- 1School of Food and Nutritional Sciences, University College Cork, Cork, Ireland
- 2Department of Neonatology, Cork University Maternity Hospital, Cork, Ireland
- 3Brookfield School of Medicine and Health, University College Cork, Cork, Ireland
- 4Teagasc Food Research Centre, Moorepark, Cork, Ireland
- 5APC Microbiome, Cork, Ireland
Introduction: Human milk provides nutrients essential for infant growth and health, levels of which are dynamic during lactation.
Methods: In this study, changes in macronutrients, fatty acids, and plasmin activities over the first six months of lactation in term milk were studied.
Results: There was a significant influence of lactation stage on levels of protein and plasmin activities, but not on levels of fat and carbohydrate in term milk. Concerning fatty acids in term milk, levels of caproic acid and α-linolenic acid increased significantly (p < 0.05), whereas those of arachidonic acid and docosahexaenoic acid decreased, in the six months after birth. Significant impacts of maternal pre-pregnancy BMI and infant gender on fatty acid profiles were also found. Multivariate statistical analysis showed that protein level, plasmin activity, and several fatty acids (α-linolenic acid, lignoceric acid, and docasadienoic acid) contributed strongly to discrimination of milk from different lactational stages.
Discussion: The study demonstrates that not all but some fatty acids were influenced by lactation, whereas protein and protease levels showed clear decreasing trends during lactation, which may help in understanding the nutritional requirements of infants.
Introduction
Human milk contains essential macro- and micro-nutrients and is rich in bioactive components, which provide short-term and long-term benefits to infants (1). Macronutrients in human milk, including protein, fat, and carbohydrate are essential to infant growth and development. Besides macronutrients, human milk also provides enzymes that break down macronutrients to aid infant digestion. Proteases that hydrolyse the peptide bonds in proteins and peptides present in human milk include plasmin, trypsin, kallikrein, cathepsins, elastase, thrombin, amino- and carboxypeptidases and matrix metalloproteinases (2, 3). Among these, plasmin has been reported to be active at the pH of the infant stomach and is the major protease that hydrolyses caseins, as well as some proteins in whey, such as polymeric immunoglobulin receptor and osteopontin (4). Therefore, plasmin may potentially influence infant growth in both nutritional and immune functions. Studies on changes in plasmin activity during lactation can help understanding the dynamics of human milk and the requirement for infants; however, knowledge in this area is still limited (5, 6).
Moreover, fatty acids (FAs) in human milk are of significance because they contribute to 95% (w/w) of neutral lipids (7). The composition of FAs can largely influence the properties of milk triglycerides, thereby influencing the energy intake and infant growth (8). Meanwhile, FAs play roles as both the precursors of important metabolic compounds and the building blocks of cell membrane (9). Human milk is also a rich source of n-3- and n-6-polyunsaturated fatty acids (n-6 PUFA and n-3 PUFA) and their long-chain PUFA (LC PUFA) derivatives, such as arachidonic acid (AA) and docosahexaenoic acid (DHA), which have been demonstrated to promote neonatal growth and brain development (10).
The levels of nutrients in human milk are dynamic and have been reported to be influenced by many factors, including lactational stages, gestational age, maternal diet, age of mothers, parity, maternal body mass index (BMI), infant birth weight, infant gender, and diurnal variation (11–13). However, the impact of those factors on human milk composition is still controversial. For example, concerning the lactational duration, the level of fat has been reported to increase in early lactation (14, 15) and then remain stable (16, 17). However, one recent study from Netherlands reported a decrease in fat and a significant decrease in energy from 1 to 3 months (18). In the same way, the levels of carbohydrate have been reported to increase during the early stages of lactation (~1-month post-partum) and remain constant afterwards (16, 19), remain stable from the first week (5, 17) or increase from 1 to 6 months (20) accroding to studies from Western countries.
Studies have also demonstrated a sex-bias effect on human milk composition, but this is still under researched (21, 22). Some studies reported higher levels of carbohydrate (15), fat, and energy in milk for male infants (17, 23), while others reported increased carbohydrate and energy levels in milk for female infants (24). In contrast, no significant effect of infant gender on macronutrients in milk has been reported (25). Some studies have also reported that the gender of a baby affects other components in human milk, including minerals and hormones, and the milk microbiome (21, 26, 27).
Differing results of the impact of maternal BMI on macronutrients have also been reported; some studies have reported that lactose levels were lower (28, 29) and fat level was higher (30–32) in milk from mothers with BMI ≥25 kg m−2, while others found no association between BMI and macronutrient levels in human milk (29, 33). Moreover, the influencing factors on FA composition, such as maternal BMI and infant gender remain unclear. Therefore, this study attempts to provide more evidence on how such factors influence the FAs.
This study reports the changes in milk composition, including macronutrients, plasmin activities, and FA profiles up to 6 months of lactation; the influence of factors including maternal BMI and infant gender on milk macronutrients and FA profiles are also examined.
Methods
Ethical approval and study design
This study was approved by the Clinical Research Ethics Committee of the Cork Teaching Hospitals. All participants were recruited at Cork University Maternity Hospital and provided written consent during the defined period of time of the study (Jan 2017 to Dec 2020), and all relevant guidelines and regulations were followed.
Healthy mothers who had given birth at full term delivery (118 recruited, 31 excluded) were enrolled in this study (Figure 1). The sample size was similar to previous studies and the number of subjects was sufficient for our study (19, 24). Term milk was collected at 1-, 4-, 8-, and 24-weeks post-partum. Questionnaires on 24-h dietary recall were collected with the milk samples.
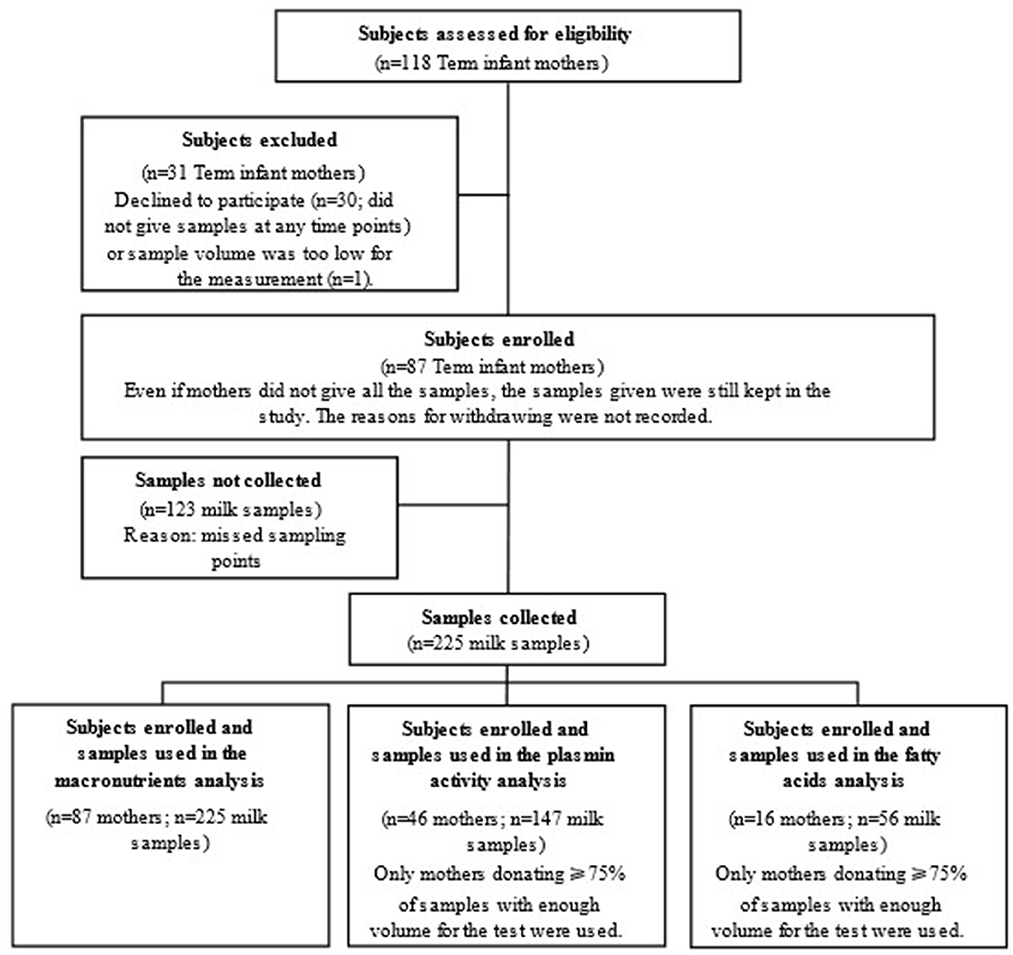
Figure 1. Study flow chart indicating subject recruitment and sample collection for analyses in the study.
Human milk collection and storage
For milk sampling, mothers expressed 10–20 ml of milk, which was collected after the infant was satisfied, and hence represented largely mid- to hind-milk. Milk was collected into a sterile container provided and stored at 4°C until delivery to the laboratory. Samples for macronutrient, plasmin activity and FA analysis were stored at −80°C until analysis. All chemicals were obtained from Merck KGaA, Darmstadt, Germany, unless otherwise stated.
Determination of levels of macronutrients
Total fat, protein, carbohydrate, and energy contents of human milk were determined with a human milk analyser (MIRIS, Uppsala, Sweden) based on mid-infrared transmission spectroscopy. The levels of energy were calculated by MIRIS as: Energy (kcal L−1) = protein (g L−1) × 4.4 + fat (g L−1) × 9.25 + carbohydrate (g L−1) × 4. Samples collected for compositional analysis were thawed at 4°C overnight, warmed to 40°C and homogenized using a MIRIS sonicator (MIRIS, Uppsala, Sweden) before analysis. Approximately 1.5 ml of human milk was injected into the human milk analyser and the measurement of each sample was in duplicate.
Determination of plasmin activity
Plasmin activity in milk was measured in duplicate using a modified version of the method of Richardson and Pearce (34). Human milk samples were thawed at 4°C overnight and centrifuged at 14,000 rpm for 20 min with 0.4 M trisodium citrate added (3:1 ratio) to obtain a clear serum phase. A reaction mix of the sample (100 μl), coumarin substrate (40 μl) and 50 mM tris-HCl buffer (pH 7.5, 60 μl) were added into a well of black 96-well microplates (VWR International Ltd, Luttervorth, UK). The fluorescence intensity was determined at 380 nm (excitation) and 460 nm (emission) using a plate reader (Spectrafluorplus, Tecan). One unit of plasmin activity is defined as the activity necessary to release 1 nmol 7-amino-4-methyl coumarin (AMC) per ml per min under the conditions of the assay.
Determination of fatty acids in human milk
Prior to the analysis, milk samples were thawed at 4°C overnight and inverted 20 times to achieve a good mixing of milk fats. Total FAs were extracted from human milk and fatty acid methyl esters (FAME) were prepared according to the method of Kelishadi et al. (35) with some modifications. Briefly, 1 ml of internal standard (1 mg ml−1 of C11:0 in methanol) was added to 1 ml milk followed by 0.7 ml of 10 N KOH and 5.3 ml of methanol in a screw-threaded clear glass tube. The tube was incubated in a water bath at 55°C for 1.5 h and vortexed for 5 s at 20 min intervals. Samples were cooled to below room temperature with ice water and 0.58 ml of 24 N H2SO4 was added. Tubes were mixed by inversion and incubated at 55°C as before for 1.5 h, vortexing for 5 s every 20 min. Tubes were then cooled and 2 ml of hexane was added. Each sample was vortexed for 2 min followed by centrifugation (Beckman J-E Highspeed, Beckman Coulter, Brea, California) for 2 min at 5,000 rpm at 4°C. The clear upper phase (FAME extract) was transferred to a screw-cap Pyrex tube with Na2SO4 (1 g) added to remove water, followed by transfer to the GC test vial. Vials were capped and stored at −80°C until gas chromatography (GC) analysis.
A Supelco 37 Component FAME mix (product CRM47885) was used as stock solution to identify and quantify FAs in milk samples. Calibration curves were produced for each individual fatty acid from 5 working standards prepared by diluting the stock solution with hexane (10, 7, 5, 2, and 1 mg ml−1 standard mixes). Calibration curves consisted of a plot of peak area vs. concentration. The response linearity of each fatty acid had r2 values higher than 0.99. The calibration was validated and tested between batches of 20 samples. The limit of quantitation and limit of detection were established using the lowest concentration standard, where all FAME compounds assessed exhibited signal-to-noise (S/N) values of at least 11. Undecanoic acid (C11:0) was used as internal standard to monitor the efficiency of the FA extraction process and to calculate recovery rates. The latter was performed by analyzing and comparing the 5 mg ml−1 working standard [1] spiked with C11:0, at a concentration similar to that added to samples [2] and C11:0 analyzed alone at this concentration [3]. In all cases, the area counts from [2] and [3] were similar at > 95%.
GC analysis of FAMEs was carried out with a Shimadzu GC-2010 Plus Gas Chromatograph (Shimadzu Scientific Instruments, Kyoto, Japan), equipped with a split/splitless injector, an AOC-20i autoinjector, an AOC-20s autosampler, a flame ionization detector (FID) and a high polarity cyanopropyl siloxane phase-based column (Agilent J&W CP-Sil 88 for FAME), 50 m × 0.25 mm with 0.2-μm film thickness (Agilent Scientific Instruments, Santa Clara, California). Helium was used as carrier gas at a linear velocity of 0.5 ml min−1. Air and hydrogen flow rates were set at 400 and 40 ml min−1, respectively. The make-up flow rate was set at 30 ml min−1, and the auto injector and the detector temperatures were set at 260 and 270°C, respectively. Two microliter of sample was injected, with the split set at 1/100. The column oven temperature was maintained at 30°C for 2 min, then increased at 10°C min−1 to 175°C and held for 15 min, followed by increasing at 5°C min−1 to 205°C, and finally by increasing at 3°C min−1 to 220°C and holding at this temperature for 24.5 min. The total runtime was 75 min. LabSolutions software (Shimadzu Scientific Instruments) was used for peak area analysis and quantification of FAs. Samples were analyzed in duplicate and the results for each FA were expressed as percentage of total FAs/peak area (%). The results for summed concentrations of saturated fatty acids (SFA), monounsaturated fatty acids (MUFA), n-3 LC PUFA, and n-6 LC PUFA are expressed as mg g−1 of total fat in a milk sample. A hexane blank was run between every 4 samples to ensure no carryover between samples.
Statistical analysis
Univariate statistical analyses of results were conducted with SPSS software version 17.0 (IBM, Armonk, New York). A mixed linear model was applied with the lactational stage (as categories) to determine whether the macronutrients, plasmin activity, and FAs changes over lactation time and to assess the impact of following factors on human milk composition: maternal pre-pregnancy BMI and infant gender. To estimate the effect of infant gender during lactation, the lactational stage (as categories), infant gender and their interaction were considered as fixed effects and the subject identification was considered as a random effect. A similar method was used to estimate pre-pregnancy BMI (as categories: BMI < 25 kg m−2 and BMI ≥ 25 kg m−2) and the interaction with age. Bonferroni post hoc tests with a level of significance at p < 0.05 were applied to analyse difference between groups. The data for each nutrient was tested for normality according to Q–Q plots and Kolmogorov–Smirnova test. Logarithmic transformation was applied to each nutrient when the data were not normally distributed.
To reduce the dimensionality of the multivariate dataset and to discriminate difference between samples from different lactational stages, principal component analysis (PCA) and partial least squares—discriminant analysis (PLS-DA), were also employed using SIMCA software version 14.1.0 (Umetrics AB, Umeå, Sweden). Unit variance auto scaling was used on the data obtained from deconvolution methods. PCA used orthogonal transformation to convert a set of potentially correlated variables into a set of values of linearly uncorrelated variables (principal components; PCs) that explain a large portion of the variance in the dataset. The PLS-DA model was computed to identify associations between the data (X variable) and lactational groups (Y variable). The model was validated using k-fold cross validation (k = 7) and a permutation test (200 permutations) (36), which measured its performance based on its R2 and Q2 values, as well as its accuracy.
Results
Participants
In total, 87 mothers who gave birth to full term neonates were enrolled in this study. As shown in Table 1, the average gestational age (mean ± SD) at birth was 39.5 ± 1.0 weeks, and the average mothers' pre-pregnancy BMI was close to the healthy weight threshold, i.e., 25 kg m−2. Meanwhile, the ratios of female infants to male infants was nearly 1:1 for the full study population, as well as for the sub-groups of participants, i.e., participants involved in the assay of FA profiles and the plasmin activity.
The total number of milk samples collected decreased during lactation as the number of mothers who stopped breast-feeding increased (Supplementary Table S1). In mothers who continued breast-feeding to 6 months, exclusive breast-feeding decreased from 82 to 56% during 24 weeks of lactation, as frequently seen (37).
Macronutrient levels during lactation
As shown in Figure 2, levels of protein (Mean ± SD) in term milk decreased significantly (p < 0.05, n = 87), from 1.7 ± 0.3 to 1.0 ± 0.2 g dl−1 from the first week to the 6 months after birth. The average total fat in term milk at each lactational stage did not show clear trends (ranged from 3.0 ± 1.8 g dl−1 at 24 weeks to 3.8 ±1.7 g dl−1 at 4 weeks). Carbohydrate content remained relatively unchanged during lactation (6.8–7.0 g dl−1). Energy content showed a similar trend to the fat content during lactation, in that the highest energy content was at 4 weeks post-partum; however, there was a significant association with lactation stage (p < 0.05, n = 87).
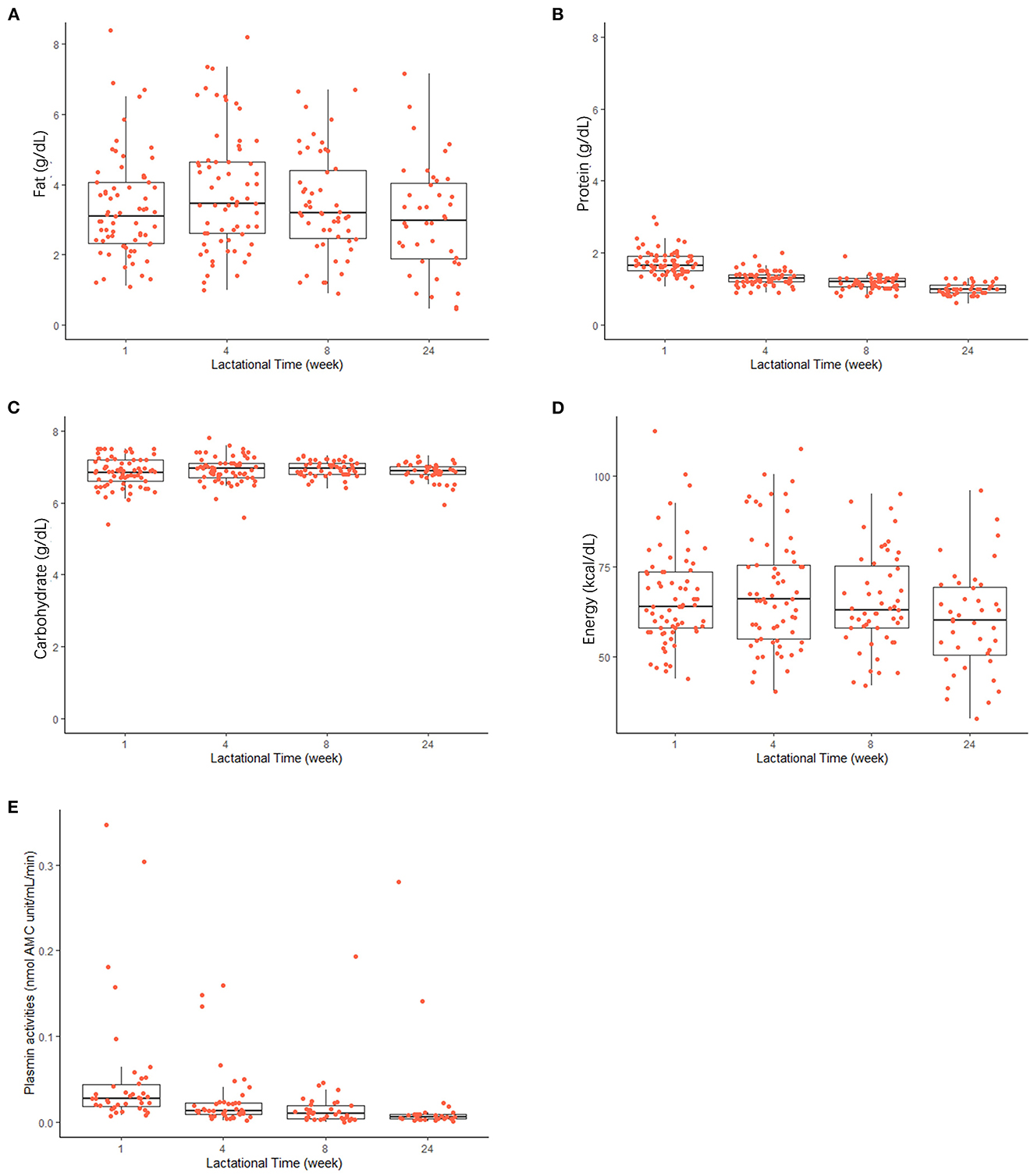
Figure 2. Changes in macronutrient levels [(A) fat; (B) protein; (C) carbohydrate], energy content (D), and plasmin activities (E) in human milk during the first 24 weeks of the lactation. Horizontal lines inside boxes indicate the median value. Lines extending vertically from the boxes indicate variability outside the upper and lower quartiles are indicated. Red points indicate the individual values in each lactational time group.
In addition, the impact of maternal BMI and infant gender on macronutrient levels in milk was estimated (Supplementary Figures S1, S2). Maternal BMI significantly (p < 0.05, n = 75) influenced levels of protein in milk at 4 weeks after birth; the protein level was lower in milk expressed by mothers with low BMI than the milk expressed form high BMI mothers after 4 weeks post-partum. No gender effect was found on levels of macronutrients and energy in milk at each lactational time point (p > 0.05, n = 87).
Plasmin activities
As shown in Figure 2E, plasmin activity decreased during lactation in human milk, with high inter-individual differences, especially at early stages after birth. Plasmin activity significantly decreased from 0.052 ± 0.07 nmol AMC units min−1 ml−1 to 0.026 ± 0.04 nmol AMC units min−1 ml−1 at 4 weeks post-partum (p < 0.05, n = 46) and remained relatively constant after that.
Fatty acid profiles
GC chromatograms of the standard mix and an example of human milk FAs are shown in Figure 3; separation was achieved with good resolution. Among 35 FAs measured, summed concentrations of SFA, MUFA, n-3 LC PUFA, and n-6 LC PUFA are summarized in Table 2; these were not significantly influenced by lactation (p > 0.05, n = 16), i.e., they remained relatively constant during lactation. ∑ SFA (mean ± SD) content was 160.4 ± 42 to 164 ± 23 mg g−1 total fat, which was higher than ∑ MUFA, which was measured as 104.4 ± 52 to 117.6 ± 43 mg g−1 total fat. In terms of LC PUFAs, levels of ∑ n-3 LCPUFAs were lower than ∑ n-6 LCPUFAs.
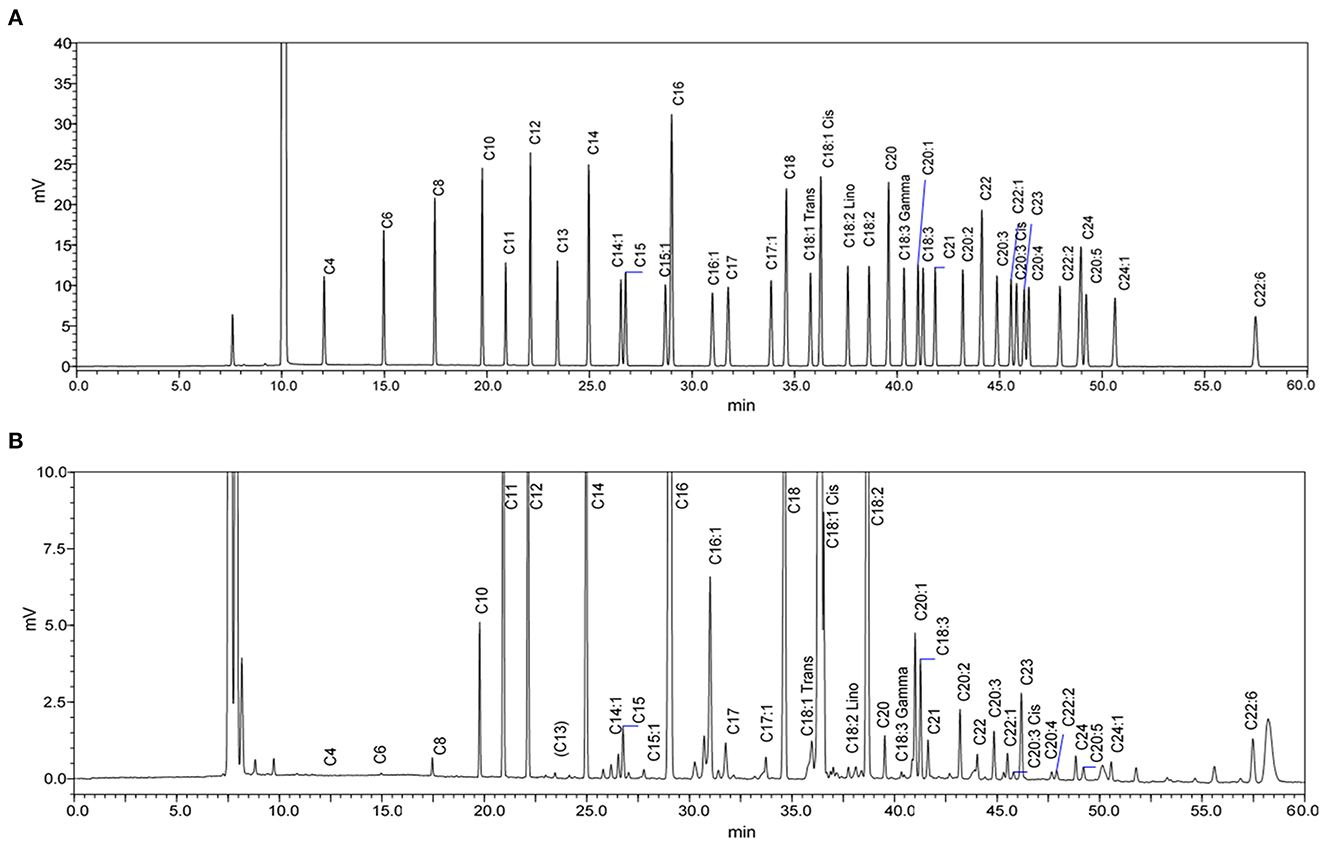
Figure 3. GC-FID chromatograms of (A) Supelco 37-Component fatty acid methyl esters (FAME) standard mix and (B) FAME from a human milk sample after one-week post-partum. The chromatogram of the milk sample is scaled to show all significant peaks.
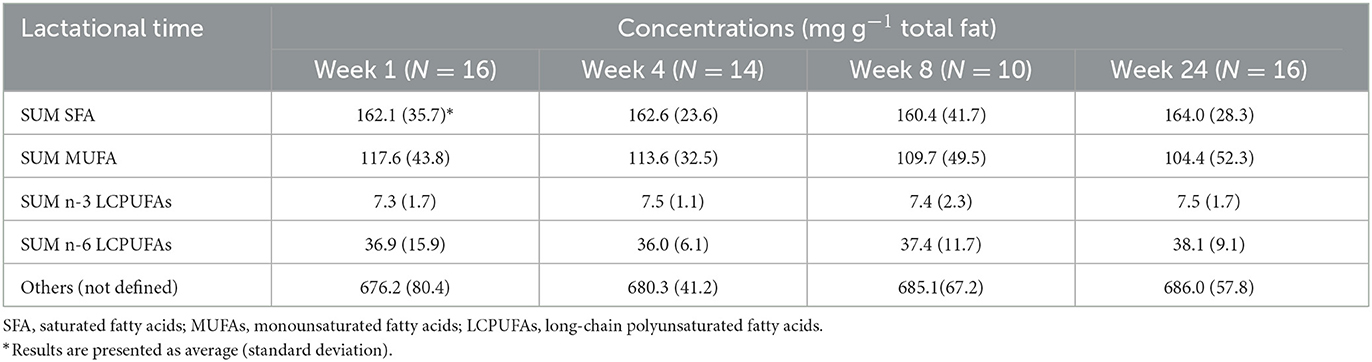
Table 2. Concentrations (mg g−1 total fat) of the sum of saturated fatty acids, monounsaturated fatty acids, n-3 long-chain poly-unsaturated fatty acids, and n-6 long-chain polyunsaturated fatty acids in human milk during lactation.
Average concentrations of individual FA in milk are shown in Table 3. According to the percentage of total FAs/peak area in GC chromatograms (see the example in Figure 3B), the most abundant FA (% total FAs) in term human milk out of 35 FAs measured in this study was oleic acid (C18:1 n-9; 28–31%). Other abundant FAs (% total FAs) in term milk included palmitic acid (C16:0; 20–21%), linoleic acid (C18:2 n-6; 10–11%), stearic acid (C18:0; 6.4–6.9%), myristic acid (C14:0; 5.8–6.4%), and lauric acid (C12:0; 5–5.1%).
There was a significant increase (p < 0.05) in levels of caproic acid (C6:0) and α-linolenic acid (ALA; C18:3 n-3) during lactation (Table 3). In contrast, levels of long-chain FAs, including AA (C20:4 n-6), erucic acid (C22:1), docasadienoic acid (C22:2), DHA (C22:6), and lignoceric acid (C24:0) decreased significantly (p < 0.05) during lactation. In addition, the ratio of linoleic acid to AA decreased from 9.8 to 8.1 during the first 6 months of lactation. Concentrations of cis-11-eicosenic acid (C20:1) and tricosanoic acid (C23:0) decreased significantly (p < 0.05) during the first 4 weeks after birth, but thereafter remained consistent until 24 weeks after birth. Lactational stage also had a significant impact on γ-linolenic acid (C18:3 n-6) levels (p < 0.05), the concentrations of which increased during 1–4 weeks after birth, but decreased from 4 to 24 weeks after birth; a summarized table of significantly changed fatty acids can be seen in Table 4.
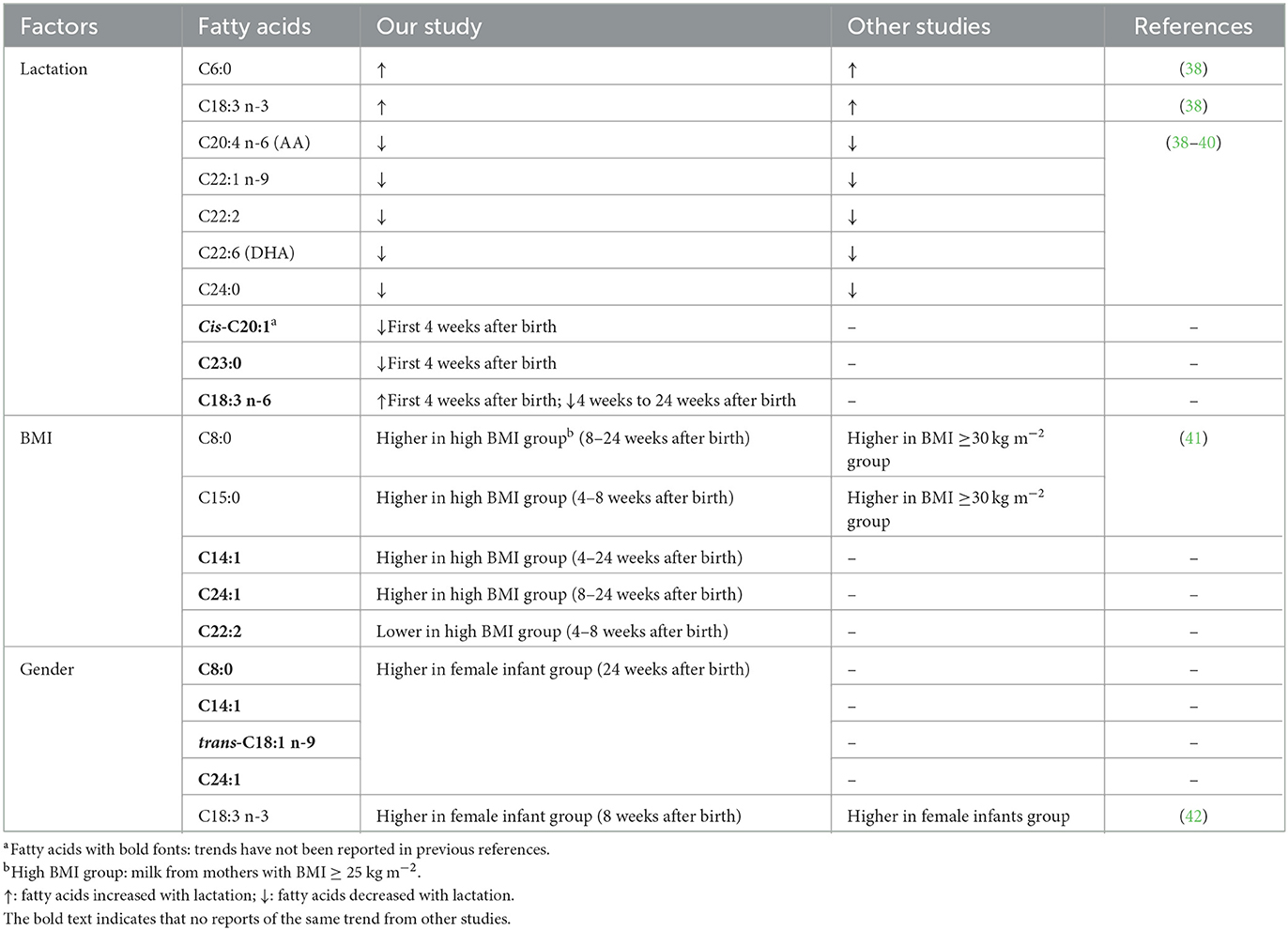
Table 4. A summary of the fatty acids with significantly changed fatty acids during the first 6 months of lactation.
In addition, maternal BMI had a significant impact (p < 0.05) on six SFAs (C8:0, C14:0, C15:0, C17:0, C21:0, and C24:0), five MUFAs (C14:1, C15:1 C16:1 C17:1, and C24:1), and six PUFAs (trans C18:2 n-6, C18:3 n-6, C20:3 n-6, C20:4 n-6, C20:5 n-3, and C22:2) in term milk (Supplementary Figure S3). Significantly (p < 0.05, n = 16) higher levels of most FAs were present in high BMI mothers (BMI ≥ 25 kg m−2) at week 4, week 8, or week 24. In contrast, term milk from high-BMI mothers contained significant (p < 0.05) lower levels of lignoceric acid (C24:0) at week 8, and lower levels AA (C20:4 n-6) and EPA (C20:5 n-3) at week 24.
When considering the impact of gender at each lactation stage (Supplementary Figure S4), there is no significant difference in each FA between milk of mothers of male or female infants after 1 or 4 weeks after birth. Significantly higher levels (p < 0.05, n = 16) of ALA (C18:3 n-3) and ∑ n-3 LCPUFAs were present in milk for female infants than in milk for male infants after 8 weeks post-partum. In milk collected 24 weeks post-partum, concentrations of SFAs, including caprylic acid (C8:0), myristic acid (C14:0), and ∑ SFA, as well as some MUFAs, including myristoleic acid (C14:1), nervonic acid (C24:1), and trans-9-elaidic acid (trans C18:1 n-9), were significantly higher in milk from mothers of female infants than in milk for male infants.
Multivariate statistical analysis
The relationship between fatty acids, macronutrients, and plasmin activities of milk were analyzed by a PCA model. The final model was summarized in seven PCs and described 78.6% of the total variance in the data. As shown in Figure 4A, the first PC and the second PC accounted for 29.0 and 12.6% of the total variation, respectively. Apart from one sample at the top left of the score plot, samples from the first week of lactation were clustered at the center and right side of the first PC, whereas samples from week 4, 8, and 24 are randomly distributed throughout the plot. According to the loading plot (Figure 4B), the first PC was positively driven by energy and fat, while it was negatively driven by trans C18:1 n-9, sum of SFA, and levels of C8:0, C21:0, C20:3 n-6, C20:0, C17:1, C17, C15:0, C14:1. The second PC showed a strong positive correlation with C20:3 n-3, C18:2 n-6, C22:2 and the sum of n-6 LCPUFAs. It can also be seen that fat and energy were highly correlated. Some fatty acids, for example, C20:1 and C18:1 n-9, C15:1 and trans C18:1 n-9, also showed high correlation with each other. By combining the score and loading plot, levels of protein, C18:0, and C16:1 as well as plasmin activities were correlated with samples from the first week of lactation that clustered at the center.
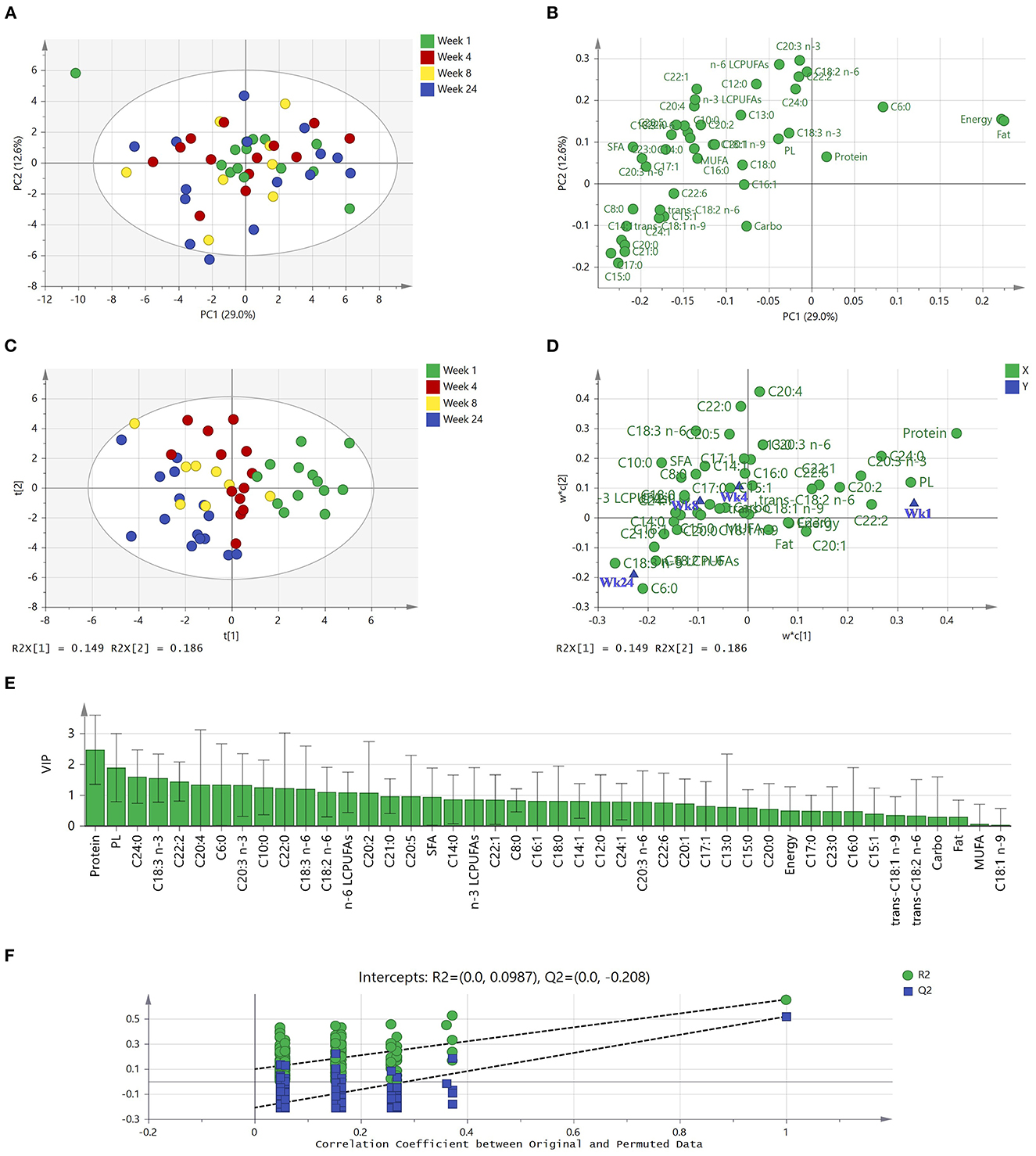
Figure 4. PCA and PLS-DA of subgroup human milk samples (n = 50) from term deliveries collected during the first 24 weeks of lactation. (A) PCA scores scatter plot of observations colored according to lactational stages. (B) Corresponding PCA loadings scatter plot of variables measured. (C) PLS-DA scores scatter plot of observations collected colored according to lactational stages (one extreme outlier was excluded). (D) Corresponding w*c loadings scatter plot applies both the X-weights (w*) and Y-weights (c) to identify associations between the collected data (X variables) and lactational stages (Y variables). (E) X variables (macronutrients, plasmin activities, and fatty acids) able to discriminate between lactational groups, ordered by VIP score. VIP scores >1 are significant and indicate important X variables that predict Y responses (lactational stages). (F) Permutation test of the PLS-DA model, indicating the absence of over-fitting in the model. The tolerance ellipse in scores scatter plots were drawn based on Hotelling's T2 with 95% confidence interval. PCA, principal component analysis; PLS-DA, partial least squares—discriminant analysis; Carbo, carbohydrate; PL, plasmin; SFA, saturated fatty acids; MUFA, monounsaturated fatty acids; LCPUFAs, long-chain poly-unsaturated fatty acids.
According to the PCA analysis, one extreme outlier (a week 1 sample) was excluded for supervised PLS-DA, for a better discrimination of milk samples between four lactational stages (Week 1, 4, 8, and 24). The model had two latent variables (R2Y = 0.35, Q2 = 0.16, p < 0.001 by CV-ANOVA) and the four groups are visualized in Figure 4C. It is clear that Week 1 samples (right side of y-axis) and Week 24 samples (left side of y-axis) can be discriminated from each other, whereas week 4 and week 8 samples can only be weakly discriminated from each other. The corresponding loading plot (Figure 4D) summarizes how the nutrients (X-variable) relate to each other as well as to lactational stage (Y-variable symbolized by a group dot). According to the location of X- and Y-variables, milk at one-week postpartum is characterized by high levels of protein and high plasmin activity, but low levels of C18:3 n-3 and C6:0. Conversely, milk at 24-weeks postpartum had low levels of protein, plasmin activities, and C20:4, while high levels of C18:3 n-3 and C6:0. Figure 4E with VIP values illustrates the important X variables (VIP >1) that predict Y responses (lactational stages), including protein, plasmin activity, C24:0, C18:3 n-3, and C22:2, followed by C20:4, C6:0, C20:3 n-3, C10:0, C22:0, C18:3 n-6, C18:2 n-6, Σ n-6 PUFAs, and C20:2.
A permutation test was used to evaluate the possible overfitting of the PLS-DA model, and a properly fitted model was identified as having values of R2-intercept <0.4 and a Q2-intercept <0.05. According to the permutation tests, the R2-intercept was 0.0987 and Q2-intercept was −0.208 (Figure 4F), indicating that the PLS-DA model had no overfitting and was credible. However, it is still notable that Q2 was below 0.5, indicating that the model showed weak predictability.
Discussion
Levels of proteins, fat, carbohydrate, and energy measured in the study were in accordance with many previous reports, being in the ranges 0.9–1.9 g dl−1, 3.2–4.1 g dl−1, 6.2–7.8 g dl−1, and 65–70 kcal dl−1, respectively (5, 19, 43–46). Analysis of mid- to hind- milk collected in the study indicated that higher levels of fat and energy are present compared to that in foremilk (13). Our results support reports that levels of protein decrease during lactation and that levels of total carbohydrate are relatively static during lactation (5). The levels of selected human milk oligosaccharides (HMOs) in the same cohort of samples were measured by Overgaard Poulsen et al. (47); those results showed a higher level of HMOs but a lower level of lactose in the early stage of lactation compared to the later stages of lactation. The increased fat and energy content from 1 to 4 weeks after birth has also been reported by previous studies (19, 48). There was no significant impact of infant gender on fat and energy content in term milk, in agreement with previous reports (17, 23, 25). When considering BMI, our results showed that milk expressed by high BMI mothers contained higher levels of protein than that of lower BMI mothers at 4 weeks post-partum, which was not found in previous reports (29, 33).
The changes in plasmin activities during lactation was measured in the study as a supplemental information for protein levels, as it may be a potential factor influencing the protein quality and digestion of infants (49, 50). Trypsin and thrombin, which have been detected in human milk, have similar specificities to plasmin (51). However, thrombin does not interfere with the assay applied in our study (34) and levels of trypsin have been reported to be low in human milk (52). The plasmin activities in term milk were in the range 0.018–0.052 nmol AMC units min−1 ml−1, and the decreasing trend of plasmin activities and high correlation to milk protein levels during lactation are in accordance with previous reports (5, 6).
Concerning FAs in milk, the major SFA presented in human milk was palmitic acid and the major MUFA was oleic acid, and the abundance of these were in accordance with previous reports (38, 53, 54). AA (C20:4 n-6), EPA (C20:5 n-3), and DHA (C22:6 n-3) are widely reported LC PUFAs in human milk, because they are structural components of cellular membranes, and are incorporated in relatively large amounts during early growth of the brain and the retina (8). In our study, the levels of EPA (C20:5 n-3) and DHA (C22:6 n-3) were in the same range, whereas AA (C20:4 n-6) were lower compared to the literature, with reports of 0.04%−0.15% of total FAs for EPA (C20:5 n-3), 0.2%−0.7% of total FAs for DHA (C22:6 n-3), and 0.45%−0.8% of total FAs for AA (C20:4 n-6) (32, 35, 39, 53). The European Food Safety Authority (EFSA) recommends a voluntary addition of AA, DHA, and EPA in infant formula, with AA <1% of total FAs, DHA < total n-6 LCPUFAs (<2% of total FAs), and EPA < DHA (55), which is consistant with our results.
Linoleic acid (C18:2 n-6) is the precursor of AA (C20:4 n-6), while ALA (C18:3 n-3) is the precursor of EPA (C20:5 n-3) and DHA (C22:6 n-3) (8). As for AA, EPA, and DHA, there is a particular need for linoleic acid and ALA in the first 6 months of life in terms of the development of nervous system. EFSA requires that the ratio of linoleic acids to AA should between 5 to 15 (55), which is in accordance with our results that the ratio decreased during the first 6 month of lactation. The levels of linoleic acid (C18:2 n-6) were lower than previous reports (17%−18% total FAs), while ALA (C18:3 n-3) levels were comparable to a Croatian study and a Spanish study (0.7%−1.4%) (53, 56). The lower content of linoleic acid may be a result of the low maternal dietary intake of this FA (57). Mothers involved in this study had a higher intake of animal oil (low linoleic acids levels), but a lower intake of vegetable oil (high linoleic acids levels) (Supplementary Table S1). Differences in FA profiles results between studies may also result from analytical methods and from population characteristics (58, 59). The GC-FID method used in our study is sensitive with a low limit of detection. However, only 37 standards applied limited the FAs that can be identified.
Concerning the lactational effect on FA profiles, significant increases in levels of caproic acid (C6:0) and ALA (C18:3 n-3) agreed with the increase of caproic acid from colostrum to mature milk reported in a meta-analysis by Floris et al. (38). Decreased levels of AA (C20:4 n-6), DHA (C22:6 n-3), lignoceric acid (C24:0), erucic acid (C22:1 n-9), and docasadienoic acid (C22:2) during lactation found in our study have also been reported previously (38–40).
Statistically significant associations between maternal BMI and FAs in human milk were found in this study. Levels of some SFA, MUFA, and PUFA were higher, while levels of lignoceric acid (C24:0), AA (C20:4 n-6) and EPA (C20:5 n-3) were lower, in milk from mothers with BMI ≥25 kg m−2 at specific lactational stages. de la Garza Puentes et al. (41) reported higher pentadecanoic acid (C15:0) and heptadecanoic acid (C17:0) levels in milk from mothers with high BMI, as shown here. They also reported higher levels of caprylic acid (C8:0) from obese mothers (BMI ≥ 30 kg m−2) compared to mothers with BMI between 25 and 30 kg m−2 in human milk. However, a statistically significant difference (p < 0.05) was only found in colostrum in their study, not in transitional or mature human milk. In terms of LC PUFA, higher levels of pentadecanoic acid (C15:0), heneicosanoic acid (C21:0) and trans-linolelaidic acid (trans C18:2 n-6) were found in mothers with higher BMI than mothers with lower BMI, similar to our results; however, they did not find statistical significance (p > 0.05) (60). Instead, these authors found significantly higher palmitic acid (C16:0) and significantly lower oleic acid (C18:1 n-9) levels in milk of mothers with BMI ≥ 25 kg m−2, which was not observed in our study. A recent study showed that milk from these two BMI groups could be distinguished by fatty acid profiles, and C21:0 and C24:0 had high discrimination coefficients, which is similar to our study (42).
To our knowledge, few reports have related levels of FAs to infant gender. Significant relationships were found between infants' gender and some FAs in this study. The major impact of infant gender was observed after 8- or 24-weeks post-partum in milk. Higher levels (mg/g fat) of ALA (C18:3 n-3) and ∑ n-3 LCPUFAs at week 8 post-partum, some SFAs and some MUFAs at week 24 post-partum were found in milk for female infants compared to milk for male infants (Supplementary Figure S4). Thakkar et al. (61) also reported higher myristic acid (C14:0), ALA (C18:3 n-3), and total SFA levels in milk for female offspring than for male offspring during a 120-day lactational observation; however, the difference was not significant (p > 0.05). They also reported significantly higher levels of linoleic acid (C18:2 n-6), 20:2 n-6 (eicosadienoic acid), and total PUFA in milk for female infants than milk for male infants, which was not observed in our study. One recent study did not find significant relationships between infant gender and FA profiles (62). Interestingly, in terms of the sex-bias of nervonic acid (C24:1) levels found in our study, this FA has been reported to decrease during lactation (not found in this study) with higher abundance in preterm human milk (40, 63). Nervonic acid is important for white matter brain development and thus contributes to the central nervous system of infants (63), and has been reported to be present at significantly higher levels in human milk compared to infant formula (64). The significance of variations in levels of this FA may need further investigation.
Multivariate statistical analysis provided a whole picture for the nutrients rather than only individual profiles (Figure 4). Although PCA could only weakly discriminate milk samples from four lactational stages using the first two PCs, it was clear that some fatty acids are closely located and thus highly correlated and that total fat content is negatively correlated with some SFA (C15:0, C17:0, C20:0, and C21:0). Interestingly, caproic acid (C6:0), a medium-chain fatty acid that increased in level during the first 6 months of lactation, showed an opposite projection on PC1 compared to other fatty acids in the PCA loading plot.
Supervised PLS-DA improved the discrimination of milk samples from lactational groups, and clearly separated week 1 and week 24 samples. The Q2 of the model is low, which could be a result of the poor discrimination of samples at lactational week 4 and week 8. Except for a few fatty acids (such as C24:0, C18:3 n-3), fatty acids showed lower contribution to predict milk from different lactational stages compared to protein and plasmin activities. This result indicates that milk proteins and proteases, moreso than fatty acids, are highly impacted by lactation stage.
The strength of this study is that it provides an overall profile of milk composition and plasmin activity and detailed comparison of FAs, as affected by lactational time points, gender, and BMI. Limitations of our study that could be addressed in future work is that numbers of mothers in different cohort are more closely balanced, information on diet could be more specific, and only fatty acids provided in the form of standards were analyzed.
Conclusion
There was a significant influence (p < 0.05) of lactation stage on levels of protein and plasmin activity in human milk during lactation. Pre-pregnancy BMI influenced the protein content of human milk. In addition, there was significant influence (p < 0.05) of lactation, pre-pregnancy maternal BMI and infant gender on individual FA profile in term human milk. Cis-C20:1 and C23:0 levels decreased during the first 4 weeks after birth, while those of C18:3 n-6 increased during the first 4 weeks but decreased from 4 to 24 weeks after birth. Levels of 12 fatty acids were higher in milk from mothers with high BMI at specific lactational stages, while only levels of C22:2 were lower in milk from mothers with high BMI from 4 to 8 weeks after birth. This study updates knowledge on the impact of human milk lactation, pre-pregnancy maternal BMI, and infants' gender on human milk macronutrients, fatty acid profiles, and protease activities, providing an overall picture on human milk macronutrients that can be useful in improving infant nutrition, for example, through strategies for targeted fortification of human breast milk in infancy.
Data availability statement
The raw data supporting the conclusions of this article will be made available by the authors, without undue reservation.
Ethics statement
The studies involving human participants were reviewed and approved by Clinical Research Ethics Committee of the Cork Teaching Hospitals. The patients/participants provided their written informed consent to participate in this study.
Author contributions
Conceptualization: AR, AK, and CS. Methodology: FM, TU-L, and EL. Formal analysis: FM, TU-L, and TD. Resources: GM and C-AO'S. Data curation and writing—original draft preparation: FM. Writing—review and editing: TU-L, FM, and AK. Supervision: AK. Funding acquisition: AK and CS. All authors have read and agreed to the published version of the manuscript.
Funding
This research was funded by Department of Agriculture, Food and the Marine, Ireland, grant number 15/F/721.
Acknowledgments
The authors would like to acknowledge the participants for their kind donations.
Conflict of interest
The authors declare that the research was conducted in the absence of any commercial or financial relationships that could be construed as a potential conflict of interest.
Publisher's note
All claims expressed in this article are solely those of the authors and do not necessarily represent those of their affiliated organizations, or those of the publisher, the editors and the reviewers. Any product that may be evaluated in this article, or claim that may be made by its manufacturer, is not guaranteed or endorsed by the publisher.
Supplementary material
The Supplementary Material for this article can be found online at: https://www.frontiersin.org/articles/10.3389/fnut.2023.1172613/full#supplementary-material
Supplementary Table S1. Diet information of mothers within 24 h of each expression.
Supplementary Figure S1. Macronutrient and energy levels in term human milk from mothers with normal weight (BMI < 25 kg m−2) and from overweight mothers (BMI ≥ 25 kg m−2) during the first 6 months of lactation. Lines extending vertically from the boxes indicate variability outside the upper and lower quartiles. Mild outliers (°, 1.5–3 times outside the interquartile range), extreme outliers (×, 3 times outside the interquartile range), and statistical significance (p < 0.05) between BMI groups are indicated. Normal, normal weight; OW, overweight.
Supplementary Figure S2. Macronutrient and energy levels in term human milk from mothers who gave birth to female infants and mothers who gave birth to male infants during the first 6 months of lactation. Lines extending vertically from the boxes indicate variability outside the upper and lower quartiles. Mild outliers (°, 1.5–3 times outside the interquartile range), extreme outliers (×, 3 times outside the interquartile range), and statistical significance (p < 0.05) between gender are indicated.
Supplementary Figure S3. Fatty acids levels in term human milk from mothers with normal weight (BMI < 25 kg m−2; white bar) and those with BMI ≥ 25 kg m−2 (gray bar) during the first 6 months of lactation. Lines extending vertically from the boxes indicate variability outside the upper and lower quartiles. Mild outliers (°, 1.5–3 times outside the interquartile range) and statistical significance (p < 0.05) between BMI groups are indicated.
Supplementary Figure S4. Fatty acids levels from mothers who gave birth to female infants (white bar) and mothers who gave birth to male infants (gray bar) during the first 6 month of lactation. Lines extending vertically from the boxes indicate variability outside the upper and lower quartiles. Mild outliers (°, 1.5–3 times outside the interquartile range) and statistical significance (p < 0.05) between gender are indicated.
References
1. Perrella S, Gridneva Z, Lai CT, Stinson L, George A, Bilston-John S, et al. Human milk composition promotes optimal infant growth, development and health. Semin Perinatol. (2021) 45:151380. doi: 10.1016/j.semperi.2020.151380
2. Chan LE, Beverly RL, Dallas DC. The enzymology of human milk. In:Kelly AL, Larsen LB, , editors. Agents of Change: Enzymes in Milk and Dairy Products. Cham: Springer International Publishing (2021), p. 209–43. doi: 10.1007/978-3-030-55482-8_9
3. Deglaire A, Oliveira SD, Jardin J, Briard-Bion V, Kroell F, Emily M, et al. Impact of human milk pasteurization on the kinetics of peptide release during in vitro dynamic digestion at the preterm newborn stage. Food Chem. (2019) 281:294–303. doi: 10.1016/j.foodchem.2018.12.086
4. Khaldi N, Vijayakumar V, Dallas DC, Guerrero A, Wickramasinghe S, Smilowitz JT, et al. Predicting the important enzymes in human breast milk digestion. J Agric Food Chem. (2014) 62:7225–32. doi: 10.1021/jf405601e
5. Caldeo V, Downey E, O'Shea C-A, Affolter M, Volger S, Courtet-Compondu M-C, et al. Protein levels and protease activity in milk from mothers of pre-term infants: a prospective longitudinal stu dy of human milk macronutrient composition. Clin Nutr. (2021) 40:3567–77. doi: 10.1016/j.clnu.2020.12.013
6. Armaforte E, Curran E, Huppertz T, Ryan CA, Caboni MF, O'Connor PM, et al. Proteins and proteolysis in pre-term and term human milk and possible implications for infant formulae. Int Dairy J. (2010) 20:715–23. doi: 10.1016/j.idairyj.2010.03.008
9. Bobiński R, Bobińska J. Fatty acids of human milk - a review. Int J Vitam Nutr Res. (2022) 92:280–91. doi: 10.1024/0300-9831/a000651
10. Demmelmair H, Prell C, Timby N, Lönnerdal B. Benefits of lactoferrin, osteopontin and milk fat globule membranes for infants. Nutrients. (2017) 9:817. doi: 10.3390/nu9080817
11. Meng F, Uniacke-Lowe T, Ryan AC, Kelly AL. The composition and physico-chemical properties of human milk: a review. Trends Food Sci Technol. (2021) 112:608–21. doi: 10.1016/j.tifs.2021.03.040
12. Andreas NJ, Kampmann B, Le-Doare KM. Human breast milk: a review on its composition and bioactivity. Early Hum Dev. (2015) 91:629–35. doi: 10.1016/j.earlhumdev.2015.08.013
13. Pham Q, Patel P, Baban B, Yu J, Bhatia J. Factors affecting the composition of expressed fresh human milk. Breastfeed Med. (2020) 15:551–8. doi: 10.1089/bfm.2020.0195
14. Anderson G, Atkinson S, Bryan M. Energy content of human milk during early lactation from mothers giving birth prematurely. Am J Clin Nutr. (1981) 34:258–65. doi: 10.1093/ajcn/34.2.258
15. Sahin S, Ozdemir T, Katipoglu N, Akcan AB, Kaynak Turkmen M. Comparison of changes in breast milk macronutrient content during the first month in preterm and term infants. Breastfeed Med. (2020) 15:56–62. doi: 10.1089/bfm.2019.0141
16. Maly J, Burianova I, Vitkova V, Ticha E, Navratilova M, Cermakova E. Preterm human milk macronutrient concentration is independent of gestational age at birth. Arch Dis Child Fetal Neonatal Ed. (2019) 104:F50–6. doi: 10.1136/archdischild-2016-312572
17. Fischer Fumeaux CJ, Garcia-Rodenas CL, De Castro CA, Courtet-Compondu M-C, Thakkar SK, Beauport L, et al. Longitudinal analysis of macronutrient composition in preterm and term human milk: a prospective cohort study. Nutrients. (2019) 11:1525. doi: 10.3390/nu11071525
18. de Fluiter KS, Kerkhof GF, van Beijsterveldt I, Breij LM, van de Heijning BJM, Abrahamse-Berkeveld M, et al. Longitudinal human milk macronutrients, body composition and infant appetite during early life. Clin Nutr. (2021) 40:3401–8. doi: 10.1016/j.clnu.2020.11.024
19. Bauer J, Gerss J. Longitudinal analysis of macronutrients and minerals in human milk produced by mothers of preterm infants. Clin Nutr. (2011) 30:215–20. doi: 10.1016/j.clnu.2010.08.003
20. Allen JC, Keller RP, Archer P, Neville MC. Studies in human lactation: milk composition and daily secretion rates of macronutrients in the first year of lactation. Am J Clin Nutr. (1991) 54:69–80. doi: 10.1093/ajcn/54.1.69
21. Galante L, Milan AM, Reynolds CM, Cameron-Smith D, Vickers MH, Pundir S. Sex-specific human milk composition: the role of infant sex in determining early life nutrition. Nutrients. (2018) 10:1194. doi: 10.3390/nu10091194
22. Tottman AC, Oliver CJ, Alsweiler JM, Cormack BE. Do preterm girls need different nutrition to preterm boys? Sex-specific nutrition for the preterm infant. Pediatr Res. (2021) 89:313–7. doi: 10.1038/s41390-020-01252-1
23. Powe CE, Knott CD, Conklin-Brittain N. Infant sex predicts breast milk energy content. Am J Hum Biol. (2010) 22:50–4. doi: 10.1002/ajhb.20941
24. Hosseini M, Valizadeh E, Hosseini N, Khatibshahidi S, Raeisi S. The role of infant sex on human milk composition. Breastfeed Med. (2020) 15:341–6. doi: 10.1089/bfm.2019.0205
25. Quinn EA. No evidence for sex biases in milk macronutrients, energy, or breastfeeding frequency in a sample of Filipino mothers. Am J Phys Anthropol. (2013) 152:209–16. doi: 10.1002/ajpa.22346
26. Moossavi S, Sepehri S, Robertson B, Bode L, Goruk S, Field CJ, et al. Composition and variation of the human milk microbiota are influenced by maternal and early-life factors. Cell Host Microbe. (2019) 25:324–35. doi: 10.1016/j.chom.2019.01.011
27. Fields DA, George B, Williams M, Whitaker K, Allison DB, Teague A, et al. Associations between human breast milk hormones and adipocytokines and infant growth and body composition in the first 6 months of life. Pediatr Obes. (2017) 12:78–85. doi: 10.1111/ijpo.12182
28. Young BE, Patinkin ZW, Pyle L, de la Houssaye B, Davidson BS, Geraghty S, et al. Markers of oxidative stress in human milk do not differ by maternal BMI but are related to infant growth trajectories. Matern Child Health J. (2017) 21:1367–76. doi: 10.1007/s10995-016-2243-2
29. Leghi GE, Netting MJ, Middleton PF, Wlodek ME, Geddes DT, Muhlhausler ABS. The impact of maternal obesity on human milk macronutrient composition: a systematic review and meta-analysis. Nutrients. (2020) 12:934. doi: 10.3390/nu12040934
30. Bzikowska-Jura A, Sobieraj P, Szostak-Wegierek D, Wesołowska A. Impact of infant and maternal factors on energy and macronutrient composition of human milk. Nutrients. (2020) 12:2591. doi: 10.3390/nu12092591
31. Dritsakou K, Liosis G, Valsami G, Polychronopoulos E, Skouroliakou M. The impact of maternal- and neonatal-associated factors on human milk's macronutrients and energy. J Matern Fetal Neonatal Med. (2017) 30:1302–8. doi: 10.1080/14767058.2016.1212329
32. Kim H, Kang S, Jung BM Yi H, Jung JA, Chang N. Breast milk fatty acid composition and fatty acid intake of lactating mothers in South Korea. Br J Nutr. (2017) 117:556–61. doi: 10.1017/S0007114517000253
33. Mäkelä J, Linderborg K, Niinikoski H, Yang B, Lagström H. Breast milk fatty acid composition differs between overweight and normal weight women: the STEPS Study. Eur J Nutr. (2013) 52:727–35. doi: 10.1007/s00394-012-0378-5
34. Richardson B, Pearce K. Determination of plasmin in dairy products. N Z J Dairy Sci Technol. (1981) 16:209–20.
35. Kelishadi R, Hadi B, Iranpour R, Khosravi-Darani K, Mirmoghtadaee P, Farajian S, et al. A study on lipid content and fatty acid of breast milk and its association with mother's diet composition. J Res Med Sci. (2012) 17:824–7.
36. Ahn WG, Jung JS, Song DK. Lipidomic analysis of plasma lipids composition changes in septic mice. Korean J Physiol Pharmacol. (2018) 22:399–408. doi: 10.4196/kjpp.2018.22.4.399
37. Theurich MA, Davanzo R, Busck-Rasmussen M, Díaz-Gómez NM, Brennan C, Kylberg E, et al. Breastfeeding rates and programs in europe: a survey of 11 national breastfeeding committees and representatives. J Pediatr Gastroenterol Nutr. (2019) 68:400–7. doi: 10.1097/MPG.0000000000002234
38. Floris LM, Stahl B, Abrahamse-Berkeveld M, Teller IC. Human milk fatty acid profile across lactational stages after term and preterm delivery: a pooled data analysis. Prostaglandins Leukot Essent Fatty Acids. (2020) 156:102023. doi: 10.1016/j.plefa.2019.102023
39. Genzel-Boroviczényy O, Wahle J, Koletzko B. Fatty acid composition of human milk during the 1st month after term and preterm delivery. Eur J Pediatr. (1997) 156:142–7. doi: 10.1007/s004310050573
40. Qi C, Sun J, Xia Y, Yu R, Wei W, Xiang J, et al. Fatty acid profile and the sn-2 position distribution in triacylglycerols of breast milk during different lactation stages. J Agric Food Chem. (2018) 66:3118–26. doi: 10.1021/acs.jafc.8b01085
41. de la Garza Puentes A, Martí Alemany A, Chisaguano AM, Montes Goyanes R, Castellote AI, Torres-Espínola FJ, et al. The effect of maternal obesity on breast milk fatty acids and its association with infant growth and cognition—the PREOBE follow-up. Nutrients. (2019) 11:2154. doi: 10.3390/nu11092154
42. Yu J, Yuan T, Zhang X, Jin Q, Wei W, Wang X. Quantification of nervonic acid in human milk in the first 30 days of lactation: influence of lactation stages and comparison with infant formulae. Nutrients. (2019) 11:1892. doi: 10.3390/nu11081892
43. Nommsen LA, Lovelady CA, Heinig MJ, Lönnerdal B, Dewey KG. Determinants of energy, protein, lipid, and lactose concentrations in human milk during the first 12 mo of lactation: the DARLING Study. Am J Clin Nutr. (1991) 53:457–65. doi: 10.1093/ajcn/53.2.457
44. Yamawaki N, Yamada M, Kan-no T, Kojima T, Kaneko T, Yonekubo A. Macronutrient, mineral and trace element composition of breast milk from Japanese women. J Trace Elem Med Biol. (2005) 19:171–81. doi: 10.1016/j.jtemb.2005.05.001
45. Wojcik KY, Rechtman DJ, Lee ML, Montoya A, Medo ET. Macronutrient analysis of a nationwide sample of donor breast milk. J Am Diet Assoc. (2009) 109:137–40. doi: 10.1016/j.jada.2008.10.008
46. Michaelsen KF, Skafte L, Badsberg JH, Jørgensen M. Variation in macronutrients in human bank milk: influencing factors and implications for human milk banking. J Pediatr Gastroenterol Nutr. (1990) 11:229–39. doi: 10.1097/00005176-199008000-00013
47. Overgaard Poulsen K, Meng F, Lanfranchi E, Yong JF, Kelly AL, Sundekilde UK. Dynamic changes in the human milk metabolome over 25 weeks of lactation. Nutrients. (2022) 9:917659. doi: 10.3389/fnut.2022.917659
48. Saarela T, Kokkonen J, Koivisto M. Macronutrient and energy contents of human milk fractions during the first six months of lactation. Acta Paediatr. (2005) 94:1176–81. doi: 10.1111/j.1651-2227.2005.tb02070.x
49. Dallas DC, Murray NM, Gan J. Proteolytic Systems in Milk: perspectives on the evolutionary function within the mammary gland and the infant. J Mammary Gland Biol Neoplasia. (2015) 20:133–47. doi: 10.1007/s10911-015-9334-3
50. Ferranti P, Traisci MV, Picariello G, Nasi A, Boschi V, Siervo M, et al. Casein proteolysis in human milk: tracing the pattern of casein breakdown and the formation of potential bioactive peptides. J Dairy Res. (2004) 71:74–87. doi: 10.1017/S0022029903006599
51. Dallas DC, Smink CJ, Robinson RC, Tian T, Guerrero A, Parker EA, et al. Endogenous human milk peptide release is greater after preterm birth than term birth. J Nutr. (2015) 145:425–33. doi: 10.3945/jn.114.203646
52. Monti JC, Mermoud AF, Jollès P. Trypsin in human milk. Experientia. (1986) 42:39–41. doi: 10.1007/BF01975884
53. Krešić G, Dujmović M, Mandić ML, Delaš I. Relationship between Mediterranean diet and breast milk fatty acid profile: a study in breastfeeding women in Croatia. Dairy Sci Technol. (2013) 93:287–301. doi: 10.1007/s13594-013-0125-6
54. Sánchez-Hernández S, Esteban-Muñoz A, Giménez-Martínez R, Aguilar-Cordero MJ, Miralles-Buraglia B, Olalla-Herrera M, et al. Comparison of changes in the fatty acid profile of human milk of spanish lactating women during the first month of lactation using gas chromatography-mass spectrometry. A comparison with infant formulas. Nutrients. (2019) 11:3055. doi: 10.3390/nu11123055
55. EFSA Panel on Dietetic Products Nutrition and Allergies (NDA). Scientific opinion on the essential composition of infant and follow-on formulae. EFSA J. (2014) 12:3760. doi: 10.2903/j.efsa.2014.3760
56. Romeu-Nadal M, Castellote AI, López-Sabater MC. Effect of cold storage on vitamins C and E and fatty acids in human milk. Food Chem. (2008) 106:65–70. doi: 10.1016/j.foodchem.2007.05.046
57. Ailhaud G, Massiera F, Weill P, Legrand P, Alessandri JM, Guesnet P. Temporal changes in dietary fats: role of n-6 polyunsaturated fatty acids in excessive adipose tissue development and relationship to obesity. Prog Lipid Res. (2006) 45:203–36. doi: 10.1016/j.plipres.2006.01.003
58. Siziba LP, Lorenz L, Stahl B, Mank M, Marosvölgyi T, Decsi T, et al. Changes in human milk fatty acid composition during lactation: the Ulm SPATZ health study. Nutrients. (2019) 11:2842. doi: 10.3390/nu11122842
59. Cruz-Hernandez C, Goeuriot S, Giuffrida F, Thakkar SK, Destaillats F. Direct quantification of fatty acids in human milk by gas chromatography. J Chromatogr A. (2013) 1284:174–9. doi: 10.1016/j.chroma.2013.01.094
60. Ellsworth L, Perng W, Harman E, Das A, Pennathur S, Gregg B. Impact of maternal overweight and obesity on milk composition and infant growth. Matern Child Nutr. (2020) 16:e12979. doi: 10.1111/mcn.12979
61. Thakkar SK, Giuffrida F, Cristina CH, De Castro CA, Mukherjee R, Tran LA, et al. Dynamics of human milk nutrient composition of women from Singapore with a special focus on lipids. Am J Hum Biol. (2013) 25:770–9. doi: 10.1002/ajhb.22446
62. Miliku K, Duan QL, Moraes TJ, Becker AB, Mandhane PJ, Turvey SE, et al. Human milk fatty acid composition is associated with dietary, genetic, sociodemographic, and environmental factors in the CHILD Cohort Study. Am J Clin Nutr. (2019) 110:1370–83. doi: 10.1093/ajcn/nqz229
63. Ntoumani E, Strandvik B, Sabel KG. Nervonic acid is much lower in donor milk than in milk from mothers delivering premature infants—of neglected importance? Prostaglandins Leukot Essent Fatty Acids. (2013) 89:241–4. doi: 10.1016/j.plefa.2013.06.005
Keywords: human milk, infant nutrition, macronutrients, plasmin, fatty acids
Citation: Meng F, Uniacke-Lowe T, Lanfranchi E, Meehan G, O'Shea C-A, Dennehy T, Ryan AC, Stanton C and Kelly AL (2023) A longitudinal study of fatty acid profiles, macronutrient levels, and plasmin activity in human milk. Front. Nutr. 10:1172613. doi: 10.3389/fnut.2023.1172613
Received: 23 February 2023; Accepted: 11 April 2023;
Published: 09 May 2023.
Edited by:
Luis Miguel Rodríguez-Alcalá, Universidade Católica Portuguesa, PortugalReviewed by:
Hans Demmelmair, Ludwig Maximilian University of Munich, GermanyLeilani Muhardi, FrieslandCampina, Netherlands
Copyright © 2023 Meng, Uniacke-Lowe, Lanfranchi, Meehan, O'Shea, Dennehy, Ryan, Stanton and Kelly. This is an open-access article distributed under the terms of the Creative Commons Attribution License (CC BY). The use, distribution or reproduction in other forums is permitted, provided the original author(s) and the copyright owner(s) are credited and that the original publication in this journal is cited, in accordance with accepted academic practice. No use, distribution or reproduction is permitted which does not comply with these terms.
*Correspondence: Alan L. Kelly, YS5rZWxseUB1Y2MuaWU=