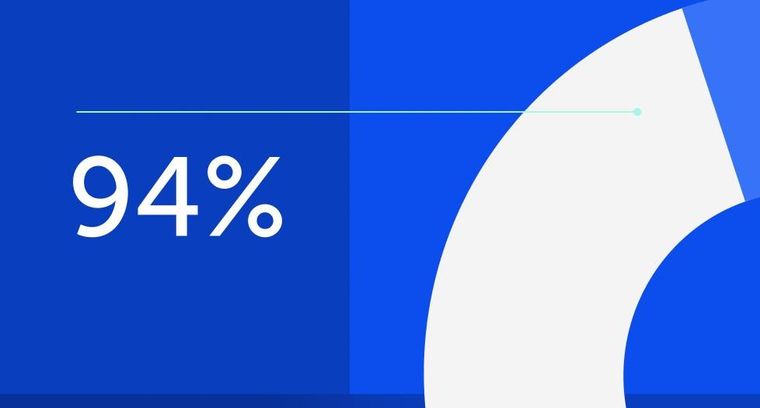
94% of researchers rate our articles as excellent or good
Learn more about the work of our research integrity team to safeguard the quality of each article we publish.
Find out more
REVIEW article
Front. Nutr., 17 January 2024
Sec. Nutrition and Food Science Technology
Volume 10 - 2023 | https://doi.org/10.3389/fnut.2023.1341583
Traditional Chinese medicine (TCM) has displayed preventive and therapeutic effects on many complex diseases. As natural biological macromolecules, TCM-derived antiobesogenic polysaccharides (TCMPOs) exhibit notable weight-loss effects and are seen to be a viable tactic in the fight against obesity. Current studies demonstrate that the antiobesity activity of TCMPOs is closely related to their structural characteristics, which could be affected by the extraction and purification methods. Therefore, the extraction, purification and structural-property correlations of TCMPOs were discussed. Investigation of the antiobesity mechanism of TCMPOs is also essential for their improved application. Herein, the possible antiobesity mechanisms of TCMPOs are systematically summarized: (1) modulation of appetite and satiety effects, (2) suppression of fat absorption and synthesis, (3) alteration of the gut microbiota and their metabolites, and (4) protection of intestinal barriers. This collated information could provide some insights and offer a new therapeutic approach for the management and prevention of obesity.
Obesity, one of the biggest challenges to public health worldwide to date, is also thought to be an important risk indicator for numerous other metabolic illnesses, such as hyperlipidemia, hypertension, myocardial infarction, stroke, type 2 diabetes as well as non-alcoholic fatty liver disorder, thereby contributing to a decline in quality of life and longevity (1–3). There has been a significant increase within the incidence of obesity globally, which is a major health risk to people and an important medical issue that needs to be resolved within various countries (2, 4, 5). Clinical strategies have been proposed to combat obesity mainly through drug administration and bariatric surgery (6, 7). Antiobesity drugs previously approved by the FDA, such as sibutramine, deoxyephedrine, carbamate and fenfluramine, have shown serious side effects, and some are no longer available or are unsuitable for long-term use (8–10). Bariatric surgeries, like Roux-en-Y gastric bypass (RYGB), gastric banding as well as sleeve gastrectomy, are confirmed to be more effective than drug therapy, but they have not been widely accepted due to their high cost, high risk, possible complications and sequelae (7, 11). Accordingly, great interest has been focused on exploring effective, healthy and low-risk alternative therapies to treat or alleviate obesity along with related metabolic disorders.
Traditional Chinese medicine (TCM) has been widely and safely applied within China for thousands of years due to its high efficiency and low side-effect, which is now becoming increasingly popular worldwide and is of vital significance to prevent disease or promote health (12, 13). As natural biological macromolecules, TCM-derived polysaccharides (TCMPs) have become highly attractive due to their remarkable biological functions and pharmacological properties, such as antioxidant, antidiabetic, antitumor, anti-inflammatory, anti-obesity, and immunomodulatory activities (5, 12, 14). In particular, the antiobesity activity of TCMPs has attracted the most attention. Multiple TCM-derived antiobesogenic polysaccharides (TCMPOs), such as Zingiber striolatum polysaccharides (13), Rosa laevigata polysaccharides (5), Lycium barbarum polysaccharides (14), Cymodocea nodosa polysaccharides (15), Polygonatum cyrtonema polysaccharides (16), Laminaria japonica polysaccharides (17), and Ganoderma lucidum polysaccharides (18), have been reported to have antiobesity effects. Considering different therapeutic leads, TCMPOs have two primary benefits over synthetic medications and bariatric procedures. Initially, the historical application of TCMs provides reliable empirical evidence for their safety. The antiobesity activity of naturally derived TCMPOs is more inclined to long-term effectiveness and safety and could be more acceptable by the public due to its distinct benefits, like affordable, few side effects as well as low risk (12, 16). Second, the antiobesity mechanism of TCMPOs is different from that of synthetic drugs; polysaccharides are hardly digestible and unabsorbable and can act on intestinal microbiota or intestinal hormones after oral administration, thereby producing potential antiobesity effects in the host (19, 20).
Numerous investigations have shown a close relationship between the structural properties for TCMPOs and their antiobesity efficacy, mainly involving the influence of molecular weights (Mw), backbone, conformational features and functional groups (12, 13, 21). Furthermore, the extraction and purification conditions of TCMPs, such as temperature, solvent and separation medium, could also have impacts on their compositions and structures, thus affecting their bioactivities (21–23). In addition, the antiobesity mechanism of TCMPOs has also become a research hotspot in recent years. Research shows that TCMPOs could exhibit antiobesity effects through multiple targets, such as regulating appetite, controlling the absorption and synthesis of fat, and modifying the intestinal flora (6, 24–26). Nevertheless, currently published reviews mostly focus on the separation, purification and biological activity of polysaccharides, there is no available review concerning the structural-property correlations and antiobesity mechanism of TCMPOs, despite having been sufficiently studied by many scholars. Therefore, our goal in this study is to methodically compile the results of contemporary research and to present a comprehensive understanding of the extraction, purification techniques, structural-property correlations and antiobesity mechanism of TCMPOs, thus drawing investigators’ attention to the application potential and utilization of TCMPOs.
The most crucial step in the separation of TCMPOs is extraction. Table 1 depicts the methods and techniques used to extract, isolate and purify the polysaccharides from TCM. Table 1 illustrates that the traditional and most popular laboratory technique for producing TCMPOs is extract within hot or boiling water. In order to get useful TCMPOs yields, this approach often requires a high extraction temperature (60∼100°C), long duration (1∼24 h), high energy, a high water-to-material proportion, as well as several extraction stages. However, due to its relative conventionality, ease of control, and affordability, this approach is still widely utilized today. Additionally, to increase the extraction efficiency, a variety of extractant and supported techniques have been employed, including treatment with dilute acid (23, 27–30) or alkali (31, 32), high pressure (18, 33–35), high-power ultrasound (36, 37). Dilute acid or dilute alkali are capable of improving the amount extracted of polysaccharides, however several studies have shown that this method tends to damage the structure for TCMPOs which thus may further affect their biological activity (12, 38). According to earlier studies, the extraction yields of Dictyophora indusiata polysaccharides prepared using various extraction methods ranged from 5.62 to 6.48% (22). Compared with simple water extraction, microwave, ultrasonic and pressurized extraction take a shorter time and have higher extraction efficiencies (Table 1). However, in view of the above facts, the heat and extractant-assisted extraction method of TCMPOs is excessively conventional and has many limitations. Hence, the development of novel technology is imperative. For example, microwave extraction, which can generate high-frequency concussion and evenly penetrate the material to destroy the cell wall (39) and promote the extraction of intracellular polysaccharides (38) in a short time, is energy-efficient and could achieve a better yield. In addition, supercritical CO2 extraction is a productive extraction method that identifies unique characteristics of the extract at crucial stages (40). During this process, the use of appropriate entrainers or modifying agents such as ethanol and methanol (41, 42) improves the probability of obtaining TCMPOs.
Table 1. The source, geographical origins, extraction, isolation and purification of various TCMPOs.
After the TCM water extracts are obtained, the isolation and purification process need to be first carried out by removing the non-polysaccharide components. The polysaccharides obtained through ethanol precipitation has been partly cleansed through deproteination as well as decoloration (38, 43, 44) before further being isolated and purified via dialysis (16), ultrafiltration (29, 45), membrane separation (18, 21), ion-exchange chromatography (46), gel filtration chromatograph (45, 47, 48), along with other methods. Figure 1 provides a schematic representation of the TCMPOs extraction and purification procedure. To obtain the pure fractions of TCMPOs, elution was carried out using the proper running buffers, then collection, concentration, dialysis, as well as lyophilization. For example, the water-extracted crude polysaccharides from Sargassum fusiforme were separated through a DEAE Sepharose Fast Flow column, as well as three polysaccharide fractions, namely, Sf-1, Sf-2, and Sf-3, were obtained from elution with water, 0.5 M NaCl, 2 M NaCl, respectively (23, 27). Wu et al. (21) fractionated the Hirsutella sinensis water extract into four fractions (H1, H2, H3 and H4) on the basis of their Mw through membrane separation. Zha et al. (49) fractionated the L. japonica water extract into the three fractions LP1, LP2, and LP3 using the ethanol precipitation method at ultimate concentrations of 40, 60, and 80%, correspondingly. Different separation and purification methods will lead to different TCMPOs components. However, some research suggests that most TCMPOs obtained by the present extraction, isolation and purification techniques are still crude products, and the quality of TCMPOs is difficult to control (12, 50, 51). First, the region of origin, the portions of the plant that are collected, the time of year it is harvested, and the technique of processing all affect how medicinally effective the TCM is (52). Second, non-template synthesized polysaccharides are never pure compounds regardless of how many procedures have been applied for purification (12). The acquired TCMPOs are always bound within either narrow or broad Mw ranges (12, 50). It is also worth noting that the extraction methods, solvent, equipment, personal operation method and so on differed from lab to lab. Therefore, special attention must be paid to the whole extraction, isolation and purification procedures to obtain high-quality TCMPOs.
Figure 1. Schematic diagram of the extraction, purification, structural features and antiobesity mechanism of TCMPOs. TCM, traditional Chinese medicine; TCMPOs, traditional Chinese medicine-derived polysaccharides.
The physiochemical and structural properties of TCMPOs, as shown in Figure 1, mainly including monosaccharide compositions, Mw, and backbone structures (configuration, type, position of glycosidic linkage, sequence of monosaccharide), as well as conformational features, have been investigated and identified by various chemical and instrumental analyses, such as acid hydrolysis, periodate oxidation analysis, methylation analysis, smith degradation, gas chromatography (GC) (49), gas chromatography–mass spectrometry (GC–MS) (53), ultraviolet (UV) detection (23, 32), ion chromatography (IC) (54), infrared spectroscopy (IR) (32), Fourier transform-infrared (FT-IR) spectroscopy (48), nuclear magnetic resonance (NMR) spectroscopy (55), high-performance liquid chromatography (HPLC) (56), high-performance size exclusion chromatography (HPSEC) (57), high-performance gel permeation chromatography (HPGPC) (58), scanning electron microscopy (SEM) (48), atomic force microscopy (AFM) and X-ray diffraction (XRD) (37, 48, 59). The structural characteristics of various TCMPOs, including their monosaccharide compositions, average Mw, backbone structures as well as conformational structures, are exhibited in Table 2, together with the sources, geographical origins and structural characterization methods.
Most often, the analysis of monosaccharide composition and proportion demands cleavage for glycosidic bonds via acid hydrolysis, followed by derivatization, detection along with measurement through HPLC, GC, or GC/MS (38, 43, 44, 57, 60, 61). In the process of derivatization, 1-phenyl-3-methyl-5-pyrazolone (PMP) is one of the best precolumn derivatization reagents in the determination of monosaccharides because its derivatives are not easy to cleave and generate isomeric peaks (48, 62). It is noteworthy that high-performance anion-exchange chromatography with pulsed amperometric detection (HPAEC-PAD) was increasingly used by investigators to detect the composition of monosaccharides because there is no need for derivatization (35, 63).
As shown in Table 2, most TCMPOs are mainly composed of glucose (Glc), galactose (Gal), mannose (Man), arabinose (Ara), rhamnose (Rha), glucuronic acid (GlcA), galacturonic acid (GalA), xylose (Xyl), and fucose (Fuc) with different molar ratios. It is noteworthy that G. lucidum polysaccharides were detected to contain glucosamine (GlcN) with a percentage of 1.1% (18). The diversity of monosaccharide composition and their molar ratio is associated with the TCM sources, geographical origins and harvest season. For example, monosaccharide composition differences were discovered for S. fusiforme harvested in Qingdao, Wenzhou, separately (27, 37). In addition, different monosaccharides were detected in the polysaccharides of S. fusiforme that were harvested in Qingdao in May, July, and August. The polysaccharides of S. fusiforme harvested in July had the highest content of Xyl and Rha (23, 28, 29, 37). The differences in monosaccharide composition in polysaccharide fractions are also closely relevant to the extraction and separation methods. For instance, DFP-H, DFP-M, DFP-U as well as DFP-P were obtained from D. indusiata with different extraction methods and possessed similar monosaccharide compositions but exhibited different molar ratios of constituent monosaccharides (22). LP1, LP2 along with LP3 were extracted and partitioned from L. japonica by the ethanol precipitation technique with various final concentrations. The dominant monosaccharides of LP1 were Ara, Man, Gal and Glu at a molar proportion for 2.63:1.46:1:0.47, whereas LP2 as well as LP3 comprised a total made up of Rha, Ara, Xyl, Man, Gal and Glu at molar proportions for 0.13:1:0.3:1.28:1.64:1.19 as well as 0.39:1:0.67:3.01:2.47:0.99, correspondingly (49).
Multiple analytical techniques, including osmometry, viscosity measurement, sedimentation, GPC, HPGPC, HPSEC, and HPLC, are employed to determine the Mw distribution of TCMPOs, of which HPGPC and HPSEC are the most commonly utilized methods (38, 39, 43, 44, 64). As mentioned above, polysaccharides are never pure compounds regardless of how many procedures have been applied for purification. The acquired TCMPOs are always within either narrow or broad Mw ranges (12). Therefore, only the average Mw of TCMPOs can be detected. In addition, the average Mw mainly depend on the separation method, such as the different final ethanol concentrations (49), running buffers (23, 27, 48), separation membranes (18). Different Mw ranging from ∼103 to ∼107 Da were discovered under a variety of experimental conditions and source materials, which are demonstrated in Tables 1, 2.
The core of backbone structural analysis of TCMPOs is the sequence of monosaccharides and the configuration, type as well as location of glycosidic linkage. Generally, the priority is to investigate the backbone of polysaccharides with its branch link sites, the ring type (pyran or furan), the linkage sequence and the absolute configuration (D- or L-type) of monosaccharide residues, the α- or β-isomeric form of each glycosidic bond and feature groups (sulfate group, acetyl group, phosphate group, methyl group and so on) that may connect (12, 20, 65). Due to the complex structure of polysaccharides, the degradation (acid hydrolysis, periodate oxidation, smith degradation, etc.) of polysaccharides is usually performed first, followed by instrumental analysis methods (FT-IR, NMR, GC–MS, ion mobility-mass spectrometry, etc.) to obtain structural information (12, 48, 66, 67).
Studies have shown that the different fractions obtained from the same TCM may share the same glycosyl linkages, while the percentage of each glycosyl bond type varies significantly. For example, a structural investigation of two polysaccharide fractions (RRP1 and RRP2) from Rhodiola rosea indicated that RRP1 consisted of 1→6 or 1→ (50.63%), 1→2 or 1→4 (25.9%) as well as 1→3 (23.47%) glycosidic connections, while RRP2 consisted of 1→6 or 1→ (23.5%), 1→2 or 1→4 (60.0%) and 1→3 (16.5%) glycosidic connections (48). Furthermore, there were three polysaccharide fractions (LP1, LP2 and RRP2) from L. japonica, among which LP1 contained non-reducing terminal residues and (1→6)-linked (60%), (1→2)-/(1→4)-linked (35%) and (1→3)-linked (5%) glycosyl bonds, LP2 contained non-reducing terminal residues and (1→6)-linked (70%), (1→3)-linked (24%) and (1→2)-/(1→4)-linked (6%) glycosyl bonds, and LP3 contained non-reducing terminal residues and (1→6)-linked (24%), (1→3)-linked (59%) and (1→2)-/(1→4)-linked (17%) glycosyl bonds (49). Three kinds of maidong polysaccharides were examined for their structures, and these polysaccharides were all composed of Fruf-(2→,→2)-Fruf-(6→, →6)-Glcp-(1→ and →1, 2)-Fruf-(6→with molar ratios of 5.0:18.2:1.0:5.3 (Liriope spicata, LSP), 6.8:15.8:1.0:5.8 (Ophiopogon japonicus, OJP), as well as 8.3:12.3:1.0:3.9 (Liriope muscari, LMP) (53). In addition, the extraction and isolation method of TCMPOs may not affect their main chain structure. For instance, DFP-H, DFP-M, DFP-U, and DFP-P were obtained from D. indusiata with different extraction techniques, although variations in the kinds of glycosidic linkages and polysaccharide structures were not noticed (22). The structural characterization of TCMPOs is shown in Table 2, which indicates that most of the researchers actually did not further explore the structure of TCMPOs. The structure of TCMPOs is tightly related to biological activities (12, 57, 67), hence, it is particularly important to focus research on primary structure identification in the future.
Generally speaking, polysaccharides within aqueous solutions show a variety of chain conformations, including aggregates, random coils, as well as distinct helical forms (single, double, and triple helices) (43, 68–70). Some of the advanced technologies have been used to investigate the conformational features of polysaccharides, such as HPLC-static light scattering (HPLC-SLS) (70), HPLC-dynamic light scattering (HPLC-DLS) (70), viscosity test using the hypothesis of diluted polymer solutions (69), the Congo red test (28, 48), differential scanning calorimetry (DSC) (19), atomic force microscopy (AFM) (28, 43), circular dichroism (CD) (57, 69), fluorescence correlation spectroscopy (FCS) (69), transmission electron microscopy (TEM) (71), scanning electron microscopy (SEM) (28), and NMR spectroscopy (19, 72). For instance, research was done on the senior structures of two distinct polysaccharide fractions (RRP1 and RRP2) obtained from R. rosea, which were eluted using distilled water as well as 0.1 M NaCl solution, correspondingly (48), RRP1 was found to have a triple-helical conformation by the Congo red test, whereas RRP2 did not. RRP1’s surface included a modest lamellar or irregular dendritic structure, according to SEM tests, while RRP2’s surface topography was smooth and featured big wrinkles and drop-shaped bulges on the margins. AFM suggested that partial aggregation was observed in the polysaccharide chains of RRP1, and RRP2 had no molecular aggregations. These findings showed that the different elution solvents may have an impact on the advanced structure of TCMPOs. As shown in Table 2, only very few of the researchers have studied the senior structures of TCMPOs. The complexity and polymorphism of polysaccharides unquestionably bring major obstacles to their higher structural identification. Since the superior structures of polysaccharides may be more related to their bioactivities than to their primary structures (12, 51), further research and confirmation still need to be conducted in the future. Moreover, future technology development needs to concentrate on characterizing the high-level structural dynamic changes of polysaccharides (69).
Few previous studies regarding the correlations between structure and antiobesity effects of various TCMPOs were stated, and it is not simple to connect the structures of TCMPOs with their antiobesity effects. Nevertheless, as shown in Figure 1, some correlations can be inferred as follows.
It is generally accepted that the biological activities for polysaccharides are closely correlated with their Mw. According to related reports, TCMPOs with relatively high Mw usually demonstrate greater antiobesogenic activity than those with lower Mw. For example, varied Mw polysaccharides from H. sinensis oral administration to high fat diet (HFD)-induced obese rats, the high-Mw polysaccharides in fraction H1 (>300 kDa) showed greater antiobesogenic effects than the low-Mw polysaccharides in fraction H2 (10–300 kDa) as well as H3 (<10 kDa) (21). Administration of fraction H1 decreased body weight by ∼50% after 12 weeks and reduced the visceral fat and homeostatic model assessment-insulin resistance (HOMA-IR) index contrasted to the HFD control group, whereas fractions H2 and H3 only reduced the visceral fat pad mass and produced no influence on body weight or HOMA-IR index. In another study, the high-Mw polysaccharide PSF (≥100 kDa) from Polygonatum kingianum displayed better regulation of lipid metabolism via enhancing insulin sensitivity and more tightly controlling the make-up and activities of the gut microbiota (73). Comparable outcomes were also observed within the investigation of G. lucidum polysaccharide (>300 kDa) (18) and Pseudostellaria heterophylla polysaccharide (50∼210 kDa) (74).
Nevertheless, the association between the Mw of TCMPOs and their antiobesogenic activity is not simply a positive or negative correlation, and there are special cases that put forward different views (22, 75, 76). For instance, the antiobesity effects of four polysaccharides (DFP-H, DFP-M, DFP-U, and DFP-P) isolated from D. indusiata were investigated. The Mw size of the four polysaccharides was arranged as follows: DFP-H > DFP-P > DFP-M > DFP-U, whereas DFP-P showed the highest cholesterol/bile acids (BAs) attaching abilities as well as strongest inhibiting efficiency upon pancreatic lipase (22). Furthermore, it is worth noting that both DFP-P as well as DFP-H displayed significantly greater in vitro attaching characteristics, which includes fat, cholesterol as well as BAs, and lipase inhibiting properties compared to DFP-M and DFP-U, which may be because the relatively high Mw could reduce its water solubility, resulting in a decrease in hydrophilicity, thus enhancing the affinity for fat/cholesterol by increasing its surface aggregation (22, 76). The aforementioned data suggests that polysaccharides’ antiobesity effect is nevertheless enhanced by a reasonably high Mw. This may be explained by the fact that polysaccharides’ structural stability can only be preserved by a sufficiently high Mw (63). However, based on previous studies, maintaining a conformation with high biological activity of polysaccharides is not negatively impacted by either an excessive or modest Mw (77, 78), and the correlation between Mw and the antiobesogenic activity of TCMPOs should be further investigated.
The biofunctional activities of TCMPOs could be affected by their backbones and conformational properties. Prior studies have demonstrated that the structural properties of polysaccharides, including types of glycosidic bonds, monosaccharide compositions and molar ratios, degrees of branching (DBs) in the backbone, are closely related to their biological activity (59, 79, 80). Studies have found that β-glycosidic linkages usually exhibit stronger antiobesity activity than α-glycosidic linkages, causing the α-amylase in human small intestine which is specific for α-glycosidic linkages (55, 75). Polysaccharides rich in β-glycosidic linkages can remain undigested and are sequentially fermented by colonic microflora, thus playing a relatively important role in weight loss (55). DFP-H, DFP-M, DFP-U, and DFP-P obtained from D. indusiata possessed similar monosaccharide compositions but exhibited different molar ratios. Findings indicated that DFP-P and DFP-H were better at binding fat than DFP-U and DFP-M, which might be related to the higher ratios of galactose and glucose (22). Two polysaccharide components (TSP-2 and TSP-1) were extracted through Toona sinensis leaves. TSP-1 demonstrated more hepatoprotective action than TSP-2, which may be related to TSP-1’s higher galactose and glucose levels as well as its higher branching residue concentration (81). It is worth mentioning that the DB in the backbone is not positively correlated with activity; only the suitable DB is advantageous to the arrangement of the triple helical configuration (79).
The conformational features (spherical, random coil, double-helix, triple-helix, worm-like, rod-like) of polysaccharides may be more related to their bioactivities than the primary structures. Polysaccharides with triple-helix conformations exhibit more potent biological effects, including anti-inflammatory, antidiabetic and immunomodulatory effects, than those with other structures, and some of them, such as lentinan and schizophyllan, have been used in clinical therapeutics (70, 82). As previously mentioned, two polysaccharide fractions (RRP1 and RRP2) isolated through R. rosea were identified with different conformational features, and subsequent research demonstrated that the triple-helix structure enabled fraction RRP1 to exhibit more prominent antioxidant and hepatoprotective properties than RRP2 (48). Although the above structure-bioactivity interaction analyses of TCMPOs were not aimed at understanding their antiobesity activities, the anti-inflammation, antioxidant and hepatoprotective activities were considered as the potential requirements for the treatment of obesity (83, 84). It can be inferred that the conformational features of TCMPOs will also affect their antiobesity activity.
The functional group contents of polysaccharides are also linked to their bioactivities, like uronic acid, sulfuric acid and aminohexose (38, 85). Uronic acid residues, in particular, have the ability to modify the physiochemical characteristics and solubility of the corresponding polysaccharide conjugates, hence augmenting their biological activities. In a recent study, five fractions (MFPs-30-60, MFPs-30-80, MFPs-90-40, MFPs-90-60 as well as MFPs-90-80) had been purified from Fructus Mori, and in vitro studies revealed that MFPs-90-40, MFPs-90-60, and MFPs-30-60 showed a better capacity to bind BAs than MFPs-90-80 and MFPs-30-80, which was attributed to the increased uronic acid concentration and indicated that the uronic acid content was a significant signal representing the polysaccharide fractions’ hypolipidemic effects (86). In addition to the functional groups originally existing in polysaccharides, structural modifications, such as sulfation, acetylation, deacetylation, phosphorylation, acetylation, hydroxy-methylation, selenylation as well as complexation with zinc or iron, are of great significance to the physical and biological activities of polysaccharides. For example, the sulfation of G. lucidum polysaccharide (GLP) improved its antioxidant activities and BA-binding capacities (87). According to a different study, selenium modification may strengthen Chinese angelica polysaccharide’s hepatoprotective as well as antioxidant properties (88). Overall, the structure-bioactivity analyses of TCMPOs should be strengthened to better clarify the structural basis of their antiobesogenic activity.
Polysaccharides intake mainly regulates appetite and satiety through physical effects and chemical humoral stimulation effects (6), hence limiting the amount of food and energy consumed to stop the development of obesity. As shown in Figure 2, with bulking and viscosity-producing capabilities, TCMPOs could contribute to postprandial satiety by inducing gastric distension and delaying gastric emptying (89). Moreover, the appropriate ingestion of TCMPOs (such as Amorphophallus konjac polysaccharides) that, when hydrated, form viscous colloidal dispersions impacts several aspects of gastrointestinal (GI) function, including slowing down the transit of the small intestine (90, 91), inhibiting dietary enzymes from adhering to their substrates (especially fats and carbohydrates) (89), and creating an absorbent barrier layer within the small intestine by interacting with the mucosa (89, 91–93). This prolongation of the intestinal phase of nutrient processing and absorption may enhance satiety and help regulate food intake (89, 94).
Figure 2. Antiobesity mechanism of TCMPOs based on the modulation of appetite and the regulation of fat absorption and synthesis. (a) TCMPOs modulate appetite and satiety effect. (b) TCMPOs regulate the digestion and absorption of exogenous lipids. (c) TCMPOs combat obesity by weakening the synthesis of endogenous lipids. TCMPOs, Traditional Chinese medicine-derived polysaccharides; PL, Pancreatic lipase; BAs, bile acids; PARP, poly (ADP-ribose) polymerase; PPARγ, peroxisome proliferator-activated receptor γ; C/EBPα, CCAAT/enhancer binding protein α; LPL, lipoprotein lipase; SREBP-1c, sterol regulatory element binding protein-1c; CPT-1α, carnitine palmitoyltransferase-1 alpha; ACC1, acetyl-CoA carboxylase 1; FAS, fatty acid synthase; ELOVL 6, long-chain elongase; DGAT, diacylglycerol acyltransferase.
More significantly, TCMPOs have the ability to control the release of gut hormones as well as peptides that influence appetite along with food intake, including leptin, adiponectin, glucagon-like peptide 1 (GLP-1), and peptide YY (PYY). A study demonstrated that Holothuria leucospilota polysaccharide (HLP) supplement outcomes with an amelioration in the levels of leptin and adiponectin, with the levels of leptin concentrations in the typical range exhibit benefits for preventing appetite, improving fatty acid oxidation, as well as decreasing body fat, but excess levels of leptin could lead to insulin resistance (95). Most notably, HLP effectively reversed the overexpression of hepatic acetyl-CoA carboxylase (ACC) and CD36 to prevent fat buildup within the liver and prevent adipocytes from secreting too much leptin (96). Another study illustrated that P. cyrtonema polysaccharides could activate the T1R2/T1R3-mediated cAMP signaling pathway to stimulate GLP-1 production and decrease appetite (97). Furthermore, some investigations have demonstrated that the microbial fermentation of TCMPOs to form short-chain fatty acids (SCFAs) may encourage the production of GLP-1 and PYY (discussed in detail later), leading to elevated insulin and reduced glucagon release as well as appetite suppression (98, 99). The sole known orexigenic or hunger hormone, ghrelin, has been linked to the management of long-term energy balance as well as has the ability to increase appetite and meal initiation (4). Cholecystokinin (CCK), another anorexigenic intestinal hormone, is released in response to meal consumption and is involved in satiety (4). However, the potential effects of TCMPOs on ghrelin and CCK responses and the TCMPOs-mediated ghrelin/CCK relation to hunger and satiety ratings have rarely been reported and still require further research.
It is common knowledge that obesity occurs when energy intake exceeds energy consumption; thus, it works well to prevent obesity by limiting the absorption of high-energy foods, especially fat from food. An essential lipolytic enzyme generated and released by the pancreas, pancreatic lipase (PL) is primarily involved in the hydrolysis of 50–70% of total dietary fats and is essential for the absorption of dietary triacylglycerols, which it does by hydrolyzing them into monoacylglycerols as well as fatty acids (6, 100–102). Emerging studies have found that TCMPOs could act as an effective inhibitor of PL to exert potentially antiobesogenic effects (Figure 2; Table 3). For example, Wu et al. (22) assessed the anti-pancreatic lipase activity in vitro about four polysaccharide fractions (DFP-H, DFP-M, DFP-U, and DFP-P) obtained from D. indusiate through different extraction techniques, as well as the inhibition of PL has been determined as 58.39 ± 0.98%, 52.65 ± 0.83%, 54.67 ± 1.03%, and 63.20 ± 1.11%, correspondingly. Other researches (103–107) also demonstrated that TCMPOs exhibit obvious inhibitory effects on PL, which indicates that TCMPOs could be developed as potential antiobesity agents to impede the digestion and absorption of dietary fat.
Bile acids, another important physiological substance that is closely related to lipid metabolism, are the end products of cholesterol catabolism in the liver and secreted into the small intestine, which might activate PL and form micelles containing dietary fat and lipophilic vitamins (A, D, E, and K), thus facilitating fat absorption, distribution, metabolism, and excretion (108–110). Studies have shown that TCMPOs could bind with BAs (Figure 2; Table 3) and destroy the mixed micelles formed by BAs with dietary lipids, thus preventing the digestion and absorption of dietary lipids. Hu et al. (106) reported the BAs-binding abilities of Plantago asiatica L. polysaccharides, which could bind more BAs than simvastatin (a commercially available hypolipidemic drug) at experimental concentrations (2.5 and 10 mg/ml). Moreover, Shi et al. (111) observed that Ophiopogon japonicus polysaccharides (MDG-1) could reduce the synthesis of cholesterol in the liver and inhibit body growth within obese mice by binding to BAs in the lumen, reducing their reflux into the liver, and suppressing the enterohepatic circulation of BAs. In addition to the two principal targets of preventing fat digestion and absorption demonstrated above, the polysaccharides extracted from some kinds of TCM could also affect how well exogenous lipids are absorbed and digested by decreasing the activity of α-amylase, pepsin and trypsin (103, 104, 106, 107), affecting the stability of the cholesterol-micelle and reducing the amount of the unbroken cholesterol-micelle (22, 103, 106).
The size (hypertrophy) and quantity (hyperplasia) of adipocytes define the bulk of adipose tissue (112–114). The hypertrophy of adipocytes is mainly caused by lipid accumulation, and the hyperplasia of adipocytes is strongly linked to the growth as well as differentiation of preadipocytes (46, 112). Multiple reports have demonstrated that polysaccharides from diverse TCM sources may reduce the production of endogenous lipids by preventing preadipocyte differentiation and promoting the apoptosis of adipocytes (Figure 2) (46, 115, 116). For example, Morus alba L. polysaccharides (JS-MP-1) could decrease the cell viability of 3T3-L1 within a dose-dependent manner to 91, 75, 68, and 54% viabilities at the experimental levels of 50, 100, 200, and 500 μg/ml, correspondingly, suggesting that it might prevent preadipocytes from growing and proliferating (46). In addition, JS-MP-1 also induced apoptosis within 3T3-L1 preadipocyte cells by inducing mitochondrial dysfunction and activating apoptosis-related proteins, like caspase 9 and 3 and poly (ADP-ribose) polymerase (PARP) (46). Poria cocos polysaccharides (PCCPs) showed the ability to decrease 3T3-L1 cell viability and inhibit intracellular lipid accumulation at relatively high concentrations (117). Xu et al. (118) reported L. barbarum polysaccharides (LBPs) presented similar inhibiting impacts upon the division for 3T3-L1 cells, which might be attributed to the lowered expression of the differentiation-related genes peroxisome proliferator-activated receptor γ (PPARγ) and CCAAT/enhancer attaching protein α (C/EBPα) after LBP treatment.
Lipoprotein lipase (LPL), a glycoprotein distinguishes free fatty acids from triglycerides in chylomicrons (CMs) as well as very low-density lipoproteins (VLDLs) (119–121). Accumulating evidence has revealed that polysaccharides from various TCM sources can reduce lipid deposition by modulating the activity of LPL (119, 122, 123). Yang et al. (123) demonstrated that Armillariella Mellea polysaccharides (AAMPs) enhanced the level of LPL and the expression of two critical lipases (adipose triglyceride lipase and hormone-sensitive lipase) and specifically inhibited the expression of sterol regulatory element binding protein-1c (SREBP-1c), leading to a significant reduction in fat accumulation in the liver. Moreover, it is found that the administration of TCM polysaccharides could also inhibit the mRNA expression concentrations of lipogenesis-related enzyme genes (acetyl-CoA carboxylase 1, ACC1; fatty acid synthase, FAS; long-chain elongase, ELOVL 6 and diacylglycerol acyltransferase, DGAT) and elevate the mRNA expression for a lipolysis-related gene (carnitine palmitoyltransferase-1 alpha, CPT-1α) (18, 118, 124–127), thus weakening the lipogenic pathway and strengthening the fat decomposition pathway.
The human intestinal cavity contains a variety of microorganisms, most notably bacteria. More and more researches are pointing to the resident microbiota’s critical roles in a range of physiological as well as pathological bodily processes (26, 114, 128). Plenty of research conducted in the last few decades have focused on the relationship between gut bacteria and obesity. Research has indicated that a rise within the ratio of Firmicutes to Bacteroidetes (F/B) was observed in obese individuals, and increased F/B ratio correlates with strengthened energy absorption capacity, which ultimately results in obesity (114, 129, 130). For example, by transplanting the faecal microbiota from adult female twin pairs that were discordant for obesity into germ-free mice, Ridaura et al. (131) discovered that metabolic abnormalities linked to obesity could be spread through uncultured faecal communities.
It has been discovered that some TCM sources include polysaccharides that can fight obesity through altering the gut microbiome (Figure 3; Table 4). Typically, for instance, Stichopus japonicus polysaccharides (SCSPs) and depolymerized SCSPs (d-SCSPs) exhibited antiobesity impacts within HFD-fed mice through changing the proportion of F/B; enhancing the abundances of Akkermansia muciniphila, Parabacteroides goldsteinii as well as SCFA-producing microbiota (Bacteroides, Alloprevotella, Ruminiclostridium, and Butyricicoccus); and decreasing the abundances of Proteobacteria and pathogenic Escherichia-Shigella (132–136). Furthermore, it was shown that purified L. japonica polysaccharide (LJP61A) may also significantly reverse the increased F/B ratio and deceased abundance of Akkermansia induced by the HFD-diet (137). A. muciniphila, a mucin-degrading bacterium commonly found in the gut, was found to have close ties to intestinal barrier integrity as well as resistant to insulin, which helps it fight obesity and related metabolic illnesses (138–140). In addition, the increasing abundance of P. goldsteinii was found to be mostly responsible for the anti-obesity benefits of H. sinensis polysaccharides, which was linked to improved intestinal integrity, decreased inflammatory levels, as well as improved adipose tissue thermogenesis (21). Overall, TCMPOs-dietary interventions were reported to combat obesity by regulating the ratio of F/B, boosting the colonization of some advantageous bacteria and decreasing the survival of detrimental bacteria linked to inflammation and the onset of obesity.
Figure 3. Antiobesity mechanism of TCMPOs based on the modulation of gut microbiota and their metabolites (① SCFAs; ② TMA; ③ Secondary BAs) and the protection of intestinal barriers. TCMPOs, traditional Chinese medicine-derived polysaccharides; GPR41(43), G-protein coupled receptors 41(43); GLP-1, glucagon-like peptide 1; PYY, peptide YY; SCFAs, short chain fatty acids; TMA, trimethylamine; TMAO, trimethylamine N-oxide; IGN, intestinal gluconeogenesis; BAs, bile acids; TGR5, takeda G protein Receptor 5; FXR, farnesoid X Receptor; BSH, bile salt hydrolase; ZO-1, zonula occludens-1; Klf4, kruppel-like factor 4; Muc2, mucin 2; LPS, lipopolysaccharide; RegIIIγ, regenerating islet-derived protein 3 γ; IL-4, interleukin-4; IL-10, interleukin-10; TNF-α, tumor necrosis factor-α; IL-1β, interleukin-1β; IL-6, interleukin-6; MCP-1, monocyte chemoattractant protein-1.
Numerous routes may be involved in how TCMPOs impact the relationship between the host’s gut flora and itself. First, as shown in Figure 3 and Table 4, microbial fermentation of TCMPOs in the intestine enhances the synthesis of metabolites like SCFAs, consisting predominantly acetate, propionate, as well as butyrate, which are essential for the health homeostasis and wellbeing of the host. TCMPOs intervention could lead to an increased production of SCFAs and a lower luminal pH, which effectively inhibits the growth of pathogenic microbiota (134, 141). SCFAs were also reported as ligands for G-protein coupled receptors (GPCRs), GPR41 and GPR43, and propionate and butyrate showed closer affinity for GPR41, which serves as gut microbe-related energy sensors within the intestines and sympathetic nervous system (142–145). Propionate and acetate were reported to be more active in activating GPR43, which prevents fat from accumulating within adipose tissue, inhibits insulin signaling within adipocytes, and increases the metabolism of glucose and unincorporated lipids within other tissues (like liver and muscle) (59, 145, 146). For instance, treatment with Morus alba. L leaf polysaccharide (MLP) significantly reduced body weight gain and in a dose-dependent manner reversed the decrease in SCFAs concentration in HFD-fed mice (84). Astragalus polysaccharides (APS) could ameliorate hepatic lipid metabolism within HFD-fed mice by enriching the abundance of a potent acetate-producing bacterium (Desulfovibrio vulgaris) (147). Moreover, SCFA-mediated GPCR activation in the gut could induce the secretion of the endocrine hormones GLP-1, PYY, and glucose-dependent insulinotropic polypeptide (GIP), which have been proven to be important in preventing or treating obesity (144, 148). Additionally, by inducing intestinal gluconeogenesis (IGN) gene expression in enterocytes, SCFAs derived from the microbial fermentation of polysaccharides might enhance a number of aspects of energy metabolism in both insulin-sensitive and insensitive states (149, 150). Propionate, being an IGN substrate, can typically directly start a gut-brain neuronal circuit that has positive effects on energy management and glucose regulation (149, 150). In summary, TCMPOs exhibit an antiobesity effect by increasing the production of SCFAs, which could decrease the pH of the lumen, activate GPR41 and GPR43, induce the secretion of certain endocrine hormones, and activate IGN to improve energy metabolism.
In addition to SCFAs, intestinal microorganisms can also generate trimethylamine (TMA), which may affect host metabolism (Figure 3). The intestinal anaerobic bacteria can metabolize choline as well as L-carnitine from food sources (e.g., animal liver, red meat, egg yolks and fish) to create TMA (151). This gut-microbially produced TMA can be further decomposed into dimethylamine (DMA) and methylamine (MA) or it can be further carried into the liver and subsequently turned into trimethylamine N-oxide (TMAO), which is linked to an increased risk of obesity (151–153). Chen et al. (154) demonstrated that Flos Lonicera polysaccharide supplementation could reverse the increase in TMAO level caused by an HFD. Similarly, after LBP treatment, the level of plasma TMAO was considerably lower within the HFPD (LBP + HFD-diet) group compared with the HFD group (155). These investigations showed that the polysaccharides of multiple TCMs could lessen the oxidation of TMA and thereby serve as a possible dietary supplement to help reduce obesity and related diseases via gut-organ axes.
Aside from generating novel metabolites, intestinal bacteria may also modify the physicochemical properties of endogenous metabolites (Figure 3). BAs, as mentioned above, are small molecules that have important functions in controlling lipid metabolism. Ninety-five percent of BAs are reabsorbed efficiently from the distal ileum, entering the enterohepatic circulation, and are circulated back to the liver (151). In addition, BAs can also be converted from primary to secondary BAs in the colon, a function that is restricted to a much narrower range of anaerobic bacteria (typically clostridial species) (156). Both primary and secondary BAs are essential signaling molecules that can modify energy metabolism through interact with receptors, Takeda G protein Receptor 5 (TGR5) (157, 158), and Farnesoid X Receptor (FXR) (6, 159). Activation of TGR5 and FXR facilitates energy expenditure and encourage thermogenesis, especially in the liver, muscles and brown adipose tissue (BAT), which lowers body weight (151, 156, 158). Studies have reported that TCMPOs supplementation (such as supplementation of PCCPs and H. sinensis polysaccharides) raises the abundance of secondary BA-producing bacteria (Clostridium), thus enabling the better activation of TGR5 and FXR, which may serve as a potential antiobesity mechanism for TCMPOs (21, 32). Moreover, HFD-induced dysbiosis can lead to the functional loss of bile salt hydrolase (BSH), which in the gut microbiota is in charge of deconjugating primary conjugated BAs, resulting in an imbalance in primary and secondary BAs in the colon, thus inducing glycolipid metabolism-related diseases (151, 158). Huang et al. (160) observed that Gracilaria lemaneiformis polysaccharide intervention markedly raised the proportional abundances of BSH-secreting microbiota (Bacteroides and Lactobacillus), hence encouraging the conversion of primary bile acids into secondary bile acids and the excretion of secondary bile acids, which in turn lowers cholesterol and helps prevent fat buildup brought on by a high-fat diet. Overall, fermentable TCMPOs could fight against obesity by generating SCFAs and TMA/TMAO and modifying the physicochemical properties of BAs. In addition, other microbially derived metabolites, such as branched-chain amino acids (BCAAs) and serotonin (5-HT), have also been found to be strongly associated with the emergence of obesity (151). Future studies on how TCMPOs affect the production of BCAAs and 5-HT should be conducted.
Numerous studies point to the interaction among intestinal permeability as well as TCMPOs as one mechanism connecting obesity and related diseases (Figure 3; Table 4). Oral treatment with TCMPOs beneficially maintained intestinal barrier integrity primarily by promoting intercellular tight junctions and strengthening the intestinal mucous layer. As shown in Figure 3 and Table 4, various studies have reported that TCMPOs, such as L. japonica polysaccharides and S. fusiforme polysaccharides, could elevate the expression of tight junction proteins (TJPs), including zonula occludens-1 (ZO-1), claudin-1 and occludin, to regulate the tight junction structure, thus protecting the integrity of the gut epithelium (18, 30, 32, 161, 162). Additionally, L. japonica polysaccharide (LJP61A) administration enhanced the mRNA levels of Kruppel-like factor 4 (Klf4, a marker of goblet cells) and mucin 2 (Muc2, a protein secreted by goblet cells) in HFD-fed mice, indicating that LJP61A may be able to counteract the HFD-induced reduction in goblet cells (137). Specifically, it has been reported that LJP61A may also elevate the relative abundance of A. muciniphila, which is reported that it may enhance the expression of regenerating islet-derived protein 3 γ (RegIIIγ) in response to gram-positive bacteria in the intestine and aid in its own survival, thereby promoting the development of the intestinal mucus layer (137, 163). Lipopolysaccharide (LPS), a cell wall component of gram-negative pathogenic bacteria, can enter the circulation and trigger a variety of transcription factors associated with inflammation, resulting in the initiation of chronic low-grade inflammation that is known as a causal factor of obesity and hepatic steatosis (32, 164). Oral treatment with TCMPOs could improve the intestinal barrier to prevent LPS from entering the circulation, hence attenuating HFD-LPS-induced inflammatory reactions and exerting the antiobesogenic effects of TCMPOs. For example, D. indusiata polysaccharides (DIPs) could maintain intestinal integrity and alleviate HFD-induced obesity by significantly reducing the levels of LPS and proinflammatory cytokines (tumor necrosis factor-α, TNF-α; interleukin-1β, IL-1β; interleukin-6, IL-6; monocyte chemoattractant protein-1, MCP-1) and enhancing the secretion of anti-inflammatory cytokines (interleukin-4, IL-4; interleukin-10, IL-10) (161, 162). It is worth noting that SCFAs, generated by microbial fermentation of TCMPOs, are considered vital energy sources for intestinal epithelial cells and can promote the development of the intestinal barrier (165).
Traditional Chinese medicine-derived polysaccharides, acting as important components with few adverse reactions and significant biological activity, have gained increasing attention during the last decade. In this review, the various extraction and purification methods, structure-activity relationship and antiobesity mechanisms of TCMPOs were systematically summarized. In particular, the antiobesity effects of TCMPOs were shown via mechanisms including the modulation of appetite and satiety, inhibition of fat absorption, modification of the gut microbiota and their metabolites as well as the protection of intestinal barriers. Furthermore, the relationship between TCMPOs’ antiobesity actions and structure is emphasized. However, there are many questions yet to be noted. (1) It is imperative to develop novel extraction technologies to improve the extraction efficiency due to the limitations of the thermal-assisted extraction of TCMPOs mentioned above. In addition, the structure of TCMPOs varies with geographical origin, the collected part of the herb, the harvesting season, and the processing method. It is unreliable to obtain TCMPOs from different sources of TCM with the same extraction method. Therefore, future research should screen out specific extraction methods based on the structural characteristics of various TCMPOs to protect the structural integrity of the target polysaccharides. (2) Consistency, repeatability, and reliability of the results are hard to maintain due to different testing circumstances and the varied qualities of raw materials. It is necessary to establish standardized procedures for the gathering and processing of the source material as well as for the extraction, isolation, and purification of TCMPOs. (3) Owing to TCMPOs’ extensive structural diversity and complexity, the senior structural characteristics have not been clarified, and the relationships between their senior structure and antiobesity activity have not been established. Therefore, we need to learn from the structural identification methods of other biomacromolecules to accelerate the structural analysis of TCMPOs. Based on the accurate structure of TCMPOs, further research and confirmation should focus on the structure-bioactivity interaction of TCMPOs to better clarify the structural basis of their antiobesogenic activity. (4) Given that obesity and associated metabolic disorders are often multigene variant, multitarget interfered and multidisease related, it is inappropriate to treat obesity in a single way, while TCMPOs possess the advantages of having multiple targets, being less toxic and having side effects in the treatment of obesity than current therapeutics. However, the feature structure and functional groups within TCMPOs that exert antiobesogenic activity, as well as the antiobesity mechanism of TCMPOs, have not been systematically clarified. Thus, there is a great need for further clarifying the feature structure in TCMPOs and its molecular targets responsible for the observed antiobesogenic activity. (5) Although the antiobesogenic activity of TCMPOs has been demonstrated in many experiments, more attention should be given to clinical studies because animal models or human body tissues and cell experiments in vitro do not accurately represent their existence in the human body.
This review may serve as a valuable reference to the extraction, purification, structural-property correlations and antiobesity mechanism of TCMPOs while offering a possible foundation for their usage to the food along with medical fields. Future studies should concentrate on resolving the aforementioned issues, and therapeutic approaches utilizing TCMPOs for the management of obesity and obesity-related disorders could represent yet another advancement in the realms of functional foods, pharmaceuticals, and medicine.
NZ: Investigation, Methodology, Writing—original draft, Writing—review and editing, Project administration. XC: Funding acquisition, Investigation, Methodology, Supervision, Writing—original draft. XW: Writing—original draft. JG: Investigation, Methodology, Writing—review and editing. JC: Investigation, Writing—review and editing. SG: Investigation, Writing—review and editing, Funding acquisition, Methodology, Project administration, Resources, Supervision.
The author(s) declare financial support was received for the research, authorship, and/or publication of this article. This work was supported by the National Natural Science Foundation of China (No. U21A20406 and 82104701), Key Project at Central Government Level: the ability establishment of sustainable use for valuable Chinese Medicine Resources (No. 2060302), the Science Fund Program for Outstanding Young Scholars in Universities of Anhui Province (No. 2022AH030064), and the Foundation of Anhui Province Key Laboratory of Pharmaceutical Preparation Technology and Application (No. 2021KFKT10).
The authors declare that the research was conducted in the absence of any commercial or financial relationships that could be construed as a potential conflict of interest.
All claims expressed in this article are solely those of the authors and do not necessarily represent those of their affiliated organizations, or those of the publisher, the editors and the reviewers. Any product that may be evaluated in this article, or claim that may be made by its manufacturer, is not guaranteed or endorsed by the publisher.
1. Bluher M. Obesity: global epidemiology and pathogenesis. Nat Rev Endocrinol. (2019) 15:288–98. doi: 10.1038/s41574-019-0176-8
2. Powell-Wiley TM, Poirier P, Burke LE, Despres JP, Gordon-Larsen P, Lavie CJ, et al. Obesity and cardiovascular disease: a scientific statement from the American Heart Association. Circulation. (2021) 143:e984–1010. doi: 10.1161/CIR.0000000000000973
3. Zhang Q, Bai Y, Wang W, Li J, Zhang L, Tang Y, et al. Role of herbal medicine and gut microbiota in the prevention and treatment of obesity. J Ethnopharmacol. (2023) 305:116127. doi: 10.1016/j.jep.2022.116127
4. Oussaada SM, van Galen KA, Cooiman MI, Kleinendorst L, Hazebroek EJ, van Haelst MM, et al. The pathogenesis of obesity. Metabolism. (2019) 92:26–36. doi: 10.1016/j.metabol.2018.12.012
5. Zhang X, Jin C, Liu H, Hu Y, Zhou Y, Wu W, et al. Polysaccharide extract from Rosa laevigata fruit attenuates inflammatory obesity by targeting redox balance and gut interface in high-fat diet-fed rats. Food Sci Hum Wellness. (2023) 12:422–53. doi: 10.1016/j.fshw.2022.07.046
6. Zhao Z, Cheung PC, You L, Xie Q, Zhang Y. Behavior of non-digestible polysaccharides in gastrointestinal tract: a mechanistic review of its anti-obesity effect. eFood (2021) 2:59. doi: 10.2991/efood.k.210310.001
7. Heffron SP, Parham JS, Pendse J, Aleman JO. Treatment of obesity in mitigating metabolic risk. Circ Res. (2020) 126:1646–65. doi: 10.1161/CIRCRESAHA.119.315897
8. Halpern A, Mancini MC. Treatment of obesity: an update on anti-obesity medications. Obes Rev. (2003) 4:25–42. doi: 10.1046/j.1467-789X.2003.00083.x
9. Rizvi MK, Rabail R, Munir S, Inam-Ur-Raheem M, Qayyum MM, Kieliszek M, et al. Astounding health benefits of jamun (Syzygium cumini) toward metabolic syndrome. Molecules. (2022) 27:7184. doi: 10.3390/molecules27217184
10. Avogaro A, de Kreutzenberg SV, Morieri ML, Fadini GP, Del Prato S. Glucose-lowering drugs with cardiovascular benefits as modifiers of critical elements of the human life history. Lancet Diabetes Endocrinol. (2022) 10:882–9. doi: 10.1016/s2213-8587(22)00247-9
11. Buchwald H, Buchwald JN. Metabolic (Bariatric and Nonbariatric) surgery for type 2 diabetes: a personal perspective review. Diabetes Care. (2019) 42:331–40. doi: 10.2337/dc17-2654
12. Zeng P, Li J, Chen Y, Zhang L. The structures and biological functions of polysaccharides from traditional Chinese herbs. Prog Mol Biol Transl Sci. (2019) 163:423–44. doi: 10.1016/bs.pmbts.2019.03.003
13. Jiang W, Hu Y, Zhu Z. Structural characteristics of polysaccharide from Zingiber striolatum and its effects on gut microbiota composition in obese mice. Front Nutr. (2022) 9:1012030. doi: 10.3389/fnut.2022.1012030
14. Li W, Li Y, Wang Q, Yang Y. Crude extracts from Lycium barbarum suppress SREBP-1c expression and prevent diet-induced fatty liver through AMPK activation. Biomed Res Int. (2014) 2014:196198. doi: 10.1155/2014/196198
15. Ben Abdallah Kolsi R, Ben Gara A, Chaaben R, El Feki A, Patti FP, El Feki L, et al. Anti-obesity and lipid lowering effects of Cymodocea nodosa sulphated polysaccharide on high cholesterol-fed-rats. Arch Physiol Biochem. (2015) 121:210–7. doi: 10.3109/13813455.2015.1105266
16. Liang J, Xu R, Zong K, Yu N, Wu Z, Wu H, et al. Structural analysis and anti-obesity effect of Polygonatum cyrtonema polysaccharide against obesity induced by high-fat diet in mice. Int J Food Sci Technol. (2021) 56:4473–83. doi: 10.1111/ijfs.15208
17. Zha XQ, Xue L, Zhang HL, Asghar MN, Pan LH, Liu J, et al. Molecular mechanism of a new Laminaria japonica polysaccharide on the suppression of macrophage foam cell formation via regulating cellular lipid metabolism and suppressing cellular inflammation. Mol Nutr Food Res. (2015) 59:2008–21. doi: 10.1002/mnfr.201500113
18. Chang CJ, Lin CS, Lu CC, Martel J, Ko YF, Ojcius DM, et al. Ganoderma lucidum reduces obesity in mice by modulating the composition of the gut microbiota. Nat Commun. (2015) 6:7489. doi: 10.1038/ncomms8489
19. Zhang Y, Li S, Wang X, Zhang L, Cheung PC. Advances in lentinan: isolation, structure, chain conformation and bioactivities. Food Hydrocolloids. (2011) 25:196–206. doi: 10.1016/j.foodhyd.2010.02.001
20. Fang J, Wang Z, Wang P, Wang M. Extraction, structure and bioactivities of the polysaccharides from Ginkgo biloba: a review. Int J Biol Macromol. (2020) 162:1897–905. doi: 10.1016/j.ijbiomac.2020.08.141
21. Wu TR, Lin CS, Chang CJ, Lin TL, Lai HC. Gut commensal Parabacteroides goldsteinii plays a predominant role in the anti-obesity effects of polysaccharides isolated from Hirsutella sinensis. Gut. (2019) 68:248–62. doi: 10.1136/gutjnl-2017-315458
22. Wu DT, Zhao YX, Guo H, Gan RY, Peng LX, Zhao G, et al. Physicochemical and biological properties of polysaccharides from Dictyophora indusiata prepared by different extraction techniques. Polymers. (2021) 13:2357. doi: 10.3390/polym13142357
23. Wei B, Zhong QW, Ke SZ, Zhou TS, Xu QL, Wang SJ, et al. Sargassum fusiforme polysaccharides prevent high-fat diet-induced early fasting hypoglycemia and regulate the gut microbiota composition. Mar Drugs. (2020) 18:444. doi: 10.3390/md18090444
24. Huang YC, Tsay HJ, Lu MK, Lin CH, Yeh CW, Liu HK, et al. Astragalus membranaceus polysaccharides ameliorates obesity, hepatic steatosis, neuroinflammation and cognition impairment without affecting amyloid deposition in metabolically stressed APPswe/PS1dE9 mice. Int J Mol Sci. (2017) 18:2746. doi: 10.3390/ijms18122746
25. Lee BH, Chen CH, Hsu YY, Chuang PT, Shih MK, Hsu WH. Polysaccharides obtained from Cordyceps militaris alleviate hyperglycemia by regulating gut microbiota in mice fed a high-fat/sucrose diet. Foods. (2021) 10:1870. doi: 10.3390/foods10081870
26. Gan L, Wang J, Guo Y. Polysaccharides influence human health via microbiota-dependent and -independent pathways. Front Nutr. (2022) 9:1030063. doi: 10.3389/fnut.2022.1030063
27. Jin W, Wu W, Tang H, Wei B, Wang H, Sun J, et al. Structure analysis and anti-tumor and anti-angiogenic activities of sulfated galactofucan extracted from Sargassum thunbergii. Mar Drugs. (2019) 17:52. doi: 10.3390/md17010052
28. Jia RB, Wu J, Li ZR, Ou ZR, Lin L, Sun B, et al. Structural characterization of polysaccharides from three seaweed species and their hypoglycemic and hypolipidemic activities in type 2 diabetic rats. Int J Biol Macromol. (2020) 155:1040–9. doi: 10.1016/j.ijbiomac.2019.11.068
29. Wei B, Xu QL, Zhang B, Zhou T, Ke S, Wang S, et al. Comparative study of Sargassum fusiforme polysaccharides in regulating cecal and fecal microbiota of high-fat diet-fed mice. Mar Drugs. (2021) 19(7):364. doi: 10.3390/md19070364
30. Zhang Y, Zuo J, Yan L, Cheng Y, Li Q, Wu S, et al. Sargassum fusiforme fucoidan alleviates high-fat diet-induced obesity and insulin resistance associated with the improvement of hepatic oxidative stress and gut microbiota profile. J Agric Food Chem. (2020) 68:10626–38. doi: 10.1021/acs.jafc.0c02555
31. Sun S, Wang K, Sun L, Cheng B, Qiao S, Dai H, et al. Therapeutic manipulation of gut microbiota by polysaccharides of Wolfiporia cocos reveals the contribution of the gut fungi-induced PGE2 to alcoholic hepatic steatosis. Gut Microbes. (2020) 12:1830693. doi: 10.1080/19490976.2020.1830693
32. Sun S, Wang K, Ma K, Bao L, Liu H. An insoluble polysaccharide from the sclerotium of Poria cocos improves hyperglycemia, hyperlipidemia and hepatic steatosis in ob/ob mice via modulation of gut microbiota. Chin J Nat Med. (2019) 17:3–14. doi: 10.1016/s1875-5364(19)30003-2
33. Lin C, Lin Y, Meng T, Lian J, Liang Y, Kuang Y, et al. Anti-fat effect and mechanism of polysaccharide-enriched extract from Cyclocarya paliurus (Batal.) Iljinskaja in Caenorhabditis elegans. Food Funct. (2020) 11:5320–32. doi: 10.1039/c9fo03058a
34. Lin C, Su Z, Luo J, Jiang L, Shen S, Zheng W, et al. Polysaccharide extracted from the leaves of Cyclocarya paliurus (Batal.) Iljinskaja enhanced stress resistance in Caenorhabditis elegans via skn-1 and hsf-1. Int J Biol Macromol. (2020) 143:243–54. doi: 10.1016/j.ijbiomac.2019.12.023
35. Yang TH, Chiu CY, Lu TJ, Liu SH, Chiang MT. The anti-obesity effect of polysaccharide-rich red algae (Gelidium amansii) hot-water extracts in high-fat diet-induced obese hamsters. Mar Drugs. (2019) 17:532. doi: 10.3390/md17090532
36. Seca AM, Pinto D. Overview on the antihypertensive and anti-obesity effects of secondary metabolites from seaweeds. Mar Drugs. (2018) 16:237. doi: 10.3390/md16070237
37. Li ZR, Jia RB, Wu J, Lin L, Ou ZR, Liao B, et al. Sargassum fusiforme polysaccharide partly replaces acarbose against type 2 diabetes in rats. Int J Biol Macromol. (2021) 170:447–58. doi: 10.1016/j.ijbiomac.2020.12.126
38. Zhang R, Zhang X, Tang Y, Mao J. Composition, isolation, purification and biological activities of Sargassum fusiforme polysaccharides: a review. Carbohydr Polym. (2020) 228:115381. doi: 10.1016/j.carbpol.2019.115381
39. Kakar MU, Kakar IU, Mehboob MZ, Zada S, Soomro H, Umair M, et al. A review on polysaccharides from Artemisia sphaerocephala Krasch seeds, their extraction, modification, structure, and applications. Carbohydr Polym. (2021) 252:117113. doi: 10.1016/j.carbpol.2020.117113
40. Chen J, Li J, Sun A, Zhang B, Qin S, Zhang Y. Supercritical CO2 extraction and pre-column derivatization of polysaccharides from Artemisia sphaerocephala Krasch. seeds via gas chromatography. Ind Crops Prod. (2014) 60:138–43. doi: 10.1016/j.indcrop.2014.06.013
41. Zou X, Liu Y, Tao C, Liu Y, Liu M, Wu J, et al. CO2 supercritical fluid extraction and characterization of polysaccharide from bamboo (Phyllostachys heterocycla) leaves. J Food Meas Charact. (2017) 12:35–44. doi: 10.1007/s11694-017-9614-2
42. Del Valle JM, Mena C, Budinich M. Extraction of garlic with supercritical CO2 and conventional organic solvents. Braz J Chem Eng. (2008) 25:532–42. doi: 10.1590/S0104-66322008000300011
43. Yan JK, Wang WQ, Wu JY. Recent advances in Cordyceps sinensis polysaccharides: mycelial fermentation, isolation, structure, and bioactivities: a review. J Funct Foods. (2014) 6:33–47. doi: 10.1016/j.jff.2013.11.024
44. Ji X, Peng Q, Yuan Y, Shen J, Xie X, Wang M. Isolation, structures and bioactivities of the polysaccharides from jujube fruit (Ziziphus jujuba Mill.): a review. Food Chem. (2017) 227:349–57. doi: 10.1016/j.foodchem.2017.01.074
45. Zhang Y, Ren C, Lu G, Cui W, Mu Z, Gao H, et al. Purification, characterization and anti-diabetic activity of a polysaccharide from mulberry leaf. Regul Toxicol Pharmacol. (2014) 70:687–95. doi: 10.1016/j.yrtph.2014.10.006
46. Choi JW, Synytsya A, Capek P, Bleha R, Pohl R, Park Y. Structural analysis and anti-obesity effect of a pectic polysaccharide isolated from Korean mulberry fruit Oddi (Morus alba L.). Carbohydr Polym. (2016) 146:187–96. doi: 10.1016/j.carbpol.2016.03.043
47. Nie Q, Hu J, Gao H, Fan L, Chen H, Nie S. Polysaccharide from Plantago asiatica L. attenuates hyperglycemia, hyperlipidemia and affects colon microbiota in type 2 diabetic rats. Food Hydrocolloids. (2019) 86:34–42. doi: 10.1016/j.foodhyd.2017.12.026
48. Xu Y, Jiang H, Sun C, Adu-Frimpong M, Deng W, Yu J, et al. Antioxidant and hepatoprotective effects of purified Rhodiola rosea polysaccharides. Int J Biol Macromol. (2018) 117:167–78. doi: 10.1016/j.ijbiomac.2018.05.168
49. Zha X, Xiao J, Zhang H, Wang J, Pan L, Yang X, et al. Polysaccharides in Laminaria japonica (LP): extraction, physicochemical properties and their hypolipidemic activities in diet-induced mouse model of atherosclerosis. Food Chem. (2012) 134:244–52. doi: 10.1016/j.foodchem.2012.02.129
50. Yi Y, Xu W, Wang HX, Huang F, Wang LM. Natural polysaccharides experience physiochemical and functional changes during preparation: a review. Carbohydr Polym. (2020) 234:115896. doi: 10.1016/j.carbpol.2020.115896
51. Qu J, Huang P, Zhang L, Qiu Y, Qi H, Leng A, et al. Hepatoprotective effect of plant polysaccharides from natural resources: a review of the mechanisms and structure-activity relationship. Int J Biol Macromol. (2020) 161:24–34. doi: 10.1016/j.ijbiomac.2020.05.196
52. Brinckmann JA. Geographical indications for medicinal plants: globalization, climate change, quality and market implications for geo-authentic botanicals. World J Tradit Chin Med. (2015) 1:16–23. doi: 10.15806/j.issn.2311-8571.2014.0020
53. Gong Y, Zhang J, Gao F, Zhou J, Xiang Z, Zhou C, et al. Structure features and in vitro hypoglycemic activities of polysaccharides from different species of Maidong. Carbohydr Polym. (2017) 173:215–22. doi: 10.1016/j.carbpol.2017.05.076
54. Chen C, You LJ, Huang Q, Fu X, Zhang B, Liu RH, et al. Modulation of gut microbiota by mulberry fruit polysaccharide treatment of obese diabetic db/db mice. Food Funct. (2018) 9:3732–42. doi: 10.1039/c7fo01346a
55. Xu J, Wang Y, Xu DS, Ruan KF, Feng Y, Wang S. Hypoglycemic effects of MDG-1, a polysaccharide derived from Ophiopogon japonicas, in the ob/ob mouse model of type 2 diabetes mellitus. Int J Biol Macromol. (2011) 49:657–62. doi: 10.1016/j.ijbiomac.2011.06.026
56. Qu J, Tan S, Xie X, Wu W, Zhu H, Li H, et al. Dendrobium officinale polysaccharide attenuates insulin resistance and abnormal lipid metabolism in obese mice. Front Pharmacol. (2021) 12:659626. doi: 10.3389/fphar.2021.659626
57. Liu C, Cui Y, Pi F, Cheng Y, Guo Y, Qian H. Extraction, purification, structural characteristics, biological activities and pharmacological applications of acemannan, a polysaccharide from Aloe vera: a review. Molecules. (2019) 24:1554. doi: 10.3390/molecules24081554
58. Chen L, Zhang L, Wang W, Qiu W, Liu L, Ning A, et al. Polysaccharides isolated from Cordyceps Sinensis contribute to the progression of NASH by modifying the gut microbiota in mice fed a high-fat diet. PLoS One. (2020) 15:e0232972. doi: 10.1371/journal.pone.0232972
59. You Y, Song H, Yan C, Ai C, Tong Y, Zhu B, et al. Dietary fibers obtained from Caulerpa lentillifera prevent high-fat diet-induced obesity in mice by regulating the gut microbiota and metabolite profiles. Food Funct. (2022) 13:11262–72. doi: 10.1039/d2fo01632j
60. Bai Y, Zang X, Ma J, Xu G. Anti-diabetic effect of Portulaca oleracea L. polysaccharideandits mechanism in diabetic rats. Int J Mol Sci. (2016) 17:1201. doi: 10.3390/ijms17081201
61. Song Y, Zhu M, Hao H, Deng J, Li M, Sun Y, et al. Structure characterization of a novel polysaccharide from Chinese wild fruits (Passiflora foetida) and its immune-enhancing activity. Int J Biol Macromol. (2019) 136:324–31. doi: 10.1016/j.ijbiomac.2019.06.090
62. Xie J, Zou L, Xie Y, Wu X, Wang L. Analysis of the monosaccharide composition of water-soluble Coriolus versicolor polysaccharides by ultra-performance liquid chromatography/photodiode array detector. Chromatographia. (2021) 84:615–22. doi: 10.1007/s10337-021-04040-z
63. Zhang J, Wen C, Zhang H, Duan Y. Review of isolation, structural properties, chain conformation, and bioactivities of psyllium polysaccharides. Int J Biol Macromol. (2019) 139:409–20. doi: 10.1016/j.ijbiomac.2019.08.014
64. Borovkova VS, Malyar YN, Sudakova IG, Chudina AI, Zimonin DV, Skripnikov AM, et al. Composition and structure of aspen (Pópulus trémula) hemicelluloses obtained by oxidative delignification. Polymers. (2022) 14:4521. doi: 10.3390/polym14214521
65. Nai J, Zhang C, Shao H, Li B, Li H, Gao L, et al. Extraction, structure, pharmacological activities and drug carrier applications of Angelica sinensis polysaccharide. Int J Biol Macromol. (2021) 183:2337–53. doi: 10.1016/j.ijbiomac.2021.05.213
66. Hofmann J, Hahm HS, Seeberger PH, Pagel K. Identification of carbohydrate anomers using ion mobility-mass spectrometry. Nature. (2015) 526:241–4. doi: 10.1038/nature15388
67. Saimaiti M, Gençcelep H. Thermal and molecular characteristics of novel water-soluble polysaccharide from the Pteridium aquilinum and Dryopteris filix-mas. Food Chem Adv. (2023) 3:100401. doi: 10.1016/j.focha.2023.100401
68. Diener M, Adamcik J, Sanchez-Ferrer A, Jaedig F, Schefer L, Mezzenga R. Primary, secondary, tertiary and quaternary structure levels in linear polysaccharides: from random coil, to single helix to supramolecular assembly. Biomacromolecules. (2019) 20:1731–9. doi: 10.1021/acs.biomac.9b00087
69. Yang L, Zhang L. Chemical structural and chain conformational characterization of some bioactive polysaccharides isolated from natural sources. Carbohydr Polym. (2009) 76:349–61. doi: 10.1016/j.carbpol.2008.12.015
70. Meng Y, Lyu F, Xu X, Zhang L. Recent advances in chain conformation and bioactivities of triple-helix polysaccharides. Biomacromolecules. (2020) 21:1653–77. doi: 10.1021/acs.biomac.9b01644
71. Li S, Huang Y, Wang S, Xu X, Zhang L. Determination of the triple helical chain conformation of beta-glucan by facile and reliable triple-detector size exclusion chromatography. J Phys Chem B. (2014) 118:668–75. doi: 10.1021/jp4087199
72. Berglund J, Angles d’Ortoli T, Vilaplana F, Widmalm G, Bergenstrahle-Wohlert M, Lawoko M, et al. A molecular dynamics study of the effect of glycosidic linkage type in the hemicellulose backbone on the molecular chain flexibility. Plant J. (2016) 88:56–70. doi: 10.1111/tpj.13259
73. Gu W, Wang Y, Zeng L, Dong J, Bi Q, Yang X, et al. Polysaccharides from Polygonatum kingianum improve glucose and lipid metabolism in rats fed a high fat diet. Biomed Pharmacother. (2020) 125:109910. doi: 10.1016/j.biopha.2020.109910
74. Hu J, Pang W, Chen J, Bai S, Zheng Z, Wu X. Hypoglycemic effect of polysaccharides with different molecular weight of Pseudostellaria heterophylla. BMC Complement Altern Med. (2013) 13:267. doi: 10.1186/1472-6882-13-267
75. Cai L, Zou S, Liang D, Luan L. Structural characterization, antioxidant and hepatoprotective activities of polysaccharides from Sophorae tonkinensis radix. Carbohydr Polym. (2018) 184:354–65. doi: 10.1016/j.carbpol.2017.12.083
76. Xu W, Mohan A, Pitts NL, Udenigwe C, Mason B. Bile acid-binding capacity of lobster shell-derived chitin, chitosan and chitooligosaccharides. Food Biosci. (2020) 33:100476. doi: 10.1016/j.fbio.2019.100476
77. Li J, Fan L, Ding S. Isolation, purification and structure of a new water-soluble polysaccharide from Zizyphus jujuba cv. Jinsixiaozao. Carbohydr Polym. (2011) 83:477–82. doi: 10.1016/j.carbpol.2010.08.014
78. Zhu Y, Tian Y, Wang N, Chang Y, Xue C, Wang J. Structure-function relationship analysis of fucoidan from sea cucumber (Holothuria tubulosa) on ameliorating metabolic inflammation. J Food Biochem. (2021) 45:e13500. doi: 10.1111/jfbc.13500
79. Wang K, Wang J, Li Q, Zhang Q, You R, Cheng Y, et al. Structural differences and conformational characterization of five bioactive polysaccharides from Lentinus edodes. Food Res Int. (2014) 62:223–32. doi: 10.1016/j.foodres.2014.02.047
80. Ji X, Shen Y, Guo X. Isolation, structures, and bioactivities of the polysaccharides from Gynostemma pentaphyllum (Thunb.) makino: a review. Biomed Res Int. (2018) 2018:6285134. doi: 10.1155/2018/6285134
81. Cao JJ, Lv QQ, Zhang B, Chen HQ. Structural characterization and hepatoprotective activities of polysaccharides from the leaves of Toona sinensis (A. Juss) Roem. Carbohydr Polym. (2019) 212:89–101. doi: 10.1016/j.carbpol.2019.02.031
82. Guo X, Kang J, Xu Z, Guo Q, Zhang L, Ning H, et al. Triple-helix polysaccharides: formation mechanisms and analytical methods. Carbohydr Polym. (2021) 262:117962. doi: 10.1016/j.carbpol.2021.117962
83. Alkhudhayri DA, Osman MA, Alshammari GM, Al Maiman SA, Yahya MA. Moringa peregrina leaf extracts produce anti-obesity, hypoglycemic, anti-hyperlipidemic, and hepatoprotective effects on high-fat diet fed rats. Saudi J Biol Sci. (2021) 28:3333–42. doi: 10.1016/j.sjbs.2021.02.078
84. Li R, Xue Z, Li S, Zhou J, Liu J, Zhang M, et al. Mulberry leaf polysaccharides ameliorate obesity through activation of brown adipose tissue and modulation of the gut microbiota in high-fat diet fed mice. Food Funct. (2022) 13:561–73. doi: 10.1039/d1fo02324a
85. Zhao Z, Huangfu L, Dong L, Liu S. Functional groups and antioxidant activities of polysaccharides from five categories of tea. Ind Crops Prod. (2014) 58:31–5. doi: 10.1016/j.indcrop.2014.04.004
86. Zhang JQ, Li C, Huang Q, You LJ, Chen C, Fu X, et al. Comparative study on the physicochemical properties and bioactivities of polysaccharide fractions extracted from Fructus Mori at different temperatures. Food Funct. (2019) 10:410–21. doi: 10.1039/c8fo02190b
87. Liu W, Wang H, Yao W, Gao X, Yu LL. Effects of sulfation on the physicochemical and functional properties of a water-insoluble polysaccharide preparation from Ganoderma lucidum. J Agric Food Chem. (2010) 58:3336–41. doi: 10.1021/jf903395g
88. Gao Z, Zhang C, Tian W, Liu K, Hou R, Yue C, et al. The antioxidative and hepatoprotective effects comparison of Chinese angelica polysaccharide (CAP) and selenizing CAP (sCAP) in CCl4 induced hepatic injury mice. Int J Biol Macromol. (2017) 97:46–54. doi: 10.1016/j.ijbiomac.2017.01.013
89. Burton-Freeman B. Dietary fiber and energy regulation. J Nutr. (2000) 130(2S Suppl.):272S–5S. doi: 10.1093/jn/130.2.272S
90. Sasaki T, Kohyama K. Influence of non-starch polysaccharides on the in vitro digestibility and viscosity of starch suspensions. Food Chem. (2012) 133:1420–6. doi: 10.1016/j.foodchem.2012.02.029
91. Qi X, Al-Ghazzewi FH, Tester RF. Dietary fibre, gastric emptying and carbohydrate digestion: a mini-review. Starch Starke. (2018) 70:1700346. doi: 10.1002/star.201700346
92. Vahouny GV, Satchithanandam S, Chen I, Tepper SA, Kritchevsky D, Lightfoot FG, et al. Dietary fiber and intestinal adaptation: effects on lipid absorption and lymphatic transport in the rat. Am J Clin Nutr. (1988) 47:201–6. doi: 10.1093/ajcn/47.2.201
93. Chen L, Gao LX, Huang QH, Zhong RQ, Zhang LL, Tang XF, et al. Viscous and fermentable nonstarch polysaccharides affect intestinal nutrient and energy flow and hindgut fermentation in growing pigs. J Anim Sci. (2017) 95:5054–63. doi: 10.2527/jas2017.1662
94. Rolls BJ. Carbohydrates, fats, and satiety. Am J Clin Nutr. (1995) 61(4 Suppl.):960S–7S. doi: 10.1093/ajcn/61.4.960S
95. Yadav A, Kataria MA, Saini V, Yadav A. Role of leptin and adiponectin in insulin resistance. Clin Chim Acta. (2013) 417:80–4. doi: 10.1016/j.cca.2012.12.007
96. Yuan Y, Liu Q, Zhao F, Cao J, Shen X, Li C. Holothuria Leucospilota polysaccharides ameliorate hyperlipidemia in high-fat diet-induced rats via short-chain fatty acids production and lipid metabolism regulation. Int J Mol Sci. (2019) 20:4738. doi: 10.3390/ijms20194738
97. Xie SZ, Yang G, Jiang XM, Qin DY, Li QM, Zha XQ, et al. Polygonatum cyrtonema hua polysaccharide promotes GLP-1 secretion from enteroendocrine L-cells through sweet taste receptor-mediated cAMP signaling. J Agric Food Chem. (2020) 68:6864–72. doi: 10.1021/acs.jafc.0c02058
98. Yao Y, Yan L, Chen H, Wu N, Wang W, Wang D. Cyclocarya paliurus polysaccharides alleviate type 2 diabetic symptoms by modulating gut microbiota and short-chain fatty acids. Phytomedicine. (2020) 77:153268. doi: 10.1016/j.phymed.2020.153268
99. Kim YA, Keogh JB, Clifton PM. Probiotics, prebiotics, synbiotics and insulin sensitivity. Nutr Res Rev. (2018) 31:35–51. doi: 10.1017/S095442241700018X
100. Seyedan A, Alshawsh MA, Alshagga MA, Koosha S, Mohamed Z. Medicinal plants and their inhibitory activities against pancreatic lipase: a review. Evid Based Complement Alternat Med. (2015) 2015:973143. doi: 10.1155/2015/973143
101. Buchholz T, Melzig MF. Medicinal plants traditionally used for treatment of obesity and diabetes mellitus - screening for pancreatic lipase and alpha-amylase inhibition. Phytother Res. (2016) 30:260–6. doi: 10.1002/ptr.5525
102. Birari RB, Bhutani KK. Pancreatic lipase inhibitors from natural sources: unexplored potential. Drug Discov Today. (2007) 12:879–89. doi: 10.1016/j.drudis.2007.07.024
103. Huang YL, Chow CJ, Tsai YH. Composition, characteristics, and in-vitro physiological effects of the water-soluble polysaccharides from Cassia seed. Food Chem. (2012) 134:1967–72. doi: 10.1016/j.foodchem.2012.03.127
104. Zhao L, Qin Y, Guan R, Zheng W, Liu J, Zhao J. Digestibility of fucosylated glycosaminoglycan from sea cucumber and its effects on digestive enzymes under simulated salivary and gastrointestinal conditions. Carbohydr Polym. (2018) 186:217–25. doi: 10.1016/j.carbpol.2018.01.029
105. Zhang T, Wu S, Ai C, Wen C, Liu Z, Wang L, et al. Galactofucan from Laminaria japonica is not degraded by the human digestive system but inhibits pancreatic lipase and modifies the intestinal microbiota. Int J Biol Macromol. (2021) 166:611–20. doi: 10.1016/j.ijbiomac.2020.10.219
106. Hu JL, Nie SP, Li C, Xie MY. In vitro effects of a novel polysaccharide from the seeds of Plantago asiatica L. on intestinal function. Int J Biol Macromol. (2013) 54:264–9. doi: 10.1016/j.ijbiomac.2012.12.011
107. Bkhairia I, Krayem N, Dhibi S, Ghorbel S, Li S, Nasri M, et al. New Water Soluble Polysaccharide From Periploca Laevigata: Functional Characterization and Evaluation of Multi-biological Activities. Preprint (version 1). Research Square (2021). doi: 10.21203/rs.3.rs-635459/v1
108. Chiang JY, Ferrell JM. Bile acids as metabolic regulators and nutrient sensors. Annu Rev Nutr. (2019) 39:175–200. doi: 10.1146/annurev-nutr-082018-124344
109. Chávez-Talavera O, Tailleux A, Lefebvre P, Staels B. Bile acid control of metabolism and inflammation in obesity, type 2 diabetes, dyslipidemia and NAFLD Short title: bile acids in meta-inflammatory disorders. Gastroenterology. (2017) 152:1679–94. doi: 10.1053/j.gastro.2017.01.055
110. Yue Y, Yadav SK, Wang C, Zhao Y, Zhang X, Wu Z. Nonabsorbable polysaccharide-functionalized polyethylenimine for inhibiting lipid absorption. Carbohydr Polym. (2018) 197:57–65. doi: 10.1016/j.carbpol.2018.05.083
111. Shi L, Wang J, Wang Y, Feng Y. MDG-1, an Ophiopogon polysaccharide, alleviates hyperlipidemia in mice based on metabolic profile of bile acids. Carbohydr Polym. (2016) 150:74–81. doi: 10.1016/j.carbpol.2016.05.008
112. Roncari DA, Lau DC, Kindler S. Exaggerated replication in culture of adipocyte precursors from massively obese persons. Metabolism. (1981) 30:425–7. doi: 10.1016/0026-0495(81)90174-8
113. Parlee SD, Lentz SI, Mori H, MacDougald OA. Quantifying size and number of adipocytes in adipose tissue. Methods Enzymol. (2014) 537:93–122. doi: 10.1016/B978-0-12-411619-1.00006-9
114. Du H, Shi L, Wang Q, Yan T, Wang Y, Zhang X, et al. Fu brick tea polysaccharides prevent obesity via gut microbiota-controlled promotion of adipocyte browning and thermogenesis. J Agric Food Chem. (2022) 70:13893–903. doi: 10.1021/acs.jafc.2c04888
115. Luo J, Qi J, Wang W, Luo Z, Liu L, Zhang G, et al. Antiobesity effect of flaxseed polysaccharide via inducing satiety due to leptin resistance removal and promoting lipid metabolism through the AMP-Activated Protein Kinase (AMPK) signaling pathway. J Agric Food Chem. (2019) 67:7040–9. doi: 10.1021/acs.jafc.9b02434
116. Zhao A, Chen Y, Li Y, Lin D, Yang Z, Wang Q, et al. Sulfated polysaccharides from Enteromorpha prolifera attenuate lipid metabolism disorders in mice with obesity induced by a high-fat diet via a pathway dependent on AMP-activated protein kinase. J Nutr. (2022) 152:939–49. doi: 10.1093/jn/nxab432
117. Wang X, Liu H, Zhao L, Dong H, Ning C, Li X, et al. Effects of the crude polysaccharides from Poria cocos on the proliferation and differentiation of 3T3-L1 cells. Res J Biol Sci. (2011) 6:597–601. doi: 10.3923/rjbsci.2011.597.601
118. Xu X, Chen W, Yu S, Lei Q, Han L, Ma W. Inhibition of preadipocyte differentiation by Lycium barbarum polysaccharide treatment in 3T3-L1 cultures. Electron J Biotechnol. (2021) 50:53–8. doi: 10.1016/j.ejbt.2021.01.003
119. Chen G, Luo YC, Li BP, Li B, Guo Y, Li Y, et al. Effect of polysaccharide from Auricularia auricula on blood lipid metabolism and lipoprotein lipase activity of ICR mice fed a cholesterol-enriched diet. J Food Sci. (2008) 73:H103–8. doi: 10.1111/j.1750-3841.2008.00821.x
120. Mead JR, Irvine SA, Ramji DP. Lipoprotein lipase: structure, function, regulation, and role in disease. J Mol Med. (2002) 80:753–69. doi: 10.1007/s00109-002-0384-9
121. Goldberg IJ. Lipoprotein lipase and lipolysis: central roles in lipoprotein metabolism and atherogenesis. J Lipid Res. (1996) 37:693–707. doi: 10.1016/s0022-2275(20)37569-6
122. Yu CH, Dai XY, Chen Q, Zang JN, Deng LL, Liu YH, et al. Hypolipidemic and antioxidant activities of polysaccharides from Rosae Laevigatae Fructus in rats. Carbohydr Polym. (2013) 94:56–62. doi: 10.1016/j.carbpol.2013.01.006
123. Yang S, Meng Y, Yan J, Wang N, Xue Z, Zhang H, et al. Polysaccharide-enriched fraction from Amillariella Mellea fruiting body improves insulin resistance. Molecules. (2018) 24:46. doi: 10.3390/molecules24010046
124. Shon M, Kim G. Anti-obese function of polysaccharides derived from Korean ginseng (panax ginseng c.a. meyer) and development of functional food material in preventing obesity. Korea J Herbol. (2016) 31:71–7. doi: 10.6116/kjh.2016.31.4.71
125. Jia L, Li W, Li J, Li Y, Song H, Luan Y, et al. Lycium barbarum polysaccharide attenuates high-fat diet-induced hepatic steatosis by up-regulating SIRT1 expression and deacetylase activity. Sci Rep. (2016) 6:36209. doi: 10.1038/srep36209
126. Postic C, Girard J. Contribution of de novo fatty acid synthesis to hepatic steatosis and insulin resistance: lessons from genetically engineered mice. J Clin Invest. (2008) 118:829–38. doi: 10.1172/JCI34275
127. Wang CM, Yuan RS, Zhuang WY, Sun JH, Wu JY, Li H, et al. Schisandra polysaccharide inhibits hepatic lipid accumulation by downregulating expression of SREBPs in NAFLD mice. Lipids Health Dis. (2016) 15:195. doi: 10.1186/s12944-016-0358-5
128. Cox AJ, West NP, Cripps AW. Obesity, inflammation, and the gut microbiota. Lancet Diabetes Endocrinol. (2015) 3:207–15. doi: 10.1016/s2213-8587(14)70134-2
129. Koliada A, Syzenko G, Moseiko V, Budovska L, Puchkov K, Perederiy V, et al. Association between body mass index and Firmicutes/Bacteroidetes ratio in an adult Ukrainian population. BMC Microbiol. (2017) 17:120. doi: 10.1186/s12866-017-1027-1
130. Mariat D, Firmesse O, Levenez F, Guimaraes V, Sokol H, Dore J, et al. The Firmicutes/Bacteroidetes ratio of the human microbiota changes with age. BMC Microbiol. (2009) 9:123. doi: 10.1186/1471-2180-9-123
131. Ridaura VK, Faith JJ, Rey FE, Cheng J, Duncan AE, Kau AL, et al. Gut microbiota from twins discordant for obesity modulate metabolism in mice. Science. (2013) 341:1241214. doi: 10.1126/science.1241214
132. Liu Z, Chen Z, Guo H, He D, Zhao H, Wang Z, et al. The modulatory effect of infusions of green tea, oolong tea, and black tea on gut microbiota in high-fat-induced obese mice. Food Funct. (2016) 7:4869–79. doi: 10.1039/c6fo01439a
133. Zhong Y, Nyman M, Fak F. Modulation of gut microbiota in rats fed high-fat diets by processing whole-grain barley to barley malt. Mol Nutr Food Res. (2015) 59:2066–76. doi: 10.1002/mnfr.201500187
134. Zhu Z, Zhu B, Sun Y, Ai C, Wang L, Wen C, et al. Sulfated polysaccharide from sea cucumber and its depolymerized derivative prevent obesity in association with modification of gut microbiota in high-fat diet-fed mice. Mol Nutr Food Res. (2018) 62:1800446. doi: 10.1002/mnfr.201800446
135. Burcelin R, Courtney M, Amar J. Gut microbiota and metabolic diseases: from pathogenesis to therapeutic perspective. In: Kochhar S, Martin F editors. Metabonomics and Gut Microbiota in Nutrition and Disease. Molecular and Integrative Toxicology. London: Springer (2015). p. 199–234. doi: 10.1007/978-1-4471-6539-2_11
136. Lai, X, Chow SH, Le Brun AP, Muir BW, Bergen PJ, White J, et al. Polysaccharide-targeting lipid nanoparticles to kill gram-negative bacteria. Small (2023) 2305052:1–13. doi: 10.1002/smll.202305052
137. Li QM, Zha XQ, Zhang WN, Liu J, Pan LH, Luo JP. Laminaria japonica polysaccharide prevents high-fat-diet-induced insulin resistance in mice via regulating gut microbiota. Food Funct. (2021) 12:5260–73. doi: 10.1039/d0fo02100h
138. Zhou K. Strategies to promote abundance of Akkermansia muciniphila, an emerging probiotics in the gut, evidence from dietary intervention studies. J Funct Foods. (2017) 33:194–201. doi: 10.1016/j.jff.2017.03.045
139. Dao MC, Everard A, Aron-Wisnewsky J, Sokolovska N, Prifti E, Verger EO, et al. Akkermansia muciniphila and improved metabolic health during a dietary intervention in obesity: relationship with gut microbiome richness and ecology. Gut. (2016) 65:426–36. doi: 10.1136/gutjnl-2014-308778
140. Anhe FF, Roy D, Pilon G, Dudonne S, Matamoros S, Varin TV, et al. A polyphenol-rich cranberry extract protects from diet-induced obesity, insulin resistance and intestinal inflammation in association with increased Akkermansia spp. population in the gut microbiota of mice. Gut. (2015) 64:872–83. doi: 10.1136/gutjnl-2014-307142
141. Shi LL, Li Y, Wang Y, Feng Y. MDG-1, an Ophiopogon polysaccharide, regulate gut microbiota in high-fat diet-induced obese C57BL/6 mice. Int J Biol Macromol. (2015) 81:576–83. doi: 10.1016/j.ijbiomac.2015.08.057
142. Ang Z, Ding JL. GPR41 and GPR43 in obesity and inflammation - protective or causative? Front Immunol. (2016) 7:28. doi: 10.3389/fimmu.2016.00028
143. Kimura I. Host energy regulation via SCFAs receptors, as dietary nutrition sensors, by gut microbiota. Pharm Soc Japan. (2014) 134:1037–42. doi: 10.1248/yakushi.14-00169
144. Lu Y, Fan C, Li P, Lu Y, Chang X, Qi K. Short chain fatty acids prevent high-fat-diet-induced obesity in mice by regulating g protein-coupled receptors and gut microbiota. Sci Rep. (2016) 6:37589. doi: 10.1038/srep37589
145. Le Poul E, Loison C, Struyf S, Springael JY, Lannoy V, Decobecq ME, et al. Functional characterization of human receptors for short chain fatty acids and their role in polymorphonuclear cell activation. J Biol Chem. (2003) 278:25481–9. doi: 10.1074/jbc.M301403200
146. Kimura I, Ozawa K, Inoue D, Imamura T, Kimura K, Maeda T, et al. The gut microbiota suppresses insulin-mediated fat accumulation via the short-chain fatty acid receptor GPR43. Nat Commun. (2013) 4:1829. doi: 10.1038/ncomms2852
147. Hong Y, Sheng L, Zhong J, Tao X, Zhu W, Ma J, et al. Desulfovibrio vulgaris, a potent acetic acid-producing bacterium, attenuates nonalcoholic fatty liver disease in mice. Gut Microbes. (2021) 13:1–20. doi: 10.1080/19490976.2021.1930874
148. Szliszka E, Czuba ZP, Domino M, Mazur B, Zydowicz G, Krol W. Ethanolic extract of propolis (EEP) enhances the apoptosis-inducing potential of TRAIL in cancer cells. Molecules. (2009) 14:738–54. doi: 10.3390/molecules
149. De Vadder F, Kovatcheva-Datchary P, Goncalves D, Vinera J, Zitoun C, Duchampt A, et al. Microbiota-generated metabolites promote metabolic benefits via gut-brain neural circuits. Cell. (2014) 156:84–96. doi: 10.1016/j.cell.2013.12.016
150. Yoshida H, Ishii M, Akagawa M. Propionate suppresses hepatic gluconeogenesis via GPR43/AMPK signaling pathway. Arch Biochem Biophys. (2019) 672:108057. doi: 10.1016/j.abb.2019.07.022
151. Agus A, Clement K, Sokol H. Gut microbiota-derived metabolites as central regulators in metabolic disorders. Gut. (2021) 70:1174–82. doi: 10.1136/gutjnl-2020-323071
152. Harris K, Kassis A, Major G, Chou C. Is the gut microbiota a new factor contributing to obesity and its metabolic disorders? J Obes. (2012) 2012:879151. doi: 10.1155/2012/879151
153. Dehghan P, Farhangi MA, Nikniaz L, Nikniaz Z, Asghari-Jafarabadi M. Gut microbiota-derived metabolite trimethylamine N-oxide (TMAO) potentially increases the risk of obesity in adults: an exploratory systematic review and dose-response meta- analysis. Obes Rev. (2020) 21:e12993. doi: 10.1111/obr.12993
154. Chen M, Lu B, Li Y, Wang Y, Zheng H, Zhong D, et al. Metabolomics insights into the modulatory effects of long-term compound polysaccharide intake in high-fat diet-induced obese rats. Nutr Metab. (2018) 15:8. doi: 10.1186/s12986-018-0246-2
155. Zhang Z, Liu H, Yu B, Tao H, Li J, Wu Z, et al. Lycium barbarum polysaccharide attenuates myocardial injury in high-fat diet-fed mice through manipulating the gut microbiome and fecal metabolome. Food Res Int. (2020) 138(Pt. B):109778. doi: 10.1016/j.foodres.2020.109778
156. Lavelle A, Sokol H. Gut microbiota-derived metabolites as key actors in inflammatory bowel disease. Nat Rev Gastroenterol Hepatol. (2020) 17:223–37. doi: 10.1038/s41575-019-0258-z
157. Thomas C, Gioiello A, Noriega L, Strehle A, Oury J, Rizzo G, et al. TGR5-mediated bile acid sensing controls glucose homeostasis. Cell Metab. (2009) 10:167–77. doi: 10.1016/j.cmet.2009.08.001
158. Wang T, Han J, Dai H, Sun J, Ren J, Wang W, et al. Polysaccharides from Lyophyllum decastes reduce obesity by altering gut microbiota and increasing energy expenditure. Carbohydr Polym. (2022) 295:119862. doi: 10.1016/j.carbpol.2022.119862
159. Jiang C, Xie C, Lv Y, Li J, Krausz K, Shi J, et al. Intestine-selective farnesoid X receptor inhibition improves obesity-related metabolic dysfunction. Nat Commun. (2015) 6:10166. doi: 10.1038/ncomms10166
160. Huang S, Pang D, Li X, You L, Zhao Z, Cheung PC, et al. A sulfated polysaccharide from Gracilaria Lemaneiformis regulates cholesterol and bile acid metabolism in high-fat diet mice. Food Funct. (2019) 10:3224–36. doi: 10.1039/c9fo00263d
161. Kanwal S, Joseph TP, Owusu L, Xiaomeng R, Meiqi L, Yi X. A polysaccharide isolated from Dictyophora indusiata promotes recovery from antibiotic-driven intestinal dysbiosis and improves gut epithelial barrier function in a mouse model. Nutrients. (2018) 10:1003. doi: 10.3390/nu10081003
162. Kanwal S, Aliya S, Xin Y. Anti-obesity effect of Dictyophora indusiata Mushroom Polysaccharide (DIP) in high fat diet-induced obesity via regulating inflammatory cascades and intestinal microbiome. Front Endocrinol. (2020) 11:558874. doi: 10.3389/fendo.2020.558874
163. Regnier M, Rastelli M, Morissette A, Suriano F, Le Roy T, Pilon G, et al. Rhubarb supplementation prevents diet-induced obesity and diabetes in association with increased Akkermansia muciniphila in mice. Nutrients. (2020) 12:2932. doi: 10.3390/nu12102932
164. Mehta NN, McGillicuddy FC, Anderson PD, Hinkle CC, Shah R, Pruscino L, et al. Experimental endotoxemia induces adipose inflammation and insulin resistance in humans. Diabetes. (2010) 59:172–81. doi: 10.2337/db09-0367
165. Zhang T, Yang Y, Liang Y, Jiao X, Zhao C. Beneficial effect of intestinal fermentation of natural polysaccharides. Nutrients. (2018) 10:1055. doi: 10.3390/nu10081055
166. Luo D, Dong X, Huang J, Huang C, Fang G, Huang Y. Pueraria lobata root polysaccharide alleviates glucose and lipid metabolic dysfunction in diabetic db/db mice. Pharm Biol. (2021) 59:382–90. doi: 10.1080/13880209.2021.1898648
167. Dong Z, Zhang M, Li H, Zhan Q, Lai F, Wu H. Structural characterization and immunomodulatory activity of a novel polysaccharide from Pueraria lobata (Willd.) Ohwi root. Int J Biol Macromol. (2020) 154:1556–64. doi: 10.1016/j.ijbiomac.2019.11.040
168. Lin CL, Wang CC, Chang SC, Inbaraj BS, Chen BH. Antioxidative activity of polysaccharide fractions isolated from Lycium barbarum Linnaeus. Int J Biol Macromol. (2009) 45:146–51. doi: 10.1016/j.ijbiomac.2009.04.014
169. Yang Y, Li W, Li Y, Wang Q, Gao L, Zhao J. Dietary Lycium barbarum polysaccharide induces Nrf2/ARE pathway and ameliorates insulin resistance induced by high-fat via activation of PI3K/AKT signaling. Oxid Med Cell Longev. (2014) 2014:145641. doi: 10.1155/2014/145641
170. Wu S, Zhang X, Liu J, Song J, Yu P, Chen P, et al. Physicochemical characterization of Sargassum fusiforme fucoidan fractions and their antagonistic effect against P-selectin-mediated cell adhesion. Int J Biol Macromol. (2019) 133:656–62. doi: 10.1016/j.ijbiomac.2019.03.218
171. Wang LY, Wang Y, Xu DS, Ruan KF, Feng Y, Wang S. MDG-1, a polysaccharide from Ophiopogon japonicus exerts hypoglycemic effects through the PI3K/Akt pathway in a diabetic KKAy mouse model. J Ethnopharmacol. (2012) 143:347–54. doi: 10.1016/j.jep.2012.06.050
172. Lee JS, Synytsya A, Kim HB, Choi DJ, Lee S, Lee J, et al. Purification, characterization and immunomodulating activity of a pectic polysaccharide isolated from Korean mulberry fruit Oddi (Morus alba L.). Int Immunopharmacol. (2013) 17:858–66. doi: 10.1016/j.intimp.2013.09.019
173. Cheng Z, Hu M, Tao J, Yang H, Yan P, An G, et al. The protective effects of Chinese yam polysaccharide against obesity-induced insulin resistance. J Funct Foods. (2019) 55:238–47. doi: 10.1016/j.jff.2019.02.023
174. Wang K, Wang H, Liu Y, Shui W, Wang J, Cao P, et al. Dendrobium officinale polysaccharide attenuates type 2 diabetes mellitus via the regulation of PI3K/Akt-mediated glycogen synthesis and glucose metabolism. J Funct Foods. (2018) 40:261–71. doi: 10.1016/j.jff.2017.11.004
175. Lee OH, Kim KI, Han CK, Kim YC, Hong HD. Effects of acidic polysaccharides from Gastrodia Rhizome on systolic blood pressure and serum lipid concentrations in spontaneously hypertensive rats fed a high-fat diet. Int J Mol Sci. (2012) 13:698–709. doi: 10.3390/ijms13010698
176. Yan Z, Fan R, Yin S, Zhao X, Liu J, Li L, et al. Protective effects of Ginkgo biloba leaf polysaccharide on nonalcoholic fatty liver disease and its mechanisms. Int J Biol Macromol. (2015) 80:573–80. doi: 10.1016/j.ijbiomac.2015.05.054
177. Liao W, Luo Z, Liu D, Ning Z, Yang J, Ren J. Structure characterization of a novel polysaccharide from Dictyophora indusiata and its macrophage immunomodulatory activities. J Agric Food Chem. (2015) 63:535–44. doi: 10.1021/jf504677r
178. Hong Y, Li B, Zheng N, Wu G, Ma J, Tao X, et al. Integrated metagenomic and metabolomic analyses of the effect of Astragalus polysaccharides on alleviating high-fat diet-induced metabolic disorders. Front Pharmacol. (2020) 11:833. doi: 10.3389/fphar.2020.00833
179. Liang J, Chen S, Chen J, Lin J, Xiong Q, Yang Y, et al. Therapeutic roles of polysaccharides from Dendrobium Officinaleon colitis and its underlying mechanisms. Carbohydr Polym. (2018) 185:159–68. doi: 10.1016/j.carbpol.2018.01.013
Keywords: obesity, traditional Chinese medicine, polysaccharides, structural-property, antiobesity mechanism
Citation: Zhi N, Chang X, Wang X, Guo J, Chen J and Gui S (2024) Recent advances in the extraction, purification, structural-property correlations, and antiobesity mechanism of traditional Chinese medicine-derived polysaccharides: a review. Front. Nutr. 10:1341583. doi: 10.3389/fnut.2023.1341583
Received: 20 November 2023; Accepted: 27 December 2023;
Published: 17 January 2024.
Edited by:
Zhaojun Wei, Hefei University of Technology, ChinaReviewed by:
Aimei Liao, Henan University of Technology, ChinaCopyright © 2024 Zhi, Chang, Wang, Guo, Chen and Gui. This is an open-access article distributed under the terms of the Creative Commons Attribution License (CC BY). The use, distribution or reproduction in other forums is permitted, provided the original author(s) and the copyright owner(s) are credited and that the original publication in this journal is cited, in accordance with accepted academic practice. No use, distribution or reproduction is permitted which does not comply with these terms.
*Correspondence: Xiangwei Chang, Y2h4d2luZ0AxNjMuY29t; Shuangying Gui, Z3Vpc2h5MDUyMEAxMjYuY29t
Disclaimer: All claims expressed in this article are solely those of the authors and do not necessarily represent those of their affiliated organizations, or those of the publisher, the editors and the reviewers. Any product that may be evaluated in this article or claim that may be made by its manufacturer is not guaranteed or endorsed by the publisher.
Research integrity at Frontiers
Learn more about the work of our research integrity team to safeguard the quality of each article we publish.