- 1Department of Basic Medicine, Zhengzhou Shuqing Medical College, Zhengzhou, China
- 2Zhoukou Vocational and Technical College, Zhoukou, China
- 3Department of Biochemistry and Molecular Biology, Medical School, Henan University, Kaifeng, China
Modifications of protein post-translation are critical modulatory processes, which alters target protein biological activity,function and/or location, even involved in pathogenesis of some diseases. So far, there are at least 16 types of post-translation modifications identified, particularly through recent mass spectrometry analysis. Among them, succinylation (Ksuc) on protein lysine residues causes a variety of biological changes. Succinylation of proteins contributes to many cellular processes such as proliferation, growth, differentiation, metabolism and even tumorigenesis. Mechanically, Succinylation leads to conformation alteration of chromatin or remodeling. As a result, transcription/expression of target genes is changed accordingly. Recent research indicated that succinylation mainly contributes to metabolism modulations, from gene expression of metabolic enzymes to their activity modulation. In this review, we will conclude roles of succinylation in metabolic regulation of glucose, fat, amino acids and related metabolic disease launched by aberrant succinylation. Our goal is to stimulate extra attention to these still not well researched perhaps important succinylation modification on proteins and cell processes.
Introduction
Protein posttranslational modifications (PTMs) regulate the biological processes of human diseases by cellular pathophysiology regulation and genetic code expansion (1). In recent years, post-translational modifications of protein, such as propionylation, glutarylation, acetylation and succinylation, have become the focus of research (2, 3). Succinylation has emerged as a key regulator of metabolic pathways in various organisms (1, 3–5). This modification involves the transfer of a succinyl group (-CO-CH2-CH2-CO-) from succinyl-CoA to the ε-amino group of lysine residues in target proteins (6–8). The transfer of the succinyl group is catalyzed by succinyltransferases or non-enzyme catalyzed. For the enzymes, they are a family of enzymes conserved across different species (9–11). The non-enzymatic catalysis of succinylation may regulate the succinylation of cytoplasmic protein lysine through the activity of cytoplasmic SIRT5 (7).
Succinyl-CoA is a key intermediate in the tricarboxylic acid (TCA) cycle, a major metabolic pathway that generates ATP and other metabolic intermediates (7). Succinyl-CoA is also involved in other metabolic pathways, including the metabolism of branched-chain amino acids and the biosynthesis of heme (12). Concentration dependence of Succinyl-CoA and resulting proteins modified can be touched on. Both The levels of succinylated proteins and concentration of succinyl-CoA are higher than any other organs of Atrial fibrillation (AF) patients (13).Succinylation has been shown to regulate the activity, stability, and subcellular localization of metabolic enzymes involved in key metabolic pathways (9, 14, 15). For example, succinylation of the TCA cycle enzyme, succinate dehydrogenase, inhibits its activity and impairs mitochondrial respiration, leading to increased production of reactive oxygen species. In contrast, succinylation of the glycolytic enzyme, glyceraldehyde-3-phosphate dehydrogenase, enhances its activity, leading to increased flux through the glycolytic pathway (16–19).
Succinylation has also been implicated in the regulation of mitochondrial function and oxidative stress response (8, 20). Dysregulation of succinylation has been associated with various metabolic disorders (21, 22). For example, decreased succinylation of key mitochondrial enzymes has been observed in the skeletal muscle of individuals with type 2 diabetes (23).
Recent studies have identified a growing number of succinylated proteins in metabolic regulation (3, 24–26). Succinylation is a highly dynamic process responsive to changes in nutrient status and redox state (22, 27). For example, the level of succinylation of key metabolic enzymes has been shown to be regulated by changes in the availability of succinyl-CoA, a key precursor for succinylation (25).
Succinylation also plays a critical role in the regulation of mitochondrial function and oxidative stress response (28). Mitochondrial dysfunction has been implicated in a variety of diseases, including metabolic disorders and neurodegenerative diseases (1, 2, 15, 29). Succinylation has been shown to regulate mitochondrial function by modulating the activity of key enzymes involved in the TCA cycle and oxidative phosphorylation (13). Reactive oxygen species (ROS) are generated during normal cellular metabolism and can cause damage to cells (30). Elevated levels of succinylation in Cancer cell hepatocellular carcinoma are associated with unfavorable survival results (31–33). Peroxisomal fatty acid oxidation,propionate and/or ketone body metabolism that lead to succinyl-CoA production, or some other yet to be discovered pathway encompassing succinyl-CoA, potentially support succinylation of extramitochondrial proteins (6, 7, 34).
Regulation of succinylation in glucose metabolism
It has been well known that glucose metabolism is a tightly regulated process, and alterations in its regulation can contribute to the development of metabolic disorders such as obesity and diabetes (35). Protein succinylation has emerged as a key player in the regulation of glucose metabolism, by influencing the activity of various enzymes involved in the metabolism (36, 37).
One important enzyme affected by succinylation is pyruvate dehydrogenase complex (PDHC), which is responsible for converting pyruvate to acetyl-CoA. An increase of total protein succinylation promotes degradation of amino acids, which in turn decrease production of acetyl-CoA, leading to decreased glucose utilization and energy production (34, 38). In addition to PDHC, succinylation also affects other enzymes involved in glucose metabolism, including those in the tricarboxylic acid (TCA) cycle and the electron transport chain (39).These modifications can alter the enzymatic activity and the flow of metabolites within these pathways, ultimately impacting glucose utilization and energy production (40).
Recent studies have highlighted specific succinylated proteins involved in glucose metabolism, providing insights into their regulatory roles. For instance, succinylation of fructose-1,6-bisphosphate aldolase has been shown to regulate glycolysis, the initial step in glucose metabolism. Additionally, succinylation of ATP citrate lyase influences the production of acetyl-CoA, a key metabolite in glucose metabolism. It has been discovered that the translocation of Pyruvate kinase M2 (PKM2) to mitochondria under glucose starvation is mediated by succinylation (41).
Furthermore, succinylation has been linked to the regulation of insulin signaling. Succinylation of insulin signaling components can modulate their activity, affecting glucose uptake and utilization (42).
Succinylation in amino acid metabolism
Protein succinylation plays a crucial role in regulating amino acid metabolism (15). One of the primary ways is by modulating the activity of key enzymes involved in amino acid biosynthesis and catabolism (43, 44). Succinylation has been shown to impact the synthesis of thyroid hormones (45). By succinylating these enzymes, their catalytic activity can be either enhanced or inhibited, thereby tightly and finely controlling the rates of amino acid production and degradation (46).
Succinylation also affects cellular signaling pathways that regulate amino acid metabolism (47). It can affect the activation or inactivation of transcription factors responsible for the expression of genes involved in amino acid synthesis, transport, and utilization. This dynamic regulation ensures that the cell can adapt to changing environmental conditions (48–50).
Furthermore, succinylation is intricately linked to the tricarboxylic acid (TCA) cycle, a central hub in glucose and amino acid metabolism. Succinyl-CoA, an intermediate in the TCA cycle, is a key substrate for succinylation reactions (26, 51). This connection allows succinylation to influence not only energy production but also the availability of carbon skeletons for amino acid biosynthesis (22).
Remarkably, succinylation emerges as a pivotal player in the fine-tuned regulation of amino acid metabolism. It can modify enzymes, modulate gene expression, and interact with central metabolic pathways underscores its importance in maintaining cellular amino acid homeostasis. Understanding succinylation’s role in this context has implications for metabolic research and potential therapeutic significancy in amino acid-related diseases (29).
Regulation of succinylation in fatty acid metabolism
Succinylation primarily regulates fatty acid metabolism is through modulating the activity of key enzymes involved in lipid synthesis and breakdown (15). The mitochondrial trifunctional protein (TFP) is responsible for the chain-shortening of long-chain fatty acids inside the mitochondria. It is subject to significant succinyl lysine post-translational modifications (PTMs) (52).
Succinylation may enhance or inhibit the catalytic activity of these enzymes depending on different enzymes, leading to finely regulating the process of fatty acid synthesis and utilization within the cell, majorly to provide energy (53, 54). Additionally, succinylation can modify signaling molecules that regulate fatty acid metabolism (55). In response to shifting metabolic demands, it can modulate the activity of transcription factors that govern the expression of a specific set of genes related to lipid metabolism. Lysine acetyltransferase 2A KAT2A, known as GCN5-mediated succinylation of histone H3K79 contributes to the epigenetic regulation of cccDNA minichromosome in HBV, which is important for the regulation of gene expression in tumor cells (11, 48).
Moreover, the link between succinylation and central metabolic pathways, such as the tricarboxylic acid (TCA) cycle, further underscores its role in fatty acid metabolism. Succinyl-CoA, a key intermediate in the TCA cycle, is a substrate for succinylation reactions. This connection not only affects energy production but also influences the availability of carbon precursors for fatty acid synthesis (56).
In conclusion, additional research is essential to fully grasp the significance of succinylation in the realm of fatty acid metabolism. A deeper understanding of how succinylation influences this metabolic process holds the potential to advance our comprehension of metabolic disorders and open doors to potential therapeutic interventions.
Succinylation in ketone body synthesis
The regulation of succinylation is a crucial factor in controlling ketone body synthesis within the intricate landscape of cellular metabolism. Succinylation emerges as a pivotal player in modulating the production of ketone bodies, essential molecules that serve as an alternative energy source (23, 57), particularly during fasting, low carbohydrate intake, or strenuous exercise (58, 59).
At its core, succinylation regulates ketone body synthesis by influencing the activity of key enzymes involved in this metabolic pathway (60). By adding succinyl groups to specific lysine residues on these enzymes, succinylation can either enhance or inhibit their catalytic function. This precise regulatory mechanism ensures that ketone body production aligns with the body’s energy requirements (61).
Succinylation’s reach extends beyond enzymatic control, encompassing the transcription factors that orchestrate gene expression related to ketone body synthesis. Mutation in the OXCT1 gene caused Succinyl-CoA:3-oxoacid CoA-transferase (SCOT/Oxct1) deficiency in plasma and muscle, which is an inborn error of ketone body utilization characterized by intermittent ketoacidosis crises (23, 60). This modification can modulate the activity of these transcription factors, consequently impacting the expression of genes responsible for ketogenesis, the process of converting fatty acids into ketone bodies. Succinyl-CoA, an intermediate in the TCA cycle, serves as a substrate for succinylation reactions, directly affecting the availability of precursors essential for ketone body synthesis (58).
The regulation of succinylation serves as a vital mechanism in finely tuning ketone body synthesis. Through its influence on enzymes, transcription factors, and integration with central metabolic pathways, succinylation ensures the body’s capacity to produce ketone bodies precisely when needed to meet varying metabolic demands (62, 63). A deeper exploration of this regulatory process holds potential for advancing our understanding of ketone body metabolism and its implications for health and disease (64, 65).
Figure 1 illustrates the schematic representation of succinylation’s role in regulating metabolism. Succinylation, a post-translational modification, plays a pivotal role in modulating key enzymes involved in metabolic processes, thereby influencing energy production. One such enzyme affected by succinylation is fructose-1,6-bisphosphate aldolase (FBPA). Succinylation activates FBPA, enhancing its activity to catalyze the synthesis of dihydroxyacetone phosphate (DHAP) and glyceraldehyde-3-phosphate (G3P) into fructose-1,6-bisphosphate (FBP). Another significant enzyme, pyruvate kinase M2 (PKM2), crucial in glycolysis, is induced by starvation and undergoes succinylation. This modification facilitates PKM2 translocation into mitochondria. The pyruvate dehydrogenase complex (PDHC), responsible for converting pyruvate to acetyl-CoA, undergoes succinylation(one component), leading to its degradation and consequently reducing acetyl-CoA production. Succinyl-CoA, a product of the tricarboxylic acid (TCA) cycle, serves as a succinyl donor to modify various enzymes involved in amino acid and fatty acid metabolism.
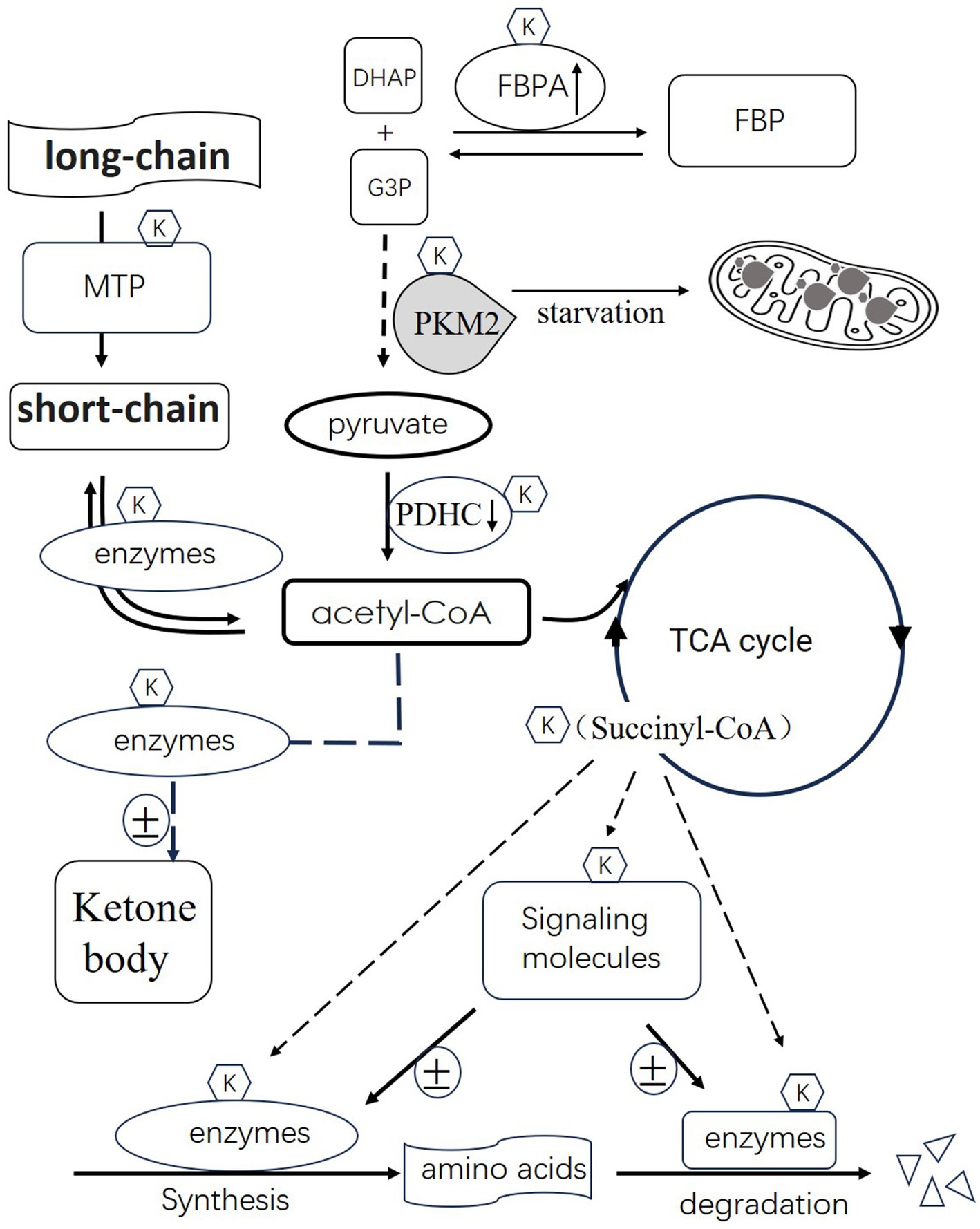
Figure 1. Illustrates the schematic representation of succinylation’s role in regulating metabolism. Succinylation activates 1,6-bisphosphate aldolase (FBPA), enhancing its activity to catalyze the synthesis of dihydroxyacetone phosphate (DHAP) and glyceraldehyde-3-phosphate (G3P) into fructose-1,6-bisphosphate (FBP). Pyruvate kinase M2 (PKM2), crucial in glycolysis, is induced by starvation and undergoes succinylation. This modification facilitates PKM2 translocation into mitochondria. The pyruvate dehydrogenase complex (PDHC), responsible for converting pyruvate to acetyl-CoA, undergoes succinylation, leading to its degradation and consequently reducing acetyl-CoA production. Succinyl-CoA, a product of the tricarboxylic acid (TCA) cycle, serves as a succinyl donor to modify various enzymes involved in amino acid and fatty acid metabolism. Additionally, certain succinylated signaling molecules can either activate or inhibit enzyme activity based on the specific enzymes involved. Furthermore, succinylation regulates the activity of enzymes involved in fatty acid metabolism and ketone body synthesis. In summary, succinylation emerges as a crucial mechanism orchestrating the activity of enzymes across various metabolic pathways, impacting energy production and metabolic homeostasis.
The succinylated component of mitochondrial trifunctional protein(MTP) affects processing of long-chain fatty acids to short-chain. The following processing of short-chain to acetyl-CoA is also affected by succinylated enzyme. Additionally, enzymes participating in both amino acid synthesis and degradation can be modified by succinylation. Certain succinylated signaling molecules can either activate or inhibit enzyme activity based on the specific enzymes involved. Furthermore, succinylation regulates the activity of enzymes involved in ketone body synthesis. The outcomes of these modifications on these enzymes are variable and contingent upon their specific metabolic functions. In summary, succinylation emerges as a crucial mechanism orchestrating the activity of enzymes across various metabolic pathways, impacting energy production and metabolic homeostasis.
Succinylation in redox regulation
Metabolism, particularly glucose metabolism plays a critical role in regulation redox balance. In recent years, research has unveiled how succinylation can intricately modulate redox regulation (27). Firstly, it directly influences the activity of enzymes involved in redox reactions. By succinylating these enzymes, succinylation can either enhance or inhibit their function, thereby affecting the delicate equilibrium between oxidants and antioxidants within the cell. Secondly, succinylation can modulate activity of transcription factors that govern the expression of genes related to redox regulation (66). This modulation can lead to adaptive responses to oxidative stress and alterations in the production of antioxidant molecules (67).
Furthermore, succinylation is intimately connected to central metabolic pathways, particularly the tricarboxylic acid (TCA) cycle, where succinyl-CoA is an essential intermediate. This connection can impact the generation of reducing equivalents like NADH, crucial for maintaining the cellular redox state. In conclusion, succinylation stands as a dynamic and emerging regulator in redox signaling, orchestrating enzyme activity and gene expression to maintain cellular redox balance and adapt to oxidative environments (7) (Table 1).
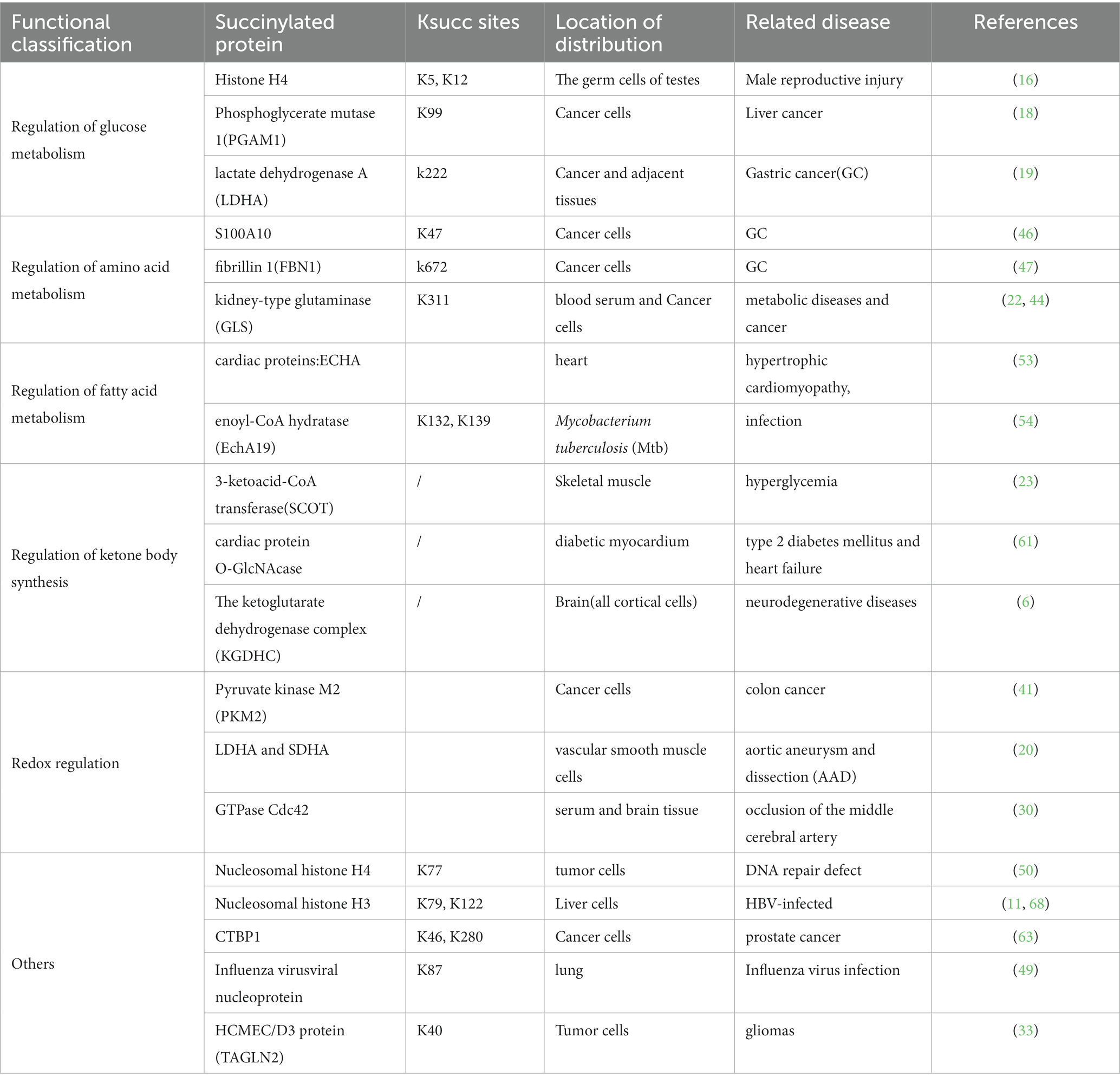
Table 1. Ksucc sites, Location of distribution, Related disease of identified succinylated proteins.
Perspective
The regulation of succinylation in metabolism represents a captivating frontier in our understanding of cellular homeostasis. This post-translational modification has emerged as a key player in fine-tuning metabolic processes.
Succinylation exerts its influence across diverse metabolic pathways, impacting enzymatic activities, gene expression, and central metabolic hubs like the tricarboxylic acid (TCA) cycle. This interconnectedness underscores its significance in modulating energy production, amino acid metabolism, fatty acid synthesis, and redox regulation.
Moreover, succinylation’s responsiveness to environmental cues, such as changes in nutrient availability or oxidative stress, highlights its role as a metabolic sensor. It enables cells to adapt swiftly to varying conditions, optimizing energy utilization and metabolic flux.
Understanding the intricate network of succinylation in metabolism holds immense promise. It may unveil novel therapeutic targets for metabolic disorders like diabetes, obesity, and cancer. Moreover, it offers insights into broader aspects of cellular health, aging, and disease. As researchers delve deeper into this fascinating field, more attention should be given to the reversible succinylation of lysines within mitochondria, as well as its potential application in anticancer drugs will be provided. we anticipate remarkable revelations that will reshape our comprehension of metabolism and its impact on human health.
Author contributions
XH: Data curation, Formal analysis, Resources, Writing – original draft. YC: Conceptualization, Data curation, Writing – original draft. XL: Resources, Writing – original draft, Methodology. XG: Writing – original draft, Methodology, Resources. WD: Writing – original draft, Formal analysis. JS: Formal analysis, Validation, Writing – original draft. SJ: Conceptualization, Funding acquisition, Supervision, Writing – review & editing.
Funding
The author(s) declare financial support was received for the research, authorship, and/or publication of this article. The study was supported by National Natural Science Foundation of China.
Conflict of interest
The authors declare that the research was conducted in the absence of any commercial or financial relationships that could be construed as a potential conflict of interest.
Publisher’s note
All claims expressed in this article are solely those of the authors and do not necessarily represent those of their affiliated organizations, or those of the publisher, the editors and the reviewers. Any product that may be evaluated in this article, or claim that may be made by its manufacturer, is not guaranteed or endorsed by the publisher.
References
1. Deng, YH, Zhang, XX, Tao, CY, Liang, YJ, Yuan, J, Yang, SH, et al. Succinylation profiles of brain injury after intracerebral hemorrhage. PLoS One. (2021) 16:e0259798. doi: 10.1371/journal.pone.0259798
2. Wang, H, Lu, J, Gao, WC, Ma, X, Li, N, Ding, Z, et al. Donepezil down-regulates propionylation, 2-hydroxyisobutyrylation, butyrylation, succinylation, and crotonylation in the brain of bilateral common carotid artery occlusion-induced vascular dementia rats. Clin Exp Pharmacol Physiol. (2020) 47:1731–9. doi: 10.1111/1440-1681.13352
3. Zhou, B, Du, Y, Xue, Y, Miao, G, Wei, T, and Zhang, P. Identification of Malonylation, Succinylation, and Glutarylation in serum proteins of acute myocardial infarction patients. Proteomics Clin Appl. (2020) 14:e1900103. doi: 10.1002/prca.201900103
4. Ye, J, and Li, J. First analyses of lysine succinylation proteome and overlap between succinylation and acetylation in Solenopsis invicta Buren (Hymenoptera: Formicidae). BMC Genomics. (2022) 23:61. doi: 10.1186/s12864-021-08285-8
5. Huang, Z, He, L, Sang, W, Wang, L, Huang, Q, and Lei, C. Potential role of lysine succinylation in the response of moths to artificial light at night stress. Ecotoxicol Environ Saf. (2021) 220:112334. doi: 10.1016/j.ecoenv.2021.112334
6. Dobolyi, A, Bago, A, Palkovits, M, Nemeria, NS, Jordan, F, Doczi, J, et al. Exclusive neuronal detection of KGDHC-specific subunits in the adult human brain cortex despite pancellular protein lysine succinylation. Brain Struct Funct. (2020) 225:639–67. doi: 10.1007/s00429-020-02026-5
7. Chinopoulos, C. The mystery of Extramitochondrial proteins lysine Succinylation. Int J Mol Sci. (2021) 22:85. doi: 10.3390/ijms22116085
8. Dai, X, Zhou, Y, Han, F, and Li, J. Succinylation and redox status in cancer cells. Front Oncol. (2022) 12:1081712. doi: 10.3389/fonc.2022.1081712
9. Chen, Y. Quantitative analysis of the Sirt5-regulated lysine Succinylation proteome in mammalian cells. Methods Mol Biol. (2016) 1410:23–37. doi: 10.1007/978-1-4939-3524-6_2
10. Yokoyama, A, Katsura, S, and Sugawara, A. Biochemical analysis of histone Succinylation. Biochem Res Int. (2017) 2017:8529404. doi: 10.1155/2017/8529404
11. Wang, Y, Guo, YR, Xing, D, Tao, YJ, and Lu, Z. Supramolecular assembly of KAT2A with succinyl-CoA for histone succinylation. Cell Discov. (2018) 4:47. doi: 10.1038/s41421-018-0048-8
12. Zhu, X, Meyers, A, Long, D, Ingram, B, Liu, T, Yoza, BK, et al. Frontline science: monocytes sequentially rewire metabolism and bioenergetics during an acute inflammatory response. J Leukoc Biol. (2019) 105:215–28. doi: 10.1002/JLB.3HI0918-373R
13. Bai, F, Ma, Y, and Liu, Q. Succinylation as a novel mode of energy metabolism regulation during atrial fibrillation. Med Hypotheses. (2018) 121:54–5. doi: 10.1016/j.mehy.2018.09.018
14. Lu, K, and Han, D. A review of the mechanism of succinylation in cancer. Medicine (Baltimore). (2022) 101:e31493. doi: 10.1097/MD.0000000000031493
15. Ning, Q, Ma, Z, Zhao, X, and Yin, M. SSKM_Succ: a novel Succinylation sites prediction method incorporating K-means clustering with a new semi-supervised learning algorithm. IEEE/ACM Trans Comput Biol Bioinform. (2022) 19:643–52. doi: 10.1109/TCBB.2020.3006144
16. Yang, Q, Li, P, Wen, Y, Li, S, Chen, J, Liu, X, et al. Cadmium inhibits lysine acetylation and succinylation inducing testicular injury of mouse during development. Toxicol Lett. (2018) 291:112–20. doi: 10.1016/j.toxlet.2018.04.005
17. Yang, Q, Liu, X, Chen, J, Wen, Y, Liu, H, Peng, Z, et al. Lead-mediated inhibition of lysine acetylation and succinylation causes reproductive injury of the mouse testis during development. Toxicol Lett. (2020) 318:30–43. doi: 10.1016/j.toxlet.2019.10.012
18. Wang, YF, Zhao, LN, Geng, Y, Yuan, HF, Hou, CY, Zhang, HH, et al. Aspirin modulates succinylation of PGAM1K99 to restrict the glycolysis through NF-kappaB/HAT1/PGAM1 signaling in liver cancer. Acta Pharmacol Sin. (2023) 44:211–20. doi: 10.1038/s41401-022-00945-z
19. Li, X, Zhang, C, Zhao, T, Su, Z, Li, M, Hu, J, et al. Lysine-222 succinylation reduces lysosomal degradation of lactate dehydrogenase a and is increased in gastric cancer. J Exp Clin Cancer Res. (2020) 39:172. doi: 10.1186/s13046-020-01681-0
20. Zhang, H, Zhang, Y, Wang, H, Yang, P, Lu, C, Liu, Y, et al. Global proteomic analysis reveals lysine succinylation contributes to the pathogenesis of aortic aneurysm and dissection. J Proteome. (2023) 280:104889. doi: 10.1016/j.jprot.2023.104889
21. Thapa, N, Chaudhari, M, McManus, S, Roy, K, Newman, RH, Saigo, H, et al. DeepSuccinylSite: a deep learning based approach for protein succinylation site prediction. BMC Bioinformatics. (2020) 21:63. doi: 10.1186/s12859-020-3342-z
22. Lukey, MJ, Greene, KS, and Cerione, RA. Lysine succinylation and SIRT5 couple nutritional status to glutamine catabolism. Mol Cell Oncol. (2020) 7:1735284. doi: 10.1080/23723556.2020.1735284
23. Mechchate, H, Abdualkader, AM, Bernacchi, JB, Gopal, K, Tabatabaei Dakhili, SA, Yang, K, et al. Defective muscle ketone body oxidation disrupts BCAA catabolism by altering mitochondrial branched-chain aminotransferase. Am J Physiol Endocrinol Metab. (2023) 324:E425–e436. doi: 10.1152/ajpendo.00206.2022
24. Xu, X, Liu, T, Yang, J, Chen, L, Liu, B, Wei, C, et al. The first succinylome profile of Trichophyton rubrum reveals lysine succinylation on proteins involved in various key cellular processes. BMC Genomics. (2017) 18:577. doi: 10.1186/s12864-017-3977-y
25. Ali, HR, Michel, CR, Lin, YH, McKinsey, TA, Jeong, MY, Ambardekar, AV, et al. Defining decreased protein succinylation of failing human cardiac myofibrils in ischemic cardiomyopathy. J Mol Cell Cardiol. (2020) 138:304–17. doi: 10.1016/j.yjmcc.2019.11.159
26. Gut, P, Matilainen, S, Meyer, JG, Pallijeff, P, Richard, J, Carroll, CJ, et al. SUCLA2 mutations cause global protein succinylation contributing to the pathomechanism of a hereditary mitochondrial disease. Nat Commun. (2020) 11:5927. doi: 10.1038/s41467-020-19743-4
27. Chen, H, Xu, H, Potash, S, Starkov, A, Belousov, VV, Bilan, DS, et al. Mild metabolic perturbations alter succinylation of mitochondrial proteins. J Neurosci Res. (2017) 95:2244–52. doi: 10.1002/jnr.24103
28. Yang, YH, Wu, SF, Zhu, YP, Yang, JT, and Liu, JF. Global profiling of lysine succinylation in human lungs. Proteomics. (2022) 22:e2100381. doi: 10.1002/pmic.202100381
29. Yang, Y, Tapias, V, Acosta, D, Xu, H, Chen, H, Bhawal, R, et al. Altered succinylation of mitochondrial proteins, APP and tau in Alzheimer's disease. Nat Commun. (2022) 13:159. doi: 10.1038/s41467-021-27572-2
30. Huang, LY, Ma, JY, Song, JX, Xu, JJ, Hong, R, Fan, HD, et al. Ischemic accumulation of succinate induces Cdc42 succinylation and inhibits neural stem cell proliferation after cerebral ischemia/reperfusion. Neural Regen Res. (2023) 18:1040–5. doi: 10.4103/1673-5374.355821
31. Bai, W, Cheng, L, Xiong, L, Wang, M, Liu, H, Yu, K, et al. Protein succinylation associated with the progress of hepatocellular carcinoma. J Cell Mol Med. (2022) 26:5702–12. doi: 10.1111/jcmm.17507
32. Zhang, Z, Chen, Y, Fang, L, Zhao, J, and Deng, S. The involvement of high succinylation modification in the development of prostate cancer. Front Oncol. (2022) 12:1034605. doi: 10.3389/fonc.2022.1034605
33. Zhang, X, Han, J, Fan, D, Wang, J, Lin, X, Zhang, H, et al. Lysine-40 succinylation of TAGLN2 induces glioma angiogenesis and tumor growth through regulating TMSB4X. Cancer Gene Ther. (2023) 30:172–81. doi: 10.1038/s41417-022-00534-6
34. Wang, M, Chang, Q, Yang, H, Liu, Y, Wang, C, Hu, F, et al. Elevated lysine crotonylation and succinylation in the brains of BTBR mice. Int J Dev Neurosci. (2019) 76:61–4. doi: 10.1016/j.ijdevneu.2019.06.011
35. Hattori, Y. Insulin resistance and heart failure during treatment with sodium glucose cotransporter 2 inhibitors: proposed role of ketone utilization. Heart Fail Rev. (2020) 25:403–8. doi: 10.1007/s10741-020-09921-3
36. Ke, X, Jiang, X, Huang, M, Tian, X, and Chu, J. Engineering of succinyl-CoA metabolism in view of succinylation regulation to improve the erythromycin production. Appl Microbiol Biotechnol. (2022) 106:5153–65. doi: 10.1007/s00253-022-12060-4
37. Zeng, J, Wu, L, Chen, Q, Wang, L, Qiu, W, Zheng, X, et al. Comprehensive profiling of protein lysine acetylation and its overlap with lysine succinylation in the Porphyromonas gingivalis fimbriated strain ATCC 33277. Mol Oral Microbiol. (2020) 35:240–50. doi: 10.1111/omi.12312
38. Artiukhov, AV, Aleshin, VA, Karlina, IS, Kazantsev, AV, Sibiryakina, DA, Ksenofontov, AL, et al. Phosphonate inhibitors of pyruvate dehydrogenase perturb homeostasis of amino acids and protein Succinylation in the brain. Int J Mol Sci. (2022) 23:186. doi: 10.3390/ijms232113186
39. Wu, J, Li, N, Huang, X, Chen, J, Jia, Y, He, Z, et al. Proteomic quantification of lysine acetylation and Succinylation profile alterations in lung adenocarcinomas of non-smoking females. Yonago Acta Med. (2022) 65:132–47. doi: 10.33160/yam.2022.05.006
40. Green, SR, and Storey, KB. Skeletal muscle of torpid Richardson's ground squirrels (Urocitellus richardsonii) exhibits a less active form of citrate synthase associated with lowered lysine succinylation. Cryobiology. (2021) 101:28–37. doi: 10.1016/j.cryobiol.2021.06.006
41. Qi, H, Ning, X, Yu, C, Ji, X, Jin, Y, McNutt, MA, et al. Succinylation-dependent mitochondrial translocation of PKM2 promotes cell survival in response to nutritional stress. Cell Death Dis. (2019) 10:170. doi: 10.1038/s41419-018-1271-9
42. Jesinkey, SR, Madiraju, AK, Alves, TC, Yarborough, OH, Cardone, RL, Zhao, X, et al. Mitochondrial GTP links nutrient sensing to β cell health, mitochondrial morphology, and insulin secretion independent of OxPhos. Cell Rep. (2019) 28:759–772.e10. doi: 10.1016/j.celrep.2019.06.058
43. Siculella, L, Giannotti, L, Di Chiara Stanca, B, Calcagnile, M, Rochira, A, Stanca, E, et al. Evidence for a negative correlation between human reactive enamine-imine intermediate deaminase a (RIDA) activity and cell proliferation rate: role of lysine Succinylation of RIDA. Int J Mol Sci. (2021) 22:804. doi: 10.3390/ijms22083804
44. Tong, Y, Guo, D, Lin, SH, Liang, J, Yang, D, Ma, C, et al. SUCLA2-coupled regulation of GLS succinylation and activity counteracts oxidative stress in tumor cells. Mol Cell. (2021) 81:2303–2316.e8. doi: 10.1016/j.molcel.2021.04.002
45. Mu, R, Ma, Z, Lu, C, Wang, H, Cheng, X, Tuo, B, et al. Role of succinylation modification in thyroid cancer and breast cancer. Am J Cancer Res. (2021) 11:4683–99.
46. Wang, C, Zhang, C, Li, X, Shen, J, Xu, Y, Shi, H, et al. CPT1A-mediated succinylation of S100A10 increases human gastric cancer invasion. J Cell Mol Med. (2019) 23:293–305. doi: 10.1111/jcmm.13920
47. Wang, X, Shi, X, Lu, H, Zhang, C, Li, X, Zhang, T, et al. Succinylation inhibits the enzymatic hydrolysis of the extracellular matrix protein Fibrillin 1 and promotes gastric Cancer progression. Adv Sci (Weinh). (2022) 9:e2200546. doi: 10.1002/advs.202200546
48. Yuan, Y, Yuan, H, Yang, G, Yun, H, Zhao, M, Liu, Z, et al. IFN-alpha confers epigenetic regulation of HBV cccDNA minichromosome by modulating GCN5-mediated succinylation of histone H3K79 to clear HBV cccDNA. Clin Epigenetics. (2020) 12:135. doi: 10.1186/s13148-020-00928-z
49. Guillon, A, Brea-Diakite, D, Cezard, A, Wacquiez, A, Baranek, T, Bourgeais, J, et al. Host succinate inhibits influenza virus infection through succinylation and nuclear retention of the viral nucleoprotein. EMBO J. (2022) 41:e108306. doi: 10.15252/embj.2021108306
50. Jing, Y, Ding, D, Tian, G, Kwan, KCJ, Liu, Z, Ishibashi, T, et al. Semisynthesis of site-specifically succinylated histone reveals that succinylation regulates nucleosome unwrapping rate and DNA accessibility. Nucleic Acids Res. (2020) 48:9538–49. doi: 10.1093/nar/gkaa663
51. Liu, Q, Wang, H, Zhang, H, Sui, L, Li, L, Xu, W, et al. The global succinylation of SARS-CoV-2-infected host cells reveals drug targets. Proc Natl Acad Sci USA. (2022) 119:e2123065119. doi: 10.1073/pnas.2123065119
52. Zhang, Y, and Goetzman, E. The enzyme activity of mitochondrial trifunctional protein is not altered by lysine acetylation or lysine succinylation. PLoS One. (2021) 16:e0256619. doi: 10.1371/journal.pone.0256619
53. Sadhukhan, S, Liu, X, Ryu, D, Nelson, OD, Stupinski, JA, Li, Z, et al. Metabolomics-assisted proteomics identifies succinylation and SIRT5 as important regulators of cardiac function. Proc Natl Acad Sci USA. (2016) 113:4320–5. doi: 10.1073/pnas.1519858113
54. Bonds, AC, Yuan, T, Werman, JM, Jang, J, Lu, R, Nesbitt, NM, et al. Post-translational Succinylation of Mycobacterium tuberculosis Enoyl-CoA hydratase EchA19 slows catalytic hydration of cholesterol catabolite 3-Oxo-chol-4,22-diene-24-oyl-CoA. ACS Infect Dis. (2020) 6:2214–24. doi: 10.1021/acsinfecdis.0c00329
55. Karunanidhi, A, Basu, S, Zhao, XJ, D'Annibale, O, Van't Land, C, Vockley, J, et al. Heptanoic and medium branched-chain fatty acids as anaplerotic treatment for medium chain acyl-CoA dehydrogenase deficiency. Mol Genet Metab. (2023) 140:107689. doi: 10.1016/j.ymgme.2023.107689
56. Collado, MS, Armstrong, AJ, Olson, M, Hoang, SA, Day, N, Summar, M, et al. Biochemical and anaplerotic applications of in vitro models of propionic acidemia and methylmalonic acidemia using patient-derived primary hepatocytes. Mol Genet Metab. (2020) 130:183–96. doi: 10.1016/j.ymgme.2020.05.003
57. Hattori, Y. Beneficial effects on kidney during treatment with sodium-glucose cotransporter 2 inhibitors: proposed role of ketone utilization. Heart Fail Rev. (2021) 26:947–52. doi: 10.1007/s10741-020-10065-7
58. Tabatabaei Dakhili, SA, Yang, K, Locatelli, CAA, Saed, CT, Greenwell, AA, Chan, JSF, et al. Ketone ester administration improves glycemia in obese mice. Am J Physiol Cell Physiol. (2023) 325:C750–c757. doi: 10.1152/ajpcell.00300.2023
59. Weis, EM, Puchalska, P, Nelson, AB, Taylor, J, Moll, I, Hasan, SS, et al. Ketone body oxidation increases cardiac endothelial cell proliferation. EMBO Mol Med. (2022) 14:e14753. doi: 10.15252/emmm.202114753
60. Amirkashani, D, Asadollahi, M, Hosseini, R, Talebi, S, Golchehre, Z, and Keramatipour, M. A novel mutation in the OXCT1 gene causing Succinyl-CoA:3-Ketoacid CoA transferase (SCOT) deficiency starting with neurologic manifestations. Iran J Child Neurol. (2023) 17:127–33. doi: 10.22037/ijcn.v17i2.35963
61. Brahma, MK, Ha, CM, Pepin, ME, Mia, S, Sun, Z, Chatham, JC, et al. Increased glucose availability attenuates myocardial ketone body utilization. J Am Heart Assoc. (2020) 9:e013039. doi: 10.1161/JAHA.119.013039
62. Shi, R, Wang, Y, Gao, Y, Xu, X, Mao, S, Xiao, Y, et al. Succinylation at a key residue of FEN1 is involved in the DNA damage response to maintain genome stability. Am J Physiol Cell Physiol. (2020) 319:C657–66. doi: 10.1152/ajpcell.00137.2020
63. Zhou, J, Yan, X, Liu, Y, and Yang, J. Succinylation of CTBP1 mediated by KAT2A suppresses its inhibitory activity on the transcription of CDH1 to promote the progression of prostate cancer. Biochem Biophys Res Commun. (2023) 650:9–16. doi: 10.1016/j.bbrc.2023.02.002
64. Jurado-Aguirre, MA, and Pérez-Verdín, AE. Succinyl CoA:3 oxoacid CoA transferase deficiency: a case report. Rev Med Inst Mex Seguro Soc. (2023) 61:691–4. doi: 10.5281/zenodo.8316483
65. Grünert, SC, Foster, W, Schumann, A, Lund, A, Pontes, C, Roloff, S, et al. Succinyl-CoA:3-oxoacid coenzyme a transferase (SCOT) deficiency: a rare and potentially fatal metabolic disease. Biochimie. (2021) 183:55–62. doi: 10.1016/j.biochi.2021.02.003
66. Smestad, J, Erber, L, Chen, Y, and Maher, LJ 3rd. Chromatin Succinylation correlates with active gene expression and is perturbed by defective TCA cycle metabolism. iScience. (2018) 2:63–75. doi: 10.1016/j.isci.2018.03.012
67. Hershberger, KA, Abraham, DM, Liu, J, Locasale, JW, Grimsrud, PA, and Hirschey, MD. Ablation of Sirtuin5 in the postnatal mouse heart results in protein succinylation and normal survival in response to chronic pressure overload. J Biol Chem. (2018) 293:10630–45. doi: 10.1074/jbc.RA118.002187
Keywords: post-translational modification, succinylation, metabolism, regulation, redox
Citation: Hou X, Chen Y, Li X, Gu X, Dong W, Shi J and Ji S (2024) Protein succinylation: regulating metabolism and beyond. Front. Nutr. 11:1336057. doi: 10.3389/fnut.2024.1336057
Edited by:
Zhi-Bo Xie, Shanghai Children’s Hospital, ChinaReviewed by:
Andrew Carley, The Ohio State University, United StatesJohn Muroski, University of California, Los Angeles, United States
Copyright © 2024 Hou, Chen, Li, Gu, Dong, Shi and Ji. This is an open-access article distributed under the terms of the Creative Commons Attribution License (CC BY). The use, distribution or reproduction in other forums is permitted, provided the original author(s) and the copyright owner(s) are credited and that the original publication in this journal is cited, in accordance with accepted academic practice. No use, distribution or reproduction is permitted which does not comply with these terms.
*Correspondence: Shaoping Ji, c2hhb3BpbmdqaUBoZW51LmVkdS5jbg==