- 1Department of Endocrinology, Shandong Provincial Hospital & Medical Integration and Practice Center, Shandong University, Jinan, Shandong, China
- 2Key Laboratory of Endocrine Glucose & Lipids Metabolism and Brain Aging, Ministry of Education, Shandong Provincial Hospital Affiliated to Shandong First Medical University, Jinan, Shandong, China
- 3Shandong Key Laboratory of Endocrinology and Lipid Metabolism, Shandong Institute of Endocrine and Metabolic Diseases, Shandong Clinical Research Center of Diabetes and Metabolic Diseases, Shandong Prevention and Control Engineering Laboratory of Endocrine and Metabolic Diseases, Shandong Provincial Hospital Affiliated to Shandong First Medical University, Jinan, Shandong, China
- 4Key Laboratory of Cardiovascular Remodeling and Function Research, Chinese Ministry of Education, Chinese National Health Commission and Chinese Academy of Medical Sciences, The State and Shandong Province Joint Key Laboratory of Translational Cardiovascular Medicine, Department of Cardiology, Qilu Hospital, Cheeloo College of Medicine, Shandong University, Jinan, Shandong, China
- 5School of Information Management, Nanjing University, Nanjing, Jiangsu, China
- 6Advanced Medical Research Institute, Cheeloo College of Medicine, Shandong University, Jinan, Shandong, China
Background: Dysregulation of feeding behavior leads to a variety of pathological manifestations ranging from obesity to anorexia. The foraging behavior of animals affected by food deficiency is not fully understood.
Methods: Home-Cage system was used to monitor the behaviors. Immunohistochemical staining was used to monitor the trend of neuronal activity. Chemogenetic approach was used to modify neuronal activity.
Results: We described here a unique mouse model of foraging behavior and unveiled that food deprivation significantly increases the general activities of mice with a daily rhythmic pattern, particularly foraging behavior. The increased foraging behavior is potentiated by food cues (mouthfeel, odor, size, and shape) and energy deficit, rather than macronutrient protein, carbohydrate, and fat. Notably, energy deficiency increases nocturnal neuronal activity in paraventricular hypothalamic nucleus (PVH), accompanying a similar change in rhythmic foraging behavior. Activating neuronal activity in PVH enhances the amplitude of foraging behavior in mice. Conversely, inactivating neuronal activity in PVH decreases the amplitude of foraging behavior and impairs the rhythm of foraging behavior.
Discussion: These results illustrate that energy status and food cues regulate the rhythmic foraging behavior via PVH neuronal activity. Understanding foraging behavior provides insights into the underlying mechanism of eating-related disorders.
Introduction
Free-eating animals exhibit a strong circadian rhythm in their feeding behavior, particularly foraging behavior (1). Foraging behavior is a fundamental physiological activity of animal survival for obtaining necessary energy. During the active phase, restricting food increases the general activities of mice, particularly wakefulness and foraging behavior (2). Consistently, brain regions associated with reward and motivation are activated during the status of energy deficit, which enhances the salience of food-related stimuli and increases the motivation to forage (3, 4).
Energy shortage is customarily recognized as the main cause of food deprivation-promoted foraging behavior. Energy mainly derives from three types of nutrients in food: carbohydrate, protein, and fat, which have been associated with the regulation of animal appetite and food intake (5). Increasing evidence has shown that food nutrients regulate animal foraging behavior. For example, high-protein and high-carbohydrate foods increased foraging behavior in herbivorous mice (6). In contrast, high-protein and high-carbohydrate foods decrease foraging behavior in omnivorous and carnivorous mice (6), and chimpanzees prefer to forage for leaves with a high carbohydrate content (7). Furthermore, foods rich in fat are more likely to increase foraging behavior in rodents (8). In addition, food cues, such as olfactory, visual signals, and mouthfeel, can increase the eating-related behavior of mice even in the absence of energy depletion (4, 9). For example, high food density reduces foraging behavior (10) and the sense of smell improves foraging efficiency (11). However, it is unknown the roles of food cues and macronutrients in the increased foraging behavior elicited by food deprivation.
The periventricular hypothalamus (PVH) is a vital brain region involved in regulating appetite and eating behavior. Appetite-related neuropeptide Y (NPY) injection to PVH decreases foraging behavior but increases hoarding and food intake (12). Moreover, melanocortin 4 receptor (MC4R)-expressing neurons in PVH regulate feeding behavior (13–16), as evidenced by food deprivation increases the activity of PVHMC4R neurons via inhibiting GABA release (17). In addition, different subpopulations of neurons in PVH control distinguishing aspects of energy balance. For example, MC4R neurons in PVH modulate the satiety by projecting to the hindbrain (18, 19). Neuronal nitric oxide synthase 1 (Nos1) neurons in PVH control both feeding behavior and energy expenditure (20). In contrast, parvocellular OXT neurons are a subpopulation of the PVHNos1 field, which modestly participates in the control of energy expenditure, rather than feeding (21, 22). Taken together, the periventricular hypothalamus acts as a central orchestrator in the intricate process of eating behavior regulation.
In this paper, to investigate the nature of the foraging behavior of caged mice, we use the Home-Cage system to monitor the fine behaviors of fasted mice in real-time and disclose the intrinsic neural regulatory mechanisms.
Materials and methods
Animals
Eight weeks C57BL/6 J male mice (Charles River Laboratory, Beijing, China) were kept under standard conditions with temperature (22 ± 1°C) and humidity (~40%) in a 12 h light/12 h dark cycle, free access to food and water. Mice were used for experiments at the age of 10–12 weeks. All of mice subjected to invasive experiments were used once. All animal experiments were permitted by the Institutional Animal Care and Use Committee at Shandong Provincial Hospital and complied with the China National Regulations on the Administration of Experimental or Laboratory Animals (No.2, 20,170,301, SSTC, China), and the ARRIVE guidelines or the U.K. Animals (Scientific Procedures) Act, 1986.
Gel diet
The gel diets were purchased from Jiangsu Xietong (Nanjing, China). Experiments were performed after 2 days of acclimation with full-energy gel. The ingredients of different gels were listed as follows: see Table 1.
Home-cage monitoring
Mice were kept in individual cages for home behavior monitoring and analysis by Home-Cage system (Shanghai Vanbi Intelligent Technology Co., Ltd.) on a 12 h light/12 h dark cycle and an ambient temperature at 24 ± 2°C in a silent room, with fluorescent light to simulate daytime and light off to simulate night, without disrupting the normal circadian rhythm of the mice. Mice were monitored by an infrared camera (Shanghai Vanbi Intelligent Technology Co., Ltd.) in the Home-Cage box mounted horizontally on the side of the cage for 24 h. The videos captured by these cameras are stored in a video processor connected to the computer. Video data were analyzed by Tracking Master V3.1.56 software (Shanghai Vanbi Intelligent Technology Co., Ltd.), and behavioral definitions were as described previously (23). Tracking Master V3.1.56 software monitored parameters as follows: contour erosin (2 pixels), contour expansion (2 pixels), animal size (500–10,000 pixels), motionless (0–4 cm/s), active (> = 4 cm/s), slow active (4–10 cm/s), fast active (> = 20 cm/s), move between two frames (1–10,000 pixels).
For the detection of home behavior in mice fed with NEG, FEG, PFG, FFG, and CFG, 2 days of FEG feeding habituation were conducted before Home-Cage monitoring. For the detection of home behaviors in virus-injected mice, 3 weeks after virus injection, a single intraperitoneal injection of clozapine-n-oxide (CNO, 1 mg/kg) was performed 5 min before the start of the experiment, and Home-Cage monitoring was performed 5 min later.
Energy intake
Energy intake in mice was performed with the Home-Cage experiment simultaneously. The weight of gels (before) was recorded at the start of and the end of the 24 h monitoring (after), respectively. Energy intake = (before-after) * 0.996/body weight. 0.996 is calories per gram of gels.
Immunohistochemical staining
Deeply anesthetized mice were perfused with 4% paraformaldehyde, and the brains were removed to the same fixative for 0–14 h. Using an automatic vibrating slicer (Leica, VT1200S), 30-um-thick brain sections were collected. Brain sections were incubated with c-fos antibody (1:500, Abcam, ab214672) and visualized with the 3,3′-diaminobenzidine (DAB) chromogen kit (DAB kit, Zhongshan Golden Bridge Bio-technology Co.Ltd). Images were acquired by positive microscope (Karl Zeiss A2, Germany). C-fos positive cells were counted in DMH (Bregma −1.70 mm), PVH (Bregma −0.94 mm), ARC (Bregma −1.70 mm), POA (Bregma 0.5 mm), VMH (Bregma −1.70 mm), LH (Bregma −1.70 mm) and SCN (Bregma −0.46 mm) by manual counting. The area (mm2) of each region of hypothalamus was calculated by image J. The density of c-fos positive cells = the number of c-fos positive cells / the area.
Viruses information and stereotaxic surgeries
Modulation of neuronal activity using Designer Receptors Exclusively Activated by Designer Drugs (DREADDs), a technology that allows for repeated activation of engineered receptors by systemic injection of otherwise inert ligand clozapine N-oxide (CNO) (24). By adeno-associated virus (AAV) as vectors, the inhibitory hM4D(Gi) DREADDs (pAAV2/9-hSyn-hM4D(Gi)-mCherry-WPRE, ObiO, AG50475, 1E+ 12 GC/ml) was used to inhibit neuronal activity, the activated DREADDs hM3D(Gq) (pAAV2/9-hSyn-hM3D(Gq)-EGFP-WPRE, ObiO, H6568, 1E+ 12 GC/ml) were used to activate neural activity. The empty control vector was pAAV-SYN-MCS-EYFP-3FLAG (AOV067, 1E+ 12 GC/ml). These AAVs were purchased from ObiO Technology (Shanghai, China). Mice received intraperitoneal injections of CNO (1 mg/kg, Sigma, C0832-5MG) to activate DREADD receptors. The dosage was chosen based on prior work showing that 1 mg/kg is an effective dose (25).
Viruses were stereotaxically infused into PVH as previous report (26), mice were placed on a stereotaxic alignment system (1900, David Kopf Instruments), and anesthetization by 2% isoflurane was maintained during surgeries. The coordinate for AAV injection (0.3ul/side) about bregma according to Mouse Brain Atlas (George Paxinos and Keith B. J. Franklin, second edition) was AP −0.82 mm, ML ±0.2 mm, DV −4.75 mm (PVH). Only animals with the correct injection site were included in the study.
Statistical analysis
Data were represented as mean ± SEM. An unpaired t-test was used to compare the means of two samples. One-way analysis of variance (ANOVA) was used to analyze and compare of means of three or more samples. Scheirer-Ray-Hare test was used to analyze sequential measurements. p < 0.05 was considered statistically significant. ANCOVA was performed with SPSS Statistics version 26 (IBM Corp.), the Scheirer-Ray-Hare test was performed with R software version 4.3.1, and the unpaired t-test was performed with Prism 9.0 (GraphPad Software Inc.).
Results
Free-feeding rodents exhibit strongly self-sustained properties in general activity with daily rhythms (27). To analyze mouse activities during the fasting period, we utilized Home-Cage system to monitor mice behaviors in real-time for 24 h under fasting or free-feeding conditions, respectively (Figure 1A). The mice were ad libitum access to water during the fasting experiment. Consistent with previous literature (28, 29), the total locomotor distance (Figures 1B–D) and general activity (Figures 1E–G) during the whole night were significantly increased in fasted mice as compared to fed mice. The rearing (Figure 1H and Supplementary Figures S1A,B) and sniffing (Figure 1I and Supplementary Figures S1C,D) behaviors of fasted mice were unaltered, even though sniffing and rearing were higher than fed mice at several time points (Figures 1H,I). Total grooming (Figure 1J and Supplementary Figures S1E,F), and digging (Figure 1K and Supplementary Figures S1G,H) were decreased. In contrast, hanging behavior was dramatically increased (Figures 1L–N) similar to total distance and general activity (Figures 1B–G). Considering the increased foraging behavior of mice under food deficiency (4, 30), we disclose that the hanging behavior of cage mice represents the foraging behavior.
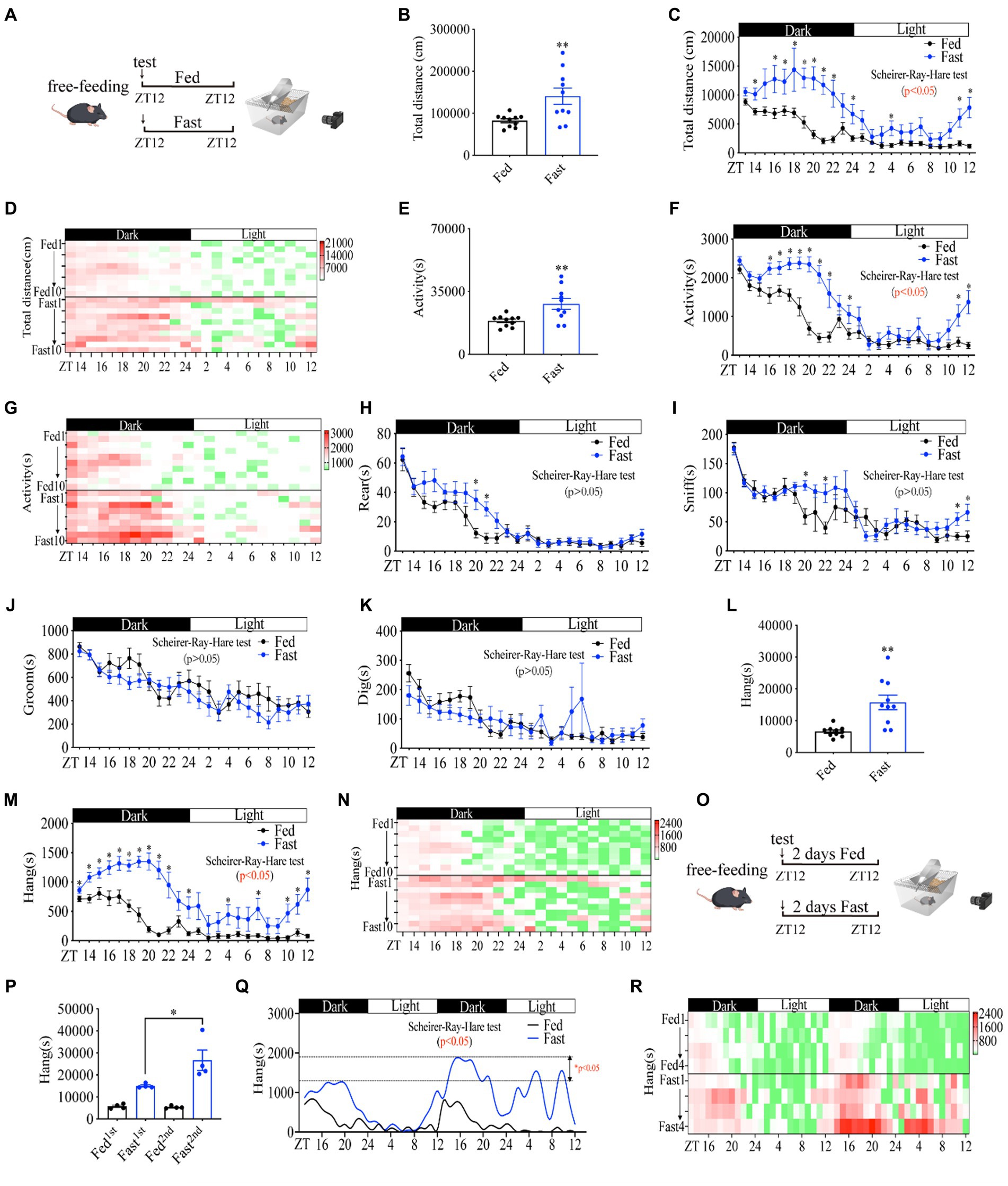
Figure 1. Food deprivation increases mouse foraging behavior in a rhythmic manner. (A) A diagram of experimental scheme (10 weeks C57BL/6 J male mice fed with chow diet or fasting, n = 10:10). (B) Total distance in 24 h. (C) Distance curve over 24 h. (D) Distance/h in 24 h for each mouse. (E) Total time of activity in 24 h. (F) Activity curve over 24 h. (G) Activity/h in 24 h for each mouse. (H) Rearing curve over 24 h. (I) Sniffing curve over 24 h. (J) Grooming curve over 24 h. (K) Digging curve over 24 h. (L) Total time of hanging in 24 h. (M) Hanging time curve over 24 h. (N) Hanging time/h in 24 h for each mouse. (O) A diagram of experimental scheme (10 weeks C57BL/6 J male mice fed with chow diet or fasting, n = 4:4). (P) Total time of hanging in 2 days. (Q) Hanging time curve over 2 days. (R) Hanging/h in 2 days for each mouse. Data are represented as mean ± SEM, *p < 0.05, **p < 0.01. Data in panels B–L were analyzed using an unpaired t-test, data in panel P was analyzed using One-way ANOVA, and other data were analyzed using the Scheirer-Ray-Hare test. ZT, zeitgeber time.
To examine whether foraging behavior has the property of self-sustained rhythm, we performed a real-time monitor of fasted mice behaviors for 48 h (Figure 1O). Notably, the lowest amplitude of foraging behavior in fed mice was always observed in the daytime at the daily ZT2-8 and the highest amplitude of foraging behavior appeared in the night from ZT12-14, revealing an intrinsic oscillator in regulating foraging behavior (Figures 1Q,R). Mice in the second 24 h of fasting displayed a dramatically higher amplitude of foraging behavior than that of mice in the first 24 h of fasting (Figure 1P). In particular, the increase in amplitude in the second daytime of fasting was extremely higher than that of the first daytime (Figures 1Q,R), suggesting a critical regulatory role of fasting in foraging behavior intensity. Together, these data demonstrate that hanging behavior is a self-sustained daily rhythmic activity and is primarily regulated by fasting or hunger signals, which reflects the properties of foraging behavior.
Food cues (mouthfeel, odor, size, and shape) are associated with the rewarding system, which is involved in the modulation of foraging behavior (31, 32). To examine whether the absence of food cues by food deprivation affects the increase in foraging behavior, we replaced the chow diet with gel diet when mice behaviors were monitored, all of mice were adapted to full energy gel (FEG) for another 2 days before this experiment (Figure 2A). As expected, none energy gel (NEG) dramatically induced a higher amplitude of distance (Supplementary Figures S2A–C), activity time (Supplementary Figures S2D–F) and foraging behavior (Figures 2B–D) than FEG did. In contrast, other behaviors of mice were not significantly altered (Supplementary Figures S2G–R), indicating a specific role of energy deficiency in foraging behavior. Of note, compared with fasted mice (Supplementary Figures S1E–H), mice fed with NEG showed unaltered grooming and digging behaviors (Supplementary Figures S2M–R), suggesting food cues were involved in regulating grooming and digging behaviors.
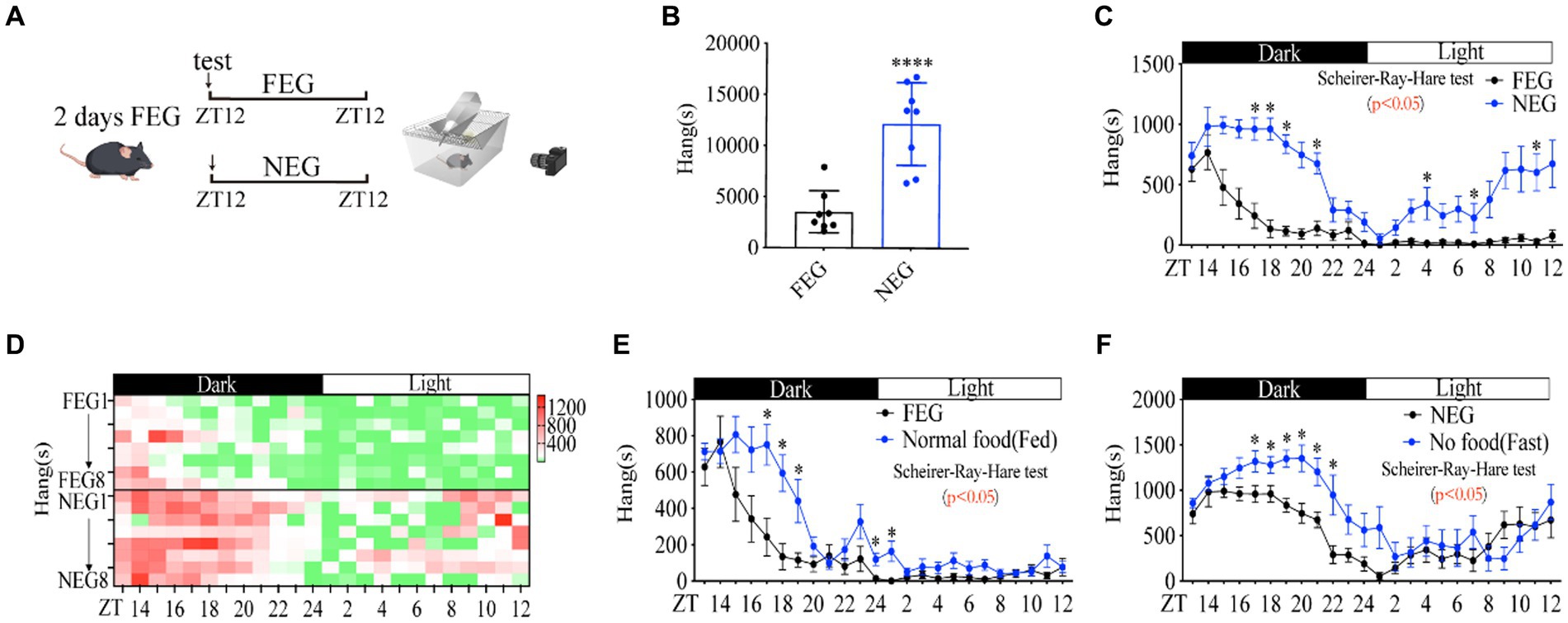
Figure 2. Food cues increase the amplitude of rhythmic foraging behavior. (A) A diagram of experimental scheme (12 weeks C57BL/6 J male mice fed with FEG or NEG, n = 8:8). (B) Total time of hanging in 24 h. (C) Hanging time curve over 24 h. (D) Hanging time/h in 24 h for each mouse. (E) Comparison of foraging behavior between FEG from C and normal diet from Figure 1M (n = 8:10). (F) Comparison of foraging behavior between NEG from C and fasted from Figure 1M (n = 8:10). Data are represented as mean ± SEM, *p < 0.05, ****p < 0.0001. Data in panel B were analyzed using an unpaired t-test and other data were analyzed using the Scheirer-Ray-Hare test. FEG, full-energy gel; NEG, non-energy gel; ZT, zeitgeber time.
Notably, comparing the data between Figures 1M and Figures 2C, we found that FEG led to a lower amplitude of foraging behavior than normal chow diet (Figure 2E), and NEG also reduced the amplitude of foraging behavior as compared to fasting by food deprivation (Figure 2F), elucidating that food cues (mouthfeel, odor, size, and shape) are involved in promoting rhythmic foraging behavior.
The calorie contents are potent natural reward and conditioning stimulus signals in the rewarding system (33). Indeed, fasting significantly increased the amplitude of foraging behavior, and subsequent refeeding after 5 h of fasting (Figure 3A) terminated the increase in distance (Supplementary Figures S3A–C), activity time (Supplementary Figures S3D–F) and foraging behavior of mice (Figures 3B–D). In contrast, rearing (Supplementary Figures S3E–G), sniffing (Supplementary Figures S3H–J), and grooming (Supplementary Figures S3K–M) in refed mice were not significantly altered compared to these in fed and fasted mice. Notably, refed mice exhibited reduced digging behavior compared to fed mice (Supplementary Figures S3N,O), which is consistent with the reduced digging behavior under food deprivation conditions (Supplementary Figures S1G, S3P).
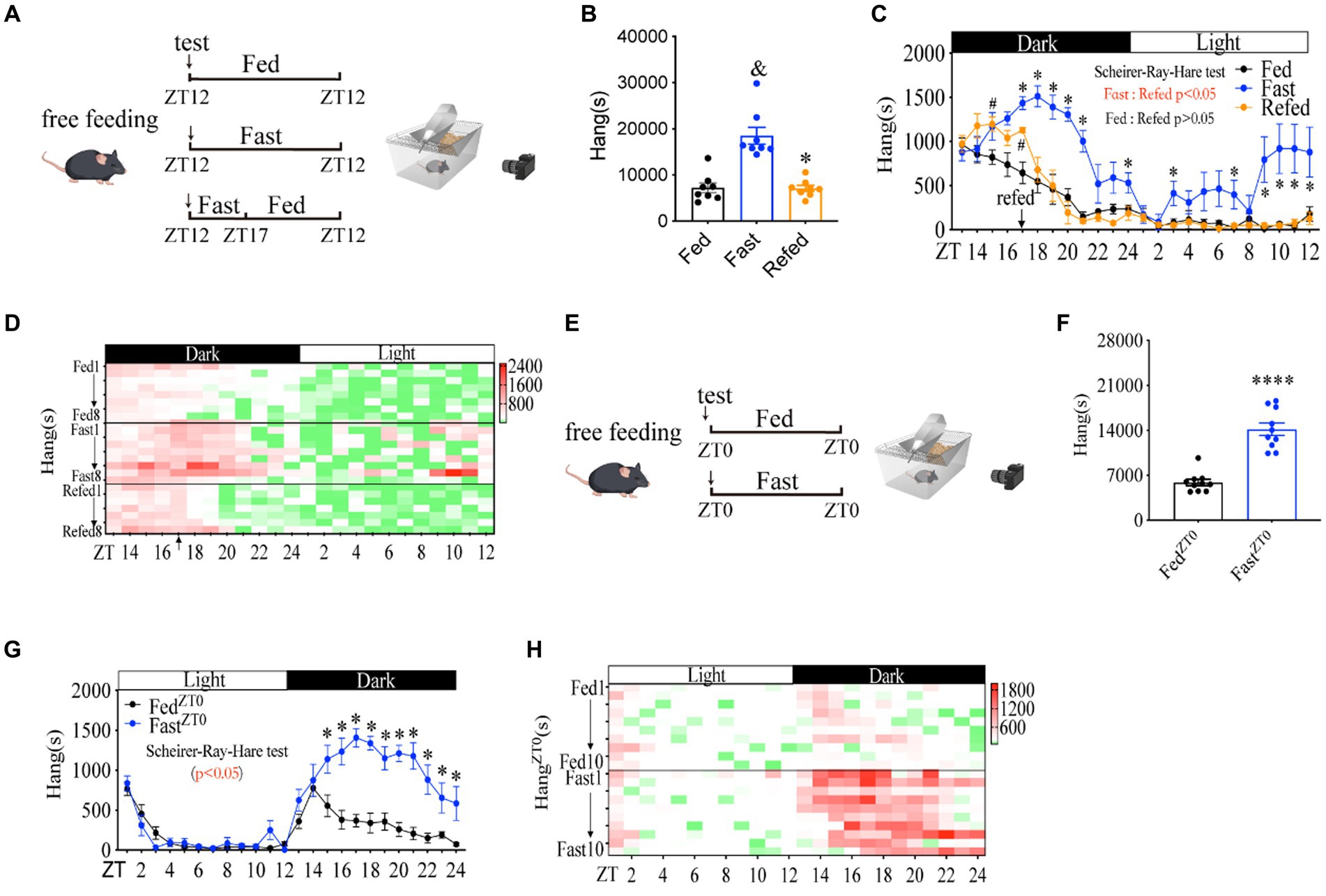
Figure 3. Habitual rhythm foraging behavior is dramatically increased by energy shortage. (A) A diagram of experimental scheme (10 weeks C57BL/6 J male mice were divided into three groups, n = 8:8:8). (B) Total time of hanging in 24 h. (C) Hanging time curve over 24 h. (D) Hanging time/h in 24 h for each mouse. (E) A diagram of experimental scheme (12 weeks C57BL/6 J male mice fed with chow diet or fasted, n = 10:10). (F) Total time of hanging in 24 h. (G) Hanging time curve over 24 h (started from ZT0), (H) hanging time/h in 24 h for each mouse(started from ZT0). Data are represented as mean ± SEM. In panels B,C, & represents the significance between fed and fast mice. # represents the significance between fed and fast mice. * represents the significance between fast and refed mice. In panels F,G, * represents the significance between fast and fast mice. &p < 0.05, #p < 0.05, *p < 0.05, ***p < 0.001, ****p < 0.0001. Data in panel B were analyzed using One-way ANOVA, data in panel F were analyzed using an unpaired t-test, and other data were analyzed using the Scheirer-Ray-Hare test. ZT, zeitgeber time.
Similarly, when mice were monitored from ZT0 (Figure 3E), we found that the distance (Supplementary Figures S4A–C), activity time (Supplementary Figures S4D–F), and foraging behavior (Figures 3F–H) in fasted mice were dramatically increased at night time. In contrast, fasted mice exhibited a similar foraging behavior as free-feeding mice within daytime ZT0-ZT12 (Figures 3G,H). The unchanged foraging behavior during the daytime was possible due to mice with sufficient energy supply after a full meal from the last night (34). Rearing (Supplementary Figures S4G–I) and sniffing (Supplementary Figures S4J–L) were not altered significantly (Scheirer-Ray-Hare test, p > 0.05), even though the total sniffing was decreased (Supplementary Figures S4J,M). Consistent with starting from 7 pm, grooming (Supplementary Figures S4M–O) also decreased and digging (Supplementary Figures S4P–R) behaviors had a decreased trend (Scheirer-Ray-Hare test, p < 0.05), even though the total digging time was not altered significantly. These results demonstrate that the increase of habitually rhythmic foraging behavior induced by fasting is primarily caused by energy shortage.
Since the energy source in food mainly derives from carbohydrate, fat, and protein (35), we next examined whether carbohydrate, fat, or protein plays an important role in the increase of foraging behavior induced by calorie content shortage. After fed with FEG for 2 days, carbohydrate-free gel (CFG), fat-free gel (FFG), protein-free gel (PFG), and FEG as control were used to feed mice, and all types of animal activity were monitored (Figure 4A). Total distance (Supplementary Figures S5A–C), activity time (Supplementary Figures S5D–F) and foraging behavior (Figures 4B–D) of mice were similar between CFG, PFG, FFG, and FEG groups (Scheirer-Ray-Hare test, p > 0.05), suggesting that the habitual rhythm foraging behavior was dramatically increased by energy deficiency rather than individual food components.
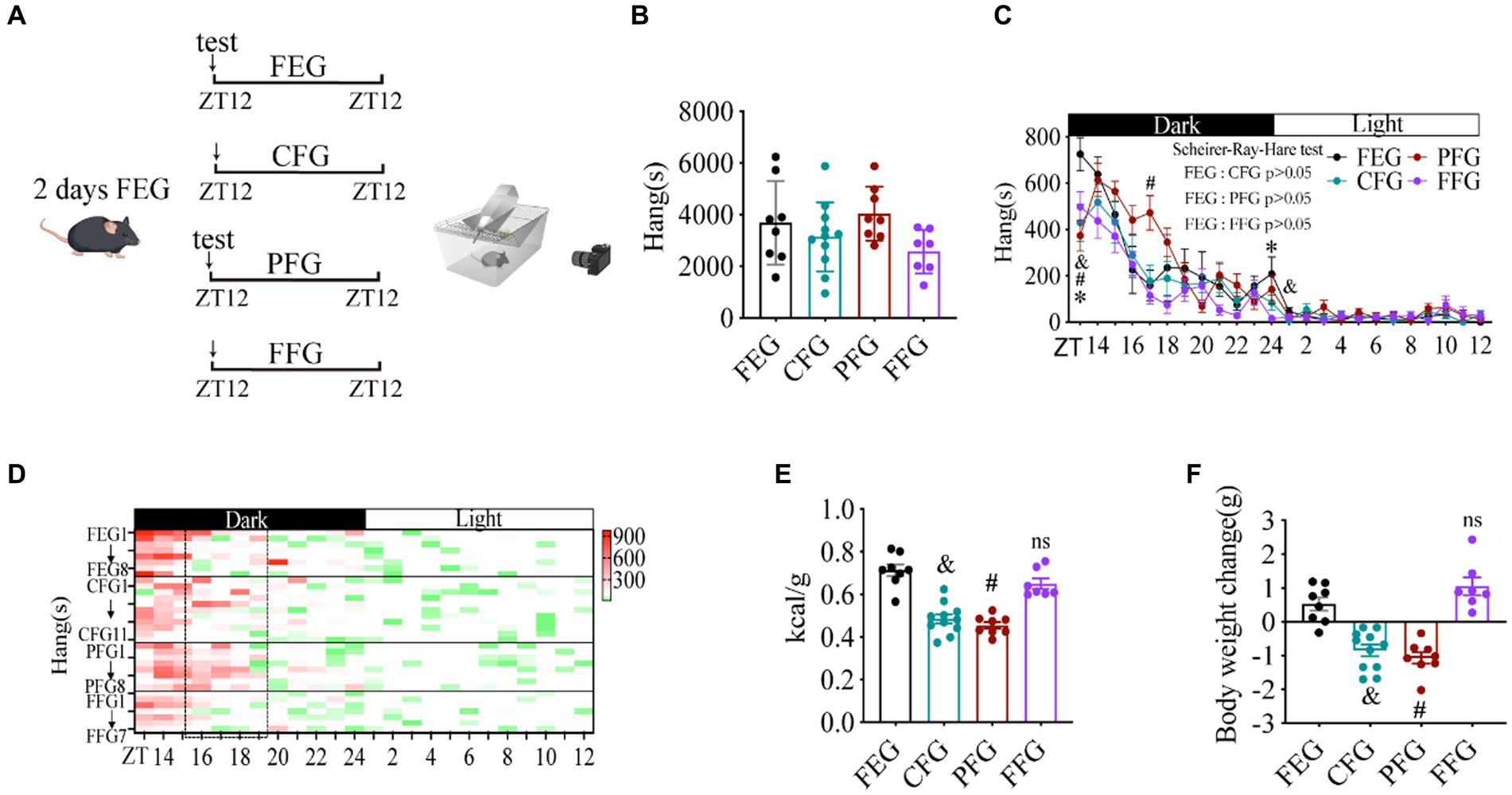
Figure 4. Habitual rhythm foraging behavior is dramatically increased by energy shortage instead of food component. (A) A diagram of experimental scheme. (B) Total time of hanging in 24 h. (C) Hanging time curve over 24 h. (D) Hanging time/h in 24 h for each mouse. (E) Calorie intake (kcal)/body weight (g) in different gel-fed mice. (F) Body weight change in different gel-fed mice. 10 weeks C57BL/6 J male mice were divided into four groups, n = 8:11:8:7. Data are represented as mean ± SEM. & represents the significance between FEG and CFG mice. # represents the significance between FEG and PFG mice. * represents the significance between FEG and FFG mice. &p < 0.05, #p < 0.05, *p < 0.05. Data in histograms were analyzed using One-way ANOVA, and data in panel c were analyzed using the Scheirer-Ray-Hare test. ZT, zeitgeber time. FEG, full-energy gel. CFG, carbohydrate-free gel; PFG, protein-free gel; FFG, fat-free gel.
Next, we found that the deficiency of carbohydrate and protein reduced the calorie intake and body weight gain of mice (Figures 4E,F), suggesting that energy shortage need to reach a threshold for promoting foraging behavior and the small amounts of energy deficits may not have a significant effect. Indeed, protein deficiency increased the foraging behavior of mice at only one time point of ZT17 (Figure 4C). In contrast, the calorie intake and body weight gain of mice were similar between FFG and FEG groups (Figures 4E,F). Consistently, rearing (Supplementary Figures S5G–I) and grooming (Supplementary Figures S5M–O) were also not altered significantly (Scheirer-Ray-Hare test, p > 0.05). However, deficiency of fat increased the sniffing (Supplementary Figures S5J–L) and digging (Supplementary Figures S5P–R) behaviors (Scheirer-Ray-Hare test, p < 0.05), suggesting that fat was involved in the regulation of these behaviors.
Since hypothalamus is the center of regulating energy balance and feeding behavior (36, 37), we next examined whether hypothalamus is associated with the increase in rhythmic foraging behavior induced by energy deficiency. Using immunohistochemistry staining for c-fos, we assessed the activity of hypothalamic neurons in POA, SCN, ARC, PVH, DMH, VMH, and LH at different time points, respectively. The number and density of c-fos positive neurons in PVH were significantly altered (Figures 5A,B. Scheirer-Ray-Hare test p = 0.078). In particular, the number and density of positive cells in PVH were increased at night (Figure 5B), which was similar in the rhythmic character of the increased foraging behavior (Figure 1M). The c-fos expression in ARC was also increased during the night, but slightly reduced during the daytime in fasted mice compared to free-fed mice (Figures 5C,D). By contrast, fasting did not change the oscillation of c-fos expression in DMH, LH, VMH, and SCN (Supplementary Figures S6A–H), but disrupted the rhythmic oscillation of c-fos expression in POA (Supplementary Figures S6I,J). Notably, PVH is one of the most important autonomic control centers in the brain, which integrates endocrine, autonomic, and somatomotor systems from ARC, DMH, and VMH to influence feeding-related behavior (38). Thus, based on the rhythm and change of both c-fos expression and foraging behavior, PVH was possibly associated with the increased foraging behavior induced by energy deficiency.
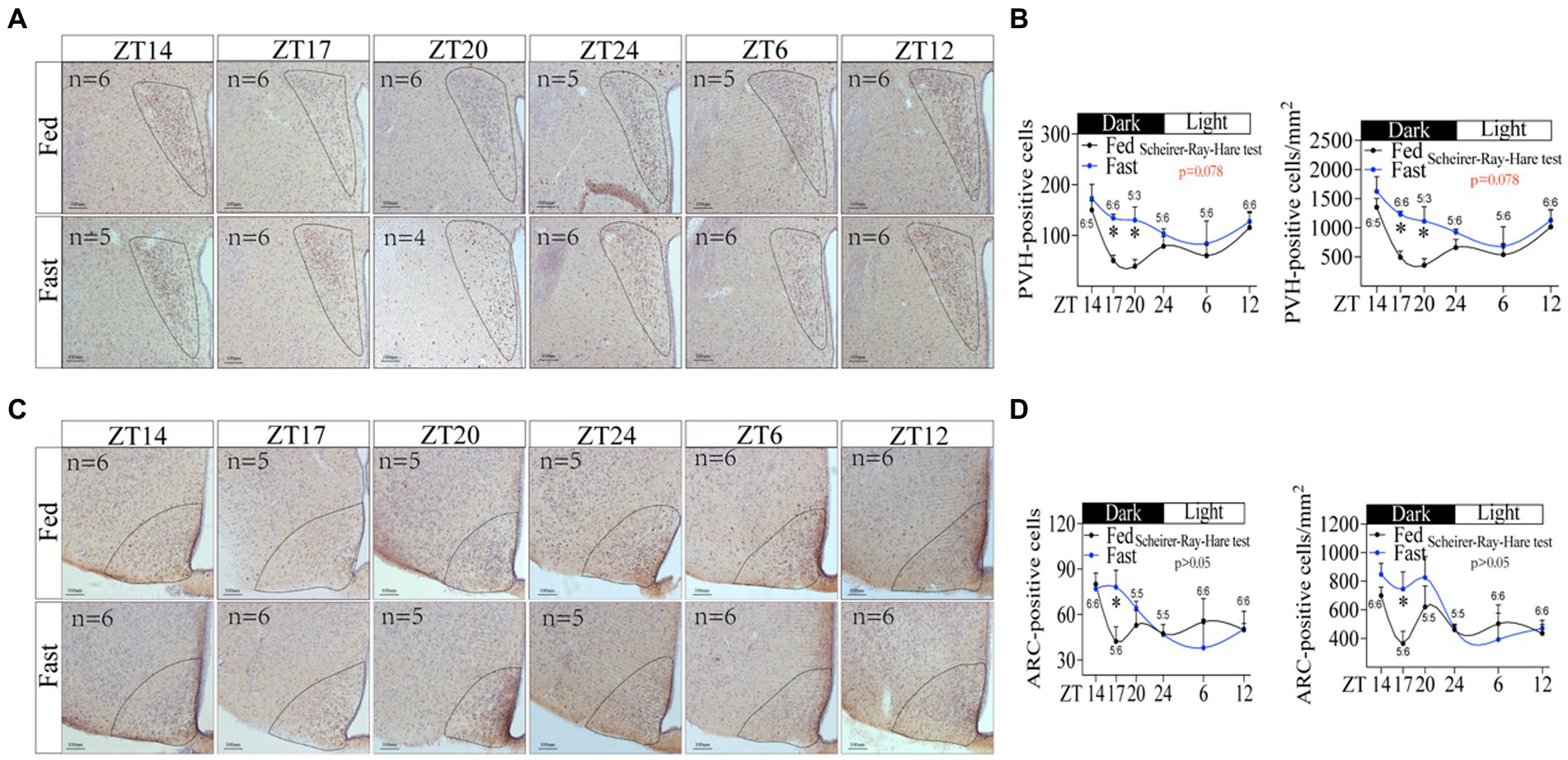
Figure 5. Neuronal activity in hypothalamic PVH is associated with the increasing rhythmic foraging behavior induced by energy deficiency. (A) Immunohistochemical staining of c-fos in PVH at different time points. (B) The number of c-fos positive cells and the density of c-fos positive cells in PVH. (C) Immunohistochemical staining of c-fos in ARC at different time points. (D) The number of c-fos positive cells and the density of c-fos positive cells in ARC. “n” numerical ratios in figures represent the number of mice per group at each time point. Only one brain slice at the corresponding location was selected for each mouse. PVH, ARC. Data were represented as mean ± SEM, *p < 0.05. Data were analyzed using the Scheirer-Ray-Hare test. ZT, zeitgeber time.
Next, we asked whether the neural activity in PVH neurons is responsible for the increase in foraging behavior. To address this issue, we used a chemogenetic approach to activate neuronal activity in PVH by expressing activated DREADDs (Gq hM3D) (Supplementary Figure S7A; Figure 6A). After CNO injection (1 mg/kg, 5 min before ZT12), PVHhM3D(Gq) mice displayed a significant increase in total distance (Figures 6B,C and Supplementary Figure S7B), general activity (Figures 6D,E and Supplementary Figure S7C) and foraging behavior (Figures 6F,G and Supplementary Figure S7D) during fed with FEG, suggesting that the neuron activity in PVH was sufficient to promote the increase of foraging behavior. However, activating neurons in PVH not only increased the foraging behavior, but also increased rearing (Figures 6H,I and Supplementary Figure S7E), sniffing (Figures 6J,K and Supplementary Figure S7F), grooming (Figures 6L,M and Supplementary Figure S7G), and digging (Figures 6N,O and Supplementary Figure S7H) behaviors. Taken together, PVH neuronal activity is sufficient for eliciting foraging behavior.
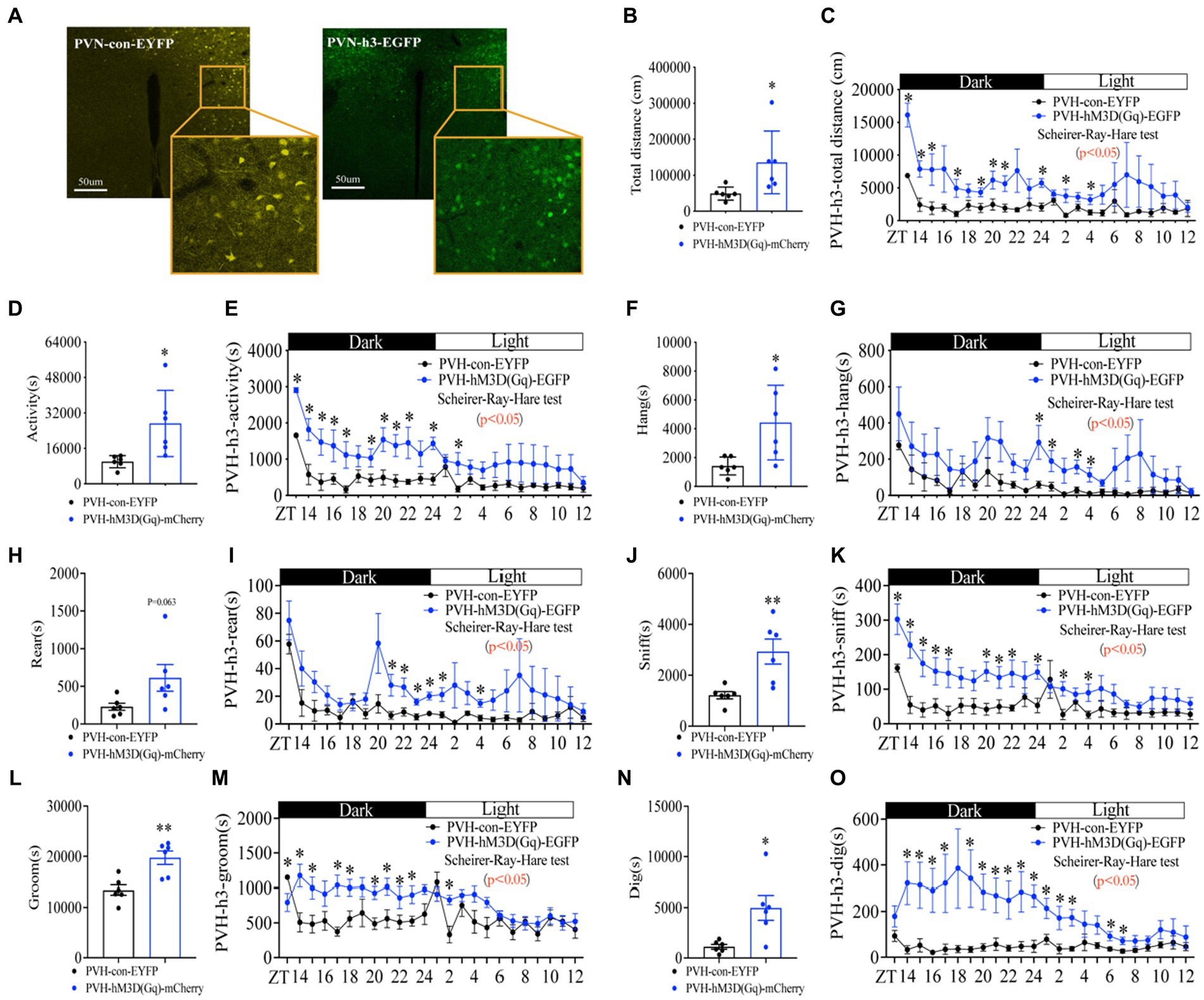
Figure 6. Activating neurons in PVH enhances the amplitude of foraging behavior. (A) hM3D(Gq) and control AAV2/9 expression in PVH. (B) Total distance in 24 h. (C) Distance curve over 24 h. (D) Total activity time in 24 h. (E) Activity time curve over 24 h. (F) Total hanging in 24 h. (G) Hanging curve over 24 h. (H) Total rearing in 24 h. (I) Rearing curve over 24 h. (J) Total sniffing in 24 h. (K) Sniffing curve over 24 h. (L) Total grooming in 24 h. (M) Grooming curve over 24 h. (N) Total digging in 24 h. (O) Digging curve over 24 h. 10 weeks C57BL/6 J male mice were injected with AAV2/9, and experiments were performed 3–4 weeks after virus injection, n = 6:6. Data are represented as mean ± SEM, *p < 0.05, **p < 0.01. Data in histograms were analyzed using an unpaired t-test, and other data were analyzed using the Scheirer-Ray-Hare test. ZT, zeitgeber time.
Next, we determined whether neuron activity in PVH is required for the increase of foraging behavior induced by energy deficiency. PVH neuron activity was inhibited by expressing the inhibitory DREADDs (Gi hM4D) in PVH (Supplementary Figure S8A; Figure 7A). After CNO injection (1 mg/kg, 5 min before ZT12), PVHhM4D(Gi) mice displayed a significant decrease in total distance (Figures 7B,C and Supplementary Figure S8B), general activity (Figures 7D,E and Supplementary Figure S8C), and foraging behavior (Figures 7F,G and Supplementary Figure S8D) during fed with NEG, suggesting that the neuron activity in PVH is required to promote the increase of foraging behavior. Notably, inactivated PVH neuron activity impaired the rhythm of foraging behavior and suppressed almost all of foraging behavior (Figure 7G), suggesting that the neuron activity in PVH is required to sustain the foraging behavior. Consistently, PVHhM4D(Gi) mice displayed a dramatic decrease in rearing (Figures 7H,I and Supplementary Figure S8E), sniffing (Figures 7J,K and Supplementary Figure S8F), grooming (Figures 7L,M and Supplementary Figure S8G), and digging (Figures 7N,O and Supplementary Figure S8H) behaviors. Taken together, neuron activity in PVH is required for the increased foraging behavior induced by energy deficiency.
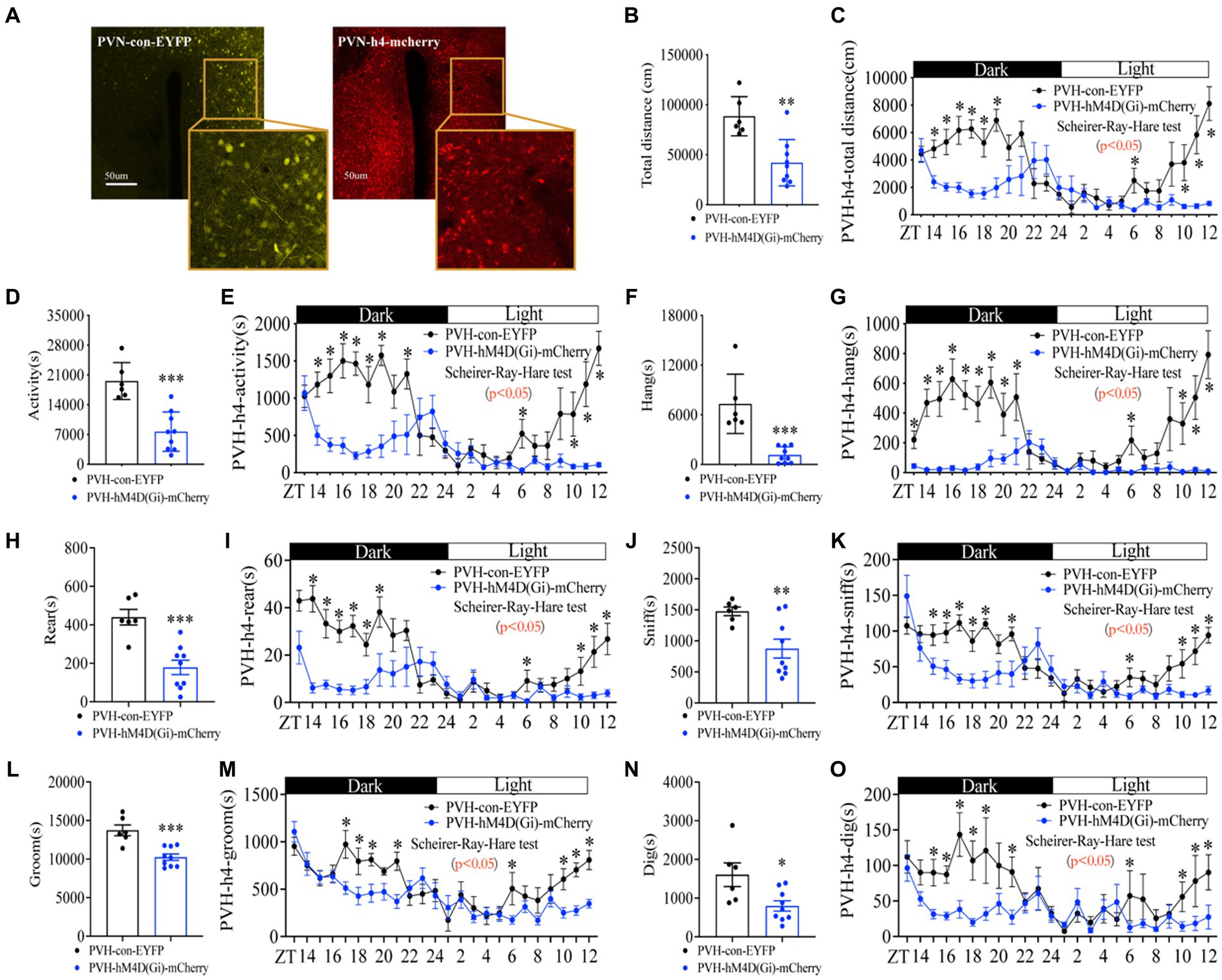
Figure 7. Inactivating neurons in PVH attenuates the amplitude of foraging behavior and disrupts the rhythm of foraging behavior. (A) hM4D(Gi) and control AAV2/9 expression in PVH. (B) Total distance in 24 h. (C) Distance curve over 24 h. (D) Total activity time in 24 h. (E) Activity time curve over 24 h. (F) Total hanging in 24 h. (G) Hanging curve over 24 h. (H) Total rearing in 24 h. (I) Rearing curve over 24 h. (J) Total sniffing in 24 h. (K) Sniffing curve over 24 h. (L) Total grooming in 24 h. (M) Grooming curve over 24 h. (N) Total digging in 24 h. (O) Digging curve over 24 h. 10 weeks C57BL/6 J male mice were injected with AAV2/9, and experiments were performed 3–4 weeks after virus injection, n = 6:6. Data are represented as mean ± SEM, *p < 0.05, **p < 0.01, ***p < 0.001. Data in histograms were analyzed using an unpaired t-test, and other data were analyzed using the Scheirer-Ray-Hare test. ZT, zeitgeber time.
Discussion
The foraging behavior is similar to feeding, reproduction, metabolism, and other physiological processes, which are essential for life (39). In this report, we demonstrate that food cues and energy shortage, rather than macronutrients deficiency, potentiate the rhythmic foraging behavior. This is the first to report that the hanging behavior of fasted mice reflects the nature or characterize of foraging behavior, and is regulated by the activity of PVH neurons.
In humans, dysregulated foraging behavior leads to a variety of pathological manifestations, such as anorexia nervosa (40), binge-eating disorder (41), night-eating syndrome (41), sleep-related eating disorder (42), and obesity (41, 43). Therefore, the study of foraging behavior is crucial for human health. Consistent with the results in animals, a study of human foraging behavior found that people tend to seek out high-calorie foods in supermarkets (44). In addition to calorie-seeking, human foraging behavior is also affected by environment and cultural rituals (45). Thus, normative studies of human foraging behavior are very difficult. In contrast, laboratory animal models are good tools for studying foraging behavior, which will be beneficial in understanding human foraging behavior.
In laboratory mice, hanging behavior is not a unique manifestation of a single function. In previous studies, researchers measured pain and motor neuron function by monitoring hanging behavior in caged mice (46, 47). In this report, we broke the behavior of fasted mice into various fine behaviors and found that only the hanging behavior was increased, similar to the increased total activity (Figure 1), suggesting that the hanging behavior reflects the nature of foraging behavior in fasted mice. Furthermore, mice have adopted the perception of food on the lid of the cage, food deprivation will elicit the hanging behavior when mice attach themselves to the cage lid with their legs off the ground to search for food. Of note, other species or genera of animals, such as bats, flying foxes, and arboreal mammals, show that hanging behavior is a typical action of searching for food (48–50). Although these species of animals are not equal to caged mice, most animal behaviors are similar or equivalent in different animal species. Thus, laboratory mice can be used as a model to study foraging behavior by monitoring the hanging behavior.
Our observations suggest that energy status or hunger signals are critical regulators for foraging behavior (Figures 1M, 2C). However, the partial energy deficit caused by inadequate CFG and PFG intake (Figure 4E) did not cause significant increases in foraging behavior (Figure 4B), suggesting that sufficient energy deficiency is required to motivate foraging behavior. Sufficient energy deficiency is a potent motivator of foraging behavior, which is modulated by evolutionary pressures and governed by hormonal (51), neural (52), and cognitive systems (53). Because the innate drive to restore energy balance is robust (54–56), small energy deficits may not be sufficient to increase foraging behavior.
Grooming and digging were decreased in mice under fasting conditions compared with fed mice (Supplementary Figures S1E–H), while mice fed with NEG showed unaltered grooming and digging behaviors compared with mice fed by FEG (Supplementary Figures S2M–R), suggesting that food cues were involved in regulating grooming and digging. Indeed, grooming and digging behaviors in many animals, especially rodents, are often related to food cues. For example, when food cues cannot predict a certain outcome (e.g., unpredictable food delivery), mice might increase grooming as a way to release stress (57). In addition, food cues might trigger an innate digging response in certain animals (e.g., the scent of food source) (58). In contrast to food deprivation, the presence of NEG provides conditions or choices for mice to dig up or bury food (gel), although NEG is energy-free.
To rule out the interference in the physiological activities of mice during 24 h of continuous Home-Cage monitoring, we administered a single injection of CNO to mice 5 min before the Home-Cage monitoring. Despite the short plasma half-life of CNO in mice (59, 60), the biological effects of CNO can also last as long as 6–10 h (59, 61). Thus, a single injection is sufficient to observe changes in foraging behavior at night in our experiments.
The habitual rhythm of foraging behavior strikingly disappeared when inactivated neurons in PVH (Figure 7G). One possible reason is that the biological effects of CNO can be maintained for over 10 h. Another possibility is that the CNO injection continuously inactivates neuronal activity by binding to DREADDs (24), which masks or disrupts the habitual rhythm of neuronal activity. Of note, extensive regulation of PVH neuronal activity altered all types of behaviors (Figures 6, 7), suggesting that multiple types of neurons in PVH play differential roles in regulating foraging behavior. Indeed, besides feeding behavior, the PVH is also a critical region of the brain involved in other behaviors. For example, activation of the PVH results in the release of corticotropin-releasing hormone (CRH), which in turn triggers the pituitary to release adrenocorticotropic hormone (ACTH) to release glucocorticoids like cortisol, which helps the body respond to stress (62). PVH is also involved in the secretion of gonadotropin-releasing hormone (GnRH), which regulates reproductive hormones and associated behaviors (63). In addition, PVH has been implicated in various social behaviors, possibly due to its role in releasing oxytocin, which is often called the “love hormone” because of its role in pair bonding, social recognition, and other prosocial behaviors (64).
This work is the first to discover the equivalent link between hanging behavior and foraging behavior in caged mice and provides a laboratory mouse model for studying foraging behavior. However, there are some limitations in this study. First, the chemogenetic approach cannot be used to control the rhythm of PVH neuronal activity in mice. Second, the different types of neurons in PVH were not distinguished or identified. Therefore, further experiments are required for the identification of the specific neurons in PVH for regulating rhythmic foraging behavior.
Understanding the foraging behavior has significance in basic biological processes and provides potentially practical applications in managing human eating behavior, preventing eating-related disorders, and developing personalized dietary styles. Considering the effect of energy status and food cues on foraging behavior, researchers and healthcare professionals can design healthier food choices to intervene in overeating behavior and malnutrition.
Conclusion
In summary, we conclude that hanging behavior reflects the properties of foraging behavior in fasted mice, and neuronal activity in PVH is required for triggering and maintaining the foraging behavior. This work will provide fresh insights into the cellular mechanisms for foraging behavior and a better therapeutic target for eating-related disorders.
Data availability statement
The original contributions presented in the study are included in the article/Supplementary material, further inquiries can be directed to the corresponding author.
Ethics statement
The animal study was approved by Institutional Animal Care and Use Committee at Shandong Provincial Hospital. The study was conducted in accordance with the local legislation and institutional requirements.
Author contributions
SW: Conceptualization, Data curation, Formal analysis, Investigation, Methodology, Project administration, Software, Supervision, Validation, Writing – original draft, Writing – review & editing. JW: Writing – review & editing. YX: Software, Writing – review & editing. ZZ: Formal analysis, Software, Writing – review & editing. XJ: Writing – review & editing. YL: Writing – review & editing. YG: Writing – review & editing. HZ: Writing – review & editing. LP: Writing – review & editing. DL: Writing – review & editing. ML: Writing – review & editing. WB: Writing – review & editing, Funding acquisition. QG: Writing – review & editing. ZH: Conceptualization, Data curation, Formal analysis, Funding acquisition, Investigation, Methodology, Project administration, Software, Supervision, Validation, Writing – review & editing.
Funding
The author(s) declare financial support was received for the research, authorship, and/or publication of this article. This research was supported by National Natural Science Foundation of China (NSFC), Grant/Award Number: 82270899 (ZH), Shandong University Grant/Award Number: 2018 TB019 (ZH), and Shandong Provincial Natural Science Foundation, Grant/Award Number: ZR2022MH121 (WB).
Acknowledgments
We thank Bo Xiang (Department of Endocrinology, Shandong Provincial Hospital Affiliated to Shandong First Medical University) and Jin Xie (Department of Endocrinology, Shandong Provincial Hospital Affiliated to Shandong First Medical University) for technical assistance.
Conflict of interest
The authors declare that the research was conducted in the absence of any commercial or financial relationships that could be construed as a potential conflict of interest.
Publisher’s note
All claims expressed in this article are solely those of the authors and do not necessarily represent those of their affiliated organizations, or those of the publisher, the editors and the reviewers. Any product that may be evaluated in this article, or claim that may be made by its manufacturer, is not guaranteed or endorsed by the publisher.
Supplementary material
The Supplementary material for this article can be found online at: https://www.frontiersin.org/articles/10.3389/fnut.2023.1278906/full#supplementary-material
References
1. Bumgarner, JR, and Nelson, RJ. Light at night and disrupted circadian rhythms Alter physiology and behavior. Integr Comp Biol. (2021) 61:1160–9. doi: 10.1093/icb/icab017
2. Cabanac, M. Influence of food and water deprivation on the behavior of the white rat foraging in a hostile environment. Physiol Behav. (1985) 35:701–9. doi: 10.1016/0031-9384(85)90400-7
3. Joseph, RJ, Alonso-Alonso, M, Bond, DS, Pascual-Leone, A, and Blackburn, GL. The neurocognitive connection between physical activity and eating behaviour. Obes Rev. (2011) 12:800–12. doi: 10.1111/j.1467-789X.2011.00893.x
4. Ngo, FY, Li, H, Zhang, H, and Lau, CG. Acute fasting modulates food-seeking behavior and neural signaling in the piriform cortex. Nutrients. (2022) 14:4156. doi: 10.3390/nu14194156
5. Mourao, DM, Bressan, J, Campbell, WW, and Mattes, RD. Effects of food form on appetite and energy intake in lean and obese young adults. Int J Obes. (2007) 31:1688–95. doi: 10.1038/sj.ijo.0803667
6. Trevelline, BK, and Kohl, KD. The gut microbiome influences host diet selection behavior. Proc Natl Acad Sci U S A. (2022) 119:e2117537119. doi: 10.1073/pnas.2117537119
7. Carlson, BA, Rothman, JM, and Mitani, JC. Diurnal variation in nutrients and chimpanzee foraging behavior. Am J Primatol. (2013) 75:342–9. doi: 10.1002/ajp.22112
8. Wang, B, and Chen, J. Effects of fat and protein levels on foraging preferences of tannin in scatter-hoarding rodents. PLoS One. (2012) 7:e40640. doi: 10.1371/journal.pone.0040640
9. Boswell, RG, and Kober, H. Food cue reactivity and craving predict eating and weight gain: a meta-analytic review. Obes Rev. (2016) 17:159–77. doi: 10.1111/obr.12354
10. Drakeley, M, Lapiedra, O, and Kolbe, JJ. Predation risk perception, food density and conspecific cues shape foraging decisions in a tropical lizard. PLoS One. (2015) 10:e0138016. doi: 10.1371/journal.pone.0138016
11. Price, CJ, and Banks, PB. Food quality and conspicuousness shape improvements in olfactory discrimination by mice. Proc Biol Sci. (2017) 284:20162629. doi: 10.1098/rspb.2016.2629
12. Dailey, MJ, and Bartness, TJ. Appetitive and consummatory ingestive behaviors stimulated by PVH and perifornical area NPY injections. Am J Physiol Regul Integr Comp Physiol. (2009) 296:R877–92. doi: 10.1152/ajpregu.90568.2008
13. Balthasar, N, Dalgaard, LT, Lee, CE, Yu, J, Funahashi, H, Williams, T, et al. Divergence of melanocortin pathways in the control of food intake and energy expenditure. Cells. (2005) 123:493–505. doi: 10.1016/j.cell.2005.08.035
14. Betley, JN, Cao, ZF, Ritola, KD, and Sternson, SM. Parallel, redundant circuit organization for homeostatic control of feeding behavior. Cells. (2013) 155:1337–50. doi: 10.1016/j.cell.2013.11.002
15. Atasoy, D, Betley, JN, Su, HH, and Sternson, SM. Deconstruction of a neural circuit for hunger. Nature. (2012) 488:172–7. doi: 10.1038/nature11270
16. Garfield, AS, Li, C, Madara, JC, Shah, BP, Webber, E, Steger, JS, et al. A neural basis for melanocortin-4 receptor-regulated appetite. Nat Neurosci. (2015) 18:863–71. doi: 10.1038/nn.4011
17. Yong, Y, Cakir, I, Lining Pan, P, Biddinger, JE, Bluett, RJ, Mackie, K, et al. Endogenous cannabinoids are required for MC4R-mediated control of energy homeostasis. Proc Natl Acad Sci U S A. (2021) 118:e2015990118. doi: 10.1073/pnas.2015990118
18. Norgren, R. Projections from the nucleus of the solitary tract in the rat. Neuroscience. (1978) 3:207–18. doi: 10.1016/0306-4522(78)90102-1
19. Herbert, H, Moga, MM, and Saper, CB. Connections of the parabrachial nucleus with the nucleus of the solitary tract and the medullary reticular formation in the rat. J Comp Neurol. (1990) 293:540–80. doi: 10.1002/cne.902930404
20. Sutton, AK, Pei, H, Burnett, KH, Myers, MG Jr, Rhodes, CJ, and Olson, DP. Control of food intake and energy expenditure by Nos1 neurons of the paraventricular hypothalamus. J Neurosci. (2014) 34:15306–18. doi: 10.1523/JNEUROSCI.0226-14.2014
21. Takayanagi, Y, Kasahara, Y, Onaka, T, Takahashi, N, Kawada, T, and Nishimori, K. Oxytocin receptor-deficient mice developed late-onset obesity. Neuroreport. (2008) 19:951–5. doi: 10.1097/WNR.0b013e3283021ca9
22. Camerino, C. Low sympathetic tone and obese phenotype in oxytocin-deficient mice. Obesity (Silver Spring). (2009) 17:980–4. doi: 10.1038/oby.2009.12
23. Steele, AD, Jackson, WS, King, OD, and Lindquist, S. The power of automated high-resolution behavior analysis revealed by its application to mouse models of Huntington's and prion diseases. Proc Natl Acad Sci U S A. (2007) 104:1983–8. doi: 10.1073/pnas.0610779104
24. Armbruster, BN, Li, X, Pausch, MH, Herlitze, S, and Roth, BL. Evolving the lock to fit the key to create a family of G protein-coupled receptors potently activated by an inert ligand. Proc Natl Acad Sci U S A. (2007) 104:5163–8. doi: 10.1073/pnas.0700293104
25. Chang, SE, Todd, TP, Bucci, DJ, and Smith, KS. Chemogenetic manipulation of ventral pallidal neurons impairs acquisition of sign-tracking in rats. Eur J Neurosci. (2015) 42:3105–16. doi: 10.1111/ejn.13103
26. Wang, J, Wu, S, Zhan, H, Bi, W, Xu, Y, Liang, Y, et al. p38alpha in the preoptic area inhibits brown adipose tissue thermogenesis. Obesity (Silver Spring). (2022) 30:2242–55. doi: 10.1002/oby.23552
27. Acosta-Rodriguez, VA, de Groot, MHM, Rijo-Ferreira, F, Green, CB, and Takahashi, JS. Mice under caloric restriction self-impose a temporal restriction of food intake as revealed by an automated feeder system. Cell Metab. (2017) 26:267–277.e2. doi: 10.1016/j.cmet.2017.06.007
28. Kennedy, PJ, and Shapiro, ML. Motivational states activate distinct hippocampal representations to guide goal-directed behaviors. Proc Natl Acad Sci U S A. (2009) 106:10805–10. doi: 10.1073/pnas.0903259106
29. Tinker, MT, Bentall, G, and Estes, JA. Food limitation leads to behavioral diversification and dietary specialization in sea otters. Proc Natl Acad Sci U S A. (2008) 105:560–5. doi: 10.1073/pnas.0709263105
30. Patton, DF, and Mistlberger, RE. Circadian adaptations to meal timing: neuroendocrine mechanisms. Front Neurosci. (2013) 7:185. doi: 10.3389/fnins.2013.00185
31. Zjacic, N, and Scholz, M. The role of food odor in invertebrate foraging. Genes Brain Behav. (2022) 21:e12793. doi: 10.1111/gbb.12793
32. Cai, L, Wang, Y, Tigabu, M, Hou, X, Wu, P, Zhou, C, et al. Strength and size of phosphorus-rich patches determine the foraging strategy of Neyraudia reynaudiana. BMC Plant Biol. (2020) 20:545. doi: 10.1186/s12870-020-02738-0
33. Kheradmand, B, Cassano, J, Gray, S, and Nieh, JC. Influence of visual targets and landmarks on honey bee foraging and waggle dancing. Insect Sci. (2020) 27:349–60. doi: 10.1111/1744-7917.12651
34. Challet, E. The circadian regulation of food intake. Nat Rev Endocrinol. (2019) 15:393–405. doi: 10.1038/s41574-019-0210-x
35. Carreiro, AL, Dhillon, J, Gordon, S, Higgins, KA, Jacobs, AG, McArthur, BM, et al. The macronutrients, appetite, and energy intake. Annu Rev Nutr. (2016) 36:73–103. doi: 10.1146/annurev-nutr-121415-112624
36. Berthoud, HR, and Morrison, C. The brain, appetite, and obesity. Annu Rev Psychol. (2008) 59:55–92. doi: 10.1146/annurev.psych.59.103006.093551
37. Sestan-Pesa, M, and Horvath, TL. Metabolism and mental illness. Trends Mol Med. (2016) 22:174–83. doi: 10.1016/j.molmed.2015.12.003
38. Bouret, SG. Development of hypothalamic circuits that control food intake and energy balance. In: nd, Harris RBS, editors. Appetite and food intake: Central control. USA: Taylor & Francis (2017). p. 135–154.
39. Catania, KC. Evolution of brains and behavior for optimal foraging: a tale of two predators. Proc Natl Acad Sci U S A. (2012) 109:10701–8. doi: 10.1073/pnas.1201885109
40. Sodersten, P, Bergh, C, Zandian, M, and Ioakimidis, I. Homeostasis in anorexia nervosa. Front Neurosci. (2014) 8:234. doi: 10.3389/fnins.2014.00234
41. McCuen-Wurst, C, Ruggieri, M, and Allison, KC. Disordered eating and obesity: associations between binge-eating disorder, night-eating syndrome, and weight-related comorbidities. Ann N Y Acad Sci. (2018) 1411:96–105. doi: 10.1111/nyas.13467
42. Irfan, M, Schenck, CH, and Howell, MJ. NonREM disorders of arousal and related parasomnias: an updated review. Neurotherapeutics. (2021) 18:124–39. doi: 10.1007/s13311-021-01011-y
43. Silventoinen, K, and Konttinen, H. Obesity and eating behavior from the perspective of twin and genetic research. Neurosci Biobehav Rev. (2020) 109:150–65. doi: 10.1016/j.neubiorev.2019.12.012
44. de Vries, R, Boesveldt, S, and de Vet, E. Locating calories: does the high-calorie bias in human spatial memory influence how we navigate the modern food environment? Food Qual Prefer. (2021) 94:104338. doi: 10.1016/j.foodqual.2021.104338
45. Ulijaszek, SJ. Human eating behaviour in an evolutionary ecological context. Proc Nutr Soc. (2002) 61:517–26. doi: 10.1079/PNS2002180
46. Zhang, H, Lecker, I, Collymore, C, Dokova, A, Pham, MC, Rosen, SF, et al. Cage-lid hanging behavior as a translationally relevant measure of pain in mice. Pain. (2021) 162:1416–25. doi: 10.1097/j.pain.0000000000002127
47. Petro, TM, Agarkova, IV, Esmael, A, Dunigan, DD, Van Etten, JL, and Pattee, GL. Chlorovirus ATCV-1 accelerates motor deterioration in SOD1-G93A transgenic mice and its SOD1 augments induction of inflammatory factors from murine macrophages. Front Neurol. (2022) 13:821166. doi: 10.3389/fneur.2022.821166
48. Meldrum, DJ, Dagosto, M, and White, J. Hindlimb suspension and hind foot reversal in Varecia variegata and other arboreal mammals. Am J Phys Anthropol. (1997) 103:85–102. doi: 10.1002/(SICI)1096-8644(199705)103:1<85::AID-AJPA6>3.0.CO;2-C
49. Chen, M, Feng, J, Li, Z, Zhou, J, Zhao, H, Zhang, S, et al. Echolocation sound waves, morphological features and foraging strategies in Hipposideros pratti. Ying Yong Sheng Tai Xue Bao. (2002) 13:1629–32.
50. Hengjan, Y, Saputra, V, Mirsageri, M, Pramono, D, Kasmono, S, Basri, C, et al. Nighttime behavioral study of flying foxes on the southern coast of West Java. Indonesia J Vet Med Sci. (2018) 80:1146–52. doi: 10.1292/jvms.17-0665
51. Schwartz, MW, Woods, SC, Porte, D Jr, Seeley, RJ, and Baskin, DG. Central nervous system control of food intake. Nature. (2000) 404:661–71. doi: 10.1038/35007534
52. Palmiter, RD. Is dopamine a physiologically relevant mediator of feeding behavior? Trends Neurosci. (2007) 30:375–81. doi: 10.1016/j.tins.2007.06.004
53. Rosati, AG. Foraging cognition: reviving the ecological intelligence hypothesis. Trends Cogn Sci. (2017) 21:691–702. doi: 10.1016/j.tics.2017.05.011
54. Reilly, SM, and Saltiel, AR. Adapting to obesity with adipose tissue inflammation. Nat Rev Endocrinol. (2017) 13:633–43. doi: 10.1038/nrendo.2017.90
55. Jones, AE, and Divakaruni, AS. Macrophage activation as an archetype of mitochondrial repurposing. Mol Asp Med. (2020) 71:100838. doi: 10.1016/j.mam.2019.100838
56. Nathan, C. Epidemic inflammation: pondering obesity. Mol Med. (2008) 14:485–92. doi: 10.2119/2008-00038.Nathan
57. Kalueff, AV, and Tuohimaa, P. The grooming analysis algorithm discriminates between different levels of anxiety in rats: potential utility for neurobehavioural stress research. J Neurosci Methods. (2005) 143:169–77. doi: 10.1016/j.jneumeth.2004.10.001
58. Rescorla, RA. Behavioral studies of Pavlovian conditioning. Annu Rev Neurosci. (1988) 11:329–52. doi: 10.1146/annurev.ne.11.030188.001553
59. Guettier, JM, Gautam, D, Scarselli, M, Ruiz de Azua, I, Li, JH, Rosemond, E, et al. A chemical-genetic approach to study G protein regulation of beta cell function in vivo. Proc Natl Acad Sci U S A. (2009) 106:19197–202. doi: 10.1073/pnas.0906593106
60. Wess, J, Nakajima, K, and Jain, S. Novel designer receptors to probe GPCR signaling and physiology. Trends Pharmacol Sci. (2013) 34:385–92. doi: 10.1016/j.tips.2013.04.006
61. Alexander, GM, Rogan, SC, Abbas, AI, Armbruster, BN, Pei, Y, Allen, JA, et al. Remote control of neuronal activity in transgenic mice expressing evolved G protein-coupled receptors. Neuron. (2009) 63:27–39. doi: 10.1016/j.neuron.2009.06.014
62. Herman, JP, Ostrander, MM, Mueller, NK, and Figueiredo, H. Limbic system mechanisms of stress regulation: hypothalamo-pituitary-adrenocortical axis. Prog Neuro-Psychopharmacol Biol Psychiatry. (2005) 29:1201–13. doi: 10.1016/j.pnpbp.2005.08.006
63. Herbison, AE. Control of puberty onset and fertility by gonadotropin-releasing hormone neurons. Nat Rev Endocrinol. (2016) 12:452–66. doi: 10.1038/nrendo.2016.70
Keywords: hanging behavior, food cues, CNO, nutrients, brain regions
Citation: Wu S, Wang J, Xu Y, Zhang Z, Jin X, Liang Y, Ge Y, Zhan H, Peng L, Luo D, Li M, Bi W, Guan Q and He Z (2023) Energy deficiency promotes rhythmic foraging behavior by activating neurons in paraventricular hypothalamic nucleus. Front. Nutr. 10:1278906. doi: 10.3389/fnut.2023.1278906
Edited by:
Theresia Mina, Nanyang Technological University, SingaporeReviewed by:
Ruey-Kuang Cheng, Institute of Molecular and Cell Biology (A*STAR), SingaporeMeng Zhao, Stanford University, United States
Copyright © 2023 Wu, Wang, Xu, Zhang, Jin, Liang, Ge, Zhan, Peng, Luo, Li, Bi, Guan and He. This is an open-access article distributed under the terms of the Creative Commons Attribution License (CC BY). The use, distribution or reproduction in other forums is permitted, provided the original author(s) and the copyright owner(s) are credited and that the original publication in this journal is cited, in accordance with accepted academic practice. No use, distribution or reproduction is permitted which does not comply with these terms.
*Correspondence: Zhao He, emhhb2hlQHNkdS5lZHUuY24=