- 1School of Bioengineering and Biosciences, Lovely Professional University, Phagwara, India
- 2Indian Scientific Education and Technology Foundation, Lucknow, India
- 3Department of Chemistry, Poona College, Savitribai Phule Pune University, Pune, India
- 4Department of Clinical Laboratory Sciences, The Faculty of Applied Medical Sciences, Taif University, Taif, Saudi Arabia
- 5Research Center for Health Sciences, Deanship of Graduate Studies and Scientific Research, Taif University, Taif, Saudi Arabia
- 6Faculty of Biotechnology, University of Agricultural Sciences and Veterinary Medicine, Bucharest, Romania
- 7Department of Zoology, Deen Dayal Upadhyay Gorakhpur University, Gorakhpur, India
- 8Centre of Genomics and Bioinformatics, Deen Dayal Upadhyay Gorakhpur University, Gorakhpur, India
Pesticides play a crucial role in modern agriculture, aiding in the protection of crops from pests and diseases. However, their indiscriminate use has raised concerns about their potential adverse effects on human health and the environment. Pesticide residues in food and water supplies are a serious health hazards to the general public since long-term exposure can cause cancer, endocrine disruption, and neurotoxicity, among other health problems. In response to these concerns, researchers and health professionals have been exploring alternative approaches to mitigate the toxic effects of pesticide residues. Bioactive substances called nutraceuticals that come from whole foods including fruits, vegetables, herbs, and spices have drawn interest because of their ability to mitigate the negative effects of pesticide residues. These substances, which include minerals, vitamins, antioxidants, and polyphenols, have a variety of biological actions that may assist in the body’s detoxification and healing of harm from pesticide exposure. In this context, this review aims to explore the potential of nutraceutical interventions as a promising strategy to mitigate the toxic effects of pesticide residues.
1 Introduction
Pesticides have become indispensable tools in modern agriculture, ensuring the protection of crops from pests and diseases, thus securing food production and global food security (1). Nevertheless, there are significant concerns about the unintended effects of pesticides on the environment and human health due to their widespread usage. Among these concerns, the presence of pesticide residues in food and water sources has emerged as a pressing issue, with potentially detrimental effects on both human and ecological systems (2). On the other hand, nutraceuticals have gained significant attention as functional foods that offer potential health benefits beyond basic nutrition (3). Derived from natural food sources, nutraceuticals contain biologically active compounds, such as vitamins, minerals, antioxidants, and phytochemicals, which can promote health, prevent chronic diseases, and enhance overall wellbeing. The increasing demand for nutraceuticals stems from the desire to seek alternative approaches to traditional medicine for maintaining and improving health (4). However, the use of pesticides in conventional agricultural practices poses a significant challenge to the concept of food-based nutraceuticals. Pesticides, despite their intended purpose of safeguarding crops, can leave residues on fruits, vegetables, and other agricultural products. These residues can contaminate the food supply chain, leading to potential health risks for consumers (5).
The presence of pesticide residues in food has raised concerns about their adverse effects on human health. Some pesticides have been linked to various health problems, including developmental disorders, hormonal disruption, neurotoxicity, and even certain types of cancer. Thus, the substances used to protect and enhance food production can inadvertently become a threat to human health (6). In contrast, food-based nutraceuticals offer a promising solution to counterbalance the potential harm caused by pesticide residues (7). These natural compounds found in fruits, vegetables, whole grains, and other food sources possess significant antioxidant and anti-inflammatory properties (8). They can help combat oxidative stress, neutralize harmful free radicals, support immune function, and contribute to overall health and wellness. The key challenge lies in minimizing pesticide residues in food products to ensure the safety and efficacy of nutraceuticals (9). Adopting sustainable agricultural practices, such as organic farming, integrated pest management (IPM), and precision agriculture, can reduce reliance on synthetic pesticides and promote the production of cleaner, pesticide-free crops (10). The use of pesticides in agricultural practices raises concerns about their potential impact on human health and the environment. While pesticide residues can pose risks, food-based nutraceuticals derived from natural sources offer a potential solution to counteract these risks (11). By adopting sustainable agricultural practices and ensuring strict monitoring, it is possible to reduce pesticide residues and promote the production of safe, nutrient-rich crops. Striking a balance between the benefits of pesticides in crop protection and the potential harm they may cause is crucial for ensuring a healthy and sustainable food system (12).
The global pesticide consumption in 2019 was approximately 4.19 million metric tons, where China was by far the largest pesticide-consuming country (1.76 million metric tons), followed by the United States (408 thousand tons), Brazil (377 thousand tons), and Argentina (204 thousand tons) (13). In southeast Asia, the WHO reported an annual increase in pesticide usage, with 20% of developing countries as pesticide consumers, including Cambodia, Laos, and Vietnam. India belongs to one of the major pesticide producing countries in Asia, having 90 thousand tons annual production of organochlorine pesticides including benzene hexachloride (14).
An increase in the usage of pesticides can cause endocrine and neurological disturbances, influencing development, reproduction, and the immune system (15). Pesticides may also influence fundamental regulatory and homeostatic systems, drive reactive oxygen species (ROS)-induced alteration of cellular components, and deplete antioxidant mechanisms, all of which contribute to the occurrence of oxidative stress (16). This review highlights various studies and research that have explored the presence of pesticide residues in food and their potential health implications. It delves into the adverse effects of chronic pesticide exposure, such as neurotoxicity, endocrine disruption, and carcinogenicity, among others. Additionally, it discusses the mechanisms by which pesticide residues can accumulate in the human body and the challenges associated with regulating their usage and monitoring their presence in food.
2 Classification of pesticides
Pesticides are categorized according to their toxicological attributes (17). The World Health Organization (WHO) has created a generally accepted pesticide classification system based on acute toxicity values. This method divides pesticides into four categories:
Class I: highly hazardous pesticides. They have high acute toxicity and constitute a serious risk to human health. Organophosphates and carbamates are examples (18).
Class II: moderately hazardous pesticides. They have moderate acute toxicity and constitute a substantial risk to human health. Some pyrethroids and herbicides are examples (19).
Class III: slightly hazardous pesticides. They have modest acute toxicity but can still be dangerous. Fungicides and insecticides are two examples (20).
Class IV: unlikely to present an acute hazard. Pesticides in this class have low toxicity. Some herbicides and insecticides are examples (21).
Here, we discuss that the classification of pesticides as highly hazardous is often based on their acute toxicity, which refers to the immediate detrimental effects that might emerge following short-term exposure. Pesticides designated very toxic can cause serious health problems even at modest levels of exposure (22).
2.1 Organochlorine
Organochlorine insecticides are organic compounds that are linked to five or even more chlorine atoms (also known as chlorinated hydrocarbons). They are used in agriculture and were among the first pesticides developed (23). There are two types of organochlorides: DDT-type substances and chlorinated alicyclic substances, comprising bornanes, cyclohexanes, and cyclodienes. Organochlorine is also a broad category of chlorinated hydrocarbons, which also includes chlorinated compounds of hexachlorobenzene (HCB), diphenyl ethane, cyclodiene (aldrin, endrin, and dieldrin), hexachlorocyclohexane (or lindane), nonachloride, chlordane, heptachlor, and heptachlor epoxide (24). Such chemicals have bioaccumulated in nature as a result of their extensive use, lipophilic nature, persistence, and narrow-spectrum action (25). The primary site of action for these substances or their metabolites in humans is the central nervous system, where they alter the electrophysiological characteristics and enzymatic neuronal membranes. These changes in the dynamics of Na+ and K+ flow through the cell membranes of neurons result in the propagation of multiple nerve impulses for each external stimulus, which can cause symptoms such as seizures and acute respiratory poisoning or even death from respiratory failure (26).
2.2 Organophosphates
They are phosphoric acid esters, which are classified as wide-spectrum insecticides because they contain a diverse range of compounds (27). This class of compounds is differentiated by the covalent substitution of one of the four carbon-oxygen-phosphorus links of phosphate ester with the carbon-phosphate bond (28). Organophosphorus insecticides fall under a variety of other chemical categories, such as organothiophosphate, aliphatic amide, aliphatic organothiophosphate, oxime organothiophosphate, benzotriazine organothiophosphate, heterocyclic organiorthophosphate, benzothiopyran organothiophosphate, isoindole organothiophosphate, benzotriazine organothiophosphate, isoxazole organothiophosphate, pyrazolopyrimidine organothiophosphate, pyrimidine organothiophosphate, pyridine organothiophosphate, thiadiazole organothiophosphate, quinoxaline organothiophosphate, triazole organothiophosphate, phosphonate phosphonothioate, phenyl organothiophosphate, phenyl ethylphosphonothioate, phosphoramidate, phenylphosphonothioate, phosphorodiamide, and phosphate oramidothioate (19). Organophosphorus pesticides include mipafox, diazinon, chlorpyrifos, ethion, dichlorvos, malathionparathion, and fenthion. The cholinesterase-inhibiting effects of organophosphorus pesticides on invertebrates and vertebrates result in acetylcholine neurotransmitter superimposition across synapses. Because nerve impulses are unable to cross the synapse, voluntary muscles contract quickly, resulting in paralysis and death (18).
2.3 Carbamates
Pesticides made from organic carbamic acid are known as carbamates. These compounds comprise aminocarb, carbaryl, and carbofuran (29). Similar to carbamates, organophosphate pesticides also work by interfering with nerve signal transmission and destroying pests by poisoning them (30). Aldicarb, trimethacarb, carbofuran, carbaryl, propoxur, ethinenocarb, methomyl, oxamyl, pirimicarb, and fenobucarb are common substances that cause hazardous exposure (31).
2.4 Pyrethroid
They are synthesized derivatives of natural pyrethrin. Compared to natural pyrethrins, they are more reliable and have prolonged residual effects (32). Although they are moderately poisonous to mammals, they are extremely detrimental to insects. For instance, cyclomethrin and decamethrin come under the pyrethroid category (33).
Overall, the classification of pesticides as highly hazardous serves as a crucial tool for identifying and managing the risks associated with their use. It emphasizes the significance of taking preventative precautions, following correct handling rules, and moving to safer and more sustainable pest management practices (34).
2.5 Pesticide exposure
Previous report demonstrated that pesticide exposure contributes to over 300,000 deaths worldwide annually (35). It is characterized by a state in which an individual ingests or inhales any pesticide beyond its threshold levels, which causes adverse effects. Pesticides can penetrate the organism by inhalation, contact with skin, and ingestion. Pesticide exposure can be occupational, as people who work in forestry, agriculture, the pesticide industry, and pesticide application are exposed to pesticides on the job (36). Domestic use (clothing) and dietary intake of tainted food and drink are the sources of nonoccupational exposure, which can lead to significant complications as they are absorbed by the environment and food chain. Although they can be eliminated through the skin, exhaled, and urine, pesticides are transported throughout the body by the bloodstream (37). Pesticides can penetrate the body through the mouth, skin, eye, and respiratory routes, which are the four most prevalent entry points. Pesticides are an essential component of fundamental agricultural processes all over the world, and farmer exposure to them is an inevitable occupational hazard (38). Farmers, according to various studies, are more prone to neurological, digestive, retinal, respiratory, and reproductive disorders than the general population and it is suspected to be influenced by the regular exposure to harmful chemicals but not only, as other factors related to way of life and hard work are also affecting their health (34). Pesticide exposure can cause immediate health problems such as acute poisoning, nausea, eye irritation, pain, and headaches. Diabetes, cancer, asthma, and other medical disorders might develop as a result of prolonged exposure. Pesticide exposure has a negative impact based on the quantity of pesticide used and the period of its retention, i.e., how prolonged it stays there. We are repeatedly exposed to pesticides in our regular activities, either directly or indirectly (39).
3 Mechanism of oxidative stress caused by some pesticides
Exposure to pesticides and many chemicals can lead to oxidative stress in living organisms. Oxidative stress occurs when there is an imbalance between the production of ROS and the body’s antioxidant defense mechanisms. Pesticides can induce oxidative stress through various mechanisms (35). Some pesticides (such as rotenone) can directly generate ROS, such as superoxide anion (O2⋅–), hydrogen peroxide (H2O2), and hydroxyl radicals (⋅OH). These ROS can damage cellular components, including lipids, proteins, and DNA, leading to oxidative stress. Pesticides can deplete the body’s antioxidant defenses, including enzymes such as superoxide dismutase (SOD), catalase (CAT), and glutathione peroxidase (GPx) (40). These enzymes play a crucial role in neutralizing ROS. Pesticides can inhibit or reduce the activity of these enzymes, impairing the antioxidant defense system. Some pesticide exposure can lead to lipid peroxidation; a process in which ROS attack and damage lipid molecules in cell membranes. This process generates lipid peroxides, which further exacerbate oxidative stress and cause membrane dysfunction. Some pesticides can directly interact with DNA, causing oxidative damage to DNA molecules (41). This can lead to mutations, DNA strand breaks, and impaired DNA repair mechanisms, which contribute to cellular dysfunction and oxidative stress. Some Pesticides can disrupt mitochondrial function, leading to increased ROS production. Mitochondria are a major source of ROS generation in cells. Pesticide-induced mitochondrial dysfunction can impair the electron transport chain, leading to increased electron leakage and ROS production. Pesticide exposure can trigger inflammation in tissues and organs. Inflammatory cells produce ROS as part of the immune response, contributing to oxidative stress. Pesticide-induced inflammation can further promote oxidative damage and disrupt cellular homeostasis (42).
Exposure to certain chemicals or toxins can initiate a cascade of events within cells. Some compounds have the potential to produce reactive oxygen species. Oxidative stress develops when there is an imbalance between the creation of ROS and the ability of cells to detoxify them. ROS can cause damage to biological structures such as lipids, proteins, and DNA. ROS can disrupt several signaling pathways within the cell. ROS, for example, can trigger signaling cascades involving transcription factors such as NF-B and AP-1, which influence gene expression in inflammation and cell survival (43). Apoptosis, also known as programmed cell death, is a natural mechanism that aids in the elimination of damaged or undesirable cells. Apoptosis can be triggered by excessive oxidative stress and disrupted signaling cascades. This is a defense mechanism designed to keep injured cells from causing more harm as illustrated in Figure 1 (25). Overall, the sequence of events demonstrates the complex link between chemical exposure, oxidative stress, altered signaling, and cellular responses (19). Understanding how pesticides cause oxidative stress can aid in the development of ways to minimize their adverse effects and encourage safer pesticide use (Table 1).
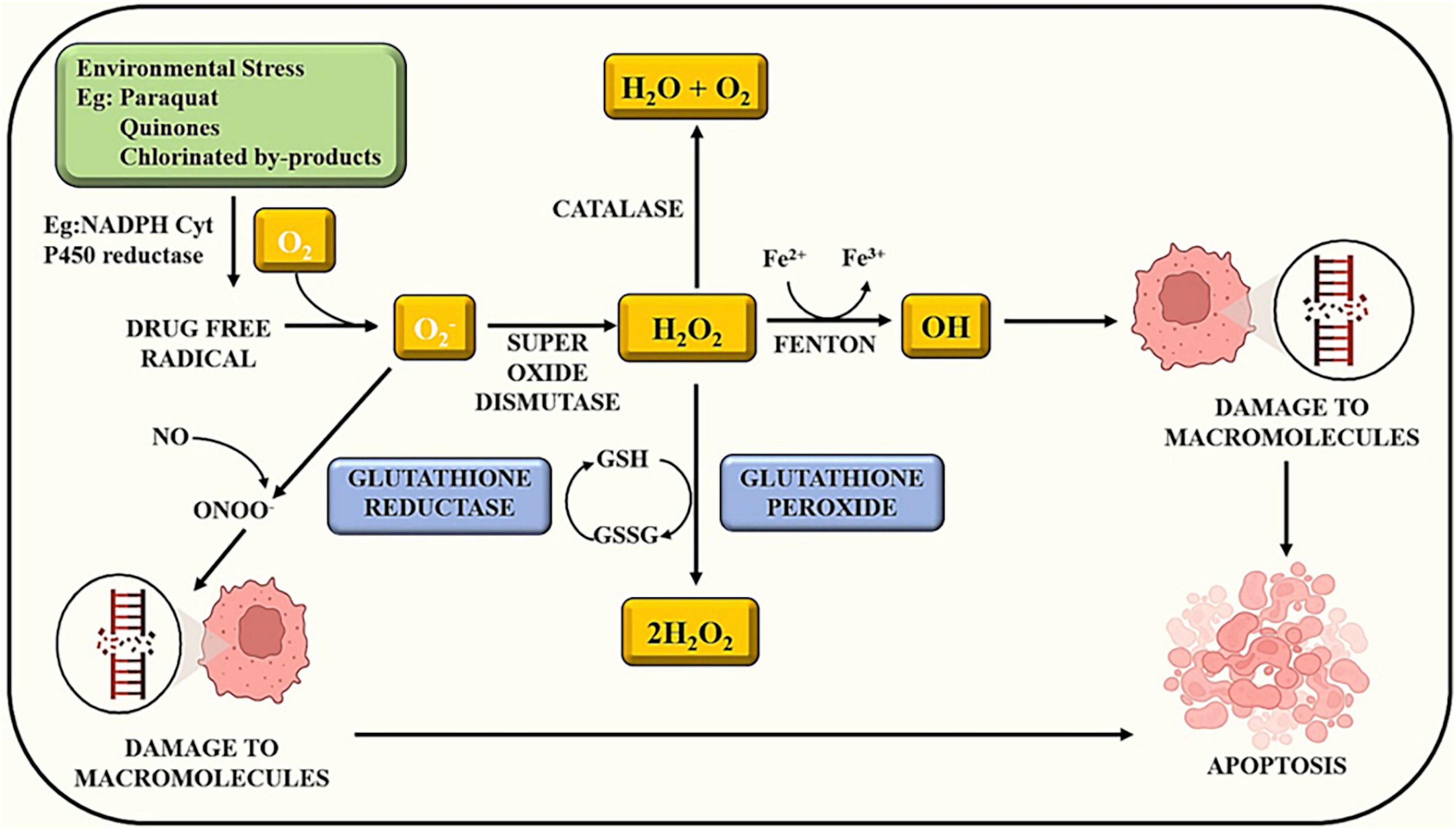
Figure 1. Chemical exposure induces reactive oxygen species and alters other signaling cascades, which results in cell apoptosis.
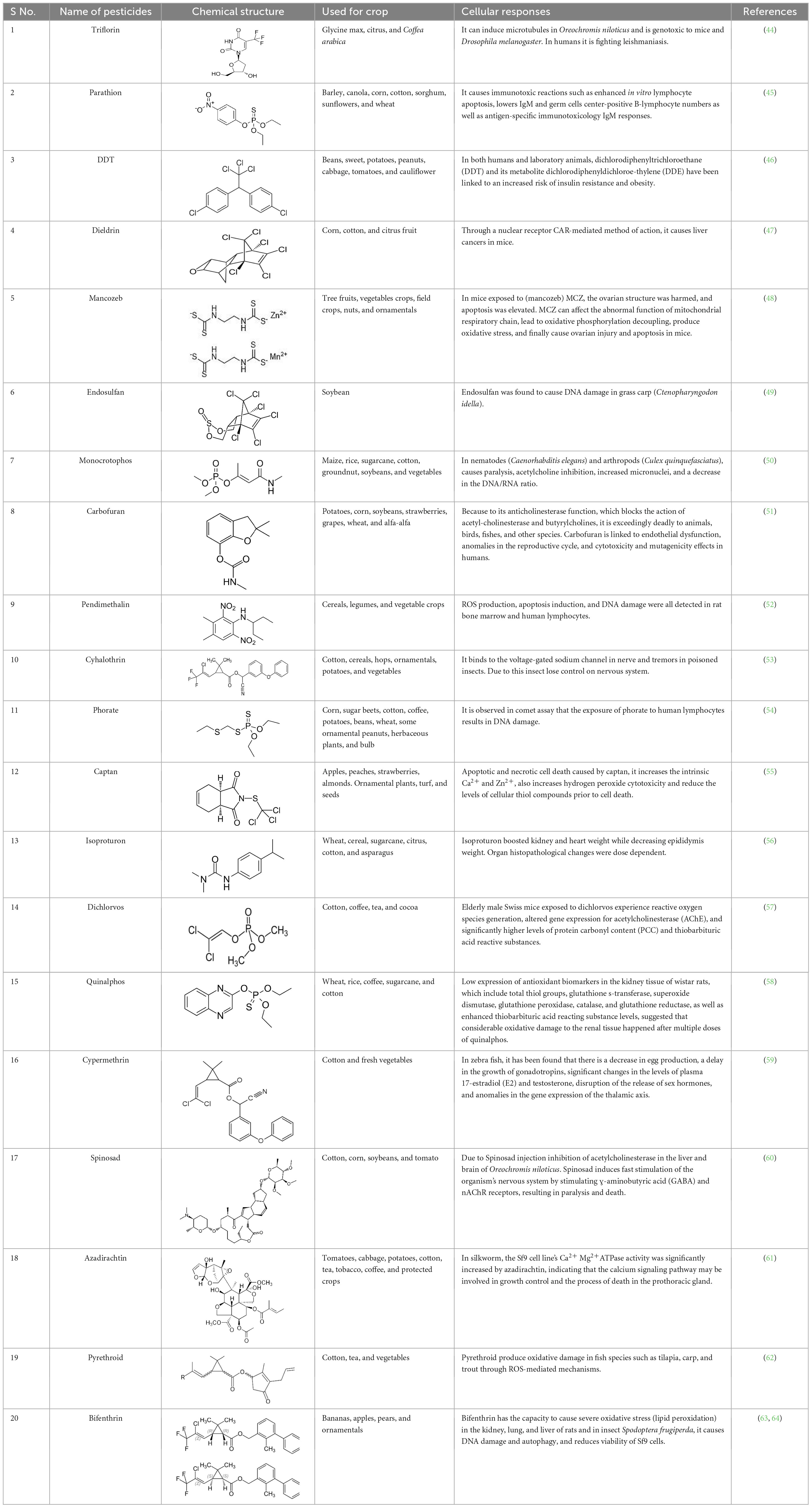
Table 1. Some examples of well-known pesticides and their oxidative stress response and related toxicity.
In contrast, high ROS concentrations cause cellular biomolecule damage, which results in the emergence of numerous illnesses (65). The human body contains a number of defense systems to mitigate the harm caused by oxidative stress. Antioxidative agents’ action is the primary mechanism (66). Since they are stable enough to eliminate free radicals by donating electrons, antioxidants are molecules that prevent or limit oxidative injury (67). Many substances have recently been discovered to have antioxidative properties, but the human body has two types of antioxidant defense systems: enzymatic and nonenzymatic. By preserving a physiologic amount of ROS, they defend both cellular structural integrity and performance from ROS harm (68). The enzymatic antioxidants include catalase (present in peroxisomes), glutathione peroxidase, which contains selenium (Se-GPX) and resides in the matrix of mitochondria and cytosol, and glutathione reductase (GSH-R), which is considered a key enzyme for the glutathione (GSH) redistribution pathway. Copper and zinc-containing SOD (Cu, Zn-SOD) is present in peroxisomes and CAT. These factors maintain the cellular homeostasis of the human body (54).
Elevated levels of oxidative stress and endocrine disruption have been linked to pesticide actions in the human body. Exposure to pesticides is a major factor in the rise in oxidative stress, which might change a person’s susceptibility to diseases. They have been discovered in the human placenta and have been linked to increased oxidative stress, intrauterine limitation, and decreased birth weight. In particular, lipid peroxidation (one of the key oxidative stress markers) and cytotoxicity have been observed in humans, primarily in pregnant women and agricultural producers (69). However, studies utilizing pesticides on mammals that directly connect the lethality of the contaminants and oxidative stress are exceedingly rare. As a result, research employing human cell lines started, and it is now being done more often. The effects of pesticides on the extent of oxidative stress in human tissues and changes in the endocrine system were demonstrated using human cell cultures. The precise action mechanisms of phenoxy analogs, triazolo pyrimidines, triazines, dinitroanilines, imidazolines, and other types of fungicides and insecticides are typically difficult to pinpoint. Particularly in cases where the effect is brought on by a dose of environmental exposure, these pathways in humans are not well understood (70).
As previously noted, in human cell lines, the oxidative stress variables under the action of pesticides were studied. It was discovered that the herbicide MCPA (4-chloro-2-methylphenoxyacetic acid) is carried across the membranes of Caco-2 cells, which is a useful study model of the human digestive tract. Caco-2 cells cultivated on semipermeable membranes were utilized to examine and characterize the mechanisms of transcellular transport of MCPA across the small intestine, where pesticides are absorbed (71). Another study looked at the impact of the sodium salts of 2,4-D-Na and 4-chloro-2-methylphenoxyacetic acid (MCPA-Na), two extensively used phenoxy herbicides, on the amount of carbonyl groups in protein substrates in erythrocytes. Because of their biological and structural simplicity, making them particularly suited for research on specific xenobiotic toxicity, human erythrocytes were chosen as such an experimental animal model (68). The results of the experiments showed that the location of the hydroxyl in the phenol ring has a significant impact on the pro-oxidative activity of phenoxy herbicides. According to previous literature, paraquat (1,1-dimethyl-4,40-bipyridinium chloride), one of the most commonly used herbicides, causes mitochondrial dysfunction in human bronchial BEAS-2B cells. Because these cells were developed from normal healthy bronchi, they are a suitable model system to investigate the pathogenic mechanisms of oxidative stress-induced respiratory ailments caused by pesticides. Furthermore, the mechanisms behind oxidative stress are frequently studied using this cell line (72).
4 Signaling mechanism related with oxidative stress in some pesticides
Although some pesticide exposure is acute or chronic, a number of events comprising various cellular pathways, including alterations in gene activation, expression, or suppression, take place (73). The exact molecular mechanism by which this happens is still unknown. Understanding the molecular and cellular level alterations is essential for determining the primary pathways associated with the induction of oxidative stress due to some pesticides and evaluating potential defensive medications or therapies (74).
4.1 TNF-α pathway
According to several investigations, one of the potential mechanisms responsible for oxidative stress is the death receptor pathway (75). The signaling of caspase-8, which catalyzes the breakdown of the caspase-3 effector either indirectly or directly through the mitochondrial pathway, is brought on by the conjugation of cell surface death receptors, including the tumor necrosis factor receptor (TNFR), which facilitates the transmitted signal of tumor necrosis factor-alpha (TNF-α). TNF- is a potent pro-inflammatory cytokine that is produced during inflammation by macrophages and monocytes and is in control of many cell signaling pathways that trigger necrosis or apoptosis (76). The association between TNF-α and the two cell surface receptors TNF-R1 and TNF-R is what causes the inflammatory responses that it triggers. TNF-α is also responsible for the activation and transcription of adhesion molecules, the stimulation of inflammatory cytokines, and the promotion of growth. The mouse fibrosarcoma cell line (L929) could experience mitochondrial dysfunction and ROS production as a result of increased TNF-α levels alone (77). Based on an experiment on mice exposed to permethrin, a rise in TNF- levels induces ROS generation and reduces the redox equilibrium, which causes oxidative stress (78). Permethrin also enhanced TNF-mRNA expression in zebrafish exposed for a period of 72 h after fertilization in a concentration-dependent manner (65).
4.2 NF-κB and Nurr1 pathway
The transcription factor orphan nuclear receptor-related 1 (Nurr1), a constituent of the nuclear receptor subclass 4 group A member 2 (NR4A2) family of proteins, is crucial for dopaminergic neuron metabolism. New research suggests that decreased Nurr1 activity may play a role in Parkinson’s disease etiology. In brain cells, Nurr1 prevents NF-B (transcription factor NF-B), which has anti-inflammatory properties (79). The pro-inflammatory nuclear factor-B, i.e., transcription factor, is expressed more, while the Nurr1 gene expression is lowered by permethrin (80). Permethrin (insecticide) enhanced the production of the pro-inflammatory cytokine TNF- and inhibited the expression of IL-13, IL-2, and IL-1 in an additional investigation in older treated rats. These findings suggest that the Nurr1, NF-κB, and TNF-α pathways may contribute to understanding some pathways associated with oxidative stress caused by pesticides (81).
4.3 STAT
According to a plethora of studies, chlorpyrifos (CPF) weakens antioxidant defenses by interfering with the electron transport chain in mitochondria (ETC) complex 1 activity, which increases the formation of free radicals and superoxide. According to an earlier investigation, CPF increased cell mortality in a dose-dependent manner and caused oxidative stress by increasing ROS production and lowering GSH levels in dopaminergic neurons and human mesencephalic cells (82). The team hypothesized that signaling transducers and activators of transcription 1 (STAT-1) regulate CPF-induced ROS production and that higher ROS ultimately result in cell apoptosis based on the findings of CPF-induced dopaminergic cell damage (Figure 2). Apoptotic cell mortality subsequently results from increased reactive oxygen species. STAT proteins are members of a group of latent cytoplasmic transcriptional regulators that are crucial for cell type-specific differentiation, growth, apoptosis, and multiplication. Both neuronal viability and cell death depend on Janus kinase (JAK)-STAT signaling (83). The activation of JAK that results from the binding of mediators to their receptors phosphorylates STAT-1 on serine 727 and tyrosine 701 residues. Phospho STAT-1 dimerizes and moves into the nucleus, where it attaches to the GAS/ISRE found on the coding region of particular target genes that control NADPH oxidase (NOX), pro-inflammatory cytokines, apoptosis, and regulators of cell cycle arrest such fas, caspases, and bax. Proapoptotic gene transcription-dependent expression as well as non-transcriptional signal transduction pathways are regulated by STAT-1 to control apoptosis. In yet another study, when organophosphate pesticides (OP) pesticides were used on STAT-1 suppression (KD) dopaminergic cells, they caused a 66% reduction in mitochondrial ROS levels compared to scrambled tiny interfering RNA (siRNA)-transfected cells subjected to identical pesticides (84). NOX-1, an NADPH oxidase isoform that produces superoxide, controls the production of ROS in a variety of cell types, including but not limited to monocytes, macrophages, vascular endothelial cells, and smooth muscle cells. The primary ROS-producing enzyme throughout inflammation is NOX1. The fact that OP pesticides boosted the recruitment of STAT-1 to the constitutive NOX1 promoter suggests that STAT-1 is responsible for controlling the transcription of NOX1. In neural cells exposed to the OP pesticide CPF, STAT-1 is critical in controlling ROS production as well as GSH levels in a NOX1-dependent manner. In human monocytes, organochlorine pesticides increased NOX-dependent ROS production. These findings collectively imply that NOX-STAT-1 activation is critical for the production of ROS during OP pesticide-induced oxidative damage (85).
4.4 Endoplasmic reticulum stress
Assembly, folding, posttranslational alteration, and protein transport are only a few of the many tasks performed by the endoplasmic reticulum (ER). Additionally, calcium, which is necessary for muscular contractions, is stored in the ER. When the ER’s (86) aptitude for folding peptides is exceeded, ER stress results, and cells with ER stress are characterized by a build-up of abnormal proteins within the ER lumen. Numerous factors, such as hypoxia, nutritional scarcity, and pesticides, can cause ER stress. Cellular senescence might be produced in the event that ER stress is substantial or prolonged. It has been demonstrated that a number of pesticides, including 2,4-dichlorophenol, paraquat, chlorpyrifos, and deltamethrin, cause ER stress. Numerous of these pesticides also cause apoptosis, although research indicates that ER stress and apoptotic cell death are caused by separate chemical mechanisms (78).
4.5 Mitochondrial dysfunction pathway (mitochondrial apoptosis)
Mitochondrial dysfunction is a typical oxidative stress-related malfunction. ROS can induce mitochondrial damage in some circumstances, but they can also cause mitochondrial damage in other circumstances. Numerous pesticides have been found to block mitochondrial ensembles, which are the primary site for ROS generation (87). As a result, it is believed that the ROS generated by damaged mitochondria play a significant role in oxidative stress. In both mammals and fish, ER stress and apoptosis are frequently linked to mitochondrial malfunction (65). Pentachlorophenol (PCP) and its metabolite tetrachlorohydroquinone (TCHQ) massively increased lipid oxidation in the rat liver and lowered the antioxidant GSH level by producing large amounts of urine 8-iso-prostaglandin-F2 (8-iso-PG-F2). The mitochondrial apoptotic pathway is another possible mechanism that may be implicated in pesticide such as atrazine (ATR), organophosphorus (OP) compounds, carbamates, and pyrethroids induced mitochondrial dysfunction, according to the available and developing evidence (88). The primary regulators of mitochondrial coherence in this system are B-cell lymphoma 2 (BCL2) and BCL2-associated X (BAX). They also affect the release of cytochrome c and the activation of caspase. Several well-known proteins linked to cell damage, BAX and BCL2, have opposing roles. In contrast to the BAX peptide, which acts as an apoptosis activator, the BCL2 protein suppresses apoptosis by its own antioxidative action (89). Following mitochondrial injury, Bax is translocated from the cytoplasm to the mitochondria, and Bcl-2 expression is significantly reduced. Mitochondrial cytochrome c is released into the cytoplasm, which is a crucial terminal event, as a result of high amounts of ROS from exposure to pesticides. TCHQ increased the transcription of Hsp70 in hepatocytes while decreasing the expression of the BCL2/BAX ratio and cellular apoptotic sensitivity (CAS), two genes involved in apoptotic and necrotic processes (90).
5 Relationship of the toxicity of some pesticides with alteration of oxidative balance
5.1 Neurotoxicity
Neurotoxins are any compounds that can damage the central nervous system, along with the brain (80). The brain is particularly prone to OP toxicity, which disrupts the balance of oxidant and antioxidant components in neuronal cells (91). OPs have a strong pro-oxidant action that disrupts neuronal mitochondrial function, resulting in a variety of neurological disorders. Higher level exposures from occupational exposures and closeness to agricultural spraying are linked to a variety of neurotoxicity in people (92).
Human neurodegenerative disorders and neurotoxicity have been associated with pyrethroid pesticides, organochlorine insecticides, and organophosphate insecticides. DDT has low toxicity (rat oral LD50 = 113 mg/kg) (93). Furthermore, oral administration of 3.5 or 35 mg DDT/day to people for up to 18 months did not result in observable toxicity or neurotoxicity. Only a few human studies have examined DDT’s possible neurotoxicity. DDT was identified more frequently in the brains of Alzheimer’s sufferers. Nonoccupational DDT exposure can potentially result in cognitive impairments (94). Dieldrin caused substantial oxidative stress, mostly owing to mitochondrial malfunction, and was associated with considerable caspase overexpression and activation, resulting in apoptosis (95). Dieldrin-induced dopaminergic neurotoxicity is caused by caspase-3-dependent proteolytic cleavage of protein kinase C (PKCδ) (96). Dieldrin has also been shown to cause epigenetic dysregulation in cell and animal models of dieldrin neurotoxicity by hyperacetylation of core histones. Endosulfan is very neurotoxic in rats, inducing both chronic neurodegeneration and developmental neurotoxicity.
Aside from inducing apoptotic cell death through mitochondrial dysfunction. According to research (97), rats gavaged with 2 mg/kg endosulfan for 6 days had damaged brain mitochondria, as evidenced by significantly lower levels of SOD, GSH, and CAT, as well as enhanced lipid peroxidation.
5.2 Disruption of the endocrine system
Every hormone has a different molecular structure and a unique production process with numerous phases. The production of the hormone may be impaired or the characteristics of the hormones may change if one component or link in the chain of hormone synthesis is disrupted. Some pesticides, including prochloraz, fenarimol, and other fungicides (imidazole), have the capacity to block testosterone to oestrogen conversion by inhibiting cytochrome 19 aromatase in vitro (98). It was predicted that substances that may decrease the activity of aromatase in vitro might also be able to alter local oestrogen and androgen levels in vivo. Aromatase induction is a biological method of xenobiotic deactivation that does not typically result in toxicity. In vitro aromatase function is induced by the pesticides simazine, atrazine, and propazine. It has been proven that p,p-DDE induces aromatase both in vivo and in vitro. Additionally, the pesticide heptachlor may operate as an inducer of testosterone 16-alpha and 16-beta hydroxylases, although pirimicarb, methomyl, iprodione, and propamocarb can only slightly stimulate aromatase activity (99). Dopamine-beta-hydroxylase activity has been demonstrated to be suppressed by thiram, sodium N-methyl-di-thio-carbamate (SMD), and other di-thio-carbamates, which results in a reduced conversion of dopamine to norepinephrine. This might affect the hypothalamic catecholamines that produce the proestrus surge in luteinizing hormone, which triggers the last stages of ovulation. It was determined that SMD has the ability to prevent rat ovulation and the LH surge. Ketoconazole blocks the synthesis of progesterone as well as a number of cytochrome 450-dependent monooxygenase enzymes (100).
5.3 Carcinogenicity
The large percentage of pesticides poses a risk to nontarget organisms, including humans. In laboratory animals, 56 pesticides have been identified as cancer-causing. In clinical studies, chemicals such as lindane, phenoxy acid herbicides, toxaphene, and several organophosphates have been linked to cancer (101). Due to the destructive effects of genotoxic substances, cellular genetic components (DNA and RNA) lose their genotoxic characteristics.
It has been studied in vivo, in vitro, and epidemiologically that certain pesticides can cause genomic toxicity (102). This genotoxicity is viewed as a fundamental health concern that, with repeated exposures, will have long-term consequences on reproductive, neurological, and cancerous systems. Pesticide use might cause mutagenic and non-mutagenic processes that cause genetic changes. Several studies have revealed a strong link between chronic pesticide exposure and a few proto-oncogenes in populations exposed owing to pesticide cytogenetic effects (103). It is linked to an increased risk of leukemia and non-lymphoma, Hodgkin’s as well as cancers of the brain, skin, thyroid, esophagus, kidney, lung, prostate, testicles, cervix, bladder, and rectum (104). These may cause oxidative stress, which results in the production of free radicals and changes in enzymatic antioxidants such as catalase, glutathione transferase and SOD (105).
6 Nutraceuticals and bioactive compounds from medicinal plants
Medicinal plants have traditionally been utilized by humans as a traditional way of treating a variety of diseases. Despite significant improvements in therapeutic development, there is still a need for effective and potent analgesic drugs (106). In this context, it has been widely described that numerous plant-derived compounds serve an essential part in the process of developing novel techniques to treat various diseases. Recent research on herbal plants or medicine has resulted in significant advances in the pharmacological assessment of diverse plants utilized in traditional medical systems (107). Secondary metabolites or substances found in medicinal plants include tannins, terpenoids, alkaloids, and flavonoids, which determine the therapeutic effectiveness of the plant, particularly its antioxidant activity. Secondary metabolites are very diverse low molecular weight substances with a wide range of biological characteristics that interact with proteins, nucleic acids, and other bio membranes and are active and volatile targets of cells (108).
Herbal remedies play a crucial part in healthcare and have various therapeutic applications. Here are some key reasons highlighting the importance of medicinal plants and their therapeutic applications:
6.1 Traditional medicine
For ages, medicinal plants have been employed in traditional medical systems including Ayurveda, Traditional Chinese Medicine, and Indigenous healing practices. They form the foundation of these systems and have been trusted for their healing properties by communities worldwide (109).
6.2 Rich source of bioactive compounds
Medicinal plants contain numerous bioactive compounds including flavonoids, alkaloids, terpenoids, and tannins, which possess medicinal properties. These compounds can have diverse impacts on the human health, including analgesic, anti-inflammatory, antimicrobial, antioxidant, anticancer, and antidiabetic activities (110).
6.3 Drug discovery and development
Many modern pharmaceutical drugs are derived from or inspired by medicinal plants. Natural plant substances serve as the foundation for the creation of novel drugs. Examples include the use of the plant-derived compound paclitaxel for cancer treatment and the development of aspirin from willow bark (111). Medicinal herbs are often the primary source of healthcare in many developing countries, where access to conventional medicine may be limited. Local communities rely on traditional herbal remedies derived from medicinal plants for treating common ailments and maintaining their wellbeing (112). Medicinal plants are an integral part of complementary and alternative medicine practices. Many people seek natural and plant-based treatments as alternatives or supplements to conventional medicine. Herbal remedies and supplements made from medicinal plants are used for various purposes, including immune support, stress reduction, and overall wellness (113, 114).
6.4 Nutraceuticals and functional foods
Medicinal herbs are also used to create functional meals and nutraceuticals. These products go beyond basic nourishment to give additional health advantages. They are created with specialized plant extracts or bioactive chemicals to promote various areas of health, such as cardiovascular health, cognitive function, and digestive wellness (115, 116).
7 Protective effects of various nutraceuticals against some pesticide-induced injuries
Various nutraceuticals have been studied for their potential protective effects against pesticide-induced toxicities. These natural compounds derived from food sources can possess antioxidant, anti-inflammatory, and detoxifying properties, which may help mitigate the harmful effects of some pesticides (Figure 3) (117).
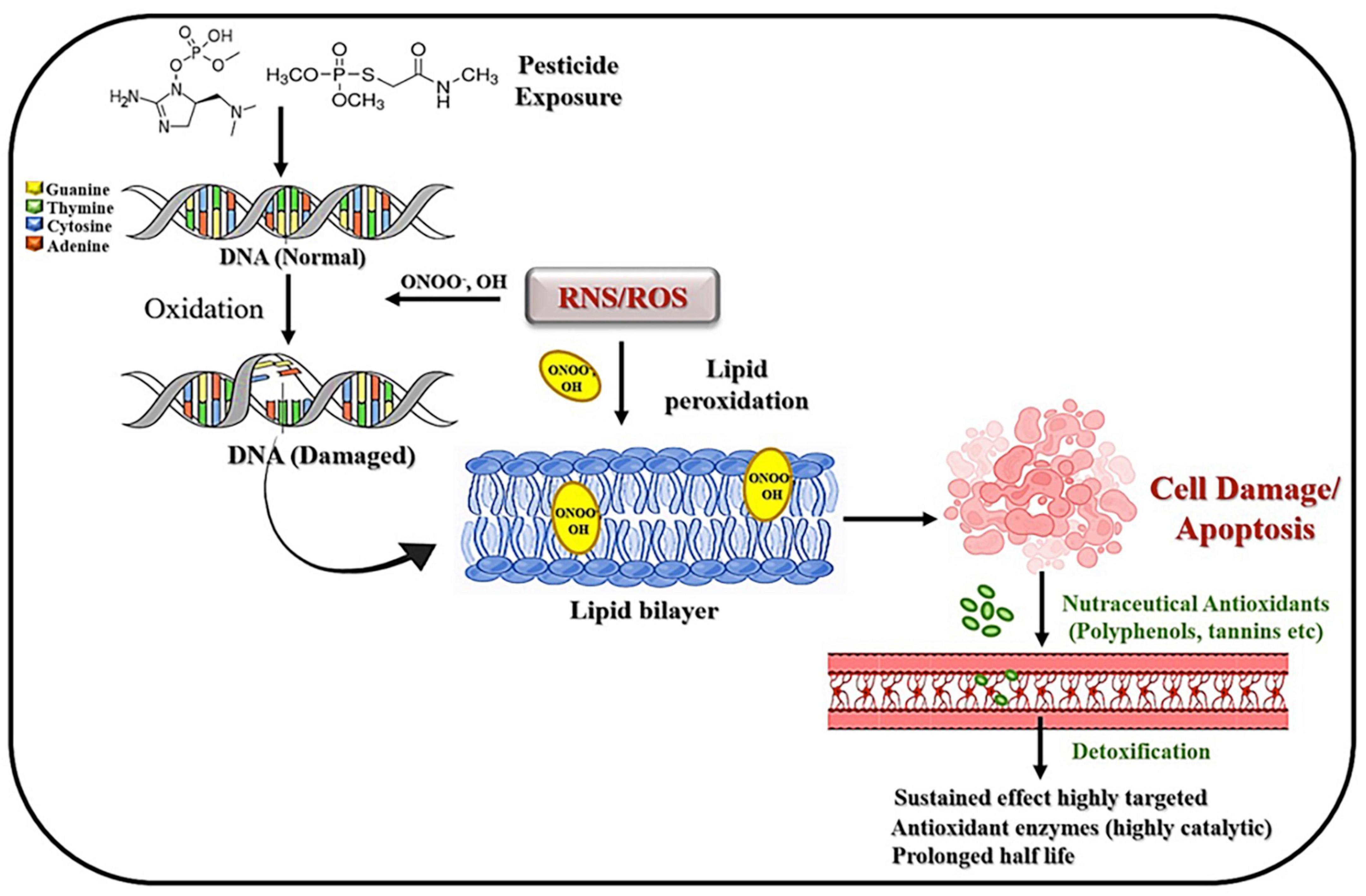
Figure 3. Schematic representation of the interplay between pesticide exposure and cellular responses, including DNA damage, lipid peroxidation, and cell apoptosis, alongside the protective effects of nutraceutical antioxidants through detoxification pathways.
Nutraceuticals rich in antioxidants, such as vitamins C and E, beta-carotene, and flavonoids, can counteract oxidative stress induced by pesticides. Examples include berries (blueberries and strawberries), citrus fruits, leafy greens (spinach and kale), and cruciferous vegetables (broccoli and cauliflower).
7.1 Curcumin
Curcumin, a bioactive component found in turmeric, has been examined for its possible protective mechanisms against pesticide-induced damage (118). While the particular methods may differ based on the pesticide and the target organ or system. Curcumin is a powerful antioxidant that can neutralize the damaging free radicals produced by pesticides (119). This helps to protect cells and tissues from oxidative damage and decreases inflammation (120). Curcumin has the ability to suppress a variety of inflammatory processes in the body. Pesticides can cause inflammation, and curcumin’s anti-inflammatory qualities may assist to mitigate this impact. It may increase the activity of detoxification enzymes in the liver, such as glutathione S-transferases (GSTs), which are important in metabolizing and removing pesticides. Pesticide poisoning frequently targets the liver. Curcumin may help protect the liver from pesticide-induced damage by lowering oxidative stress and inflammation. Curcumin’s anti-apoptotic characteristics may aid in the prevention or reduction of cell death caused by pesticide exposure (121). Some pesticides have been related to an increased cancer risk. By suppressing tumor development and metastasis, curcumin’s anticancer effects may help lessen the carcinogenic risk of some pesticides. Curcumin may boost the generation and activity of natural antioxidant enzymes including SOD and catalase, boosting the body’s ability to counteract pesticide-induced oxidative stress. Curcumin can improve gut health by encouraging the development of good gut bacteria and decreasing inflammation in the gastrointestinal system. This can help reduce some of the negative effects of pesticides on the digestive system (122). A recent study demonstrated that rats treated with mancozeb alone exhibited significant liver damage, characterized by elevated levels of liver enzymes [aspartate transaminase (AST) and alanine transaminase (ALT)] and histopathological alterations indicative of hepatotoxicity. In contrast, rats co-administered with curcumin and mancozeb showed a significant reduction in liver enzyme levels and less severe histopathological changes, indicating the protective effects of curcumin against pesticide-induced hepatotoxicity (88).
7.2 Resveratrol
Resveratrol is a polyphenolic chemical found naturally in foods such as red grapes, red wine, and some berries. Its possible preventive mechanisms against pesticide-induced damage have been investigated. Resveratrol is a powerful antioxidant that may neutralize pesticide-generated free radicals. This helps to minimize oxidative stress and tissue damage. Resveratrol has the ability to suppress a variety of inflammatory pathways in the body (123). Pesticides can cause inflammation, which resveratrol’s anti-inflammatory qualities may help to mitigate. Resveratrol may increase the activity of liver detoxification enzymes such as GSTs. These enzymes are essential for pesticide metabolization and elimination. Pesticide poisoning frequently affects the liver. Some pesticides have the ability to cause cell death in diverse tissues. The anti-apoptotic characteristics of resveratrol may help prevent or minimize cell death in response to pesticide exposure (124). Resveratrol has been explored for its neuroprotective properties. It may help protect nerve cells from pesticide damage, potentially lowering the likelihood of neurodegenerative consequences. Some insecticides may be harmful to the cardiovascular system. The cardiovascular advantages of resveratrol, such as its capacity to enhance blood vessel function and lower inflammation, may protect against these consequences. Resveratrol has been linked to anticancer activity. By suppressing tumor development and metastasis, it may help lessen the carcinogenic risk of some pesticides. Resveratrol may promote metabolic health by regulating blood sugar levels and improving lipid profiles. These advantages may assist in mitigating some pesticide-induced metabolic abnormalities. Some pesticides have the potential to damage the endocrine system. The ability of resveratrol to promote hormonal homeostasis may assist to mitigate these abnormalities (94). Kumar et al. (119) provides evidence supporting the potential neuroprotective effects of resveratrol against pesticide-induced neurotoxicity in rats. The findings suggest that resveratrol may offer therapeutic benefits in mitigating the adverse neurological effects associated with pesticide exposure (125).
7.3 Flavonoids and flavone glycosides
Flavonoids and flavone glycosides constitute a diverse class of phytochemicals known for their potent antioxidant and anti-inflammatory properties. These compounds have demonstrated significant potential in preventing oxidative damage induced by pesticides. Quercetin is a flavonoid, which is a polyphenolic component present in a variety of fruits, vegetables, and cereals (126, 127). Quercetin is a powerful antioxidant that may neutralize pesticide-generated free radicals. This helps to minimize oxidative stress and tissue damage (128). Histopathological analysis of brain tissues revealed that rats treated with chlorpyrifos alone displayed neuronal damage and gliosis, whereas rats co-administered with quercetin and chlorpyrifos showed preservation of neuronal morphology and reduced gliosis, supporting the neuroprotective effects of quercetin. Rutin is well-known for its antioxidant properties. Pesticides can cause free radicals and oxidative stress in the body, which can lead to cell damage and inflammation (129, 130). Organophosphate insecticides, for example, cause toxicity by blocking cholinesterase enzymes. The ability of rutin to inhibit these enzymes may give protection against pesticide-induced toxicity (131). A recent study measured levels of pro-inflammatory cytokines (such as TNF-α and IL-6) and apoptotic markers (such as caspase-3 activity) in liver and kidney tissues. Rats treated with deltamethrin alone showed increased levels of pro-inflammatory cytokines and caspase-3 activity, indicative of inflammation and apoptosis. However, rats co-administered with rutin and deltamethrin exhibited reduced levels of pro-inflammatory cytokines and caspase-3 activity, suggesting the anti-inflammatory and anti-apoptotic effects of rutin (132). Flavonols like kaempferol may be found in a variety of fruits and vegetables. It is useful in preventing oxidative stress brought on by pesticides because of its potent anti-inflammatory and antioxidant qualities. A prevalent flavone in celery, parsley, and chamomile tea is apigenin. By scavenging free radicals and adjusting the activity of antioxidant enzymes, it has been demonstrated to mitigate oxidative damage caused by pesticides (133).
7.4 N-acetylcysteine
N-acetylcysteine (NAC) is an amino acid supplement and pharmaceutical renowned for its mucolytic and antioxidant characteristics. NAC is a precursor of glutathione, one of the most essential antioxidants in the body. NAC can help neutralize damaging free radicals produced by pesticides by raising glutathione levels, lowering oxidative stress and cellular damage (134). NAC helps the body’s detoxification functions, notably in the liver. It can aid in increasing the liver’s ability to metabolize and remove pesticides and other pollutants. NAC is widely used to treat acetaminophen (paracetamol) overdoses. While not specifically related to pesticide toxicity, it demonstrates NAC’s potential to combat toxic chemicals by increasing detoxification mechanisms in the liver. In situations of pesticide inhalation, NAC’s mucolytic characteristics can assist lower the thickness of mucus in the respiratory system, making hazardous chemicals easier to remove from the lungs (135). NAC may have anti-inflammatory characteristics that can aid to reduce the inflammatory response caused by some pesticides. NAC may affect the immune system, perhaps improving the body’s immunological response against pesticide-induced illnesses or inflammatory processes. NAC may protect against the negative effects of pesticides on several organs, including the liver, kidneys, and nervous system, by lowering oxidative stress and oxidative damage in cells and tissues. NAC has been examined for its possible neuroprotective benefits, and it may help protect nerve cells against pesticide damage, lowering the likelihood of neurodegeneration (136). A report assessed biochemical markers of oxidative stress and neuroinflammation in the brain tissues of rats. Rats treated with chlorpyrifos alone showed increased levels of oxidative stress markers (such as malondialdehyde) and pro-inflammatory cytokines (such as TNF-α and IL-1β) compared to control rats. In contrast, rats co-administered with NAC and chlorpyrifos exhibited reduced levels of oxidative stress markers and pro-inflammatory cytokines, indicating the antioxidant and anti-inflammatory properties of NAC (137).
7.5 Omega-3 fatty acids
Omega-3 fatty acids, polyunsaturated fats present in foods such as fatty fish, flaxseeds, and walnuts, have been investigated for their possible protective mechanisms against pesticide-induced toxicity. Anti-inflammatory properties of omega-3 fatty acids are well established. Pesticides, notably organophosphates and pyrethroids, have the potential to cause inflammation in the body (138). Omega-3 fatty acids can help decrease inflammation, potentially reducing pesticide harm. Omega-3 fatty acids have antioxidant qualities, which means they can help neutralize the damaging free radicals produced by pesticide exposure. Some research suggests that omega-3 fatty acids can boost the activity of detoxification enzymes in the liver, such as cytochrome P450 enzymes. These enzymes are in charge of breaking down and removing numerous poisons, including pesticides, from the body. Omega-3 fatty acids may alter the immune system’s response to pesticide exposure. They may help the body deal with the harmful effects of pesticides by altering immunological function. Pesticides, particularly some organophosphates, can be neurotoxic (139). Docosahexaenoic acid (DHA), in particular, is critical for brain health and development. They may provide protection against pesticide-induced nervous system harm. Cell membranes rely heavily on omega-3 fatty acids. They can assist preserve the fluidity and integrity of cell membranes, which may protect cells against pesticide-induced disruption. Pro-inflammatory cytokines, which are chemicals involved in the inflammatory response, have been demonstrated to be reduced by omega-3s. This decrease in cytokine production may aid in reducing the inflammatory response caused by certain pesticides (140). The study evaluated the neurobehavioral effects of organophosphate pesticide exposure and the potential protective effects of omega-3 fatty acids using various behavioral tests, including the Y-maze test and the elevated plus maze test. Rats treated with organophosphate pesticide exhibited impaired spatial memory and increased anxiety-like behavior compared to control rats. However, rats co-administered with omega-3 fatty acids and pesticide showed significant improvements in spatial memory and reduced anxiety-like behavior, suggesting the neuroprotective effects of omega-3 fatty acids against pesticide-induced toxicity (141).
7.6 Vitamins
Vitamins are essential micronutrients known for their diverse physiological functions, including antioxidant defense and immune regulation. Vitamin C is a potent antioxidant that scavenges free radicals and regenerates other antioxidants, such as vitamin E. It plays a crucial role in protecting cells from oxidative damage induced by pesticides. Elzoghby et al. (139) evaluated markers of liver function, such as serum levels of ALT and AST, as well as histopathological changes in liver tissues. Rats treated with malathion alone exhibited elevated levels of ALT and AST, indicative of liver damage. However, rats co-administered with vitamin showed reduced levels of ALT and AST, suggesting the protective effects of vitamin C against pesticide-induced hepatotoxicity. Histopathological examination also revealed less severe liver damage (142). Vitamin E comprises a group of fat-soluble antioxidants, including alpha-tocopherol and gamma-tocotrienol. It acts as a lipid-soluble antioxidant, protecting cell membranes from oxidative stress caused by pesticides (143). The experimental study provides evidence supporting the protective effects of vitamin E against organophosphate pesticide-induced oxidative stress in rats. The findings suggest that supplementation with vitamin E enhances the activity of key antioxidant enzymes, such as SOD, CAT, and GPx, thereby mitigating the adverse effects associated with pesticide exposure (144).
Vitamin A and its precursors, such as beta-carotene, possess antioxidant properties that contribute to cellular defense against pesticide-induced oxidative damage (145). Rats treated with atrazine alone showed decreased activities of antioxidant enzymes, such as SOD and catalase, and increased levels of lipid peroxidation, indicative of oxidative stress. In contrast, rats co-administered with vitamin E and atrazine exhibited restored antioxidant enzyme activities and reduced lipid peroxidation levels, suggesting the antioxidant properties of vitamin E (146). Additionally, vitamin A is involved in maintaining epithelial integrity and immune function. Vitamin D regulates calcium and phosphorus metabolism, thereby promoting bone health. Moreover, emerging research suggests its potential role in modulating immune responses and reducing inflammation, which may aid in mitigating pesticide-induced toxicity (147).
7.7 Coenzyme Q10
Coenzyme Q10 (CoQ10), also known as ubiquinone, is a naturally occurring molecule present in the body that plays an important role in the creation of cellular energy. CoQ10 is a powerful antioxidant that aids in the neutralization of damaging free radicals and the reduction of oxidative stress. Pesticides can cause free radicals to form in the body, causing cellular damage and inflammation (147). The antioxidant capabilities of CoQ10 may assist to offset these effects and reduce pesticide-induced oxidative stress. CoQ10 is essential in mitochondria, the energy-producing organelles found within cells. Pesticides can interfere with cellular energy generation, causing weariness and tissue damage. CoQ10 supplementation may maintain mitochondrial activity, guaranteeing an appropriate energy supply to cells and maybe assisting cells in coping with pesticide-induced stress. Some insecticides, especially those with neurotoxic characteristics, can cause mitochondrial damage. Because CoQ10 is an essential component of the mitochondrial electron transport chain, it may help shield mitochondria from such damage, sustaining cellular function (148). CoQ10 has been linked to anti-inflammatory properties. Pesticides can cause inflammation in the body, which can lead to tissue damage. CoQ10 has been shown to improve the body’s natural defense systems against pollutants such as pesticides. It may boost the activity of detoxification enzymes in the liver and aid in the elimination of pesticides from the body. CoQ10 has being investigated for its neuroprotective effects. Some pesticides can injure the neurological system, and CoQ10 may provide protection. Some insecticides have been linked to cardiovascular damage. CoQ10 is proven to benefit heart health and may help guard against pesticide-related cardiovascular problems (129). A report provides evidence supporting the potential cardioprotective, antioxidant, and anti-inflammatory effects of coenzyme Q10 against pesticide-induced cardiac toxicity in rats. The findings suggest that coenzyme Q10 supplementation may offer therapeutic benefits in mitigating the adverse effects of pesticide exposure on cardiac health (149).
A report by Mohamed et al. (148) measured SOD activity in rat tissues as an indicator of antioxidant defense. Rats treated with acetamiprid alone showed decreased SOD activity compared to control rats, indicative of reduced antioxidant capacity. However, rats co-administered with curcumin and pesticide exhibited restored SOD activity, suggesting the ability of these phyto-protective nutraceuticals to enhance antioxidant defense against pesticide-induced oxidative stress (150). As an additional indicator of antioxidant defense, CAT activity in rat tissues was evaluated in an earlier report. When compared to control rats, animals treated with deltamethrin alone had lower CAT activity, a sign of compromised antioxidant defense systems. On the other hand, rats who received both the pesticide and rutin concurrently showed increased CAT activity, indicating that these phyto-protective nutraceuticals can improve CAT-mediated antioxidant defense against oxidative stress caused by pesticides (151).
As another indicator of antioxidant defense, GPx activity was also evaluated in rat tissues in previous report. When compared to control rats, animals treated with metribuzin alone had lower GPx activity, a sign of impaired antioxidant defense systems. Rats co-administered with quercetin plus metribuzin, however, showed restored GPx activity, indicating that these phyto-protective nutraceuticals can improve GPx-mediated antioxidant protection against oxidative stress caused by pesticides (152). The findings suggest that supplementation with these phyto-protective nutraceuticals can enhance the activity of key antioxidant enzymes, such as SOD, CAT, and GPx, thereby mitigating the adverse effects associated with pesticide exposure.
The substances curcumin, resveratrol, flavonoids, n-acetylcysteine, omega-3 fatty acids, vitamins, and coenzyme Q10 are highlighted as representative examples due to their relevance in providing protective effects against certain injuries induced by pesticide exposure. These substances have been studied for their potential to counteract the harmful effects of pesticides on the body, such as oxidative stress, inflammation, and cellular damage. By presenting these examples, it is suggested that they may offer promising avenues for mitigating the negative impacts of pesticide exposure on human health (153).
These compounds also possess anti-inflammatory properties, further protecting against pesticide-induced injuries (154). Furthermore, certain nutraceuticals have been found to enhance detoxification mechanisms in the body. Similarly, certain herbs and spices, such as ginger and turmeric, have been found to alleviate pesticide-induced liver and kidney injuries through their antioxidant and anti-inflammatory properties (Table 2).
8 Conclusion
Specific pesticide-mediated toxicities pose significant risks to human health and the environment. Pesticides are widely used in agricultural practices to control pests and increase crop yield, but they can have detrimental effects on nontarget organisms, including humans. However, there is growing evidence that certain food-based nutraceuticals can help mitigate the toxic effects of pesticides. Natural compounds found in various foods, such as antioxidants, vitamins, minerals, and phytochemicals, possess protective properties against pesticide toxicity. These nutraceuticals can act as scavengers of free radicals, reduce oxidative stress, enhance detoxification processes, and boost the immune system. Additionally, they may have the ability to repair damaged DNA and protect vital organs such as the liver and kidneys. Furthermore, incorporating a balanced diet rich in fruits, vegetables, whole grains, and legumes can provide essential nutrients that support overall health and resilience against pesticide toxicities. Additionally, further research is needed to better understand the mechanisms by which nutraceuticals protect against pesticide toxicity and to identify optimal dosages and combinations for effective amelioration.
Author contributions
MS: Formal analysis, Writing – original draft. SS: Investigation, Methodology, Writing – original draft. SKS: Resources, Writing – review & editing. RB: Formal analysis, Writing – original draft. WA: Resources, Writing – original draft. ASA: Software, Writing – original draft. MA: Formal analysis, Writing – original draft. AA: Methodology, Writing – original draft. EV: Resources, Writing – review & editing. MPS: Project administration, Writing – review & editing.
Funding
The author(s) declare that no financial support was received for the research, authorship, and/or publication of this article.
Conflict of interest
The authors declare that the research was conducted in the absence of any commercial or financial relationships that could be construed as a potential conflict of interest.
The author(s) declared that they were an editorial board member of Frontiers, at the time of submission. This had no impact on the peer review process and the final decision.
Publisher’s note
All claims expressed in this article are solely those of the authors and do not necessarily represent those of their affiliated organizations, or those of the publisher, the editors and the reviewers. Any product that may be evaluated in this article, or claim that may be made by its manufacturer, is not guaranteed or endorsed by the publisher.
References
1. Premanandh J. Factors affecting food security and contribution of modern technologies in food sustainability. J Sci Food Agric. (2011) 91:2707–14. doi: 10.1002/jsfa.4666
2. Cerda R, Avelino J, Gary C, Tixier P, Lechevallier E, Allinne C. Primary and secondary yield losses caused by pests and diseases: Assessment and modeling in coffee. PLoS One. (2017) 12:e0169133. doi: 10.1371/journal.pone.0169133
3. Gaur N, Diwan B, Choudhary R. Bioremediation of organic pesticides using nanomaterials. nano-bioremediation: Fundamentals and Applications. Micro Nano Technol. (2022) 2022:517–40. doi: 10.1016/B978-0-12-823962-9.00024-6
4. Pandey MM, Rastogi S, Rawat AKS. Indian traditional ayurvedic system of medicine and nutritional supplementation. Evid Based Complement Alternat Med. (2013) 2013:376327. doi: 10.1155/2013/376327
5. Van Boxstael S, Habib I, Jacxsens L, De Vocht M, Baert L, Van de Perre E, et al. Food safety issues in fresh produce: Bacterial pathogens, viruses and pesticide residues indicated as major concerns by stakeholders in the fresh produce chain. Food Control. (2013) 32:190–7. doi: 10.1016/j.foodcont.2012.11.038
6. Simoglou KB, Roditakis E. Consumers’ Benefit–risk perception on pesticides and food safety–a survey in Greece. Agriculture. (2022) 12:192. doi: 10.3390/agriculture12020192
7. Kim KH, Kabir E, Jahan SA. Exposure to pesticides and the associated human health effects. Sci Total Environ. (2017) 575:525–35. doi: 10.1016/j.scitotenv.2016.09.009
8. Bernardes MFF, Pazin M, Pereira LC, Dorta DJ. Impact of pesticides on environmental and human health. In: Andreazza A, Scola G editors. Toxicology studies-cells, drugs, and environment. London: IntechOpen (2015). p. 195–233. doi: 10.5772/59710
9. Fang Y, Nie Z, Die Q, Tian Y, Liu F, He J, et al. Organochlorine pesticides in soil, air, and vegetation at and around a contaminated site in southwestern China: Concentration, transmission, and risk evaluation. Chemosphere. (2017) 178:340–9. doi: 10.1016/j.chemosphere.2017.02.151
10. Liu Y, Mo R, Tang F, Fu Y, Guo Y. Influence of different formulations on chlorpyrifos behavior and risk assessment in bamboo forest of China. Environ Sci Pollut Res. (2015) 22:20245–54. doi: 10.1007/s11356-015-5272-2
11. Tudi M, Daniel Ruan H, Wang L, Lyu J, Sadler R, Connell D, et al. Agriculture development, pesticide application and its impact on the environment. Int J Environ Res Public Health. (2021) 18:1112. doi: 10.3390/ijerph18031112
12. Zhang W. Global pesticide use: Profile, trend, cost/benefit and more. Proc Int Acad Ecol Environ Sci. (2018) 8:1.
13. Pathak VM, Verma VK, Rawat BS, Kaur B, Babu N, Sharma A, et al. Current status of pesticide effects on environment, human health, and it is eco-friendly management as bioremediation: A comprehensive review. Front Microbiol. (2022) 13:962619. doi: 10.3389/fmicb.2022.962619
14. Ali Mekouar M. 15. Food and agriculture organization of the United Nations (FAO). Yearbook Int Environ Law. (2017) 28:506–20. doi: 10.1093/yiel/yvy073
15. Xavier R, Rekha K, Bairy KL. Health perspective of pesticide exposure and dietary management. Malaysian J Nutr. (2004) 10:39–51.
16. Sachdev S, Ansari SA, Ansari MI, Fujita M, Hasanuzzaman M. Abiotic stress and reactive oxygen species: Generation, signaling, and defense mechanisms. Antioxidants. (2021) 10:277. doi: 10.3390/antiox10020277
17. Nardelli V, D’Amico V, Ingegno M, Della Rovere I, Iammarino M, Casamassima F, et al. Pesticides contamination of cereals and legumes: Monitoring of samples marketed in Italy as a contribution to risk assessment. Appl Sci. (2021) 11:7283. doi: 10.3390/app11167283
18. Terziev V, Petkova-Georgieva S. Human health problems and classification of the most toxic pesticides. Int E J Adv Soc Sci. (2019) 5:8. doi: 10.2139/ssrn.3513837
19. Ahamad A, Kumar J. Pyrethroid pesticides: An overview on classification, toxicological assessment and monitoring. J Hazard Mater Adv. (2023) 10:100284. doi: 10.1016/j.hazadv.2023.100284
20. DiBartolomeis M, Kegley S, Mineau P, Radford R, Klein K. An assessment of acute insecticide toxicity loading (AITL) of chemical pesticides used on agricultural land in the United States. PLoS One. (2019) 14:e0220029. doi: 10.1371/journal.pone.0220029
21. Lushchak VI, Matviishyn TM, Husak VV, Storey JM, Storey KB. Pesticide toxicity: A mechanistic approach. EXCLI J. (2018) 17:1101.
22. Garcia FP, Ascencio SC, Oyarzún JCG, Hernandez AC, Alavarado PV. Pesticides: Classification, uses and toxicity. Measures of exposure and genotoxic risks. J Res Environ Sci Toxicol. (2012) 1:279–93.
23. Abubakar Y, Tijjani H, Egbuna C, Adetunji CO, Kala S, Kryeziu TL, et al. Pesticides, history, and classification. Natural remedies for pest, disease and weed control. Cambridge, MA: Academic Press (2020). p. 29–42. doi: 10.1016/B978-0-12-819304-4.00003-8
24. Lovleen. Differential specification of various insecticides and their environmental degradation. Toxicol Int. (2020) 26:110–28.
25. Chmiel T, Mieszkowska A, Kempińska-Kupczyk D, Kot-Wasik A, Namieśnik J, Mazerska Z. The impact of lipophilicity on environmental processes, drug delivery and bioavailability of food components. Microchem J. (2019) 146:393–406. doi: 10.1016/j.microc.2019.01.030
26. Kauts S, Shabir S, Yousuf S, Mishra Y, Bhardwaj R, Milibari AA, et al. The evidence of in-vivo and in-vitro studies on microplastic and nano plastic toxicity in mammals: A possible threat for an upcoming generation? Phys Chem Earth. (2023) 132:103511. doi: 10.1016/j.pce.2023.103511
27. Bhatt P, Zhou X, Huang Y, Zhang W, Chen S. Characterization of the role of esterases in the biodegradation of organophosphate, carbamate, and pyrethroid pesticides. J Hazard Mater. (2021) 411:125026. doi: 10.1016/j.jhazmat.2020.125026
28. Banjare P, Singh J, Roy PP. Predictive classification-based QSTR models for toxicity study of diverse pesticides on multiple avian species. Environ Sci Pollut Res. (2021) 28:17992–8003. doi: 10.1007/s11356-020-11713-z
29. Kaur R, Mavi GK, Raghav S, Khan I. Pesticides classification and its impact on environment. Int J Curr Microbiol Appl Sci. (2021) 8:1889–97. doi: 10.20546/ijcmas.2019.803.224
30. Yadav IC, Devi NL. Pesticides classification and its impact on human and environment. Environ Sci Eng. (2018) 6:140–58.
31. Mdeni NL, Adeniji AO, Okoh AI, Okoh OO. Analytical evaluation of carbamate and organophosphate pesticides in human and environmental matrices: A review. Molecules. (2022) 27:618. doi: 10.3390/molecules27030618
32. Oguh CE, Okpaka CO, Ubani CS, Okekeaji U, Joseph PS, Amadi EU. Natural pesticides (biopesticides) and uses in pest management-a critical review. Asian J Biotechnol Genet Eng. (2019) 2:1–18.
33. Field LM, Emyr Davies TG, O’reilly AO, Williamson MS, Wallace BA. Voltage-gated sodium channels as targets for pyrethroid insecticides. Eur Biophys J. (2017) 46:675–9. doi: 10.1007/s00249-016-1195-1
34. Damalas CA, Eleftherohorinos IG. Pesticide exposure, safety issues, and risk assessment indicators. Int J Environ Res Public Health. (2011) 8:1402–19. doi: 10.3390/ijerph8051402
35. Baghel AS, Bhardwaj A, Ibrahim W. Optimization of pesticides spray on crops in agriculture using machine learning. Comp Intell Neurosci. (2022) 2022:9408535. doi: 10.1155/2022/9408535
36. Sabarwal A, Kumar K, Singh RP. Hazardous effects of chemical pesticides on human health–Cancer and other associated disorders. Environ Toxicol Pharmacol. (2022) 63:103–14. doi: 10.1016/j.etap.2018.08.018
37. Vopham T, Bertrand KA, Hart JE, Laden F, Brooks MM, Yuan JM, et al. Pesticide exposure and liver cancer: A review. Cancer Causes Control. (2017) 28:177–90. doi: 10.1007/s10552-017-0854-6
38. Muhammad Muddassir MM, Muhammad Shahid MS, Altalb AAT, Ahsan SMW, Muhammad Mubushar MM, Zia MA, et al. Paradigm shift from non-Bt to Bt cotton and factors conducing Bt cotton production in a southern Punjab’s district of Pakistan. AgroLife Sci J. (2017) 6.
39. Carvalho FP. Pesticides, environment, and food safety. Food Energy Secur. (2017) 6:48–60. doi: 10.1002/fes3.108
40. Yin K, Wang Y, Zhao H, Wang D, Guo M, Mu M, et al. A comparative review of microplastics and nanoplastics: Toxicity hazards on digestive, reproductive and nervous system. Sci Total Environ. (2021) 774:145758. doi: 10.1016/j.scitotenv.2021.145758
41. Fuhrimann S, Van den Brenk I, Atuhaire A, Mubeezi R, Staudacher P, Huss A, et al. Recent pesticide exposure affects sleep: A cross-sectional study among smallholder farmers in Uganda. Environ Int. (2022) 158:106878. doi: 10.1016/j.envint.2021.106878
42. Bhardwaj JK, Kumar V, Saraf P, Panchal H, Rathee V, Sachdeva SN. Efficacy of N-acetyl-l-cysteine against glyphosate induced oxidative stress and apoptosis in testicular germ cells preventing infertility. J Biochem Mol Toxicol. (2022) 36:e22979. doi: 10.1002/jbt.22979
43. Ali SN, Ahmad MK, Mahmood R. Sodium chlorate, an herbicide and major water disinfectant byproduct, generates reactive oxygen species and induces oxidative damage in human erythrocytes. Environ Sci Pollut Res. (2017) 24:1898–909. doi: 10.1007/s11356-016-7980-7
44. Khan AU, Jamshaid H, Ud Din F, Zeb A, Khan GM. Designing, optimization and characterization of Trifluralin transfersomal gel to passively target cutaneous leishmaniasis. J Pharm Sci. (2022) 111:1798–811. doi: 10.1016/j.xphs.2022.01.010
45. Fukuyama T, Kosaka T, Hayashi K, Miyashita L, Tajima Y, Wada K, et al. Immunotoxicity in mice induced by short-term exposure to methoxychlor, parathion, or piperonyl butoxide. J Immunotoxicol. (2013) 10:150–9. doi: 10.3109/1547691X.2012.703252
46. Vonder Embse AN, Elmore SE, Jackson KB, Habecker BA, Manz KE, Pennell KD, et al. Developmental exposure to DDT or DDE alters sympathetic innervation of brown adipose in adult female mice. Environ Health. (2021) 20:1–16. doi: 10.1186/s12940-021-00721-2
47. Wang Z, Wu Q, Li X, Klaunig JE. Constitutive androstane receptor (CAR) mediates dieldrin-induced liver tumorigenesis in mouse. Arch Toxicol. (2020) 94:2873–84. doi: 10.1007/s00204-020-02781-8
48. Bao J, Zhang Y, Wen R, Zhang L, Wang X. Low level of mancozeb exposure affects ovary in mice. Ecotoxicol Environ Saf. (2022) 239:113670. doi: 10.1016/j.ecoenv.2022.113670
49. Khisroon M, Hassan N, Khan A, Farooqi J. Assessment of DNA damage induced by endosulfan in grass carp (Ctenopharyngodon idella Valenciennes, 1844). Environ Sci Pollut Res. (2021) 28:15551–5. doi: 10.1007/s11356-021-12727-x
50. Singh S, Kumar V, Kanwar R, Wani AB, Gill JPK, Garg VK, et al. Toxicity, and detoxification of monocrotophos from ecosystem using different approaches: A. review. Chemosphere. (2021) 275:130051. doi: 10.1016/j.chemosphere.2021.130051
51. Mishra S, Zhang W, Lin Z, Pang S, Huang Y, Bhatt P, et al. Carbofuran toxicity and its microbial degradation in contaminated environments. Chemosphere. (2020) 259:127419. doi: 10.1016/j.chemosphere.2020.127419
52. Arici M, Abudayyak M, Boran T, Özhan G. Does pendimethalin develop in pancreatic cancer induced inflammation? Chemosphere. (2020) 252:126644. doi: 10.1016/j.chemosphere.2020.126644
53. Alavi J, Maroufi A, Mirzaghaderi G. Trifluralin-mediated polyploidization of fenugreek (Trigonella feonum-graecum L.) using in vitro embryo culture. Acta Physiol Plant. (2022) 44:97. doi: 10.1007/s11738-022-03436-0
54. Saquib Q, Faisal M, Ansari SM, Wahab R. Phorate triggers oxidative stress and mitochondrial dysfunction to enhance micronuclei generation and DNA damage in human lymphocytes. Saudi J Biol Sci. (2019) 26:1411–7. doi: 10.1016/j.sjbs.2019.04.008
55. Inoue T, Kinoshita M, Oyama K, Kamemura N, Oyama Y. Captan-induced increase in the concentrations of intracellular Ca2+ and Zn2+ and its correlation with oxidative stress in rat thymic lymphocytes. Environ Toxicol Pharmacol. (2018) 63:78–83. doi: 10.1016/j.etap.2018.08.017
56. Sarkar S, Kotteeswaran V. Green synthesis of silver nanoparticles from aqueous leaf extract of Pomegranate (Punica granatum) and their anticancer activity on human cervical cancer cells. Adv Nat Sci Nanosci Nanotechnol. (2018) 9:025014. doi: 10.1088/2043-6254/aac590
57. Bist R, Chaudhary B, Bhatt DK. Defensive proclivity of bacoside A and bromelain against oxidative stress and AChE gene expression induced by dichlorvos in the brain of Mus musculus. Sci Rep. (2021) 11:1–11. doi: 10.1038/s41598-021-83289-8
58. Singh P, Verma PK, Raina R, Sood S, Sharma P. Maximum contaminant level of arsenic in drinking water potentiates quinalphos-induced renal damage on coadministration of both arsenic and quinalphos in Wistar rats. Environ Sci Pollut Res. (2020) 27:21331–40. doi: 10.1007/s11356-020-08643-1
59. Lu J, Wu Q, Yang Q, Li G, Wang R, Liu Y, et al. Molecular mechanism of reproductive toxicity induced by beta-cypermethrin in zebrafish. Comp Biochem Physiol C Toxicol Pharmacol. (2021) 239:108894. doi: 10.1016/j.cbpc.2020.108894
60. Piner P, Üner N. In vivo acetylcholinesterase inhibition in the tissues of spinosad exposed Oreochromis niloticus. Environ Toxicol Pharmacol. (2012) 34:473–7. doi: 10.1016/j.etap.2012.06.012
61. Zhang J, Liu H, Sun Z, Xie J, Zhong G, Yi X. Azadirachtin induced apoptosis in the prothoracic gland in Bombyx mori and a pronounced Ca2+ release effect in Sf9 cells. Int J Biol Sci. (2017) 13:1532. doi: 10.7150/ijbs.22175
62. Yang C, Lim W, Song G. Mediation of oxidative stress toxicity induced by pyrethroid pesticides in fish. Comp Biochem Physiol C Toxicol Pharmacol. (2020) 234:108758. doi: 10.1016/j.cbpc.2020.108758
63. Dar MA, Khan AM, Raina R, Verma PK, Wani NM. Effect of bifenthrin on oxidative stress parameters in the liver, kidneys, and lungs of rats. Environ Sci Pollut Res. (2019) 26:9365–70. doi: 10.1007/s11356-019-04362-4
64. Xu Z, Zhu L, Yang Y, Zhang Y, Lu M, Tao L, et al. Bifenthrin induces DNA damage and autophagy in Spodoptera frugiperda (Sf9) insect cells. Vitro Cell Dev Biol Anim. (2021) 57:264–71. doi: 10.1007/s11626-021-00554-w
65. Sule RO, Condon L, Gomes AV. A common feature of pesticides: Oxidative stress–The role of oxidative stress in pesticide-induced toxicity. Oxid Med Cell Longev. (2022) 2022:5563759. doi: 10.1155/2022/5563759
66. Cicchetti F, Drouin-Ouellet J, Gross RE. Environmental toxins, and Parkinson’s disease: What have we learned from pesticide-induced animal models. Trends Pharmacol Sci. (2009) 30:475–83. doi: 10.1016/j.tips.2009.06.005
67. Kaur K, Kaur R. Occupational pesticide exposure, impaired DNA repair, and diseases. Indian J Occup Environ Med. (2018) 22:74. doi: 10.4103/ijoem.IJOEM_45_18
68. Shah HK, Sharma T, Banerjee BD. Organochlorine pesticides induce inflammation, ROS production, and DNA damage in human epithelial ovary cells: An in vitro study. Chemosphere. (2020) 246:125691. doi: 10.1016/j.chemosphere.2019.125691
69. Colle D, Farina M, Ceccatelli S, Raciti M. Paraquat and maneb exposure alters rat neural stem cell proliferation by inducing oxidative stress: New insights on pesticide-induced neurodevelopmental toxicity. Neurotox Res. (2021) 34:820–33. doi: 10.1007/s12640-018-9916-0
70. Gupta PK. Toxicity of herbicides. In: Gupta RC editor. Veterinary toxicology. Cambridge, MA: Academic Press (2018). p. 553–67. doi: 10.1016/B978-0-12-811410-0.00044-1
71. Chedik L, Bruyere A, Bacle A, Potin S, Le Vée M, Fardel O. Interactions of pesticides with membrane drug transporters: Implications for toxicokinetics and toxicity. Expert Opin Drug Metab Toxicol. (2018) 14:739–52. doi: 10.1080/17425255.2018.1487398
72. Maheshwari N, Khan FH, Mahmood R. Pentachlorophenol-induced cytotoxicity in human erythrocytes: Enhanced generation of ROS and RNS, lowered antioxidant power, inhibition of glucose metabolism, and morphological changes. Environ Sci Pollut Res. (2019) 26:12985–3001. doi: 10.1007/s11356-019-04736-8
73. Eleršek T, Filipič M. Organophosphorus pesticides-mechanisms of their toxicity. In: M Stoytcheva editor. Pesticides-the impacts of pesticide exposure. New York, NY: Intech (2011). p. 243–60.
74. Bhardwaj JK, Saraf PN-. acetyl-l-cysteine mediated regulation of DNA fragmentation, an apoptotic event, against methoxychlor toxicity in the granulosa cells of ovarian antral follicles. Mutat Res Genet Toxicol Environ Mutagen. (2020) 858:503222. doi: 10.1016/j.mrgentox.2020.503222
75. Li H, Wang F, Li J, Deng S, Zhang S. Adsorption of three pesticides on polyethylene microplastics in aqueous solutions: Kinetics, isotherms, thermodynamics, and molecular dynamics simulation. Chemosphere. (2021) 264:128556. doi: 10.1016/j.chemosphere.2020.128556
76. Decourt B, Lahiri D, Sabbagh M. Targeting tumor necrosis factor alpha for Alzheimer’s disease. Curr Alzheimer Res. (2017) 14:412–25. doi: 10.2174/1567205013666160930110551
77. Zhu S, Liu Y, Li Y, Yi J, Yang B, Li Y, et al. The potential risks of herbicide butachlor to immunotoxicity via induction of autophagy and apoptosis in the spleen. Chemosphere. (2022) 286:131683. doi: 10.1016/j.chemosphere.2021.131683
78. Wang X, Martínez MA, Dai M, Chen D, Ares I, Romero A, et al. Permethrin-induced oxidative stress and toxicity and metabolism. A review. Environ Res. (2016) 149:86–104. doi: 10.1016/j.envres.2016.05.003
79. Murphy EP, Crean D. Molecular interactions between NR4A orphan nuclear receptors and NF-κB are required for appropriate inflammatory responses and immune cell homeostasis. Biomolecules. (2015) 5:1302–18. doi: 10.3390/biom5031302
80. Bordoni L, Nasuti C, Mirto M, Caradonna F, Gabbianelli R. Intergenerational effect of early life exposure to permethrin: Changes in global DNA methylation and in Nurr1 gene expression. Toxics. (2015) 3:451–61. doi: 10.3390/toxics3040451
81. Kamal S, Junaid M, Bibi I, Rehman S, Rehman K, Akash MSH. Role of pesticides as EDCs in metabolic disorders. In: Akash MS, Rehman K, and Hashmi MZ editors. Endocrine disrupting chemicals-induced metabolic disorders and treatment strategies. New York, NY: Springer International Publishing (2021). p. 265–300. doi: 10.1007/978-3-030-45923-9_17
82. Lee JH, Mohan CD, Basappa S, Rangappa S, Chinnathambi A, Alahmadi TA, et al. The IκB kinase inhibitor ACHP targets the STAT3 signaling pathway in human non-small cell lung carcinoma cells. Biomolecules. (2019) 9:875. doi: 10.3390/biom9120875
83. Khalifa AG, Moselhy WA, Mohammed HM, Khalil F, Shaban M, El-Nahass ES, et al. Deltamethrin and its nanoformulations induce behavioral alteration and toxicity in rat brain through oxidative stress and JAK2/STAT3 signaling pathway. Toxics. (2022) 10:303. doi: 10.3390/toxics10060303
84. Singh NS, Sharma R, Parween T, Patanjali PK. Pesticide contamination and human health risk factor. In: Oves M, Zain Khan M, Ismail M editors. Modern age environmental problems and their remediation. Cham: Springer (2018). p. 49–68. doi: 10.1007/978-3-319-64501-8_3
85. Sharma H, Hirko AC, King MA, Liu B. Role of NADPH oxidase in cooperative reactive oxygen species generation in dopaminergic neurons induced by combined treatment with dieldrin and lindane. Toxicol Lett. (2018) 299:47–55. doi: 10.1016/j.toxlet.2018.09.006
86. Lee GH, Choi KC. Adverse effects of pesticides on the functions of immune system. Comp Biochem Physiol C Toxicol Pharmacol. (2020) 235:108789. doi: 10.1016/j.cbpc.2020.108789
87. Shabir S, Singh MP. The aging: Introduction, theories, principles, and future prospective. Anti-aging drug discovery on the basis of hallmarks of aging. Amsterdam: Elsevier (2022). p. 1–17. doi: 10.1016/B978-0-323-90235-9.00017-3
88. Farkhondeh T, Mehrpour O, Forouzanfar F, Roshanravan B, Samarghandian S. Oxidative stress and mitochondrial dysfunction in organophosphate pesticide-induced neurotoxicity and its amelioration: A review. Environ Sci Pollut Res. (2020) 27:24799–814. doi: 10.1007/s11356-020-09045-z
89. Bouaziz C, Graiet I, Salah A, Ben Salem I, Abid S. Influence of bifentrin, a pyrethriod pesticide, on human colorectal HCT-116 cells attributed to alterations in oxidative stress involving mitochondrial apoptotic processes. J Toxicol Environ Health. (2020) 83:331–40. doi: 10.1080/15287394.2020.1755756
90. Dash AP, Raghavendra K, Pillai MKK. Resurrection of DDT: A critical appraisal. Indian J Med Res. (2007) 126:1.
91. Chopra G, Shabir S, Yousuf S, Kauts S, Bhat SA, Mir AH, et al. Proteinopathies: Deciphering physiology and mechanisms to develop effective therapies for neurodegenerative diseases. Mol Neurobiol. (2022) 59:7513–40. doi: 10.1007/s12035-022-03042-8
92. Richardson JR, Fitsanakis V, Westerink RH, Kanthasamy AG. Neurotoxicity of pesticides. Acta Neuropathol. (2019) 138:343–62. doi: 10.1007/s00401-019-02033-9
93. Singh MP, Chakrabarty R, Shabir S, Yousuf S, Obaid AA, Moustafa M, et al. Influence of the gut microbiota on the development of neurodegenerative diseases. Mediat Inflamm. (2022) 2022:3300903. doi: 10.1155/2022/3300903
94. Zeng X, Du Z, Ding X, Jiang W. Protective effects of dietary flavonoids against pesticide-induced toxicity: A review. Trends Food Sci Technol. (2021) 109:271–9. doi: 10.1016/j.tifs.2021.01.046
95. Wong HL, Garthwaite DG, Ramwell CT, Brown CD. Assessment of occupational exposure to pesticide mixtures with endocrine-disrupting activity. Environ Sci Pollut Res. (2019) 26:1642–53. doi: 10.1007/s11356-018-3676-5
96. Bretveld RW, Thomas CM, Scheepers PT, Zielhuis GA, Roeleveld N. Pesticide exposure: The hormonal function of the female reproductive system disrupted? Reprod Biol Endocrinol. (2006) 4:1–14. doi: 10.1186/1477-7827-4-30
97. Johansson HK, Christiansen S, Draskau MK, Svingen T, Boberg J. Classical toxicity endpoints in female rats are insensitive to the human endocrine disruptors diethylstilbestrol and ketoconazole. Reprod Toxicol. (2021) 101:9–17. doi: 10.1016/j.reprotox.2021.01.003
98. Jacobsen-Pereira CH, Dos Santos CR, Maraslis FT, Pimentel L, Feijó AJL, Silva CI, et al. Markers of genotoxicity and oxidative stress in farmers exposed to pesticides. Ecotoxicol Environ Saf. (2018) 148:177–83. doi: 10.1016/j.ecoenv.2017.10.004
99. Perumalla Venkata R, Rahman MF, Mahboob M, Indu Kumari S, Chinde S, Dumala N, et al. Assessment of genotoxicity in female agricultural workers exposed to pesticides. Biomarkers. (2017) 22:446–54. doi: 10.1080/1354750X.2016.1252954
100. Bolognesi C, Creus A, Ostrosky-Wegman P, Marcos R. Micronuclei, and pesticide exposure. Mutagenesis. (2011) 26:19–26. doi: 10.1093/mutage/geq070
101. Blair A, Freeman LB. Epidemiologic studies in agricultural populations: Observations and future directions. J Agromed. (2009) 14:125–31. doi: 10.1080/10599240902779436
102. Benigni R, Laura Battistelli C, Bossa C, Giuliani A, Fioravanzo E, Bassan A, et al. Evaluation of the applicability of existing (Q) SAR models for predicting the genotoxicity of pesticides and similarity analysis related with genotoxicity of pesticides for facilitating of grouping and read across. EFSA Support Publ. (2019) 16:1598E. doi: 10.1016/j.yrtph.2020.104658
103. Radha, Kumar M, Puri S, Pundir A, Bangar SP, Changan S, et al. Evaluation of nutritional, phytochemical, and mineral composition of selected medicinal plants for therapeutic uses from cold desert of Western Himalaya. Plants. (2021) 10:1429. doi: 10.3390/plants10071429
104. Pathak K, Pathak MP, Saikia R, Gogoi U, Sahariah JJ, Zothantluanga JH, et al. Cancer chemotherapy via natural bioactive compounds. Curr Drug Discov Technol. (2022) 19:4–23. doi: 10.2174/1570163819666220331095744
105. De Filippis LF. Plant secondary metabolites: From molecular biology to health products. In: Azooz MM, Ahmad P editors. Plant-environment interaction: Responses and approaches to mitigate stress. New York, NY: Wiley (2016). p. 263–99. doi: 10.1002/9781119081005.ch15
106. Keihanian F, Moohebati M, Saeidinia A, Mohajeri SA, Madaeni S. Therapeutic effects of medicinal plants on isoproterenol-induced heart failure in rats. Biomed Pharmacother. (2021) 134:111101. doi: 10.1016/j.biopha.2020.111101
107. Zhu T, Wang L, Wang LP, Wan Q. Therapeutic targets of neuroprotection and neurorestoration in ischemic stroke: Applications for natural compounds from medicinal herbs. Biomed Pharmacother. (2022) 148:112719. doi: 10.1016/j.biopha.2022.112719
108. Dutra RC, Campos MM, Santos AR, Calixto JB. Medicinal plants in Brazil: Pharmacological studies, drug discovery, challenges, and perspectives. Pharmacol Res. (2016) 112:4–29. doi: 10.1016/j.phrs.2016.01.021
109. Barkat MA, Goyal A, Barkat HA, Salauddin M, Pottoo FH, Anwer ET. Herbal medicine: Clinical perspective and regulatory status. Comb Chem High Throughput Screen. (2021) 24:1573–82. doi: 10.2174/1386207323999201110192942
110. Jahangir MA, Anand C, Muheem A, Gilani SJ, Taleuzzaman M, Zafar A, et al. Nano phytomedicine based delivery system for CNS disease. Curr Drug Metab. (2020) 21:661–73. doi: 10.2174/1389200221666200523161003
111. Maqbool Z, Arshad MS, Ali A, Aziz A, Khalid W, Afzal MF, et al. Potential role of phytochemical extract from saffron in development of functional foods and protection of brain-related disorders. Oxid Med Cell Longev. (2022) 2022:6480590. doi: 10.1155/2022/6480590
112. Dey P, Kundu A, Chakraborty HJ, Kar B, Choi WS, Lee BM, et al. Therapeutic value of steroidal alkaloids in cancer: Current trends and future perspectives. Int J Cancer. (2019) 145:1731–44. doi: 10.1002/ijc.31965
113. Tiwari P, Sangwan RS, Sangwan NS. Plant secondary metabolism linked glycosyltransferases: An update on expanding knowledge and scopes. Biotechnol Adv. (2016) 34:714–39. doi: 10.1016/j.biotechadv.2016.03.006
114. Pagare S, Bhatia M, Tripathi N, Pagare S, Bansal YK. Secondary metabolites of plants and their role: Overview. Curr Trends Biotechnol Pharm. (2015) 9:293–304.
115. Jafari-Nozad AM, Jafari A, Aschner M, Farkhondeh T, Samarghandian S. Curcumin combats against organophosphate pesticides toxicity: A review of the current evidence and molecular pathways. Curr Med Chem. (2023) 30:2312–39. doi: 10.2174/0929867329666220817125800
116. Jabłońska-Trypuć A, Wiater J. Protective effect of plant compounds in pesticides toxicity. J Environ Health Sci Eng. (2022) 20:1035–45. doi: 10.1007/s40201-022-00823-0
117. Hossen MS, Tanvir EM, Prince MB, Paul S, Saha M, Ali MY, et al. Protective mechanism of turmeric (Curcuma longa) on carbofuran-induced hematological and hepatic toxicities in a rat model. Pharm Biol. (2022) 55:1937–45. doi: 10.1080/13880209.2017.1345951
118. Alp H, Aytekin I, Hatipoglu NK, Alp A, Ogun M. Effects of sulforophane and curcumin on oxidative stress created by acute malathion toxicity in rats. Eur Rev Med Pharmacol Sci. (2012) 16:144–8.
119. Kumar R, Ali M, Kumar A, Gahlot V. Comparative bioremedial effect of Withania somnifera and curcuma longa on ovaries of pesticide induced mice. Eur J Pharm Med Res. (2015) 2:249–53.
120. Saber TM, Abo-Elmaaty AMA, Abdel-Ghany HM. Curcumin mitigates mancozeb-induced hepatotoxicity and genotoxicity in rats. Ecotoxicol Environ Saf. (2019) 183:109467. doi: 10.1016/j.ecoenv.2019.109467
121. Sharma P, Huq AU, Singh R. Cypermethrin-induced reproductive toxicity in the rat is prevented by resveratrol. J Hum Reprod Sci. (2014) 7:99. doi: 10.4103/0974-1208.138867
122. Jahan S, Kumar D, Singh S, Kumar V, Srivastava A, Pandey A, et al. Resveratrol prevents the cellular damages induced by monocrotophos via PI3K signalling pathway in human cord blood mesenchymal stem cells. Mol Neurobiol. (2018) 55:8278–92. doi: 10.1007/s12035-018-0986-z
123. Kumar V, Tripathi VK, Singh AK, Lohani M, Kuddus M. Trans-resveratrol restores the damages induced by organophosphate pesticide-monocrotophos in neuronal cells. Toxicol Int. (2013) 20:48–55. doi: 10.4103/0971-6580.111571
124. Akinmoladun AC, Oladejo CO, Josiah SS, Famusiwa CD, Ojo OB, Olaleye MT. Catechin, quercetin and taxifolin improve redox and biochemical imbalances in rotenone-induced hepatocellular dysfunction: Relevance for therapy in pesticide-induced liver toxicity. Pathophysiology. (2018) 25:365–71. doi: 10.1016/j.pathophys.2018.07.002
125. Ahmadian E, Eftekhari A, Kavetskyy T, Khosroushahi AY, Turksoy VA, Khalilov R. Effects of quercetin loaded nanostructured lipid carriers on the paraquat-induced toxicity in human lymphocytes. Pestic Biochem Physiol. (2020) 167:104586. doi: 10.1016/j.pestbp.2020.104586
126. Baldissera MD, Souza CF, Parmeggiani B, Vendrusculo RG, Ribeiro LC, Muenchen DK. Protective effects of diet containing rutin against trichlorfon-induced muscle bioenergetics disruption and impairment on fatty acid profile of silver catfish Rhamdia quelen. Ecotoxicol Environ Saf. (2020) 205:111127. doi: 10.1016/j.ecoenv.2020.111127
127. Banerjee BD, Seth V, Ahmed RS. Pesticide-induced oxidative stress: Perspective and trends. Rev Environ Health. (2001) 16:1–40. doi: 10.1515/reveh.2001.16.1.1
128. Gandhi S, Abramov AY. Mechanism of oxidative stress in neurodegeneration. Oxid Med Cell Longev. (2012) 2012:428010. doi: 10.1155/2012/428010
129. Küçükler S, Kandemir FM, Özdemir S, Çomaklı S, Caglayan C. Protective effects of rutin against deltamethrin-induced hepatotoxicity and nephrotoxicity in rats via regulation of oxidative stress, inflammation, and apoptosis. Environ Sci Pollut Res Int. (2021) 28:62975–90. doi: 10.1007/s11356-021-15190-w
130. Nimse SB, Pal D. Free radicals, natural antioxidants, and their reaction mechanisms. RSC Adv. (2015) 5:27986–8006. doi: 10.1039/C4RA13315C
131. Dhouib IE, Jallouli M, Annabi A, Gharbi N, Elfazaa S, Lasram MM. A minireview on N-acetylcysteine: An old drug with new approaches. Life Sci. (2016) 151:359–63. doi: 10.1016/j.lfs.2016.03.003
132. Aboubakr HM, Elzohairy EA, Ali AA, Rashed LA, Elkady NK, Soliman AS. Therapeutic effects of N-acetylcysteine against malathion-induced hepatotoxicity. Egypt J Forens Sci. (2019) 9:1–9. doi: 10.1186/s41935-019-0142-6
133. Ahmed T, Tripathi AK, Ahmed RS, Das S, Suke SG, Pathak R, et al. Endosulfan-induced apoptosis, and glutathione depletion in human peripheral blood mononuclear cells: Attenuation by N-acetylcysteine. J Biochem Mol Toxicol. (2008) 22:299–304. doi: 10.1002/jbt.20240
134. Mahmoud SM, Abdel Moneim AE, Qayed MM, El-Yamany NA. Potential role of N-acetylcysteine on chlorpyrifos-induced neurotoxicity in rats. Environ Sci Pollut Res Int. (2019) 26:20731–41. doi: 10.1007/s11356-019-05366-w
135. Emam H, Ahmed E, Abdel-Daim M. Antioxidant capacity of omega-3-fatty acids and vitamin E against imidacloprid-induced hepatotoxicity in Japanese quails. Environ Sci Pollut Res. (2018) 25:11694–702. doi: 10.1007/s11356-018-1481-9
136. Moneim AEA, Dkhil MA, Al-Quraishy S. The potential role of Portulaca oleracea as a neuroprotective agent in rotenone-induced neurotoxicity and apoptosis in the brain of rats. Pestic Biochem Physiol. (2013) 105:203–12. doi: 10.1016/j.pestbp.2013.02.004
137. Aljadani NA, Elnaggar MH, Assaggaff AI. The role of fish oil and evening primrose oil against the toxicity of fenitrothion pesticide in male rats. Int J Pharm Res Allied Sci. (2020) 9:108–22.
138. Avci B, Bilge SS, Arslan G, Alici O, Darakci O, Baratzada T, et al. Protective effects of dietary omega-3 fatty acid supplementation on organophosphate poisoning. Toxicol Ind Health. (2018) 34:69–82. doi: 10.1177/0748233717737646
139. Elzoghby RR, Ahlam FH, Abdel-Fatah A, Farouk M. Protective role of vitamin C and green tea extract on malathion-induced hepatotoxicity and nephrotoxicity in rats. Am J Pharmacol Toxicol. (2014) 9:177. doi: 10.3844/ajptsp.2014.177.188
140. Aly N, Kawther EG, Mahmoud F, El-Sebae AK. Protective effect of vitamin C against chlorpyrifos oxidative stress in male mice. Pestic Biochem Physiol. (2010) 97:7–12. doi: 10.1016/j.pestbp.2009.11.007
141. Sun Y, Zhang J, Song W, Shan A. Vitamin E alleviates phoxim-induced toxic effects on intestinal oxidative stress, barrier function, and morphological changes in rats. Environ Sci Pollut Res Int. (2018) 25:26682–92. doi: 10.1007/s11356-018-2666-y
142. Sharma RK, Sharma B. In-vitro carbofuran induced genotoxicity in human lymphocytes and its mitigation by vitamins C and E. Dis Mark. (2012) 32:153–63. doi: 10.3233/DMA-2011-0870
143. Singh M, Kaur P, Sandhir R, Kiran R. Protective effects of vitamin E against atrazine-induced genotoxicity in rats. Mutat Res Genet Toxicol Environ Mutagen. (2008) 654:145–9. doi: 10.1016/j.mrgentox.2008.05.010
144. Eroglu S, Pandir D, Uzun FG, Bas H. Protective role of vitamins C and E in diclorvos-induced oxidative stress in human erythrocytes in vitro. Biol Res. (2013) 46:33–8. doi: 10.4067/S0716-97602013000100005
145. Malik V, Singh J, Kumar A, Kumar V. Protective effect of coenzyme Q10 nanoparticles against monocrotophos induced oxidative stress in kidney tissues of rats. Biologia. (2021) 76:1849–57. doi: 10.1007/s11756-021-00732-x
146. Amit K, Jagjeet S, Annu P, Vijay K. Protective effects of coenzyme Q10 nanoparticles on hepatotoxicity induced by monocrotophos in rats. Res J Biotechnol. (2022) 17:8. doi: 10.25303/1708rjbt001008
147. Mantle D, Hargreaves IP. Organophosphate poisoning and coenzyme Q10: An overview. Br J Neurosci Nurs. (2018) 14:206–14. doi: 10.12968/bjnn.2018.14.5.206
148. Mohamed Z, El-Kader AEK, Salah-Eldin AE, Mohamed O, Awadalla EA. Protective effects of curcumin against acetamiprid-induced neurotoxicity in male albino rats. Biol Bull. (2023) 24:1–13.
149. Kadeche L, Bourogaa E, Boumendjel A, Djeffal A, Abdennour C, El Feki A, et al. Quercetin attenuates metribuzin-induced biochemical and hematological toxicity in adult rats. Int J Pharm Sci Rev Res. (2016) 40:38–46.
150. Medithi S, Jonnalagadda PR, Jee B. Predominant role of antioxidants in ameliorating the oxidative stress induced by pesticides. Arch Environ Occup Health. (2021) 76:61–74. doi: 10.1080/19338244.2020.1750333
151. Saafi EB, Louedi M, Elfeki A, Zakhama A, Najjar MF, Hammami M, et al. Protective effect of date palm fruit extract (Phoenix dactylifera L.) on dimethoate induced-oxidative stress in rat liver. Exp Toxicol Pathol. (2011) 63:433–41. doi: 10.1016/j.etp.2010.03.002
152. Rice PJ, Rice PJ, Arthur EL, Barefoot AC. Advances in pesticide environmental fate and exposure assessments. J Agric Food Chem. (2007) 55:5367–76. doi: 10.1021/jf063764s
153. Alavanja MC, Hoppin JA, Kamel F. Health effects of chronic pesticide exposure: Cancer and neurotoxicity. Annu Rev Public Health. (2004) 25:155–97. doi: 10.1146/annurev.publhealth.25.101802.123020
154. Bhatia R, Wernham A. Integrating human health into environmental impact assessment: An unrealized opportunity for environmental health and justice. Environ Health Perspect. (2008) 116:991–1000. doi: 10.1289/ehp.11132
155. Al-Jawhari H, Bin-Thiyab H, Elbialy N. In vitro antioxidant and anticancer activities of cupric oxide nanoparticles synthesized using spinach leaves extract. Nano Struct Nano Objects. (2022) 29:100815. doi: 10.1016/j.nanoso.2021.100815
156. Singh MP, Shabir S, Deopa AS, Raina SR, Bantun F, Jalal NA, et al. Synthesis of green engineered silver nanoparticles through Urtica dioica: An inhibition of microbes and alleviation of cellular and organismal toxicity in Drosophila melanogaster. Antibiotics. (2022) 11:1690. doi: 10.3390/antibiotics11121690
157. Yousuf S, Shabir S, Kauts S, Minocha T, Obaid AA, Khan AA, et al. Appraisal of the antioxidant activity, polyphenolic content, and characterization of selected himalayan herbs: Anti-proliferative potential in HepG2 cells. Molecules. (2022) 27:8629. doi: 10.3390/molecules27238629
158. Singh MP, Himalian R, Shabir S, Obaid AA, Alamri AS, Galanakis CM, et al. Protection of phytoextracts against rotenone-induced organismal toxicities in drosophila melanogaster via the attenuation of ROS generation. Appl Sci. (2022) 12:9822. doi: 10.3390/app12199822
159. Panighel G, Ferrarese I, Lupo MG, Sut S, Dall’Acqua S, Ferri N. Investigating the in vitro mode of action of okra (Abelmoschus esculentus) as hypocholesterolemic, anti-inflammatory, and antioxidant food. Food Chem Mol Sci. (2022) 5:100126. doi: 10.1016/j.fochms.2022.100126
160. Khor KZ, Lim V, Moses EJ, Abdul Samad N. The in vitro and in vivo anticancer properties of Moringa oleifera. Evid Based Complement Alternat Med. (2018) 2018:1071243. doi: 10.1155/2018/1071243
161. Sidorova YS, Shipelin VA, Petrov NA, Zorin SN, Mazo VK. Anxiolytic and antioxidant effect of phytoecdysteroids and polyphenols from Chenopodium quinoa on an in vivo restraint stress model. Molecules. (2022) 27:9003. doi: 10.3390/molecules27249003
162. Peng J, Hu T, Li J, Du J, Zhu K, Cheng B, et al. Shepherd’s purse polyphenols exert its anti-inflammatory and antioxidative effects associated with suppressing MAPK and NF-κB pathways and heme oxygenase-1 activation. Oxid Med Cell Longev. (2019) 2019:7202695. doi: 10.1155/2019/7202695
163. Shabir S, Yousuf S, Singh SK, Vamanu E, Singh MP. Ethnopharmacological Effects of Urtica dioica, Matricaria chamomilla, and Murraya koenigii on rotenone-exposed, D. melanogaster: An attenuation of cellular, biochemical, and organismal markers. Antioxidants. (2022) 11:1623. doi: 10.3390/antiox11081623
164. Termini D, Den Hartogh DJ, Jaglanian A, Tsiani E. Curcumin against prostate cancer: Current evidence. Biomolecules. (2020) 10:1536. doi: 10.3390/biom10111536
165. Banerjee S, Katiyar P, Kumar V, Saini SS, Varshney R, Krishnan V, et al. Black pepper and piperine induce anticancer effects on leukemia cell line. Toxicol Res. (2021) 10:169–82. doi: 10.1093/toxres/tfab001
166. Thuphairo K, Sornchan P, Suttisansanee U. Bioactive compounds, antioxidant activity and inhibition of key enzymes relevant to Alzheimer’s disease from sweet pepper (Capsicum annuum) extracts. Prevent Nutr Food Sci. (2019) 24:327. doi: 10.3746/pnf.2019.24.3.327
167. Kulkarni RA, Deshpande AR. Anti-inflammatory, and antioxidant effect of ginger in tuberculosis. J Complement Integr Med. (2016) 13:201–6. doi: 10.1515/jcim-2015-0032
168. Yousuf S, Shabir S, Singh MP. Protection against drug-induced liver injuries through nutraceuticals via amelioration of Nrf-2 signaling. J Am Nutr Assoc. (2022) 42:495–515. doi: 10.1080/27697061.2022.2089403
169. Jiang Y, Jiang Z, Ma L, Huang Q. Advances in nano delivery of green tea catechins to enhance the anticancer activity. Molecules. (2021) 26:3301. doi: 10.3390/molecules26113301
170. Lee SJ, Oh S, Kim MJ, Sim GS, Moon TW, Lee J. Oxidative stability of extracts from red ginseng and puffed red ginseng in bulk oil or oil-in-water emulsion matrix. J.Ginseng Res. (2018) 42:320–6. doi: 10.1016/j.jgr.2017.04.002
171. Shi Q, Lang W, Wang S, Li G, Bai X, Yan X, et al. Echinacea polysaccharide attenuates lipopolysaccharide induced acute kidney injury by inhibiting inflammation, oxidative stress and the MAPK signaling pathway. Int J Mol Med. (2021) 47:243–55. doi: 10.3892/ijmm.2020.4769
172. Sethi G, Shanmugam MK, Warrier S, Merarchi M, Arfuso F, Kumar AP, et al. Pro-apoptotic and anticancer properties of diosgenin: A comprehensive and critical review. Nutrients. (2018) 10:645. doi: 10.3390/nu10050645
173. Wijekoon C, Netticadan T, Siow YL, Sabra A, Yu L, Raj P, et al. Potential associations among bioactive molecules, antioxidant activity and resveratrol production in Vitis vinifera fruits of North America. Molecules. (2022) 27:336. doi: 10.3390/molecules27020336
174. Baby B, Antony P, Vijayan R. Antioxidant and anticancer properties of berries. Crit rev Food Sci Nutr. (2018) 58:2491–507. doi: 10.1080/10408398.2017.1329198
175. Maya-Cano DA, Arango-Varela S, Santa-Gonzalez GA. Phenolic compounds of blueberries (Vaccinium spp) as a protective strategy against skin cell damage induced by ROS: A review of antioxidant potential and antiproliferative capacity. Heliyon. (2021) 7:e06297. doi: 10.1016/j.heliyon.2021.e06297
176. Soleimanifar M, Niazmand R, Jafari SM. Evaluation of oxidative stability, fatty acid profile, and antioxidant properties of black cumin seed oil and extract. J Food Measur Character. (2019) 13:383–9. doi: 10.1007/s11694-018-9953-7
177. Zhao D, Wei J, Hao J, Han X, Ding S, Yang L, et al. Effect of sodium carbonate solution pretreatment on drying kinetics, antioxidant capacity changes, and final quality of wolfberry (Lycium barbarum) during drying. LWT. (2019) 99:254–61. doi: 10.1016/j.lwt.2018.09.066
178. Yu L, Gao B, Li Y, Wang TT, Luo Y, Wang J, et al. Home food preparation techniques impacted the availability of natural antioxidants and bioactivities in kale and broccoli. Food Funct. (2018) 9:585–93. doi: 10.1039/c7fo00948h
179. Hossain MN, De Leo V, Tamborra R, Laselva O, Ingrosso C, Daniello V, et al. Characterization of anti-proliferative and antioxidant effects of nanosized vesicles from Brassica oleracea L.(Broccoli). Sci Rep. (2022) 12:14362. doi: 10.1038/s41598-022-17899-1
180. Babu KD, Sharma J, Maity A, Singh NV, Patil PG, Shilpa P, et al. Pomegranate: An ancient fruit for health and nutrition. Progr Hortic. (2021) 53:3–13. doi: 10.5958/2249-5258.2021.00001.4
181. Hadadi SA, Li H, Rafie R, Kaseloo P, Witiak SM, Siddiqui RA. Antioxidation properties of leaves, skin, pulp, and seeds extracts from green papaya and their anticancer activities in breast cancer cells. J Cancer Metastas Treat. (2018) 4:25. doi: 10.20517/2394-4722.2018.22
182. Jovanović M, Milutinović M, Kostić M, Miladinović B, Kitić N, Branković S, et al. Antioxidant capacity of pineapple (Ananas comosus (L.) Merr.) extracts and juice. Lek Sirov. (2018) 38:27–30. doi: 10.5937/leksir1838027J
183. Rashad MM, Mahmoud AE, Ali MM, Nooman MU, Al-Kashef AS. Antioxidant and anticancer agents produced from pineapple waste by solid-state fermentation. Int J Toxicol Pharmacol Res. (2015) 7:287–96.
184. Alansari WS, Eskandrani AA. The anticarcinogenic effect of the apple polyphenol phloretin in an experimental rat model of hepatocellular carcinoma. Arabian J Sci Eng. (2020) 45:4589–97. doi: 10.1007/s13369-020-04478-7
185. Adilah ZM, Jamilah B, Hanani ZN. Functional and antioxidant properties of protein-based films incorporated with mango kernel extract for active packaging. Food Hydrocoll. (2018) 74:207–18. doi: 10.1016/j.foodhyd.2017.08.017
186. Hamed M. Estimation of capsaicinoid compounds and other nutritionally important compounds in Colorado grown pepper cultivars. Ph.D. thesis. Fort Collins, CO: Colorado State University (2021).
187. Yousuf S, Shabir S, Mehdi MM, Srivastav S, Mohammed Saleh ZM, Bassfar Z, et al. Investigation of the Protective Effects of Urtica dioica, Capsella bursa-pastoris and Inula racemosa on Acetaminophen-Induced nephrotoxicity in Swiss albino male mice. Appl Sci. (2023) 13:3925. doi: 10.3390/app13063925
188. Shabir S, Sehgal A, Dutta J, Devgon I, Singh SK, Alsanie WF, et al. Therapeutic potential of green-engineered ZnO nanoparticles on rotenone-exposed D. melanogaster (Oregon R+): Unveiling ameliorated biochemical, cellular, and behavioral parameters. Antioxidants. (2023) 12:1679. doi: 10.3390/antiox12091679
Keywords: pesticides, nutraceuticals, reactive oxygen species, apoptosis, cytotoxicity
Citation: Sajad M, Shabir S, Singh SK, Bhardwaj R, Alsanie WF, Alamri AS, Alhomrani M, Alsharif A, Vamanu E and Singh MP (2024) Role of nutraceutical against exposure to pesticide residues: power of bioactive compounds. Front. Nutr. 11:1342881. doi: 10.3389/fnut.2024.1342881
Received: 23 November 2023; Accepted: 25 March 2024;
Published: 17 April 2024.
Edited by:
Marco Iammarino, Experimental Zooprophylactic Institute of Puglia and Basilicata (IZSPB), ItalyReviewed by:
Kangkon Saikia, Ministry of Science and Technology, IndiaFacundo Ibañez, National Institute for Agricultural Research (INIA), Uruguay
Copyright © 2024 Sajad, Shabir, Singh, Bhardwaj, Alsanie, Alamri, Alhomrani, Alsharif, Vamanu and Singh. This is an open-access article distributed under the terms of the Creative Commons Attribution License (CC BY). The use, distribution or reproduction in other forums is permitted, provided the original author(s) and the copyright owner(s) are credited and that the original publication in this journal is cited, in accordance with accepted academic practice. No use, distribution or reproduction is permitted which does not comply with these terms.
*Correspondence: Emanuel Vamanu, ZW1hbnVlbC52YW1hbnVAZ21haWwuY29t; Mahendra P. Singh, bXByYXRhcHMwMUBnbWFpbC5jb20=; bWFoZW5kcmEuem9vbEBkZHVndS5hYy5pbg==
†These authors have contributed equally to this work