- 1Université Grenoble Alpes, Inserm, Laboratory of Fundamental and Applied Bioenergetics (LBFA), Grenoble, France
- 2Université Grenoble Alpes, Inserm, Laboratory HP2, Grenoble, France
Obstructive sleep apnea syndrome (OSAS) is associated with chronic intermittent hypoxia (cIH) that causes disturbances in glucose and lipid metabolism. Animals exposed to cIH show lower body weight and food intake, but the protein-energy metabolism has never been investigated. Here, to address the gap, we studied the impact of cIH on nutritional status in rats. A total of 24 male Wistar rats were randomized into 3 groups (n = 8): a control group (Ctrl), a cIH group (cIH) exposed to cIH (30 s 21–30 s 5% fraction of inspired oxygen, 8 h per day, for 14 days), and a pair-fed group (PF) exposed to normoxia with food intake adjusted to the intake of the cIH group rats with anorexia. Body weight and food intake were measured throughout the study. After 14 days, the rats were euthanized, the organs were collected, weighed, and the liver, intestine mucosa, and muscles were snap-frozen to measure total protein content. Food intake was decreased in the cIH group. Body weight was significantly lower in the cIH group only (−11%, p < 0.05). Thymus and liver weight as well as EDL protein content tended to be lower in the cIH group than in the Ctrl and PF groups. Jejunum and ileum mucosa protein contents were lower in the cIH group compared to the PF group. cIH causes a slight impairment of nutritional status and immunity. This pre-clinical work argues for greater consideration of malnutrition in care for OSAS patients. Further studies are warranted to devise an adequate nutritional strategy.
Introduction
Obstructive sleep apnea syndrome (OSAS) is characterized by recurrent episodes of collapse of the upper airway during sleep resulting in chronic intermittent hypoxia (cIH). OSAS induces disturbances in glucose and lipid metabolism (1, 2). As OSAS is strongly associated with obesity (1), the issue of whether OSAS alters protein-energy metabolism has never been explored, despite the growing body of evidence that sarcopenic obesity negatively affects patient outcomes, increasing the risks for frailty, disability, and morbimortality (3–5).
A preclinical model demonstrated that cIH, which is the major feature of OSAS, is a critical component of glucose homeostasis disturbances, including insulin resistance characterized by decreased insulin sensitivity and increased HOMA-IR (homeostasis model assessment of insulin resistance), and dyslipidemia characterized by increased total cholesterol, LDL (low density lipoprotein), or triglyceride levels (6–11). However, animals exposed to cIH showed decreased food intake, especially along hypoxia exposure, that was associated with a lower body weight (7, 8, 12), which suggests an alteration of nutritional status.
To the best of our knowledge, altered protein-energy metabolism under cIH has never been studied. Here, to address this gap, this study set out to investigate the impact of cIH on nutritional status in rats.
Materials and methods
Experimental design
Animals
All the procedures were registered as compliant with European Directives on the care and use of animals for research purposes, and approved by the French Ministry of Research (APAFIS #201603301129626-V3).
The study used 24 male Wistar rats aged 6–7 weeks old. The rats were housed 6 per cage in a temperature-controlled facility (22 ± 2°C) on a 12 h light/dark cycle for an 8-day acclimatization period. Water and food (16.9% proteins, 4.3% lipids; LASQCDiet R16-R) were provided ad libitum.
The rats were then randomized into 3 groups (n = 8 per group): a healthy control group (Ctrl) exposed to normoxia and fed ad libitum; a cIH group (cIH) exposed to cIH (see below) and fed ad libitum; a pair-fed group (PF) exposed to normoxia with food intake adjusted to intake of the cIH group who suffered of anorexia to rule out the effect of decreased food intake decrease observed in cIH.
During the study, rats were separated into individual cages in order to measure their food intake (the difference between the food given the day before and the food still in the cage) (13, 14). Body weight and food intake were monitored throughout the study to evaluate nutritional status (15).
Intermittent hypoxia
Briefly, rats were exposed to cIH (cIH; 30 s-O2 21%/30 s-O2 5%; 8 h.d–1) in their cages for 14 days or to normoxia (Ctrl and PF; 30 s-O2 21%/30 s-O2 21%; 8 h.d–1) to reproduce equivalent levels of noise and air turbulence related to gas circulation. Fraction of inspired oxygen (FIO2) was monitored with a ML206-model gas analyzer (ADInstruments, Dunedin, New Zealand) (7, 8).
Euthanasia and sample collection
At D14, the rats were fasted for 3 h -then euthanized by exsanguination. Blood was collected into capillary tubes for hematocrit determination.
The heart, liver, kidney, spleen, thymus, the extensor digitorum longus (EDL), tibialis and soleus muscles (right and left), and the jejunum and ileum (proximal part) mucosa were weighed. The muscles, liver and intestinal mucosa were snap-frozen in liquid nitrogen and stored at −80°C until analysis.
Protein content analysis
Frozen EDL, tibialis, soleus, liver, jejunum mucosa and ileum mucosa were ground and homogenized in 10 volumes of ice-cold 10% trichloroacetic acid, 0.5 mmol/l EDTA. After delipidation with ethanol/ether (1:1 vol/vol), the pellets were dissolved in NaOH 1N (4 mL/100 mg tissue, 12 h at +40°C). Then, total protein content was determined by a method based on bicinchoninic acid (Pierce™ BCA Protein Assay Kit; ThermoScientific, Rockford, IL, USA) (13, 16).
Statistical analysis
Results are reported as means ± SEM. After checking the data for normality, food intake and body weight change were studied using two-way (group × time) repeated-measures analysis of variance (ANOVA). For the other parameters, if D’Agostino-Pearson’s K2 test for normality passed (p > 0.05), we used ANOVA followed by Tukey’s post-hoc test, and if the normality test failed, we used a Kruskal–Wallis one-way analysis followed by Dunn’s post-hoc test. Differences were considered statistically significant at p < 0.05.
Results
Hematocrit
Hematocrit was higher in the cIH group compared to the other groups (Figure 1A), thus confirming the exposure to hypoxia.
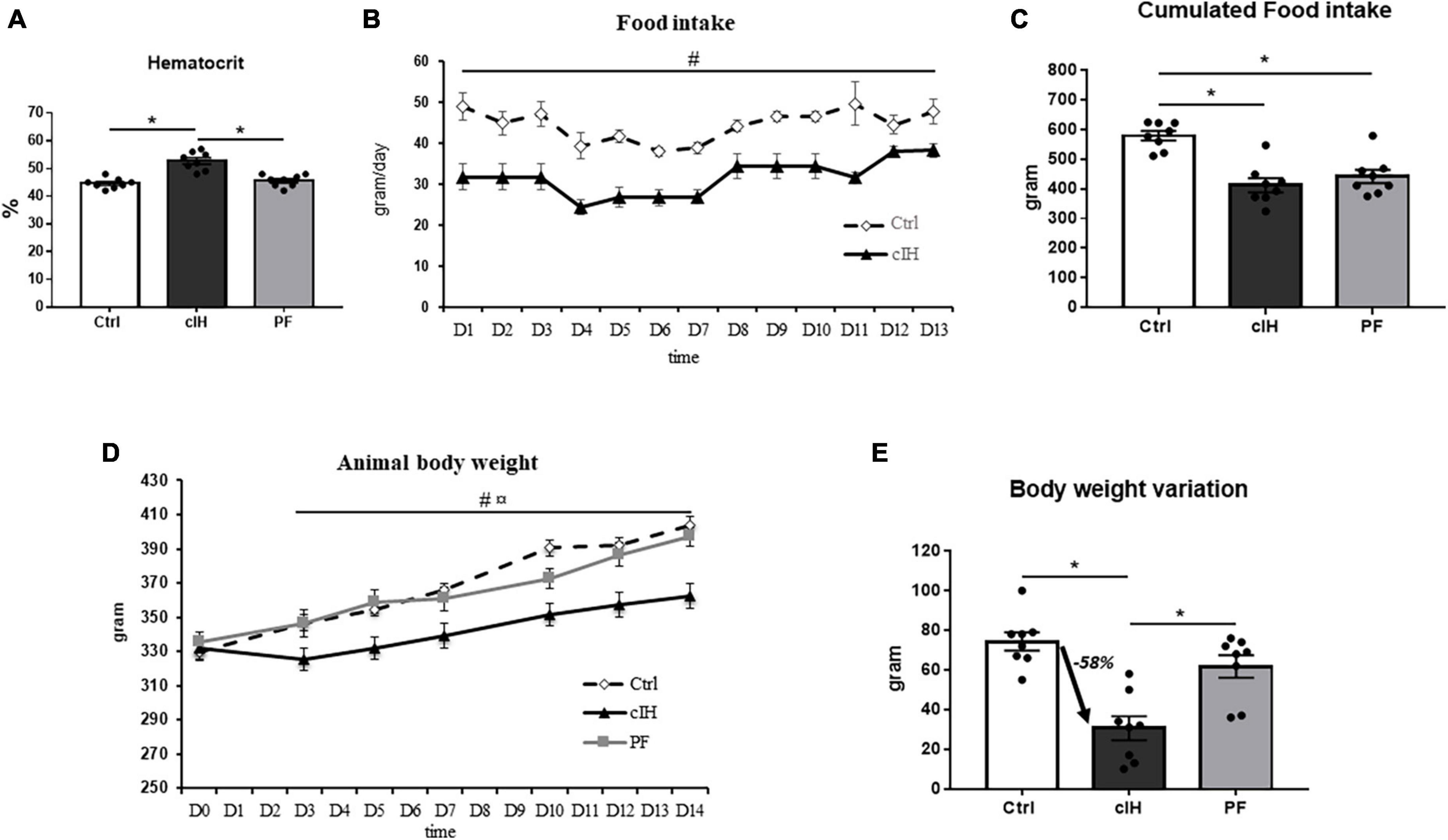
Figure 1. Model validation and rat food intake and body weight variation. (A) Hematocrit, (B) rat food intake, (C) 14-day cumulative food intake, (D) rat body weight, (E) rat body weight variation between D0 and D14. Healthy control rats (Ctrl; n = 8) were under normoxia and fed ad libitum. The chronic intermittent hypoxia group (cIH; n = 8) was exposed to intermittent hypoxia [60-s intermittent hypoxia cycles: 30 s at 5% fraction of inspired oxygen (FIO2) and 30 s at 21% FIO2, for 8 h a day] and fed ad libitum. The pair-fed group (PF; n = 8) was under normoxia and had the same food consumption as the cIH-group rats that showed anorexia. The rats were euthanized at D14. Results are expressed as means ± SEM. *p < 0.05; # cIH vs. Ctrl, p < 0.05; cIH vs. PF, p < 0.05.
Food intake and body weight
Food intake was lower in cIH rats than in Ctrl rats throughout the exposure (Figure 1B). A total of 30% lower cumulative food intake was observed after 14 days of cIH (Figure 1C).
Body weight was lower in cIH rats than in Ctrl and PF rats throughout the exposure (Figure 1D), with a 58% lower total body weight gain after 14 days of cIH (Figure 1E).
Organ weights
Thymus weight was lower in cIH rats compared to Ctrl and PF rats (Figure 2A). Liver weight was lower and ileum mucosa weight tended to be lower (p = 0.0597) in the cIH group compared to the PF group (Figures 2B, C). Heart, kidney, spleen, jejunum mucosa and muscle weights were not modified by cIH or anorexia (Figures 2D–J).
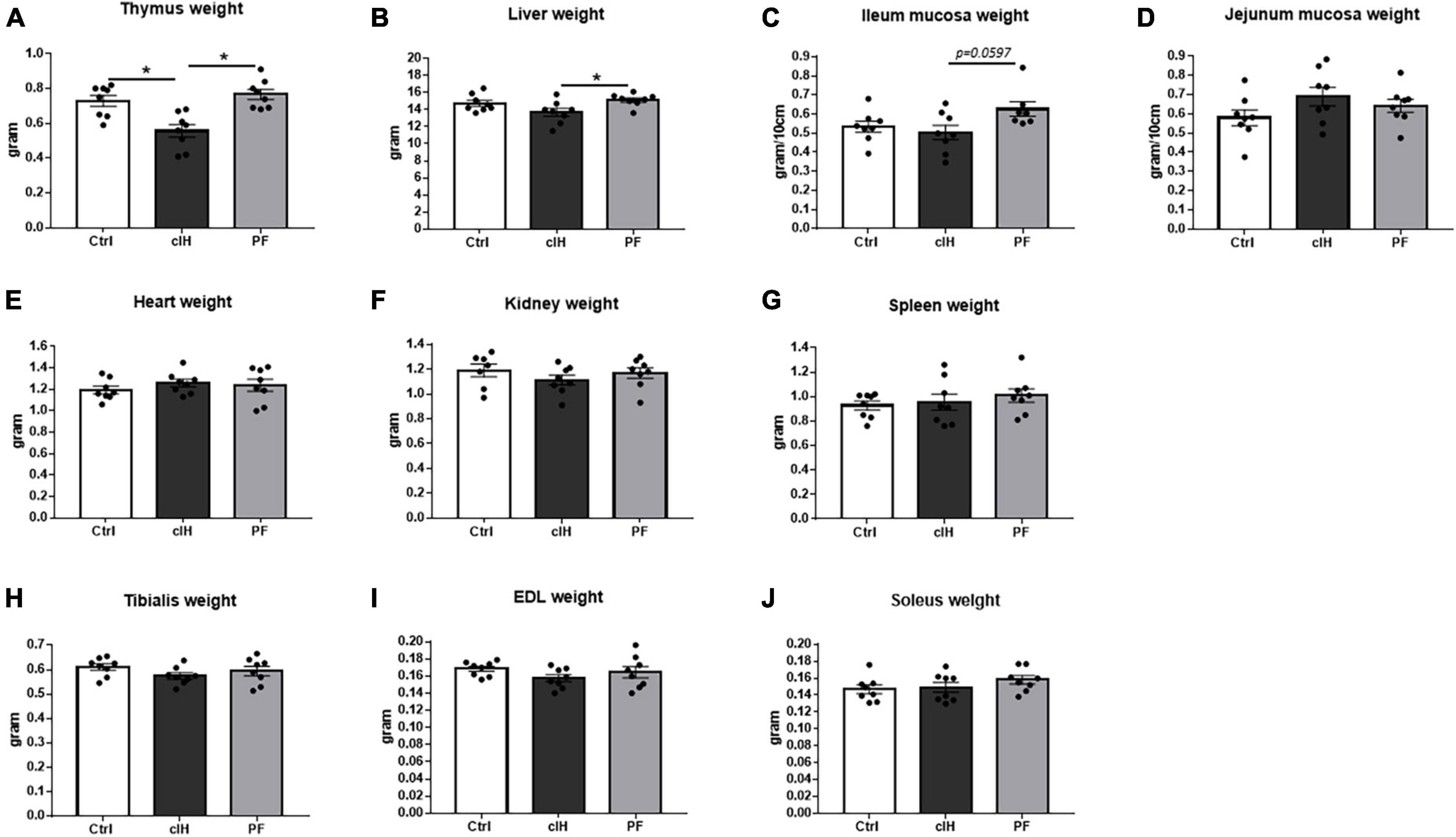
Figure 2. Organ weights. Weights of (A) thymus, (B) liver, (C) ileum mucosa, (D) jejunum mucosa, (E) heart, (F) kidney, (G) spleen, (H) tibialis muscle, (I) EDL muscle, and (J) soleus muscle. Healthy control rats (Ctrl; n = 8) were under normoxia and fed ad libitum. The chronic intermittent hypoxia group (cIH; n = 8) was exposed to intermittent hypoxia [60-s intermittent hypoxia cycles: 30 s at 5% fraction of inspired oxygen (FIO2) and 30 s at 21% FIO2, for 8 h a day] and fed ad libitum. The pair-fed group (PF; n = 8) was under normoxia and had the same food consumption as the cIH-group rats that showed anorexia. The rats were euthanized at D14. Results are expressed as means ± SEM. *p < 0.05.
Tissue protein contents
Liver protein content showed no difference between the 3 groups (Figure 3A). Ileum mucosa protein content was lower in cIH rats compared to PF rats, whereas jejunum mucosa protein content tended to be higher (p = 0.0588) in PF rats compared to Ctrl-group rats (Figures 3B, C). In muscle, EDL protein content tended to be lower in cIH and PF rats than in Ctrl rats (p = 0.0534 and p = 0.0722, respectively) whereas tibialis and soleus protein content showed no difference between the 3 groups (Figures 3D–F).
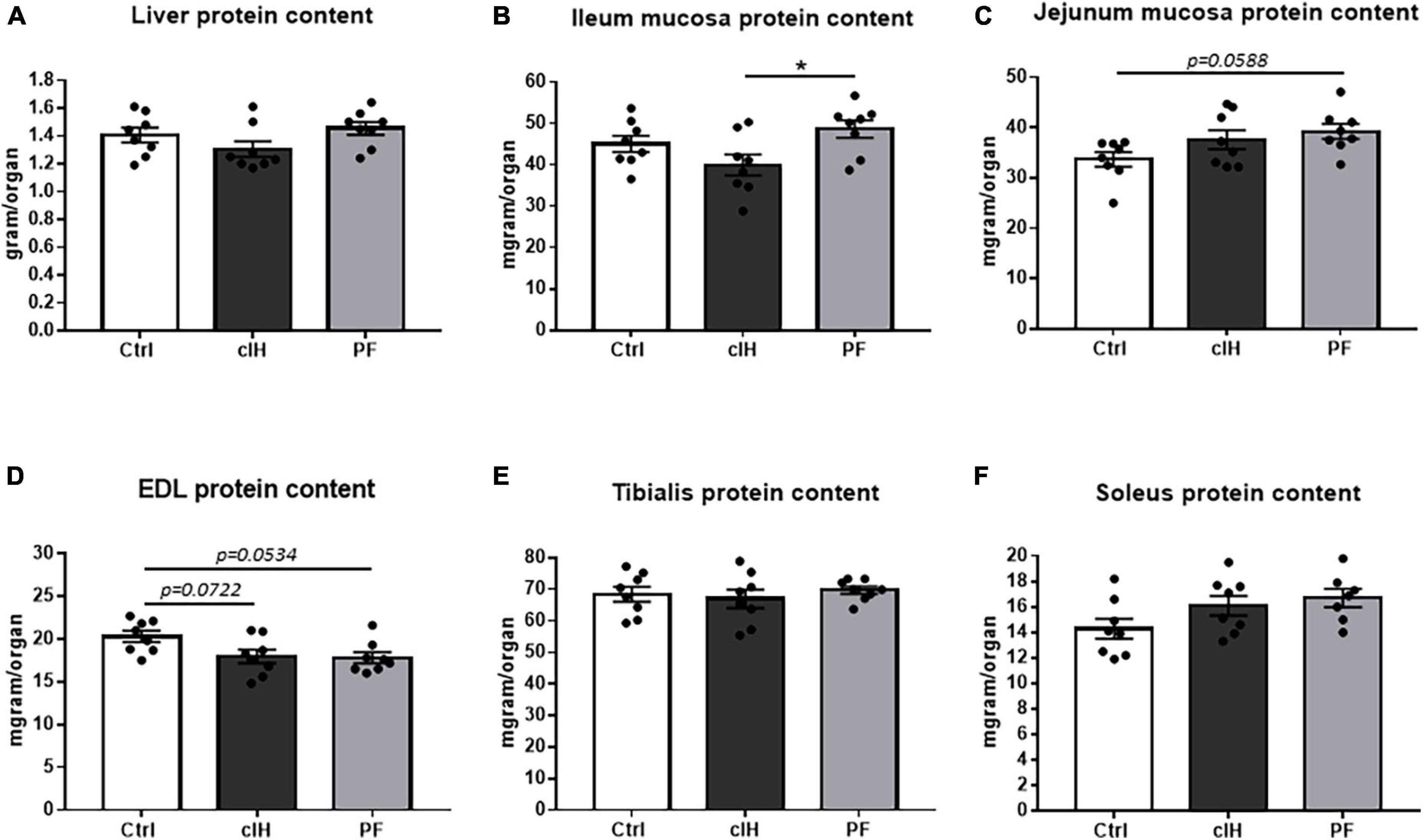
Figure 3. Tissue protein content. Protein content of (A) liver, (B) ileum mucosa, (C) jejunum mucosa, (D) EDL muscle, (E) tibialis muscle, and (F) soleus muscle. Healthy control rats (Ctrl; n = 8) were under normoxia and fed ad libitum. The chronic intermittent hypoxia group (cIH; n = 8) was exposed to intermittent hypoxia [60-s intermittent hypoxia cycles: 30 s at 5% fraction of inspired oxygen (FIO2) and 30 s at 21% FIO2, for 8 h a day] and fed ad libitum. The pair-fed group (PF; n = 8) was under normoxia and had the same food consumption as the cIH-group rats that showed anorexia. The rats were euthanized at D14. Results are expressed as means ± SEM. *p < 0.05.
Discussion
OSAS is found mainly in obese patients. For this reason, no study yet has tested whether OSAS-induced cIH affects nutritional status, whereas malnutrition during obesity (i.e., sarcopenic obesity) is emerging as a major problem (3–5). First, as previously described, we validated our model by demonstrating that cIH induced an increase in hematocrit (12). The hypoxia inducible factor-1 (HIF-1)-related erythropoietin (EPO) gene transcription is an adaptative answer to the decrease in PO2 and is responsible for an increase in hematocrit (17). In the context of cIH, HIF-1 is strongly activated (18); thus its activation on EPO gene is one of the main explanation to observe an increase in hematocrit induced by cIH. Then, here, for the first time, we evaluated the effect of cIH, the critical component in terms of metabolic effects of OSAS, on nutritional status in rats. We showed that cIH slightly negatively impacts nutritional status, independently of the associated anorexia, causing decreased body weight and a modest alteration of protein metabolism in intestine and muscle.
cIH had modest effects on nutritional status but with signs of altered protein metabolism. As expected, we observed anorexia, as reported in animal models of cIH (7, 8, 12). In the same way, previous studies reported an impact of cIH on feeding behavior that is tightly controlled by the central nervous system. The increase in plasma leptin level has been proposed to explain an anorexigen effect (7). However, in this study we suggest that cIH alters some specific O2-sensitive pathways controlling feeding behavior. Among them, adiponectin, an adipokine recognized to counteract the effect of leptin, is decreased under IH and in sleep apnea patients (19). Anorexia is usually observed in stress situations (e.g., sepsis, cancer-related chemotherapy) (13, 14, 20), but most of these stresses have been applied acutely and are associated with short-term anorexia followed by a rapid increase in food intake to redress the loss of energy intake. Here, the anorexia persisted throughout the 14 days of exposure. This suggests that long-term anorexia (even if quite moderate, i.e., 35%) in cIH could lead to malnutrition and thus all the consequences of malnutrition such as sarcopenia and dysimmunity, as well as decreased quality of life and increased morbimortality (3–5). Interestingly, the loss of body weight was not related to anorexia (no effect of pair feeding) but was specifically related to cIH. One explanation for this cIH-related body weight loss could be the metabolic disturbances induced by cIH, particularly in adipose tissue, such as morphological changes in adipose tissue including altered inflammatory profiles, activation of HIF-1, and sympathetic activation-induced lipolysis (21).
As body weight loss is usually associated with sarcopenia and dysimmunity, we explored protein metabolism and observed some alterations caused by cIH itself. First, cIH clearly induced disturbances in protein metabolism, especially in the intestinal tract, with no intestinal mucosa response to anorexia. This is consistent with previous works showing that hypoxia leads to gastrointestinal disorders, due in part to disrupted intestinal microbiota (22), and that OSAS is characterized by microbiota disruption and impaired intestinal barrier function (23), but there has been no previous work on intestinal protein metabolism under cIH.
Second, cIH induced an alteration in the liver response to anorexia. Substantial alterations in hepatic glucose metabolism (i.e., systemic insulin resistance) have already been observed in our rodent cIH model (7, 8), but here we found no change in hepatic protein content. However, since the canonical response to insulin (i.e., 308Thr/473Ser–PPKB/PKB) has been demonstrated to be altered in liver, adipose tissue and striated skeletal muscle (7), we have to consider a direct impact of canonical insulin signaling pathway impairment on protein synthesis. In line with systemic metabolic disturbances, mitochondrial function and structure alterations could also explain an alteration in protein metabolism since adenosine triphosphate (ATP) production is essential for protein synthesis. Besides cIH induces mitochondrial dysfunction and structural damages in several organs such as striated skeletal muscle (24), liver (25), heart (26) and brain (27). Finally, since a decade, cIH has also been demonstrated to induce endoplasmic reticulum (ER) stress in several organs such as liver (28), heart (29) and brain (30). Since ER is involved in protein folding, we cannot exclude the impact of cIH on misfolded protein clearance and metabolism alteration.
Third, as previously observed by Iiyori et al. (31), we expected to see an alteration of protein metabolism mainly in oxidative muscle (i.e., soleus) in response to the lack of oxygen. Instead, we observed that protein metabolism tended to be altered in glycolytic muscle (i.e., EDL), and in both anorexic groups, suggesting that this response is not specifically related to cIH. The lack of effects of cIH on muscle protein metabolism observed in our study could be explained by metabolic adaptations of the muscle [for review see (32)]. Hence, previous data on hypoxia showed that muscle protein synthesis was maintained in myotubes whereas oxygen consumption was declined (33), and was even increased in rat submitted to hypoxia (34). As protein synthesis is an important driver of energy expenditure (i.e., accounting for approximately 20% of energy consumption in cells) (35), the increase of muscle protein synthesis should be supported by a modulation of energy metabolism. Therefore, the increase of muscle protein synthesis could be associated with an increase of the energy expenditure. Such results suggest that even if muscle weight and muscle protein content are not modified, an increase in muscle protein turnover, raising energy expenditure and leading to body weight loss, cannot be excluded. Further studies exploring deeply the effect of cIH on body composition and energy expenditure would be of major interest.
According to the literature, metabolic modifications (i.e., body weight and lipid metabolism), like the cardiovascular alterations associated with cIH, are highly dependent on the severity of cIH (5 vs. 10% FIO2) and the cIH pattern (number of hours/day and FIO2 percentage) (9, 31, 36). For example, body weight was not modified in rats under slight cIH (10%) but was decreased under severe cIH (5% FIO2) (9, 37). Here, under severe cIH (5% FIO2), we observed the same body weight loss (i.e., 10%) but only slight alterations in protein metabolism, suggesting that protein metabolism alterations may not be dependent on the severity and pattern of cIH. Further investigations are needed to better understand the impact of cIH on protein metabolism.
The consequences of hypoxia on immune cells are well known from decades [For recent review see (38)], but the knowledge on the effects of cIH are much more scattered. In our study, cIH caused thymic involution, which reflects immunosuppression and malnutrition (39). Immune dysfunction, at cellular level, has been found in OSAS, but it is difficult to separate the cause between cIH and OSA-related comorbidities (40, 41). In our work, we confirm a direct effect of cIH on immune status. Among the explanations which could be proposed, one is a crosstalk between IH and innate immunity previously revealed to involve the activation of HIF-1 and NF-kB (42). Although further experiments are needed to more deeply explore immune function, this study nevertheless showed that cIH has a share of responsibility in immune dysfunction, which is not surprising given that immune cell function requires oxygen.
Conclusion
This preliminary study suggests that cIH itself can affect both nutritional and immune status. This pre-clinical work argues strongly for greater consideration of malnutrition in care for OSAS patients, and further research is warranted in order to devise an adequate nutritional strategy for OSAS patients.
Data availability statement
The original contributions presented in this study are included in this article/supplementary material, further inquiries can be directed to the corresponding author.
Ethics statement
The animal study was approved by the French Ministry of Research (APAFIS #201603301129626-V3). The study was conducted in accordance with the local legislation and institutional requirements.
Author contributions
CB, SM, CM, and EB contributed to conception and design of the study. CB, SM, SB, MC, and EB performed the study. CB analyzed the results, performed the statistical analysis, and wrote the first draft of the manuscript. All authors contributed to manuscript revision, read, and approved the submitted version.
Funding
This study was supported by a recurrent grant from the Grenoble Alpes University.
Acknowledgments
We thank Cindy Tellier, Arthur Goron, and Geoffrey Maillard for providing valuable technical support.
Conflict of interest
The authors declare that the research was conducted in the absence of any commercial or financial relationships that could be construed as a potential conflict of interest.
Publisher’s note
All claims expressed in this article are solely those of the authors and do not necessarily represent those of their affiliated organizations, or those of the publisher, the editors and the reviewers. Any product that may be evaluated in this article, or claim that may be made by its manufacturer, is not guaranteed or endorsed by the publisher.
References
1. Lévy P, Kohler M, McNicholas W, Barbé F, McEvoy R, Somers V, et al. Obstructive sleep apnoea syndrome. Nat Rev Dis Primers. (2015) 1:15015. doi: 10.1038/nrdp.2015.15
2. Lv R, Liu X, Zhang Y, Dong N, Wang X, He Y, et al. Pathophysiological mechanisms and therapeutic approaches in obstructive sleep apnea syndrome. Signal Transduct Target Ther. (2023) 8:218. doi: 10.1038/s41392-023-01496-3
3. Barazzoni R, Bischoff S, Boirie Y, Busetto L, Cederholm T, Dicker D, et al. Sarcopenic obesity: time to meet the challenge. Clin Nutr. (2018) 37:1787–93. doi: 10.1016/j.clnu.2018.04.018
4. Donini L, Busetto L, Bischoff S, Cederholm T, Ballesteros-Pomar M, Batsis J, et al. Definition and diagnostic criteria for sarcopenic obesity: ESPEN and EASO consensus statement. Clin Nutr. (2022) 41:990–1000. doi: 10.1016/j.clnu.2021.11.014
5. Cappellari G, Guillet C, Poggiogalle E, Pomar M, Batsis J, Boirie Y, et al. Sarcopenic obesity research perspectives outlined by the sarcopenic obesity global leadership initiative (SOGLI) - Proceedings from the SOGLI consortium meeting in Rome November 2022. Clin Nutr. (2023) 42:687–99. doi: 10.1016/j.clnu.2023.02.018
6. Barnes L, Mesarwi O, Sanchez-Azofra A. The cardiovascular and metabolic effects of chronic hypoxia in animal models: a mini-review. Front Physiol. (2022) 13:873522. doi: 10.3389/fphys.2022.873522
7. Thomas A, Belaidi E, Moulin S, Horman S, van der Zon G, Viollet B, et al. Chronic intermittent hypoxia impairs insulin sensitivity but improves whole-body glucose tolerance by activating skeletal muscle AMPK. Diabetes. (2017) 66:2942–51. doi: 10.2337/db17-0186
8. Poulain L, Mathieu H, Thomas A, Borel A, Remy C, Levy P, et al. Intermittent hypoxia-induced insulin resistance is associated with alterations in white fat distribution. Sci Rep. (2017) 7:11180. doi: 10.1038/s41598-017-11782-0
9. Li J, Savransky V, Nanayakkara A, Smith P, O’Donnell C, Polotsky V. Hyperlipidemia and lipid peroxidation are dependent on the severity of chronic intermittent hypoxia. J Appl Physiol. (2007) 102:557–63. doi: 10.1152/japplphysiol.01081.2006
10. Murphy AM, Thomas A, Crinion SJ, Kent BD, Tambuwala MM, Fabre A, et al. Intermittent hypoxia in obstructive sleep apnoea mediates insulin resistance through adipose tissue inflammation. Eur Respir J. (2017) 49:1601731. doi: 10.1183/13993003.01731-2016
11. Drager LF, Jun JC, Polotsky VY. Metabolic consequences of intermittent hypoxia: relevance to obstructive sleep apnea. Best Pract Res Clin Endocrinol Metab. (2010) 24:843–51. doi: 10.1016/j.beem.2010.08.011
12. Ozcan B, Blachot-Minassian B, Paradis S, Mazière L, Chambion-Diaz M, Bouyon S, et al. L-citrulline supplementation reduces blood pressure and myocardial infarct size under chronic intermittent hypoxia, a major feature of sleep apnea syndrome. Antioxidants. (2022) 11:2326. doi: 10.3390/antiox11122326
13. Breuillard C, Moinard C, Goron A, Neveux N, De Reviers A, Mazurak V, et al. Dietary citrulline does not modify rat colon tumor response to chemotherapy, but failed to improve nutritional status. Clin Nutr. (2021) 40:4560–8. doi: 10.1016/j.clnu.2021.05.035
14. Boutière M, Cottet-Rousselle C, Coppard C, Couturier K, Féart C, Couchet M, et al. Protein intake in cancer: does it improve nutritional status and/or modify tumour response to chemotherapy? J Cachexia Sarcopenia Muscle. (2023) 14:2003–15. doi: 10.1002/jcsm.13276
15. Cederholm T, Jensen G, Correia M, Gonzalez M, Fukushima R, Higashiguchi T, et al. GLIM criteria for the diagnosis of malnutrition - A consensus report from the global clinical nutrition community. J Cachexia Sarcopenia Muscle. (2019) 10:207–17. doi: 10.1002/jcsm.12383
16. Breuillard C, Darquy S, Curis E, Neveux N, Garnier J, Cynober L, et al. Effects of a diabetes-specific enteral nutrition on nutritional and immune status of diabetic, obese, and endotoxemic rats: interest of a graded arginine supply. Crit Care Med. (2012) 40:2423–30. doi: 10.1097/CCM.0b013e31825334da
17. Semenza G. O2-regulated gene expression: transcriptional control of cardiorespiratory physiology by HIF-1. J Appl Physiol. (2004) 96:1173–7. doi: 10.1152/japplphysiol.00770.2003
18. Belaidi E, Morand J, Gras E, Pépin J, Godin-Ribuot D. Targeting the ROS-HIF-1-endothelin axis as a therapeutic approach for the treatment of obstructive sleep apnea-related cardiovascular complications. Pharmacol Ther. (2016) 168:1–11. doi: 10.1016/j.pharmthera.2016.07.010
19. Gauda E, Conde S, Bassi M, Zoccal D, Almeida Colombari D, Colombari E, et al. Leptin: master regulator of biological functions that affects breathing. Compr Physiol. (2020) 10:1047–83. doi: 10.1002/cphy.c190031
20. Patel A, Frikke-Schmidt H, Bezy O, Sabatini P, Rittig N, Jessen N, et al. LPS induces rapid increase in GDF15 levels in mice, rats, and humans but is not required for anorexia in mice. Am J Physiol Gastrointest Liver Physiol. (2022) 322:G247–55. doi: 10.1152/ajpgi.00146.2021
21. Ryan S, Arnaud C, Fitzpatrick S, Gaucher J, Tamisier R, Pépin J. Adipose tissue as a key player in obstructive sleep apnoea. Eur Respir Rev. (2019) 28:190006. doi: 10.1183/16000617.0006-2019
22. Kleessen B, Schroedl W, Stueck M, Richter A, Rieck O, Krueger M. Microbial and immunological responses relative to high-altitude exposure in mountaineers. Med Sci Sports Exerc. (2005) 37:1313–8. doi: 10.1249/01.mss.0000174888.22930.e0
23. Li Q, Xu T, Shao C, Gao W, Wang M, Dong Y, et al. Obstructive sleep apnea is related to alterations in fecal microbiome and impaired intestinal barrier function. Sci Rep. (2023) 13:778. doi: 10.1038/s41598-023-27784-0
24. Bannow L, Bonaterra G, Bertoune M, Maus S, Schulz R, Weissmann N, et al. Effect of chronic intermittent hypoxia (CIH) on neuromuscular junctions and mitochondria in slow- and fast-twitch skeletal muscles of mice-the role of iNOS. Skelet Muscle. (2022) 12:6. doi: 10.1186/s13395-022-00288-7
25. Gaucher J, Vial G, Montellier E, Guellerin M, Bouyon S, Lemarie E, et al. Intermittent hypoxia rewires the liver transcriptome and fires up fatty acids usage for mitochondrial respiration. Front Med. (2022) 9:829979. doi: 10.3389/fmed.2022.829979
26. Moulin S, Thomas A, Wagner S, Arzt M, Dubouchaud H, Lamarche F, et al. Intermittent hypoxia-induced cardiomyocyte death is mediated by HIF-1 dependent MAM disruption. Antioxidants. (2022) 11:1462. doi: 10.3390/antiox11081462
27. Laouafa S, Roussel D, Marcouiller F, Soliz J, Gozal D, Bairam A, et al. Roles of oestradiol receptor alpha and beta against hypertension and brain mitochondrial dysfunction under intermittent hypoxia in female rats. Acta Physiol. (2019) 226:e13255. doi: 10.1111/apha.13255
28. Hou Y, Yang H, Cui Z, Tai X, Chu Y, Guo X. Tauroursodeoxycholic acid attenuates endoplasmic reticulum stress and protects the liver from chronic intermittent hypoxia induced injury. Exp Ther Med. (2017) 14:2461–8. doi: 10.3892/etm.2017.4804
29. Belaidi E, Thomas A, Bourdier G, Moulin S, Lemarié E, Levy P, et al. Endoplasmic reticulum stress as a novel inducer of hypoxia inducible factor-1 activity: its role in the susceptibility to myocardial ischemia-reperfusion induced by chronic intermittent hypoxia. Int J Cardiol. (2016) 210:45–53. doi: 10.1016/j.ijcard.2016.02.096
30. Cai X, Li X, Jin S, Liang D, Wen Z, Cao H, et al. Endoplasmic reticulum stress plays critical role in brain damage after chronic intermittent hypoxia in growing rats. Exp Neurol. (2014) 257:148–56. doi: 10.1016/j.expneurol.2014.04.029
31. Iiyori N, Alonso L, Li J, Sanders M, Garcia-Ocana A, O’Doherty R, et al. Intermittent hypoxia causes insulin resistance in lean mice independent of autonomic activity. Am J Respir Crit Care Med. (2007) 175:851–7.
32. Favier F, Britto F, Freyssenet D, Bigard X, Benoit H. HIF-1-driven skeletal muscle adaptations to chronic hypoxia: molecular insights into muscle physiology. Cell Mol Life Sci. (2015) 72:4681–96. doi: 10.1007/s00018-015-2025-9
33. Arthur P, Giles J, Wakeford C. Protein synthesis during oxygen conformance and severe hypoxia in the mouse muscle cell line C2C12. Biochim Biophys Acta. (2000) 1475:83–9. doi: 10.1016/s0304-4165(00)00046-5
34. Etheridge T, Atherton P, Wilkinson D, Selby A, Rankin D, Webborn N, et al. Effects of hypoxia on muscle protein synthesis and anabolic signaling at rest and in response to acute resistance exercise. Am J Physiol Endocrinol Metab. (2011) 301:E697–702. doi: 10.1152/ajpendo.00276.2011
35. Buttgereit F, Brand MD. A hierarchy of ATP-consuming processes in mammalian cells. Biochem J. (1995) 312:163–7. doi: 10.1042/bj3120163
36. Belaidi E, Khouri C, Harki O, Baillieul S, Faury G, Briançon-Marjollet A, et al. Cardiac consequences of intermittent hypoxia: a matter of dose? A systematic review and meta-analysis in rodents. Eur Respir Rev. (2022) 31:210269. doi: 10.1183/16000617.0269-2021
37. Hazlehurst J, Lim T, Charlton C, Miller J, Gathercole L, Cornfield T, et al. Acute intermittent hypoxia drives hepatic de novo lipogenesis in humans and rodents. Metabol Open. (2022) 14:100177.
38. Burtscher J, Pasha Q, Chanana N, Millet G, Burtscher M, Strasser B. Immune consequences of exercise in hypoxia: a narrative review. J Sport Health Sci. (2023) 19:S2095-2546(23)00092-3. doi: 10.1016/j.jshs.2023.09.007
39. Savino W, Durães J, Maldonado-Galdeano C, Perdigon G, Mendes-da-Cruz D, Cuervo P. Thymus, undernutrition, and infection: approaching cellular and molecular interactions. Front Nutr. (2022) 9:948488. doi: 10.3389/fnut.2022.948488
40. Ludwig K, Huppertz T, Radsak M, Gouveris H. Cellular immune dysfunction in obstructive sleep apnea. Front Surg. (2022) 9:890377. doi: 10.3389/fsurg.2022.890377
41. Mohit, Pateriya A, Tomar M, Shrivastava A, Chand P. Immunometabolism: an evolutionary perspective in obstructive sleep apnea. Sleep Med Rev. (2022) 65:101668. doi: 10.1016/j.smrv.2022.101668
Keywords: chronic intermittent hypoxia, obstructive sleep apnea, nutritional status, anorexia, protein metabolism
Citation: Breuillard C, Moulin S, Bouyon S, Couchet M, Moinard C and Belaidi E (2023) Chronic intermittent hypoxia due to obstructive sleep apnea slightly alters nutritional status: a pre-clinical study. Front. Nutr. 10:1250529. doi: 10.3389/fnut.2023.1250529
Received: 30 June 2023; Accepted: 13 October 2023;
Published: 30 October 2023.
Edited by:
Owen Kelly, Sam Houston State University, United StatesReviewed by:
Dulce González-Islas, National Institute of Respiratory Diseases-Mexico (INER), MexicoLinyi Li, Capital Medical University, China
Copyright © 2023 Breuillard, Moulin, Bouyon, Couchet, Moinard and Belaidi. This is an open-access article distributed under the terms of the Creative Commons Attribution License (CC BY). The use, distribution or reproduction in other forums is permitted, provided the original author(s) and the copyright owner(s) are credited and that the original publication in this journal is cited, in accordance with accepted academic practice. No use, distribution or reproduction is permitted which does not comply with these terms.
*Correspondence: Charlotte Breuillard, Y2hhcmxvdHRlLmJyZXVpbGxhcmRAdW5pdi1ncmVub2JsZS1hbHBlcy5mcg==