- 1Department of Dairy Science and Food Technology, Institute of Agricultural Sciences, Banaras Hindu University, Varanasi, India
- 2Department of Livestock Production Management, Sri Karan Narendra Agriculture University, Jobner, India
- 3Department of Agriculture Economics, Sri Karan Narendra Agriculture University, Jobner, India
- 4Division of Dairy Technology, ICAR—National Dairy Research Institute, Karnal, India
- 5Department of Food Technology and Science, Faculty of Agriculture and Veterinary Medicine, Ibb University, Ibb, Yemen
Human milk is considered the most valuable form of nutrition for infants for their growth, development and function. So far, there are still some cases where feeding human milk is not feasible. As a result, the market for infant formula is widely increasing, and formula feeding become an alternative or substitute for breastfeeding. The nutritional value of the formula can be improved by adding functional bioactive compounds like probiotics, prebiotics, human milk oligosaccharides, vitamins, minerals, taurine, inositol, osteopontin, lactoferrin, gangliosides, carnitine etc. For processing of infant formula, diverse thermal and non-thermal technologies have been employed. Infant formula can be either in powdered form, which requires reconstitution with water or in ready-to-feed liquid form, among which powder form is readily available, shelf-stable and vastly marketed. Infants’ gut microbiota is a complex ecosystem and the nutrient composition of infant formula is recognized to have a lasting effect on it. Likewise, the gut microbiota establishment closely parallels with host immune development and growth. Therefore, it must be contemplated as an important factor for consideration while developing formulas. In this review, we have focused on the formulation and manufacturing of safe and nutritious infant formula equivalent to human milk or aligning with the infant’s needs and its ultimate impact on infants’ gut microbiota.
1. Introduction
Nowadays, the major driving forces for the acceptance and consumption of food items on a global scale are their functionality and the health benefits imparted by them. Food production is a foremost and significant driver of environmental changes like biodiversity loss, water pollution and deforestation (1). Alongside, malnutrition in its varied forms involving undernutrition, non-communicable diseases related to diet, and unhealthy diets are the chief contributors to the global burden of several diseases (2, 3). The prevalence of malnutrition heralds a global epidemic that presents difficulties for public health (4). Viewing all such issues, food security and nutrition must be the global political priority (5). Consequently, the development and formulation of health-imparting formulas are gaining more attention from policymakers, researchers, and advocates (6, 7).
Regarding infant nutrition, breastfeeding is a compelling force contributing to a child’s sustainable growth and development. It is a biological first food that is nutritionally optimized, safe for consumption and protects the child against many infections (8). It can reduce stress for both the infant and mother, imparting the child–mother bonding and can literally be called ‘packed with love’ (9). Breastfed children are more likely to achieve full intellectual potential and can ultimately perform better in later life (7). Breastfeeding has advantages for mothers in terms of birth spacing and decreased incidence of breast and ovarian cancer. Furthermore, the environmental cost of breastfeeding is negligible, whereas the economic loss from non-breastfeeding is estimated to be greater than USD 300 billion annually (10, 11). However, some mothers are unable to breastfeed their infants because of medical or physiological reasons like poor mammary gland development (mammary hypoplasia) and hormonal imbalances (12, 13). Nonetheless, promotion and marketing of breastmilk substitutes are thought to be the supplementary hurdle for breastfeeding. Exposure of individuals to this strategic marketing led to reduced breastfeeding initiation and duration, irrespective of the country (7, 14, 15).
Commercial substitutes for breastfeeding milk formulas are consumed worldwide, defining a partial or complete replacement for breast milk to feed young children or infants between 0–36 months of age (16). Milk formulas are distinguished into three main parts, including standard infant formula (IF) (0–6 months), follow-up formula (6–12 months) and toddler formula (13–36 months). Milk formulas are processed food items with a typical formulation of milk proteins, lactose or other sugars, vegetable oils, micronutrients, and some other additives (17, 18). The IFs are for both the small and large populations of mothers who cannot breastfeed their infants. On the other hand, the follow-up or the toddler formulas are superfluous to human needs. These formulas are manifold expensive compared to regular cow or buffalo milk (19, 20). IF is generally available in 3 forms: liquid, powder and ready-to-feed. The powdered form is the least expensive and is mixed with water for feeding. The concentrated liquid form is supposed to be mixed with an equal amount of water. Among all three, the most expensive is the ready-to-feed form which requires no mixing before feeding (21).
In terms of the IF market, there is a broadening of geographical reach and product ranges (7). In 2021, the infant formula market registered a revenue of approximately 38.17 billion USD. The sector is anticipated to expand at a compound annual growth rate (CARG) of over 10% between 2022 to 2030, driven by the increased prevalence of premature birth (22). A few players, including Nestle, Danone, Abbott, FrieslandCampina and Heinz, dominate the IF market. Together, these companies control nearly 60% global IF market share (23). Moreover, the consumption of these infant formulas is rising day by day because of key drivers such as the increasing number of working mothers, rising cases of malnutrition, a concern about infant nutrition and the growing income of the middle class (7).
Undernourished children in poor or undeveloped countries will likely be deficient in foods rich in high-quality proteins comprising the essential amino acids that are the building block of cognitive development and linear growth (24). Milk possesses physical and nutritional characteristics, making it ideal for complementary food. The digestibility-corrected amino acid score of dairy items is higher than other foods. Milk also contains unique plasma insulin-like growth factor 1 (IGF-1), a growth hormone that increases amino acid uptake (25). It is dense in fat, calories, and many micronutrients, like Vitamin A & B12 and rich in calcium, phosphorous, magnesium and potassium (26). Milk and its products possess antioxidant potential, and this might be due to the sulfur-containing amino acids (cysteine and methionine), vitamins (A, E), antioxidative enzymes (glutathione peroxidase, superoxide dismutase and catalase) and the appreciable amount of the daidzein, a polyphenolic metabolite (27). All of these play a significant role in numerous functions like immunomodulation, cardiovascular, neural, and metabolic growth and the gut microbiome’s establishment (28). In this paper, we have focused on the several components engaged in the formulation of infant formula, their role in the infant’s gut, the manufacturing process, and non-thermal technologies utilized to preserve nutritional value and extension of shelf-life.
2. Choice of milk or milk substitute for IF formulations
The chemical nature of human milk is complex. The general composition involves 87–88% water, 1.0% protein, 3.8% fat and about 7% lactose (29). Lactose and fat majorly contribute to total energy (30). Although its composition is very dynamic and varies over time according to the needs of a growing child, the lactose content remains fairly constant (after 21 days of parturition). Another function of lactose is maintaining constant osmotic pressure and aiding in mineral absorption (21). Human milk possesses two classes of protein, namely whey and casein. The whey remains liquid in an infant’s stomach, making it easier to digest, while the casein forms clots or curds. The major whey proteins include lactoferrin, alpha-lactalbumin and secretary IgA. Other proteins include folate-binding protein, lipase, amylase, lysozyme, Bifidus factor, anti-chymotrypsin and alpha-1-antitrypsin, and haptocorrin (31). In terms of vitamins and minerals, human milk possesses an adequate amount to support the normal growth of an infant, except vitamins K and D. The role of minerals is their contribution in various physiological functions and formation of essential amino acids and are biologically crucial to structural and catalytic molecules (21).
Besides human milk, bovine milk is also a good source of fats and lipids, proteins, carbohydrates, minerals, and vitamins. The protein and mineral content of bovine milk is higher when compared to human milk. Also, the lactose content of human milk is approximately 7 percent [involving 1% of oligosaccharides (OSs)], while bovine milk has a 4.5% lactose content. In contrast, the amount of whey protein in both kinds of milk is approximately similar (32). The casein in bovine milk is eight folds higher if compared to human milk. All such differences should be considered while formulating IF. Also, the whey-to-casein ratio (20, 80) of bovine milk should be modified in such a way that it mimics the mature human milk’s whey-to-casein ratio, i.e., 60:40 (33). For a quick illustration, a brief mention of the nutritional composition of milk from different milk species is presented in Table 1. Furthermore, mare, camel and llama milks have a higher Ig (Ig) content than cow, goat, sheep, and human milk. Donkey milk is comparable to human milk as it contains lower casein and higher serum protein. Even the lysozyme level of donkey milk is two times higher than human milk, corresponding to its higher antimicrobial activity. Moreover, human milk and mare’s milk contain less saturated fatty acids than the milks of other species (34). Lipid classes in selected milks include cerebrosides in camel, deer & buffalo; gangliosides in deer, sheep & camel; and plasmalogens in buffalo, deer & goat (35).
Bovine milk is used as a primary base material for the formulation of infant formulas because of its higher volume production, well-established chain distributions, and many recognized functional attributes of its components (36). Nonetheless, sometimes infants suffer from cow’s milk allergies (CMA), which is an immune-mediated reaction. Here IgE antibodies bind to the surface of mast cells, and subsequent exposure leads to degranulation of mast cells and release of mediators, including histamine & leukotrienes. All this causes symptoms involving throat tightness, urticaria, angioedema, abdominal pain, diarrhea, vomiting and dizziness (37, 38). In such cases, camel, donkey milk-based IF can be an alternative option. Human and donkey milk has a similar composition as well as antigenic and protein homogeneity (39). Camel milk-based IF has superior anti-inflammatory activity, so this can be a practical option in hypo-allergic IF production (40). Further, other reasons for using alternative formulas can be the disorders related to carbohydrate metabolisms, like deficiency of primary lactase, galactosemia or adopting a vegan lifestyle. However, non-dairy formulas like soy-based formulas can also be given in these cases. At the same time, protein hydrolysates are also developed for infants suffering from soy and milk protein intolerance (33). Consequently, developing newer infant formulas and improving existing ones by enhancing functional properties is a task for researchers and scientists and can be achieved by using milk from different species (41).
2.1. Bioactive peptides in milk
The milk proteins are the primary source of bioactive peptides, which are short-chained sequences of the amino acids and may be released through enzymatic action or in vivo fermentation with starter (lactic acid bacteria) (46). These bioactive peptides are generally small in size and range from 2–50 amino acid residues, and these can be used in the manufacturing of infant formulas (47). In general, Igs, α-LA, β-LG, protease-peptide fractions, lactoferrin, caseins and a minimal amount of whey proteins like transferrin and serum albumin are the major fractions of the proteins present inside bovine milk. These peptides are formed in-vivo by the gastrointestinal (GI) processes. These can also be produced in vitro by enzymatic hydrolysis, encoded in precursor sequences of the native protein. The peptides are later purified by various separation techniques like ultrafiltration, size exclusion, reversed-phased high-performance liquid and ion exchange chromatography (28, 48).
Several bioactive compounds in bovine and caprine milk are effective against cardiovascular, digestive, neurological, immunological and endocrine system disorders, displaying various functional properties like antithrombotic, anti-hypertensive, anti-microbial, anti-oxidant, immunomodulatory and anti-hypertensive activities (49, 50). For example, α- Lactalbumin is broken down into smaller peptides depicting antibacterial, immunostimulatory and prebiotic properties. It also improves the absorption of minerals (51). Parastouei et al. (52) assessed the content of bioactive peptides in milk from different species, including cow, buffalo, goat, camel, sheep, donkey horse and humans. The lowest and highest concentrations of the total peptides resistant to digestion were found in human and sheep’s milk. The casein of donkey milk contained a higher inhibitory peptide of dipeptidyl peptidase IV (DPP- IV) & DPP- III, and angiotensin-converting enzyme (ACE). However, whey protein in camel milk contains inflated ACE-inhibitory peptides. Kumar et al. (53) employed commercial proteases viz. alcalase, papain and chymotrypsin for hydrolyzation of casein fraction in camel milk protein. It was established that the hydrolysate derived possessed higher antioxidant potential and antimicrobial activity.
In another investigative work, Chen et al. (54) utilized Lactobacillus plantarum to ferment goat milk and later evaluated its ability to generate ACE-inhibitory peptides. The produced hydrolysate showed an ACE-inhibitory activity of 88.91%, and after purification by RP-HPLC and ultrafiltration, it displayed 91.62% ACE inhibitory activity. Pei et al. (55) hydrolyzed yak milk by the action of pepsin, and the hydrolysate generated depicted antimicrobial activity against Salmonella paratyphi, E. coli, Enterobacter cloacae and Listeria innocua. Moreover, the glycol-macropeptide of sheep casein has been illustrated to have antiplatelet aggregation activity, and this property increased further with hydrolysis by trypsin. Thus, the increasing interest of researchers in bioactive peptides has lately been augmented exploration of milk bioactive peptides from other species (47). Nowadays, some of these novel components isolated from bovine and caprine milk are commercially available. The important consideration is that while adding these bioactive peptides to IF, lowering the formula’s overall protein concentration is crucial (21). Figure 1 illustrates various classes of bio-peptides derived from milk, along with their functional roles in the health of mankind. For more details about bioactive peptides in milk, readers may refer to Mohanty et al. (46), Guha et al. (47), Nielsen et al. (56) and Park and Nam (57).
3. Formulation of infant formula: ingredients and nutrients
Infant Formulas are developed in a way to maintain a balance of macro and microelements. Typical ingredients for formulating IF are carbohydrates, fats, proteins, vitamins and minerals (33). The ingredients [whey protein isolate (WPI), skim milk powders (SMP), whey protein concentrate (WPC) etc.] made from milk are generally used as a protein source. Mainly vegetable fats are used as the fat source, but animal milk fats can also replace them (58, 59). No matter where the macro and micro elements are isolated, they still affect the physiological properties of the IF to be developed. Numerous clinical trials have been conducted to determine the efficacy of these elements in the formulation of IF; some of them are mentioned in Table 2. Various components and their effect on IF are discussed well in the subsequent sections.
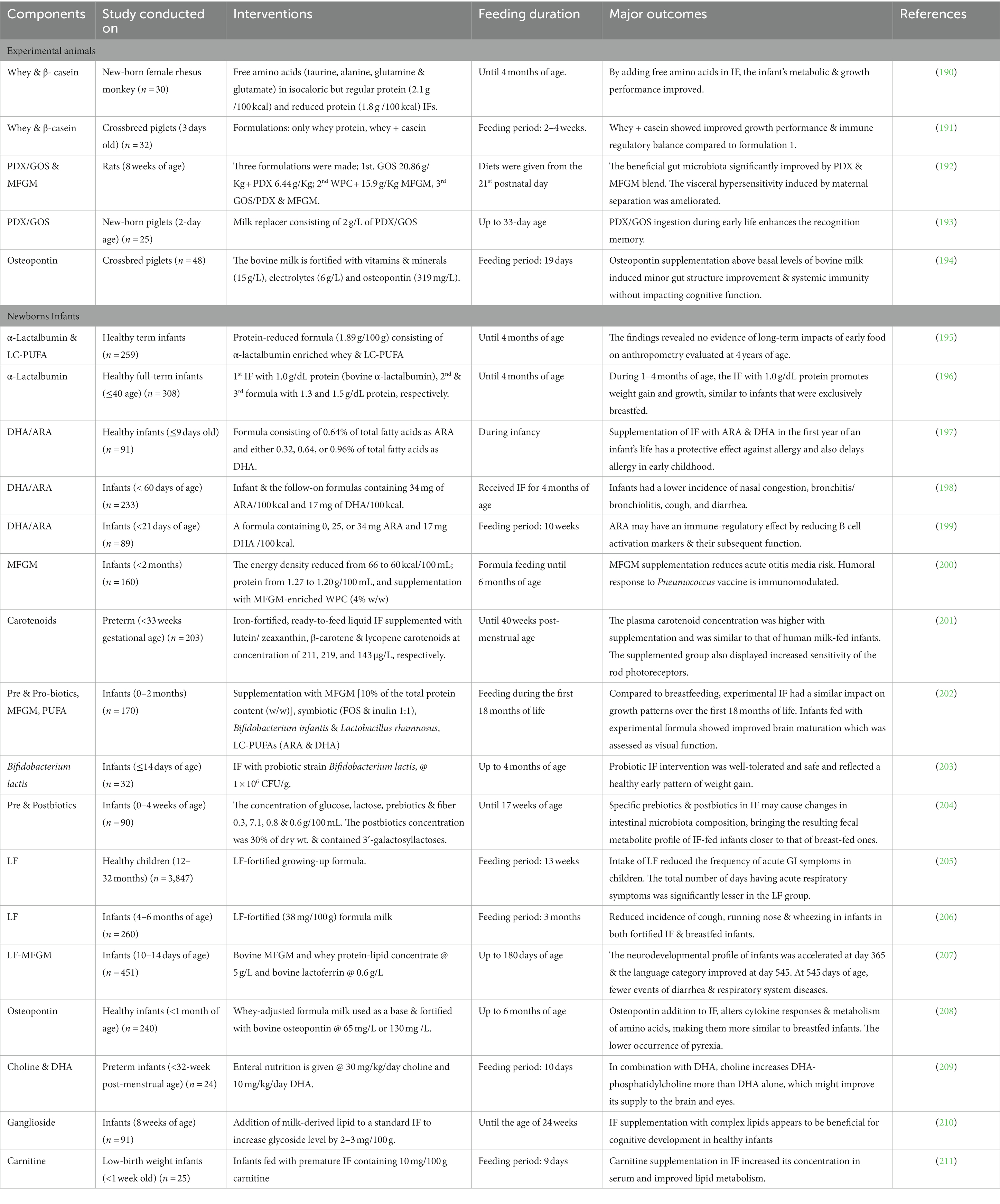
Table 2. Clinical research conducted on the inclusion of various components in formulations of infant formula.
3.1. Carbohydrates
There are two broad categorizations of carbohydrates: non-glycemic and glycemic carbohydrates. The glycemic ones are absorbed and digested in the small intestine and are later followed by increased blood sugar levels. Whereas non-glycemic pass undigested to the large intestine and does not lead to an increase in blood glucose, hence exerting prebiotic effects (60). The EU legislation for the IF specifies the minimum content of the total carbohydrates, which is 9 g/100 kcal (61). The glycemic carbohydrates act as the potential energy source for infants (62). Regarding the bovine and caprine milk-based IFs, lactose is the primary source, consisting of approximately 7–7.5 g/100 mL lactose content. Pure lactose is available from the processing of whey and bovine milk, which is subsequently used for the fortification of IF for matching human milk lactose levels (45). On the contrary, some other sources of carbohydrates are also permitted in IF, like sucrose, maltose, and glucose. These are generally permitted in IF, which are made from protein hydrolysates and are helpful in palatability improvement as they can be bitter (36, 61, 63). The maltodextrin incorporation in food powder has improved properties like resistance to caking and crystallization, reducing Maillard browning, lowering bulk density, and enhancing stability and dispersibility (64).
Non-glycemic carbohydrates involve the OSs present in milk (45). The Human milk OSs (HMOS) are resisted for digestion in the upper GI tract and show the prebiotic effect by acting as a substrate for the growth of many beneficial bacteria like bifidobacteria (65). They can also serve as decoys that prevent toxins and pathogenic bacteria from binding to the target epithelial cells, showing antibacterial activity (66). The composition of different milk sources has already been presented in Table 1. Besides, the concentration of HMOSs is 5–20 g/L, i.e., higher than the milk of other domesticated animals, e.g., bovine 30–60 mg/L, sheep 20–40 mg/L, goat 60–350 mg/L and donkey 250–300 mg/L, respectively (67, 68). The bovine colostrum (day 0) contains more 6′-sialyllactose, disialyllacto-N-tetraose, and LS-tetrasaccharide. Subsequently, their concentration decreases as milk matures (days 3–5) (69). Martin-Ortiz et al. (70) demonstrated that caprine milk OSs decreased with lactation stage like at 488 mg/L (day 1) to 112 mg/L (day 120). The sialylation is higher in colostrum (>80%) but later decreases to approximately 40% on day 120 (71). Likewise in donkey milk lower sialylated OSs were observed at 15th day of lactation (72).
Moreover, the biosynthetic pathways of HMOSs are considerably different from that of free bovine milk OSs (73). The neutral OSs are the predominant ones in human milk, while these are merely present in bovine and caprine milk. Although bovine milk contains fewer OS structures than human milk, these two shares at least ten common OS structures, including the acidic 6′-sialyllactose and 3′-sialyllactose (74). Contrary to that, porcine milk has a higher concentration of neutral OSs (75). Shi et al. (76) reported that camel milk OSs were most similar to human milk compared to cow, goat, camel and sheep milk OSs. Furthermore, the structure of caprine milk OSs is more similar to human milk OSs (45, 66). Lacto-N-biose unit, regarded as a building block of type 1 HMOS, is also found in goat milk OSs. Again, the presence of neutral structures (galactosyl-lactose & lacto-N-hexaose) and sialylated structures (3-6-sialyl-lactose & disialyl-lactose) in human and goat milk OSs, explains the similarities among them (77). Only three acidic OSs in donkey milk have been quantified: 3-sialyllactose, disialyl-lacto-N-tetraose and 6-sialyllactose. But they are present in lower concentrations than in human milk (78). Leong et al. (79) suggested that OSs naturally present in IF based on goats’ milk exhibit strong anti-pathogen adhesion and prebiotic properties and might enhance newborns’ gut health.
3.2. Proteins
The IF composition is strictly regulated by various guidelines set up by governing bodies like the Food and Drug Administration (FDA) in the USA, the EU in Europe and internationally, and the Codex Alimentarius Commission (80). The milk-based IF is formulated in such a way as to contain 1.3–1.5 g/100 mL protein approximately. It is still higher than human milk to compensate for the amount of essential amino acids (81). The L-form of amino acids is only permitted to be added, whereas D-form is not allowed because it can cause D-lactic acidosis (21). The high protein also includes a faster weight gain in infancy and has been later correlated to obesity. Thus, a lower protein intake (1.8 g/100 kcal in IF) is suggested to stimulate health effects for longer period of time (65). In the case of bovine milk, protein content is generally achieved by combining WPC, WPI and SMP (36). Although, for caprine milk based IF, whole milk is used as a base to achieve the required protein concentration (82). Additionally, partially or fully hydrolyzed whey proteins and demineralized powdered whey or hydrolyzed demineralized powdered whey are also utilized to produce IF (83–85). Infants allergic to soy and bovine protein hydrolysates are given the amino acids-based formulas (86).
The protein quality of the IF is its ability to fulfill the metabolic requirements for amino acids and nitrogen. The determination of protein quality is generally assessed by protein-digestibility-corrected amino acid scores (PDCAAS) (87). This method has some limitations, such as the values of PDCAAS are calculated on the fecal digestibility basis of the crude protein, and the digestibility of amino acids is determined accurately at the ileum (88). As a result of all such limitations, FAO recommended digestible indispensable amino acid scores (DIAAS) to replace PDCAAS. In DIAAS method, the digestibility is generally based on the true ileal digestibility in rat or pig models, but most preferably in humans. The pattern of reference to amino-acid scoring of human milk is used for the development of IF (80). Moreover, the source of protein used in IF must also contribute to the required levels of conditionally essential amino acids (61). Mathai et al. (88) reported the values of DIAAS demonstrated in pigs for WPI, WPC, SPI, SMP, and milk protein concentrate (MPC) were 67, 71, 81, 68 and 85, respectively. The drawbacks of this study included the raw protein as a feed, so the study was not reflective of the proteins present in IFs which undergo numerous processing during manufacturing. In another investigation by Maathuis et al. (89), the kinetics of DIAAS and true ileal digestion of protein were determined for human milk (HM), goat milk-based IF (GIF), and cow milk-based IF (CIF). The results showed that the protein quality was not significantly different between HM, GIF and CIF. Still, the protein digestion kinetics of GIF was more comparable to HM than the CIF. The Biological value (BV) of milk is 91%, with the BV of lactalbumin at 104% and casein at 77% (90, 91). In the case of goat and sheep milk protein, BV is 90.9 and 97%, respectively (92, 93). The BV of milk protein can be affected by processing like sterilization due to the loss of lysine and methionine (94). Besides, gamma irradiation also leads to a fall in BV (95).
Protein hydrolysates and peptides generated from major milk proteins, viz., whey and casein proteins, exert immunomodulatory effects involving antibody synthesis, lymphocyte proliferation and cytokine regulation. Immunomodulatory peptides generated from chymosin-pepsin hydrolysis of milk include β-CN f193-202, f191-193, f63-68 (immunopeptides), and αs1-CN f194-199 (αs1-immunocasokinin) (57). Sharma et al. (96) reported some goat milk immunomodulatory peptides, namely, dipeptidyl peptidase, Interleukin 12 subunit, transcription factor A, oligodendrocyte transcription factor 2. The authors also outlined their biological significance in signaling processes of the cytokines and generating cytotoxic lymphocyte, consecutively enhancing immunity. Interleukin 6 (IL-6) is a cytokine that plays a role in hematopoiesis, inflammation, and immunological regulation. Diseases are associated with greater levels of IL-6 expression. Goat milk peptides are capable of binding IL-6 receptors, owing to immunomodulatory activity of these peptides (97). Donkey milk peptides have also been shown to increase the cytokines involved in the onset of acute local inflammatory response and the regulation of innate immunity. Furthermore, whey protein fraction of donkey milk has a molecular mass greater than 10 kDa and stimulate the productivity of certain immune regulatory cytokines like interferon γ (IFN-γ), interleukin-2 (IL-2) by murine splenocytes (98). Ma et al. (99) regarded β-lactoglobulin40-60 from cow milk as an immunomodulatory peptide which directs T lymphocyte activation toward the Th1 phenotype. It can be concluded that there are numerous immunogenic peptides in milk derived from different species. All such components can be isolated and later incorporated while formulating IF based on the varied growing needs of infants.
3.3. Fat
The primary energy source in IF is fats, providing nearly half of the infant’s requirement of energy (100). According to the current guidelines of EU legislation, the fat content of IF must range between 4.4 to 6.0 g/ 100 kcal. In addition, the minimum requirements for essential fatty acids like α-linolenic acid and linoleic acid are also set, which the infants cannot synthesize. Hence, their diet must supply these (61). About 70% of bovine milk has saturated fatty acids. The most common fatty acids are the odd chain fatty acids, butyrate and conjugated linoleic acid (58). Arachidonic acid (ARA) and docosahexaenoic acid (DHA) have roles in the plasma membrane constituent’s development and are lower in bovine milk when compared to human milk. Infants fed with ARA and DHA have lower incidences of allergies, nasal congestion, bronchitis, upper respiratory tract infections, cough, diarrhea, eczema and contact dermatitis (101). In human milk, palmitic acid accounts for approximately 10% of the total energy intake of infants. Henceforth, it is a key nutrient for the development of IF (102). Incorporating various structured triglycerides in IF has been associated with reduced intestinal inflammation and colic instances, modified gut microflora, and improved bone development (103). However, more research is still required to determine the exact PUFA ratio and dose for optimal development and growth of cell-mediated and humoral immunity (101, 104).
Hageman et al. (58) compared and reviewed the role of vegetable oil and bovine milk in the nutrition of infants. The researchers suggested that blending vegetable oil and bovine milk could impart additional health benefits associated with various nutrients possessing similar attributes to human milk fat. This developed model utilizes the specific composition of oils and fats (triacylglycerol, polyunsaturated fatty acids and fatty acids). It also determines the degree of differences and similarities to human milk, ultimately leading to the development of realistic substitutes. A study conducted by Mehrotra et al. (105) revealed that the long-chain saturated fats present in the vegetable oil-based IF are associated with the formation of calcium fatty-acids soaps, which in turn contributes to constipation. Their work suggested that reducing palmitic acid may be a solution for improving stool consistency.
In caprine and bovine milk-based IF, the proteins responsible for the stabilization of fat micelles are whey proteins and casein (106). These protein fractions lead to heat coagulation stability, emulsifying ability and emulsifying stability while processing IF (107, 108). The composition and size of the lipid globules of the lipid/water interface have been demonstrated and shown to affect digestibility (109). There have been attempts to modify the lipid/water interface composition, for example, modification in the phospholipid content of IF via adding the milk phospholipids (110). Since the structure and composition of milk fat globules (MFGs) differ between human, bovine and caprine milk. It is therefore necessary to evaluate the MFGs of these milks, in order to explore a more suitable substitute for breast milk. The content of saturated fatty acids is higher in bovine & caprine MFGs, which is about 60% greater than in human MFGs, but unsaturated fatty acids (C18:2) in human MFGs are seven times higher (111). Furthermore, fat globules of donkey milk are very small (average: 1.92 μm). Their small size represents a bigger surface accessible for lipase action, which may help explain its higher digestibility (112). There is considerable variation in the mean size of MFG for human (4.2–5.1 μm), caprine (2.2–3.9 μm), bovine (2.5–5.7 μm) & ovine mature milk (2.8–4.0 μm) (113). In case of caprine-based milk IF, mostly the goat milk is used as a raw material to achieve desired protein content. IF having goat milk also retains components of Milk Fat Globular Membrane (MFGM) and are linked to metabolic, cognitive, and immune development of the infant (114).
Exosomes are another class of secreted biomolecular nanostructures of lipid bilayers with size ranging from 30–150 nm. These extracellular vesicles are linked to human metabolism, physiology and immunomodulation and have remained as an excellent carrier of biomolecules like proteins, lipids, mRNA, DNA etc. (115). Hock et al. (116) reported that milk derived exosomes (MDEs) promote the viability of intestinal epithelial cells, their proliferation and cell activity. The findings also recommended the use of MDEs as a preventative in the treatment of necrotizing enterocolitis, a lethal disorder affecting the intestines of infants. Moreover, higher proportions of sphingomyelin and phosphatidylserine are being reported in bovine and human exosomes as compared to MFGM (117). The camel milk exosomes have anticancer effects possibly via inhibition of oxidative stress and induction of apoptosis, metastasis and angiogenesis in tumor microenvironment (118). Yak-MDEs help in the improvement of GI development under hypoxic conditions (119). Still, the removal of fat globules and cream before long-term storage of milk is necessary to acquire a higher yield of MDE (120).
3.4. Minerals and vitamins
The human body cannot synthesize many vitamins and minerals, so these must be supplied in the diet. Both caprine and bovine milk are richer in minerals that can contribute to a high load of renal solute in infants, which leads to a higher risk of hypertonic dehydration (36, 121). Various minerals like phosphorous, calcium, sodium, potassium, magnesium and chloride are added in the form of citrates, chlorides, carbonates, phosphates or hydroxides (33). Reducing the protein content in caprine and bovine milk can also lower the ash content, making it suitable for feeding infants. In some cases where the innate mineral levels derived from carbohydrate sources are insufficient, the fortification of mineral salts is necessary. But it is preferable to amplify the amount of intrinsic minerals because the fortified minerals can lead to instability of IF (36). Besides, there is a reduction in bovine milk heat stability by soluble calcium salt addition. This occurred due to increased ionic Ca and decreased pH levels, which in turn led to reduced heat stability, resulting in the formation of undesirable firm coagulum when sterilized (122). Goat milk contains higher free calcium ions because of insufficient citrate levels. Hence, it is necessary to add phosphate and citrate salts for the enhancement of heat stability while preparing the IF from goat milk (36).
Like minerals, IFs are also fortified with vitamins to meet the requirements of infants. The administration of vitamins is not mandatory, though if infants drink less than 500 mL of IF per day, additional A, D, and C vitamins are recommended (123). While manufacturing IF, the ingredients are exposed to several heat treatments, which causes the deterioration of heat-labile vitamins like thiamine and Vitamin C. Therefore, it is essential to compensate for all such losses while processing by fortification. Heat-labile vitamins must be dry-blended with all powdered ingredients after heat treatment in order to avoid such issues (36, 121). As stated by Wang et al. (124), with the addition of vitamins, metal ions and PUFA, the thiobarbituric acid value increased, and the peroxide value decreased in the early storage periods, indicating oxidative deterioration in IF.
Both vitamins and minerals possess the capacity to elicit a particular immune response known as immunogenicity. This depends upon molecular weight, chemical complexity, route of exposure and dose of immunogen (125). Morante-Palacios et al. (126) demonstrated that vitamin C enhances epigenomic reprogramming of nuclear factor-kappa-light-chain-enhancer of B cells (NF-κB), which in turn boosts immunogenic properties of the dendritic cells. Likewise, vitamin E affects the immune system via modulation of protein kinase C. Vitamin A is also involved in immune system development and plays a regulatory role in humoral immunity and cellular immune regulation (127). Calcium, selenium, Phosphorous, and iodine minerals are essential for brain function development; they also help in strengthening the immune system (128). In addition, the low availability of phosphorus weakens the immune responses. Manganese is another micronutrient whose deficiency in the body may lead to impaired antibody production. Sulfur plays a significant role in transport across membranes, biocatalytic processes and immune functions (129). However, it must be acknowledged that excessive consumption of some minerals can have a negative impact on the immune system (130). Molska et al. (131) concluded that even though the concentration of minerals was higher in IF when compared to human milk yet, their absorption was lower.
Venema et al. (132) demonstrated the importance of lower gastric phase pH for the bioaccessibility and solubility of minerals. Bioaccessibility is the fraction of compound liberated during digestion and made available for absorption. Venema et al. (132) also validated that the type of mineral salt had a direct impact on bioaccessibility; salts with a higher solubility (i.e., dipotassium phosphate, calcium glycerophosphate) result in better bioaccessibility as compared to inadequately soluble salts (i.e., calcium carbonate, calcium phosphate). Besides, on average, the bioaccessibility of Zn and Fe is lower than that of other elements. In contrast, the elements K and Mg are known for their higher bioavailability and efficient utilization (133). To overcome the problem of low bioavailability, complexing mineral with certain organic compounds like amino acid chelators has been exploited. For example, caseinophosphopeptides are widely used in the formulation of mineral enriched IF and can improve the bioavailability of Ca, Zn, and Fe (134). Furthermore, the bioavailability of Fe can be enhanced by fortification of water-soluble Fe compounds in IF, such as ferrous fumarate, ferrous sulfate, ferric pyrophosphate or ferric ammonium sulfate (135).
Although the low bioavailability of carotenoids from IF is unclear but can be due to either micellization or poorer release from the matrix, cellular uptake & transport, or further biodistribution and absorption. Interestingly, the bioaccessibility of carotenoids has been reported not to vary significantly between IF and mother’s milk (136). The bioaccessibility of folic acid and vitamin C from IF is also low compared to breast milk, indicating that vitamin C can be encapsulated in a way that it is not quickly released or react with other ingredients of IF. Also, as far as vitamin A is concerned, the lipid content in the IF influences its bioaccessibility (137). Regarding the more bioavailable source of folate, calcium L-methylfolate is safe and is recommended for use in IF supplementation (138). Microcapsules can be prepared by loading vitamins to improve their bioavailability (135). Otadi and Zabihial (139) formulated a heat-cured vitamin E microcapsule with ethyl cellulose whose release was 10–12% slower than a typical microcapsule. Therefore, all the vitamins and minerals must be evaluated well with their effects on the infant’s gut and their ultimate absorption. Secondly, they must be quantified accurately in infant formula using advance analytical tools like liquid chromatography mass spectrometry (LC–MS) or High Performance Liquid Chromatography (140, 141).
3.5. Other ingredients
3.5.1. Lactoferrin
Lactoferrin is one of the most significant bio-activators in milk and other external secretions. It plays a variety of biological tasks, including modulation of the immune responses, iron absorption regulation, antiviral, antimicrobial, antioxidant, anti-inflammatory and anticancer activities (142). Lactoferrin was also successfully added to the formulations of IF, and the resultant formula was safe and tolerated well by the infant, with an expected growth pattern (143). Numerous preclinical investigations using rat and piglet models have shown improved learning and memory capacities due to the lactoferrin-enriched formula (144, 145). According to Wazed et al. (146), the thermal treatment showed better lactoferrin retention than the High-Pressure Processing (HPP) treatment. At a higher temperature, substantial denaturation of lactoferrin was observed. Ultimately the High Temperature Short Time (HTST) pasteurization retained the best quality of Lactoferrin, confirming its possibility to be added to the products of HTST pasteurization.
3.5.2. Osteopontin
Osteopontin is another multifunctional protein involved in many biological processes, including cell proliferation, bone remodeling, immune-modulatory functions and biomineralization. The levels of osteopontin in bovine milk are significantly lower when compared to human milk, and only traces are found in infant formula. This protective mechanism may be an essential factor in a breastfed infant’s ability to ward against sickness. Osteopontin, like lactoferrin, is resistant to in-vivo gastric digestion, and this characteristic is maintained across mammalian species (65). Donovan et al. (147) using rhesus monkeys, reported that the consumption of a formula containing bovine osteopontin had an impact on the expression of many regulatory genes. Moreover, this change produced an expression profile resembling breastfed newborns. This research implies that osteopontin might have health advantages. All these findings support the idea of osteopontin fortification in IF. Further, the FDA recommendation for IF supplementation is 160 mg/L osteopontin (148).
3.5.3. Probiotics and prebiotics
Most of the probiotic strains incorporated into IF are isolated from fecal or food microbiota (21). As validated by several studies conducted so far, the Lactobacillus and Bifidobacterium strains are considered to be the most suitable probiotics for infants. Furthermore, Streptococcus and Propionibacterium are other promising strains to be used as probiotics (149). Prebiotics are indigestible OSs that stimulate bacterial growth and function (150, 151). The infection and stool rate are also lessened in the case of infants fed with prebiotic-supplemented IF (152). It was also found that infants fed with HMO-supplemented formula had a decreased incidence of bronchitis and use of medications such as antibiotics and antipyretics (153). Nowadays, there are seven approved food-grade OSs for inclusion in IF involving lactulose (LOS), inulin, polydextrose (PDX), 2′fucosyllactose (2′-FL), galacto-OS (GOS), fructo-OS (FOS) and N-neo-tetraose (LNnT) (154). Nevertheless, IFs are generally fortified with FOS, GOS and polydextrose prebiotics (151). The addition of lacto-N-neo-tetraose and 2′fucosyllactose has contributed to narrowing the compositional gap between IF and human milk (155).
3.5.4. Choline
Choline is the precursor of the phospholipids, acetylcholine and platelet-activating factor (156). In the case of lactating women intake of 550 mg per day of choline is required, whereas 450 mg per day is needed for pregnant women (157). Choline presence alters the spinal cord and brain structure and can lower the risk of defects in neural tubes (158). Choline supplementation during pregnancy, at nearly twice the recommended dose (930 mg), speeds up the infant’s ability to process information (159). Additional choline may enhance cognitive, affective and neurological functioning when consumed by mothers carrying Down syndrome fetuses (160). Inadequate choline levels can hinder vitamin B12 and folic acid metabolism (65). The IF must contain 25 to 50 mg per 100 kcal of choline, as recommended by the EU legislation (61). If the raw materials lack choline, the IF is fortified with choline salts like choline chloride (80).
3.5.5. miRNA
MicroRNA (miRNA) are short non-coding molecules involved in post-transcriptional gene regulation and have been discovered in cells, lipid fractions and skim milk of humans, which originate from mammary glands (161, 162). miRNA is involved in cell proliferation, apoptosis, differentiation and immune response (163, 164). These are bioavailable in human milk and are transmitted to infants during lactation (165). miRNA is enriched in bovine and human milk but is present in a lesser amount in caprine milk (166). Much research has been conducted to highlight the effects of miRNA on obesity, diabetes, inflammation and cardiovascular diseases in the offspring tissue (167). In addition, Yun et al. (168) confirmed the presence of immune-related miRNAs in both colostrum & mature milk of human, bovine, as well as caprines. Yet, no miRNA has been detected in human or bovine milk-based IF to date. This might be due to the degradation caused by heating or homogenization (169). Although according to Golan-Gerstl et al. (164), pasteurization has a minor effect on the miRNA 148-3p profile expression in skim milk and fat fractions of caprine and bovine milks.
3.5.6. L-carnitine
IF must also contain a compound called L-carnitine, which is an essential nutrient for neonates as they cannot synthesize it for a short period of time. It is a water-soluble molecule that resembles vitamins and is present in various plants, microorganisms and mammalian species. It plays a primary physiological role in the metabolism of fatty acids (80, 170). According to EU legislation, the minimum concentration of 1.2 mg/100 kcal L-carnitine must be present in IF (61). Mikhael (171) suggested carnitine is an essential ingredient of IF, and its deficiency can cause anomalies or infections in infants.
3.5.7. Lutein
Lutein is a carotenoid present in human milk. Its concentration varies according to the maternal diet, i.e., with the intake of vegetables and fruits (172). The function of lutein is to be a structural component of the eye, to act as a filter for blue light and as an antioxidant. It plays physiological and biological roles in an infant’s visual development and function (172, 173). More than two-thirds of the carotenoids, specifically lutein, are enriched in varied sections of the human brain, i.e., temporal and occipital, frontal and cerebellum cortices (174). The bioavailability of lutein in human milk is almost four times higher compared to lutein-fortified IF (175). Recent studies have stated that lutein supplementation in IF is safe and supports cognitive and visual development (176, 177). In present days there are several infant formulas containing lutein (178). Among all the available lutein, crystalline lutein-zeaxanthin is the most popular commercially available form of lutein (179).
3.5.8. Taurine
Another nutrient, namely taurine, is sulfur-containing amino acid, possessing a sulphonic-acid group and is manufactured from either monoethanolamine or ethylene oxide. It is a beneficial dietary supplement commonly added to nutritional supplements, infant formula, energy drinks and pet foods (180). Taurine is present in higher amounts in human milk than in bovine milk; therefore, the IFs are usually fortified with it, with levels not exceeding 12 mg/100 kcal (61, 121). It is believed to play a role in intestinal fat absorption, hepatic function and the development of the long-term nervous system. Long-term administration of formula milk lacking in parenteral nutrition or taurine has been linked to hepatic cholestasis, retinal degeneration, lower fat absorption, decreased bile production and delayed auditory maturation (181).
3.5.9. Ganglioside
Gangliosides are commonly found in the cell’s lipid membrane and are sialylated glycosphingolipids. They play a role in gut integrity, neurological development, immune cell signaling, preventing infections and intracellular trafficking (182, 183). The supplementation of disialoganglioside has shown a favorable effect on the infant’s neurological development. Infants with an age of 2 to 8 weeks, when supplemented with 2–3 mg ganglioside per 100 g of IF, showed increased General IQ, Performance IQ, and Hand & Eye Coordination IQ on Griffiths scales (184). Moreover, very few studies have been directed until now to understand this bioactive compound’s tolerability, efficacy and safety (101).
3.5.10. Inositol
Inositol is a carbocyclic sugar alcohol and is reported to play a role in many biological functions like phospholipid production, cell osmolarity regulation and cell signaling (185). It is naturally made in humans from glucose and is only half as sweet as sucrose (186). Human milk contains more inositol than bovine and caprine milk (187). Following EU legislation, the IF must contain a minimum of 4 mg per 100 kcal of inositol (61). Inositol is helpful in treating preterm infants who have infant respiratory distress syndrome or are at risk for it (188). Myo-inositol also prevents neural tube abnormalities, especially when paired with folic acid (189).
4. Manufacturing of infant formula
Manufacturing of IF involves multifarious processing techniques resulting in different forms of the formulas. The most commonly available form in the market is the powder one. The liquid formula has certain drawbacks like lower shelf life, demands extra packaging care to avoid contamination and has elevated prices (212). For the production of powdered IF, diverse technologies are employed. Generally, the powder IF is manufactured by dry blending and wet blending (213, 214). On an industrial scale, the combination of both dry and wet blending is implemented (33). Liquid infant formula is also manufactured by time temperature processing, majorly by ultra-high Temperature for extending shelf life (215). The common processing steps involved in the manufacturing of infant formula are schematically presented in Figure 2.
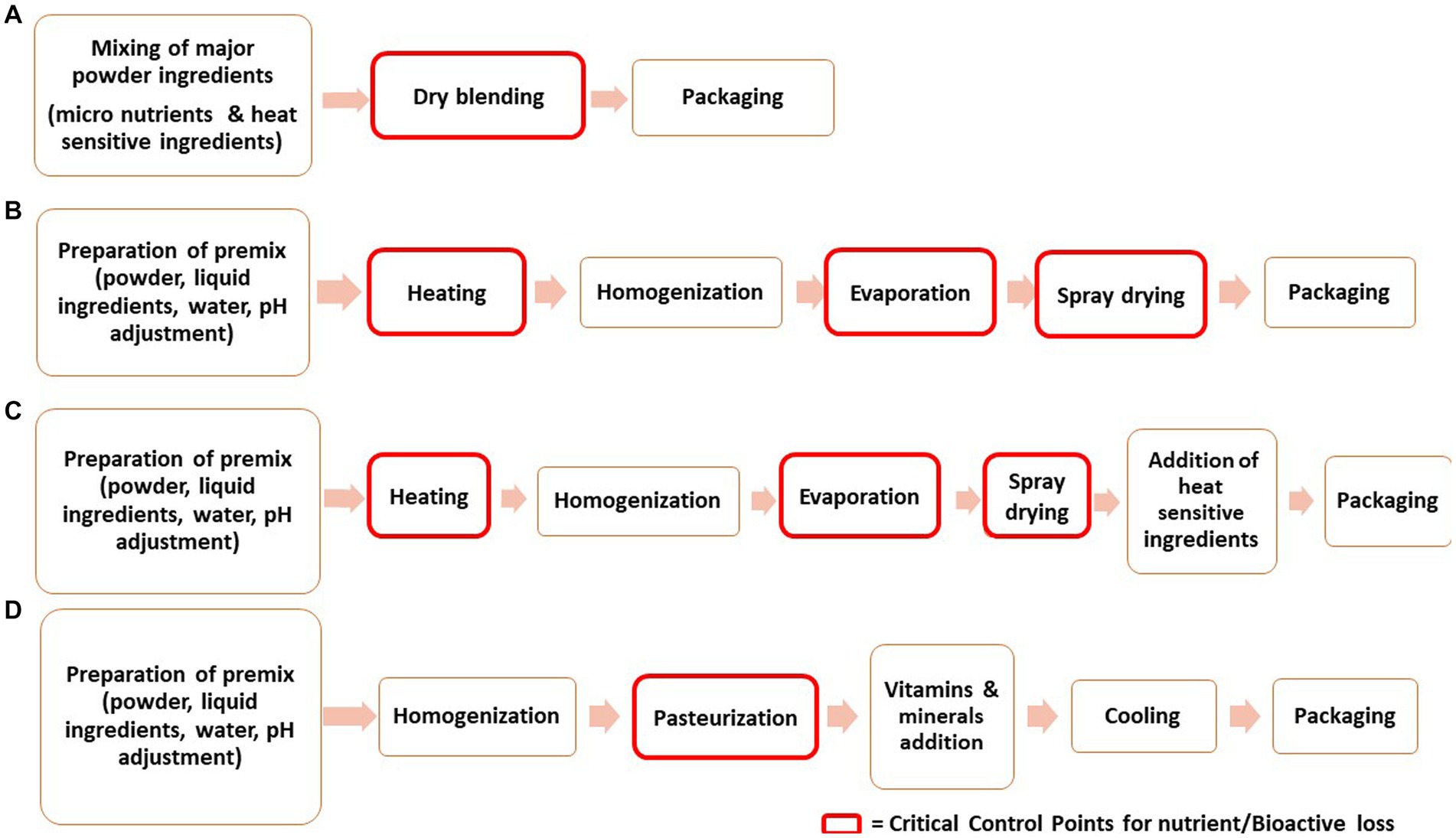
Figure 2. Schematic diagram presenting the processing steps involved in manufacturing of Infant Formula (A) Dry-blending, (B) Wet-blending, (C) Combination of dry and wet blending (D) Liquid infant formula.
4.1. Thermal processing
Traditional thermal methods have been an important tool for the processing by application of heat to acquire powder products or potential pasteurized and sterilized effects. It is unambiguous that by this treatment, there is an efficient reduction of microorganisms resulting in a shelf-stable product (216). Moreover, it is also important to keep the proper balance between heat treatment and control of chemical changes (217). These are based on heat generation outside the product and later heat transfer by either convection or conduction mechanism. The heat application could be by direct or indirect contact with the matrix. On top of that, in thermal processing, an absolute knowledge of thermophysical and thermal properties is vital to determine product behavior. These characteristics are heat diffusivity, penetration coefficient, thermal conductivity, chemical composition, dielectric constant, and modulating product structure. Moreover, these treatments are cost-effective, and numerous studies have been conducted so far to optimize a particular temperature and time combination treatment (218).
4.1.1. Dry blending
In “dry blending,” all the dry ingredients are first mixed well and then packed (219). A large-scale blender or Ribbon blender is employed for the uniform blending of large batches. The blend is then passed through a sifter to remove extraneous matter or oversized particles. The blended material passes to the packaging line and is filled into their respective packaging material by a filler hopper (217). The empty flexible pouches are flushed with nitrogen to prevent oxidation (220). This method has some advantages, like lower cost of equipment and maintenance. Further, there is less energy expenditure during production (221). Besides these benefits, microbial contamination is possible as it depends on raw material quality for assembling the final product. Moreover, considerable problems are related to post-processing contamination with Enterobacteriaceae and Salmonella (222, 223). As the densities of ingredients used vary, they tend to segregate during storage and transportation, leading to a nonhomogeneous state which is not preferred by the consumer. Secondly, the solubility and wettability of dry-blended IFs are not as good as wet-blended IF (217).
4.1.2. Wet blending
To minimize the microbial risk associated with dry mixing, the wet blending method offers better process control and monitoring in manufacturing steps (213, 214, 224). Due to better control, wet blending produces powdered IF with better microbial and physicochemical properties (225). In wet mixing, the dried ingredients are dissolved in skimmed milk or preheated water. Typically, a low Total Solid level (20–30%) wet-mix is obtained to prevent problems related to high viscosities (224). Subsequently, the mix is pasteurized and then homogenized. The pasteurization of the mix is carried out at 75°C to 100°C for 18 s (226). The effect of heating after and before homogenization was evaluated by Buggy et al. (227) and the results depicted that the particle size of aggregates/droplets was smaller in the case of heating application before the homogenization step. Thus, pasteurization is carried out before homogenization. The homogenization process is generally implemented to reduce fat particle size, increase surface area and produce stable emulsions (64). But it also includes stabilizing the protein layer (228). The wet mixes are subjected to two-stage homogenization, applying 13.8 MPa and 3.5 MPa pressure in the first and seconds stage, respectively (64, 84, 213).
Afterward, this preparation is concentrated in a vacuum evaporator to achieve 45–55% total solids and then spray dried (223, 224). The main aim of the evaporation step is to reduce the spray drying cost (224). Additionally, the physicochemical properties of IF are also better. In the dairy and food industries, falling film evaporators are frequently used for evaporation. IF wet-mix is typically concentrated at 50°C and 70°C (64, 213). The spray drying is characterized by the atomization of concentrated premix into tiny droplets of size 10–400 μm. The water molecules of the liquid are evaporated by heated air flow with inlet and outlet temperatures ranging from 180–200°C and 80–100°C (229). As this exposure is very short, so the core temperature does not exceed 45°C. In order to maximize the thermal efficiency and evaporative capacity of the dryer, it is crucial to choose the proper inlet–outlet temperatures (230, 231). Inlet–outlet temperatures also affect water activity, moisture content, glass transition temperature and particle size (229).
In the last phase, the dried powder is recovered in the cyclone separator (232). The recovery system is coupled with adequate packaging steps resulting in minimum microbial contamination. The appropriate packaging method extends the product’s shelf life by preventing oxidation, deterioration and particle agglomeration (212). The resulting IF is sensitive to browning, lipid oxidation and caking induced due to moisture. Thus, commonly used packaging materials are polyolefin-coated metal cans (233). An and co-workers (234) demonstrated the effect of the modified atmosphere on the quality of IF packed in metal cans. The results showed that a high CO2 environment helped in oxidation prevention and increased the survival of incorporated Bifidobacterium. Although MAP packaging is the most widely used technology for powdered products, it cannot generally attain complete oxygen removal (235). To strengthen MAP, the incorporation of antioxidants in the polymer can be effective for inhibition of oxidation and scavenging of headspace oxygen (236). Jo et al. (237) incorporated ascorbic acid in Al- laminated film pouches with CO2 flushing under MAP conditions and then compared it to plain film. This resulted in further lipid oxidation suppression and extended the storage period of powdered IF. Hence, newer and more advanced packaging technologies are required to extend the shelf life and keeping quality of IF.
4.1.3. Processing of liquid infant formula
For the manufacturing of liquid IF, the initial processing steps involved are similar to wet blending (212). At first, the emulsion is formed by mixing vegetable oil with 90°C preheated water and then lactose, milk, OSs, WPC & fat-soluble vitamins are added. Homogenization is done for the stabilization of the emulsion. After this step, emulsion is pasteurized (72°C for 10 s) or sterilized (12°C for 3 s), followed by rapid cooling at 10°C (215, 238). A careful selection of thermal treatment is necessary to optimize the physiological effects possessed by bioactive peptides, which are released after the digestion of IF (239). All minerals and water-soluble vitamins are added to the obtained emulsion. Eventually, this solution is diluted with water to achieve the desired nutrient concentration of the liquid formula. Later, for even mixing, homogenization is again carried out at 240 bar, followed by a sterilization step at 136°C for 35 s (238). The sterilized formulation is cooled to 25°C and aseptically filled in PET (polyethylene terephthalate) bottles (215).
4.2. Challenges associated with thermal processing for manufacturing of infant formula
Processes like pasteurization, homogenization and spray drying have the most pronounced impact on the properties of infant formula due to the interactions between protein-carbohydrate, protein–protein and protein-lipid. These thermal processes may also be responsible for the interaction among other components and the changes in the physicochemical, structural and reconstitution properties of IF (240). Subjecting the formula to several heat processes while its production promotes the Maillard reaction and aggregation or denaturation of proteins, as they are most sensitive to heat (241). In particular, when aggregation occurs, there is a rise in viscosity, a decrease in emulsion stability and a reduction in the overall performance during processing (242). Most proteins lose their biological activity due to aggregation, which ultimately encourages protein coagulation (243–245). Casein protein is more heat stable when compared to whey (246). Nevertheless, intense heating of casein can lead to its cleavage, causing dephosphorylation. Additionally, there is a loss of the protective function of κ-casein if glycosylated. Further, as β-casein is mainly responsible for the bioavailability of zinc and calcium, its functionality loss may result in many future problems in infants (247).
Whey proteins are more prone to heat treatment, which could change their nutritional and functional properties (248). Milk protein solubility decreases when there is an interaction between whey protein and casein micelles leading to their aggregation or dissociation. This ultimately results in the alteration of functionality and hydrophobicity of reconstituted products (243–245). The most sensitive proteins are Igs, and these are present in lower concentrations (225). Upon heating, the bioavailability of zinc and calcium declines as the α-lactalbumin loses its ability to bind with these minerals (249). Although there is no presence of β-lactoglobulin in human milk, still when cow’s milk-derived IF is exposed to heat, it can become insoluble or can get denatured (250). To prevent the aggregation of β-lactoglobulin during thermal processing, the increment of α-lactoglobulin concentration can be an alternative (227). Protein denaturation induced by heat treatment can modulate protein allergenicity. This can occur due to exposure or masking of epitomes, depending on heat treatment intensities (248).
The Maillard reaction can take place when IF is heat treated as it contains proteins and sugar. It is a chemical reaction between the reducing sugar’s carbonyl group and free amino acids, forming Schiff’s base, i.e., lactosyl-lysine (231). Lysine is the most reactive amino acid, but sugars can also react with histidine, arginine, tryptophan and methionine (251, 252). The Schiff’s bases formed are chemically unstable and are susceptible to additional isomerization, known as Amadori rearrangement, which results in the creation of lactulose-lysine (the Amadori product). Consequently, the bioavailability of lysine can be affected, causing nutritive loss (81, 251, 253). Prolonged heating in acidic or neutral conditions, there is a formation of various furfural compounds like 5-methyl-2-furaldehyde, 5-hydroxymethylfurfural, 2-furyl-methyl ketone and 2-furaldehyde (254, 255). Nε-carboxymethyl-lysine is an advanced glycation product that can cause potential pro-oxidant and pro-inflammatory health effects (256). Aldehydes, reductones, furfurals and some other intermediate products tend to react with amines and form dark polymeric compounds of higher molecular mass called melanoidins (257). Melanoidins have the ability to chelate metals, which can be harmful if they influence nutritionally essential metals like Zn, Ca, Cu, Mg, and Fe and thus impair mineral absorption and metabolism (258, 259). Over time, there has been a revolution in newer techniques for studying the generation of all these undesirable compounds formed during thermal processing. For example, the advanced glycation end-products (AGE) were predicted by the Molecular Transformer model curated with data from the literature (260).
MFGM has recently been permitted to be incorporated into the IF. Heat treatment can also affect it, leading to its enhanced permeability and hence reducing its stability (261). A combination of thermal and homogenization treatments causes fat structure differences in terms of size, interfacial architecture, composition and fatty acid profile (262, 263). Also, there is phospholipid breakdown in heat treatment with a consequent increment in inorganic phosphate (264). Heat processing results in the loss of vitamins and minerals and the isomerization of lactose to lactulose (143, 265, 266). Heat treatment intensities determine the extent of alterations in the mineral balance between serum and colloidal phases (246). Compared to macro-elements, significantly less information is available on heat treatment effects in trace mineral concentration and distribution. Nonetheless, trace minerals can also be lost during heat treatment or in other unit processing involving heat, such as in fouling deposits during drying and evaporation (267, 268). Moreover, due to heat, there is partial degradation of water-soluble vitamins like C, B1, B6, B12, some hormones and unsaturated fatty acids. Both drying and evaporation operations can be detrimental to oxygen-sensitive components, including most fat- and water-soluble vitamins and unsaturated fatty acids (264).
4.3. Non-thermal processing of infant formula
With the aim of combating organoleptic and nutritional changes occurring during heat processing, various nonthermal technologies have been introduced in the manufacturing of infant formula. These novel techniques include HPP, Ultrasound, Ionizing radiations, Pulsed electric field (PEF), Ultraviolet irradiations and Cold plasma (269). Over the past few years, numerous researchers have been actively working on non-thermal technologies to study their impact on pathogenic microbes and nutritional profiles. Yet, the aforementioned technologies have a limited impact, higher equipment & processing cost, and stringent operating requirements (270). Table 3 summarizes the application of non-thermal technologies for processing Infant Formula and inactivation of microbiological contamination, while retaining nutritional and other quality attributes.
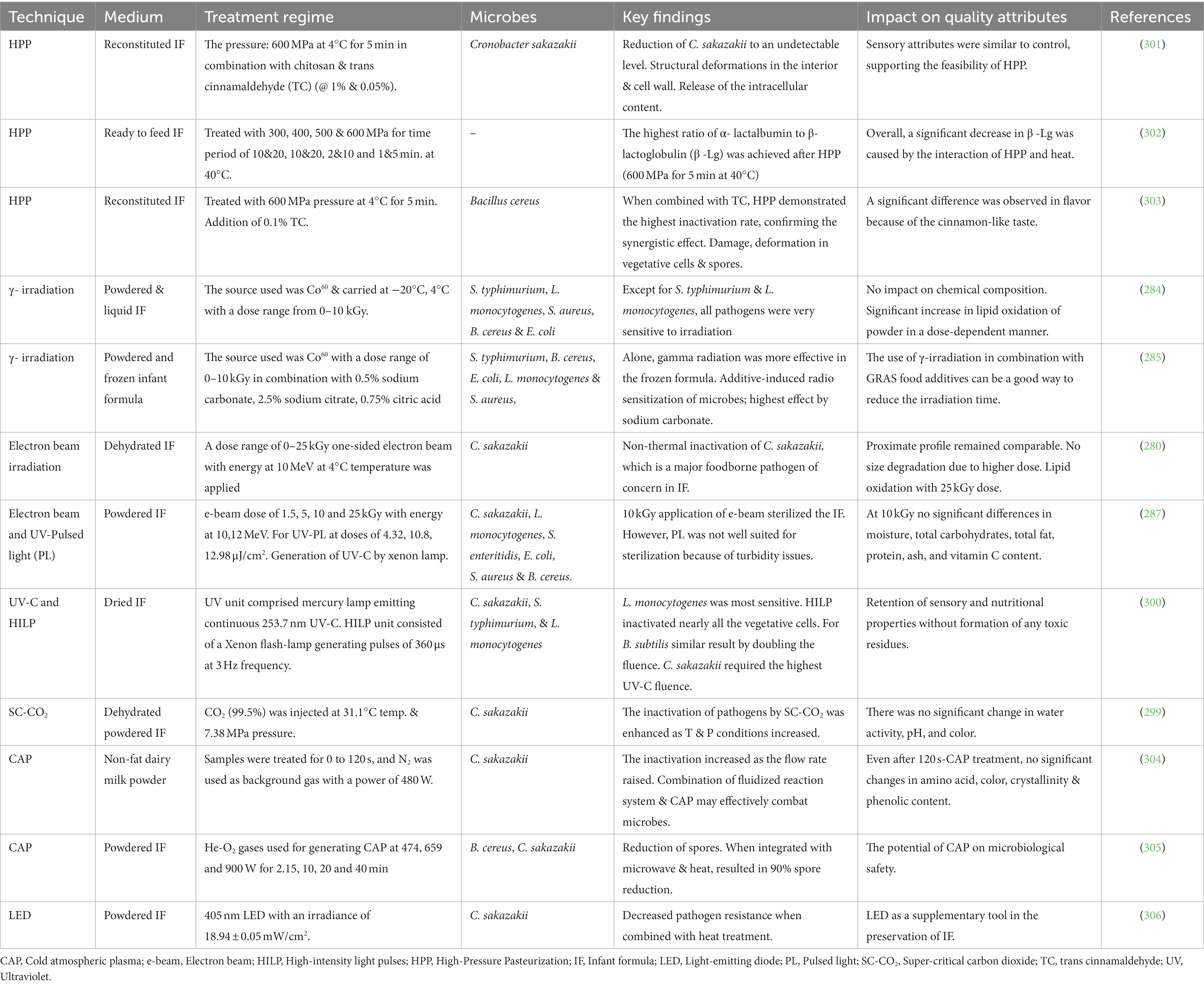
Table 3. Application of non-thermal technologies for processing of infant formula and its effects on pathogenic microbes and quality attributes.
The High-Pressure Processing technology finds its potential application in wet mixtures, which are later subjected to spray drying or in the production of non-thermally pasteurized liquid IF (271). Due to economic and technical constraints, the pressure limit is about 600 MPa (272). It can also be combined with other non-thermal technologies to enhance the effect of microbial inactivation (273). As previously said, pasteurization is characterized as a heat-based intervention, and HPP is now included in the definition of pasteurization as a non-thermal pasteurization technology (274). Apart from HPP, PEF is another non-thermal technology applied for IF processing and is generally employed on non-liquid food items (275). PEF effectively eliminates enzymes linked to quality degradation, pathogenic bacteria, and spoilage-causing microbes without diminishing consumer demand (276). The inactivation induced is due to the dielectric breakdown of the cell membrane and electroporation. Several factors affecting PEF are pulse intensity, number of pulses, flow rate, shape and pulse width. Besides these physiological parameters of microbes and conductivity, the temperature can also affect the microbial inactivation rate (277). PEF implies the application of strong electric fields in short pulses in intestines ranging from 10–80 KV/cm for microseconds (278).
The ionizing radiation methods, such as gamma and beta radiation, effectively inactivate foodborne bacterial pathogens while maintaining the macronutrient composition. But, the preservation of the nutritional matrix depends upon the food composition, environmental conditions and irradiation dosage (279, 280). Both the γ and β radiations comprise the kinetic energy of photons and electrons. When it comes in contact with the target, excitation and ionization occur in the form of a physiochemical effect (281). These technologies affect living cells through the induction of physiological, genomic, morphological and biochemical changes (282). One of the significant effects is damage in macromolecules via direct energy dissipation leading to the breakage of double and single bonds, ultimately causing cell apoptosis. On the other hand, the indirect effect involves the formation of free radicals and highly reactive oxygen species, causing damage to cellular material and nucleic acids (283). Gamma irradiation technology has also been applied to infant formula, whether in liquid or powdered formulation, for the inactivation of these pathogens with a dosage range of 0–10 kGy (284, 285). The most commonly used radioactive source are cobalt-60 and cesium-137. On the industrial scale, Co60 is mainly utilized for radiation generation from Co59, which is a non-radioactive stable metal (286). For Electron Beam, a high voltage power supply is the source of electrons applied to IF with a dose range of 0–25 kGy and 10 MeV energy (280, 287). In order to monitor radiation levels and create regulations on radiation safety, it is crucial to determine the radioactivity levels in IF and the associated doses (288).
Cold Atmospheric Plasma (CAP), or simply the Cold Plasma, among emerging non-thermal technologies, is utilized for sterilization and microbial decontamination. The plasma species are responsible for the oxidation of macromolecules (proteins and lipids) and the destruction of the cell membrane (289). Certain benefits aided by cold plasma consist of lower operating temperatures, minimal destruction of flavor and nutrients and no toxic by-product generation (290). Nevertheless, some studies have shown that CAP impacts the functional and structural properties of various food components (291–294). Gao et al. (295) reported that CP treatment at 70 kV for about 180 s trigger lipids oxidation during storage. Pulsed light also has the potential for rapid inactivation of microbes on food surfaces by application of short pulses rich in UV light (100-1,100 nm). There is no deleterious effect of pulsed light on lipid oxidation and protein composition. Despite microbial inactivation, its drawback is the agglomeration of powder particles due to inter-particle forces developed by moisture absorption. All such parameters must be kept in mind while selecting particular non-thermal processing (287).
Within the infant formula manufacturing sector, bacteriophage application might be seen as a new-generation approach to non-thermal biocontrol. Bacteriophages (phages) are obligatory parasites that lyse live bacterial hosts. The lytic protein produced by the phages can hinder the growth of Cronobacter sakazakii and Staphylococcus aureus (296). Kim et al. (297) delineated that bacteriophages can control the C. sakazakii growth and its biofilm-forming ability when IF milk is treated with two representative bacteriophages (PBES19 & PBES04). The results depicted that bacteriophages can be effectively used to increase food safety in commercial facilities. However, the methods in optimization for enhancing viable phage propagation are not cheap and also require considerable investment when it comes to upscale (65). On top of that, natural antimicrobials such as copper sulphate, lactic acid, nisin, lactoferrin, trans-cinnamaldehyde, caprylic acid, etc., can be considered efficacious in controlling the microbial spoilage of IF (271).
The use of supercritical carbon dioxide has been an alternative pasteurization technology for many food items. The treatment involves contact of a pasteurized item with super or sub-critical CO2 for a defined amount of time in a continuous, batch or semi-batch manner. Supercritical CO2 is the CO2 at pressure and temperature above its critical point and exists as a single-phase, having the ability to solubilize materials and diffuse through the solids. It is majorly used for the pasteurization of liquid items involving ready-to-feed formulas (298, 299). Further, newer non-thermal technologies based on light, such as broad-spectrum pulsed light (200–1,100 nm) and UV-C (254 nm), are some potential hurdles that can be applied as post-production processes in dried IF. Nonetheless, UV-C is traditionally used for the decontamination of drinking water, wastewater, air and solid foods surfaces. On the contrary, High-Intensity Pulsed Light (HIPL) is a modified version of UV-C and is claimed to be an improved method for UV-C delivery and be applied to powdered IF (300). The advantages and disadvantages of several processing technologies used for manufacturing IF are presented in Figure 3.
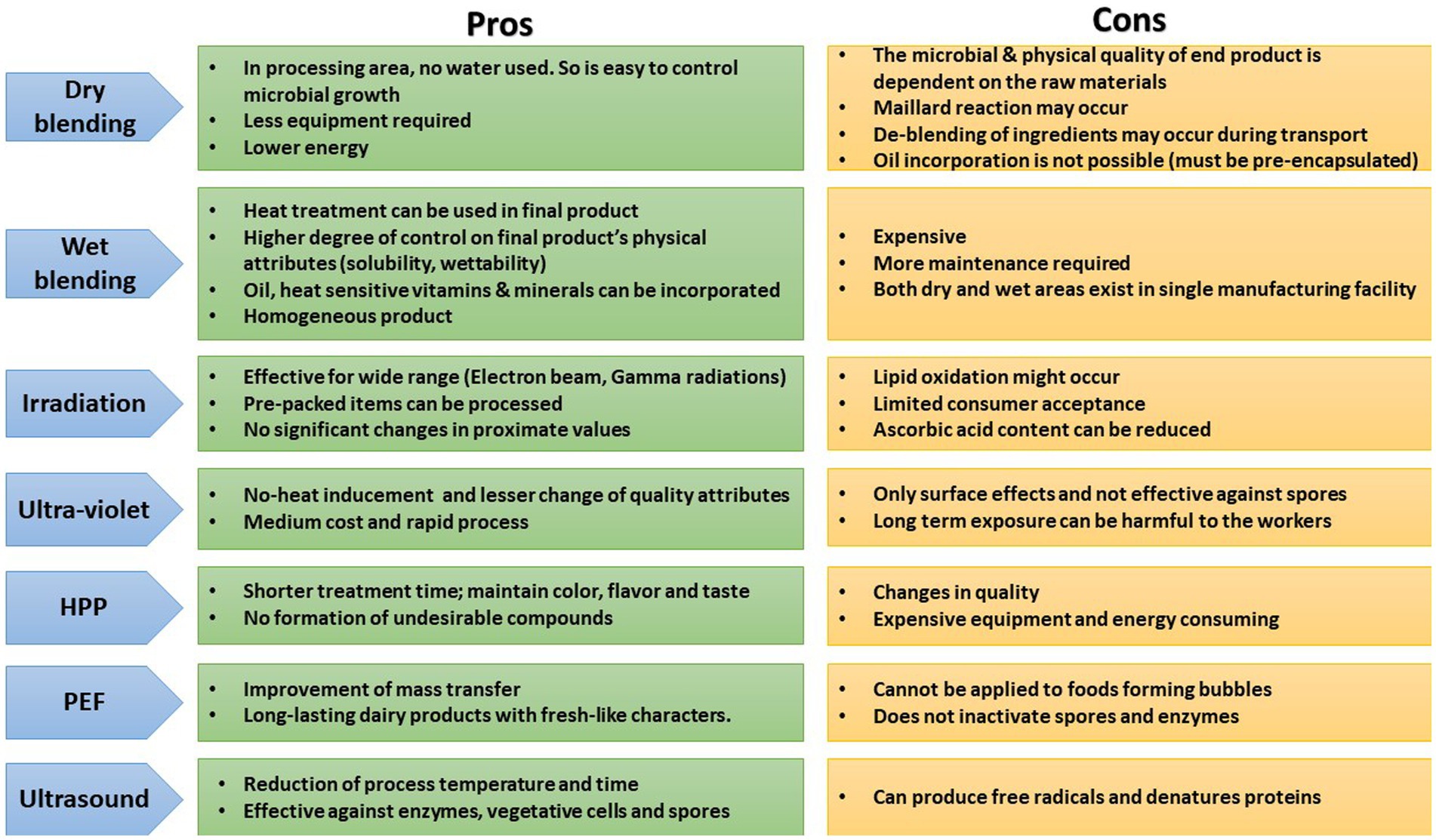
Figure 3. Pros and cons of different processing technologies applied for manufacturing infant formula. HPP, High pressure processing; PEF, Pulsed electric filed.
5. The action of infant formula on infant’s gut microbiota and their possible mechanistic connection
5.1. Establishment of infant’s gut microbiome
Infants’ physiological development includes the growth of their gut flora, which should be considered in clinical practice for manufacturing infant formulas (307). The gut microbiota exhibits several functions like the synthesis of vitamins and de novo synthesis of amino acids, facilitating the utilization of nutrients, metabolic system’s programming and immune system development etc. It also modulates body composition and infant growth. All of these factors have implications for infants’ short- and long-term health (308). Many studies have reported varied qualitative bacterial flora composition among breastfed infants. In addition, an increase of fermentative bacteria and a decrease in the growth of putrefactive flora was also observed (309). This switch aids in the improvement of intestinal function, such as the absorptive and digestive functions of several nutrients, especially vitamins. Ultimately, there is stimulation of the GI-associated immune system, which reduces the risk of allergies in infants (310).
In the early weeks of life, there are majorly obligatory and facultative anaerobes in the infant’s gut. The establishment of obligatory anaerobes is made possible by the initial colonization of the gut by facultative anaerobes. Later, the microbiota of the gut achieves structural complexity (311, 312). Hence, initial microbial colonization is critical in defining the bacterial flora of adults. Once the microbiota of adults is established, it remains constant, with the exception of potential alterations brought on by a variety of causes, such as a change in dietary habits or the progression of diseases (313). Many longitudinal and cross-sectional studies indicate that undernutrition in childhood is associated with immaturity of gut microbiota, altered diversity, depletion in obligate anaerobes, and enrichment in potentially inflammatory and pathogenic species accompanied by low nutrient utilization (314).
Besides, the gut microbiota is also involved in the nutrient-sensing mechanisms of the intestine (315). One of the probable mechanisms underlying the interaction between host metabolism and microbiota is via appetite-regulating hormones (involving ghrelin, leptin, and glucagon-like peptide-1). Gut microbes can produce numerous neuroactive compounds (acetylcholine, dopamine, serotonin & GABA), which can act on both the central nervous and enteric systems. They have the potential to modulate cognitive function and behavior; bind to receptors expressed in the brain (bile acids & indoles). Meanwhile, dietary precursors in the synthesis of host neurotransmitters, like tyrosine (for dopamine) and tryptophan (for serotonin), can be metabolized by microbial enzymes (316). Short Chain Fatty Acids (SCFAs), the end product formed after bacterial fermentation of non-digestible carbohydrates, might be able to alter metabolism and energy harvest through adipogenesis, enteroendocrine cells and production of insulin-like growth factor-1 (317). Host microbial-genomic interaction has recently been found to be an important mechanism associated with copious aspects of human health and diseases. According to metagenomic research, host genes play a role in shaping the microbiome, which in turn, regulates the gene expression of the host (314). The integrative approach by Charton et al. (318) underlined specific bacteria (Veillonellaceae, Prevotellaceae, Enterobacteriaceae, Rikenellaceae and Lachnospiraceae) associated with commutation of the gut-brain axis. A potential pathway depicting the relationship between neonatal feeding, gut microbiota and the metabolism of the infant is presented in Figure 4.
In addition, it is also necessary to investigate the functionality of gut microbiota belonging to various taxonomic groups and clearer picture of microbiome functionality can be derived from metatranscriptomics (319). A minor overall functional difference, formula-fed infants showed a faster functional maturity when compared to breastfed infants at four months (320, 321). Further, a meta-analysis study by Ho et al. (322) inferred that infants who were not exclusively breastfed had lower levels of vitamin metabolism, lipid metabolism and detoxification. However, microbiological processes involved in carbohydrate metabolism were more prevalent. At the same time, the oxidative phosphorylation and synthesis of vitamin B are also the characteristics of exclusive breastfeeding (320). At the age of 3 & 6 months, formula-fed infants also have higher levels of SCFAs in their stools, including free amino acids, butyrate, propionate, acetate and 5-amino valerate. Additionally, the breastfed infants had higher levels of lactic acid and FLs in their stools due to higher HMOSs fermentation (323). Therefore, microbiome composition is modulated by the type of feed. The impact of feeding on preterm and term gut microbiota may be potentially beneficial to metabolism, intestinal function and the immune system, along with persisting subject for life (324).
5.2. Nutrient profile and processing impact on gut microbiome
Compared to breastfeeding, formula feeding has been linked to a less stable microbiome across time, a remarkably different bacterial composition, and increased bacterial diversity and richness (325–327). In an investigation that operated on 91 infants, the composition of fecal bacteria was more diverse in formula-fed infants. Also, this diversification varies from one formula to another (326). Moreover, the alteration of the microbial profile in the first months of life may lead to overweight development in the infant (328, 329). During the first 18 months, Selma-Royo et al. (330) outlined a lower concentration of Bifidobacterium and Bacteroidetes genus in infants with higher Weight/Length and Body Mass Index scores. In another study, infants who were not exclusively breastfed had more Bacteroides count (331). The exact role played by specific bacterial genera or families in weight management is still unclear (309, 332). Overall, these data imply that prior colonization of the gut microbiota is crucial, and it may influence neonatal growth trajectories (333). Moreover, the disruption in gut microbiota (i.e., dysbiosis) has been related to necrotizing enterocolitis and several other chronic illnesses, including inflammatory bowel disease, allergies, obesity, asthma, diabetes, cancer and neurological conditions associated with the gut-brain axis (334–336).
Besides, IF-fed infants have a faster development curve than age-matched breastfed newborns, which is generally associated with advanced adiposity, greater weight gain, and an elevated risk of childhood obesity (337). Piglets raised in containment facilities on bovine-based IF exhibit differences in the mucosal immune system’s three compartments, including more rapid recruitment of antigen presenting cells, lesser regulatory T-cells and increased B-cells, as well as distinctive microbiomes (338, 339). The introduction of IF before the third month of life is associated with a higher risk of rapid growth at six months and increased body mass index in their adulthood. Therefore, the time of formula administration is also a factor in determining health in neonatal and adulthood (340). The consequence of fast gut maturation due to IF feeding is a greater abundance of different Clostridium genera, particularly C. difficile, with subsequent development of atopy (341). However, only a few research has sought to shed light on the dynamics of the metabolic and functional processes related to the gut microbiota evolution and maturation with impact on long term health, even though many papers have characterized community membership in the gut microbiota (Figure 4).
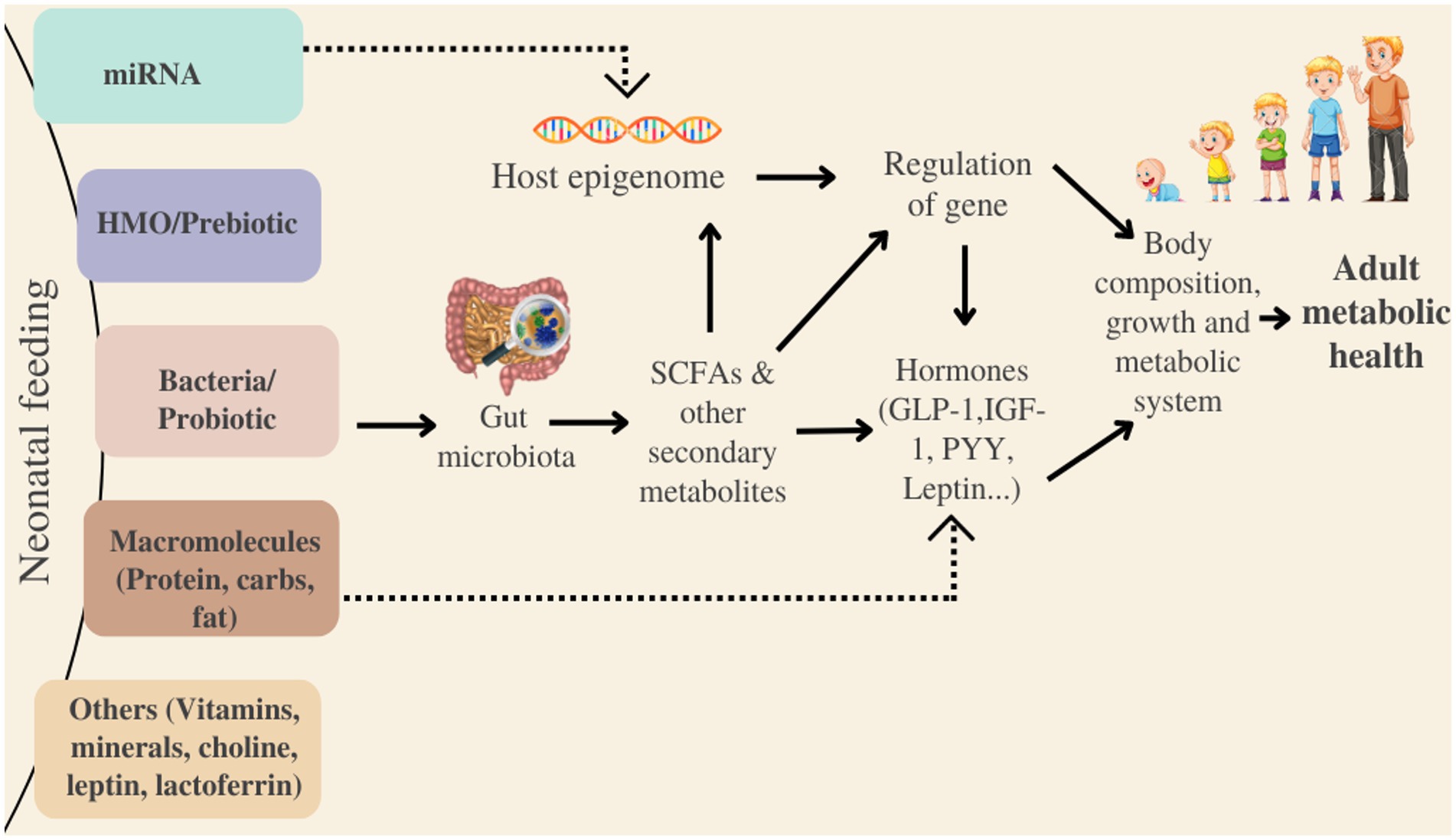
Figure 4. Potential pathway entailing gut microbiota and its link to long-metabolic health. HMO, Human milk oligosaccharides; SCFAs, Short-chain fatty acids; PYY, Peptide YY; IGF-1, Insulin-like factor-1; miRNA, non-coding microRNA; GLP-1, Glucagon-like peptide-1.
The macronutrient profile of IFs has the ability to influence the gut microbiome (308). Compared to a casein-predominant IF, a whey-predominant IF resulted in a fecal microbiota that was more similar to that of 2-month-old breastfed infants (342). Nowak-Wegrzyn et al. (86) assessed the safety and clinical hypoallergenicity of a whey-based extensively hydrolyzed formula containing lacto-N-neotetraose and 2′fucosyl-lactose. They suggested that this formula could be recommended for the management of cow’s milk protein allergy in young children and infants. The findings of Rosa et al. (343) indicated that the neonatal diet had an impact on the microbial proteins and bacterial taxa of the gut before weaning. Proteins like lactoferrin may also serve as prebiotics and affect the composition of gut microbiota. Manzoni (344) documented the in vivo effect of lactoferrin on the diversity and growth of intestinal microbiota. The advantage of lactoferrin via the microbiota might involve several processes, including intestinal cell differentiation and maturation, energy supply for growth of the intestine and gut-brain-microbiota axis signaling for the development of nerve fibers (345–347). Gomez-Gallego et al. (348) investigated weaned pups (n = 12) fed with low-concentration polyamines-enriched IF (putrescine, spermidine & spermine). The microbiota changes were higher on supplementation, followed by increased levels of Akkermansia-like bacteria, Bifidobacterium group and Lactobacillus–Enterococcus group bacteria.
The triglyceride structure may potentially have an impact on bacteria in the gut. Compared to infants receiving a low-palmitate IF, high-palmitate IF increased Bifidobacterium and Lactobacillus count in 6-week-old infants’ faces at quantities similar to that of breastfed children (349). In accordance with a study carried out on germ-free female and male pups (n = 36), the establishment of the gut microbiota may be modulated by the differences in phospholipids types and the fatty acids profile. The results suggest that the emulsifiers and fat type used in IF may affect gut flora setup differently in formula-fed infants (350). 1,3-olein-2-palmitin is a triglyceride used in the supplementation of IF, demonstrated a slight increase of SCFA content and an abundance of gut bifidobacteria (351). In 28-day-old piglets, the addition of MFGM alone did not affect the gut microbiota composition compared to standard IFs. When compared to piglets fed on a regular vegetable-oil IF, there was a rise in Proteobacteria and fall in Firmicutes phyla showing the impact of MFGM and dairy lipids. This incorporation of MFGM and milk fat in IF modifies fecal microbiota composition, the process of gut immunity development and protein digestion (352).
Interventions containing exogenous microbes have recently gained attention in the modulation of gut microbiota (353, 354). Lemaire et al. (355) investigated the long-term effects of Lactobacillus fermentum and dairy lipids on gut microbiota, host entero-insular axis & metabolism. The intervention specifically lowered the fecal content of lysine and 2-oxoglutarate. It also increased SCFA content and had a beneficial impact on endocrine function. In line with this, Bifidobacterium strains work as ecosystem engineers in extremely preterm infants, speeding up the development of their microbiomes and having an impact on their immune systems (356). Since Limosilactobacillus reuteri has been found both in human gut and breast milk, Alliet et al. (357) evaluated the efficacy and safety of IF supplemented with 2′-fucosyllactose & L. reuteri. Results suggest that doing so supports age-appropriate growth, is well-tolerated and may play a role in shifting the gut microbial pattern toward that of breastfed infants. Meanwhile, by generating SCFAs, probiotic metabolites can support an anti-inflammatory effect (358, 359). For example, butyrate reduces the expression of the pro-inflammatory cytokines and enhances the expression of the anti-inflammatory cytokines suggesting that it is a key negative regulator of inflammation. Acetate is another abundantly produced SCFA that exerts an anti-inflammatory effect on receptors expressed in peripheral blood cells and adipose tissue (360).
Again, various types of prebiotic substrates might perhaps act diversely on intestinal bacteria (316). In the colon, prebiotics reach intact and serve as substrates for bacterial growth. Therefore, the Bifidobacterium count and metabolic activity of gut microbiota of infants supplemented with the prebiotics is comparable to that of breastfed infants (361). Furthermore, the infants fed with 2′-FL & LNnT-supplemented IF had fecal microbiota similar to breastfed infants in terms of overall genus composition, microbial diversity and abundance of major genera (153, 362, 363). Infants fed on IF supplemented with 2′-FL expressed lower levels of plasma inflammatory cytokine profile (IL1- α, IL1-β, TNFα & IL 6), which resembles that of breastfed infant group (364). Following this, the incorporation of a prebiotic mix in IF resulted in an elevated concentration of stool secretory IgA and reduced infection rates. Therefore, prebiotics are also indicated to have an influence on intestinal immune development (361). Lagkouvardos et al. (365) demonstrated that synbiotic intervention containing GOS and Limosilactobacillus fermentum influenced milieu parameters and fecal microbiota at an early age, sharing fewer similarities to breastfed infants. The use of a probiotic-supplemented IF, notably with S. thermophilus and B. lactis, has displayed a lower incidence of antibiotic-associated diarrhea. Due to the heterogeneity and limited numbers of studies, it is challenging to draw more robust conclusions about the exact intervention of both pro- and prebiotics for supplementation in IF to modulate the gut microbiome.
Likewise, some food additives may also disrupt gut homeostasis and contribute to inflammatory responses related to tissue damage (366). Several studies in animal models, as reviewed by Rinninella et al. (367), reported that higher salt doses are associated with changes in bacterial group abundance. These results depicted decreased group abundance in Oscillibacter, Lactobacillus spp., Pseudoflavonifractor, Rothia, Clostridium and Johnsonella; and increased group abundance in Ruminococcus, Parasutterella spp., Lachnospiraceae, Erwinia genus, Corynebacteriaceae and Christensenellaceae. Furthermore, work on the effect of vitamin D supplementation in infant gut microbiota (age 3–6 months) demonstrated that, Vit. D was associated with decreased Lactococcus and increased Lachnobacterium. These studies on Vit. D also suggested the possibilities of correlations in the incidence and prevalence of allergies/asthma with long-term immune system implications (368). The B vitamins are biosynthesized by the cooperation of several phyla of gut bacteria, including Bacteroidetes, Proteobacteria and Fusobacteria (369). Emulsifiers as an additive lower gut microbial diversity, i.e., increasing Verrumicrobia and decreasing Bacteroides abundance. These alterations in gut microbiota led to dysbiosis and promoted colitis, chronic gut inflammation and metabolic syndrome (370). Therefore, all such considerations must be considered while designing infant formula mimicking human milk.
Processing causes significant alteration in the composition and structure of different components present in food. Because of heat treatment, the Maillard reaction occurs, resulting in the formation of melanoidins which possess prebiotic role and, in turn, affect gut microbiota composition. For example, melanoidin-enriched malts were fed to mice (n = 75) and relative reduction in pathogenic bacterial groups, namely Dorea, Alistipe, and Oscillibacter; and an increase in beneficial Lactobacillus, Akkermansia, Parasutterella, Barnesiella, Bifidobacterium were observed (371). In addition, prior to spray drying, heat treatment of milk was shown to improve the residual casein immunoreactivity significantly (372). Ye et al. (239) demonstrated that storage and UHT treatment of ready-to-feed liquid IF affects in vitro digestibility and release of bioactive peptides, principally due to the induction of protein aggregation and structural changes. These peptides are more readily absorbed, improving the digestibility. The intense cooking technologies sometimes increase the abundance of beneficial microbes like Bifidobacterium spp. and Ruminococcus spp. (373). Reviewing different studies, we have concluded that there is still lack of more vigorous research about processing of IF and their impact on gut microbiota.
The formation of the gut microbiota is important, but since it is so complex, it is still not entirely understood how various pathways affect the biology of the infant (308). In order to investigate the early nutritional effect in the long term and to control some confounding factors discovered in human studies, preclinical models like animals such as neonatal piglets and nonhuman primates are found to be excellent for such studies (374–376). Alongside, targeting microbiome reprogramming for the treatment and prevention of metabolic illnesses in the future will be made possible by a comprehensive knowledge of relationships between gut microbiome establishment, metabolism and human health (377). Correlations between particular bacteria and certain macronutrients have been documented; however, more research is required to fully comprehend these associations and assess their long-term health effects (378).
6. Conclusion
Infant Formula consumption is increasing enormously in this modern era, creating a striving need to develop formulas that fulfil the growth and developmental requirements of infants who cannot breastfeed. Researchers and scientists are working on adding different components and applying the latest technologies to formulate products like human milk in many possible ways. The paramount interest is shifting toward adding functional bioactive compounds that can promote health outcomes other than growth, namely cognition, immunity, and temperament. There are numerous technologies involved when it comes to infant formula processing. Most production processes involve heat for microbial inactivation and powder production, causing heat-induced deteriorative changes in IF. Many non-thermal technologies have been utilized at different stages of IF manufacturing to overcome the drawbacks of nutritive loss from heat treatments. In terms of IF action in the gut, the development of an infant’s gut microbiota plays a pivotal role, but because of its complexity, underlying pathways having an impact on an infant’s gut biology remain unknown. Additionally, scientists have reported the beneficial effect of milk derived constituents on gut microbiota development which is related to the development and maintenance of metabolic health and immunity. Yet due to the lack of an exact relationship between formula feeding, gut microbiota establishment and its long-term health implications, greater in-depth and extensive research in these fields are of utmost importance. Likewise, a sound understanding of the physiological properties of IF during storage, handling and transportation must be evaluated. On top of that, ensuring sustainability and reducing production costs are also crucial for Infant formula success.
Author contributions
SB, VP, and DB: conceptualization. SB and BB: methodology. SB, VP, and SK: data curation. SB, VP, SY, ZA-Z, and HR: writing and original draft preparation. DB, BB, SK, PK, and VK: review and editing of the manuscript. SB, PK, VK, and ZA-Z: visualization and illustrations. VP, DB, and SY: supervision. All authors have read and agreed to the published version of the manuscript.
Funding
VP acknowledges the IoE Scheme research grant from Banaras Hindu University, Varanasi, India.
Conflict of interest
The authors declare that the research was conducted in the absence of any commercial or financial relationships that could be construed as a potential conflict of interest.
Publisher’s note
All claims expressed in this article are solely those of the authors and do not necessarily represent those of their affiliated organizations, or those of the publisher, the editors and the reviewers. Any product that may be evaluated in this article, or claim that may be made by its manufacturer, is not guaranteed or endorsed by the publisher.
Supplementary material
The Supplementary material for this article can be found online at: https://www.frontiersin.org/articles/10.3389/fnut.2023.1194679/full#supplementary-material
References
1. Pope, DH, Karlsson, JO, Baker, P, and McCoy, D. Examining the environmental impacts of the dairy and baby food industries: are first-food systems a crucial missing part of the healthy and sustainable food systems agenda now underway? Int J Environ Res Public Health. (2021) 18:12678. doi: 10.3390/ijerph182312678
2. Kamangar, F, Nasrollahzadeh, D, Safiri, S, Sepanlou, SG, Fitzmaurice, C, Ikuta, KS, et al. The global, regional, and national burden of oesophageal cancer and its attributable risk factors in 195 countries and territories, 1990–2017: a systematic analysis for the global burden of disease study 2017. Lancet Gastroenterol Hepatol. (2020) 5:582–97. doi: 10.1016/S2468-1253(20)30007-8
3. Swinburn, BA, Kraak, VI, Allender, S, Atkins, VJ, Baker, PI, Bogard, JR, et al. The global syndemic of obesity, undernutrition, and climate change: the lancet commission report. Lancet. (2019) 393:791–846. doi: 10.1016/S0140-6736(18)32822-8
4. Sotiraki, M, Malliou, A, Tachirai, N, Kellari, N, Grammatikopoulou, MG, Sergentanis, TN, et al. Burden of childhood malnutrition: a roadmap of global and European policies promoting healthy nutrition for infants and young children. Children. (2022) 9:1179. doi: 10.3390/children9081179
5. Emadi, MH, and Rahmanian, M. Commentary on challenges to taking a food systems approach within the food and agriculture organization (FAO). Food Security and Land Use Change under Conditions of Climatic Variability: A Multidimensional Perspective. (2020):19–31. doi: 10.1007/978-3-030-36762-6_2
6. Almeida, CC, Mendonça Pereira, BF, Leandro, KC, Costa, MP, Spisso, BF, and Conte-Junior, CA. Bioactive compounds in infant formula and their effects on infant nutrition and health: a systematic literature review. Int J Food Sci. (2021) 2021:1–31. doi: 10.1155/2021/8850080
7. Baker, P, Russ, K, Kang, M, Santos, TM, Neves, PAR, Smith, J, et al. Globalization, first-foods systems transformations and corporate power: a synthesis of literature and data on the market and political practices of the transnational baby food industry. Glob Health. (2021) 17:58. doi: 10.1186/s12992-021-00708-1
8. Smith, JP. Markets, breastfeeding and trade in mothers’ milk. Int Breastfeed J. (2015) 10:1–7. doi: 10.1186/s13006-015-0034-9
9. UvnäsMoberg, K, Ekström-Bergström, A, Buckley, S, Massarotti, C, Pajalic, Z, Luegmair, K, et al. Maternal plasma levels of oxytocin during breastfeeding—a systematic review. PLoS One. (2020) 15:e0235806. doi: 10.1371/journal.pone.0235806
10. Rollins, NC, Bhandari, N, Hajeebhoy, N, Horton, S, Lutter, CK, Martines, JC, et al. Breastfeeding 2: why invest, and what it will take to improve breastfeeding practices. Lancet. (2016) 387:491–504. doi: 10.1016/S0140-6736(15)01044-2
11. Walters, DD, Phan, LTH, and Mathisen, R. The cost of not breastfeeding: global results from a new tool. Health Policy Plan. (2019) 34:407–17. doi: 10.1093/heapol/czz050
12. Williams, J, Namazova-Baranova, L, Weber, M, Vural, M, Mestrovic, J, Carrasco-Sanz, A, et al. The importance of continuing breastfeeding during coronavirus disease-2019: in support of the World Health Organization statement on breastfeeding during the pandemic. J Pediatr. (2020) 223:234–6. doi: 10.1016/j.jpeds.2020.05.009
13. Bever Babendure, J, Reifsnider, E, Mendias, E, Moramarco, MW, and Davila, YR. Reduced breastfeeding rates among obese mothers: a review of contributing factors, clinical considerations and future directions. Int Breastfeed J. (2015) 10:1–11. doi: 10.1186/s13006-015-0046-5
14. World Health Organization (ed.). Scope and impact of digital marketing strategies for promoting breastmilk substitutes. WHO TEAM Maternal, Newborn, Child and Adolescent Health and Ageing, Nutrition and Food Safety. (2022) 36.
15. Pérez-Escamilla, R, Tomori, C, Hernández-Cordero, S, Baker, P, Barros, AJD, Bégin, F, et al. Breastfeeding: crucially important, but increasingly challenged in a market-driven world. Lancet. (2023) 401:472–85. doi: 10.1016/S0140-6736(22)01932-8
16. WHO. (2017). Guidance on ending the inappropriate promotion of foods for infants and young children: Implementation manual. Available at: https://www.who.int/publications/i/item/9789241513470 (Accessed February 14, 2023)
17. Baker, P, Santos, T, Neves, PA, Machado, P, Smith, J, Piwoz, E, et al. First-food systems transformations and the ultra-processing of infant and young child diets: the determinants, dynamics and consequences of the global rise in commercial milk formula consumption. Matern Child Nutr. (2021) 17:e13097. doi: 10.1111/mcn.13097
18. Khandpur, N, Neri, DA, Monteiro, C, Mazur, A, Frelut, M-L, Boyland, E, et al. Ultra-processed food consumption among the paediatric population: an overview and call to action from the European childhood obesity group. Ann Nutr Metab. (2020) 76:109–13. doi: 10.1159/000507840
19. McCann, JR, Russell, GC, Campbell, KJ, and Woods, JL. Nutrition and packaging characteristics of toddler foods and milks in Australia. Public Health Nutr. (2021) 24:1153–65. doi: 10.1017/S1368980020004590
20. Pries, AM, Mulder, A, Badham, J, Sweet, L, Yuen, K, and Zehner, E. Sugar content and nutrient content claims of growing-up milks in Indonesia. Matern Child Nutr. (2021) 17:e13186. doi: 10.1111/mcn.13186
21. Martin, CR, Ling, P-R, and Blackburn, GL. Review of infant feeding: key features of breast milk and infant formula. Nutrients. (2016) 8:279. doi: 10.3390/nu8050279
22. Global Market Insight. (2022). Infant formula market size, by product (standard, follow-on, toddler, specialty), by distribution channel (hypermarket, supermarket, convenience stores, online), industry analysis report, regional outlook, end-user development potential, Price trends, Competitive Market Share & Forecast, 2023–2032. Available at: https://www.gminsights.com/industry-analysis/infant-formula-market (Accessed December 22, 2022).
23. Future Market Insights. (2021). Market insights on infant formula covering sales outlook, demand forecast & up-to-date key trends. Available at: https://www.futuremarketinsights.com/reports/infant-formula-market#thankyou (Accessed December 14, 2022).
24. Semba, RD. The rise and fall of protein malnutrition in global health. Ann Nutr Metab. (2016) 69:79–88. doi: 10.1159/000449175
25. Visioli, F, and Strata, A. Milk, dairy products, and their functional effects in humans: a narrative review of recent evidence. Adv Nutr. (2014) 5:131–43. doi: 10.3945/an.113.005025
26. Dror, DK, and Allen, LH. Dairy product intake in children and adolescents in developed countries: trends, nutritional contribution, and a review of association with health outcomes. Nutr Rev. (2014) 72:68–81. doi: 10.1111/nure.12078
27. Grażyna, C, Hanna, C, Adam, A, and Magdalena, BM. Natural antioxidants in milk and dairy products. Int J Dairy Technol. (2017) 70:165–78. doi: 10.1111/1471-0307.12359
28. Punia, H, Tokas, J, Malik, A, Sangwan, S, Baloda, S, Singh, N, et al. Identification and detection of bioactive peptides in milk and dairy products: remarks about agro-foods. Molecules. (2020) 25:3328. doi: 10.3390/molecules25153328
29. Yi, DY, and Kim, SY. Human breast milk composition and function in human health: from nutritional components to microbiome and microRNAs. Nutrients. (2021) 13:3094. doi: 10.3390/nu13093094
30. Fischer Fumeaux, CJ, Garcia-Rodenas, CL, De Castro, CA, Courtet-Compondu, M-C, Thakkar, SK, Beauport, L, et al. Longitudinal analysis of macronutrient composition in preterm and term human milk: a prospective cohort study. Nutrients. (2019) 11:1525. doi: 10.3390/nu11071525
31. Shah, R, Sabir, S, and Alhawaj, AF. Physiology, breast milk. Treasure Island, FL: StatPearls Publishing (2022).
32. Taponen, S, McGuinness, D, Hiitiö, H, Simojoki, H, Zadoks, R, and Pyörälä, S. Bovine milk microbiome: a more complex issue than expected. Vet Res. (2019) 50:1–15. doi: 10.1186/s13567-019-0662-y
33. Masum, AKM, Chandrapala, J, Huppertz, T, Adhikari, B, and Zisu, B. Production and characterization of infant milk formula powders: a review. Dry Technol. (2021) 39:1492–512. doi: 10.1080/07373937.2020.1767645
34. Numpaque, M, Şanlı, T, and Anli, EA. Diversity of milks other than cow, sheep, goat and buffalo: in terms of nutrition and technological use. Turkish J Agricult Food Sci Technol. (2019) 7:2047–53. doi: 10.24925/turjaf.v7i12.2047-2053.2623
35. Lagutin, K, MacKenzie, A, Bloor, S, Scott, D, and Vyssotski, M. HPLC-MS, GC and NMR profiling of bioactive lipids of human milk and milk of dairy animals (cow, sheep, goat, buffalo, camel, red deer). Separations. (2022) 9:145. doi: 10.3390/separations9060145
36. Crowley, SV, Kelly, AL, Lucey, JA, and O’Mahony, JA. Potential applications of non-bovine mammalian Milk in infant nutrition In: Handbook of Milk of non-bovine mammals. eds. Y. W. Park, G. F. W. Haenlein, and W. L. Wendorff (Online Wiley Library) (2017). 625–54.
37. Flom, JD, and Sicherer, SH. Epidemiology of cow’s milk allergy. Nutrients. (2019) 11:1051. doi: 10.3390/nu11051051
38. Schoemaker, AA, Sprikkelman, AB, Grimshaw, KE, Roberts, G, Grabenhenrich, L, Rosenfeld, L, et al. Incidence and natural history of challenge-proven cow’s milk allergy in European children–EuroPrevall birth cohort. Allergy. (2015) 70:963–72. doi: 10.1111/all.12630
39. Souroullas, K, Aspri, M, and Papademas, P. Donkey milk as a supplement in infant formula: benefits and technological challenges. Food Res Int. (2018) 109:416–25. doi: 10.1016/j.foodres.2018.04.051
40. Mudgil, P, Baba, WN, Alneyadi, M, Redha, AA, and Maqsood, S. Production, characterization, and bioactivity of novel camel milk-based infant formula in comparison to bovine and commercial sources. Lwt. (2022) 154:112813. doi: 10.1016/j.lwt.2021.112813
41. Belinska, K, Falendysh, N, and Marusei, T. Study of efficiency and quality of dry milk mixtures for baby nutrition in the storage process. Food Sci. Technol. (2021) 15:77–86. doi: 10.15673/fst.v15i4.2261
42. Roy, D, Ye, A, Moughan, PJ, and Singh, H. Composition, structure, and digestive dynamics of milk from different species—a review. Front Nutr. (2020) 7:577759. doi: 10.3389/fnut.2020.577759
43. Alichanidis, E, Moatsou, G, and Polychroniadou, A. Composition and properties of non-cow milk and products In: Non-bovine milk and milk products. eds. E. Tsakalidou and K. Papadimitriou (Amsterdam: Elsevier, Academic Press) (2016). 81–116.
44. Agne-Djigo, A, Kwadjode, KM, Idohou-Dossou, N, Diouf, A, Guiro, AT, and Wade, S. Energy intake from human milk covers the requirement of 6-month-old Senegalese exclusively breast-fed infants. Br J Nutr. (2013) 110:1849–55. doi: 10.1017/S0007114513001074
45. Claeys, WL, Verraes, C, Cardoen, S, De Block, J, Huyghebaert, A, Raes, K, et al. Consumption of raw or heated milk from different species: An evaluation of the nutritional and potential health benefits. Food Control. (2014) 42:188–201. doi: 10.1016/j.foodcont.2014.01.045
46. Mohanty, DP, Mohapatra, S, Misra, S, and Sahu, PS. Milk derived bioactive peptides and their impact on human health–a review. Saudi J Biol Sci. (2016) 23:577–83. doi: 10.1016/j.sjbs.2015.06.005
47. Guha, S, Sharma, H, Deshwal, GK, and Rao, PS. A comprehensive review on bioactive peptides derived from milk and milk products of minor dairy species. Food Prod Process Nutr. (2021) 3:1–21. doi: 10.1186/s43014-020-00045-7
48. Zaky, AA, Shim, J-H, and Abd El-Aty, AM. A review on extraction, characterization, and applications of bioactive peptides from pressed black cumin seed cake. Front Nutr. (2021) 8:743909. doi: 10.3389/fnut.2021.743909
49. Hsieh, C-C, Hernández-Ledesma, B, Fernández-Tomé, S, Weinborn, V, Barile, D, and de Moura, BJMLN. Milk proteins, peptides, and oligosaccharides: effects against the 21st century disorders. Biomed Res Int. (2015) 2015:6840. doi: 10.1155/2015/146840
50. Lucey, JA, Otter, D, and Horne, DS. A 100-year review: Progress on the chemistry of milk and its components. J Dairy Sci. (2017) 100:9916–32. doi: 10.3168/jds.2017-13250
51. Layman, DK, Lönnerdal, B, and Fernstrom, JD. Applications for α-lactalbumin in human nutrition. Nutr Rev. (2018) 76:444–60. doi: 10.1093/nutrit/nuy004
52. Parastouei, K, Jabbari, M, Javanmardi, F, Barati, M, Mahmoudi, Y, Khalili-Moghadam, S, et al. Estimation of bioactive peptide content of milk from different species using an in silico method. Amino Acids. (2022):1–18. doi: 10.1007/s00726-022-03152-6 [Epub ahead of print].
53. Kumar, D, Chatli, MK, Singh, R, Mehta, N, and Kumar, P. Enzymatic hydrolysis of camel milk casein and its antioxidant properties. Dairy Sci Technol. (2016) 96:391–404. doi: 10.1007/s13594-015-0275-9
54. Chen, L, Zhang, Q, Ji, Z, Shu, G, and Chen, H. Production and fermentation characteristics of angiotensin-I-converting enzyme inhibitory peptides of goat milk fermented by a novel wild Lactobacillus plantarum 69. LWT. (2018) 91:532–40. doi: 10.1016/j.lwt.2018.02.002
55. Pei, J, Jiang, H, Li, X, Jin, W, and Tao, Y. Antimicrobial peptides sourced from post-butter processing waste yak milk protein hydrolysates. AMB Express. (2017) 7:1–6. doi: 10.1186/s13568-017-0497-8
56. Nielsen, SD, Beverly, RL, Qu, Y, and Dallas, DC. Milk bioactive peptide database: a comprehensive database of milk protein-derived bioactive peptides and novel visualization. Food Chem. (2017) 232:673–82. doi: 10.1016/j.foodchem.2017.04.056
57. Park, YW, and Nam, MS. Bioactive peptides in milk and dairy products: a review. Korean J Food Sci Anim Resour. (2015) 35:831. doi: 10.5851/kosfa.2015.35.6.831
58. Hageman, JHJ, Danielsen, M, Nieuwenhuizen, AG, Feitsma, AL, and Dalsgaard, TK. Comparison of bovine milk fat and vegetable fat for infant formula: implications for infant health. Int Dairy J. (2019) 92:37–49. doi: 10.1016/j.idairyj.2019.01.005
59. Lund, P, Bechshøft, MR, Ray, CA, and Lund, MN. Effect of processing of whey protein ingredient on Maillard reactions and protein structural changes in powdered infant formula. J Agric Food Chem. (2022) 70:319–32. doi: 10.1021/acs.jafc.1c05612
60. Wahlqvist, ML, and Wattanapenpaiboon, N. Macronutrients: carbohydrates In: Food and nutrition. Abingdon: Routledge (2020). 208–24.
61. EU. (2016). Commission delegated regulation (EU) 2016/127 of 25 September 2015 supplementing regulation (EU) no 609/2013 of the European Parliament and of the council as regards the specific compositional and information requirements for infant formula and follow-on formula and as regards requirements on information relating to infant and young child feeding. eds. D. Gallegos and M. L. Wahlqvist (Official Journal of the European Union). Available at: http://data.europa.eu/eli/reg_del/2016/127/oj (Accessed December 14, 2022).
62. Sjöblad, S. Could the high consumption of high glycaemic index carbohydrates and sugars, associated with the nutritional transition to the Western type of diet, be the common cause of the obesity epidemic and the worldwide increasing incidences of type 1 and type 2 d. Med Hypotheses. (2019) 125:41–50. doi: 10.1016/j.mehy.2019.02.027
63. EFSA Panel on Dietetic Products N and A (NDA). Scientific opinion on the essential composition of infant and follow-on formulae. EFSA J. (2014) 12:3760. doi: 10.2903/j.efsa.2014.3760
64. Masum, AKM, Chandrapala, J, Adhikari, B, Huppertz, T, and Zisu, B. Effect of lactose-to-maltodextrin ratio on emulsion stability and physicochemical properties of spray-dried infant milk formula powders. J Food Eng. (2019) 254:34–41. doi: 10.1016/j.jfoodeng.2019.02.023
65. Ahern, GJ, Hennessy, AA, Ryan, CA, Ross, RP, and Stanton, C. Advances in infant formula science. Annu Rev Food Sci Technol. (2019) 10:75–102. doi: 10.1146/annurev-food-081318-104308
66. Giorgio, D, Di Trana, A, and Claps, S. Oligosaccharides, polyamines and sphingolipids in ruminant milk. Small Rumin Res. (2018) 160:23–30. doi: 10.1016/j.smallrumres.2018.01.006
67. van Leeuwen, SS, te Poele, EM, Chatziioannou, AC, Benjamins, E, Haandrikman, A, and Dijkhuizen, L. Goat Milk oligosaccharides: their diversity, quantity, and functional properties in comparison to human Milk oligosaccharides. J Agric Food Chem. (2020) 68:13469–85. doi: 10.1021/acs.jafc.0c03766
68. Wadhwani, KN, Bhavsar, M, Islam, MM, Patel, JH, and Lunagariya, PM. Modern health concept of non-bovine milk: a review. Indian J Anim Product Manag. (2023) 37:12–22. doi: 10.48165/ijapm.2023.37.1.8
69. Quinn, EM, O’Callaghan, TF, Tobin, JT, Murphy, JP, Sugrue, K, Slattery, H, et al. Changes to the oligosaccharide profile of bovine milk at the onset of lactation. Dairy. (2020) 1:284–96. doi: 10.3390/dairy1030019
70. Martín-Ortiz, A, Barile, D, Salcedo, J, Moreno, FJ, Clemente, A, Ruiz-Matute, AI, et al. Changes in caprine milk oligosaccharides at different lactation stages analyzed by high performance liquid chromatography coupled to mass spectrometry. J Agric Food Chem. (2017) 65:3523–31. doi: 10.1021/acs.jafc.6b05104
71. Tao, N, DePeters, EJ, German, JB, Grimm, R, and Lebrilla, CB. Variations in bovine milk oligosaccharides during early and middle lactation stages analyzed by high-performance liquid chromatography-chip/mass spectrometry. J Dairy Sci. (2009) 92:2991–3001. doi: 10.3168/jds.2008-1642
72. Licitra, R, Li, J, Liang, X, Altomonte, I, Salari, F, Yan, J, et al. Profile and content of sialylated oligosaccharides in donkey milk at early lactation. LWT. (2019) 115:108437. doi: 10.1016/j.lwt.2019.108437
73. Weng, W-C, Liao, H-E, Huang, S-P, Tsai, S-T, Hsu, H-C, Liew, CY, et al. Unusual free oligosaccharides in human bovine and caprine milk. Sci Rep. (2022) 12:10790. doi: 10.1038/s41598-022-15140-7
74. Robinson, RC. Structures and metabolic properties of bovine milk oligosaccharides and their potential in the development of novel therapeutics. Front Nutr. (2019) 6:50. doi: 10.3389/fnut.2019.00050
75. Li, W, Wang, J, Lin, Y, Li, Y, Ren, F, and Guo, H. How far is it from infant formula to human milk? A look at the human milk oligosaccharides. Trends Food Sci Technol. (2021) 118:374–87. doi: 10.1016/j.tifs.2021.09.021
76. Shi, Y, Han, B, Zhang, L, and Zhou, P. Comprehensive identification and absolute quantification of milk oligosaccharides in different species. J Agric Food Chem. (2021) 69:15585–97. doi: 10.1021/acs.jafc.1c05872
77. Nayik, GA, Jagdale, YD, Gaikwad, SA, Devkatte, AN, Dar, AH, and Ansari, MJ. Nutritional profile, processing and potential products: a comparative review of goat milk. Dairy. (2022) 3:622–47. doi: 10.3390/dairy3030044
78. Altomonte, I, Salari, F, Licitra, R, and Martini, M. Donkey and human milk: insights into their compositional similarities. Int Dairy J. (2019) 89:111–8. doi: 10.1016/j.idairyj.2018.09.005
79. Leong, A, Liu, Z, Almshawit, H, Zisu, B, Pillidge, C, Rochfort, S, et al. Oligosaccharides in goats’ milk-based infant formula and their prebiotic and anti-infection properties. Br J Nutr. (2019) 122:441–9. doi: 10.1017/S000711451900134X
80. Byrne, ME, O’Mahony, JA, and O’Callaghan, TF. Compositional and functional considerations for bovine-, caprine-and plant-based infant formulas. Dairy. (2021) 2:695–715. doi: 10.3390/dairy2040054
81. Fenelon, MA, Hickey, RM, Buggy, A, McCarthy, N, and Murphy, EG. “Whey proteins in infant formula,” in Whey proteins. eds. H. C. Deeth and N. Bansal (Amsterdam: Elsevier) (2019). 439–94.
82. Prosser, CG. Compositional and functional characteristics of goat milk and relevance as a base for infant formula. J Food Sci. (2021) 86:257–65. doi: 10.1111/1750-3841.15574
83. Drapala, KP, Auty, MAE, Mulvihill, DM, and O’Mahony, JA. Influence of emulsifier type on the spray-drying properties of model infant formula emulsions. Food Hydrocoll. (2017) 69:56–66. doi: 10.1016/j.foodhyd.2016.12.024
84. Murphy, EG, Roos, YH, Hogan, SA, Maher, PG, Flynn, CG, and Fenelon, MA. Physical stability of infant milk formula made with selectively hydrolysed whey proteins. Int Dairy J. (2015) 40:39–46. doi: 10.1016/j.idairyj.2014.08.012
85. Toikkanen, O, Outinen, M, Malafronte, L, and Rojas, OJ. Formation and structure of insoluble particles in reconstituted model infant formula powders. Int Dairy J. (2018) 82:19–27. doi: 10.1016/j.idairyj.2018.03.001
86. Nowak-Wegrzyn, A, Czerkies, L, Reyes, K, Collins, B, and Heine, RG. Confirmed hypoallergenicity of a novel whey-based extensively hydrolyzed infant formula containing two human milk oligosaccharides. Nutrients. (2019) 11:1447. doi: 10.3390/nu11071447
87. Katz, DL, Doughty, KN, Geagan, K, Jenkins, DA, and Gardner, CD. Perspective: the public health case for modernizing the definition of protein quality. Adv Nutr. (2019) 10:755–64. doi: 10.1093/advances/nmz023
88. Mathai, JK, Liu, Y, and Stein, HH. Values for digestible indispensable amino acid scores (DIAAS) for some dairy and plant proteins may better describe protein quality than values calculated using the concept for protein digestibility-corrected amino acid scores (PDCAAS). Br J Nutr. (2017) 117:490–9. doi: 10.1017/S0007114517000125
89. Maathuis, A, Havenaar, R, He, T, and Bellmann, S. Protein digestion and quality of goat and cow milk infant formula and human milk under simulated infant conditions. J Pediatr Gastroenterol Nutr. (2017) 65:661. doi: 10.1097/MPG.0000000000001740
90. Újvári, G, Bencsik, D, and Zsótér, BT. Sport habits and food purchasing and consuming patterns of vegetarians and vegans in Hungary. Quaestus Multidiscipl Res J. (2021) 18:112–22.
91. Layman, DK, and Rodriguez, NR. Egg protein as a source of power, strength, and energy. Nutr Today. (2009) 44:43–8. doi: 10.1097/NT.0b013e3181959cb2
92. Gerchev, G, Iliev, T, Slavkova, S, and Mihaylova, G. Chemical composition and biological protein value of milk of Tsigai sheep and their F2 cross-breeds of Chios. Biotechnol Animal Husbandry. (2014) 30:243–50. doi: 10.2298/BAH1402243G
93. Belewu, MA, and Aiyegbusi, OF. Comparison of the mineral content and apparent biological value of milk from human, cow and goat. J Food Technol Africa. (2002) 7:9–11. doi: 10.4314/jfta.v7i1.19310
94. Bezie, A. The effect of different heat treatment on the nutritional value of Milk and Milk products and shelf-life of Milk products. A review. JDVS. (2019) 11:555822. doi: 10.19080/JDVS.2019.11.555822
95. Metta, VC, and Johnson, BC. The effect of radiation sterilization on the nutritive value of foods: I. biological value of Milk and beef proteins. J Nutr. (1956) 59:479–90. doi: 10.1093/jn/59.4.479
96. Sharma, G, Rout, PK, Kaushik, R, and Singh, G. Identification of bioactive peptides in goat milk and their health application. J Adv Dairy Res. (2017) 5:1000191. doi: 10.4172/2329-888X.1000191
97. Bhavaniramya, S, Sibiya, A, Alothaim, AS, Al Othaim, A, Ramar, V, Veluchamy, A, et al. Evaluating the structural and immune mechanism of Interleukin-6 for the investigation of goat milk peptides as potential treatments for COVID-19. J King Saud Univer Sci. (2022) 34:101924. doi: 10.1016/j.jksus.2022.101924
98. Martini, M, Altomonte, I, Tricò, D, Lapenta, R, and Salari, F. Current knowledge on functionality and potential therapeutic uses of donkey milk. Animals. (2021) 11:1382. doi: 10.3390/ani11051382
99. Ma, X, Yang, F, Meng, X, Wu, Y, Tong, P, Gao, J, et al. Immunomodulatory role of BLG-derived peptides based on simulated gastrointestinal digestion and DC-T cell from mice allergic to Cow’s Milk. Foods. (2022) 11:1450. doi: 10.3390/foods11101450
100. Mazzocchi, A, D’Oria, V, De, CV, Bettocchi, S, Milani, GP, Silano, M, et al. The role of lipids in human milk and infant formulae. Nutrients. (2018) 10:567. doi: 10.3390/nu10050567
101. Skolnick, J, Chou, C, and Miklavcic, J. Insights into novel infant milk formula bioactives. Nutr Diet Suppl. (2020) 12:11–9. doi: 10.2147/NDS.S192099
102. Innis, SM. Palmitic acid in early human development. Crit Rev Food Sci Nutr. (2016) 56:1952–9. doi: 10.1080/10408398.2015.1018045
103. Bar-Yoseph, F, Lifshitz, Y, and Cohen, T. Review of sn-2 palmitate oil implications for infant health. Prostaglandins Leukot Essent Fatty Acids. (2013) 89:139–43. doi: 10.1016/j.plefa.2013.03.002
104. Sharma, R, Diwan, B, Sharma, A, and Witkowski, JM. Emerging cellular senescence-centric understanding of immunological aging and its potential modulation through dietary bioactive components. Biogerontology. (2022) 23:699–729. doi: 10.1007/s10522-022-09995-6
105. Mehrotra, V, Sehgal, SK, and Bangale, NR. Fat structure and composition in human milk and infant formulas: implications in infant health. Clin Epidemiol Glob Health. (2019) 7:153–9. doi: 10.1016/j.cegh.2018.03.005
106. Chen, M, and Sun, Q. Current knowledge in the stabilization/destabilization of infant formula emulsions during processing as affected by formulations. Trends Food Sci Technol. (2021) 109:435–47. doi: 10.1016/j.tifs.2021.01.036
107. Liang, Y, Wong, S-S, Pham, SQ, and Tan, JJ. Effects of globular protein type and concentration on the physical properties and flow behaviors of oil-in-water emulsions stabilized by micellar casein–globular protein mixtures. Food Hydrocoll. (2016) 54:89–98. doi: 10.1016/j.foodhyd.2015.09.024
108. Liang, Y, Matia-Merino, L, Gillies, G, Patel, H, Ye, A, and Golding, M. The heat stability of milk protein-stabilized oil-in-water emulsions: a review. Curr Opin Colloid Interface Sci. (2017) 28:63–73. doi: 10.1016/j.cocis.2017.03.007
109. Sun, Y, Ma, S, Liu, Y, Jia, Z, Li, X, Liu, L, et al. Changes in interfacial composition and structure of milk fat globules are crucial regulating lipid digestion in simulated in-vitro infant gastrointestinal digestion. Food Hydrocoll. (2023) 134:108003. doi: 10.1016/j.foodhyd.2022.108003
110. Gallier, S, Vocking, K, Post, JA, Van De Heijning, B, Acton, D, Van Der Beek, EM, et al. A novel infant milk formula concept: mimicking the human milk fat globule structure. Colloids Surf B Biointerfaces. (2015) 136:329–39. doi: 10.1016/j.colsurfb.2015.09.024
111. Yao, Y, Zhao, G, Xiang, J, Zou, X, Jin, Q, and Wang, X. Lipid composition and structural characteristics of bovine, caprine and human milk fat globules. Int Dairy J. (2016) 56:64–73. doi: 10.1016/j.idairyj.2015.12.013
112. Martini, M, Altomonte, I, Licitra, R, and Salari, F. Nutritional and nutraceutical quality of donkey milk. J Equine Vet Sci. (2018) 65:33–7. doi: 10.1016/j.jevs.2017.10.020
113. Thum, C, Roy, NC, Everett, DW, and McNabb, WC. Variation in milk fat globule size and composition: a source of bioactives for human health. Crit Rev Food Sci Nutr. (2023) 63:87–113. doi: 10.1080/10408398.2021.1944049
114. Gallier, S, Tolenaars, L, and Prosser, C. Whole goat milk as a source of fat and milk fat globule membrane in infant formula. Nutrients. (2020) 12:3486. doi: 10.3390/nu12113486
115. Adriano, B, Cotto, NM, Chauhan, N, Jaggi, M, Chauhan, SC, and Yallapu, MM. Milk exosomes: Nature’s abundant nanoplatform for theranostic applications. Bioact Mater. (2021) 6:2479–90. doi: 10.1016/j.bioactmat.2021.01.009
116. Hock, A, Miyake, H, Li, B, Lee, C, Ermini, L, Koike, Y, et al. Breast milk-derived exosomes promote intestinal epithelial cell growth. J Pediatr Surg. (2017) 52:755–9. doi: 10.1016/j.jpedsurg.2017.01.032
117. García-Martínez, J, Pérez-Castillo, ÍM, Salto, R, López-Pedrosa, JM, Rueda, R, and Girón, MD. Beneficial effects of bovine milk exosomes in metabolic interorgan cross-talk. Nutrients. (2022) 14:1442. doi: 10.3390/nu14071442
118. Badawy, AA, El-Magd, MA, and AlSadrah, SA. Therapeutic effect of camel milk and its exosomes on MCF7 cells in vitro and in vivo. Integr Cancer Ther. (2018) 17:1235–46. doi: 10.1177/1534735418786000
119. Rashidi, M, Bijari, S, Khazaei, AH, Shojaei-Ghahrizjani, F, and Rezakhani, L. The role of milk-derived exosomes in the treatment of diseases. Front Genet. (2022) 13:1009338. doi: 10.3389/fgene.2022.1009338
120. Wijenayake, S, Eisha, S, Tawhidi, Z, Pitino, MA, Steele, MA, Fleming, AS, et al. Comparison of methods for pre-processing, exosome isolation, and RNA extraction in unpasteurized bovine and human milk. PLoS One. (2021) 16:e0257633. doi: 10.1371/journal.pone.0257633
121. McSweeney, S, O’Regan, J, and O’Callaghan, D. Nutritional formulae for infants and young children In: Milk and dairy products in human nutrition. eds. Y. W. Park and G. F. W. Haenlein (Oxford: John Wiley & Sons) (2013). 458–76.
122. Dumpler, J, Huppertz, T, and Kulozik, U. Invited review: heat stability of milk and concentrated milk: past, present, and future research objectives. J Dairy Sci. (2020) 103:10986–1007. doi: 10.3168/jds.2020-18605
123. Bartleman, J. Infant and child nutrition. Medicine (Abingdon). (2019) 47:195–8. doi: 10.1016/j.mpmed.2018.12.002
124. Wang, W, Li, Y, Cai, L, and Fang, L. Characteristics on the oxidation stability of infant formula powder with different ingredients during storage. Food Sci Nutr. (2020) 8:6392–400. doi: 10.1002/fsn3.1928
125. Therattil, JM, and Bahna, SL. Hypoallergenic formulas In: Food hypersensitivity and adverse reactions. eds. M. Frieri and B. Kettelhut (Boca Raton, FL: CRC Press) (2019). 477–83.
126. Morante-Palacios, O, Godoy-Tena, G, Calafell-Segura, J, Ciudad, L, Martínez-Cáceres, EM, Sardina, JL, et al. Vitamin C enhances NF-κB-driven epigenomic reprogramming and boosts the immunogenic properties of dendritic cells. Nucleic Acids Res. (2022) 50:10981–94. doi: 10.1093/nar/gkac941
127. Ahvanooei, MRR, Norouzian, MA, and Vahmani, P. Beneficial effects of vitamins, minerals, and bioactive peptides on strengthening the immune system against COVID-19 and the role of cow’s milk in the supply of these nutrients. Biol Trace Elem Res. (2021) 200:4664–77. doi: 10.1007/s12011-021-03045-x
128. Karacabey, K, and Ozdemir, N. The effect of nutritional elements on the immune system. J Obes Wt Loss Ther. (2012) 2:152. doi: 10.4172/2165-7904.1000152
129. Kumar, P, Kumar, M, Bedi, O, Gupta, M, Kumar, S, Jaiswal, G, et al. Role of vitamins and minerals as immunity boosters in COVID-19. Inflammopharmacology. (2021) 29:1001–16. doi: 10.1007/s10787-021-00826-7
130. Weyh, C, Krüger, K, Peeling, P, and Castell, L. The role of minerals in the optimal functioning of the immune system. Nutrients. (2022) 14:644. doi: 10.3390/nu14030644
131. Molska, A, Gutowska, I, Baranowska-Bosiacka, I, Noceń, I, and Chlubek, D. The content of elements in infant formulas and drinks against mineral requirements of children. Biol Trace Elem Res. (2014) 158:422–7. doi: 10.1007/s12011-014-9947-1
132. Venema, K, Verhoeven, J, and Verbruggen, S. Calcium and phosphorus bioaccessibility from different amino acid-based medical nutrition formulas for infants and children under in vitro digestive conditions. Clin Nutr Exp. (2020) 32:20–8. doi: 10.1016/j.yclnex.2020.06.002
133. Moraes, MR, do Nascimento da Silva, E, Sanches, VL, Cadore, S, and Godoy, HT. Bioaccessibility of some minerals in infant formulas. J Food Sci Technol. (2022) 59:2004–12. doi: 10.1007/s13197-021-05215-0
134. Sun, X, Sarteshnizi, RA, Boachie, RT, Okagu, OD, Abioye, RO, Pfeilsticker Neves, R, et al. Peptide–mineral complexes: understanding their chemical interactions, bioavailability, and potential application in mitigating micronutrient deficiency. Foods. (2020) 9:1402. doi: 10.3390/foods9101402
135. Wang, Y, Ye, A, Hou, Y, Jin, Y, Xu, X, Han, J, et al. Microcapsule delivery systems of functional ingredients in infant formulae: research progress, technology, and feasible application of liposomes. Trends Food Sci Technol. (2022) 119:36–44. doi: 10.1016/j.tifs.2021.11.016
136. Bohn, T. Determinants and determination of carotenoid bioavailability from infant food formulas and adult nutritionals including liquid dairy products. J AOAC Int. (2019) 102:1044–58. doi: 10.5740/jaoacint.19-0015
137. Brandon, EFA, Bakker, MI, Kramer, E, Bouwmeester, H, Zuidema, T, and Alewijn, M. Bioaccessibility of vitamin A, vitamin C and folic acid from dietary supplements, fortified food and infant formula. Int J Food Sci Nutr. (2014) 65:426–35. doi: 10.3109/09637486.2013.869795
138. Turck, D, Castenmiller, J, De Henauw, S, Hirsch-Ernst, KI, Kearney, J, Maciuk, A, et al. Calcium l-methylfolate as a source of folate added for nutritional purposes to infant and follow-on formula, baby food and processed cereal-based food. EFSA Journal (2020).
139. Otadi, M, and Zabihi, F. Vitamin E microcapsulation by Ethylcellulose through emulsion solvent evaporation technique; An operational condition study. World Appl Sci J. (2011) 14:20–5.
140. Cellar, NA, McClure, SC, Salvati, LM, and Reddy, TM. A new sample preparation and separation combination for precise, accurate, rapid, and simultaneous determination of vitamins B1, B2, B3, B5, B6, B7, and B9 in infant formula and related nutritionals by LC-MS/MS. Anal Chim Acta. (2016) 934:180–5. doi: 10.1016/j.aca.2016.05.058
141. Woollard, DC, Bensch, A, Indyk, H, and McMahon, A. Determination of vitamin a and vitamin E esters in infant formulae and fortified milk powders by HPLC: use of internal standardisation. Food Chem. (2016) 197:457–65. doi: 10.1016/j.foodchem.2015.10.077
142. Hao, L, Shan, Q, Wei, J, Ma, F, and Sun, P. Lactoferrin: major physiological functions and applications. Curr Protein Pept Sci. (2019) 20:139–44. doi: 10.2174/1389203719666180514150921
143. Li, Y, Gill, BD, Grainger, MNC, and Manley-Harris, M. The analysis of vitamin B12 in milk and infant formula: a review. Int Dairy J. (2019) 99:104543. doi: 10.1016/j.idairyj.2019.104543
144. Van De Looij, Y, Ginet, V, Chatagner, A, Toulotte, A, Somm, E, Hüppi, PS, et al. Lactoferrin during lactation protects the immature hypoxic-ischemic rat brain. Ann Clin Transl Neurol. (2014) 1:955–67. doi: 10.1002/acn3.138
145. Chen, Y, Zheng, Z, Zhu, X, Shi, Y, Tian, D, Zhao, F, et al. Lactoferrin promotes early neurodevelopment and cognition in postnatal piglets by upregulating the BDNF signaling pathway and polysialylation. Mol Neurobiol. (2015) 52:256–69. doi: 10.1007/s12035-014-8856-9
146. Wazed, MA, Ismail, M, and Farid, M. Pasteurized ready-to-feed (RTF) infant formula fortified with lactoferrin: a potential niche product. J Food Eng. (2020) 273:109810. doi: 10.1016/j.jfoodeng.2019.109810
147. Donovan, SM, Monaco, MH, Drnevich, J, Kvistgaard, AS, Hernell, O, and Lönnerdal, B. Bovine osteopontin modifies the intestinal transcriptome of formula-fed infant rhesus monkeys to be more similar to those that were breastfed. J Nutr. (2014) 144:1910–9. doi: 10.3945/jn.114.197558
148. FDA. (2017). FDA. U.S., GRAS notice no. GRN 716, bovine Milk Osteopontin (bOPN). Available at: https://www.fda.gov/files/food/published/GRAS-Notice-000716--Bovine-milk-osteopontin.pdf (Accessed December 20, 2022).
149. Song, MW, Kim, K-T, and Paik, H-D. Probiotics as a functional health supplement in infant formulas for the improvement of intestinal microflora and immunity. Food Rev Intl. (2021) 39:858–74. doi: 10.1080/87559129.2021.1928178
150. Bertelsen, RJ, Jensen, ET, and Ringel-Kulka, T. Use of probiotics and prebiotics in infant feeding. Best Pract Res Clin Gastroenterol. (2016) 30:39–48. doi: 10.1016/j.bpg.2016.01.001
151. Vandenplas, Y, De, GE, and Veereman, G. Prebiotics in infant formula. Gut Microbes. (2014) 5:681–7. doi: 10.4161/19490976.2014.972237
152. Skórka, A, Pieścik-Lech, M, Kołodziej, M, and Szajewska, H. Infant formulae supplemented with prebiotics: are they better than unsupplemented formulae? An updated systematic review. Br J Nutr. (2018) 119:810–25. doi: 10.1017/S0007114518000120
153. Puccio, G, Alliet, P, Cajozzo, C, Janssens, E, Corsello, G, Sprenger, N, et al. Effects of infant formula with human milk oligosaccharides on growth and morbidity: a randomized multicenter trial. J Pediatr Gastroenterol Nutr. (2017) 64:624. doi: 10.1097/MPG.0000000000001520
154. Vandenplas, Y, Zakharova, I, and Dmitrieva, Y. Oligosaccharides in infant formula: more evidence to validate the role of prebiotics. Br J Nutr. (2015) 113:1339–44. doi: 10.1017/S0007114515000823
155. Donovan, SM, and Comstock, SS. Human milk oligosaccharides influence neonatal mucosal and systemic immunity. Ann Nutr Metab. (2016) 69:41–51. doi: 10.1159/000452818
156. Hedtke, V, and Bakovic, M. Choline transport for phospholipid synthesis: an emerging role of choline transporter-like protein 1. Exp Biol Med. (2019) 244:655–62. doi: 10.1177/1535370219830997
157. Davenport, C, Yan, J, Taesuwan, S, Shields, K, West, AA, Jiang, X, et al. Choline intakes exceeding recommendations during human lactation improve breast milk choline content by increasing PEMT pathway metabolites. J Nutr Biochem. (2015) 26:903–11. doi: 10.1016/j.jnutbio.2015.03.004
158. Derbyshire, E. Could we be overlooking a potential choline crisis in the United Kingdom? BMJ Nutr Prev Health. (2019) 2:86. doi: 10.1136/bmjnph-2019-000037
159. Caudill, MA, Strupp, BJ, Muscalu, L, Nevins, JEH, and Canfield, RL. Maternal choline supplementation during the third trimester of pregnancy improves infant information processing speed: a randomized, double-blind, controlled feeding study. FASEB J. (2018) 32:2172. doi: 10.1096/fj.201700692RR
160. Strupp, J, Powers, BE, Velazquez, R, Ash, JA, Kelley, CM, Alldred, MJ, et al. Maternal choline supplementation: a potential prenatal treatment for down syndrome and Alzheimer’s disease. Curr Alzheimer Res. (2016) 13:97–106. doi: 10.2174/1567205012666150921100311
161. Van Hese, I, Goossens, K, Vandaele, L, and Opsomer, G. Invited review: MicroRNAs in bovine colostrum—focus on their origin and potential health benefits for the calf. J Dairy Sci. (2020) 103:1–15. doi: 10.3168/jds.2019-16959
162. Lukasik, A, Brzozowska, I, Zielenkiewicz, U, and Zielenkiewicz, P. Detection of plant miRNAs abundance in human breast milk. Int J Mol Sci. (2017) 19:37. doi: 10.3390/ijms19010037
163. Mosca, F, and Giannì, ML. Human milk: composition and health benefits. Pediatr Med Chir. (2017) 39:155. doi: 10.4081/pmc.2017.155
164. Golan-Gerstl, R, Elbaum Shiff, Y, Moshayoff, V, Schecter, D, Leshkowitz, D, and Reif, S. Characterization and biological function of milk-derived miRNAs. Mol Nutr Food Res. (2017) 61:1700009. doi: 10.1002/mnfr.201700009
165. Denzler, R, and Stoffel, M. Uptake and function studies of maternal milk-derived microRNAs. J Biol Chem. (2015) 290:23680–91. doi: 10.1074/jbc.M115.676734
166. Alsaweed, M, Hartmann, PE, Geddes, DT, and Kakulas, F. MicroRNAs in breastmilk and the lactating breast: potential immunoprotectors and developmental regulators for the infant and the mother. Int J Environ Res Public Health. (2015) 12:13981–4020. doi: 10.3390/ijerph121113981
167. Casas-Agustench, P, Iglesias-Gutierrez, E, and Davalos, A. Mother’s nutritional miRNA legacy: nutrition during pregnancy and its possible implications to develop cardiometabolic disease in later life. Pharmacol Res. (2015) 100:322–34. doi: 10.1016/j.phrs.2015.08.017
168. Yun, B, Kim, Y, Park, DJ, and Oh, S. Comparative analysis of dietary exosome-derived microRNAs from human, bovine and caprine colostrum and mature milk. J Anim Sci Technol. (2021) 63:593. doi: 10.5187/jast.2021.e39
169. Zempleni, J, Aguilar-Lozano, A, Sadri, M, Sukreet, S, Manca, S, Wu, D, et al. Biological activities of extracellular vesicles and their cargos from bovine and human milk in humans and implications for infants. J Nutr. (2017) 147:3–10. doi: 10.3945/jn.116.238949
170. Bene, J, Szabo, A, Komlósi, K, and Melegh, B. Mass spectrometric analysis of L-carnitine and its esters: potential biomarkers of disturbances in carnitine homeostasis. Curr Mol Med. (2020) 20:336. doi: 10.2174/1566524019666191113120828
171. Mikhael, EM. Comparison among commonly available infant formula milks in the Iraqi market. Glob Pediatr Health. (2015) 2:2333794X15608716. doi: 10.1177/2333794X15608716
172. Zielińska, MA, Wesołowska, A, Pawlus, B, and Hamułka, J. Health effects of carotenoids during pregnancy and lactation. Nutrients. (2017) 9:838. doi: 10.3390/nu9080838
173. Capeding, R, Gepanayao, CP, Calimon, N, Lebumfacil, J, Davis, AM, Stouffer, N, et al. Lutein-fortified infant formula fed to healthy term infants: evaluation of growth effects and safety. Nutr J. (2010) 9:1–9. doi: 10.1186/1475-2891-9-22
174. Eggersdorfer, M, and Wyss, A. Carotenoids in human nutrition and health. Arch Biochem Biophys. (2018) 652:18–26. doi: 10.1016/j.abb.2018.06.001
175. Lipkie, TE, Morrow, AL, Jouni, ZE, McMahon, RJ, and Ferruzzi, MG. Longitudinal survey of carotenoids in human milk from urban cohorts in China, Mexico, and the USA. PLoS One. (2015) 10:e0127729. doi: 10.1371/journal.pone.0127729
176. Stringham, JM, Johnson, EJ, and Hammond, BR. Lutein across the lifespan: from childhood cognitive performance to the aging eye and brain. Curr Dev Nutr. (2019) 3:nzz066. doi: 10.1093/cdn/nzz066
177. Li, LH, Lee, JCY, Leung, HH, Lam, WC, Fu, Z, and Lo, ACY. Lutein supplementation for eye diseases. Nutrients. (2020) 12:1721. doi: 10.3390/nu12061721
178. Li, X, Holt, RR, Keen, CL, Morse, LS, Zivkovic, AM, Yiu, G, et al. Potential roles of dietary zeaxanthin and lutein in macular health and function. Nutr Rev. (2022) 80:1755–68. doi: 10.1093/nutrit/nuac005
179. Tso, P, Vurma, M, Ko, C-W, Lee, D, and DeMichele, S. Effect of mono-and diglycerides on the digestion and absorption of lutein in lymph fistula rats. Am J Physiol Gastroint Liver Physiol. (2018) 315:G95–G103. doi: 10.1152/ajpgi.00236.2017
180. Ma, C-C, Butler, D, Milligan, V, Hammann, BA, Luo, H, Brazdil, JF, et al. Continuous process for the production of taurine from monoethanolamine. Ind Eng Chem Res. (2020) 59:13007–15. doi: 10.1021/acs.iecr.0c02277
181. Chawla, D. Taurine and neonatal nutrition. Indian J Pediatrics. (2018) 85:829. doi: 10.1007/s12098-018-2781-2
182. Ryan, JM, Rice, GE, and Mitchell, MD. The role of gangliosides in brain development and the potential benefits of perinatal supplementation. Nutr Res. (2013) 33:877–87. doi: 10.1016/j.nutres.2013.07.021
183. Cutillo, G, Saariaho, A-H, and Meri, S. Physiology of gangliosides and the role of antiganglioside antibodies in human diseases. Cell Mol Immunol. (2020) 17:313–22. doi: 10.1038/s41423-020-0388-9
184. Zheng, L, Fleith, M, Giuffrida, F, O’Neill, BV, and Schneider, N. Dietary polar lipids and cognitive development: a narrative review. Adv Nutr. (2019) 10:1163–76. doi: 10.1093/advances/nmz051
185. Bashiri, Z, Sheibak, N, Amjadi, F, and Zandieh, Z. The role of myo-inositol supplement in assisted reproductive techniques. Hum Fertil. (2022):1–17. doi: 10.1080/14647273.2022.2073273 [Epub ahead of print]
186. Awuchi, CG, and Echeta, KC. Current developments in sugar alcohols: chemistry, nutrition, and health concerns of sorbitol, xylitol, glycerol, arabitol, inositol, maltitol, and lactitol. Int J Adv Acad Res. (2019) 5:1–33.
187. Wu, R, Chen, J, Zhang, L, Wang, X, Yang, Y, and Ren, X. LC/MS-based metabolomics to evaluate the milk composition of human, horse, goat and cow from China. Eur Food Res Technol. (2021) 247:663–75. doi: 10.1007/s00217-020-03654-1
188. Howlett, A, Ohlsson, A, and Plakkal, N. Inositol in preterm infants at risk for or having respiratory distress syndrome. Cochrane Database Syst Rev. (2015):CD000366. doi: 10.1002/14651858.CD000366.pub3
189. Cavalli, P, and Ronda, E. Myoinositol: the bridge (PONTI) to reach a healthy pregnancy. Int J Endocrinol. (2017) 2017:1–5. doi: 10.1155/2017/5846286
190. He, X, Sotelo-Orozco, J, Rudolph, C, Lönnerdal, B, and Slupsky, CM. The role of protein and free amino acids on intake, metabolism, and gut microbiome: a comparison between breast-fed and formula-fed rhesus monkey infants. Front Pediatr. (2020) 7:563. doi: 10.3389/fped.2019.00563
191. Rafiee-Tari, N, Fan, MZ, Archbold, T, Arranz, E, and Corredig, M. Effect of milk protein composition and amount of β-casein on growth performance, gut hormones, and inflammatory cytokines in an in vivo piglet model. J Dairy Sci. (2019) 102:8604–13. doi: 10.3168/jds.2018-15786
192. O’Mahony, SM, McVey Neufeld, K, Waworuntu, RV, Pusceddu, MM, Manurung, S, Murphy, K, et al. The enduring effects of early-life stress on the microbiota–gut–brain axis are buffered by dietary supplementation with milk fat globule membrane and a prebiotic blend. Eur J Neurosci. (2020) 51:1042–58. doi: 10.1111/ejn.14514
193. Fleming, SA, Monaikul, S, Patsavas, AJ, Waworuntu, RV, Berg, BM, and Dilger, RN. Dietary polydextrose and galactooligosaccharide increase exploratory behavior, improve recognition memory, and alter neurochemistry in the young pig. Nutr Neurosci. (2019) 22:499–512. doi: 10.1080/1028415X.2017.1415280
194. Aasmul-Olsen, K, Henriksen, NL, Nguyen, DN, Heckmann, AB, Thymann, T, Sangild, PT, et al. Milk Osteopontin for gut, immunity and brain development in preterm pigs. Nutrients. (2021) 13:2675. doi: 10.3390/nu13082675
195. Fleddermann, M, Demmelmair, H, Hellmuth, C, Grote, V, Trisic, B, Nikolic, T, et al. Association of infant formula composition and anthropometry at 4 years: follow-up of a randomized controlled trial (BeMIM study). PLoS One. (2018) 13:e0199859. doi: 10.1371/journal.pone.0199859
196. Oropeza-Ceja, LG, Rosado, JL, Ronquillo, D, García, OP, Caamaño, M, García-Ugalde, C, et al. Lower protein intake supports normal growth of full-term infants fed formula: a randomized controlled trial. Nutrients. (2018) 10:886. doi: 10.3390/nu10070886
197. Foiles, AM, Kerling, EH, Wick, JA, Scalabrin, DMF, Colombo, J, and Carlson, SE. Formula with long-chain polyunsaturated fatty acids reduces incidence of allergy in early childhood. Pediatr Allergy Immunol. (2016) 27:156–61. doi: 10.1111/pai.12515
198. Lapillonne, A, Pastor, N, Zhuang, W, and Scalabrin, DMF. Infants fed formula with added long chain polyunsaturated fatty acids have reduced incidence of respiratory illnesses and diarrhea during the first year of life. BMC Pediatr. (2014) 14:1–8. doi: 10.1186/1471-2431-14-168
199. Miklavcic, JJ, Larsen, BMK, Mazurak, VC, Scalabrin, DMF, MacDonald, IM, Shoemaker, GK, et al. Reduction of arachidonate is associated with increase in B-cell activation marker in infants: a randomized trial. J Pediatr Gastroenterol Nutr. (2017) 64:446–53. doi: 10.1097/MPG.0000000000001283
200. Timby, N, Hernell, O, Vaarala, O, Melin, M, Lönnerdal, B, and Domellöf, M. Infections in infants fed formula supplemented with bovine milk fat globule membranes. J Pediatr Gastroenterol Nutr. (2015) 60:384–9. doi: 10.1097/MPG.0000000000000624
201. Rubin, LP, Chan, GM, Barrett-Reis, BM, Fulton, AB, Hansen, RM, Ashmeade, TL, et al. Effect of carotenoid supplementation on plasma carotenoids, inflammation and visual development in preterm infants. J Perinatol. (2012) 32:418–24. doi: 10.1038/jp.2011.87
202. Nieto-Ruiz, A, García-Santos, JA, Bermúdez, MG, Herrmann, F, Diéguez, E, Sepúlveda-Valbuena, N, et al. Cortical visual evoked potentials and growth in infants fed with bioactive compounds-enriched infant formula: results from COGNIS randomized clinical trial. Nutrients. (2019) 11:2456. doi: 10.3390/nu11102456
203. Spalinger, J, Nydegger, A, Belli, D, Furlano, RI, Yan, J, Tanguy, J, et al. Growth of infants fed formula with evolving nutrition composition: a single-arm non-inferiority study. Nutrients. (2017) 9:219. doi: 10.3390/nu9030219
204. Rodriguez-Herrera, A, Tims, S, Polman, J, Porcel Rubio, R, Muñoz Hoyos, A, Agosti, M, et al. Early-life fecal microbiome and metabolome dynamics in response to an intervention with infant formula containing specific prebiotics and postbiotics. Am J Physiol Gastroint Liver Physiol. (2022) 322:G571–82. doi: 10.1152/ajpgi.00079.2021
205. Motoki, N, Mizuki, M, Tsukahara, T, Miyakawa, M, Kubo, S, Oda, H, et al. Effects of lactoferrin-fortified formula on acute gastrointestinal symptoms in children aged 12–32 months: a randomized, double-blind, placebo-controlled trial. Front Pediatr. (2020) 8:233. doi: 10.3389/fped.2020.00233
206. Chen, K, Chai, L, Li, H, Zhang, Y, Xie, H-M, Shang, J, et al. Effect of bovine lactoferrin from iron-fortified formulas on diarrhea and respiratory tract infections of weaned infants in a randomized controlled trial. Nutrition. (2016) 32:222–7. doi: 10.1016/j.nut.2015.08.010
207. Li, F, Wu, SS, Berseth, CL, Harris, CL, Richards, JD, Wampler, JL, et al. Improved neurodevelopmental outcomes associated with bovine milk fat globule membrane and lactoferrin in infant formula: a randomized, controlled trial. J Pediatr. (2019) 215:24–31. doi: 10.1016/j.jpeds.2019.08.030
208. Lönnerdal, B, Kvistgaard, AS, Peerson, JM, Donovan, SM, and Peng, Y. Growth, nutrition, and cytokine response of breast-fed infants and infants fed formula with added bovine osteopontin. J Pediatr Gastroenterol Nutr. (2016) 62:650–7. doi: 10.1097/MPG.0000000000001005
209. Bernhard, W, Böckmann, K, Maas, C, Mathes, M, Hövelmann, J, Shunova, A, et al. Combined choline and DHA supplementation: a randomized controlled trial. Eur J Nutr. (2020) 59:729–39. doi: 10.1007/s00394-019-01940-7
210. Gurnida, DA, Rowan, AM, Idjradinata, P, Muchtadi, D, and Sekarwana, N. Association of complex lipids containing gangliosides with cognitive development of 6-month-old infants. Early Hum Dev. (2012) 88:595–601. doi: 10.1016/j.earlhumdev.2012.01.003
211. Seong, S-H, Cho, S-C, Park, Y, and Cha, Y-S. L-carnitine-supplemented parenteral nutrition improves fat metabolism but fails to support compensatory growth in premature Korean infants. Nutr Res. (2010) 30:233–9. doi: 10.1016/j.nutres.2010.04.004
212. Francisquini JANunes, L, Martins, E, Stephani, R, Perrone, ÍT, and Carvalho, AF. How the heat treatment affects the constituents of infant formulas: a review. Brazilian J Food Technol. (2020) 23:27219. doi: 10.1590/1981-6723.27219
213. Jiang, YJ. Processing Technology for Infant Formula In: M Guo, editor. Human milk biochemistry and infant formula manufacturing technology. Cambridge, UK: Woodhead (2014). 211–29.
214. Schmitz-Schug, I, Kulozik, U, and Foerst, P. Modeling spray drying of dairy products–impact of drying kinetics, reaction kinetics and spray drying conditions on lysine loss. Chem Eng Sci. (2016) 141:315–29. doi: 10.1016/j.ces.2015.11.008
215. Lund, P, Nielsen, SB, Nielsen, CF, Ray, CA, and Lund, MN. Impact of UHT treatment and storage on liquid infant formula: complex structural changes uncovered by centrifugal field-flow fractionation with multi-angle light scattering. Food Chem. (2021) 348:129145. doi: 10.1016/j.foodchem.2021.129145
216. Varghese, KS, Pandey, MC, Radhakrishna, K, and Bawa, AS. Technology, applications and modelling of ohmic heating: a review. J Food Sci Technol. (2014) 51:2304–17. doi: 10.1007/s13197-012-0710-3
217. Jiang, SL, and Guo, MR. Processing technology for infant formula In: Human milk biochemistry and infant formula manufacturing technology. ed. M. Guo (Amsterdam: Elsevier) (2021). 223–40.
218. Lafarga, T, Queralt, AV, Bobo, G, Abadias, M, and Aguiló-Aguayo, I. “Thermal processing technologies,” in Food Formulation: Novel Ingredients and Processing Techniques. eds. (2021) 165–81. doi: 10.1002/9781119614760.ch9
219. Murphy, EG, Regost, NE, Roos, YH, and Fenelon, MA. Powder and reconstituted properties of commercial infant and follow-on formulas. Foods. (2020) 9:84. doi: 10.3390/foods9010084
220. Jo, MG, An, DS, and Lee, DS. Characterization and enhancement of package O2 barrier against oxidative deterioration of powdered infant formula. Korean J Packag Sci Technol. (2018) 16:24–13. doi: 10.20909/kopast.2018.24.1.13
221. Song, X, Shukla, S, and Kim, M. Detection of Cronobacter species in powdered infant formula using immunoliposome-based immunomagnetic concentration and separation assay. Food Microbiol. (2018) 72:23–30. doi: 10.1016/j.fm.2017.11.002
222. Guo, M, and Ahmad, S. Formulation guidelines for infant formula In: Human milk biochemistry and infant formula manufacturing technology. ed. M. Guo (Amsterdam: Elsevier) (2014). 141–71.
223. Happe, RP, and Gambelli, L. Infant formula In: Specialty oils and fats in food and nutrition. ed. G. Talbot (Amsterdam: Elsevier) (2015). 285–315.
224. Blanchard, E, Zhu, P, and Schuck, P. Infant formula powders In: Handbook of food powders. eds. B. Bhandari, N. Bansal, M. Zhang, and P. Schuck (Amsterdam: Elsevier) (2013). 465–83.
225. McCarthy, NA, Magan, JB, Kelleher, CM, Kelly, AL, O’Mahony, JA, and Murphy, EG. Heat treatment of milk: effect on concentrate viscosity, powder manufacture and end-product functionality. Int Dairy J. (2022) 128:105289. doi: 10.1016/j.idairyj.2021.105289
226. Arzuaga, MR, Aalaei, K, da Silva, DF, Barjon, S, Añón, MC, Abraham, AG, et al. Infant milk formulae processing: effect of wet-mix total solids and heat treatment temperature on rheological, emulsifying and nutritional properties. J Food Eng. (2021) 290:110194. doi: 10.1016/j.jfoodeng.2020.110194
227. Buggy, AK, McManus, JJ, Brodkorb, A, Carthy, NM, and Fenelon, MA. Stabilising effect of α-lactalbumin on concentrated infant milk formula emulsions heat treated pre-or post-homogenisation. Dairy Sci Technol. (2017) 96:845–59. doi: 10.1007/s13594-016-0306-1
228. Bourlieu, C, Bouzerzour, K, Ferret-Bernard, S, Le Bourgot, C, Chever, S, Menard, O, et al. Infant formula interface and fat source impact on neonatal digestion and gut microbiota. Eur J Lipid Sci Technol. (2015) 117:1500–12. doi: 10.1002/ejlt.201500025
229. Masum, AKM, Chandrapala, J, Huppertz, T, Adhikari, B, and Zisu, B. Influence of drying temperatures and storage parameters on the physicochemical properties of spray-dried infant milk formula powders. Int Dairy J. (2020) 105:104696. doi: 10.1016/j.idairyj.2020.104696
230. Lang, E, and Sant’Ana, AS. Microbial contaminants in powdered infant formula: what is the impact of spray-drying on microbial inactivation? Curr Opin Food Sci. (2021) 42:195–202. doi: 10.1016/j.cofs.2021.06.007
231. Nunes, L, Martins, E, Perrone, IT, and de Carvalho, AF. The Maillard reaction in powdered infant formula. J Food Nutr Res. (2019) 7:33–40. doi: 10.12691/jfnr-7-1-5
232. Keshani, S, Daud, WRW, Nourouzi, MM, Namvar, F, and Ghasemi, M. Spray drying: An overview on wall deposition, process and modeling. J Food Eng. (2015) 146:152–62. doi: 10.1016/j.jfoodeng.2014.09.004
233. Zhang, S, Liu, L, Li, B, Xie, Y, Ouyang, J, and Wu, Y. Concentrations of migrated mineral oil/polyolefin oligomeric saturated hydrocarbons (MOSH/POSH) in Chinese commercial milk powder products. Food Add Contamin. (2019) 36:1261–72. doi: 10.1080/19440049.2019.1627001
234. An, DS, Wang, HJ, Jaisan, C, Lee, JH, Jo, MG, and Lee, DS. Effects of modified atmosphere packaging conditions on quality preservation of powdered infant formula. Packag Technol Sci. (2018) 31:441–6. doi: 10.1002/pts.2372
235. Wang, HJ, An, DS, and Lee, DS. A model to tune modified atmosphere conditions of powdered infant formula packaging. J Food Process Eng. (2017) 40:e12380. doi: 10.1111/jfpe.12380
236. Lee, DS. Antioxidative packaging system In: Innovations in food packaging. ed. J. H. Han (Amsterdam: Elsevier) (2014). 111–31.
237. Jo, MG, An, DS, and Lee, DS. Antioxidant packaging as additional measure to augment CO2-enriched modified atmosphere packaging for preserving infant formula powder. Pope John XXI. (2020) 26:19–23. doi: 10.20909/kopast.2020.26.1.19
238. Seo, CW, Hong, S, Shin, YK, and Kang, SH. Physicochemical properties of liquid infant formula stored at different temperatures. Korean J Food Sci Anim Resour. (2018) 38:995. doi: 10.5851/kosfa.2018.e31
239. Ye, Y, Engholm-Keller, K, Fang, Y, Nielsen, CF, Jordà, A, Lund, MN, et al. UHT treatment and storage of liquid infant formula affects protein digestion and release of bioactive peptides. Food Funct. (2022) 13:344–55. doi: 10.1039/D1FO02619D
240. Sun, X, Wang, C, Wang, H, and Guo, M. Effects of processing on structure and thermal properties of powdered preterm infant formula. J Food Sci. (2018) 83:1685–94. doi: 10.1111/1750-3841.14162
241. Brodkorb, A, Croguennec, T, Bouhallab, S, and Kehoe, JJ. “Heat-induced denaturation, aggregation and gelation of whey proteins,” in Advanced Dairy Chemistry. eds. P. McSweeney and J. O’Mahony (New York, NY: Springer) (2016) 155–78.
242. Joyce, AM, Brodkorb, A, Kelly, AL, and O’Mahony, JA. Separation of the effects of denaturation and aggregation on whey-casein protein interactions during the manufacture of a model infant formula. Dairy Sci Technol. (2017) 96:787–806. doi: 10.1007/s13594-016-0303-4
243. Qian, F, Sun, J, Cao, D, Tuo, Y, Jiang, S, and Mu, G. Experimental and Modelling study of the denaturation of Milk protein by heat treatment. Korean J Food Sci Anim Resour. (2017) 37:44–51. doi: 10.5851/kosfa.2017.37.1.44
244. de Souza, AB, Costa, LCG Jr, Stephani, R, de Oliveira, MAL, Perrone, ÍT, and Costa, RGB. Evaluation of the viscosity profile obtained for dispersions containing different proportions of milk protein concentrate/whey protein concentrate during simulated conditions of thermal processing. LWT Food Sci Technol. (2015) 64:536–9. doi: 10.1016/j.lwt.2015.05.058
245. Borad, SG, Kumar, A, and Singh, AK. Effect of processing on nutritive values of milk protein. Crit Rev Food Sci Nutr. (2017) 57:3690–702. doi: 10.1080/10408398.2016.1160361
246. Fox, PF, Uniacke-Lowe, T, McSweeney, PLH, O’Mahony, JA, Fox, PF, Uniacke-Lowe, T, et al. “Heat-induced changes in milk,” in Dairy Chemistry and Biochemistry. eds. P. F. Fox, T. Uniacke-Lowe, P. L. H. McSweeney, and J. A. O’Mahony (Cham.: Springer). (2015) 345–75.
247. Lönnerdal, B, Erdmann, P, Thakkar, SK, Sauser, J, and Destaillats, F. Longitudinal evolution of true protein, amino acids and bioactive proteins in breast milk: a developmental perspective. J Nutr Biochem. (2017) 41:1–11. doi: 10.1093/jn/133.5.1490S
248. Golkar, A, Milani, JM, and Vasiljevic, T. Altering allergenicity of cow’s milk by food processing for applications in infant formula. Crit Rev Food Sci Nutr. (2019) 59:159–72. doi: 10.1080/10408398.2017.1363156
249. Golinelli, LP, Del Aguila, EM, Paschoalin, VMF, Silva, JT, and Conte-Junior, CA. Functional aspect of colostrum and whey proteins in human milk. J Hum Nutr Food Sci. (2014) 2:1035.
250. Rafe, A, and Razavi, SMA. Effect of thermal treatment on chemical structure of β-lactoglobulin and basil seed gum mixture at different states by ATR-FTIR spectroscopy. Int J Food Prop. (2015) 18:2652–64. doi: 10.1080/10942912.2014.999864
251. Mehta, BM, and Deeth, HC. Blocked lysine in dairy products: formation, occurrence, analysis, and nutritional implications. Compr Rev Food Sci Food Saf. (2016) 15:206–18. doi: 10.1111/1541-4337.12178
252. Kamdem, JP, and Tsopmo, A. Reactivity of peptides within the food matrix. Food Biochem. (2019) 43:e12489. doi: 10.1111/jfbc.12489
253. Zenker, HE, van Lieshout, GAA, van Gool, MP, Bragt, MCE, and Hettinga, KA. Lysine blockage of milk proteins in infant formula impairs overall protein digestibility and peptide release. Food Funct. (2020) 11:358–69. doi: 10.1039/C9FO02097G
254. Chávez-Servín, JL, de la Torre Carbot, K, García-Gasca, T, Castellote, AI, and López-Sabater, MC. Content and evolution of potential furfural compounds in commercial milk-based infant formula powder after opening the packet. Food Chem. (2015) 166:486–91. doi: 10.1016/j.foodchem.2014.06.050
255. Shen, Z, Ma, X, Ali, MM, Liang, J, and Du, Z. Analysis of the evolution of potential and free furfural compounds in the production chain of infant formula and risk assessment. Food Chem. (2022) 368:130814. doi: 10.1016/j.foodchem.2021.130814
256. Elmhiri, G, Mahmood, DFD, Niquet-Leridon, C, Jacolot, P, Firmin, S, Guigand, L, et al. Formula-derived advanced glycation end products are involved in the development of long-term inflammation and oxidative stress in kidney of IUGR piglets. Mol Nutr Food Res. (2015) 59:939–47. doi: 10.1002/mnfr.201400722
257. Singh, K, Tripathi, S, and Chandra, R. Maillard reaction product and its complexation with environmental pollutants: a comprehensive review of their synthesis and impact. Bioresour Technol Rep. (2021) 15:100779. doi: 10.1016/j.biteb.2021.100779
258. Rannou, C, Laroque, D, Renault, E, Prost, C, and Sérot, T. Mitigation strategies of acrylamide, furans, heterocyclic amines and browning during the Maillard reaction in foods. Food Res Int. (2016) 90:154–76. doi: 10.1016/j.foodres.2016.10.037
259. Roncero-Ramos, I, Pastoriza, S, Navarro, MP, and Delgado-Andrade, C. Assessing the effects of model Maillard compound intake on iron, copper and zinc retention and tissue delivery in adult rats. Food Funct. (2016) 7:164–70. doi: 10.1039/C5FO00790A
260. Yang, H, Bai, X, Feng, B, Wang, Q, Meng, L, Wang, F, et al. Application of molecular transformer approach for predicting the potential reactions to generate advanced glycation end products in infant formula. Food Chem. (2023) 407:135143. doi: 10.1016/j.foodchem.2022.135143
261. Liu, Z, Roy, NC, Guo, Y, Jia, H, Ryan, L, Samuelsson, L, et al. Human breast Milk and infant formulas differentially modify the intestinal microbiota in human infants and host physiology in rats. J Nutr. (2016) 146:191–9. doi: 10.3945/jn.115.223552
262. Lopez, C, Cauty, C, and Guyomarc’h, F. Organization of lipids in milks, infant milk formulas and various dairy products: role of technological processes and potential impacts. Dairy Sci Technol. (2015) 95:863–93. doi: 10.1007/s13594-015-0263-0
263. Cilla, A, Diego Quintaes, K, Barberá, R, and Alegría, A. Phospholipids in human milk and infant formulas: benefits and needs for correct infant nutrition. Crit Rev Food Sci Nutr. (2016) 56:1880–92. doi: 10.1080/10408398.2013.803951
264. Kilic-Akyilmaz, M, Ozer, B, Bulat, T, and Topcu, A. Effect of heat treatment on micronutrients, fatty acids and some bioactive components of milk. Int Dairy J. (2022) 126:105231. doi: 10.1016/j.idairyj.2021.105231
265. Nooshkam, M, and Madadlou, A. Maillard conjugation of lactulose with potentially bioactive peptides. Food Chem. (2016) 192:831–6. doi: 10.1016/j.foodchem.2015.07.094
266. Borschel, MW. “Vitamin B-6 in infancy: requirements and current feeding practices,” in Vitamin B-6 Metabol Pregnancy Lactat Infancy. ed. D. J. Raiten (CRC Press) (2021):109–24. doi: 10.1201/9781003210368-7
267. Huppertz, T, and Nieuwenhuijse, H. Constituent fouling during heat treatment of milk: a review. Int Dairy J. (2022) 126:105236. doi: 10.1016/j.idairyj.2021.105236
268. Peixoto, RRA, Fernández-Menéndez, S, Fernández-Colomer, B, Vaquero, AIP, Cadore, S, Sanz-Medel, A, et al. Impact of holder pasteurization on essential elements from human donor milk: Total contents and protein-binding profiles. J Food Compos Anal. (2020) 87:103395. doi: 10.1016/j.jfca.2019.103395
269. Shabbir, MA, Ahmed, H, Maan, AA, Rehman, A, Afraz, MT, Iqbal, MW, et al. Effect of non-thermal processing techniques on pathogenic and spoilage microorganisms of milk and milk products. Food Sci Technol. (2020) 41:279–94. doi: 10.1590/fst.05820
270. Umair, M, Jabbar, S, Ayub, Z, Muhammad Aadil, R, Abid, M, Zhang, J, et al. Recent advances in plasma technology: influence of atmospheric cold plasma on spore inactivation. Food Rev Intl. (2022) 38:789–811. doi: 10.1080/87559129.2021.1888972
271. Pina-Pérez, MC, Rodrigo, D, and Martínez, A. Nonthermal inactivation of Cronobacter sakazakii in infant formula milk: a review. Crit Rev Food Sci Nutr. (2016) 56:1620–9. doi: 10.1080/10408398.2013.781991
272. Cebrián, G, Mañas, P, and Condón, S. Comparative resistance of bacterial foodborne pathogens to non-thermal technologies for food preservation. Front Microbiol. (2016) 7:734. doi: 10.3389/fmicb.2016.00734
273. Aaliya, B, Valiyapeediyekkal Sunooj, K, Navaf, M, Parambil Akhila, P, Sudheesh, C, Ahmad Mir, S, et al. Recent trends in bacterial decontamination of food products by hurdle technology: a synergistic approach using thermal and non-thermal processing techniques. Food Res Int. (2021) 147:110514. doi: 10.1016/j.foodres.2021.110514
274. Wang, C-Y, Huang, H-W, Hsu, C-P, and Yang, BB. Recent advances in food processing using high hydrostatic pressure technology. Crit Rev Food Sci Nutr. (2016) 56:527–40. doi: 10.1080/10408398.2012.745479
275. Ray, PR, Haldar, L, Sen, C, and Bhattacharyya, M. Decontamination of Milk and Milk products In: Microbial decontamination of food. eds. A. Demirci and M. O. Ngadi (Berlin: Springer) (2022). 259–75.
276. Alirezalu, K, Munekata, PES, Parniakov, O, Barba, FJ, Witt, J, Toepfl, S, et al. Pulsed electric field and mild heating for milk processing: a review on recent advances. J Sci Food Agric. (2020) 100:16–24. doi: 10.1002/jsfa.9942
277. Sharma, P, Bremer, P, Oey, I, and Everett, DW. Bacterial inactivation in whole milk using pulsed electric field processing. Int Dairy J. (2014) 35:49–56. doi: 10.1016/j.idairyj.2013.10.005
278. Pal, M, Ayele, Y, Hadush, A, Kundu, P, and Jadhav, VJ. (2017). Public Health Significance of Foodborne Helminthiasis: A Systematic Review. J Exp Food Chem. 4:135. doi: 10.4172/2472-0542.1000135
279. Balaji, AS, Allahdad, Z, and Lacroix, M. Effect of γ-irradiation in combination with natural antimicrobial formulation on microbial inactivation, protein digestibility and quality of mothers’ milk. Int Dairy J. (2022) 131:105386. doi: 10.1016/j.idairyj.2022.105386
280. Tesfai, A, Beamer, SK, Matak, KE, and Jaczynski, J. Effect of electron beam on chemical changes of nutrients in infant formula. Food Chem. (2014) 149:208–14. doi: 10.1016/j.foodchem.2013.10.110
281. Kalaiselvan, RR, Sugumar, A, and Radhakrishnan, M. Gamma irradiation usage in fruit juice extraction In: Fruit juices. eds. G. Rajauria and B. K. Tiwari (Amsterdam: Elsevier) (2018) 423–35.
282. Xie, L, Solhaug, KA, Song, Y, Brede, DA, Lind, OC, Salbu, B, et al. Modes of action and adverse effects of gamma radiation in an aquatic macrophyte Lemna minor. Sci Total Environ. (2019) 680:23–34. doi: 10.1016/j.scitotenv.2019.05.016
283. Munir, MT, and Federighi, M. Control of foodborne biological hazards by ionizing radiations. Foods. (2020) 9:878. doi: 10.3390/foods9070878
284. Robichaud, V, Bagheri, L, Aguilar-Uscanga, BR, Millette, M, and Lacroix, M. Effect of ɣ-irradiation on the microbial inactivation, nutritional value, and antioxidant activities of infant formula. LWT. (2020) 125:109211. doi: 10.1016/j.lwt.2020.109211
285. Robichaud, V, Bagheri, L, Salmieri, S, Aguilar-Uscanga, BR, Millette, M, and Lacroix, M. Effect of γ-irradiation and food additives on the microbial inactivation of foodborne pathogens in infant formula. LWT. (2021) 139:110547. doi: 10.1016/j.lwt.2020.110547
286. Joshua, AO. An overview of irradiation as a food preservation technique. Novel Res Microbiol J. (2020) 4:779–89. doi: 10.21608/nrmj.2020.95321
287. McFadden, E, Costa Ramos, A-L, Bradley, D, Vrain, O, McEvoy, B, and Rowan, NJ. Comparative studies on the novel sterilisation of Irish retailed infant milk formula using electron beam and pulsed light treatments. International Journal of Science, Environment and Technology (2016) 5:4375–4377. Available at: http://research.thea.ie/handle/20.500.12065/3297
288. Jemii, E, and Alharbi, T. Measurements of natural radioactivity in infant formula and radiological risk assessment. J Radioanal Nucl Chem. (2018) 315:157–61. doi: 10.1007/s10967-017-5646-7
289. Sarangapani, C, Patange, A, Bourke, P, Keener, K, and Cullen, PJ. Recent advances in the application of cold plasma technology in foods. Annu Rev Food Sci Technol. (2018) 9:609–29. doi: 10.1146/annurev-food-030117-012517
290. Tolouie, H, Mohammadifar, MA, Ghomi, H, and Hashemi, M. Cold atmospheric plasma manipulation of proteins in food systems. Crit Rev Food Sci Nutr. (2018) 58:2583–97. doi: 10.1080/10408398.2017.1335689
291. Venkataratnam, H, Sarangapani, C, Cahill, O, and Ryan, CB. Effect of cold plasma treatment on the antigenicity of peanut allergen Ara h 1. Innovative Food Sci Emerg Technol. (2019) 52:368–75. doi: 10.1016/j.ifset.2019.02.001
292. Misra, NN, Kaur, S, Tiwari, BK, Kaur, A, Singh, N, and Cullen, PJ. Atmospheric pressure cold plasma (ACP) treatment of wheat flour. Food Hydrocoll. (2015) 44:115–21. doi: 10.1016/j.foodhyd.2014.08.019
293. Thirumdas, R, Trimukhe, A, Deshmukh, RR, and Annapure, US. Functional and rheological properties of cold plasma treated rice starch. Carbohydr Polym. (2017) 157:1723–31. doi: 10.1016/j.carbpol.2016.11.050
294. Segat, A, Misra, NN, Cullen, PJ, and Innocente, N. Atmospheric pressure cold plasma (ACP) treatment of whey protein isolate model solution. Innov Food Sci Emerg Technol. (2015) 29:247–54. doi: 10.1016/j.ifset.2015.03.014
295. Gao, Y, Zhuang, H, Yeh, H-Y, Bowker, B, and Zhang, J. Effect of rosemary extract on microbial growth, pH, color, and lipid oxidation in cold plasma-processed ground chicken patties. Innov Food Sci Emerg Technol. (2019) 57:102168. doi: 10.1016/j.ifset.2019.05.007
296. Gutiérrez, D, Rodríguez-Rubio, L, Martínez, B, Rodríguez, A, and García, P. Bacteriophages as weapons against bacterial biofilms in the food industry. Front Microbiol. (2016) 7:825. doi: 10.3389/fmicb.2016.00825
297. Kim, HS, Ashrafudoulla, M, Kim, B-R, Mizan, MFR, Jung, S-J, Sadekuzzaman, M, et al. The application of bacteriophage to control Cronobacter sakazakii planktonic and biofilm growth in infant formula milk. Biofouling. (2021) 37:606–14. doi: 10.1080/08927014.2021.1943741
298. Callanan, M, Paes, M, Iversen, C, Kleijn, R, Almeida, CB, Peñaloza, W, et al. Behavior of Enterobacter pulveris in amorphous and crystalline powder matrices treated with supercritical carbon dioxide. J Dairy Sci. (2012) 95:6300–6. doi: 10.3168/jds.2012-5541
299. Kim, SA, Kim, OY, and Rhee, MS. Direct application of supercritical carbon dioxide for the reduction of Cronobacter spp.(Enterobacter sakazakii) in end products of dehydrated powdered infant formula. J Dairy Sci. (2010) 93:1854–60. doi: 10.3168/jds.2009-2738
300. Arroyo, C, Dorozko, A, Gaston, E, O’Sullivan, M, Whyte, P, and Lyng, JG. Light based technologies for microbial inactivation of liquids, bead surfaces and powdered infant formula. Food Microbiol. (2017) 67:49–57. doi: 10.1016/j.fm.2017.06.001
301. Cetin-Karaca, H, and Morgan, MC. Antimicrobial efficacy of cinnamaldehyde, chitosan and high pressure processing against Cronobacter sakazakii in infant formula. J Food Saf. (2020) 40:e12845. doi: 10.1111/jfs.12845
302. Wazed, MA, and Farid, M. Hypoallergenic and low-protein ready-to-feed (rtf) infant formula by high pressure pasteurization: a novel product. Foods. (2019) 8:408. doi: 10.3390/foods8090408
303. Cetin-Karaca, H, and Morgan, MC. Inactivation of Bacillus cereus spores in infant formula by combination of high pressure and trans-cinnamaldehyde. LWT. (2018) 97:254–60. doi: 10.1016/j.lwt.2018.07.001
304. Chen, D, Peng, P, Zhou, N, Cheng, Y, Min, M, Ma, Y, et al. Evaluation of Cronobacter sakazakii inactivation and physicochemical property changes of non-fat dry milk powder by cold atmospheric plasma. Food Chem. (2019) 290:270–6. doi: 10.1016/j.foodchem.2019.03.149
305. Oh, YJ, Lee, H, Kim, JE, Lee, SH, Cho, HY, and Min, SC. Cold plasma treatment application to improve microbiological safety of infant milk powder and onion powder. Korean J Food Sci Technol. (2015) 47:486–91. doi: 10.9721/KJFST.2015.47.4.486
306. Zheng, Z, Xie, Y, Ma, S, Tu, J, Li, J, Liang, S, et al. Effect of 405-nm light-emitting diode on environmental tolerance of Cronobacter sakazakii in powdered infant formula. Food Res Int. (2021) 144:110343. doi: 10.1016/j.foodres.2021.110343
307. Korpela, K. Impact of delivery mode on infant gut microbiota. Ann Nutr Metab. (2021) 77:11–9. doi: 10.1159/000518498
308. Lemaire, M, Le Huërou-Luron, I, and Blat, S. Effects of infant formula composition on long-term metabolic health. J Dev Orig Health Dis. (2018) 9:573–89. doi: 10.1017/S2040174417000964
309. Abenavoli, L, Scarpellini, E, Colica, C, Boccuto, L, Salehi, B, Sharifi-Rad, J, et al. Gut microbiota and obesity: a role for probiotics. Nutrients. (2019) 11:2690. doi: 10.3390/nu11112690
310. Goldsmith, F, O’Sullivan, A, Smilowitz, JT, and Freeman, SL. Lactation and intestinal microbiota: how early diet shapes the infant gut. J Mammary Gland Biol Neoplasia. (2015) 20:149–58. doi: 10.1007/s10911-015-9335-2
311. Ahlawat, S, and Sharma, KK. Gut–organ axis: a microbial outreach and networking. Lett Appl Microbiol. (2021) 72:636–68. doi: 10.1111/lam.13333
312. Li, C, Chen, J, and Li, SC. Understanding horizontal gene transfer network in human gut microbiota. Gut Pathog. (2020) 12:1–20. doi: 10.1186/s13099-020-00370-9
313. Voreades, N, Kozil, A, and Weir, TL. Diet and the development of the human intestinal microbiome. Front Microbiol. (2014) 5:494. doi: 10.3389/fmicb.2014.00494
314. Cong, X, Xu, W, Romisher, R, Poveda, S, Forte, S, Starkweather, A, et al. Focus: microbiome: gut microbiome and infant health: brain-gut-microbiota axis and host genetic factors. Yale J Biol Med. (2016) 89:299–308.
315. Duca, FA, and Lam, TKT. Gut microbiota, nutrient sensing and energy balance. Diabetes Obes Metab. (2014) 16:68–76. doi: 10.1111/dom.12340
316. Fabiano, V, Indrio, F, Verduci, E, Calcaterra, V, Pop, TL, Mari, A, et al. Term infant formulas influencing gut microbiota: an overview. Nutrients. (2021) 13:4200. doi: 10.3390/nu13124200
317. Pekmez, CT, Dragsted, LO, and Brahe, LK. Gut microbiota alterations and dietary modulation in childhood malnutrition – the role of short chain fatty acids. Clin Nutr. (2019) 38:615–30. doi: 10.1016/j.clnu.2018.02.014
318. Charton, E, Bourgeois, A, Bellanger, A, Le-Gouar, Y, Dahirel, P, Romé, V, et al. Infant nutrition affects the microbiota-gut-brain axis: comparison of human milk vs. infant formula feeding in the piglet model. Front Nutr. (2022) 9:9. doi: 10.3389/fnut.2022.976042
319. Pham, VT, Lacroix, C, Braegger, CP, and Chassard, C. Early colonization of functional groups of microbes in the infant gut. Environ Microbiol. (2016) 18:2246–58. doi: 10.1111/1462-2920.13316
320. Bäckhed, F, Roswall, J, Peng, Y, Feng, Q, Jia, H, Kovatcheva-Datchary, P, et al. Dynamics and stabilization of the human gut microbiome during the first year of life. Cell Host Microbe. (2015) 17:852. doi: 10.1016/j.chom.2015.05.012
321. Bokulich, NA, Chung, J, Battaglia, T, Henderson, N, Jay, M, Li, H, et al. Antibiotics, birth mode, and diet shape microbiome maturation during early life. Sci Transl Med. (2016) 8:343ra82–2. doi: 10.1126/scitranslmed.aad7121
322. Ho, NT, Li, F, Lee-Sarwar, KA, Tun, HM, Brown, BP, Pannaraj, PS, et al. Meta-analysis of effects of exclusive breastfeeding on infant gut microbiota across populations. Nat Commun. (2018) 9:4169. doi: 10.1038/s41467-018-06473-x
323. Martin, F-PJ, Moco, S, Montoliu, I, Collino, S, Da Silva, L, Rezzi, S, et al. Impact of breast-feeding and high-and low-protein formula on the metabolism and growth of infants from overweight and obese mothers. Pediatr Res. (2014) 75:535–43. doi: 10.1038/pr.2013.250
324. Dimitrakopoulou, EI, Pouliakis, A, Falaina, V, Xanthos, T, Zoumpoulakis, P, Tsiaka, T, et al. The metagenomic and Metabolomic profile of the infantile gut: can they be “predicted” by the feed type? Children. (2022) 9:154. doi: 10.3390/children9020154
325. Flint, HJ, and Flint, HJ. “Variability and stability of the human gut microbiome,” in Why Gut Microbes Matter. ed. H. J. Flint (Cham.: Springer). (2020):63–79. doi: 10.1007/978-3-030-43246-1_6
326. Ma, J, Li, Z, Zhang, W, Zhang, C, Zhang, Y, Mei, H, et al. Comparison of gut microbiota in exclusively breast-fed and formula-fed babies: a study of 91 term infants. Sci Rep. (2020) 10:15792. doi: 10.1038/s41598-020-72635-x
327. Thompson, AL, Monteagudo-Mera, A, Cadenas, MB, Lampl, ML, and Azcarate-Peril, MA. Milk-and solid-feeding practices and daycare attendance are associated with differences in bacterial diversity, predominant communities, and metabolic and immune function of the infant gut microbiome. Front Cell Infect Microbiol. (2015) 5:3. doi: 10.3389/fcimb.2015.00003
328. Sarkar, A, Yoo, JY, Valeria Ozorio Dutra, S, Morgan, KH, and Groer, M. The association between early-life gut microbiota and long-term health and diseases. J Clin Med. (2021) 10:459. doi: 10.3390/jcm10030459
329. Rodríguez, JM, Murphy, K, Stanton, C, Ross, RP, Kober, OI, Juge, N, et al. The composition of the gut microbiota throughout life, with an emphasis on early life. Microb Ecol Health Dis. (2015) 26:26050. doi: 10.3402/mehd.v26.26050
330. Selma-Royo, M, Calatayud Arroyo, M, García-Mantrana, I, Parra-Llorca, A, Escuriet, R, Martínez-Costa, C, et al. Perinatal environment shapes microbiota colonization and infant growth: impact on host response and intestinal function. Microbiome. (2020) 8:1–19. doi: 10.1186/s40168-020-00940-8
331. Pannaraj, PS, Li, F, Cerini, C, Bender, JM, Yang, S, Rollie, A, et al. Association between breast milk bacterial communities and establishment and development of the infant gut microbiome. JAMA Pediatr. (2017) 171:647–54. doi: 10.1001/jamapediatrics.2017.0378
332. Mathur, R, and Barlow, GM. Obesity and the microbiome. Expert Rev Gastroenterol Hepatol. (2015) 9:1087–99. doi: 10.1586/17474124.2015.1051029
333. Yang, Z, Liu, X, Wu, Y, Peng, J, and Wei, H. Effect of the microbiome on intestinal innate immune development in early life and the potential strategy of early intervention. Front Immunol. (2022) 13:3782. doi: 10.3389/fimmu.2022.936300
334. McKenzie, C, Tan, J, Macia, L, and Mackay, CR. The nutrition-gut microbiome-physiology axis and allergic diseases. Immunol Rev. (2017) 278:277–95. doi: 10.1111/imr.12556
335. Trivedi, R, and Barve, K. Gut microbiome a promising target for management of respiratory diseases. Biochem J. (2020) 477:2679–96. doi: 10.1042/BCJ20200426
336. Warner, BB, Deych, E, Zhou, Y, Hall-Moore, C, Weinstock, GM, Sodergren, E, et al. Gut bacteria dysbiosis and necrotising enterocolitis in very low birthweight infants: a prospective case-control study. Lancet. (2016) 387:1928–36. doi: 10.1016/S0140-6736(16)00081-7
337. LaTuga, MS, Stuebe, A, and Seed, PC. A review of the source and function of microbiota in breast milk. Semin Reprod Med. (2014) 32:68–73. doi: 10.1055/s-0033-1361824
338. Lewis, MC, Inman, CF, Patel, D, Schmidt, B, Mulder, I, Miller, B, et al. Direct experimental evidence that early-life farm environment influences regulation of immune responses. Pediatr Allergy Immunol. (2012) 23:265–9. doi: 10.1111/j.1399-3038.2011.01258.x
339. Inman, CF, Haverson, K, Konstantinov, SR, Jones, PH, Harris, C, Smidt, H, et al. Rearing environment affects development of the immune system in neonates. Clin Exp Immunol. (2010) 160:431–9. doi: 10.1111/j.1365-2249.2010.04090.x
340. Rzehak, P, Oddy, WH, Mearin, ML, Grote, V, Mori, TA, Szajewska, H, et al. Infant feeding and growth trajectory patterns in childhood and body composition in young adulthood. Am J Clin Nutr. (2017) 106:568–80. doi: 10.3945/ajcn.116.140962
341. Yang, I, Corwin, EJ, Brennan, PA, Jordan, S, Murphy, JR, and Dunlop, A. The infant microbiome: implications for infant health and neurocognitive development. Nurs Res. (2016) 65:76. doi: 10.1097/NNR.0000000000000133
342. Hascoët, J-M, Hubert, C, Rochat, F, Legagneur, H, Gaga, S, Emady-Azar, S, et al. Effect of formula composition on the development of infant gut microbiota. J Pediatr Gastroenterol Nutr. (2011) 52:756–62. doi: 10.1097/MPG.0b013e3182105850
343. Rosa, F, Zybailov, BL, Glazko, GV, Rahmatallah, Y, Byrum, S, Mackintosh, SG, et al. Milk formula diet alters bacterial and host protein profile in comparison to human milk diet in neonatal piglet model. Nutrients. (2021) 13:3718. doi: 10.3390/nu13113718
344. Manzoni, P. Clinical benefits of lactoferrin for infants and children. J Pediatr. (2016) 173:S43–52. doi: 10.1016/j.jpeds.2016.02.075
345. Berding, K, Wang, M, Monaco, MH, Alexander, LS, Mudd, AT, Chichlowski, M, et al. Prebiotics and bioactive milk fractions affect gut development, microbiota, and neurotransmitter expression in piglets. J Pediatr Gastroenterol Nutr. (2016) 63:688–97. doi: 10.1097/MPG.0000000000001200
346. Hu, W, Zhao, J, Wang, J, Yu, T, Wang, J, and Li, N. Transgenic milk containing recombinant human lactoferrin modulates the intestinal flora in piglets. Biochem Cell Biol. (2012) 90:485–96. doi: 10.1139/o2012-003
347. Yang, C, Zhu, X, Liu, N, Chen, Y, Gan, H, Troy, FA II, et al. Lactoferrin up-regulates intestinal gene expression of brain-derived neurotrophic factors BDNF, UCHL1 and alkaline phosphatase activity to alleviate early weaning diarrhea in postnatal piglets. J Nutr Biochem. (2014) 25:834–42. doi: 10.1016/j.jnutbio.2014.03.015
348. Gómez-Gallego, C, Collado, MC, Ilo, T, Jaakkola, U-M, Bernal, MJ, Periago, MJ, et al. Infant formula supplemented with polyamines alters the intestinal microbiota in neonatal BALB/cOlaHsd mice. J Nutr Biochem. (2012) 23:1508–13. doi: 10.1016/j.jnutbio.2011.10.003
349. Yaron, S, Shachar, D, Abramas, L, Riskin, A, Bader, D, Litmanovitz, I, et al. Effect of high β-palmitate content in infant formula on the intestinal microbiota of term infants. J Pediatr Gastroenterol Nutr. (2013) 56:376–81. doi: 10.1097/MPG.0b013e31827e1ee2
350. Nejrup, RG, Licht, TR, and Hellgren, LI. Fatty acid composition and phospholipid types used in infant formulas modifies the establishment of human gut bacteria in germ-free mice. Sci Rep. (2017) 7:1–11. doi: 10.1038/s41598-017-04298-0
351. Chen, Q, Xie, Q, Jiang, C, Evivie, SE, Cao, T, Wang, Z, et al. Infant formula supplemented with 1, 3-olein-2-palmitin regulated the immunity, gut microbiota, and metabolites of mice colonized by feces from healthy infants. J Dairy Sci. (2022) 105:6405–21. doi: 10.3168/jds.2021-21736
352. Le Huërou-Luron, I, Bouzerzour, K, Ferret-Bernard, S, Ménard, O, Le Normand, L, Perrier, C, et al. A mixture of milk and vegetable lipids in infant formula changes gut digestion, mucosal immunity and microbiota composition in neonatal piglets. Eur J Nutr. (2018) 57:463–76. doi: 10.1007/s00394-016-1329-3
353. Korpela, K, Helve, O, Kolho, K-L, Saisto, T, Skogberg, K, Dikareva, E, et al. Maternal fecal microbiota transplantation in cesarean-born infants rapidly restores normal gut microbial development: a proof-of-concept study. Cells. (2020) 183:324–34. doi: 10.1016/j.cell.2020.08.047
354. Dominguez-Bello, MG, De Jesus-Laboy, KM, Shen, N, Cox, LM, Amir, A, Gonzalez, A, et al. Partial restoration of the microbiota of cesarean-born infants via vaginal microbial transfer. Nat Med. (2016) 22:250–3. doi: 10.1038/nm.4039
355. Lemaire, M, Dou, S, Cahu, A, Formal, M, Le Normand, L, Romé, V, et al. Addition of dairy lipids and probiotic Lactobacillus fermentum in infant formula programs gut microbiota and entero-insular axis in adult minipigs. Sci Rep. (2018) 8:11656. doi: 10.1038/s41598-018-29971-w
356. Samara, J, Moossavi, S, Alshaikh, B, Ortega, VA, Pettersen, VK, Ferdous, T, et al. Supplementation with a probiotic mixture accelerates gut microbiome maturation and reduces intestinal inflammation in extremely preterm infants. Cell Host Microbe. (2022) 30:696–711. doi: 10.1016/j.chom.2022.04.005
357. Alliet, P, Vandenplas, Y, Roggero, P, Jespers, SNJ, Peeters, S, Stalens, J-P, et al. Safety and efficacy of a probiotic-containing infant formula supplemented with 2′-fucosyllactose: a double-blind randomized controlled trial. Nutr J. (2022) 21:11. doi: 10.1186/s12937-022-00764-2
358. Xiong, W, Brown, CT, Morowitz, MJ, Banfield, JF, and Hettich, RL. Genome-resolved metaproteomic characterization of preterm infant gut microbiota development reveals species-specific metabolic shifts and variabilities during early life. Microbiome. (2017) 5:1–13. doi: 10.1186/s40168-017-0290-6
359. Fu, RH, Wu, DC, Yang, W, Scoffield, J, and Benveniste, EN. Probiotic metabolites promote anti-inflammatory functions of immune cells. FASEB J. (2017) 31:1041–8. doi: 10.1096/fasebj.31.1_supplement.1048.1
360. Miqdady, M, Al Mistarihi, J, Azaz, A, and Rawat, D. Prebiotics in the infant microbiome: the past, present, and future. Pediatr Gastroenterol Hepatol Nutr. (2020) 23:1. doi: 10.5223/pghn.2020.23.1.1
361. Béghin, L, Tims, S, Roelofs, M, Rougé, C, Oozeer, R, Rakza, T, et al. Fermented infant formula (with Bifidobacterium breve C50 and Streptococcus thermophilus O65) with prebiotic oligosaccharides is safe and modulates the gut microbiota towards a microbiota closer to that of breastfed infants. Clin Nutr. (2021) 40:778–87. doi: 10.1016/j.clnu.2020.07.024
362. Hegar, B, Wibowo, Y, Basrowi, RW, Ranuh, RG, Sudarmo, SM, Munasir, Z, et al. The role of two human milk oligosaccharides, 2′-fucosyllactose and lacto-N-neotetraose, in infant nutrition. Pediatr Gastroenterol Hepatol Nutr. (2019) 22:330–40. doi: 10.5223/pghn.2019.22.4.330
363. Berger, B, Porta, N, Foata, F, Grathwohl, D, Delley, M, Moine, D, et al. Linking human milk oligosaccharides, infant fecal community types, and later risk to require antibiotics. MBio. (2020) 11:e03196–19. doi: 10.1128/mBio.03196-19
364. Goehring, KC, Marriage, BJ, Oliver, JS, Wilder, JA, Barrett, EG, and Buck, RH. Similar to those who are breastfed, infants fed a formula containing 2′-fucosyllactose have lower inflammatory cytokines in a randomized controlled trial. J Nutr. (2016) 146:2559–66. doi: 10.3945/jn.116.236919
365. Lagkouvardos, I, Intze, E, Schaubeck, M, Rooney, JPK, Hecht, C, Piloquet, H, et al. Early life gut microbiota profiles linked to synbiotic formula effects: a randomized clinical trial in European infants. Am J Clin Nutr. (2023) 117:326–39. doi: 10.1016/j.ajcnut.2022.11.012
366. Laudisi, F, Stolfi, C, and Monteleone, G. Impact of food additives on gut homeostasis. Nutrients. (2019) 11:2334. doi: 10.3390/nu11102334
367. Rinninella, E, Cintoni, M, Raoul, P, Lopetuso, LR, Scaldaferri, F, Pulcini, G, et al. Food components and dietary habits: keys for a healthy gut microbiota composition. Nutrients. (2019) 11:2393. doi: 10.3390/nu11102393
368. Sordillo, JE, Zhou, Y, McGeachie, MJ, Ziniti, J, Lange, N, Laranjo, N, et al. Factors influencing the infant gut microbiome at age 3-6 months: findings from the ethnically diverse vitamin D antenatal asthma reduction trial (VDAART). J Allergy Clin Immunol. (2017) 139:482–91. doi: 10.1016/j.jaci.2016.08.045
369. Magnúsdóttir, S, Ravcheev, D, de Crécy-Lagard, V, and Thiele, I. Systematic genome assessment of B-vitamin biosynthesis suggests co-operation among gut microbes. Front Genet. (2015) 6:148. doi: 10.3389/fgene.2015.00148
370. Chassaing, B, Koren, O, Goodrich, JK, Poole, AC, Srinivasan, S, Ley, RE, et al. Dietary emulsifiers impact the mouse gut microbiota promoting colitis and metabolic syndrome. Nature. (2015) 519:92–6. doi: 10.1038/nature14232
371. Aljahdali, N, Gadonna-Widehem, P, Anton, PM, and Carbonero, F. Gut microbiota modulation by dietary barley malt melanoidins. Nutrients. (2020) 12:241. doi: 10.3390/nu12010241
372. Dupont, D, Boutrou, R, Menard, O, Jardin, J, Tanguy, G, Schuck, P, et al. Heat treatment of milk during powder manufacture increases casein resistance to simulated infant digestion. Food Dig. (2010) 1:28–39. doi: 10.1007/s13228-010-0003-0
373. Pérez-Burillo, S, Pastoriza, S, Jiménez-Hernández, N, D’Auria, G, Francino, MP, and Rufián-Henares, JA. Effect of food thermal processing on the composition of the gut microbiota. J Agric Food Chem. (2018) 66:11500–9. doi: 10.1021/acs.jafc.8b04077
374. Brink, LR, Matazel, K, Piccolo, BD, Bowlin, AK, Chintapalli, SV, Shankar, K, et al. Neonatal diet impacts bioregional microbiota composition in piglets fed human breast milk or infant formula. J Nutr. (2019) 149:2236–46. doi: 10.1093/jn/nxz170
375. Nunez, N, Réot, L, and Menu, E. Neonatal immune system ontogeny: the role of maternal microbiota and associated factors. How might the non-human primate model enlighten the path? Vaccines (Basel). (2021) 9:584. doi: 10.3390/vaccines9060584
376. Roura, E, Koopmans, S-J, Lallès, J-P, Le Huerou-Luron, I, de Jager, N, Schuurman, T, et al. Critical review evaluating the pig as a model for human nutritional physiology. Nutr Res Rev. (2016) 29:60–90. doi: 10.1017/S0954422416000020
377. Moszak, M, Szulińska, M, and Bogdański, P. You are what you eat—the relationship between diet, microbiota, and metabolic disorders—a review. Nutrients. (2020) 12:1096. doi: 10.3390/nu12041096
Keywords: breastfeeding, milk formulas, infant gut microbiome, non-thermal processing techniques, thermal processing, pediatric nutrition, infant diet formulation, human milk
Citation: Bakshi S, Paswan VK, Yadav SP, Bhinchhar BK, Kharkwal S, Rose H, Kanetkar P, Kumar V, Al-Zamani ZAS and Bunkar DS (2023) A comprehensive review on infant formula: nutritional and functional constituents, recent trends in processing and its impact on infants’ gut microbiota. Front. Nutr. 10:1194679. doi: 10.3389/fnut.2023.1194679
Edited by:
Ana Maria Calderon De La Barca, National Council of Science and Technology (CONACYT), MexicoReviewed by:
Abraham Wall-Medrano, Universidad Autónoma de Ciudad Juárez, MexicoLina Zhang, Jiangnan University, China
Copyright © 2023 Bakshi, Paswan, Yadav, Bhinchhar, Kharkwal, Rose, Kanetkar, Kumar, Al-Zamani and Bunkar. This is an open-access article distributed under the terms of the Creative Commons Attribution License (CC BY). The use, distribution or reproduction in other forums is permitted, provided the original author(s) and the copyright owner(s) are credited and that the original publication in this journal is cited, in accordance with accepted academic practice. No use, distribution or reproduction is permitted which does not comply with these terms.
*Correspondence: Vinod Kumar Paswan, dmtwYXN3YW4uZHNmdEBiaHUuYWMuaW4=