- College of Bioscience and Biotechnology, Hunan Agricultural University, Hunan Provincial Engineering Research Center of Applied Microbial Resources Development for Livestock and Poultry, Changsha, China
Tryptophan (Trp) is an essential amino acid that can be metabolized via endogenous and exogenous pathways, including the Kynurenine Pathway, the 5-Hydroxyindole Pathway (also the Serotonin pathway), and the Microbial pathway. Of these, the Microbial Trp metabolic pathways in the gut have recently been extensively studied for their production of bioactive molecules. The gut microbiota plays an important role in host metabolism and immunity, and microbial Trp metabolites can influence the development and progression of various diseases, including inflammatory, cardiovascular diseases, neurological diseases, metabolic diseases, and cancer, by mediating the body’s immunity. This review briefly outlines the crosstalk between gut microorganisms and Trp metabolism in the body, starting from the three metabolic pathways of Trp. The mechanisms by which microbial Trp metabolites act on organism immunity are summarized, and the potential implications for disease prevention and treatment are highlighted.
1. Introduction
Tryptophan (Trp), one of the functional amino acids (FAAs) for mammals, can synthesize proteins and is also a precursor for a range of biologically active molecules (1). Trp is metabolized via the Kynurenine Pathway, the 5-Hydroxyindole Pathway (Serotonin pathway), and the Microbial pathway. The metabolites serotonin, melatonin, indole, and its derivatives are essential in regulating organismal immunity (2, 3).
The gut possesses a complex ecosystem that provides a habitat for numerous microbiota. Over a long evolutionary period, the gut microbiota and its host have mutually benefited and maintained certain homeostasis (4, 5). Dysbiosis of the intestinal ecology will lead to dysfunction accompanied by increased intestinal permeability, immune system abnormalities, and organismal inflammation (6, 7). These effects can be mediated directly through intercellular interactions. Besides, they can also be mediated by metabolites produced by microorganisms, the environment or host molecules (8). As a veritable endocrine powerhouse, the gut microbiota generates various bioactive molecules. These bioactive compounds can exert effects both locally, within the gut environment, and distally, throughout the body, eliciting a range of responses that can impact multiple organ systems (8). Disruption in the reciprocal communication between the host and the microbiota may incite or intensify the development of disease pathogenesis (9, 10), which includes the mediation of disease through the immune system. The indole and indole derivatives, such as indole-3-acrylic acid (IA), indole-3-propionic acid (IPA), indole-3-lactic acid (ILA), indole-3-aldehyde (IAld), and indole-3-acetic acid (IAA), act as essential signaling molecules within the microbial community, mediating host-microbial crosstalk to affect the body’s immune system causing the development of related diseases (11–13). It is worth mentioning that indole and indole derivatives metabolized by Trp can act as activators of aryl hydrocarbon receptor (AhR) or pregnane X receptor (PXR) signaling to regulate host immune responses (14, 15).
While several previous studies have comprehensively explored the impact of Trp metabolism and gut microbes on related diseases and the crosstalk between the two (16–22), there remains a notable gap in the literature regarding the relationship between microbial Trp metabolites and organismal immunity. To address this issue, we present a concise summary of recent discoveries regarding the gut microbiota’s role in Trp metabolism. This encompasses the direct conversion of Trp into bioactive molecules by the gut microbiota, as well as their modulation of Trp metabolism in various pathways. Additionally, we provide an overview of the potential function of microbial-derived Trp metabolites as signaling molecules in the communication between the gut microbiota and organismal immunity, as well as their involvement in the pathophysiology of related diseases. Overall, this review aims to elucidate the complex interactions between microbial Trp metabolism, gut microbiota, and organismal immunity that which have important implications for human health.
2. Tryptophan metabolism
2.1. Tryptophan uptake, absorption, and metabolism
Tryptophan, also referred to as β-indolylalanine, is an aromatic amino acid characterized by the presence of a β-carbon attached to the 3-position of the indole group. With a chemical formula of C11H12N2O2 (23), Trp is the largest molecular weight of the standard amino acids and the least stored within the cell, making it more susceptible to a deficiency under conditions of nutrient deprivation and increased catabolism (12). Poultry, fish, dairy, soybean, and other foods have high Trp content (24). Humans consume Trp in the form of both ingested protein and gut microbial secretion, and the majority of Trp is absorbed in the intestine. Dietary habits, hormones, geographic location, and stress are some factors that affect Trp absorption and utilization (25). In addition, the simultaneous intake of other neutral amino acids or carbohydrates can also limit the absorption and availability of Trp (26, 27). Studies have shown that Trp uptake in the brain is enhanced during intense physical activity, activation of the neurosympathetic system, lipolysis, and increased plasma levels of non-esterified fatty acid (NEFA) (28, 29). The body’s deficiency of Trp and related metabolites is associated with various diseases, including neurological disorders, inflammatory bowel disease (IBD), metabolic diseases, cardiovascular diseases, and depression (11, 30).
Tryptophan, a neutral amino acid, is absorbed through apical membranes in the small intestine by amino acid transporters, including ATB0+ (SLC6A14 gene), a symporter utilizing 2Na/1Cl−, and B0AT1 (SLC6A19 gene), Na-dependent transmembrane protein (2, 31). It is then transported into the portal circulation through basolateral membranes via TAT1 (SLC16A10 gene), a uniporter, or LAT2-4F2hc (SLC7A8-SLC3A2 gene) and LAT1-4F2hc (SLC7A5-SLC3A2 gene), antiporters (32–34). The insertion of these transporters into the cell membrane, the modulation of their activity, and an increase in substrate supply require their association with other molecules, such as ACE2, CD98/CD147, or aminopeptidase N. Nonetheless, comprehension of the factors that affect the expression of these receptors and proteins remains insufficient. After being absorbed into the circulation, Trp binds mainly to albumin (75–90%) (30). Trp enters the liver with the blood circulation for protein synthesis, and the rest of Trp continues to reach tissue cells throughout the body with the blood circulation for protein synthesis (35). Trp that does not undergo protein synthesis will enter various metabolic pathways, mainly through Endogenous Trp metabolism, including the kynurenine and the serotonin pathway (36, 37). Within these pathways, the formation of endogenous metabolites such as kynurenine (KYN), kynurenic acid (KYNA), 3-hydroxykynurenine (3-HOK), and 5-hydroxytryptophan (5-HTP) takes place (38, 39). In addition, a portion of Trp that does not enter the circulation will be metabolized and degraded by intestinal microorganisms (Bacterial Trp metabolism) (40), also known as exogenous Trp metabolism. Notably, exogenous metabolites indole and indole derivatives are produced during this process (39).
2.2. Kynurenine pathway
The kynurenine pathway (KP) is the primary catabolic route for Trp. Once Trp is transported to the bloodstream from the intestine, approximately 90% of the unbound Trp, which is not used for protein synthesis, undergoes catabolism through this pathway in the liver (41, 42). The enzymatic activities involved in the KP and the availability of Trp are crucial factors affecting this process. Specifically, the initial and rate-limiting step for the production of downstream metabolites involves the oxidation of Trp to N-formyl-kynurenine (Nfk) by indoleamine 2,3-dioxygenase 1 and 2 (IDO1, IDO2) as well as Trp 2,3-dioxygenase (TDO). IDO and TDO are subject to stabilization by its substrate, leading to reduced inactivation rates (43–45). IDO and TDO are tissue specific. The IDO is present in various organs including the brain, gastrointestinal tract and liver, and is related to the body’s inflammatory response. IFN-γ, TNF-α, and IL-6, inflammatory cytokines effectively induce IDO activity. TDO is expressed almost exclusively in the liver, and the hypothalamic–pituitary–adrenal axis positively affects TDO expression through glucocorticoids, and to a lesser extent in the brain (45–47). Under the mediation of arylformamidase, N-formylkynurenine is catalyzed to kynurenine concisely. Kynurenine (KYN) can form kynurenic acid (KYNA) in the presence of kynurenine aminotransferase (KAT) (42, 48).
In addition to this, kynurenine can be formed to form 3-hydroxykynurenine (3-HOK) catalyzed by kynurenine 3-monooxygenase (KMO). 3-HOK can be converted to xanthurenic acid (XA) catalyzed by KAT. In addition, 3-hydroxyanthranilic acid (3-HAA) can also be formed under the catalysis of Kynureninase (49, 50). 3-HAA is metabolized in three ways. (1) As one of the most critical products of the KPquinolinic acid (QUIN) is formed from 3-HAA catalyzed by 3-HAA oxygenase (36). QUIN can be converted to NAD as the end product of the kynurenine-quinolinic acid pathway (17). (2) 3-HAA is catalyzed by 3-hydroxyanthranilate 3,4-dioxygenase to form 2-amino-3-carboxymuconate semialdehyde, and then in 2-amino-3-carboxymuconate semialdehyde carboxy-lyase catalyzed formation of Picolinic acid (51). (3) Conversion of 2-amino-3-carboxymuconate semialdehyde to acetyl CoA (complete oxidation) (52, 53). Among the three possible sub-metabolic pathways of KP, Trp is completely oxidized to carbon dioxide and water via acetyl CoA for the majority of reasons that we will not discuss here.
2.3. 5-Hydroxyindole pathway
The Serotonin Pathway is another pathway involved in the endogenous metabolism of Trp. Serotonin (5-hydroxytryptamine, 5-HT) is a monoamine molecule that is primarily synthesized in mammals through the catabolism of Trp (54). 5-HT, a key inhibitory neurotransmitter, is found in high levels in the cerebral cortex and synapses, where it is involved in mood control, regulation of sleep and pain, etc. (55, 56). In addition, 5-HT acts as a strong vasoconstrictor and smooth muscle contraction stimulator in peripheral tissues. Initially, Trp hydroxylase (TPH)-1/2 converts Trp to 5-hydroxytryptophan (5-HTP). Enterochromaffin cells located in the gastrointestinal tract express the enzyme TPH-1, and serotonergic neurons in both the central nervous system (CNS) and enteric nervous system (ENS) express the enzyme TPH-2 (17, 37, 57). Serotonin is formed from 5-HTP by the catalytic action of aromatic amino acid decarboxylase. In the intestine, TPH-1 catalyzes the production of serotonin, which can act locally or be transported by platelets to various distal sites, such as the liver, bones, and cardiovascular system (58). Although 5-HT is essential in maintaining the normal function of the central and peripheral nervous system, it cannot cross the blood–brain barrier under physiological conditions (59). 5-HT catalyzed by TPH-2 is produced in the CNS and periphery in small but significant amounts to act locally (60). Melatonin is metabolically synthesized from Trp in the pineal gland or the EC in the gastrointestinal tract (61). Trp is converted to 5-HT by the catalytic action of various enzymes. Initially, 5-HT is converted to N-acetyl-5-hydroxytryptamine catalyzed by serotonin-N-acetyltransferase, then to melatonin catalyzed by acetylserotonin O-methyltransferase (62). Besides that, Monoamine oxidase (MAO) breaks down 5-HT through catabolism, resulting in the formation of 5-hydroxyindole (5-HI) acetaldehyde. Subsequently, aldehyde dehydrogenase further metabolizes the acetaldehyde to form 5-HI acetic acid (5-HIAA), which is eliminated from the body through urine excretion (63) (Figure 1).
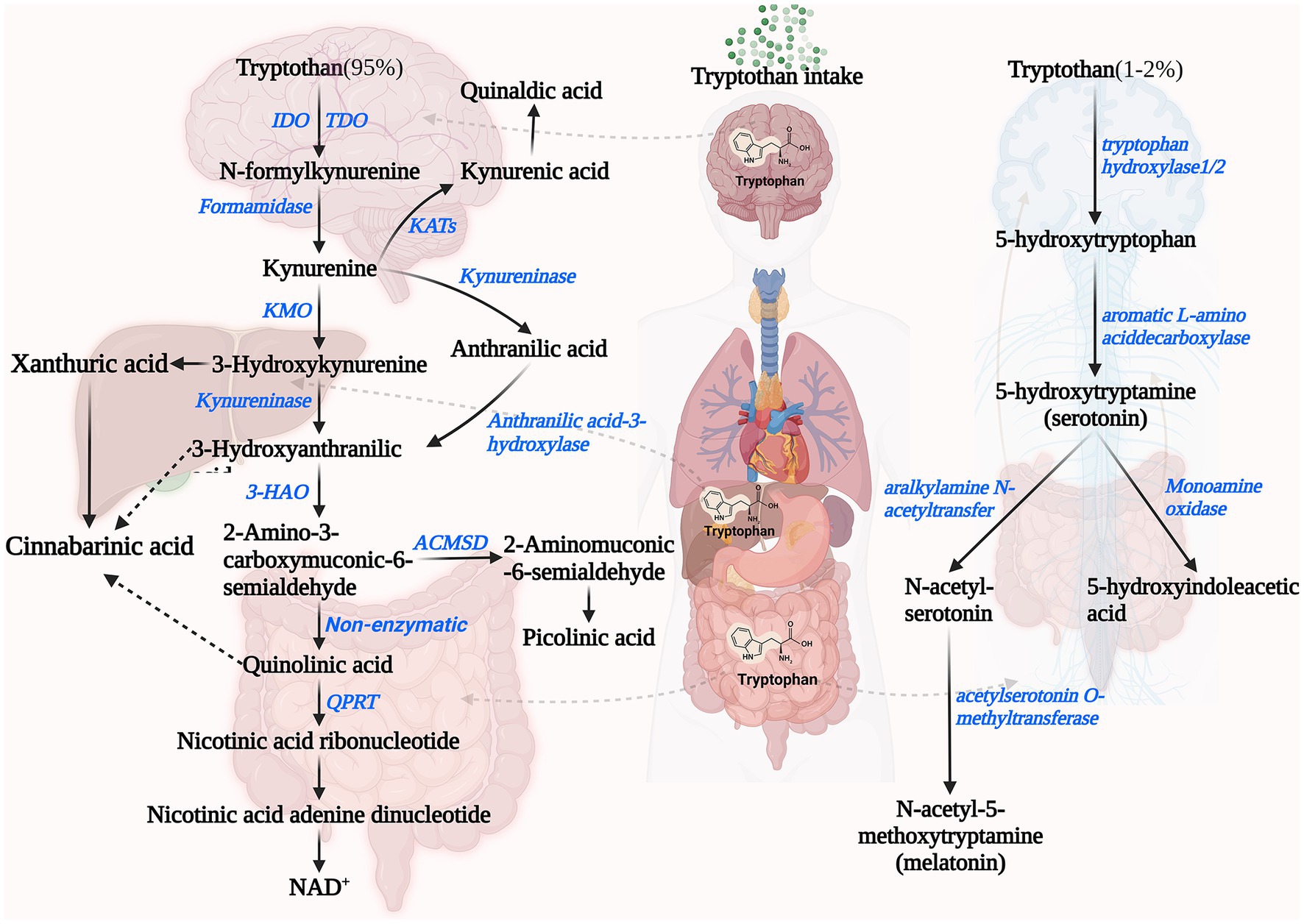
Figure 1. Endogenous pathways of tryptophan metabolism including Kynurenine Pathway (left) and 5-Hydroxyindole Pathway (right). IDO, indoleamine 2,3-dioxygenase; TDO, tryptophan 2,3-dioxygenase; KMO, kynurenine 3-monooxygenase; KAT, kynurenine aminotransferase; 3-HAO, 3-hydroxyanthranilicacid 3,4-dioxygenase; ACMSD, 2-amino-3-carboxymuconate-6-semialdehydedecarboxylase; QPRT, quinolinate phosphoribosyltransferase; and NAD+, nicotinamide adenine dinucleotides+.
2.4. Microbiome pathways
In addition to the 5-HT pathway and KP, a small fraction of Trp enters the large intestine to be metabolized by microorganisms. The metabolic breakdown of Trp is proportional to intake, carbohydrate consumption and colonic pH (11, 64). As carbohydrate substrates are progressively depleted along the colon from proximal to distal regions, bacterial metabolism shifts from utilizing carbohydrates to proteins via fermentation. The transition leads to a notable rise in phenolic compound levels, resulting from the breakdown of Trp in the gut content, which are considerably more abundant in the distal colon compared to the proximal colon (65).
In Microbiome Pathways, Trp is degraded by intestinal microorganisms to indole and its derivatives, including IAA, IAld, IPA, IA, Indole-3-Acetamide (IAM), etc., which is a complex process and accompanied by many enzymatic reactions. Microbiome Pathways can be broadly classified into four pathways (Figure 2). Path I: Trp is converted to IAM (by Tryptophan 2-monooxygenase) and then to IAA (by indole-3-acetamidehydrolase) (9, 28). Path II: Trp is converted to tryptamine and then to Indole-3-acetaldehyde(IAAld) by Tryptophan decarboxyolase, Diamine oxidase, and MAO. IAAld can be converted to Tryptophol or IAA catalyzed by Indole-3-acetaldehydeoxidase. IAA can be converted to skatole (by Indoleacetate dexarbaxylase) or IAId (28). Path III: Trp gradually transforms to Indole-3-Pyruvate(IPyA; by Aromatic amino acid aminotransferase), ILA (by phenyllactate dehydrogenase), and IA (by phenyllactate dehydratase), and eventually to IPA(by acyl-CoA dehydrogenase) (9, 40). Path IV: Trp is catalyzed by Tryptophanase to indole, which can be further converted to Indoxyl sulfate (I3S) (13).
Many intestinal microorganisms are involved in metabolism, and most mediate metabolism by forming the different types of enzymes required for the pathway (66). One of the most well-studied microorganisms is Escherichia coli (E. coli). In E. coli, the indole pathway is regulated by a transcriptional regulator called tryptophanase (TnaA), which catalyzes the conversion of Trp to indole and pyruvate (67). Additionally, E. coli also possesses an indole importer (YhcY) and an efflux pump (MdtEF), which regulate the intracellular concentration of indole and control its secretion (68). Another microorganism involved in the indole pathway is Clostridium sporogenes (69). Trp is converted to indole by the enzyme tryptophanase in this microorganism, which is then further metabolized to IAld by an aldehyde dehydrogenase. IAld can then be oxidized to IAA by an oxidoreductase or converted to tryptamine by a decarboxylase. Several other microorganisms, including Bacteroides thetaiotaomicron, Proteus vulgaris, and Pseudomonas aeruginosa, have also been shown to possess enzymes involved in the indole pathway of Trp metabolism (70–73). Furthermore, Pseudomonas aeruginosa has been found to have a tryptophanase enzyme regulated by a two-component system, which is vital for regulating virulence (74).
3. Crosstalk between intestinal microbes and tryptophan metabolism
An increasing body of literature suggests that Trp catabolites, produced by the gut microbiota, play a critical role as signaling molecules in both microbial communities and communication between hosts and microbes (9). These metabolites may have a role in maintaining immune system stability within the body (75). As seen previously, bacterial Trp metabolites include indoles and indole derivatives, most of which are produced by intestinal microorganisms, including indole, IAA, IA, IPA, tryptamine, and skatole. Bacterial Trp metabolites can act as potent bioactive compounds to mediate organismal immunity. Metabolites can affect immune cells and intestinal barrier integrity by activating human receptors such as PXR or AhR, both highlighted in subsequent sections (9, 65). Different bacteria produce different enzymes to catalyze different metabolites. Since the production of some metabolites requires multiple enzymatic reactions to produce them, multiple bacterial mediators are required (Table 1).
In addition to Bacterial Trp metabolism, numerous studies have shown that Endogenous Trp metabolism is also mediated by microorganisms (41, 65). Serotonin is one of the critical products of Endogenous Trp metabolism and is mainly released from intestinal chromogranin cells (103). It has been demonstrated to function as an intestinal signaling molecule, conveying signals from the gut to intrinsic or extrinsic neurons and influencing intestinal peristalsis, motility, secretion, vasodilation, and nutrient absorption (104–107). These effects suggest a potentially significant role in the regulation of gastrointestinal physiology (104). Investigations employing germ-free mice have demonstrated a noteworthy reduction in the levels of serotonin present in both the colon and blood (108). A further investigation utilizing germ-free mice colonized with specific pathogen-free fecal microbiota has shown that commensal microbiota synthesizes serotonin within the gut lumen by deconjugating glucuronide-conjugated serotonin, which is excreted via the bile duct through a β-glucuronidase-dependent mechanism (109). This discovery emphasizes the significant role of commensal microbiota in regulating gut serotonin levels, which can be modulated in multiple ways. Studies have revealed that commensal bacteria promote serotonin biosynthesis in colonic enterochromaffin cells through a mechanism involving metabolites and cell components (110, 111). Hafnia alvei (NCIMB, 11999), Escherichia coli K-12, Lactobacillus plantarum FI8595, L. lactis subsp. lactis IL1403, Klebsiella pneumoniae (NCIMB, 673), Morganella morganii (NCIMB, 10466), Lactococcus lactis subsp. cremoris MG1363, and Streptococcus thermophilus NCFB2392 have been reported to produce serotonin by expressing Trp synthetase (37, 112). Moreover, several other factors have been found to modulate the gut microbiota’s effects on serotonin production. For instance, dietary components such as fiber have been shown to stimulate serotonin production by promoting the growth of specific gut bacteria (113). Microbial biotransformation of bile acids changes secondary bile acids to deoxycholic acid inducing 5-HT synthesis (114). Similarly, alterations in the gut microbiota composition resulting from disease, medication use, or other factors can impact serotonin biosynthesis (105, 115, 116).
As the most critical enzyme in KP, IDO, the effect of the gut microbiota on IDO1 activity has been extensively studied (53, 117). Germ-free mice with a deficient intestinal microbiota reduce IDO1 activity, and antibiotic treatment of mice with established microbiota results in a similar reduction in IDO1 activity (118). It is worth noting that introducing some gut microbes (e.g., Bifidobacterium infantis) can normalize the kynurenine-to-tryptophan ratio (119). This is due to the ability of the intestinal microorganisms to degrade Trp into a variety of metabolites (Trp microbial pathway), thereby limiting tryptophan metabolism in the KP and serotonin pathways (59). Besides that, intestinal microorganisms indirectly regulate IDO1 activity through the metabolite butyrate, a short-chain fatty acid (SCFA), which modulates the kynurenine pathway (120). Butyrate serves as the primary energy source for enterocytes and can downregulate intestinal IDO expression through two mechanisms. First, it inhibits the expression of STAT1 (121), which reduces the transcriptional activity of IDO. Second, it acts as a histone deacetylase inhibitor to suppress IDO transcription, thereby inhibiting kynurenine production from Trp (122). These researches demonstrate that gut microbes and their metabolites are essential regulator of the KP.
4. AHR and PXR recognize microbial pathway tryptophan metabolites
4.1. Aryl hydrocarbon receptor signaling pathway
The AHR is a transcription factor that regulates various physiological and pathological processes in living organisms, including detoxification, metabolism, cell proliferation, differentiation, inflammation, and immune responses (123). AHR is typically present in the cytoplasm as part of a protein complex, which includes the AhR-interacting protein Ara9, the c-SRC protein kinase (c-SRC), the 90-kDa heat shock protein (HSP90), the 23 kDa heat shock protein (p23) and AHR, when not bound to a ligand (123). Ligand binding induces a conformational change in AHR, exposing it to protein kinase C-mediated phosphorylation and promoting its nuclear translocation, where it dissociates from the protein complex and rapidly interacts with the AhR nuclear translocator (ARNT) (124). The AhR/ARNT heterodimeric complex binds to specific DNA sequences, known as dioxin-responsive elements (DREs) or xenobiotic-responsive elements (XREs), located at 5’-TNGCGTG-3′, to regulate gene transcription (125).
The most frequently mentioned genes are several genes involved in xenobiotic metabolism encoding for microsomal cytochrome P450-dependent monooxygenases. These include CYP1A1, CYP1A2, CYP1B1, and NAD(P)H-quinone oxidoreductase (126). These enzymes play a critical role in the metabolism of xenobiotics, such as environmental toxins, drugs, and carcinogens, by catalyzing their oxidation and conjugation with endogenous molecules to facilitate their elimination from the body (127). Furthermore, the AhR signaling pathway is crucial for the regulation of immunity. AhR activation regulates the differentiation, migration, and activation of immune cells, the production of inflammatory factors, and helps maintain immune homeostasis (128) (Figure 3).
4.2. Microbial TRP metabolites as AHR ligands
Aryl hydrocarbon receptor is a remarkably versatile nuclear receptor with the ability to bind to a wide range of diverse ligands. The ligands of AHR include endogenous ligands (Kynurenine, 6-Formylindolo [3,2-b]carbazole, and Tryptamine, etc.) and exogenous ligands (Benzo [a]pyrene and Indole-3-carbinol, 2,3,7,8-Tetrachlorodibenzo-p-dioxin, TCDD; etc.) (123, 129). AHR receptors involved in Microbiome Trp metabolism include IA, IAA, IAAld, IAId, and Tryptamine, which are important sources of receptors.
4.3. AHR and organismal immunity
Aryl hydrocarbon receptor is highly expressed in innate and adaptive immune cells, and recent investigations have elucidated its significant involvement in immune regulation (130). The role of AhR in regulating adaptive immunity is primarily discussed with respect to B cells and T cells (123). Experimental findings indicate that AhR-deficient mice expression display a marked increase in the overall population of B220 cells within their bone marrow compared to their wild-type counterparts (131). This effect was attributed to an increase in the number of pro/pre-B cells and immature B cells, without impacting the total number of mature B cells (131). Moreover, the potential contribution of AhR to B-cell malignancies has been examined (132). To summarize, AhR modulates B cell proliferation, differentiation, and the development of malignancies.
Aryl hydrocarbon receptor has different effects on the development and function of different T cells. AhR binding to ligands is activated to promote Tregs/Th17 cell differentiation in a ligand-specific manner (133). For example, TCDD-induced activation of AhR promotes the generation of Tregs (134); FICZ promotes the generation of Th17 cells (134). In addition to this, the AhR signaling pathway can promote the differentiation of T helper 17(Th17) cells by limiting the activation of STAT-1 and STAT-5 (135, 136), thereby reducing the expression of Th2 cytokines and modulating the Th1/Th2 balance (137).
In AhR-mediated innate immunity, Macrophages, Dendritic cells, and Lymphoid Cells (ILCs) are significantly affected. Notably, AhR exerts a negative regulatory effect on the production of proinflammatory cytokine IL-6 by inhibiting histamine production in macrophages stimulated by lipopolysaccharide (LPS) (138). Furthermore, ILCs express AhR and its genetic disruption can cause functional defects in ILCs and loss of specific subpopulations (139). Specifically, AhR is necessary for the activation of certain types of ILCs, such as liver-resident NK cells, and for the secretion of cytokines and anti-microbial peptides by ILC3s in the gastrointestinal tract (137). In addition, AhR controls DC differentiation, function and antigen presentation with profound effects on T-cell immunity (25). Overall, these studies suggest that AhR affects organismal immunity by directly and indirectly influencing the maturation and function of multiple immune cells.
In general, the AhR signaling pathway is widely recognized as a critical component of the immune defense mechanism at barrier sites (65, 140). This pathway is crucial in maintaining intestinal homeostasis by regulating various processes, including barrier stability, and immune cell function (141). However, Microbial metabolism has a dominant influence on the activity of AhR in the intestine. In the next section, the effects of gut microbial Trp metabolites on organismal immunity are explicitly described, with the AhR signaling pathway, which is activated with gut microbial Trp metabolites as ligands, occupying an important position.
4.4. Pregnane X receptor
Similar to the AhR, the pregnane X receptor (PXR) serves as a prominent target for the interaction with microbial tryptophan metabolites. PXR also belongs to a family of nuclear receptors (NR1I2) (142),which plays a crucial role in regulating the expression of genes involved in both drug and endobiotic metabolism, including those encoding UDP-glucuronosyl-transferases, cytochrome P450s (CYPs), drug efflux pumps, and glutathione-S-transferases (143–145). The PXR is a major regulator of the expression of the CYPs isoform, which metabolizes more than half of all human drugs (146). Therefore, PXR activation protects the body from exogenous or endogenous toxic substances and plays an essential role in the process of detoxification. PXR is expressed in a variety of cells including intestinal epithelial cells, immune cells (B cells, T cells, dendritic cells, etc.), and hepatocytes (147, 148). Activation of PXR occurs upon binding of ligands to its ligand-binding domain (LBD), leading to the formation of a heterodimeric complex with the retinoid X receptor (RXR), subsequently initiating gene transcription. PXR serves as an essential regulator of intestinal epithelial barrier function, and PXR deletion or PXR low expression decreases intestinal homeostasis and increases intestinal inflammation (149, 150).
The microbial Trp metabolites indole and indole derivatives including skatole, IAA, and IPA have been extensively studied as PXR ligands. IPA activates its receptor PXR by binding directly to the genomic region of PXR or by indirectly crosstalk with other transcription factors, such as AHR (151). These transcription factors control many genes involved in transport, inflammation, apoptosis, and oxidative stress (152). For example, PXR reduces the secretion of proinflammatory cytokines and controls inflammation by inhibiting the NF-κB signaling pathway (153, 154). In Addition, indole and IAM induced PXR regulatory genes CYPs and MDR1 in human intestinal cancer cells. I3S exerts cytostatic properties via the PXR in breast cancer models (151, 155). Clarifying the microbial Trp metabolite mediated PXR pathway would further shed light on the mechanisms that govern the metabolic cross-talk between host and microbiome with regard to Trp.
5. Microbial tryptophan metabolites and immunity
Recent studies have highlighted the crucial role of Trp catabolites produced by the gut microbiota in serving as signaling molecules within the microbial community and host–microbe interactions (65). In turn, this significantly contributes to maintaining immune system homeostasis in the body (Figure 4).
5.1. Regulation of Trp metabolites from gut microbiota in the innate immunity
The innate immune system consists of skin and mucous membranes, and immune cells (neutrophils, dendritic cells, macrophages and NK cells, etc.) (156). Non-specific immunity plays a vital role in the immune system as it rapidly responds to a broad spectrum of pathogens even without prior exposure (157). In this section, the effects of microbial Trp metabolites on non-specific immune cells and the regulation of cytokine (produced by non-specific immune cells) expression are discussed.
The microbial Trp metabolite indole and its derivatives can directly regulate the growth and differentiation of non-specific immune cells (65). For example, IAld and IAA have been shown to promote the differentiation of monocytes into dendritic cells, whereas IAA also enhances the phagocytic activity of human neutrophils and macrophages (158, 159). Moreover, indole derivatives also facilitate the differentiation of tolerogenic dendritic cells, leading to the generation of regulatory T cells that suppress the inflammatory response. These notable effects are mediated through the activation of the AHR in dendritic cells, thereby triggering the upregulation of genes associated with tolerogenic dendritic cell functionality and the production of immunomodulatory cytokines (160–162). NK cells are also a critical component of the innate immune system that is involved in defending against viral infections and tumors. Several recent studies have revealed that IAld can activate human NK cells and enhance their ability to kill cancer cells (163–165). Moreover, tryptamine has been found to activate the AHR pathway in NK cells, resulting in an augmented cytotoxicity toward tumor cells (166–168). Overall, these studies suggest that microbial Trp metabolites have a major impact on the formation and function of many non-specific immune cells and might be involved in the regulation of immune responses.
In addition to direct modulatory effects on non-specific immune cells, numerous studies have shown that microbial Trp metabolites can mediate the inflammatory response by modulating the expression of cytokines during the infection phase, with a particular emphasis on the pivotal role played by macrophages (167, 169). Macrophages, derived predominantly from bone marrow monocytes, represent a critical subset of immune cells in the body (170). As one of the most important parts of innate immunity, M1 macrophages can be induced by IFN-γ, LPS or FFA to promote inflammatory responses, kill intracellularly infected pathogens and fight tumors by releasing inflammatory cytokines such as IL-1, IL-12, and TNF (171). M2 macrophages are capable of synthesizing and releasing anti-inflammatory factors (TGF-β, IL-10, etc.), immunosuppressive factors and various tumor-promoting cytokines, which can suppress inflammatory responses and promoting tumor cell growth and metastasis (172). Numerous studies have shown that microbial Trp metabolites can suppress the inflammatory response in macrophages during the infection phase. In patients with NAFLD, IAA attenuated the effect of TNF-α secreted by macrophages on bile acid metabolism, which reversed the ratio of CDCA to CA increased by FFA and macrophage inflammatory factors (123, 173). Similar results were found in the liver of mice, where IAA attenuated HFD-induced hepatotoxicity in a dose-dependent manner. It inhibited the expression of inflammatory factors such as IL-1β, IL-23, TNF-α, MCP-1, IL-17A, and IL-6, while elevating the level of the anti-inflammatory factor IL-10. The reduced pro/anti-inflammatory cytokines ratio was also reduced (14, 174, 175). In addition to this, IPA can reduce serum inflame, matory factors TNF-α and IL-1β (176). Similarly, indole has a similar function while decreasing the inflammatory cytokine expression and increasing the anti-inflammatory cytokine expression (177). Collectively, these investigations have shown that indole and indole derivatives of microbial Trp metabolites such as IPA and IAA can reduce the expression of proinflammatory factors in a dose-dependent manner and help regulate the inflammatory response.
The regulation of cytokines by microbial Trp metabolites is mainly mediated through AHR. It was found that macrophage TNF expression was reduced in response to co-stimulation by LPS and the AhR ligands IAld and IAA. In which IAld leads to a decrease in inflammatory vesicle pathway and IL-6 signaling capacity that reduces TNF transcription (178); IAA neutralizes free radicals, thus attenuating the inflammatory response of RAW264.7 macrophages to LPS and increasing IL-8 signaling (179). Similarly, indole-3-methanol suppressed the generation of LPS-mediated inflammatory cytokines such as IL-6 in bone marrow-derived macrophages (BMM) of mice (180). Tryptamine decreased the mRNA expression levels of IL-7 and IL-6 in LPS-stimulated RAW 6.264 cells via AHR (175, 181). Microbial Trp metabolite ligands of AhR affect the metabolic and immunomodulatory processes of nonspecific immune cells. Pathways such as oxidative phosphorylation, fatty acid β-oxidation or amino acid degradation are upregulated in most cases.
5.2. Regulation of Trp metabolites from gut microbiota in the adaptive immunity
Apart from innate immunity, microbial Trp metabolites are believed to have a significant impact on adaptive immunity. Specifically, the focus is on the role of T cells in the adaptive immune response, which recognize and respond to specific antigens (182). Upon activation, T cells differentiate into different subtypes, such as Th cells, cytotoxic T cells, and regulatory T cells, each with a distinct function in regulating the immune response (183). Several studies have reported that indole derivatives can regulate the differentiation, activation, and proliferation of T cells, thereby modulating their function.
Indole derivatives can regulate T-cell differentiation by modulating the expression of cytokines and transcription factors. For example, the indole derivative Indole-3-carbinol (I3C) has been shown to promote Th1 cell differentiation by upregulating the expression of interferon-γ and T-bet transcription factors (184). This is crucial in the defense against the intracellular pathogens (e.g., viruses and bacteria) (185, 186). Moreover, I3C inhibit the differentiation of Th2 cells by downregulating the expression of interleukin-4-related genes and GATA3 transcription factors (184), which mediate the development of allergy and asthma (186, 187). Another indole derivative, Indole-3-propionic acid (I3S), has also exhibited the ability to regulate T-cell activation and proliferation. I3S suppresses the expression of CD25 and CD69 surface markers (188), which are critical indicators of early T-cell activation and proliferation (189, 190). Besides, I3S reduced the frequency of IL-4-producing CD4 T cells and inhibited Th2 differentiation (191). This effect was attributed to the suppression of STAT5 and STAT6 phosphorylation, which are transcription factors involved in Th2 differentiation. I3S can also induce T cell apoptosis, or programmed cell death, by upregulating the expression of pro-apoptotic proteins (192). Other indole derivatives, IAld and IPA, have also been shown to modulate immune responses by regulating T-cell function (193, 194). IAld induces T-cell apoptosis and inhibits T-cell activation by regulating the expression of pro-and anti-apoptotic proteins (195).
Most notably, T-regulatory (Treg) and T-helper 17 (Th17) cells are key T-cell lineages that predominate at mucosal sites, including the gastrointestinal tract (196). Tregs play a pivotal role in inducing immune tolerance via cytokine secretion and modulation of antigen-presenting cell or effector lymphocyte function, making them critical cellular determinants of immune homeostasis in most tissues (197). In the thymus, “natural” Tregs (nTregs) develop when T lymphocytes tightly bind to self-peptide–MHC complexes on thymic stromal cells, and FOXP3 expression is induced. In the periphery, “induced” Tregs (iTregs) arise when Antigen-presenting cells activate Th cells in the presence of IL-2 and TGF-β (198). Autoimmune disorders and IBD are associated with dysregulation of the Treg population in circulation and afflicted tissue (196). Th17 cells develop following the activation of Th cells in the presence of inflammatory cytokines like IL-1β, IL-6, and IL-23, coupled with TGF-β. Mouse models have demonstrated the essential role of Th17 cells and their secreted cytokines in the pathogenesis of IBD, with elevated levels found in affected individuals (199). Notably, the Th17 response and inhibition of Tregs are considered significant contributors to the development of aberrant inflammation in the GI tract (200). Numerous studies have shown that the microbiota Trp metabolite indole and indole derivatives modulate Treg/Th17 differentiation, suggesting that the persistence of indole promotes tolerance and suppresses colonic inflammation (201). For example, I3C treatment decreases the occurrence of colitis by reducing Th17 cells and increasing Tregs (202). Besides that, I3C increased the production of CD4Foxp3 cells in mice and ultimately reduced CD4 IL-17 cells that induce neuroinflammation (203). IAA and IPA promote Treg differentiation and function by upregulating the expression of Foxp3 and other Treg-related genes (14, 40, 204). IAA also promotes Tregs differentiation by activating the AHR pathway (14).
The AhR pathway has been described in detail above as a key pathway involved in regulating the immune response by several ligands, including indole derivatives. For example, I3S inhibited Th2 differentiation by suppressing the phosphorylation of the related transcription factors STAT5 and STAT6 (191). However, the effect of I3S on Th2 was inhibited by the AhR antagonist α-naphthoflavone, which laterally suggests that the regulation of Th2 differentiation by I3S is AhR-dependent (191). Thus, boosting circulating AHR ligands, whether from endogenous or exogenous sources, is crucial for managing inflammatory diseases in various tissues. Furthermore, L. reuteri-produced Trp catabolites ILA or IAAld activated AhR and enhanced IL-17 production by T cells (205). This activation of AhR by ILA derived from Trp metabolism leads to the downregulation of Thpok and the reprogramming of CD4 intraepithelial lymphocytes (IELs) into double-positive (DP) IELs (96), highlighting an AHR-mediated mechanism distinct from AhR’s impact on regulatory T cells (Tr1, Tregs), intraepithelial γδ T cells and DCs (96). Moreover, tryptamine has been shown to activate mechanistic target of rapamycin (mTOR) in Treg cells in vitro, induce increased expression of p4EBP1 in effector memory T (Tem) cells, and enhance phosphorylated ribosomal pS6 expression in Tem cells (206), suggesting that exogenous tryptamine promotes glycolysis in TC (cytotoxic) T cells, thereby influencing the regulation of CD4 T cell function (207). Indole derivatives can modulate immune function and maintain immune homeostasis through their effects on Th1, Th2, and Treg cells as well as the AhR pathway (15, 208). Understanding the role of indole derivatives in T-cell regulation may lead to developing new therapies to treat immune-related diseases.
In addition to their effects on T lymphocytes, indole derivatives have been shown to have inhibitory and stimulatory effects on B cells. I3C can regulate B-cell function by inhibiting the production of immunoglobulins IgM and IgG in response to various stimuli. Reducing the expression of CD69, a marker of B-cell activation, induces B-cell apoptosis and inhibits B-cell proliferation (209, 210). Tryptamine has also been shown to stimulate IgA production by B cells and to activate the transcription factor AhR to regulate B cell function (211, 212). In conclusion, indole derivatives with inhibitory and stimulatory effects on B cells may have the potential as immunomodulators for treating immune diseases. However, further research is needed to fully understand the mechanisms of action of these compounds and their potential therapeutic applications.
5.3. Microbial Trp metabolites maintain intestinal barrier function and mucosal integrity
Intestinal microbial homeostasis and intestinal barrier integrity are closely linked with intestinal immunity. The intestinal barrier comprises the epithelial and mucus barriers, consisting of a layer of epithelial cells. Both Tight junction (TJ) and adherens junction (AJ) proteins play an essential role in this regard (213). TJ proteins, such as ZO1 and occludin, act as a protective barrier at the apical region of neighboring epithelial cell membranes (214). Their role is to prevent the unregulated diffusion of molecules between cells, thereby maintaining the integrity of the cell–cell barrier. On the other hand, AJ proteins like E-cadherin and β-catenin, located basolaterally but subjacent to TJs, play a crucial role in adhesive forces between adjacent epithelial cells (215). These proteins aid in sealing the intestinal barrier, ensuring its integrity. The intestinal barrier integrity is maintained through the collaboration of numerous factors, including mucus proteins, active molecules, and immune factors (216). However, disruptions to these factors may lead to various conditions, such as diabetes, asthma, autism, acne, allergies, and several intestinal disorders, including inflammatory bowel disease (IBD) (217, 218). A properly functioning gut-organ axis relies on a healthy intestinal microbiota structure and an integrated intestinal barrier. Disturbances to the gut microbiota composition have been associated with disruptions in intestinal homeostasis and intestinal barrier dysfunction (219). Therefore, regulating the interaction between the intestinal microbiota and the intestinal barrier presents a new approach to treating some intestinal diseases. The function of intestinal microbial Trp metabolites in maintaining the intestinal barrier and mucosal integrity has been extensively studied.
Indole derivatives, including indole-3-ethanol (IEt), IPyA, and IAld, have been shown to regulate the integrity of the intestinal barrier by modulating TJs and AJs, which together form the apical junction complex, ultimately reducing the incidence of DSS colitis in mouse models (100, 220). Treatment with IAld, IEt, and IPyA in mice inhibited the expression of TNFR1 during DSS colitis and prevented TJ and adherens junction complex (AJC) catabolism caused by activation of NF-κB by TNFα (93, 221–223). Furthermore, these metabolites protect the intestine from AJC destruction caused by proinflammatory cytokines by promoting the intestinal epithelial cells IL-10R expression (224, 225). Similarly, in the colitis model, oral administration of indole-containing capsules enhances the expression of molecules associated with TJs and AJs in colonic epithelial cells of germ-free mice, thus mitigating epithelial injury (226). Indole is known to fortify the epithelial barrier by inducing the upregulation of genes associated with the functions of TJs, AJs, actin cytoskeleton, and mucin production, among others (177). From the above studies, it can be hypothesized that microbial Trp metabolites have multiple targets in vivo, but their actions are synergistic. Highly relevant to the intestinal epithelium, and interacting directly with the microbiota, they work together to maintain intestinal health and immune function. Regulation of TJ by indole derivatives can be mediated by mediating gene expression. IPA and ICOOH treatment increased the gene expression of occludin, ZO-1, and MUC-2 in IECs (227, 228), and also reduced the expression of LPS-induced inflammatory factors such as IL-1β and IL-8, ultimately decreasing the permeability between the intestinal barriers. Administration of IEt, IPyA, and indole-3-carboxaldehyde increased the expression of genes such as ocludin and ZO-1, thereby enhancing the adhesion between adjacent epithelial cells (220). The findings of these studies collectively demonstrate that bacterial TRP metabolites promote TJ protein expression and reduce proinflammatory cytokine expression.
IL-22 plays a key role in maintaining intestinal epithelial integrity and antimicrobial defense, in addition to enhancing intestinal stem cells (ISC)-mediated regeneration and proliferation of epithelial cells. The primary producers of IL-22 in the intestine, a process controlled by microbiota, are innate ILCs and ILCs that express the nuclear hormone receptor RORt (229, 230). Symbiotic microbiota inhibits IL-22 production by ILCs in the healthy mouse intestine by expressing IL-25 by epithelial cells (231). In addition, the microbiota has been shown to modulate IL-22 production via indole and indole derivatives. Treatment with IAld alleviated dextran sulfate sodium (DSS)-induced colitis in mice, improved the reduction in body weight and colonic length, and restored damage to the jejunum and colon (232). IAld activation of AHR prompts lamina propria lymphocytes (LPLs), a type of Lymphoid Cells (ILCs), to secrete IL-22. IL-22 enhances intestinal epithelial regeneration and proliferation by inducing phosphorylation of STAT3 and accelerating ISC differentiation (100). Furthermore, it has been shown that IL-22-mediated STAT3 activation reduces crypt damage and stimulates crypt formation (233). Ultimately, the proliferation of intestinal epithelial cells is accelerated, restoring the damaged intestinal mucosa and barrier function.
Indole-3-propionic acid, a ligand for PXR, promoted intestinal barrier integrity by downregulating enterocyte TNF-α while it upregulated ligand-encoded mRNAs, which led to the induction of MDR1 and regulation of the epithelial nodal complex (149). Furthermore, metabolite IA reduce inflammatory response and enhance the function of the intestinal barrier by downregulating inflammation and oxidative stress genes, including CD14, CCL2, MT2A, CYBB, IL-6, and PTAFR (89).
6. Diseases
Bacterial TRP metabolites are critical in regulating various immune diseases, including inflammatory, cardiovascular, neurological, metabolic diseases, and cancer. This review focuses on the impact of bacterial TRP metabolites on the pathophysiology of inflammatory bowel disease (IBD), neurological disorders, and metabolic diseases.
6.1. Inflammatory bowel disease
Inflammatory bowel disease is a recurrent, chronic, non-specific inflammatory condition affecting the gastrointestinal tract (234). The two most commonly observed forms of IBD are Crohn’s disease and ulcerative colitis (UC) (235). IBD is known to result from the interplay of genetic and environmental factors, such as dysregulation of the mucosal immune system, disruption of the mucosal barrier of the intestine, and imbalances in the intestinal microbial community (183). Of note, recent research has demonstrated the significant involvement of dysregulated gut microbiota in the pathogenesis of IBD (12). Moreover, several studies have suggested a possible association between microbial Trp metabolism and IBD. For example, one study found that a tryptophan-free diet increased the susceptibility of mice to colitis (236). Trp indole metabolites would be selectively reduced in the serum and colon of IBD mice (237). The levels of IAA and other indole derivatives with anti-inflammatory effects were also significantly reduced in IBD (238, 239).
Recent research has indicated that there is a potential involvement of the Th17 subpopulation in the pathogenesis of colitis models induced by TNBS and DSS. Specifically, increased inflammation and Th17 subpopulations were observed in the colon and colon-associated mesenteric lymph nodes (MLN) (240–242). Notably, treatment with I3C can significantly reduce Th17 populations while increasing Tregs. Additionally, in mice with colitis treated with I3C, normal crypt formation and colonic tissue structure were maintained, while cellular infiltration was reduced (202). In parallel, the intestinal mucus layer was thinner and the amount of IPA was significantly reduced in patients with IBD, but its levels recovered with disease remission (237). In addition, IPA-treated mice showed attenuated inflammatory infiltration and reduced structural loss of epithelium and tissue. Microbial metabolites, including IPA, can help maintain intestinal homeostasis by promoting normal secretory function of goblet cells and contributing to a stable and healthy gut microbiota (243). Therefore, it can be speculated that treatment of IBD patients to normalize IPA can reduce the disease and promote intestinal homeostasis.
The most noteworthy anti-inflammatory factor in IBD is IL-10. IL-10 or IL-10 receptor deficient mice spontaneously develop severe colitis (244). During organismal inflammation, IL-10 binds to IL-10R1 and signals to reduce the proinflammatory factors production in a variety of cells, including intestinal epithelial cells (245). Functional IL-10 signaling is essential to maintain mucin production by cupped cells, which ensures mucosal barrier function and epithelial cell homeostasis (246). Mutations in IL-10 receptors have been associated with IBD and serve as a critical marker for inflammation resolution in colitis (247). Furthermore, IA has been shown to enhance IL-10 and mucin gene expression production. Thus, restoration of Trp metabolism in the gut of IBD patients leading to an increase in IA production may promote an anti-inflammatory response to produce therapeutic effects in IBD patients (89). In summary, gut microbial Trp metabolism is expected to be a therapeutic target for patients with IBD, and microbial Trp metabolites have been demonstrated laterally in the pathophysiology of other inflammatory diseases.
6.2. Neurological diseases
Microbial TRP metabolites can signal to the brain suggesting a potential role for metabolites in communication between the gut microbiota and the CNS (9, 248). AhR is known to be a receptor expressed not only in the gastrointestinal tract but also in CNS cells (neurons, astrocytes, and microglia). AhR reduces proinflammatory cytokine expression in astrocytes and microglia and affects the development of neurological diseases such as Parkinson’s disease (PD), multiple sclerosis (MS) (249, 250), Alzheimer’s disease (AD), and epilepsy (251, 252). Besides, AhR significantly impacts neuronal differentiation, proliferation, and survival (253). Indole derivatives, serving as ligands for AhR, including IPA and IAA, exhibit the ability to traverse the blood–brain barrier (BBB), thereby imparting particular relevance to their mediating role in the gut-brain axis (GBA). For example, indole, I3S, IPA, and IAld can activate AhR signaling in astrocytes and inhibit inflammation in the CNS (254). IPA has importance in neurological diseases due to its potent free radical scavenging ability and antioxidant capacity to protect primary neurons and neuroblastoma cells from oxidative damage (255).
Alzheimer’s disease as the most prevalent neurodegenerative disease, β-amyloid (Aβ) abnormal accumulation, and phosphorylation of tau aberrantly are widely recognized as key events leading to AD (256). Numerous studies have increasingly shown the positive effects of indoles and their derivatives in ameliorating AD pathology (257–259). Notably, in AD patients, the expression of indole derivatives IAA, ILA, and IPA was significantly reduced (260, 261). Similarly, in a study involving APP/PS1 mice with AD, indole, ILA, IAA, and I3C were found to prevent Tau hyperphosphorylation, abnormal Aβ accumulation, synaptic damage, and to promote behavioral and cognitive recovery (251). These effects are attributed to the ability of indole derivatives to elevate the levels of PSD-95 and synaptophysin, thereby mitigating synaptic damage and promoting synaptic maturation (251). Additionally, indole derivatives suppress the release of inflammatory cytokines (IL-18, IL-1β, IL-6, and TNF-α) by upregulating the expression of AhR, inhibiting the activation of the NF-κB signaling pathway, and impeding the formation of NLRP3 inflammasome. Consequently, indole derivatives contribute to the reduction of AD inflammatory responses (262). PD is the second most prevalent neurodegenerative disease after AD (263). Similar to AD, the therapeutic potential of indole derivatives for PD has been extensively investigated. For instance, I3C ameliorates lipopolysaccharide-induced neuroinflammation models of PD, delay neuronal degeneration, and improve cognitive function (264, 265). These effects are attributed to the inhibition of the NF-κB signaling pathway, decreased activity of inflammatory cytokines such as TNF-α and IL-6, and increased levels of catalase and superoxide dismutase (265). Furthermore, Pael receptors were associated with delayed proliferation of cells overexpressed in PD. IPA treatment significantly improved the proliferation of SH-SY5Y cells overexpressing Pael receptors (266). In summary, indole derivatives hold promise as potential therapeutic agents for both AD and PD. Their ability to mitigate key pathological events, including Aβ accumulation, tau phosphorylation, synaptic damage, and inflammatory responses, highlights their potential for improving the underlying neurodegenerative processes associated with these diseases (255, 260). Further research in academia is warranted to fully elucidate the mechanisms involved and explore the translational potential of indole derivatives in the treatment of AD and PD.
In contrast, recent studies have shown that L. reuteri, which is involved in one of the intestinal microbial Trp metabolisms, will exacerbate Experimental autoimmune encephalomyelitis (EAE) when colonized in the host intestine (267). This is due to the activation of AHR by indole derivatives such as IAA, IAAld, and tryptamine metabolites of L. reuteri, which promote the value-added of IL-17-producing γδ T cells and infiltration into the CNS. In parallel, production of inflammatory factors IL-17, IFN-γ, and GM-CSF increases. When reduced dietary tryptophan consumption will suppress EAE and inflammatory T cell responses in the CNS (205). In conclusion, the communication between Trp microbial metabolites and the CNS is a complex and dynamic process involving the combined effects of various receptors (e.g., AhR and PXR) and cytokines. The activation of AhR by indole derivatives to mediate inflammation in the CNS appears to act bidirectionally, but further studies are needed. These findings suggest that a better understanding of the gut-brain connection and the role of Trp microbial metabolites in this connection may lead to new therapeutic strategies for treating neurological disorders. By promoting a healthy gut microbiota and modulating Trp microbial metabolites, we may be able to positively impact brain health and prevent the development of neurological disorders.
6.3. Metabolic diseases
The prevalence of metabolic diseases is increasing with the increase in high-calorie diets and the decrease in exercise in humans (268). Metabolic disorders are clustered by a variety of interrelated pathological conditions, including obesity, nonalcoholic steatohepatitis (NASH), dyslipidemia, glucose intolerance, insulin resistance, hypertension, and diabetes mellitus (269). When these disorders occur together, they strongly increase cardiovascular morbidity and mortality.
Interestingly, the role of microbial TRP metabolites in metabolic diseases has been increasingly studied by scientists in recent years. A strong negative correlation exists between IAA abundance and body mass index (BMI) (270). IAA affects high-fat diet-mediated obesity through PXR and TLR4-mediated production of IL-35 B cells (271). Moreover, indole, IPA and I3S were lower in patients with type 2 diabetes and higher IPA serum concentrations were associated with a lower incidence of T2D (272). Metabolic disorders decrease the production of AHR ligands from Trp metabolites, thereby inhibiting the AhR pathway. This reduction also lowers the production of GLP-1 and IL-22, contributing to gut permeability and transfer of LPS, which results in inflammation, insulin resistance, and liver fat deposition. Recently, it has been shown that indole reduced key protein expression in the NF-κB pathway and inhibited proinflammatory factor expression (273). Besides, Indole prevents LPS-induced cholesterol metabolism alterations by increasing 4β-hydroxycholesterol hepatic levels via transcriptional regulation, which ultimately counters the adverse effects of LPS on liver. Additionally, IAA and ILA can reduce insulin resistance via aryl hydrocarbon receptors (11).
7. Summary
In conclusion, microbial Trp metabolites, especially those produced by gut microorganisms, significantly influence the regulation of host immunity. The metabolites produced by gut bacteria, such as indole and its derivatives, can activate the AhR and PXR signaling pathways, which play a crucial role in immune function and maintaining intestinal barrier integrity. These metabolites are involved in modulating non-specific and adaptive immunity, stimulating the generation of anti-inflammatory cytokines and enhancing the activity of immune cells. Moreover, bacterial Trp metabolites are also involved in maintaining intestinal homeostasis and mucosal integrity, thereby contributing to the prevention of inflammation and the development of immune-related diseases. Dysbiosis of the intestinal microbiota can lead to an imbalance in Trp metabolism, contributing to the pathophysiology of variety diseases (e.g., inflammatory bowel disease, neurological diseases, and metabolic diseases).
The crosstalk between gut microbes and Trp metabolism is a critical research area still in its early stages. However, the findings presented in this review suggest that targeting the gut microbiota and its metabolites may be a promising strategy for the prevention and treatment of immune-related diseases. To fully comprehend the mechanisms by which bacterial Trp metabolites impact immunity and to identify potential therapeutic targets, further research is necessary. The significance of the gut microbiota in regulating host immunity emphasizes the necessity for continued exploration of this intricate and constantly changing system.
Author contributions
The author confirms being the sole contributor of this work and has approved it for publication.
Funding
This study was supported by Hunan Provincial Science and Technology Department (2021JJ30008, 2020NK2004, and 2019TP2004), Double first-class construction project of Hunan Agricultural University (SYL201802003), Postgraduate Scientific Research Innovation Project of Hunan Province (CX20210654), and Science and Technology Innovation and Entrepreneurship Project for University Students of Hunan Province (2021RC1004).
Conflict of interest
The author declares that the research was conducted in the absence of any commercial or financial relationships that could be construed as a potential conflict of interest.
Publisher’s note
All claims expressed in this article are solely those of the authors and do not necessarily represent those of their affiliated organizations, or those of the publisher, the editors and the reviewers. Any product that may be evaluated in this article, or claim that may be made by its manufacturer, is not guaranteed or endorsed by the publisher.
References
1. Poeggeler, B, Singh, SK, and Pappolla, MA. Tryptophan in nutrition and health. Int J Mol Sci. (2022) 23:5455. doi: 10.3390/ijms23105455
2. Grifka-Walk, HM, Jenkins, BR, and Kominsky, DJ. Amino acid Trp: the far out impacts of host and commensal tryptophan metabolism. Front Immunol. (2021) 12:653208. doi: 10.3389/fimmu.2021.653208
3. Peters, JC. Tryptophan nutrition and metabolism: an overview. Adv Exp Med Biol. (1991) 294:345–58.
4. Brown, EM, Ke, X, Hitchcock, D, Jeanfavre, S, Avila-Pacheco, J, Nakata, T, et al. Bacteroides-derived sphingolipids are critical for maintaining intestinal homeostasis and Symbiosis. Cell Host Microbe. (2019) 25:668–680.e7. doi: 10.1016/j.chom.2019.04.002
5. Jandhyala, SM, Talukdar, R, Subramanyam, C, Vuyyuru, H, Sasikala, M, and Nageshwar Reddy, D. Role of the normal gut microbiota. World J Gastroenterol. (2015) 21:8787–803. doi: 10.3748/wjg.v21.i29.8787
6. Maynard, CL, Elson, CO, Hatton, RD, and Weaver, CT. Reciprocal interactions of the intestinal microbiota and immune system. Nature. (2012) 489:231–41. doi: 10.1038/nature11551
7. Liu, X, Nagy, P, Bonfini, A, Houtz, P, Bing, XL, Yang, X, et al. Microbes affect gut epithelial cell composition through immune-dependent regulation of intestinal stem cell differentiation. Cell Rep. (2022) 38:110572. doi: 10.1016/j.celrep.2022.110572
8. Zhang, LS, and Davies, SS. Microbial metabolism of dietary components to bioactive metabolites: opportunities for new therapeutic interventions. Genome Med. (2016) 8:46. doi: 10.1186/s13073-016-0296-x
9. Agus, A, Planchais, J, and Sokol, H. Gut microbiota regulation of tryptophan metabolism in health and disease. Cell Host Microbe. (2018) 23:716–24. doi: 10.1016/j.chom.2018.05.003
10. Tang, WH, Wang, Z, Levison, BS, Koeth, RA, Britt, EB, Fu, X, et al. Intestinal microbial metabolism of phosphatidylcholine and cardiovascular risk. N Engl J Med. (2013) 368:1575–84. doi: 10.1056/NEJMoa1109400
11. Roager, HM, and Licht, TR. Microbial tryptophan catabolites in health and disease. Nat Commun. (2018) 9:3294. doi: 10.1038/s41467-018-05470-4
12. Lavelle, A, and Sokol, H. Gut microbiota-derived metabolites as key actors in inflammatory bowel disease. Nat Rev Gastroenterol Hepatol. (2020) 17:223–37. doi: 10.1038/s41575-019-0258-z
13. Fiore, A, and Murray, PJ. Tryptophan and indole metabolism in immune regulation. Curr Opin Immunol. (2021) 70:7–14. doi: 10.1016/j.coi.2020.12.001
14. Shen, J, Yang, L, You, K, Chen, T, Su, Z, Cui, Z, et al. Indole-3-acetic acid alters intestinal microbiota and alleviates ankylosing spondylitis in mice. Front Immunol. (2022) 13:762580. doi: 10.3389/fimmu.2022.1104646
15. Sun, M, Ma, N, He, T, Johnston, LJ, and Ma, X. Tryptophan (Trp) modulates gut homeostasis via aryl hydrocarbon receptor (AhR). Crit Rev Food Sci Nutr. (2020) 60:1760–8. doi: 10.1080/10408398.2019.1598334
16. Davidson, M, Rashidi, N, Nurgali, K, and Apostolopoulos, V. The role of tryptophan metabolites in neuropsychiatric disorders. Int J Mol Sci. (2022) 23:9968. doi: 10.3390/ijms23179968
17. Correia, AS, and Vale, N. Tryptophan metabolism in depression: a narrative review with a focus on serotonin and kynurenine pathways. Int J Mol Sci. (2022) 23:8493. doi: 10.3390/ijms23158493
18. Badawy, AA. Tryptophan availability for kynurenine pathway metabolism across the life span: control mechanisms and focus on aging, exercise, diet and nutritional supplements. Neuropharmacology. (2017) 112:248–63. doi: 10.1016/j.neuropharm.2015.11.015
19. Lovelace, MD, Varney, B, Sundaram, G, Lennon, MJ, Lim, CK, Jacobs, K, et al. Recent evidence for an expanded role of the kynurenine pathway of tryptophan metabolism in neurological diseases. Neuropharmacology. (2017) 112:373–88. doi: 10.1016/j.neuropharm.2016.03.024
20. Körtési, T, Spekker, E, and Vécsei, L. Exploring the tryptophan metabolic pathways in migraine-related mechanisms. Cells. (2022) 11:3795. doi: 10.3390/cells11233795
21. Badawy, AA. Targeting tryptophan availability to tumors: the answer to immune escape? Immunol Cell Biol. (2018) 96:1026–34. doi: 10.1111/imcb.12168
22. Platten, M, Nollen, EAA, Röhrig, UF, Fallarino, F, and Opitz, CA. Tryptophan metabolism as a common therapeutic target in cancer, neurodegeneration and beyond. Nat Rev Drug Discov. (2019) 18:379–401. doi: 10.1038/s41573-019-0016-5
23. Kałużna-Czaplińska, J, Gątarek, P, Chirumbolo, S, Chartrand, MS, and Bjørklund, G. How important is tryptophan in human health? Crit Rev Food Sci Nutr. (2019) 59:72–88. doi: 10.1080/10408398.2017.1357534
24. Nongonierma, AB, and Fitz Gerald, RJ. Milk proteins as a source of tryptophan-containing bioactive peptides. Food Funct. (2015) 6:2115–27. doi: 10.1039/C5FO00407A
25. Madella, AM, Van Bergenhenegouwen, J, Garssen, J, Masereeuw, R, and Overbeek, SA. Microbial-derived tryptophan catabolites kidney disease and gut inflammation. Toxins. (2022) 14:645. doi: 10.3390/toxins14090645
26. Tse, FL, Christiano, JR, and Talbot, KC. Relative absorption of tryptophan ethyl ester amide derivatives with various fatty acid chains in the dog. J Pharm Pharmacol. (1984) 36:633–6. doi: 10.1111/j.2042-7158.1984.tb04916.x
27. Sanger, GJ. 5-hydroxytryptamine and the gastrointestinal tract: where next? Trends Pharmacol Sci. (2008) 29:465–71. doi: 10.1016/j.tips.2008.06.008
28. Gao, K, Mu, CL, Farzi, A, and Zhu, WY. Tryptophan metabolism: a link between the gut microbiota and brain. Adv Nutr. (2020) 11:709–23. doi: 10.1093/advances/nmz127
29. Maitre, M, Klein, C, Patte-Mensah, C, and Mensah-Nyagan, AG. Tryptophan metabolites modify brain Aβ peptide degradation: a role in Alzheimer's disease? Prog Neurobiol. (2020) 190:101800. doi: 10.1016/j.pneurobio.2020.101800
30. Comai, S, Bertazzo, A, Brughera, M, and Crotti, S. Tryptophan in health and disease. Adv Clin Chem. (2020) 95:165–218. doi: 10.1016/bs.acc.2019.08.005
31. Barik, S. The uniqueness of tryptophan in biology: properties, metabolism, interactions and localization in proteins. Int J Mol Sci. (2020) 21:8776. doi: 10.3390/ijms21228776
32. Kim, DK, Kanai, Y, Matsuo, H, Kim, JY, Chairoungdua, A, Kobayashi, Y, et al. The human T-type amino acid transporter-1: characterization, gene organization, and chromosomal location. Genomics. (2002) 79:95–103. doi: 10.1006/geno.2001.6678
33. Liu, S, Xu, JZ, and Zhang, WG. Advances and prospects in metabolic engineering of Escherichia coli for L-tryptophan production. World J Microbiol Biotechnol. (2022) 38:22. doi: 10.1007/s11274-021-03212-1
34. Wood, H, Fehlner-Gardner, C, Berry, J, Fischer, E, Graham, B, Hackstadt, T, et al. Regulation of tryptophan synthase gene expression in Chlamydia trachomatis. Mol Microbiol. (2003) 49:1347–59. doi: 10.1046/j.1365-2958.2003.03638.x
35. Klaessens, S, Stroobant, V, De Plaen, E, and Van den Eynde, BJ. Systemic tryptophan homeostasis. Front Mol Biosci. (2022) 9:897929. doi: 10.3389/fmolb.2022.897929
36. Chen, LM, Bao, CH, Wu, Y, Liang, SH, Wang, D, Wu, LY, et al. Tryptophan-kynurenine metabolism: a link between the gut and brain for depression in inflammatory bowel disease. J Neuroinflammation. (2021) 18:135. doi: 10.1186/s12974-021-02175-2
37. O'Mahony, SM, Clarke, G, Borre, YE, Dinan, TG, and Cryan, JF. Serotonin, tryptophan metabolism and the brain-gut-microbiome axis. Behav Brain Res. (2015) 277:32–48. doi: 10.1016/j.bbr.2014.07.027
38. Joisten, N, Ruas, JL, Braidy, N, Guillemin, GJ, and Zimmer, P. The kynurenine pathway in chronic diseases: a compensatory mechanism or a driving force? Trends Mol Med. (2021) 27:946–54. doi: 10.1016/j.molmed.2021.07.006
39. Hubková, B, Valko-Rokytovská, M, Čižmárová, B, Zábavníková, M, Mareková, M, and Birková, A. Tryptophan: its metabolism along the kynurenine, serotonin, and indole pathway in malignant melanoma. Int J Mol Sci. (2022) 23:9160. doi: 10.3390/ijms23169160
40. Su, X, Gao, Y, and Yang, R. Gut microbiota-derived tryptophan metabolites maintain gut and systemic homeostasis. Cells. (2022) 11:2296. doi: 10.3390/cells11152296
41. Cervenka, I, Agudelo, LZ, and Ruas, JL. Kynurenines: Tryptophan's metabolites in exercise, inflammation, and mental health. Science. (2017) 357:eaaf9794. doi: 10.1126/science.aaf9794
42. Castro-Portuguez, R, and Sutphin, GL. Kynurenine pathway, NAD(+) synthesis, and mitochondrial function: targeting tryptophan metabolism to promote longevity and healthspan. Exp Gerontol. (2020) 132:110841. doi: 10.1016/j.exger.2020.110841
43. Li, F, Zhang, R, Li, S, and Liu, J. IDO1: an important immunotherapy target in cancer treatment. Int Immunopharmacol. (2017) 47:70–7. doi: 10.1016/j.intimp.2017.03.024
44. Merlo, LM, and Mandik-Nayak, L. IDO2: a pathogenic mediator of inflammatory autoimmunity. Clin Med Insights Pathol. (2016) 9:21–8. doi: 10.4137/CPath.S39930
45. Yu, CP, Song, YL, Zhu, ZM, Huang, B, Xiao, YQ, and Luo, DY. Targeting TDO in cancer immunotherapy. Med Oncol. (2017) 34:73. doi: 10.1007/s12032-017-0933-2
46. Kim, M, and Tomek, P. Tryptophan: a rheostat of Cancer immune escape mediated by immunosuppressive enzymes IDO1 and TDO. Front Immunol. (2021) 12:636081. doi: 10.3389/fimmu.2021.636081
47. O'Farrell, K, and Harkin, A. Stress-related regulation of the kynurenine pathway: relevance to neuropsychiatric and degenerative disorders. Neuropharmacology. (2017) 112:307–23. doi: 10.1016/j.neuropharm.2015.12.004
48. Guillemin, GJ, Smythe, G, Takikawa, O, and Brew, BJ. Expression of indoleamine 2, 3-dioxygenase and production of quinolinic acid by human microglia, astrocytes, and neurons. Glia. (2005) 49:15–23. doi: 10.1002/glia.20090
49. Hughes, TD, Güner, OF, Iradukunda, EC, Phillips, RS, and Bowen, JP. The kynurenine pathway and kynurenine 3-monooxygenase inhibitors. Molecules. (2022) 27:273. doi: 10.3390/molecules27010273
50. Dehhaghi, M, Panahi, HKS, Kavyani, B, Heng, B, Tan, V, Braidy, N, et al. The role of kynurenine pathway and NAD(+) metabolism in Myalgic encephalomyelitis/chronic fatigue syndrome. Aging Dis. (2022) 13:698–711. doi: 10.14336/AD.2021.0824
51. Tanaka, M, Tóth, F, Polyák, H, Szabó, Á, Mándi, Y, and Vécsei, L. Immune influencers in action: metabolites and enzymes of the tryptophan-kynurenine metabolic pathway. Biomedicine. (2021) 9:734. doi: 10.3390/biomedicines9070734
52. Muneer, A. Kynurenine pathway of tryptophan metabolism in neuropsychiatric disorders: pathophysiologic and therapeutic considerations. Clin Psychopharmacol Neurosci. (2020) 18:507–26. doi: 10.9758/cpn.2020.18.4.507
53. Wang, Q, Liu, D, Song, P, and Zou, MH. Tryptophan-kynurenine pathway is dysregulated in inflammation, and immune activation. Front Biosci. (2015) 20:1116–43. doi: 10.2741/4363
54. Gibson, EL. Tryptophan supplementation and serotonin function: genetic variations in behavioural effects. Proc Nutr Soc. (2018) 77:174–88. doi: 10.1017/S0029665117004451
55. Paredes, S, Cantillo, S, Candido, KD, and Knezevic, NN. An Association of Serotonin with pain disorders and its modulation by estrogens. Int J Mol Sci. (2019) 20:5729. doi: 10.3390/ijms20225729
56. Roth, W, Zadeh, K, Vekariya, R, Ge, Y, and Mohamadzadeh, M. Tryptophan metabolism and gut-brain homeostasis. Int J Mol Sci. (2021) 22:2973. doi: 10.3390/ijms22062973
57. Waider, J, Araragi, N, Gutknecht, L, and Lesch, KP. Tryptophan hydroxylase-2 (TPH2) in disorders of cognitive control and emotion regulation: a perspective. Psychoneuroendocrinology. (2011) 36:393–405. doi: 10.1016/j.psyneuen.2010.12.012
58. Jenkins, TA, Nguyen, JC, Polglaze, KE, and Bertrand, PP. Influence of tryptophan and serotonin on mood and cognition with a possible role of the gut-brain Axis. Nutrients. (2016) 8:56. doi: 10.3390/nu8010056
59. Clarke, G, Grenham, S, Scully, P, Fitzgerald, P, Moloney, RD, Shanahan, F, et al. The microbiome-gut-brain axis during early life regulates the hippocampal serotonergic system in a sex-dependent manner. Mol Psychiatry. (2013) 18:666–73. doi: 10.1038/mp.2012.77
60. Höglund, E, Øverli, Ø, and Winberg, S. Tryptophan metabolic pathways and brain serotonergic activity: a comparative review. Front Endocrinol. (2019) 10:158. doi: 10.3389/fendo.2019.00158
61. Alcantara-Zapata, DE, Lucero, N, De Gregorio, N, Astudillo Cornejo, P, Ibarra Villanueva, C, Baltodano-Calle, MJ, et al. Women's mood at high altitude. Sexual dimorphism in hypoxic stress modulation by the tryptophan-melatonin axis. Front Physiol. (2022) 13:1099276. doi: 10.3389/fphys.2022.1099276
62. Slominski, AT, Hardeland, R, Zmijewski, MA, Slominski, RM, Reiter, RJ, and Paus, R. Melatonin: a cutaneous perspective on its production, metabolism, and functions. J Invest Dermatol. (2018) 138:490–9. doi: 10.1016/j.jid.2017.10.025
63. De Giovanni, M, Tam, H, Valet, C, Xu, Y, Looney, MR, and Cyster, JG. GPR35 promotes neutrophil recruitment in response to serotonin metabolite 5-HIAA. Cells. (2022) 185:815–830.e19. doi: 10.1016/j.cell.2022.01.010
64. Wrzosek, L, Ciocan, D, Hugot, C, Spatz, M, Dupeux, M, Houron, C, et al. Microbiota tryptophan metabolism induces aryl hydrocarbon receptor activation and improves alcohol-induced liver injury. Gut. (2021) 70:1299–308. doi: 10.1136/gutjnl-2020-321565
65. Gao, J, Xu, K, Liu, H, Liu, G, Bai, M, Peng, C, et al. Impact of the gut microbiota on intestinal immunity mediated by tryptophan metabolism. Front Cell Infect Microbiol. (2018) 8:13. doi: 10.3389/fcimb.2018.00013
66. Hyland, NP, Cavanaugh, CR, and Hornby, PJ. Emerging effects of tryptophan pathway metabolites and intestinal microbiota on metabolism and intestinal function. Amino Acids. (2022) 54:57–70. doi: 10.1007/s00726-022-03123-x
67. Wei, GZ, Martin, KA, Xing, PY, Agrawal, R, Whiley, L, Wood, TK, et al. Tryptophan-metabolizing gut microbes regulate adult neurogenesis via the aryl hydrocarbon receptor. Proc Natl Acad Sci U S A. (2021) 118:e2021091118. doi: 10.1073/pnas.2021091118
68. Zhang, XS, García-Contreras, R, and Wood, TK. Escherichia coli transcription factor Ync C (Mcb R) regulates colanic acid and biofilm formation by repressing expression of periplasmic protein YbiM (McbA). ISME J. (2008) 2:615–31. doi: 10.1038/ismej.2008.24
69. Dodd, D, Spitzer, MH, Van Treuren, W, Merrill, BD, Hryckowian, AJ, Higginbottom, SK, et al. A gut bacterial pathway metabolizes aromatic amino acids into nine circulating metabolites. Nature. (2017) 551:648–52. doi: 10.1038/nature24661
70. Bhattarai, Y, Jie, S, Linden, DR, Ghatak, S, Mars, RAT, Williams, BB, et al. Bacterially derived tryptamine increases mucus release by activating a host receptor in a mouse model of inflammatory bowel disease. iScience. (2020) 23:101798. doi: 10.1016/j.isci.2020.101798
71. Phillips, RS, Buisman, AA, Choi, S, Hussaini, A, and Wood, ZA. The crystal structure of Proteus vulgaris tryptophan indole-lyase complexed with oxindolyl-L-alanine: implications for the reaction mechanism. Acta Crystallogr D Struct Biol. (2018) 74:748–59. doi: 10.1107/S2059798318003352
72. Cruz-Vera, LR, Yang, R, and Yanofsky, C. Tryptophan inhibits Proteus vulgaris TnaC leader peptide elongation, activating tna operon expression. J Bacteriol. (2009) 191:7001–6. doi: 10.1128/JB.01002-09
73. Bortolotti, P, Hennart, B, Thieffry, C, Jausions, G, Faure, E, Grandjean, T, et al. Tryptophan catabolism in Pseudomonas aeruginosa and potential for inter-kingdom relationship. BMC Microbiol. (2016) 16:137. doi: 10.1186/s12866-016-0756-x
74. Hwang, HJ, Li, XH, Kim, SK, and Lee, JH. Anthranilate acts as a signal to modulate biofilm formation, virulence, and antibiotic tolerance of Pseudomonas aeruginosa and surrounding Bacteria. Microbiol Spectr. (2022) 10:e0146321. doi: 10.1128/spectrum.01463-21
75. Teunis, C, Nieuwdorp, M, and Hanssen, N. Interactions between tryptophan metabolism, the gut microbiome and the immune system as potential drivers of non-alcoholic fatty liver disease (NAFLD) and metabolic diseases. Meta. (2022) 12:514. doi: 10.3390/metabo12060514
76. Lee, JH, and Lee, J. Indole as an intercellular signal in microbial communities. FEMS Microbiol Rev. (2010) 34:426–44. doi: 10.1111/j.1574-6976.2009.00204.x
77. Keszthelyi, D, Troost, FJ, and Masclee, AA. Understanding the role of tryptophan and serotonin metabolism in gastrointestinal function. Neurogastroenterol Motil. (2009) 21:1239–49. doi: 10.1111/j.1365-2982.2009.01370.x
78. Smith, EA, and Macfarlane, GT. Enumeration of human colonic bacteria producing phenolic and indolic compounds: effects of pH, carbohydrate availability and retention time on dissimilatory aromatic amino acid metabolism. J Appl Bacteriol. (1996) 81:288–302. doi: 10.1111/j.1365-2672.1996.tb04331.x
79. Devlin, AS, Marcobal, A, Dodd, D, Nayfach, S, Plummer, N, Meyer, T, et al. Modulation of a circulating uremic solute via rational genetic manipulation of the gut microbiota. Cell Host Microbe. (2016) 20:709–15. doi: 10.1016/j.chom.2016.10.021
80. Fang, Z, Pan, T, Wang, H, Zhu, J, Zhang, H, Zhao, J, et al. Limosilactobacillus reuteri attenuates atopic dermatitis via changes in gut Bacteria and indole derivatives from tryptophan metabolism. Int J Mol Sci. (2022) 23:7735. doi: 10.3390/ijms23147735
81. Isenberg, HD, and Sundheim, LH. Indole reactions in bacteria. J Bacteriol. (1958) 75:682–90. doi: 10.1128/jb.75.6.682-690.1958
82. Laird, TS, Flores, N, and Leveau, JHJ. Bacterial catabolism of indole-3-acetic acid. Appl Microbiol Biotechnol. (2020) 104:9535–50. doi: 10.1007/s00253-020-10938-9
83. Russell, WR, Duncan, SH, Scobbie, L, Duncan, G, Cantlay, L, Calder, AG, et al. Major phenylpropanoid-derived metabolites in the human gut can arise from microbial fermentation of protein. Mol Nutr Food Res. (2013) 57:523–35. doi: 10.1002/mnfr.201200594
84. Elsden, SR, Hilton, MG, and Waller, JM. The end products of the metabolism of aromatic amino acids by Clostridia. Arch Microbiol. (1976) 107:283–8. doi: 10.1007/BF00425340
85. Lamas, B, Richard, ML, Leducq, V, Pham, HP, Michel, ML, Da Costa, G, et al. CARD9 impacts colitis by altering gut microbiota metabolism of tryptophan into aryl hydrocarbon receptor ligands. Nat Med. (2016) 22:598–605. doi: 10.1038/nm.4102
86. Cook, KL, Rothrock, MJ Jr, Loughrin, JH, and Doerner, KC. Characterization of skatole-producing microbial populations in enriched swine lagoon slurry. FEMS Microbiol Ecol. (2007) 60:329–40. doi: 10.1111/j.1574-6941.2007.00299.x
87. Whitehead, TR, Price, NP, Drake, HL, and Cotta, MA. Catabolic pathway for the production of skatole and indoleacetic acid by the acetogen Clostridium drakei, Clostridium scatologenes, and swine manure. Appl Environ Microbiol. (2008) 74:1950–3. doi: 10.1128/AEM.02458-07
88. Jensen, MT, Cox, RP, and Jensen, BB. 3-Methylindole (skatole) and indole production by mixed populations of pig fecal bacteria. Appl Environ Microbiol. (1995) 61:3180–4. doi: 10.1128/aem.61.8.3180-3184.1995
89. Wlodarska, M, Luo, C, Kolde, R, d'Hennezel, E, Annand, JW, Heim, CE, et al. Indoleacrylic acid produced by commensal Peptostreptococcus species suppresses inflammation. Cell Host Microbe. (2017) 22:25–37.e6. doi: 10.1016/j.chom.2017.06.007
90. Matchett, WH. Inhibition of tryptophan synthetase by indoleacrylic acid. J Bacteriol. (1972) 110:146–54. doi: 10.1128/jb.110.1.146-154.1972
91. Lu, W, Qian, L, Fang, Z, Wang, H, Zhu, J, Lee, YK, et al. Probiotic strains alleviated OVA-induced food allergy in mice by regulating the gut microbiota and improving the level of indoleacrylic acid in fecal samples. Food Funct. (2022) 13:3704–19. doi: 10.1039/D1FO03520G
92. Jiang, H, Chen, C, and Gao, J. Extensive summary of the important roles of indole propionic acid, a gut microbial metabolite in host health and disease. Nutrients. (2022) 15:151. doi: 10.3390/nu15010151
93. Williams, BB, Van Benschoten, AH, Cimermancic, P, Donia, MS, Zimmermann, M, Taketani, M, et al. Discovery and characterization of gut microbiota decarboxylases that can produce the neurotransmitter tryptamine. Cell Host Microbe. (2014) 16:495–503. doi: 10.1016/j.chom.2014.09.001
94. Serger, E, Luengo-Gutierrez, L, Chadwick, JS, Kong, G, Zhou, L, Crawford, G, et al. The gut metabolite indole-3 propionate promotes nerve regeneration and repair. Nature. (2022) 607:585–92. doi: 10.1038/s41586-022-04884-x
95. Honoré, AH, Aunsbjerg, SD, Ebrahimi, P, Thorsen, M, Benfeldt, C, Knøchel, S, et al. Metabolic footprinting for investigation of antifungal properties of Lactobacillus paracasei. Anal Bioanal Chem. (2016) 408:83–96. doi: 10.1007/s00216-015-9103-6
96. Cervantes-Barragan, L, Chai, JN, Tianero, MD, Di Luccia, B, Ahern, PP, Merriman, J, et al. Lactobacillus reuteri induces gut intraepithelial CD4(+)CD8αα(+) T cells. Science. (2017) 357:806–10. doi: 10.1126/science.aah5825
97. Aragozzini, F, Ferrari, A, Pacini, N, and Gualandris, R. Indole-3-lactic acid as a tryptophan metabolite produced by Bifidobacterium spp. Appl Environ Microbiol. (1979) 38:544–6. doi: 10.1128/aem.38.3.544-546.1979
98. Paeslack, N, Mimmler, M, Becker, S, Gao, Z, Khuu, MP, Mann, A, et al. Microbiota-derived tryptophan metabolites in vascular inflammation and cardiovascular disease. Amino Acids. (2022) 54:1339–56. doi: 10.1007/s00726-022-03161-5
99. Wilck, N, Matus, MG, Kearney, SM, Olesen, SW, Forslund, K, Bartolomaeus, H, et al. Salt-responsive gut commensal modulates T(H)17 axis and disease. Nature. (2017) 551:585–9. doi: 10.1038/nature24628
100. Zelante, T, Iannitti, RG, Cunha, C, De Luca, A, Giovannini, G, Pieraccini, G, et al. Tryptophan catabolites from microbiota engage aryl hydrocarbon receptor and balance mucosal reactivity via interleukin-22. Immunity. (2013) 39:372–85. doi: 10.1016/j.immuni.2013.08.003
101. Smith, EA, and Macfarlane, GT. Formation of phenolic and Indolic compounds by anaerobic Bacteria in the human large intestine. Microb Ecol. (1997) 33:180–8. doi: 10.1007/s002489900020
102. Koga, J, Adachi, T, and Hidaka, H. Molecular cloning of the gene for indolepyruvate decarboxylase from Enterobacter cloacae. Mol Gen Genet. (1991) 226:10–6. doi: 10.1007/BF00273581
103. Chen, Z, Luo, J, Li, J, Kim, G, Stewart, A, Urban, JF Jr, et al. Interleukin-33 promotes serotonin release from Enterochromaffin cells for intestinal homeostasis. Immunity. (2021) 54:151–163.e6. doi: 10.1016/j.immuni.2020.10.014
104. De Deurwaerdère, P, and Di Giovanni, G. Serotonin in health and disease. Int J Mol Sci. (2020) 21:3500. doi: 10.3390/ijms21103500
105. Pourhamzeh, M, Moravej, FG, Arabi, M, Shahriari, E, Mehrabi, S, Ward, R, et al. The roles of serotonin in neuropsychiatric disorders. Cell Mol Neurobiol. (2022) 42:1671–92. doi: 10.1007/s10571-021-01064-9
106. Sugisawa, E, Takayama, Y, Takemura, N, Kondo, T, Hatakeyama, S, Kumagai, Y, et al. RNA sensing by gut Piezo1 is essential for systemic serotonin synthesis. Cells. (2020) 182:609–624.e21. doi: 10.1016/j.cell.2020.06.022
107. Mawe, GM, and Hoffman, JM. Serotonin signalling in the gut--functions, dysfunctions and therapeutic targets. Nat Rev Gastroenterol Hepatol. (2013) 10:473–86. doi: 10.1038/nrgastro.2013.105
108. Reigstad, CS, Salmonson, CE, Rainey, JF 3rd, Szurszewski, JH, Linden, DR, Sonnenburg, JL, et al. Gut microbes promote colonic serotonin production through an effect of short-chain fatty acids on enterochromaffin cells. FASEB J. (2015) 29:1395–403. doi: 10.1096/fj.14-259598
109. Hata, T, Asano, Y, Yoshihara, K, Kimura-Todani, T, Miyata, N, Zhang, XT, et al. Regulation of gut luminal serotonin by commensal microbiota in mice. PLoS One. (2017) 12:e0180745. doi: 10.1371/journal.pone.0180745
110. Mandić, AD, Woting, A, Jaenicke, T, Sander, A, Sabrowski, W, Rolle-Kampcyk, U, et al. Clostridium ramosum regulates enterochromaffin cell development and serotonin release. Sci Rep. (2019) 9:1177. doi: 10.1038/s41598-018-38018-z
111. Yano, JM, Yu, K, Donaldson, GP, Shastri, GG, Ann, P, Ma, L, et al. Indigenous bacteria from the gut microbiota regulate host serotonin biosynthesis. Cells. (2015) 161:264–76. doi: 10.1016/j.cell.2015.02.047
112. Shishov, VA, Kirovskaia, TA, Kudrin, VS, and Oleskin, AV. Amine neuromediators, their precursors, and oxidation products in the culture of Escherichia coli K-12. Prikl Biokhim Mikrobiol. (2009) 45:550–4.
113. Li, Y, Yang, M, Zhang, L, Mao, Z, Lin, Y, Xu, S, et al. Dietary Fiber supplementation in gestating sow diet improved fetal growth and placental development and function through serotonin signaling pathway. Front Vet Sci. (2022) 9:831703. doi: 10.3389/fvets.2022.1080927
114. Xiao, L, Liu, Q, Luo, M, and Xiong, L. Gut microbiota-derived metabolites in irritable bowel syndrome. Front Cell Infect Microbiol. (2021) 11:729346. doi: 10.3389/fcimb.2021.729346
115. van Galen, KA, Ter Horst, KW, and Serlie, MJ. Serotonin, food intake, and obesity. Obes Rev. (2021) 22:e13210. doi: 10.1111/obr.13210
116. Nawrot, J, Napierała, M, Kaczerowska-Pietrzak, K, Florek, E, Gornowicz-Porowska, J, Pelant, E, et al. The anti-serotonin effect of Parthenolide derivatives and standardised extract from the leaves of Stizolophus balsamita. Molecules. (2019) 24:4131. doi: 10.3390/molecules24224131
117. Labadie, BW, Bao, R, and Luke, JJ. Reimagining IDO pathway inhibition in Cancer immunotherapy via downstream focus on the tryptophan-kynurenine-aryl hydrocarbon Axis. Clin Cancer Res. (2019) 25:1462–71. doi: 10.1158/1078-0432.CCR-18-2882
118. Alvarado, DM, Chen, B, Iticovici, M, Thaker, AI, Dai, N, VanDussen, KL, et al. Epithelial Indoleamine 2,3-dioxygenase 1 modulates aryl hydrocarbon receptor and notch signaling to increase differentiation of secretory cells and Alter mucus-associated microbiota. Gastroenterology. (2019) 157:1093–1108.e11. doi: 10.1053/j.gastro.2019.07.013
119. Desbonnet, L, Garrett, L, Clarke, G, Bienenstock, J, and Dinan, TG. The probiotic Bifidobacteria infantis: an assessment of potential antidepressant properties in the rat. J Psychiatr Res. (2008) 43:164–74. doi: 10.1016/j.jpsychires.2008.03.009
120. Deng, Y, Zhou, M, Wang, J, Yao, J, Yu, J, Liu, W, et al. Involvement of the microbiota-gut-brain axis in chronic restraint stress: disturbances of the kynurenine metabolic pathway in both the gut and brain. Gut Microbes. (2021) 13:1–16. doi: 10.1080/19490976.2021.1902719
121. Martin-Gallausiaux, C, Larraufie, P, Jarry, A, Béguet-Crespel, F, Marinelli, L, Ledue, F, et al. Butyrate produced by commensal Bacteria Down-regulates Indolamine 2,3-dioxygenase 1 (IDO-1) expression via a dual mechanism in human intestinal epithelial cells. Front Immunol. (2018) 9:2838. doi: 10.3389/fimmu.2018.02838
122. Gao, K, Pi, Y, Peng, Y, Mu, CL, and Zhu, WY. Time-course responses of ileal and fecal microbiota and metabolite profiles to antibiotics in cannulated pigs. Appl Microbiol Biotechnol. (2018) 102:2289–99. doi: 10.1007/s00253-018-8774-2
123. Shinde, R, and McGaha, TL. The aryl hydrocarbon receptor: connecting immunity to the microenvironment. Trends Immunol. (2018) 39:1005–20. doi: 10.1016/j.it.2018.10.010
124. Lamorte, S, Shinde, R, and McGaha, TL. Nuclear receptors, the aryl hydrocarbon receptor, and macrophage function. Mol Asp Med. (2021) 78:100942. doi: 10.1016/j.mam.2021.100942
125. Habano, W, Miura, T, Terashima, J, and Ozawa, S. Aryl hydrocarbon receptor as a DNA methylation reader in the stress response pathway. Toxicology. (2022) 470:153154. doi: 10.1016/j.tox.2022.153154
126. Dong, F, and Perdew, GH. The aryl hydrocarbon receptor as a mediator of host-microbiota interplay. Gut Microbes. (2020) 12:1859812. doi: 10.1080/19490976.2020.1859812
127. Murray, IA, Patterson, AD, and Perdew, GH. Aryl hydrocarbon receptor ligands in cancer: friend and foe. Nat Rev Cancer. (2014) 14:801–14. doi: 10.1038/nrc3846
128. Gutiérrez-Vázquez, C, and Quintana, FJ. Regulation of the immune response by the aryl hydrocarbon receptor. Immunity. (2018) 48:19–33. doi: 10.1016/j.immuni.2017.12.012
129. Sun, Y, Shi, Z, Lin, Y, Zhang, M, Liu, J, Zhu, L, et al. Benzo(a)pyrene induces MUC5AC expression through the AhR/mitochondrial ROS/ERK pathway in airway epithelial cells. Ecotoxicol Environ Saf. (2021) 210:111857. doi: 10.1016/j.ecoenv.2020.111857
130. Gasaly, N, de Vos, P, and Hermoso, MA. Impact of bacterial metabolites on gut barrier function and host immunity: a focus on bacterial metabolism and its relevance for intestinal inflammation. Front Immunol. (2021) 12:658354. doi: 10.3389/fimmu.2021.658354
131. Thurmond, TS, Staples, JE, Silverstone, AE, and Gasiewicz, TA. The aryl hydrocarbon receptor has a role in the in vivo maturation of murine bone marrow B lymphocytes and their response to 2,3,7,8-tetrachlorodibenzo-p-dioxin. Toxicol Appl Pharmacol. (2000) 165:227–36. doi: 10.1006/taap.2000.8942
132. Sherr, DH, and Monti, S. The role of the aryl hydrocarbon receptor in normal and malignant B cell development. Semin Immunopathol. (2013) 35:705–16. doi: 10.1007/s00281-013-0390-8
133. Prasad Singh, N, Nagarkatti, M, and Nagarkatti, P. From suppressor T cells to regulatory T cells: how the journey that began with the discovery of the toxic effects of TCDD led to better understanding of the role of AhR in Immunoregulation. Int J Mol Sci. (2020) 21:7849. doi: 10.3390/ijms21217849
134. Singh, NP, Singh, UP, Singh, B, Price, RL, Nagarkatti, M, and Nagarkatti, PS. Activation of aryl hydrocarbon receptor (AhR) leads to reciprocal epigenetic regulation of FoxP3 and IL-17 expression and amelioration of experimental colitis. PLoS One. (2011) 6:e23522. doi: 10.1371/journal.pone.0023522
135. Kimura, A, Naka, T, and Kishimoto, T. IL-6-dependent and-independent pathways in the development of interleukin 17-producing T helper cells. Proc Natl Acad Sci U S A. (2007) 104:12099–104. doi: 10.1073/pnas.0705268104
136. Yang, XP, Ghoreschi, K, Steward-Tharp, SM, Rodriguez-Canales, J, Zhu, J, Grainger, JR, et al. Opposing regulation of the locus encoding IL-17 through direct, reciprocal actions of STAT3 and STAT5. Nat Immunol. (2011) 12:247–54. doi: 10.1038/ni.1995
137. Cervantes-Barragan, L, and Colonna, M. AHR signaling in the development and function of intestinal immune cells and beyond. Semin Immunopathol. (2018) 40:371–7. doi: 10.1007/s00281-018-0694-9
138. Masuda, K, Kimura, A, Hanieh, H, Nguyen, NT, Nakahama, T, Chinen, I, et al. Aryl hydrocarbon receptor negatively regulates LPS-induced IL-6 production through suppression of histamine production in macrophages. Int Immunol. (2011) 23:637–45. doi: 10.1093/intimm/dxr072
139. Yang, W, Yu, T, Huang, X, Bilotta, AJ, Xu, L, Lu, Y, et al. Intestinal microbiota-derived short-chain fatty acids regulation of immune cell IL-22 production and gut immunity. Nat Commun. (2020) 11:4457. doi: 10.1038/s41467-020-18262-6
140. Trikha, P, and Lee, DA. The role of AhR in transcriptional regulation of immune cell development and function. Biochim Biophys Acta Rev Cancer. (2020) 1873:188335. doi: 10.1016/j.bbcan.2019.188335
141. Stockinger, B, Shah, K, and Wincent, E. AHR in the intestinal microenvironment: safeguarding barrier function. Nat Rev Gastroenterol Hepatol. (2021) 18:559–70. doi: 10.1038/s41575-021-00430-8
142. Illés, P, Krasulová, K, Vyhlídalová, B, Poulíková, K, Marcalíková, A, Pečinková, P, et al. Indole microbial intestinal metabolites expand the repertoire of ligands and agonists of the human pregnane X receptor. Toxicol Lett. (2020) 334:87–93. doi: 10.1016/j.toxlet.2020.09.015
143. Kliewer, SA. The nuclear pregnane X receptor regulates xenobiotic detoxification. J Nutr. (2003) 133:2444s–7s. doi: 10.1093/jn/133.7.2444S
144. Gerbal-Chaloin, S, Daujat, M, Pascussi, JM, Pichard-Garcia, L, Vilarem, MJ, and Maurel, P. Transcriptional regulation of CYP2C9 gene. Role of glucocorticoid receptor and constitutive androstane receptor. J Biol Chem. (2002) 277:209–17. doi: 10.1074/jbc.M107228200
145. Geick, A, Eichelbaum, M, and Burk, O. Nuclear receptor response elements mediate induction of intestinal MDR1 by rifampin. J Biol Chem. (2001) 276:14581–7. doi: 10.1074/jbc.M010173200
146. Noble, SM, Carnahan, VE, Moore, LB, Luntz, T, Wang, H, Ittoop, OR, et al. Human PXR forms a tryptophan zipper-mediated homodimer. Biochemistry. (2006) 45:8579–89. doi: 10.1021/bi0602821
147. Owen, A, Chandler, B, Back, DJ, and Khoo, SH. Expression of pregnane-X-receptor transcript in peripheral blood mononuclear cells and correlation with MDR1 mRNA. Antivir Ther. (2004) 9:819–21.
148. Schote, AB, Turner, JD, Schiltz, J, and Muller, CP. Nuclear receptors in human immune cells: expression and correlations. Mol Immunol. (2007) 44:1436–45. doi: 10.1016/j.molimm.2006.04.021
149. Venkatesh, M, Mukherjee, S, Wang, H, Li, H, Sun, K, Benechet, AP, et al. Symbiotic bacterial metabolites regulate gastrointestinal barrier function via the xenobiotic sensor PXR and toll-like receptor 4. Immunity. (2014) 41:296–310. doi: 10.1016/j.immuni.2014.06.014
150. Xing, Y, Yan, J, and Niu, Y. PXR: a center of transcriptional regulation in cancer. Acta Pharm Sin B. (2020) 10:197–206. doi: 10.1016/j.apsb.2019.06.012
151. Sári, Z, Mikó, E, Kovács, T, Jankó, L, Csonka, T, Lente, G, et al. Indolepropionic acid, a metabolite of the microbiome, has cytostatic properties in breast Cancer by activating AHR and PXR receptors and inducing oxidative stress. Cancers. (2020) 12:2411. doi: 10.3390/cancers12092411
152. Du, L, Qi, R, Wang, J, Liu, Z, and Wu, Z. Indole-3-propionic acid, a functional metabolite of Clostridium sporogenes, promotes muscle tissue development and reduces muscle cell inflammation. Int J Mol Sci. (2021) 22:12435. doi: 10.3390/ijms222212435
153. Mencarelli, A, Renga, B, Palladino, G, Claudio, D, Ricci, P, Distrutti, E, et al. Inhibition of NF-κB by a PXR-dependent pathway mediates counter-regulatory activities of rifaximin on innate immunity in intestinal epithelial cells. Eur J Pharmacol. (2011) 668:317–24. doi: 10.1016/j.ejphar.2011.06.058
154. Wang, S, Xie, X, Lei, T, Zhang, K, Lai, B, Zhang, Z, et al. Statins attenuate activation of the NLRP3 Inflammasome by oxidized LDL or TNFα in vascular endothelial cells through a PXR-dependent mechanism. Mol Pharmacol. (2017) 92:256–64. doi: 10.1124/mol.116.108100
155. Sári, Z, Mikó, E, Kovács, T, Boratkó, A, Ujlaki, G, Jankó, L, et al. Indoxylsulfate, a metabolite of the microbiome, has cytostatic effects in breast Cancer via activation of AHR and PXR receptors and induction of oxidative stress. Cancers. (2020) 12:2915. doi: 10.3390/cancers12102915
156. Hillion, S, Arleevskaya, MI, Blanco, P, Bordron, A, Brooks, WH, Cesbron, JY, et al. The innate part of the adaptive immune system. Clin Rev Allergy Immunol. (2020) 58:151–4. doi: 10.1007/s12016-019-08740-1
157. Place, DE, and Kanneganti, TD. The innate immune system and cell death in autoinflammatory and autoimmune disease. Curr Opin Immunol. (2020) 67:95–105. doi: 10.1016/j.coi.2020.10.013
158. Zhu, J, Luo, L, Tian, L, Yin, S, Ma, X, Cheng, S, et al. Aryl hydrocarbon receptor promotes IL-10 expression in inflammatory macrophages through Src-STAT3 signaling pathway. Front Immunol. (2018) 9:2033. doi: 10.3389/fimmu.2018.02033
159. Chng, SH, Kundu, P, Dominguez-Brauer, C, Teo, WL, Kawajiri, K, Fujii-Kuriyama, Y, et al. Ablating the aryl hydrocarbon receptor (AhR) in CD11c+ cells perturbs intestinal epithelium development and intestinal immunity. Sci Rep. (2016) 6:23820. doi: 10.1038/srep23820
160. Cui, X, Ye, Z, Wang, D, Yang, Y, Jiao, C, Ma, J, et al. Aryl hydrocarbon receptor activation ameliorates experimental colitis by modulating the tolerogenic dendritic and regulatory T cell formation. Cell Biosci. (2022) 12:46. doi: 10.1186/s13578-022-00780-z
161. Barroso, A, Mahler, JV, Fonseca-Castro, PH, and Quintana, FJ. Therapeutic induction of tolerogenic dendritic cells via aryl hydrocarbon receptor signaling. Curr Opin Immunol. (2021) 70:33–9. doi: 10.1016/j.coi.2021.02.003
162. Salazar, F, Awuah, D, Negm, OH, Shakib, F, and Ghaemmaghami, AM. The role of indoleamine 2,3-dioxygenase-aryl hydrocarbon receptor pathway in the TLR4-induced tolerogenic phenotype in human DCs. Sci Rep. (2017) 7:43337. doi: 10.1038/srep43337
163. Riazati, N, Kable, ME, Newman, JW, Adkins, Y, Freytag, T, Jiang, X, et al. Associations of microbial and indoleamine-2,3-dioxygenase-derived tryptophan metabolites with immune activation in healthy adults. Front Immunol. (2022) 13:917966. doi: 10.3389/fimmu.2022.917966
164. Tintelnot, J, Xu, Y, Lesker, TR, Schönlein, M, Konczalla, L, Giannou, AD, et al. Microbiota-derived 3-IAA influences chemotherapy efficacy in pancreatic cancer. Nature. (2023) 615:168–74. doi: 10.1038/s41586-023-05728-y
165. Huang, C, Liu, LY, Song, TS, Ni, L, Yang, L, Hu, XY, et al. Apoptosis of pancreatic cancer BXPC-3 cells induced by indole-3-acetic acid in combination with horseradish peroxidase. World J Gastroenterol. (2005) 11:4519–23. doi: 10.3748/wjg.v11.i29.4519
166. Frankel, TL, and Pasca di Magliano, M. Immune sensing of microbial metabolites: action at the tumor. Immunity. (2022) 55:192–4. doi: 10.1016/j.immuni.2022.01.009
167. Hezaveh, K, Shinde, RS, Klötgen, A, Halaby, MJ, Lamorte, S, Ciudad, MT, et al. Tryptophan-derived microbial metabolites activate the aryl hydrocarbon receptor in tumor-associated macrophages to suppress anti-tumor immunity. Immunity. (2022) 55:324–340.e8. doi: 10.1016/j.immuni.2022.01.006
168. Sipos, A, Ujlaki, G, Mikó, E, Maka, E, Szabó, J, Uray, K, et al. The role of the microbiome in ovarian cancer: mechanistic insights into oncobiosis and to bacterial metabolite signaling. Mol Med. (2021) 27:33. doi: 10.1186/s10020-021-00295-2
169. Delfini, M, Stakenborg, N, Viola, MF, and Boeckxstaens, G. Macrophages in the gut: masters in multitasking. Immunity. (2022) 55:1530–48. doi: 10.1016/j.immuni.2022.08.005
170. Yang, Y, Li, L, Xu, C, Wang, Y, Wang, Z, Chen, M, et al. Cross-talk between the gut microbiota and monocyte-like macrophages mediates an inflammatory response to promote colitis-associated tumourigenesis. Gut. (2020) 70:1495–506. doi: 10.1136/gutjnl-2020-320777
171. Shapouri-Moghaddam, A, Mohammadian, S, Vazini, H, Taghadosi, M, Esmaeili, SA, Mardani, F, et al. Macrophage plasticity, polarization, and function in health and disease. J Cell Physiol. (2018) 233:6425–40. doi: 10.1002/jcp.26429
172. Mantovani, A, Sozzani, S, Locati, M, Allavena, P, and Sica, A. Macrophage polarization: tumor-associated macrophages as a paradigm for polarized M2 mononuclear phagocytes. Trends Immunol. (2002) 23:549–55. doi: 10.1016/S1471-4906(02)02302-5
173. Whitfield-Cargile, CM, Cohen, ND, Chapkin, RS, Weeks, BR, Davidson, LA, Goldsby, JS, et al. The microbiota-derived metabolite indole decreases mucosal inflammation and injury in a murine model of NSAID enteropathy. Gut Microbes. (2016) 7:246–61. doi: 10.1080/19490976.2016.1156827
174. Ji, Y, Gao, Y, Chen, H, Yin, Y, and Zhang, W. Indole-3-acetic acid alleviates nonalcoholic fatty liver disease in mice via attenuation of hepatic lipogenesis, and oxidative and inflammatory stress. Nutrients. (2019) 11:2062. doi: 10.3390/nu11092062
175. Krishnan, S, Ding, Y, Saedi, N, Choi, M, Sridharan, GV, Sherr, DH, et al. Gut microbiota-derived tryptophan metabolites modulate inflammatory response in hepatocytes and macrophages. Cell Rep. (2018) 23:1099–111. doi: 10.1016/j.celrep.2018.03.109
176. Fang, H, Fang, M, Wang, Y, Zhang, H, Li, J, Chen, J, et al. Indole-3-propionic acid as a potential therapeutic agent for Sepsis-induced gut microbiota disturbance. Microbiol Spectr. (2022) 10:e0012522. doi: 10.1128/spectrum.00125-22
177. Bansal, T, Alaniz, RC, Wood, TK, and Jayaraman, A. The bacterial signal indole increases epithelial-cell tight-junction resistance and attenuates indicators of inflammation. Proc Natl Acad Sci U S A. (2010) 107:228–33. doi: 10.1073/pnas.0906112107
178. Walter, K, Grosskopf, H, Karkossa, I, von Bergen, M, and Schubert, K. Proteomic characterization of the cellular effects of AhR activation by microbial tryptophan catabolites in endotoxin-activated human macrophages. Int J Environ Res Public Health. (2021) 18:10336. doi: 10.3390/ijerph181910336
179. Ji, Y, Yin, W, Liang, Y, Sun, L, Yin, Y, and Zhang, W. Anti-inflammatory and anti-oxidative activity of Indole-3-acetic acid involves induction of HO-1 and neutralization of free radicals in RAW264.7 cells. Int J Mol Sci. (2020) 21:1579. doi: 10.3390/ijms21051579
180. Ishihara, Y, Kado, SY, Hoeper, C, Harel, S, and Vogel, CFA. Role of NF-kB RelB in aryl hydrocarbon receptor-mediated ligand specific effects. Int J Mol Sci. (2019) 20:2652. doi: 10.3390/ijms20112652
181. Agista, AZ, Tanuseputero, SA, Koseki, T, Ardiansyah, S, Budijanto, H, Sultana, Y, et al. Tryptamine, a microbial metabolite in fermented Rice bran suppressed lipopolysaccharide-induced inflammation in a murine macrophage model. Int J Mol Sci. (2022) 23:11209. doi: 10.3390/ijms231911209
182. Carrasco, E, de Las Heras, MMG, Gabandé-Rodríguez, E, Desdín-Micó, G, Aranda, JF, and Mittelbrunn, M. The role of T cells in age-related diseases. Nat Rev Immunol. (2022) 22:97–111. doi: 10.1038/s41577-021-00557-4
183. Schirmer, M, Garner, A, Vlamakis, H, and Xavier, RJ. Microbial genes and pathways in inflammatory bowel disease. Nat Rev Microbiol. (2019) 17:497–511. doi: 10.1038/s41579-019-0213-6
184. Weng, JR, Tsai, CH, Kulp, SK, and Chen, CS. Indole-3-carbinol as a chemopreventive and anti-cancer agent. Cancer Lett. (2008) 262:153–63. doi: 10.1016/j.canlet.2008.01.033
185. Iliev, ID, and Cadwell, K. Effects of intestinal Fungi and viruses on immune responses and inflammatory bowel diseases. Gastroenterology. (2021) 160:1050–66. doi: 10.1053/j.gastro.2020.06.100
186. Romagnani, S. Th1/Th2 cells. Inflamm Bowel Dis. (1999) 5:285–94. doi: 10.1097/00054725-199911000-00009
187. Kokubo, K, Onodera, A, Kiuchi, M, Tsuji, K, Hirahara, K, and Nakayama, T. Conventional and pathogenic Th2 cells in inflammation, tissue repair, and fibrosis. Front Immunol. (2022) 13:945063. doi: 10.3389/fimmu.2022.945063
188. Hung, SC, Kuo, KL, Huang, HL, Lin, CC, Tsai, TH, Wang, CH, et al. Indoxyl sulfate suppresses endothelial progenitor cell-mediated neovascularization. Kidney Int. (2016) 89:574–85. doi: 10.1016/j.kint.2015.11.020
189. Létourneau, S, Krieg, C, Pantaleo, G, and Boyman, O. IL-2-and CD25-dependent immunoregulatory mechanisms in the homeostasis of T-cell subsets. J Allergy Clin Immunol. (2009) 123:758–62. doi: 10.1016/j.jaci.2009.02.011
190. Cibrián, D, and Sánchez-Madrid, F. CD69: from activation marker to metabolic gatekeeper. Eur J Immunol. (2017) 47:946–53. doi: 10.1002/eji.201646837
191. Hwang, YJ, Yun, MO, Jeong, KT, and Park, JH. Uremic toxin indoxyl 3-sulfate regulates the differentiation of Th2 but not of Th1 cells to lessen allergic asthma. Toxicol Lett. (2014) 225:130–8. doi: 10.1016/j.toxlet.2013.11.027
192. Hwang, WB, Kim, DJ, Oh, GS, and Park, JH. Aryl hydrocarbon receptor ligands Indoxyl 3-sulfate and Indole-3-carbinol inhibit FMS-like tyrosine kinase 3 ligand-induced bone marrow-derived plasmacytoid dendritic cell differentiation. Immune Netw. (2018) 18:e35. doi: 10.4110/in.2018.18.e35
193. Kamal, A, Rao, MP, Swapna, P, Srinivasulu, V, Bagul, C, Shaik, AB, et al. Synthesis of β-carboline-benzimidazole conjugates using lanthanum nitrate as a catalyst and their biological evaluation. Org Biomol Chem. (2014) 12:2370–87. doi: 10.1039/C3OB42236D
194. Nyström, S, Govender, M, Yap, SH, Kamarulzaman, A, Rajasuriar, R, and Larsson, M. HIV-infected individuals on ART with impaired immune recovery have altered plasma metabolite profiles. Open forum. Infect Dis Ther. (2021) 8:ofab288. doi: 10.1093/ofid/ofab288
195. Borghi, M, Pariano, M, Solito, V, Puccetti, M, Bellet, MM, Stincardini, C, et al. Targeting the aryl hydrocarbon receptor with Indole-3-aldehyde protects from vulvovaginal candidiasis via the IL-22-IL-18 cross-talk. Front Immunol. (2019) 10:2364. doi: 10.3389/fimmu.2019.02364
196. Yan, JB, Luo, MM, Chen, ZY, and He, BH. The function and role of the Th17/Treg cell balance in inflammatory bowel disease. J Immunol Res. (2020) 2020:8813558. doi: 10.1155/2020/8813558
197. Scheinecker, C, Göschl, L, and Bonelli, M. Treg cells in health and autoimmune diseases: new insights from single cell analysis. J Autoimmun. (2020) 110:102376. doi: 10.1016/j.jaut.2019.102376
198. Göschl, L, Scheinecker, C, and Bonelli, M. Treg cells in autoimmunity: from identification to Treg-based therapies. Semin Immunopathol. (2019) 41:301–14. doi: 10.1007/s00281-019-00741-8
199. Jiang, P, Zheng, C, Xiang, Y, Malik, S, Su, D, Xu, G, et al. The involvement of TH17 cells in the pathogenesis of IBD. Cytokine Growth Factor Rev. (2023) 69:28–42. doi: 10.1016/j.cytogfr.2022.07.005
200. Ma, F, Hao, H, Gao, X, Cai, Y, Zhou, J, Liang, P, et al. Melatonin ameliorates necrotizing enterocolitis by preventing Th17/Treg imbalance through activation of the AMPK/SIRT1 pathway. Theranostics. (2020) 10:7730–46. doi: 10.7150/thno.45862
201. Liu, YJ, Tang, B, Wang, FC, Tang, L, Lei, YY, Luo, Y, et al. Parthenolide ameliorates colon inflammation through regulating Treg/Th17 balance in a gut microbiota-dependent manner. Theranostics. (2020) 10:5225–41. doi: 10.7150/thno.43716
202. Busbee, PB, Menzel, L, Alrafas, HR, Dopkins, N, Becker, W, Miranda, K, et al. Indole-3-carbinol prevents colitis and associated microbial dysbiosis in an IL-22-dependent manner. JCI Insight. (2020) 5:e127551. doi: 10.1172/jci.insight.127551
203. Rouse, M, Singh, NP, Nagarkatti, PS, and Nagarkatti, M. Indoles mitigate the development of experimental autoimmune encephalomyelitis by induction of reciprocal differentiation of regulatory T cells and Th17 cells. Br J Pharmacol. (2013) 169:1305–21. doi: 10.1111/bph.12205
204. Li, K, Hao, Z, Du, J, Gao, Y, Yang, S, and Zhou, Y. Bacteroides thetaiotaomicron relieves colon inflammation by activating aryl hydrocarbon receptor and modulating CD4(+)T cell homeostasis. Int Immunopharmacol. (2021) 90:107183. doi: 10.1016/j.intimp.2020.107183
205. Montgomery, TL, Eckstrom, K, Lile, KH, Caldwell, S, Heney, ER, Lahue, KG, et al. Lactobacillus reuteri tryptophan metabolism promotes host susceptibility to CNS autoimmunity. Microbiome. (2022) 10:198. doi: 10.1186/s40168-022-01408-7
206. Brown, J, Abboud, G, Ma, L, Choi, SC, Kanda, N, Zeumer-Spataro, L, et al. Microbiota-mediated skewing of tryptophan catabolism modulates CD4(+) T cells in lupus-prone mice. iScience. (2022) 25:104241. doi: 10.1016/j.isci.2022.104241
207. Zhu, C, Xie, Q, and Zhao, B. The role of AhR in autoimmune regulation and its potential as a therapeutic target against CD4 T cell mediated inflammatory disorder. Int J Mol Sci. (2014) 15:10116–35. doi: 10.3390/ijms150610116
208. Liu, Y, Zhou, N, Zhou, L, Wang, J, Zhou, Y, Zhang, T, et al. IL-2 regulates tumor-reactive CD8(+) T cell exhaustion by activating the aryl hydrocarbon receptor. Nat Immunol. (2021) 22:358–69. doi: 10.1038/s41590-020-00850-9
209. Yan, XJ, Qi, M, Telusma, G, Yancopoulos, S, Madaio, M, Satoh, M, et al. Indole-3-carbinol improves survival in lupus-prone mice by inducing tandem B-and T-cell differentiation blockades. Clin Immunol. (2009) 131:481–94. doi: 10.1016/j.clim.2009.01.013
210. Safa, M, Tavasoli, B, Manafi, R, Kiani, F, Kashiri, M, Ebrahimi, S, et al. Indole-3-carbinol suppresses NF-κB activity and stimulates the p53 pathway in pre-B acute lymphoblastic leukemia cells. Tumour Biol. (2015) 36:3919–30. doi: 10.1007/s13277-014-3035-1
211. Pierre, S, Chevallier, A, Teixeira-Clerc, F, Ambolet-Camoit, A, Bui, LC, Bats, AS, et al. Aryl hydrocarbon receptor-dependent induction of liver fibrosis by dioxin. Toxicol Sci. (2014) 137:114–24. doi: 10.1093/toxsci/kft236
212. Heath-Pagliuso, S, Rogers, WJ, Tullis, K, Seidel, SD, Cenijn, PH, Brouwer, A, et al. Activation of the ah receptor by tryptophan and tryptophan metabolites. Biochemistry. (1998) 37:11508–15. doi: 10.1021/bi980087p
213. Allaire, JM, Crowley, SM, Law, HT, Chang, SY, Ko, HJ, and Vallance, BA. The intestinal epithelium: central coordinator of mucosal immunity: (trends in immunology 39, 677-696, 2018). Trends Immunol. (2019) 40:174. doi: 10.1016/j.it.2018.12.008
214. Kirschner, N, and Brandner, JM. Barriers and more: functions of tight junction proteins in the skin. Ann N Y Acad Sci. (2012) 1257:158–66. doi: 10.1111/j.1749-6632.2012.06554.x
215. Lessey, LR, Robinson, SC, Chaudhary, R, and Daniel, JM. Adherens junction proteins on the move-from the membrane to the nucleus in intestinal diseases. Front Cell Dev Biol. (2022) 10:998373. doi: 10.3389/fcell.2022.998373
216. Perez-Lopez, A, Behnsen, J, Nuccio, SP, and Raffatellu, M. Mucosal immunity to pathogenic intestinal bacteria. Nat Rev Immunol. (2016) 16:135–48. doi: 10.1038/nri.2015.17
217. Huang, W, Cho, KY, Meng, D, and Walker, WA. The impact of indole-3-lactic acid on immature intestinal innate immunity and development: a transcriptomic analysis. Sci Rep. (2021) 11:8088. doi: 10.1038/s41598-021-87353-1
218. Mu, Q, Kirby, J, Reilly, CM, and Luo, XM. Leaky gut as a danger signal for autoimmune diseases. Front Immunol. (2017) 8:598. doi: 10.3389/fimmu.2017.00598
219. Bertola, A, Mathews, S, Ki, SH, Wang, H, and Gao, B. Mouse model of chronic and binge ethanol feeding (the NIAAA model). Nat Protoc. (2013) 8:627–37. doi: 10.1038/nprot.2013.032
220. Scott, SA, Fu, J, and Chang, PV. Microbial tryptophan metabolites regulate gut barrier function via the aryl hydrocarbon receptor. Proc Natl Acad Sci U S A. (2020) 117:19376–87. doi: 10.1073/pnas.2000047117
221. Ma, TY, Boivin, MA, Ye, D, Pedram, A, and Said, HM. Mechanism of TNF-{alpha} modulation of Caco-2 intestinal epithelial tight junction barrier: role of myosin light-chain kinase protein expression. Am J Physiol Gastrointest Liver Physiol. (2005) 288:G422–30. doi: 10.1152/ajpgi.00412.2004
222. Ma, TY, Iwamoto, GK, Hoa, NT, Akotia, V, Pedram, A, Boivin, MA, et al. TNF-alpha-induced increase in intestinal epithelial tight junction permeability requires NF-kappa B activation. Am J Physiol Gastrointest Liver Physiol. (2004) 286:G367–76. doi: 10.1152/ajpgi.00173.2003
223. Wang, F, Graham, WV, Wang, Y, Witkowski, ED, Schwarz, BT, and Turner, JR. Interferon-gamma and tumor necrosis factor-alpha synergize to induce intestinal epithelial barrier dysfunction by up-regulating myosin light chain kinase expression. Am J Pathol. (2005) 166:409–19. doi: 10.1016/S0002-9440(10)62264-X
224. Madsen, KL, Lewis, SA, Tavernini, MM, Hibbard, J, and Fedorak, RN. Interleukin 10 prevents cytokine-induced disruption of T84 monolayer barrier integrity and limits chloride secretion. Gastroenterology. (1997) 113:151–9. doi: 10.1016/S0016-5085(97)70090-8
225. Madsen, KL, Malfair, D, Gray, D, Doyle, JS, Jewell, LD, and Fedorak, RN. Interleukin-10 gene-deficient mice develop a primary intestinal permeability defect in response to enteric microflora. Inflamm Bowel Dis. (1999) 5:262–70. doi: 10.1097/00054725-199911000-00004
226. Shimada, Y, Kinoshita, M, Harada, K, Mizutani, M, Masahata, K, Kayama, H, et al. Commensal bacteria-dependent indole production enhances epithelial barrier function in the colon. PLoS One. (2013) 8:e80604. doi: 10.1371/journal.pone.0080604
227. Park, I, Nam, H, Goo, D, Wickramasuriya, SS, Zimmerman, N, Smith, AH, et al. Gut microbiota-derived Indole-3-carboxylate influences mucosal integrity and immunity through the activation of the aryl hydrocarbon receptors and nutrient transporters in broiler chickens challenged with Eimeria maxima. Front Immunol. (2022) 13:867754. doi: 10.3389/fimmu.2022.867754
228. Li, J, Zhang, L, Wu, T, Li, Y, Zhou, X, and Ruan, Z. Indole-3-propionic acid improved the intestinal barrier by enhancing epithelial barrier and mucus barrier. J Agric Food Chem. (2021) 69:1487–95. doi: 10.1021/acs.jafc.0c05205
229. Rutz, S, Wang, X, and Ouyang, W. The IL-20 subfamily of cytokines--from host defence to tissue homeostasis. Nat Rev Immunol. (2014) 14:783–95. doi: 10.1038/nri3766
230. Sonnenberg, GF, and Artis, D. Innate lymphoid cell interactions with microbiota: implications for intestinal health and disease. Immunity. (2012) 37:601–10. doi: 10.1016/j.immuni.2012.10.003
231. Sawa, S, Lochner, M, Satoh-Takayama, N, Dulauroy, S, Bérard, M, Kleinschek, M, et al. RORγt+ innate lymphoid cells regulate intestinal homeostasis by integrating negative signals from the symbiotic microbiota. Nat Immunol. (2011) 12:320–6. doi: 10.1038/ni.2002
232. Hou, Q, Ye, L, Liu, H, Huang, L, Yang, Q, Turner, JR, et al. Lactobacillus accelerates ISCs regeneration to protect the integrity of intestinal mucosa through activation of STAT3 signaling pathway induced by LPLs secretion of IL-22. Cell Death Differ. (2018) 25:1657–70. doi: 10.1038/s41418-018-0070-2
233. Wang, L, Fouts, DE, Stärkel, P, Hartmann, P, Chen, P, Llorente, C, et al. Intestinal REG3 lectins protect against alcoholic steatohepatitis by reducing mucosa-associated microbiota and preventing bacterial translocation. Cell Host Microbe. (2016) 19:227–39. doi: 10.1016/j.chom.2016.01.003
234. Zhang, YZ, and Li, YY. Inflammatory bowel disease: pathogenesis. World J Gastroenterol. (2014) 20:91–9. doi: 10.3748/wjg.v20.i1.91
235. Le Berre, C, Ananthakrishnan, AN, Danese, S, Singh, S, and Peyrin-Biroulet, L. Ulcerative colitis and Crohn's disease have similar burden and goals for treatment. Clin Gastroenterol Hepatol. (2020) 18:14–23. doi: 10.1016/j.cgh.2019.07.005
236. Hashimoto, T, Perlot, T, Rehman, A, Trichereau, J, Ishiguro, H, Paolino, M, et al. ACE2 links amino acid malnutrition to microbial ecology and intestinal inflammation. Nature. (2012) 487:477–81. doi: 10.1038/nature11228
237. Alexeev, EE, Lanis, JM, Kao, DJ, Campbell, EL, Kelly, CJ, Battista, KD, et al. Microbiota-derived indole metabolites promote human and murine intestinal homeostasis through regulation of Interleukin-10 receptor. Am J Pathol. (2018) 188:1183–94. doi: 10.1016/j.ajpath.2018.01.011
238. Liu, S, Zhao, W, Lan, P, and Mou, X. The microbiome in inflammatory bowel diseases: from pathogenesis to therapy. Protein Cell. (2021) 12:331–45. doi: 10.1007/s13238-020-00745-3
239. Yang, Y, Zheng, X, Wang, Y, Tan, X, Zou, H, Feng, S, et al. Human fecal microbiota transplantation reduces the susceptibility to dextran sulfate sodium-induced germ-free mouse colitis. Front Immunol. (2022) 13:836542. doi: 10.3389/fimmu.2022.1081546
240. Endharti, AT, and Permana, S. Extract from mango mistletoes Dendrophthoe pentandra ameliorates TNBS-induced colitis by regulating CD4+ T cells in mesenteric lymph nodes. BMC Complement Altern Med. (2017) 17:468. doi: 10.1186/s12906-017-1973-z
241. Lin, H, Zhang, W, Jiang, X, Chen, R, Huang, X, and Huang, Z. Total glucosides of paeony ameliorates TNBS-induced colitis by modulating differentiation of Th17/Treg cells and the secretion of cytokines. Mol Med Rep. (2017) 16:8265–76. doi: 10.3892/mmr.2017.7598
242. Yang, F, Wang, D, Li, Y, Sang, L, Zhu, J, Wang, J, et al. Th1/Th2 balance and Th17/Treg-mediated immunity in relation to murine resistance to dextran sulfate-induced colitis. J Immunol Res. (2017) 2017:7047201. doi: 10.1155/2017/7047201
243. Flannigan, KL, Nieves, KM, Szczepanski, HE, Serra, A, Lee, JW, Alston, LA, et al. The Pregnane X receptor and Indole-3-propionic acid shape the intestinal mesenchyme to restrain inflammation and fibrosis. Cell Mol Gastroenterol Hepatol. (2023) 15:765–95. doi: 10.1016/j.jcmgh.2022.10.014
244. Rogler, G, Singh, A, Kavanaugh, A, and Rubin, DT. Extraintestinal manifestations of inflammatory bowel disease: current concepts, treatment, and implications for disease management. Gastroenterology. (2021) 161:1118–32. doi: 10.1053/j.gastro.2021.07.042
245. Li, MC, and He, SH. IL-10 and its related cytokines for treatment of inflammatory bowel disease. World J Gastroenterol. (2004) 10:620–5. doi: 10.3748/wjg.v10.i5.620
246. Hasnain, SZ, Tauro, S, Das, I, Tong, H, Chen, AC, Jeffery, PL, et al. IL-10 promotes production of intestinal mucus by suppressing protein misfolding and endoplasmic reticulum stress in goblet cells. Gastroenterology. (2013) 144:357–368.e9. doi: 10.1053/j.gastro.2012.10.043
247. Begue, B, Verdier, J, Rieux-Laucat, F, Goulet, O, Morali, A, Canioni, D, et al. Defective IL10 signaling defining a subgroup of patients with inflammatory bowel disease. Am J Gastroenterol. (2011) 106:1544–55. doi: 10.1038/ajg.2011.112
248. Li, D, Yu, S, Long, Y, Shi, A, Deng, J, Ma, Y, et al. Tryptophan metabolism: mechanism-oriented therapy for neurological and psychiatric disorders. Front Immunol. (2022) 13:985378. doi: 10.3389/fimmu.2022.1072573
249. Portaccio, E, Fonderico, M, Iaffaldano, P, Pastò, L, Razzolini, L, Bellinvia, A, et al. Disease-modifying treatments and time to loss of ambulatory function in patients with primary progressive multiple sclerosis. JAMA Neurol. (2022) 79:869–78. doi: 10.1001/jamaneurol.2022.1929
250. Rothhammer, V, Borucki, DM, Tjon, EC, Takenaka, MC, Chao, CC, Ardura-Fabregat, A, et al. Microglial control of astrocytes in response to microbial metabolites. Nature. (2018) 557:724–8. doi: 10.1038/s41586-018-0119-x
251. Sun, J, Zhang, Y, Kong, Y, Ye, T, Yu, Q, Kumaran Satyanarayanan, S, et al. Microbiota-derived metabolite indoles induced aryl hydrocarbon receptor activation and inhibited neuroinflammation in APP/PS1 mice. Brain Behav Immun. (2022) 106:76–88. doi: 10.1016/j.bbi.2022.08.003
252. Salminen, A. Activation of aryl hydrocarbon receptor (AhR) in Alzheimer's disease: role of tryptophan metabolites generated by gut host-microbiota. J Mol Med. (2023) 101:201–22. doi: 10.1007/s00109-023-02289-5
253. Juricek, L, and Coumoul, X. The aryl hydrocarbon receptor and the nervous system. Int J Mol Sci. (2018) 19:2504. doi: 10.3390/ijms19092504
254. Rothhammer, V, Mascanfroni, ID, Bunse, L, Takenaka, MC, Kenison, JE, Mayo, L, et al. Type I interferons and microbial metabolites of tryptophan modulate astrocyte activity and central nervous system inflammation via the aryl hydrocarbon receptor. Nat Med. (2016) 22:586–97. doi: 10.1038/nm.4106
255. Chyan, YJ, Poeggeler, B, Omar, RA, Chain, DG, Frangione, B, Ghiso, J, et al. Potent neuroprotective properties against the Alzheimer beta-amyloid by an endogenous melatonin-related indole structure, indole-3-propionic acid. J Biol Chem. (1999) 274:21937–42. doi: 10.1074/jbc.274.31.21937
256. Serrano-Pozo, A, Das, S, and Hyman, BT. APOE and Alzheimer's disease: advances in genetics, pathophysiology, and therapeutic approaches. Lancet Neurol. (2021) 20:68–80. doi: 10.1016/S1474-4422(20)30412-9
257. Qian, C, Yang, C, Lu, M, Bao, J, Shen, H, Deng, B, et al. Activating AhR alleviates cognitive deficits of Alzheimer's disease model mice by upregulating endogenous Aβ catabolic enzyme Neprilysin. Theranostics. (2021) 11:8797–812. doi: 10.7150/thno.61601
258. Wu, L, Han, Y, Zheng, Z, Peng, G, Liu, P, Yue, S, et al. Altered gut microbial metabolites in amnestic mild cognitive impairment and Alzheimer's disease: signals in host-microbe interplay. Nutrients. (2021) 13. doi: 10.3390/nu13010228
259. Shao, Y, Ouyang, Y, Li, T, Liu, X, Xu, X, Li, S, et al. Alteration of metabolic profile and potential biomarkers in the plasma of Alzheimer's disease. Aging Dis. (2020) 11:1459–70. doi: 10.14336/AD.2020.0217
260. Bendheim, PE, Poeggeler, B, Neria, E, Ziv, V, Pappolla, MA, and Chain, DG. Development of indole-3-propionic acid (OXIGON) for Alzheimer's disease. J Mol Neurosci. (2002) 19:213–7. doi: 10.1007/s12031-002-0036-0
261. Koyambo-Konzapa, SJ, Mbesse Kongbonga, GY, Ramlina Vamhindi, PRBSD, Nsangou, M, and Franklin Benial, AM. Spectroscopic, quantum chemical, molecular docking and molecular dynamics investigations of hydroxylic indole-3-pyruvic acid: a potent candidate for nonlinear optical applications and Alzheimer's drug. J Biomol Struct Dyn. (2022) 40:10651–64. doi: 10.1080/07391102.2021.1947380
262. Shen, H, Guan, Q, Zhang, X, Yuan, C, Tan, Z, Zhai, L, et al. New mechanism of neuroinflammation in Alzheimer's disease: the activation of NLRP3 inflammasome mediated by gut microbiota. Prog Neuro-Psychopharmacol Biol Psychiatry. (2020) 100:109884. doi: 10.2147/JIR.S350109
263. Tolosa, E, Garrido, A, Scholz, SW, and Poewe, W. Challenges in the diagnosis of Parkinson's disease. Lancet Neurol. (2021) 20:385–97. doi: 10.1016/S1474-4422(21)00030-2
264. Mohamad, KA, El-Naga, RN, and Wahdan, SA. Neuroprotective effects of indole-3-carbinol on the rotenone rat model of Parkinson's disease: impact of the SIRT1-AMPK signaling pathway. Toxicol Appl Pharmacol. (2022) 435:115853. doi: 10.1016/j.taap.2021.115853
265. Saini, N, Akhtar, A, Chauhan, M, Dhingra, N, and Pilkhwal Sah, S. Protective effect of Indole-3-carbinol, an NF-κB inhibitor in experimental paradigm of Parkinson's disease: in silico and in vivo studies. Brain Behav Immun. (2020) 90:108–37. doi: 10.1016/j.bbi.2020.08.001
266. Mimori, S, Kawada, K, Saito, R, Takahashi, M, Mizoi, K, Okuma, Y, et al. Indole-3-propionic acid has chemical chaperone activity and suppresses endoplasmic reticulum stress-induced neuronal cell death. Biochem Biophys Res Commun. (2019) 517:623–8. doi: 10.1016/j.bbrc.2019.07.074
267. Montgomery, TL, Künstner, A, Kennedy, JJ, Fang, Q, Asarian, L, Culp-Hill, R, et al. Interactions between host genetics and gut microbiota determine susceptibility to CNS autoimmunity. Proc Natl Acad Sci U S A. (2020) 117:27516–27. doi: 10.1073/pnas.2002817117
268. Li, D, Li, Y, Yang, S, Lu, J, Jin, X, and Wu, M. Diet-gut microbiota-epigenetics in metabolic diseases: from mechanisms to therapeutics. Biomed Pharmacother. (2022) 153:113290. doi: 10.1016/j.biopha.2022.113290
269. Aron-Wisnewsky, J, Warmbrunn, MV, Nieuwdorp, M, and Clément, K. Metabolism and metabolic disorders and the microbiome: the intestinal microbiota associated with obesity, lipid metabolism, and metabolic health-pathophysiology and therapeutic strategies. Gastroenterology. (2021) 160:573–99. doi: 10.1053/j.gastro.2020.10.057
270. Natividad, JM, Agus, A, Planchais, J, Lamas, B, Jarry, AC, Martin, R, et al. Impaired aryl hydrocarbon receptor ligand production by the gut microbiota is a key factor in metabolic syndrome. Cell Metab. (2018) 28:737–749.e4. doi: 10.1016/j.cmet.2018.07.001
271. Su, X, Zhang, M, Qi, H, Gao, Y, Yang, Y, Yun, H, et al. Gut microbiota-derived metabolite 3-idoleacetic acid together with LPS induces IL-35(+) B cell generation. Microbiome. (2022) 10:13. doi: 10.1186/s40168-021-01205-8
272. de Mello, VD, Paananen, J, Lindström, J, Lankinen, MA, Shi, L, Kuusisto, J, et al. Indolepropionic acid and novel lipid metabolites are associated with a lower risk of type 2 diabetes in the Finnish diabetes prevention study. Sci Rep. (2017) 7:46337. doi: 10.1038/srep46337
Keywords: tryptophan, indole derivatives, immunity, AhR, microorganism
Citation: Li S (2023) Modulation of immunity by tryptophan microbial metabolites. Front. Nutr. 10:1209613. doi: 10.3389/fnut.2023.1209613
Edited by:
George Grant, University of Aberdeen, United KingdomReviewed by:
Laurence Morel, The University of Texas Health Science Center at San Antonio, United StatesYogesh Singh, University of Tübingen, Germany
Copyright © 2023 Li. This is an open-access article distributed under the terms of the Creative Commons Attribution License (CC BY). The use, distribution or reproduction in other forums is permitted, provided the original author(s) and the copyright owner(s) are credited and that the original publication in this journal is cited, in accordance with accepted academic practice. No use, distribution or reproduction is permitted which does not comply with these terms.
*Correspondence: Siying Li, bGlzaXlpbmdAc3R1Lmh1bmF1LmVkdS5jbg==