- 1Oxford Centre for Diabetes, Endocrinology and Metabolism, University of Oxford, Oxford, United Kingdom
- 2Department of Experimental Medicine, Sapienza University of Rome, Rome, Italy
- 3National Institute for Health Research Oxford Biomedical Research Centre, John Radcliffe Hospital, Oxford, United Kingdom
Sleep disturbances are an emerging risk factor for metabolic diseases, for which the burden is particularly worrying worldwide. The importance of sleep for metabolic health is being increasingly recognized, and not only the amount of sleep plays an important role, but also its quality. In this review, we studied the evidence in the literature on macronutrients and their influence on sleep, focusing on the mechanisms that may lay behind this interaction. In particular, we focused on the effects of macronutrients on circadian and homeostatic processes of sleep in preclinical models, and reviewed the evidence of clinical studies in humans. Given the importance of sleep for health, and the role of circadian biology in healthy sleep, it is important to understand how macronutrients regulate circadian clocks and sleep homeostasis.
1. Introduction
1.1. Sleep disturbances and metabolic disturbances: an inseparable duo
The association between obesity and sleep has been increasingly studied over the last years. The duration of sleep has been put in relation to metabolic disturbances in many clinical studies. Metabolism and sleep mutually influence each other, in a complex relationship. Lack of sleep is associated to a higher body mass index (1–5) and to central obesity (4, 6–10). The recommended amount of sleep, according to the consensus statement of the American Academy of Sleep Medicine and Sleep Research Society, is at least 7 h per night on a regular basis (11).
Central obesity, which is characterized by visceral fat deposition, is a metabolic feature associated with a higher cardiovascular risk. Lack of sleep is associated to glucose metabolism impairment and type 2 diabetes (12, 13). A sleep duration longer of just 1 h per night is associated with a lower prevalence of obesity and type 2 diabetes (14, 15). Similarly, a reduction of just 1 h of sleep per night is associated with weight gain (1).
It is known that sleep restriction can stimulate appetite (16–18) and seeking for high calorie food (16, 19, 20). This is particularly evident in the conditions in which the sleep–wake cycle is disturbed, as in shift work (21). In fact, it may lead to an increased preference for calorie-dense foods (22), reduction in energy expenditure (23) and alterations in appetite-controlling hormones (23). On the other hand, food acts as an external regulator of the circadian rhythm of the individual, of which sleep is a major manifestation (24).
There is some evidence that the relationship between BMI and sleep duration is U-shaped, so that long or short sleep durations are both associated to increased adiposity (15, 25). Similarly, the relationship between sleep duration and impaired glucose tolerance or diabetes is U-shaped (12, 13), so that we may suppose that metabolic disturbances, whatever they are, are similarly interconnected with sleep duration, but that sleep duration cannot be considered as a linear variable. The variability in sleep duration can be itself considered a risk factor for metabolic alterations (26), diabetes (14) and adiposity parameters (4, 27, 28) at any age. Also sleep quality has its own importance. Given the fact that sleep is composed by quantitative features that are objectively measurable, as sleep latency and sleep duration, and some aspects that are mainly subjective and impossible to measure objectively, as depth and restfulness (29), sleep quality is a parameter that is difficult to define in its entirety. In fact, it is determined by the combination of many factors, as sleep duration, latency, fragmentation, and the perception of restorative sleep, which are strictly connected to the presence of daytime dysfunction. A higher degree of sleep fragmentation, calculated considering movement and immobile epochs with the actigraphy, is related to a shorter total sleep time and associated with obesity (25), and a reduced sleep efficiency is associated to central obesity in women (30).
On the other hand, obesity is a risk factor for developing sleep disturbances. Obesity can increase the risk of developing obstructive sleep apnoea (31), and the psychological and environmental implications of obesity can affect sleep quality, so that sleep disturbances and obesity sit in a vicious circle in which one worsen the other (32).
The mechanisms of the association between lack of sleep and metabolic disturbances are not clarified yet. Metabolic disturbances, included diabetes and non-alcoholic liver disease, are strictly connected among each other, and they similarly interact with lack of sleep. Metabolic disturbances are often associated to hormonal imbalances, which may play a role in their development, in a complex relationship of mutual influence (33–36). Some hormones may be involved in the association between metabolic disturbances and reduced sleep. The higher levels of cortisol due to the condition of chronic stress related to sleep deprivation may explain this relationship, through the increased levels of insulin resistance and inflammation (37). Moreover, the appetite regulation hormones are altered, and lead to an increased energy intake. Lack of sleep is associated to higher levels of pro-inflammatory cytokines and altered sympathetic activity, which lead not only to weight gain itself, but also to the development of a higher cardiovascular risk through the induction of endothelial dysfunction (32, 38).
1.2. Sleep structure and functioning
1.2.1. Sleep architecture
Sleep is a physiological element which is particularly important for health, and specifically, for metabolic and cardiovascular health. It is composed by a rapid-eye movement phase (REM) and non-REM phases. The non-REM phases include the phases N1 and N2, which are the “light sleep” phases, and N3 that is the “slow wave sleep” phase (SWS), that is a deep sleep phase. Both REM and slow-waves sleep contribute to the restorative function of sleep. Sleep stages succeed one after the other in cycles that last from 90 to 110 min. Each stage lasts differently: N1 last from 1 to 5 min, N2 from 10 to 60 min and SWS from 20 to 40 min, while REM phase lasts from 10 to 60 min. These lengths vary during the sleep, and REM length increases along the night, and the non-REM phase gets shorter. The majority of sleep is spent in N2 phase, and non-REM phase lasts about 75% of sleep. During a night, normally there are 4 o 5 sleep cycles, with the progression across sleep stages as N1, N2, N3, N2, REM (39).
1.2.2. Sleep components: the homeostatic and circadian processes
Four decades ago, Borbély proposed the two-process model of sleep (40): a conceptual model that has shaped the field of sleep research and is still the primary model used to describe sleep processes. This model describes the continuous interaction of two processes, termed process S and C, that are responsible for driving sleep and wake timing (see Figure 1). Process S, or the homeostatic process, represents sleep debt, which builds up during the wake phase and decreases during sleep. When it reaches its lowest value at the end of sleep, it triggers the body to awaken, and when it reaches its highest value after a long period of being awake, it triggers sleep. Meanwhile, process C represents the circadian process. This is controlled by the master circadian regulator, which resides within the Suprachiasmatic Nuclei (SCN). Process C oscillates across a day-night cycle, as entrained by external light cues, and controls the timing of sleep and arousal. The two components are interconnected and mutually influence each other to determine sleep propensity (40) in a complex relationship, not yet completely elucidated, that influences some characteristics of sleep, as length and depth; in fact, circadian amplitude may be reduced when sleep pressure increases (41). In physiological conditions, the two processes are harmoniously concordant, so that sleep onset is favored by both processes during the night, when it is dark, and sleep debt has accumulated during the day. Clock genes, the fundamental bricks of the circadian process, are also implicated in sleep homeostasis; the expression of some clock genes changes with wake and sleep phases and with sleep deprivation, and conversely sleep homeostasis is altered in many clock gene mutants (42).
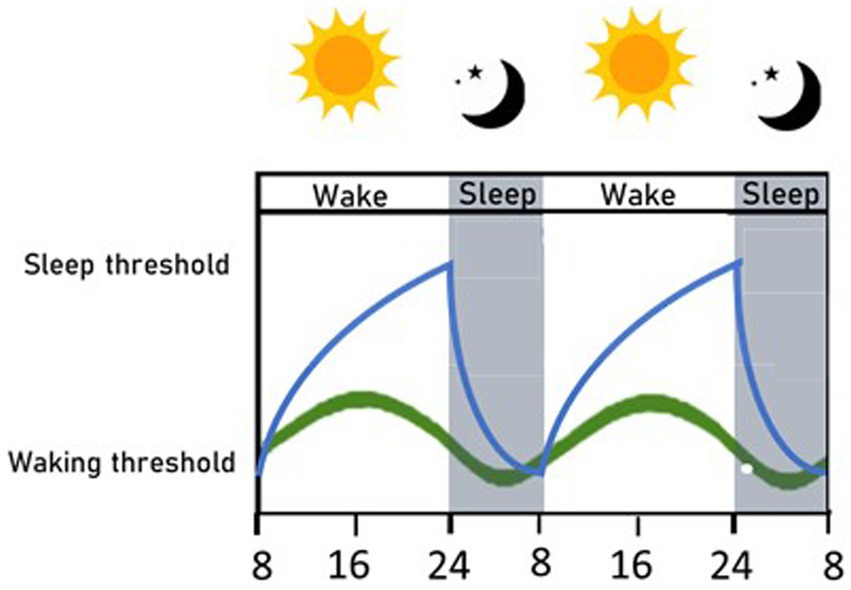
Figure 1. Circadian and homeostatic sleep processes. A simplified graphical representation of the two sleep processes, within a 2 days-time, in physiological conditions. The circadian process (green line) helps set the timing of sleep, and its fluctuations are regulated by the alternance of light and dark. The homeostatic process (blue line) is related to sleep debt, which accumulates while awake, and once the sleep threshold is reached, promotes sleep; then sleep debt decreases and awakening occurs when the waking threshold is reached. The two processes are interconnected and mutually interact, so that sleep is harmoniously regulated (40).
For the purpose of this review, we will focus on how macronutrients influence the sleep components.
2. Circadian process and macronutrients in pre-clinical models
2.1. The circadian clock and the role of dietary nutrients on its entrainment and function
The two-process model of sleep regulation describes the interaction of a homeostatic process S dependent on time spent asleep/awake, and process C which is controlled by the central circadian pacemaker (43). The ‘master’ circadian clock resides within the Suprachiasmatic Nuclei (SCN) which is located in the hypothalamus. The SCN receives direct light input from the retinal ganglia in order to ‘tell time’. In practice, this means that the master clock of the SCN is entrained by exposure to external light cues, and can shift by approximately 1 h per day in humans and mice. The period of the circadian clock in the SCN (the amount of time it takes to complete one cycle) is approximately 24 h in order to match the rotation of the Earth about its axis. The exact amount of time for one oscillation in the absence of external stimulation is known as the ‘free-running period’ and varies on average between species and individuals, and may be slightly more or less than 24 h. The core circadian clock controls 24-h cycles not only of sleep/wake, but also other physiological parameters, such as core body temperature and blood pressure, as well as secretion of hormones such as melatonin, cortisol, and prolactin.
At a molecular level, the circadian clock is composed of a transcription-translation feedback loop. The positive arm of this loop includes the proteins BMAL1 and CLOCK, which activate the transcription of the inhibitory PERIOD and CRYPTOCHROME (PER1-3 and CRY1&2) proteins. The oscillations are stabilized by an auxiliary loop including REVERB and ROR proteins. This molecular clock operates not only in the SCN but also in peripheral tissues and cell types, with signals from the central SCN pacemaker synchronizing the peripheral clocks (44). Peripheral clocks are influenced by feeding and fasting states and are kept synchronized with the central clock. In this physiological situation, light and dark cycles succeed harmoniously, in accordance with feeding and fasting status. However, peripheral clocks can become decoupled from the central clock, due to stimuli including, for instance, mis-timed feeding, i.e., eating out of synchrony with the biological clock, during normal physiological rest phase (45). This decoupling has been linked to the pathogenesis of a wide variety of diseases, including metabolic disorders. Both central and peripheral circadian clocks are extremely important for regulating the metabolism of macronutrients and maintaining energy homeostasis (46).
Metabolites from feeding play a role in regulating cellular rhythmicity, therefore diet composition play a role in influencing circadian clock activity and reprogram the clock in mice (47). The mechanisms through which nutrients impact circadian metabolome, and consequently biological rhythm, have not yet been fully elucidated and results are not completely concordant (see Figure 2). Research in animal models showed that different diets have a different impact on circadian phase.
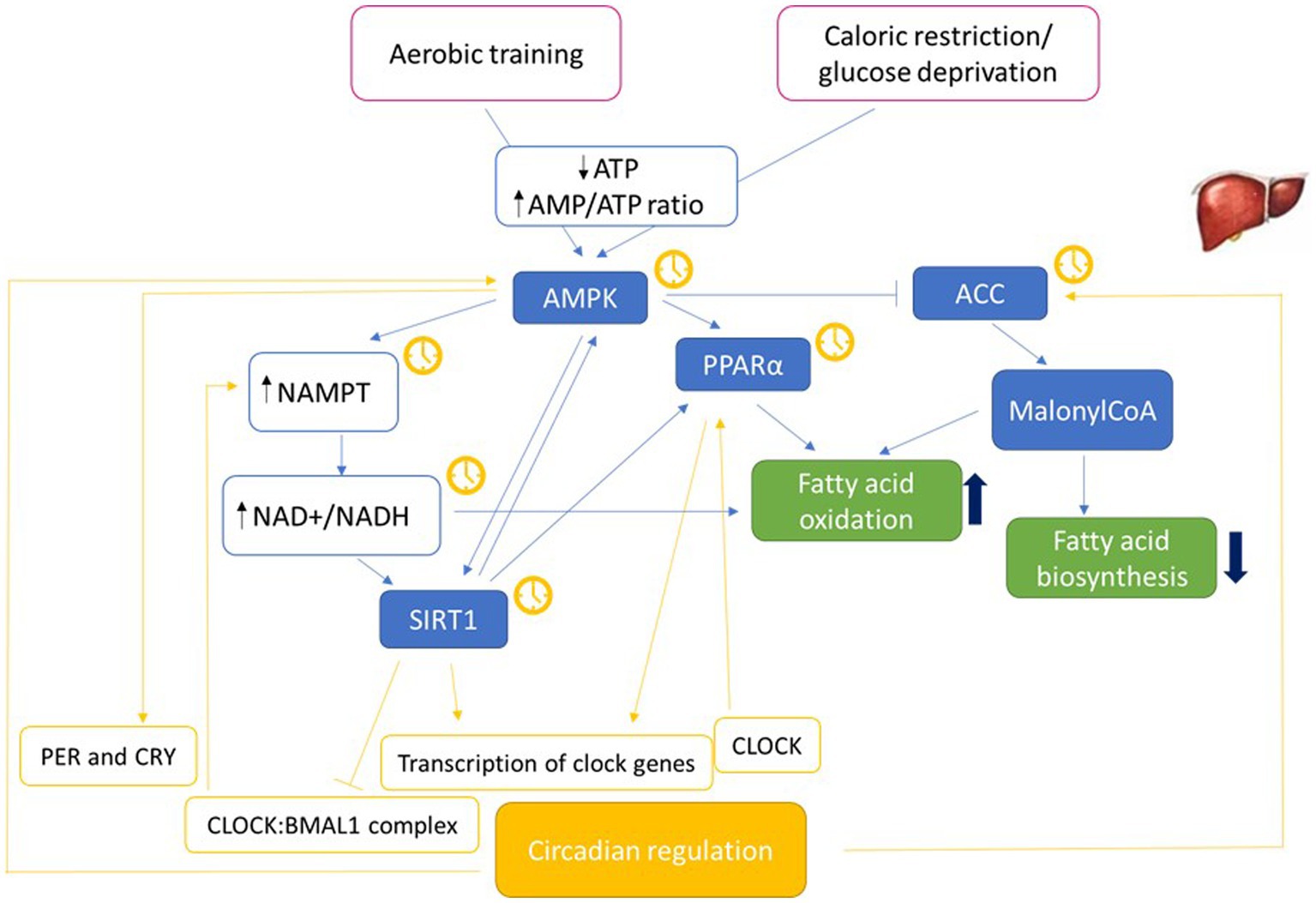
Figure 2. Molecular pathways possibly involving metabolic functions and circadian rhythm. Molecular pathways that may represent the connection between metabolism and circadian machinery (46, 71, 175–184). NAD+ acts as a cofactor for fatty acid oxidation and SIRT1 activity. The yellow clock indicates the molecules whose expression is circadian. Adenosine monophosphate-activated protein kinase (AMPK); Nicotinamide phosphoribosyltransferase (NAMPT); nicotinamide adenine dinucleotide (NAD+/NADH); Sirtuin 1 (SIRT1); Peroxisome proliferator-activated receptors (PPAR); Acetyl-CoA carboxylase (ACC).
Nutrients act mainly on the peripheral clocks, but some preliminary evidence suggest that food is able to directly interact with the central clock (48) (see Figure 3). During fasting conditions, the production of ghrelin from the stomach activated AgRP/NPY neurons, that project to the arcuate nucleus of the hypothalamus, and then connect to the CNS. Also the intergeniculate leaflet receives information form the periphery and is able to project to the CNS. Another mechanism could involve the pancreas, that in response to food intake produces pancreatic polypeptide that activate NPY6-receptor (Npy6r) that is coexpressed with vasoactive intestinal polypeptide (VIP) in the CNS of mice, and synchronizes CNS neurons. Leptin may be involved as well, since it has been observed that in vitro is able to forward shift the peak time of CNS and has a role in modulating the CNS response to light. Mendoza et al. (49) administered HF diet to mice and observed that the induction of the early gene c-fos by light in CNS was reduced, maybe through VIP signaling, so that it is possible hypothesize that a HF diet modifies circadian synchronization to light. c-fos is a regulatory protein found within the SCN, considered as a molecular marker of SCN resetting. The production of ghrelin, VIP and leptin is differently influenced by different macronutrients, as for example glucose markedly suppresses ghrelin concentration, medium-chain triglycerides reduce it less strongly, while amino acids determine a rise in ghrelin levels (50), therefore we may hypothesize that different macronutrients, through gastrointestinal hormones levels, differentially impact central clocks.
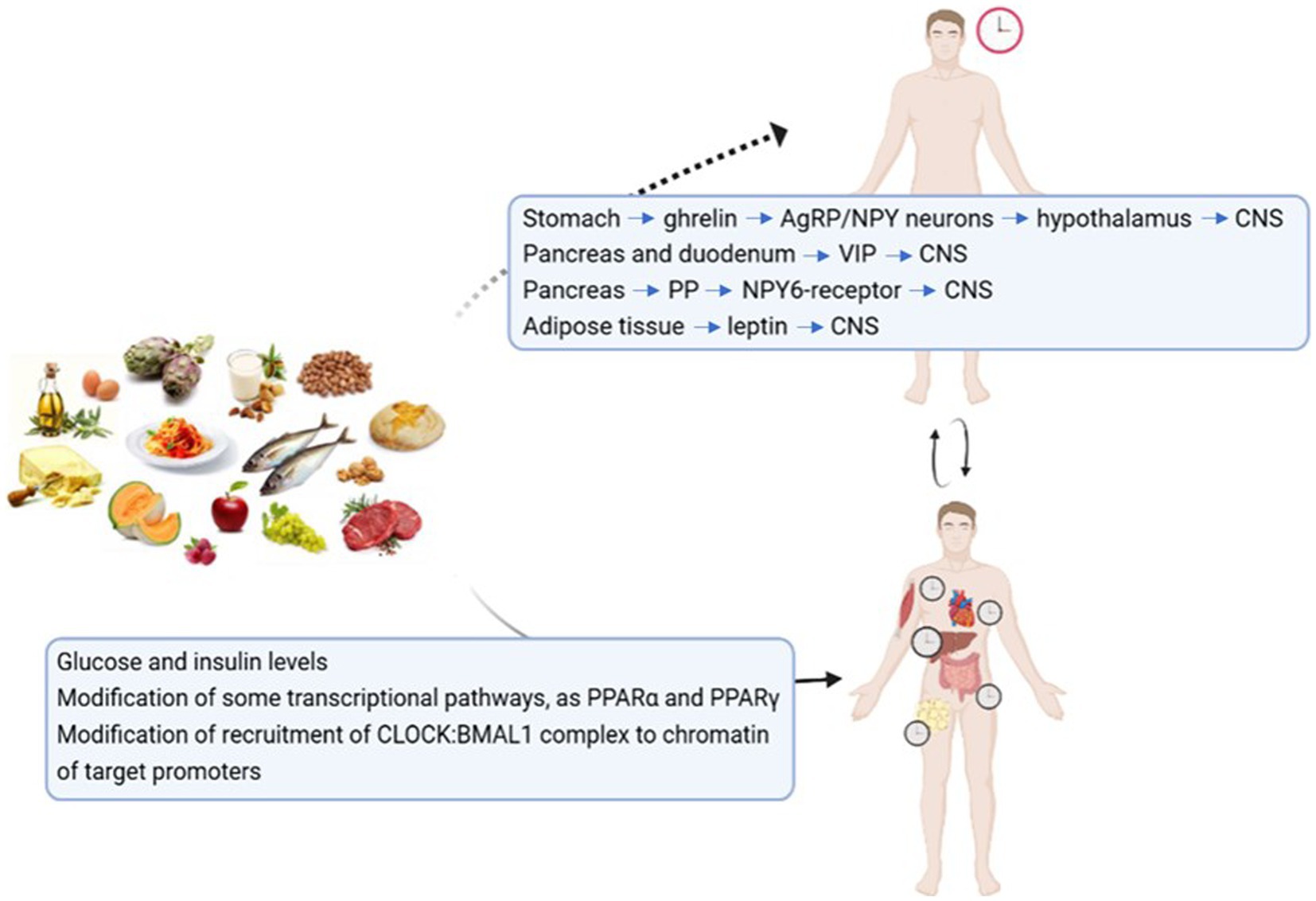
Figure 3. How food may affect the central and the peripheral clocks. Possible mechanisms through which nutrients may act on the central and the peripheral clocks (47–49, 185–187). Central Nervous System (CNS); Vasoactive intestinal Peptide (VIP); Pancreatic Polypeptide (PP); Peroxisome-Proliferator Activated Receptor (PPAR).
2.2. Impact of macronutrients on circadian process in pre-clinical models
2.2.1. Dietary fat effects on central and peripheral clocks
Experiments in mice have served as the main model for examination of the mechanisms of circadian clock function, and it is important to note that laboratory mice are naturally nocturnally active whereas humans are naturally diurnally active, i.e., the circadian active phase of mice is at night and the active phase of humans is during the day. Importantly, studies in mice have shown that the circadian phase of the SCN is not affected by restricting food intake to the rest phase, whereas the phase of peripheral organs, especially the liver, can be (51, 52). This observation indicates that circadian clocks in different tissues can become uncoupled, and this uncoupling, or internal desynchrony, has been associated with multiple human diseases (53). Examination of this phenomenon in mice using jet lag studies and genetic knockouts has shown a direct link between the circadian clock genes and metabolic disease phenotypes (54). Furthermore, disruption of the circadian clock by high-fat diet (HFD) and constant light have independent and additive effects on weight gain in mice, indicating that disrupted circadian rhythms potentiate the detrimental effects of high-fat diet (55).
The SCN receives metabolic feedback from peripheral organs, as evidenced by the observation that the free-running period of mice is altered by exposure to high fat diet (49). The induction of the gene c-fos by light was reduced in the SCN of mice fed high-fat diet, and this effect may be mediated by vasoactive intestinal peptide (VIP) and neuropeptide Y (NPY) (49). Metabolic entrainment of the SCN may also occur through the adipokine leptin, which can cause dose-dependent phase-shift of SCN tissue in vitro (56). Administration of exogenous leptin did not shift the behavioral circadian rhythm of mice in vivo, but did potentiate the effect of light-pulse induced phase-shift through induction of the clock genes Per1 and Per2 (57). Other studies have found that neurons from the arcuate nucleus and intergeniculate leaflet, which are sensitive to the appetite related hormone ghrelin, transmit signals to the SCN, and thus NPY and GABA may also provide metabolic feedback to the central circadian clock (58).
While dietary fat has been shown to exert some feedback on the central pacemaker, a much greater effect is observed on clocks in peripheral tissues. Clocks in peripheral metabolic tissues, such as adipose tissue, are hypothesized to regulate energy partition in response to regular daily feeding patterns to help maintain energy homeostasis. Therefore, timing of macronutrient intake may be of high importance, and indeed mice fed HFD at the end of the active phase exhibit greater adiposity than those fed HFD at the beginning of the active period. This phenomenon may be an effect of disturbed temporal regulation of β-oxidation (59). Other studies have shown that restriction of HFD to the rest phase leads to significantly higher weight gain than restriction of HFD to the active phase (60). Furthermore, restriction of HFD to active phase when compared to ad libitum HFD reduces body weight gain, increases glucose tolerance, and prevents the development of metabolic liver disease. This may be related to a restored efficiency in the pathways function of CREB, mTOR and AMPK, and improved oscillation of the clocks and their target genes (61). HFD significantly alters the rhythmic expression of clock genes in the liver, as well as clock output genes (62, 63) (see Figure 4). This includes, Pparα and Ampk which promote β-oxidation as part of the adiponectin signaling pathway, and disruption of their normal rhythmic expression may lead to impaired liver lipid metabolism. HFD determines the loss of rhythmic metabolite expression, such as NAD+, that parallels the dampened cyclic transcription of Nampt. These modifications in the rhythm of gene expression induced by the HFD may alter the circadian clock by disrupting, through a phase-shift or a reduction, of the recruitment of BMAL1: CLOCK to its target promoters on chromatin (47). At the same time, HFD determines oscillatory gain of normally non-rhythmic transcripts with known or predicted methyltransferase activity, such as Ehmt, Trmt2b, Whsc1, and Dph5. The transcriptional reprogramming by a HFD is related to the oscillation and chromatin recruitment of PPARγ, whose expression is induced by a HFD (47).
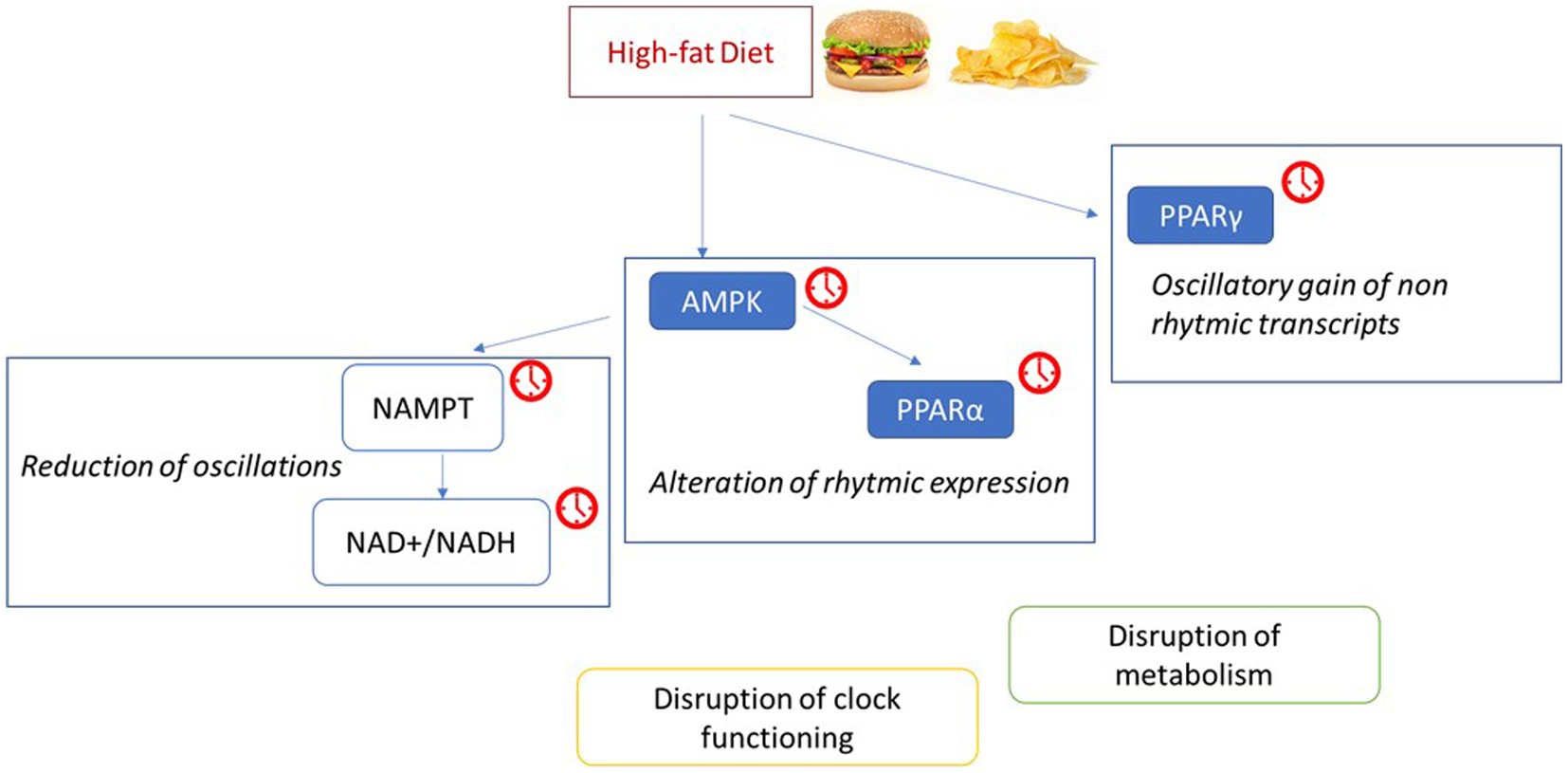
Figure 4. The high-fat diet induces a reprogramming of metabolic pathways and circadian rhythm. Possible mechanisms through which a high fat diet disrupts clock function and metabolism (47, 62, 188), inducing an altered or a phase shifted recruitment to chromatin of target genes by CLOCK:BMAL1. The red clocks indicate the molecules whose expression during the day is altered by the consumption of a high-fat diet. Adenosine monophosphate-activated protein kinase (AMPK); Nicotinamide phosphoribosyltransferase (NAMPT); nicotinamide adenine dinucleotide (NAD+/NADH); Peroxisome proliferator-activated receptors (PPAR).
2.2.2. Glucose effects on central and peripheral clocks
Glucose homeostasis is subject to circadian control, as surgical lesion of the SCN in rats abolishes the 24-h rhythm of basal blood glucose and insulin (64). But glucose may also exert a reciprocal effect on the circadian clock, and there is evidence of regulation in the SCN. Parenteral administration of glucose caused a phase-shift in SCN Per2 mRNA expression in rats (65). Furthermore, in vivo administration of insulin to induce hypoglycaemia in mice decreased the magnitude of light-pulse induced phase shifts, indicating that brain glucose levels are important for circadian entrainment (66).
In vitro, glucose has been shown to downregulate Per1 and Per2 gene expression in rat fibroblasts, possibly mediated by transforming growth factor β-inducible early gene 1 (Tieg1 (67, 68);. Conversely, insulin upregulates Per1 and Per2 gene expression in rat fibroblasts (69). Mice treated in vivo with streptozotocin to induce non-obese insulin resistance, showed alterations in hepatic expression of Bmal1, Per2, and Cry1, which were reversible by PPARα agonist pioglitazone (70). This might suggest that PPARα mediates the feedback of glucose homeostasis on to the core circadian clock. Indeed, multiple studies have shown that PPARα agonists change the rhythmic expression of core clock genes in multiple mouse tissues (71–74). Interestingly, PPARα agonist bezafibrate caused behavioral phase-advance in SCN-lesioned mice, indicating that the peripheral PPARα may be able to exert circadian control independently of the SCN master clock (75). Other potential mediators include the kinases Mapk and Gsk3, both of which are regulated by insulin signaling. Mapk can downregulate Bmal1, whereas GSK3 phosphorylates and thus degrades CRY2 (76, 77). The exact role of GSK3 in circadian entrainment is poorly understood, with opposing observations in different systems. Pharmacological inhibition and siRNA knockdown of GSK3 caused period lengthening in cultured mouse SCN and fibroblasts (78, 79). Whereas in cultured human U2OS cells, inhibitors and knockdown of GSK3 lead to period shortening (80). Another glucose sensing kinase, Ampk, can also phosphorylate and destabilize CRY1 which may add another layer of complexity to the feedback mechanisms between glucose and the circadian clock (81).
2.2.3. Dietary protein effects on central and peripheral clocks
Relatively less research has been conducted on the role of dietary protein in circadian clock entrainment. Most of the few research articles examining this have used “Essence of Chicken” (EC), which is a mixture of water-soluble substances derived from cooking whole chicken. Whilst EC contains many proteins and peptides, it will also contain many other water-soluble factors, and it is important to note that standardization is likely to be difficult to achieve. However, supplementary dietary protein has been shown to enhance the circadian oscillation of glucocorticoid secretion in mice (82), indicating a potential role for certain amino acids. In mice exposed to 12 weeks of light-induced circadian disruption, dietary EC supplementation was reported to decrease the time to reset clock genes in the pineal gland (83). Further examination of this model, which induces decoupling of hepatic clock genes (Per1, Cry1, Bmal1) and key metabolic regulators (mTOR, AMPK), and leads to liver injury, showed that dietary EC reduced markers of liver inflammation and improved synchronicity of clock genes in liver (84). It should be stressed that the role of specific proteins or amino acids cannot be delineated from other non-protein constituents of the dietary supplement in these studies. However, another study, showed that protein-only diet and/or amino acid (cysteine) administration, can elicit entrainment of liver clock via glucagon secretion and production of IGF-1 (85). Furthermore, an in vitro study demonstrated that exogenous methionine and arginine can alter the phosphorylation of mTOR and the expression of circadian clock proteins, offering a plausible mechanism for the in vivo observations mentioned above (86). Lastly, it is noteworthy that protein ingestion has differential effects on intestinal microbiota in different circadian phases, and intestinal microbiota may play a role in regulating peripheral circadian clocks (87).
3. Homeostatic process and macronutrients in pre-clinical models
3.1. Molecular mechanisms of process S
Unlike the rhythmic circadian process C, homeostatic process S is cumulative – with sleep debt accumulating to its maximal value while awake, and decreasing exponentially during sleep. Higher levels of sleep debt result in an increased intensity in sleep, affecting the amount of stimuli required to awaken (88–91). While the exact molecular mechanisms involved in process S have yet to be elucidated, current thinking in the field centers around the hypothesis that process S is driven by the build-up of neuro-modulating substances in the brain, as originally proposed by Feinberg et al. and Borbély et al. (92, 93).
The primary marker used to measure process S is the presence and amplitude of slow wave activity (SWA) as recorded by electroencephalogram (EEG). This is typically considered to be in the range of 0.5–4.0 Hz (43). SWA correlates almost perfectly with sleep debt: slowly increasing over the course of the waking period, peaking during early sleep, and declining over the course of sleep. SWA is caused by the rhythmic firing of cortical neurons, particularly during non-rapid eye movement (NREM) sleep, in which neurons synchronously switch between a bursting and resting phase. As sleep need decreases, synchrony is diminished and the SWA pattern is lost (94).
One of the proposed neuro-modulating substances responsible for driving homeostatic sleep debt is adenosine (95). This was first proposed by Benington and Heller, where they postulated that the role of sleep was to replenish depleted glycogen stores, and that SWA was caused by the release of extracellular adenosine by glycogen-depleted astrocytes (96). While this model has proved to be a very simplistic view of sleep (97), the proposed links to adenosine secretion have been verified by further studies (98). Adenosine is bound via the adenosine A1/A2 receptor in various parts of the brain and, interestingly, can trigger certain pathways typically involved in light-sensing via cAMP-CREB and ERK signaling (99). This may indicate a potential cross-over point for process C and process S. However, knockdown of adenosine A1 receptor has been described to disrupt SWA, a readout of homeostatic sleep drive, but not total sleep duration, which would typically be controlled by process C (100).
More recently, a phosphorylation-centric model of sleep debt has been proposed in which synaptic protein phosphorylation may be the neuro-modulator responsible for dictating sleep pressure (101). This is evidenced by the discovery of sleep-inducing kinases, including calmodulin-dependent protein kinase (CaMK) II (102), salt-inducible kinases (SIK)3 (103), and extracellular signal-regulated kinases (ERKs) (104), which increase in activity over the wake period and show elevated function during sleep deprivation. In addition, the synaptic phospho-proteome shows a peak cluster of phosphorylation events at sleep–wake transition periods that is independent from circadian control (105), as demonstrated by sleep deprivation studies. It is therefore plausible that phosphorylation events are a key molecular mechanism underpinning process S. Moreover, these sleep-inducing kinases are implicated in metabolic pathways as well. CaMKII is activated by exercise and influence lipid metabolism (106), SIK3 belongs to the AMP-activated protein kinase (AMPK) family, regulates cholesterol and bile acid metabolism and influence lipid metabolism (107, 108), while the MAPK/ERK pathway recently emerged as a key regulator of the Warburg effect, an aerobic glycolysis metabolic reprogramming occurring in cancer cell proliferation and some physiological processes (109).
There is a growing body of evidence that different macronutrients can affect various sleep parameters, including sleep intensity and sleep pressure. The next section of this review will focus on the impact of different macronutrient groups on the homeostatic component of sleep in pre-clinical models.
Circadian rhythm is tightly controlled and fundamental for the health of the living organism. In fact, the regulation of circadian rhythm is redundant, given the role of neuronal PAS domain protein 2 (NPAS2), which can functionally substitute CLOCK in the central clock in mice (110). NPAS2 is a conserved gene whose transcript can dimerize with BMAL1 and activate the transcription of genes related to circadian rhythm, as PER1/2/3 and CRY1/2 (111). Sleep deprivation reduces the binding of CLOCK, NPAS2 and BMAL1 to the promoters of target circadian genes. In particular, NPAS2 is able to induce sleep when the sleep need increase, even when it is not the time to sleep, therefore playing a major role in sleep homeostasis, probably acting at the thalamus and cortex, where it is more expressed (112). In addition, NPAS2 has also a metabolic role, since it boosts the Warburg effect in hepatocellular carcinoma cells through increasing the expression of glycolytic genes (111).
3.2. Impact of macronutrients on homeostatic component of sleep in pre-clinical models
3.2.1. Carbohydrates and homeostatic sleep
Carbohydrates are one of the best described macronutrients that affect sleep. In particular, it has been proposed that high levels of glucose result in the uptake of tryptophan (Trp) in the brain (113). Following ingestion of a high carbohydrate meal, insulin influences tryptophan supply to the brain by both promoting the binding of tryptophan to albumin, and at the same time reducing the levels of several other amino acid species that would usually compete with tryptophan for transport proteins into the brain (114). Tryptophan is a precursor for serotonin and ultimately melatonin, which is the primary sleep-promoting hormone, suggesting that carbohydrate intake could promote sleep pressure.
Unfortunately, few studies have been performed looking at the role of carbohydrates during sleep in rodent models. However, one paper found that glucose activates sleep-promoting neurons in the ventrolateral preoptic nucleus in mice (115), which are essential for the initiation and maintenance of SWA. The authors found that physiological levels of glucose led to the selective excitation of sleep-promoting neurons, which is normally gated by KATP channels. Induction of SWA is a marker of increased sleep pressure, and therefore suggests that glucose intake promotes sleep in mice. SWA has also been shown to regulate glycolytic metabolism directly (116). Measurement of lactate levels in real time reveal that lactate builds up during wakefulness and depletes during SWA sleep proportionally to the amplitude of SWA oscillations. Therefore, this suggests that SWA reduces the glycolytic rate of the cerebral cortex, and thus glucose metabolism is an essential part of homeostatic sleep.
The sleep modulating role of carbohydrates has been better studied in Drosophila, where response to sugar intake appears to be dose dependent. Sleep pathways between Drosophila and mammals are generally considered to be very highly conserved, with both exhibiting the two-process model described by Borbély et al. (43), therefore conclusions from Drosophila are likely to be relevant to human biology. Starvation in flies results in a dampening of sleep, leading to hyperactivity as they redirect their priorities to locating a new food source (117). This is conserved in mice, with food restriction promoting wakefulness and delayed onset of sleep during daytime (118). This can be rescued by detection of a sweet gustatory stimulus, promoting an induction of sleep and reduction in locomotor activity, such as feeding of low sucrose concentrations (119–121). This gustatory rescue of the starvation effect may be of interest in humans, for maintaining a good sleep in cases of food shortages and unstable environmental conditions (119). Interestingly, meals containing high sucrose concentrations (between 5 and 33%) have a polar effect, instead resulting in increased movement and delayed sleep (122). When maintained on a high sucrose diet over a long time period, flies show a reduction in number of sleep bouts but increased average sleep length compared to low-sucrose fed flies (123). As flies age, their sleep patterns fragment and sleep quality drops. Chronic feeding of high sucrose in aged flies has no effect on reduction of age-induced sleep fragmentation, however a controlled short exposure to a high sucrose feed can rescue this phenotype (124), suppressing sleep fragmentation in aged flies for a short time period, highlighting that the feeding of different macronutrients may be used as a treatment for certain types of sleep disruption. The effect of dietary sugar on sleep behavior is independent of circadian rhythm (123), as it is conserved even in models where circadian rhythm is disrupted, indicating that it primarily acts on the homeostatic sleep process.
Similarly to taste, also smell has a connection with sleep, as the perception of odors is reduced in some sleep stages and, conversely, odors can affect sleep, through the modulations of arousal and sleep latency, duration and quality. Moreover, sleep improves the odor memory (125). Some odors are related to ancestral mechanisms, so that the sleep of newborn infants is ameliorated by the smell of the mother. In rodents, the odor of food stimulates the awakening, which is stronger when the animal is food-deprived (125). The odor itself is able to determine a metabolic response in mice, so that increased or reduced smell ability is associated to modifications in body weight, probably due to modifications of energy expenditure, and not only to the modifications in food intake that may occur (126). Metabolism is strictly connected with aging and life span, therefore the connection between smell, metabolism and sleep may be of particular interest. If these findings are applicable to humans, which express much less olfactory receptor genes, and have a smaller olfactory bulb, is still to be elucidated.
3.2.2. Protein and homeostatic sleep
Consumption of protein has also been described to affect sleep architecture. In particular, addition of 2% yeast protein to the diet of drosophila resulted in increased locomotor behavior, reduced sleep duration, and lowered the arousal threshold required to awaken, indicating shallower sleep (122). However, this effect may depend on the specific ratio of carbohydrate: protein, as the addition of protein to a high sucrose diet instead promotes the consolidation of sleep in flies (123), reverting the sleep disruption normally observed in high sucrose diet fed flies. Therefore, it may be more useful to look at the relative composition of different macronutrients in a meal for therapeutic purposes, rather than study each macronutrient in isolation.
In mice, maternal diet composition plays a significant role on offspring health (127). Offspring born to murine dams fed a restricted protein diet display altered energy expenditure and disrupted sleep architecture compared to normal protein fed controls, despite being fed identical diets after weaning. Interestingly, these changes were independent from circadian machinery, and may indicate that protein consumption during gestation is particularly important for development of homeostatic sleep circuitry.
Tryptophan-rich proteins have a particular role in sleep promotion, as mentioned in the previous section. Consumption of the essential amino acid tryptophan directly facilitates sleep via the generation of melatonin (114). This is dependent upon tryptophan availability in the brain, which is controlled by the ratio of tryptophan to branched chain amino acids in circulation. This is aided by insulin signaling, which actively reduces the availability of branched chain amino acids and therefore promotes the uptake of tryptophan by transport proteins in the brain. However, melatonin appears to control sleep primarily via circadian factors, as it does not seem to alter sleep need as measured by sleep duration, and therefore this is unlikely to affect the homeostatic process (128). Some other authors observed that tryptophan has a direct effect on the homeostatic regulation of sleep. The re-feeding of rats after 4 days of fasting with a diet rich in α-lactalbumin was able to restore the time spent in SWS and wakefulness in just 1 day of refeeding, contrarily to the rats fed with a control-chow with lower amount of lactalbumin, who needed 4 to 6 days of refeeding to restore the alteration in the sleep architecture induced by the fasting (129). Probably, this happened through an increased transport of tryptophan through the brain–blood barrier, as lactalbumin has a higher Trp: large neutral amino acids (LNAA) ratio, and therefore the availability of brain serotonin increased. In fact, tryptophan is the serotonin precursor, through a short metabolic pathway of two enzymes, the tryptophan hydroxylase and the amino acid decarboxylase (130). Serotonin is a neurotransmitter implicated in the regulation of several behavioral and physiological function, as mood, cognition, but also sleep and wakefulness (130), and serves as a precursor for the synthesis of melatonin in the pineal gland during the night.
3.2.3. High fat diet and sleep need
The effects of lipid intake on sleep have primarily been studied in high fat diet obesity models, rather than isocaloric meals containing altered lipid proportions, so it is difficult to separate the effects of dietary fats from that of obesity-related behavioral changes. In mice, high fat diets have been shown to increase sleep pressure, reduce wakefulness, and fragment sleep, as supported by a significant increase in SWA during both wake and rest phases (131, 132). Alterations to fatty acid metabolism also alter sleep parameters. For example, ACADS, a component of the β-oxidation pathway, has been shown to affect rapid eye movement sleep, as its deficiency affects theta oscillations during sleep (133).
Fatty acid metabolism is also disrupted by high fat diets in drosophila (134), which may therefore have a downstream consequence on sleep. Interestingly, it has been found that medium- and long-chain dietary fatty acids, which range from approximately 6-10 carbons in length, promote sleep in flies (135), although their varying effects on sleep duration, number of sleep bouts, and activity during wakefulness vary massively. This may be as a result of differential oxidation of fatty acid species (136), as free fatty acids can be either used to produce Acetyl-CoA or stored as triglycerides, which may affect their relative effects on sleep architecture.
4. Clinical evidence in humans
The composition of the diet can influence both sleep quality and structure (137–146). Anyway, in humans, establishing the effects of a specific nutrient on sleep parameters is very complex, because they are not consumed isolated but within dietary patterns. A recent interesting review summarized many findings regarding the effects of macronutrients on sleep in humans (147).
Some studies observed that a healthy dietary pattern, with a large consumption of fruit, vegetables, fish and fibers, and regular meals, is associated to a better sleep quality (139, 148–150). The consumption of specific variety of cherries or their juice, which are rich in melatonin and serotonin, is often reported to be associated to a better sleep (151, 152). Vegetarians seem to have less sleep disorder, quantified with a lower Athens Insomnia Scale score (given by the sum of the scores for difficulty in sleep induction, awakenings during the night, early-morning awakening, total sleep time, overall quality of sleep and day functioning) and lower diurnal sleepiness, than non-vegetarians, and this is particularly evident in women (153). Anyway, the unprocessed red meat is not clearly related to a better or a poorer sleep (148). The Mediterranean diet, which is based on vegetables, fruit, whole grain and fish consumption, is associated with a better sleep quality, in particular with adequate sleep duration, reduced sleep latency, better sleep efficiency and reduced day dysfunction (154, 155).
Some studies reported that protein intake is associated to a better sleep quality and duration (142, 144–146, 156) but not all studies are completely concordant (138, 144, 145).
As already discussed, Trp is an essential amino acid that, after passing the brain blood barrier, is converted in serotonin and ultimately to melatonin, which promotes sleep. Sleep duration is positively associated with the dietary Trp: LNAA ratio (37). The reason for which this ratio among dietary intake of Trp and LNAA is related to sleep duration may be due to the fact that LNAA compete with Trp to bind to a carrier protein to pass the blood–brain barrier (157), influencing brain Trp bioavailability.
A study in more than 1,900 Japanese healthy adults observed an association between self-reported quality of sleep and self-assessed dietary habits. In terms of food, the intake of pulses, bread, fish and shellfish was correlated with sleep duration. Interestingly, these associations were present only in men, and not in women. The authors observed that sleep duration was directly related to the protein intake (140). A study in 104 healthy adults and older individuals from Singapore investigated the relationship among protein intake and sleep, focusing on tryptophan intake (37). Sleep duration was positively associated to dietary Trp: LNAA ratio but also to plant Trp and plant Trp: LNAA, and this interestingly suggest that the source of protein may play a role. Plant sources had a higher Trp:LNNA ratio, in comparison with animal sources, and moreover, they contain carbohydrates, which can increase plasma Trp:LNAA up to 50%. Dairy protein intake was negatively associated with sleep duration, despite its high content of tryptophan. Also sleep efficiency seemed to be negatively influenced by protein from diary sources, since people with a sleep efficiency of more than 85% ate less protein from diary sources in comparison with people with lower sleep efficiency (p = 0.033). This result may be related to the fact the dairy products contain not just alpha-lactalbumin, but also other proteins, which may increase the LNAA amount and therefore reduce the ratio. The different effect of the protein quality on sleep may also be related to the interaction of the gut microbiota with the peptide produced with the protein digestion, with the eventual development of dysbiosis and inflammation (150), but not all studies observed this different effect on sleep by proteins of different sources (146). Similarly, the ingestion of an evening whey protein supplementation, rich in tryptophan, did not improve sleep parameters in 15 elite male athletes. However, as authors point out, the high protein/energy intake snack did not negatively affect sleep, which is interesting for the importance of this kind of snacks for athlete recovery (158). Another study in 36 elite male football players observed that evening protein intake was associated with shortened sleep onset latency (159).
Tryptophan supplementation from non-diary sources is not invariably associated to an amelioration of sleep quality. In a recent study, 22 women with fibromyalgia were administered an eucaloric Mediterranean diet enriched with walnuts as source of tryptophan and magnesium for 16 weeks, but sleep quality did not ameliorate (160).
Data on carbohydrate intake and sleep are controversial. High- CHO (carbohydrate) diets may reduce the sleep onset latency (161). The glycaemic index may influence this parameter, as a study observed that the consumption of a high glycaemic index meal 4 h before bedtime shortened the sleep latency (113). Other sleep parameters were not affected. High-glycaemic index carbohydrates can increase the ratio tryptophan: LNAA through insulin action, which promotes the uptake of LNAA from muscles (162). Furthermore, the timing of carbohydrate intake plays a role, as the same high glycaemic index meal given 1 h before bedtime did not have a significant effect on sleep latency (113). On the other hand, another study reported no changes in sleep onset nor sleep efficiency, but a reduction in the restorative sleep in the first sleep cycle, after consumption of a high CHO meal 4 h before bedtime (163), and another one reported a reduced sleep quality associated to a high CHO intake (139). In fact, the consumption of higher percentage of energy from carbohydrates has been associated with arousals (137). A study in 32 female athletes reported an increase in wake after sleep onset and decrease of sleep efficiency along with increase in CHO intake, and on the contrary a decrease of sleep onset latency along with saturated fat intake (164).
In healthy adults administered a very low carbohydrate diet, the slow wave phase increased, and the rapid eye movement (REM) sleep decreased, in comparison to an isocaloric control mixed diet (165). Another study observed a reduction of sleepiness during a very low-calorie ketogenic diet during the reduced ketosis phase, but no evidence of modifications of other sleep parameters (166).
Regarding fat intake, less data is available. A short sleep is often associated to a higher fat intake (143), but this is not invariably observed (167). Conversely, saturated fats, which are commonly present in the Western diet, have a negative effect on sleep quality according to some studies. Ad libitum eating over 3 days, with a great intake of saturated fat and sugars, is associated to a less restorative sleep and increased latency (137). In particular, the percent of energy from saturated fat predicted a reduced SWS. On the other hand, other studies reported a better self-reported sleep with a high-fat diet (168), or a neutral effect (161, 163).
The effects on sleep of omega-3, of which fatty fishes are rich, and omega-6 long-chain polyunsaturated fatty acids, are not completely elucidated yet (169, 170). Fish consumption has been often associated to a better sleep quality evaluated with the Pittsburgh Sleep Quality Index Questionnaire and a longer sleep duration in men (139, 140).
As some interesting reviews and metanalysis recently pointed out, at the moment there are no striking evidence about the effects of a specific nutrient on sleep, and further research is warranted (150, 156, 171).
Conversely, deprivation of sleep can induce a modification in the pattern of the preferred foods. In fact, short sleepers, very short sleepers and individuals after a nightshift tend to eat less healthy food, increase their intake of saturated fats and carbohydrates, and consume less vegetables (20, 22). The mechanisms through which this happens may be related to a longer time of food availability, or research for gratification through calorie-dense food, or to higher energy needs to maintain the extended wakefulness, or to imbalances in appetite hormones (172).
The time of the administration of food may influence sleep and circadian rhythm. Time-restricted eating (TRE) consists in eating within a restricted time window during the day, and fast for the rest of the day. TRE is used mainly in obesity, since it favors weight loss and an amelioration of metabolic disturbances (173). Since time-restricted feeding (TRF) has been linked with an alignment of circadian rhythms in animal models, and the prolonged fasting stimulates SIRT-1 activity and ketone bodies production, which can influence circadian rhythm, it has been hypothesized that TRE may influence sleep. A recent review of human trials studied the effects of TRE on sleep in overweight and obese humans, trough questionnaires, self-reported diaries, and accelerometers (174). The authors reported that overall sleep quality did not change, sleep duration did not increase, nor insomnia was modified. Of note, the effects on sleep latency and efficiency did not align across studies. The insignificant impact on sleep quality may be attributed to the minimal weight loss occurred; the duration of sleep as well as the presence of insomnia remained unchanged probably due to the fact that participants in the trials were already sleeping for more than 7 h per night and did not suffer from insomnia. Therefore, TRE deserves more studies on larger samples of patients.
Some minerals and vitamins have been related to sleep duration and efficiency, in particular sodium, iron, zinc and vitamins D, B9, and B12 (37, 140, 164), but this topic goes beyond the aim of our review.
5. Conclusion
Dietary composition and sleep are bidirectionally related. Macronutrients influences sleep, and on the other hand, lack of sleep is related to the preferential choice of certain food. The mechanisms through which this mutual influence takes place, remain poorly defined. Both sleep processes C and S are involved. The role of circadian clocks in metabolic homoeostasis and the implications for health and disease are gradually being elucidated. However, besides regulating the metabolism of macronutrients, conversely the circadian clock is also regulated by macronutrients, both centrally and peripherally. Furthermore, regulation of peripheral circadian clocks can feed back on to the central pacemaker in the SCN.
Clinical studies have been inconclusive regarding the effects of a single macronutrient on sleep parameters in humans, probably also because the inevitable association of the macronutrients in dietary patterns.
The effects of food on sleep are not connected just to the food itself, but comprehend the response that its taste and its smell elicit in the organism. In fact, sweet taste is able to modify sleep and reduces sleep deprivation due to starvation, and the odors are able to influence sleep latency, duration and quality, and to induce a metabolic effect in animal models. These data may be of interest for ameliorating the management of patients suffering from sleep deprivation. If these results are translatable in humans, it has still to be determined.
Further research should be conducted to explore TRE as a tool for managing sleep disruption in humans: in obese patients, where the effect may be related to the weight loss achieved, but also in normal weight individuals suffering from sleep disturbances.
Further studies aimed to better understand the relationship between nutrients and sleep are needed, given the importance of sleep for metabolic health in humans.
Author contributions
EG: conceptualization. EG, MB, and MV: writing and original draft preparation. AL, LG, and DR: review and editing. All authors contributed to the article and approved the submitted version.
Funding
This research was funded by PRIN 2020 (grant no. 2020NCKXBR), Italian Ministry of Education, Universities and Research; co-funding of the European Union - Next Generation EU, Mission 4 Component 2 Investment 1.5, project Rome Technopole - code ECS 00000024 (CUP: B83C22002820006).
Conflict of interest
The authors declare that the research was conducted in the absence of any commercial or financial relationships that could be construed as a potential conflict of interest.
Publisher’s note
All claims expressed in this article are solely those of the authors and do not necessarily represent those of their affiliated organizations, or those of the publisher, the editors and the reviewers. Any product that may be evaluated in this article, or claim that may be made by its manufacturer, is not guaranteed or endorsed by the publisher.
References
1. Cappuccio, FP, Taggart, FM, Kandala, NB, Currie, A, Peile, E, Stranges, S, et al. Meta-analysis of short sleep duration and obesity in children and adults. Sleep. (2008) 31:619–26. doi: 10.1093/sleep/31.5.619
2. Xiao, Q, Gu, F, Caporaso, N, and Matthews, CE. Relationship between sleep characteristics and measures of body size and composition in a nationally-representative sample. BMC Obes. (2016) 3:1–8. doi: 10.1186/s40608-016-0128-y
3. Patel, SR, and Hu, FB. Short sleep duration and weight gain: a systematic review. Obesity. (2008) 16:643–53. doi: 10.1038/oby.2007.118
4. Ogilvie, RP, Redline, S, Bertoni, AG, Chen, X, Ouyang, P, Szklo, M, et al. Actigraphy measured sleep indices and adiposity:the multi-ethnic study of atherosclerosis (MESA). Sleep. (2016) 39:1701–8. doi: 10.5665/sleep.6096
5. Chaput, J, and Tremblay, A. Insufficient sleep as a contributor to weight gain:An update. Curr Obes Rep. (2012) 1:245–56. doi: 10.1007/s13679-012-0026-7
6. Sperry, SD, Scully, ID, Gramzow, RH, and Jorgensen, RS. Sleep duration and waist circumference in adults: a meta-analysis. Sleep. (2015) 38:1269–76. doi: 10.5665/sleep.4906
7. Kim, K, Shin, D, Jung, GU, Lee, D, and Park, SM. Association between sleep duration, fat mass, lean mass and obesity in Korean adults: the fourth and fifth Korea National Health and nutrition examination surveys. J Sleep Res. (2017) 26:453–60. doi: 10.1111/jsr.12504
8. Ford, ES, Li, C, Wheaton, AG, Chapman, DP, Perry, GS, and Croft, JB. Sleep duration and body mass index and waist circumference among us adults. Obesity. (2014) 22:598–607. doi: 10.1002/oby.20558
9. Potter, GDM, Cade, JE, and Hardie, LJ. Longer sleep is associated with lower BMI and favorable metabolic profiles in UK adults: findings from the National Diet and nutrition survey. PLoS One. (2017) 12:1–14. doi: 10.1371/journal.pone.0182195
10. Gangitano, E, Martinez-Sanchez, N, Bellini, MI, Urciuoli, I, Monterisi, S, Mariani, S, et al. Weight loss and sleep, current evidence in animal models and humans. Nutrients. (2023) 15:3431. doi: 10.3390/nu15153431
11. Watson, NF, Badr, MS, Belenky, G, Bliwise, DL, Buxton, OM, Buysse, D, et al. Recommended amount of sleep for a healthy adult: a joint consensus statement of the American Academy of sleep medicine and Sleep Research Society. JCSM. (2015) 11:591–2. doi: 10.5664/jcsm.4758
12. Chaput, JP, Després, JP, Bouchard, C, and Tremblay, A. Association of sleep duration with type 2 diabetes and impaired glucose tolerance. Diabetologia. (2007) 50:2298–304. doi: 10.1007/s00125-007-0786-x
13. Jackson, CL, Redline, S, Kawachi, I, and Hu, FB. Association between sleep duration and diabetes in black and white adults. Diabetes Care. (2013) 36:3557–65. doi: 10.2337/dc13-0777
14. Rosique-esteban, N, et al. Cross-sectional associations of objectively-measured sleep characteristics with obesity and type 2 diabetes in the PREDIMED-plus trial. Sleep J. (2018) 41:1–10. doi: 10.1093/sleep/zsy190
15. Chaput, JP, Bouchard, C, and Tremblay, A. Change in sleep duration and visceral fat accumulation over 6 years in adults. Obesity. (2014) 22:9–12. doi: 10.1002/oby.20701
16. Zhu, B, Shi, C, Park, CG, Zhao, X, and Reutrakul, S. Effects of sleep restriction on metabolism-related parameters in healthy adults: a comprehensive review and meta-analysis of randomized controlled trials. Sleep Med Rev. (2019) 45:18–30. doi: 10.1016/j.smrv.2019.02.002
17. McNeil, J, et al. The effects of partial sleep restriction and altered sleep timing on appetite and food reward. Appetite. (2017) 109:48–56. doi: 10.1016/j.appet.2016.11.020
18. Spiegel, K, Tasali, E, Penev, P, and Van Cauter, E. Brief communication: sleep curtailment in healthy young men is associated with decreased leptin levels, elevated ghrelin levels, and increased hunger and appetite. Ann Intern Med. (2004) 141:846–50.
19. Franckle, RL, et al. Insufficient sleep among elementary and middle school students is linked with elevated soda consumption and other unhealthy dietary behaviors. Prev Med. (2015) 74:36–41. doi: 10.1016/j.ypmed.2015.02.007
20. Simon, SL, Field, J, Miller, LE, DiFrancesco, M, and Beebe, DW. Sweet/dessert foods are more appealing to adolescents after sleep restriction. PLoS One. (2015) 10:4–11. doi: 10.1371/journal.pone.0115434
21. Broussard, JL, and Van Cauter, E. Disturbances of sleep and circadian rhythms: novel risk factors for obesity. Curr Opin Endocrinol Diabetes Obes. (2016) 23:353–9. doi: 10.1097/MED
22. Cain, S, Filtness, A, Phillips, C, and Anderson, C. Enhanced preference for high-fat foods following a simulated night shift. Scand J Work Environ Health. (2015) 41:288–93. doi: 10.5271/sjweh.3486
23. Mchill, AW, et al. Impact of circadian misalignment on energy metabolism during simulated nightshift work. Proc Natl Acad Sci U S A. (2014) 111:17302–7. doi: 10.1073/pnas.1412021111
24. Gangitano, E, Gnessi, L, Lenzi, A, and Ray, D. Chronobiology and metabolism: is ketogenic diet able to influence circadian rhythm? Front Neurosci. (2021) 15:1–8. doi: 10.3389/fnins.2021.756970
25. Van Den Berg, JF, et al. Actigraphic sleep duration and fragmentation are related to obesity in the elderly: the Rotterdam study. Int J Obes. (2008) 32:1083–90. doi: 10.1038/ijo.2008.57
26. Spruyt, AK, Molfese, DL, and Gonzal, D. Sleep duration, sleep regularity, body weight, and metabolic homeostasis in school-aged children. Pediatrics. (2011) 127:345–52. doi: 10.1542/peds.2010-0497
27. He, F, et al. Habitual sleep variability, mediated by nutrition intake, is associated with abdominal obesity in adolescents. Sleep Med. (2015) 16:1489–94. doi: 10.1016/j.sleep.2015.07.028
28. Kobayashi, D, Takahashi, O, Shimbo, T, Okubo, T, Arioka, H, and Fukui, T. High sleep duration variability is an independent risk factor for weight gain. Sleep Breath. (2013) 17:167–72. doi: 10.1007/s11325-012-0665-7
29. Buysse, DJ, Reynolds, CF, Monk, TH, Berman, SR, and Kupfer, DJ. The Pittsburgh sleep quality index: a new instrument for psychiatric practice and research. Psychiatry Res. (1989) 28:193–213. doi: 10.1016/0165-1781(89)90047-4
30. Mezick, EJ, Wing, RR, and McCaffery, JM. Associations of self-reported and actigraphy-assessed sleep characteristics with body mass index and waist circumference in adults: moderation by gender. Sleep Med. (2014) 15:64–70. doi: 10.1016/j.sleep.2013.08.784
31. Kuvat, N, Tanriverdi, H, and Armutcu, F. The relationship between obstructive sleep apnea syndrome and obesity: a new perspective on the pathogenesis in terms of organ crosstalk. Clin Respir J. (2020) 14:595–604. doi: 10.1111/crj.13175
32. Rodrigues, GD, Fiorelli, EM, Furlan, L, Montano, N, and Tobaldini, E. Obesity and sleep disturbances: the ‘chicken or the egg’ question. Eur J Intern Med. (2021) 92:11–6. doi: 10.1016/J.EJIM.2021.04.017
33. Gangitano, E, Ginanni Corradini, S, Lubrano, C, and Gnessi, L. La Non-Alcoholic Fatty Liver Disease, una patologia epatica di interesse endocrinologico. L’Endocrinologo. (2021) 5:436–40. doi: 10.1007/s40619-021-00955-9
34. Bellini, MI, et al. Nonalcoholic fatty liver disease and diabetes. World J Diabetes. (2022) 13:668–82. doi: 10.4239/wjd.v13.i9.668
35. Gangitano, E, Gnessi, L, and Merli, M. Protein catabolism and the dysregulation of energy intake-related hormones may play a major role in the worsening of malnutrition in hospitalized cirrhotic patients. Liver. (2022) 2:158–70. doi: 10.3390/livers2030014
36. Gangitano, E, et al. Growth hormone secretory capacity is associated with cardiac morphology and function in overweight and obese patients: a controlled, cross-sectional study. Cells. (2022) 11:2420. doi: 10.3390/cells11152420
37. Sutanto, CN, Loh, WW, Toh, DWK, Lee, DPS, and Kim, JE. Association between dietary protein intake and sleep quality in middle-aged and older adults in Singapore. Front Nutr. (2022) 9:1–10. doi: 10.3389/fnut.2022.832341
38. Tobaldini, E, et al. One night on-call: sleep deprivation affects cardiac autonomic control and inflammation in physicians. Eur J Intern Med. (2013) 24:664–70. doi: 10.1016/J.EJIM.2013.03.011
39. Patel, A. K., Reddy, V., and Araujo, J. F., (2022). “Physiology, sleep stages,” StatPearls, Available at: https://www.ncbi.nlm.nih.gov/books/NBK526132/ [Accessed: September 20, 2022]
41. Deboer, T., “Sleep homeostasis and the circadian clock: do the circadian pacemaker and the sleep homeostat influence each other’s functioning?,” Neurobiol Sleep Circ Rhythms, vol. 5. Elsevier Inc, pp. 68–77, 2018. doi: 10.1016/j.nbscr.2018.02.003.
42. Striz, M, and O’Hara, BF. Clock genes and sleep homeostasis: a fundamental link within the two-process model? Sleep. (2013) 36:301–2. doi: 10.5665/sleep.2430
43. Borbély, A, Daan, S, Wirz-Justice, A, and Deboer, T. The two-process model of sleep regulation: a reappraisal. J Sleep Res. (2016) 25:131–43. doi: 10.1111/jsr.12371
44. Mohawk, JA, Green, CB, and Takahashi, JS. Central and peripheral circadian clocks in mammals. Annu Rev Neurosci. (2012) 35:445–62. doi: 10.1146/annurev-neuro-060909-153128
45. Oosterman, JE, Wopereis, S, and Kalsbeek, A. The circadian clock, shift work, and tissue-specific insulin resistance. Endocrinology. (2020) 161:1–11. doi: 10.1210/endocr/bqaa180
46. Reinke, H, and Asher, G. Crosstalk between metabolism and circadian clocks. Nat Rev Mol Cell Biol. (2019) 20:227–41. doi: 10.1038/s41580-018-0096-9
47. Eckel-Mahan, KL, Patel, VR, de Mateo, S, Orozco-Solis, R, Ceglia, NJ, Sahar, S, et al. Reprogramming of the circadian clock by nutritional challenge. Cells. (2013) 155:1464–78. doi: 10.1016/j.cell.2013.11.034
48. Oosterman, JE, Kalsbeek, A, La Fleur, SE, and Belsham, DD. Impact of nutrients on circadian rhythmicity. Am J Phys Regul Integr Comp Phys. (2015) 308:R337–50. doi: 10.1152/ajpregu.00322.2014
49. Mendoza, J, Pévet, P, and Challet, E. High-fat feeding alters the clock synchronization to light. J Physiol. (2008) 586:5901–10. doi: 10.1113/jphysiol.2008.159566
50. Yin, X, Li, Y, Xu, G, An, W, and Zhang, W. Ghrelin fluctuation, what determines its production? Acta Biochim Biophys Sin Shanghai. (2009) 41:188–97. doi: 10.1093/abbs/gmp001
51. Damiola, F, Le Minh, N, Preitner, N, Fleury-olela, F, and Schibler, U. Restricted feeding uncouples circadian oscillators in peripheral tissues from the central pacemaker in the suprachiasmatic nucleus. Genes Dev. (2000) 14:2950–61. doi: 10.1101/gad.183500.moral
52. Stokkan, K-A, Yamazaki, S, Tei, H, Sakaki, Y, and Menaker, M. Entrainment of the circadian clock in the liver by feeding. Science. (2001) 291:490–3. doi: 10.1126/science.291.5503.490
53. Maidstone, RJ, Turner, J, Vetter, C, Dashti, HS, Saxena, R, Scheer, FAJL, et al. Night shift work is associated with an increased risk of asthma. Thorax. (2021) 76:53–60. doi: 10.1136/thoraxjnl-2020-215218
54. Kettner, NM, Voicu, H, Finegold, MJ, Coarfa, C, Sreekumar, A, Putluri, N, et al. Circadian homeostasis of liver metabolism suppresses Hepatocarcinogenesis. Cancer Cell. (2016) 30:909–24. doi: 10.1016/j.ccell.2016.10.007
55. Coomans, CP, Berg, SAA, Houben, T, Klinken, JB, Berg, R, Pronk, ACM, et al. Detrimental effects of constant light exposure and high-fat diet on circadian energy metabolism and insulin sensitivity. FASEB J. (2013) 27:1721–32. doi: 10.1096/fj.12-210898
56. Prosser, RA, and Bergeron, HE. Leptin phase-advances the rat suprachiasmatic circadian clock in vitro. Neurosci Lett. (2003) 336:139–42. doi: 10.1016/S0304-3940(02)01234-X
57. Mendoza, J, Lopez-Lopez, C, Revel, FG, Jeanneau, K, Delerue, F, Prinssen, E, et al. Dimorphic effects of leptin on the circadian and Hypocretinergic Systems of Mice. J Neuroendocrinol. (2011) 23:28–38. doi: 10.1111/j.1365-2826.2010.02072.x
58. Saderi, N, et al. The NPY intergeniculate leaflet projections to the suprachiasmatic nucleus transmit metabolic conditions. Neuroscience. (2013) 246:291–300. doi: 10.1016/j.neuroscience.2013.05.004
59. Bray, MS, and Young, ME. Regulation of fatty acid metabolism by cell autonomous circadian clocks: time to fatten up on information? J Biol Chem. (2011) 286:11883–9. doi: 10.1074/JBC.R110.214643
60. Arble, DM, Bass, J, Laposky, AD, Vitaterna, MH, and Turek, FW. Circadian timing of food intake contributes to weight gain. Obesity. (2009) 17:2100–2. doi: 10.1038/oby.2009.264
61. Hatori, M, Vollmers, C, Zarrinpar, A, DiTacchio, L, Bushong, EA, Gill, S, et al. Time-restricted feeding without reducing caloric intake prevents metabolic diseases in mice fed a high-fat diet. Cell Metab. (2012) 15:848–60. doi: 10.1016/j.cmet.2012.04.019
62. Barnea, M, Madar, Z, and Froy, O. High-fat diet delays and fasting advances the circadian expression of adiponectin signaling components in mouse liver. Endocrinology. (2009) 150:161–8. doi: 10.1210/en.2008-0944
63. Quagliarini, F, et al. Cistromic reprogramming of the diurnal glucocorticoid hormone response by high-fat diet. Mol Cell. (2019) 76:531–545.e5. doi: 10.1016/j.molcel.2019.10.007
64. la Fleur, SE, Kalsbeek, A, Wortel, J, and Buijs, RM. A suprachiasmatic nucleus generated rhythm in basal glucose concentrations. J Neuroendocrinol. (1999) 11:643–52. doi: 10.1046/j.1365-2826.1999.00373.x
65. Iwanaga, H, Yano, M, Miki, H, Okada, K, Azama, T, Takiguchi, S, et al. Per2 gene expressions in the suprachiasmatic nucleus and liver differentially respond to nutrition factors in rats. J Parenter Enter Nutr. (2005) 29:157–61. doi: 10.1177/0148607105029003157
66. Challet, E, Losee-Olson, S, and Turek, FW. Reduced glucose availability attenuates circadian responses to light in mice. Am J Phys Regul Integr Comp Phys. (1999) 276:R1063–70. doi: 10.1152/ajpregu.1999.276.4.R1063
67. Hirota, T, Kon, N, Itagaki, T, Hoshina, N, Okano, T, and Fukada, Y. Transcriptional repressor TIEG1 regulates Bmal1 gene through GC box and controls circadian clockwork. Genes Cells. (2010) 15:111–21. doi: 10.1111/j.1365-2443.2009.01371.x
68. Hirota, T, Okano, T, Kokame, K, Shirotani-Ikejima, H, Miyata, T, and Fukada, Y. Glucose Down-regulates Per1 and Per2mRNA levels and induces circadian gene expression in cultured Rat-1 fibroblasts. J Biol Chem. (2002) 277:44244–51. doi: 10.1074/jbc.M206233200
69. Balsalobre, A, Marcacci, L, and Schibler, U. Multiple signaling pathways elicit circadian gene expression in cultured Rat-1 fibroblasts. Curr Biol. (2000) 10:1291–4. doi: 10.1016/S0960-9822(00)00758-2
70. Yang, S-C, Tseng, H-L, and Shieh, K-R. Circadian-clock system in mouse liver affected by insulin resistance. Chronobiol Int. (2013) 30:796–810. doi: 10.3109/07420528.2013.766204
71. Canaple, L, Rambaud, J, Dkhissi-Benyahya, O, Rayet, B́, Tan, NS, Michalik, L, et al. Reciprocal regulation of brain and muscle Arnt-like protein 1 and peroxisome proliferator-activated receptor α defines a novel positive feedback loop in the rodent liver circadian clock. Mol Endocrinol. (2006) 20:1715–27. doi: 10.1210/me.2006-0052
72. Chen, L, and Yang, G. PPARs integrate the mammalian clock and energy metabolism. PPAR Res. (2014) 2014:1–6. doi: 10.1155/2014/653017
73. Gervois, P, Chopin-Delannoy, S, Fadel, A, Dubois, G, Kosykh, V, Fruchart, JC, et al. Fibrates increase human REV-ERBα expression in liver via a novel peroxisome proliferator-activated receptor response element. Mol Endocrinol. (1999) 13:400–9. doi: 10.1210/mend.13.3.0248
74. Oishi, K, Atsumi, GI, Sugiyama, S, Kodomari, I, Kasamatsu, M, Machida, K, et al. Disrupted fat absorption attenuates obesity induced by a high-fat diet in clock mutant mice. FEBS Lett. (2006) 580:127–30. doi: 10.1016/j.febslet.2005.11.063
75. Shirai, H, Oishi, K, Kudo, T, Shibata, S, and Ishida, N. PPARα is a potential therapeutic target of drugs to treat circadian rhythm sleep disorders. Biochem Biophys Res Commun. (2007) 357:679–82. doi: 10.1016/j.bbrc.2007.04.002
76. Sanada, K, Okano, T, and Fukada, Y. Mitogen-activated protein kinase phosphorylates and negatively regulates basic Helix-loop-Helix-PAS transcription factor BMAL1. J Biol Chem. (2002) 277:267–71. doi: 10.1074/jbc.M107850200
77. Harada, Y, Sakai, M, Kurabayashi, N, Hirota, T, and Fukada, Y. Ser-557-phosphorylated mCRY2 is degraded upon synergistic phosphorylation by glycogen synthase kinase-3β. J Biol Chem. (2005) 280:31714–21. doi: 10.1074/jbc.M506225200
78. Kaladchibachi, SA, Doble, B, Anthopoulos, N, Woodgett, JR, and Manoukian, AS. Glycogen synthase kinase 3, circadian rhythms, and bipolar disorder: a molecular link in the therapeutic action of lithium. J Circadian Rhythms. (2007) 5:3. doi: 10.1186/1740-3391-5-3
79. Abe, M, Herzog, ED, and Block, GD. Lithium lengthens the circadian period of individual suprachiasmatic nucleus neurons. Neuroreport. (2000) 11:3261–4. doi: 10.1097/00001756-200009280-00042
80. Hirota, T, Lewis, WG, Liu, AC, Lee, JW, Schultz, PG, and Kay, SA. A chemical biology approach reveals period shortening of the mammalian circadian clock by specific inhibition of GSK-3β. Proc Natl Acad Sci. (2008) 105:20746–51. doi: 10.1073/pnas.0811410106
81. Lamia, KA, Sachdeva, UM, DiTacchio, L, Williams, EC, Alvarez, JG, Egan, DF, et al. AMPK regulates the circadian clock by Cryptochrome phosphorylation and degradation. Science. (2009) 326:437–40. doi: 10.1126/science.1172156
82. Dilixiati, A, Koyanagi, S, Kusunose, N, Matsunaga, N, and Ohdo, S. Dietary supplementation with essence of chicken enhances daily oscillations in plasma glucocorticoid levels and behavioral adaptation to the phase-shifted environmental light–dark cycle in mice. J Pharmacol Sci. (2017) 134:211–7. doi: 10.1016/j.jphs.2017.07.004
83. Wu, T, Watanabe, H, Hong, LK, Abe, K, Ni, Y, and Fu, Z. Effect of BRAND’s essence of chicken on the resetting process of circadian clocks in rats subjected to experimental jet lag. Mol Biol Rep. (2011) 38:1533–40. doi: 10.1007/s11033-010-0261-5
84. Wu, T, Yao, C, Tsang, F, Huang, L, Zhang, W, Jiang, J, et al. Facilitated physiological adaptation to prolonged circadian disruption through dietary supplementation with essence of chicken. Chronobiol Int. (2015) 32:1458–68. doi: 10.3109/07420528.2015.1105252
85. Ikeda, Y, et al. Glucagon and/or IGF-1 production regulates resetting of the liver circadian clock in response to a protein or amino acid-only diet. EBioMedicine. (2018) 28:210–24. doi: 10.1016/j.ebiom.2018.01.012
86. Hu, L, Chen, Y, Cortes, IM, Coleman, DN, Dai, H, Liang, Y, et al. Supply of methionine and arginine alters phosphorylation of mechanistic target of rapamycin (mTOR), circadian clock proteins, and α-s1-casein abundance in bovine mammary epithelial cells. Food Funct. (2020) 11:883–94. doi: 10.1039/C9FO02379H
87. Tamura, K, Sasaki, H, Shiga, K, Miyakawa, H, and Shibata, S. The timing effects of soy protein intake on mice gut microbiota. Nutrients. (2019) 12:87. doi: 10.3390/nu12010087
88. Williams, HL, Hammack, JT, Daly, RL, Dement, WC, and Lubin, A. Responses to auditory stimulation, sleep loss and the eeg stages of sleep. Electroencephalogr Clin Neurophysiol. (1964) 16:269–79. doi: 10.1016/0013-4694(64)90109-9
89. Ferrara, M. Auditory arousal thresholds after selective slow-wave sleep deprivation. Clin Neurophysiol. (1999) 110:2148–52. doi: 10.1016/S1388-2457(99)00171-6
90. Neckelmann, D, and Ursin, R. Sleep stages and EEG power Spectrum in relation to acoustical stimulus arousal threshold in the rat. Sleep. (1993) 16:467–77. doi: 10.1093/SLEEP/16.5.467
91. Ermis, U, Krakow, K, and Voss, U. Arousal thresholds during human tonic and phasic REM sleep. J Sleep Res. (2010) 19:400–6. doi: 10.1111/j.1365-2869.2010.00831.x
92. Feinberg, I, March, JD, Fein, G, Floyd, TC, Walker, JM, and Price, L. Period and amplitude analysis of 0.5–3c/sec activity in NREM sleep of young adults. Electroencephalogr Clin Neurophysiol. (1978) 44:202–13. doi: 10.1016/0013-4694(78)90266-3
93. Borbély, AA, Baumann, F, Brandeis, D, Strauch, I, and Lehmann, D. Sleep deprivation: effect on sleep stages and EEG power density in man. Electroencephalogr Clin Neurophysiol. (1981) 51:483–93. doi: 10.1016/0013-4694(81)90225-X
94. Vyazovskiy, VV, Olcese, U, Lazimy, YM, Faraguna, U, Esser, SK, Williams, JC, et al. Cortical firing and sleep homeostasis. Neuron. (2009) 63:865–78. doi: 10.1016/J.NEURON.2009.08.024
95. Peng, W, Wu, Z, Song, K, Zhang, S, Li, Y, and Xu, M. Regulation of sleep homeostasis mediator adenosine by basal forebrain glutamatergic neurons. Science. (2020) 369. doi: 10.1126/SCIENCE.ABB0556
96. Benington, JH, and Craig Heller, H. Restoration of brain energy metabolism as the function of sleep. Prog Neurobiol. (1995) 45:347–60. doi: 10.1016/0301-0082(94)00057-O
97. Petit, JM, Burlet-Godinot, S, Magistretti, PJ, and Allaman, I. Glycogen metabolism and the homeostatic regulation of sleep. Metab Brain Dis. (2015) 30:263–79. doi: 10.1007/S11011-014-9629-X
98. Pascual, O., et al., “Astrocytic purinergic signaling coordinates synaptic networks,” Science, vol. 310, no., pp. 113–116, Oct. 2005, doi: 10.1126/SCIENCE.1116916
99. Jagannath, A, Varga, N, Dallmann, R, Rando, G, Gosselin, P, Ebrahimjee, F, et al. Adenosine integrates light and sleep signalling for the regulation of circadian timing in mice. Nat Commun. (2021) 12:2113. doi: 10.1038/s41467-021-22179-z
100. Bjorness, TE, Kelly, CL, Gao, T, Poffenberger, V, and Greene, RW. Control and function of the homeostatic sleep response by adenosine A1 receptors. J Neurosci. (2009) 29:1267–76. doi: 10.1523/JNEUROSCI.2942-08.2009
101. Ode, K. L., and Ueda, H. R., “Phosphorylation hypothesis of sleep,” Front Psychol, vol. 11, p.:2700, Oct. 2020, doi: 10.3389/FPSYG.2020.575328/BIBTEX.
102. Tatsuki, F, Sunagawa, GA, Shi, S, Susaki, EA, Yukinaga, H, Perrin, D, et al. Involvement of ca(2+)-dependent hyperpolarization in sleep duration in mammals. Neuron. (2016) 90:70–85. doi: 10.1016/J.NEURON.2016.02.032
103. Funato, H, Miyoshi, C, Fujiyama, T, Kanda, T, Sato, M, Wang, Z, et al. Forward-genetics analysis of sleep in randomly mutagenized mice. Nature. (2016) 539:378–83. doi: 10.1038/nature20142
104. Vanderheyden, WM, Gerstner, JR, Tanenhaus, A, Yin, JC, and Shaw, PJ. ERK phosphorylation regulates sleep and plasticity in Drosophila. PLoS One. (2013) 8:1–14. doi: 10.1371/JOURNAL.PONE.0081554
105. Brüning, F, Noya, SB, Bange, T, Koutsouli, S, Rudolph, JD, Tyagarajan, SK, et al. Sleep-wake cycles drive daily dynamics of synaptic phosphorylation. Science. (2019) 366. doi: 10.1126/science.aav3617
106. Joseph, JS, Anand, K, Malindisa, ST, and Fagbohun, OF. Role of CaMKII in the regulation of fatty acids and lipid metabolism. Diabetes Metab Syndr Clin Res Rev. (2021) 15:589–94. doi: 10.1016/J.DSX.2021.02.037
107. Sun, Z, Jiang, Q, Li, J, and Guo, J. The potent roles of salt-inducible kinases (SIKs) in metabolic homeostasis and tumorigenesis. Signal Transduct Target Ther. (2020) 5:150. doi: 10.1038/s41392-020-00265-w
108. Uebi, T, Itoh, Y, Hatano, O, Kumagai, A, Sanosaka, M, Sasaki, T, et al. Involvement of SIK3 in glucose and lipid homeostasis in mice. PLoS One. (2012) 7:e37803. doi: 10.1371/journal.pone.0037803
109. Papa, S, Choy, PM, and Bubici, C. The ERK and JNK pathways in the regulation of metabolic reprogramming. Oncogene. (2019) 38:2223–40. doi: 10.1038/s41388-018-0582-8
110. DeBruyne, JP, Weaver, DR, and Reppert, SM. CLOCK and NPAS2 have overlapping roles in the suprachiasmatic circadian clock. Nat Neurosci. (2007) 10:543–5. doi: 10.1038/nn1884
111. Peng, LU, Bai, G, and Pang, Y. Roles of NPAS2 in circadian rhythm and disease. Acta Biochim Biophys Sin Shanghai. (2021) 53:1257–65. doi: 10.1093/abbs/gmab105
112. Franken, P, Dudley, CA, Estill, SJ, Barakat, M, Thomason, R, O’Hara, BF, et al. NPAS2 as a transcriptional regulator of non-rapid eye movement sleep: genotype and sex interactions. Proc Natl Acad Sci. (2006) 103:7118–23. doi: 10.1073/pnas.0602006103
113. Afaghi, A, O’Connor, H, and Chow, CM. High-glycemic-index carbohydrate meals shorten sleep onset. Am J Clin Nutr. (2007) 85:426–30. doi: 10.1093/ajcn/85.2.426
114. Daniel, PM, Love, ER, Moorhouse, SR, and Pratt, OE. The effect of insulin upon the influx of tryptophan into the brain of the rabbit. J Physiol. (1981) 312:551–62. doi: 10.1113/jphysiol.1981.sp013643
115. Varin, C, Rancillac, A, Geoffroy, H, Arthaud, S, Fort, P, and Gallopin, T. Glucose induces slow-wave sleep by exciting the sleep-promoting neurons in the ventrolateral preoptic nucleus: a new link between sleep and metabolism. J Neurosci. (2015) 35:9900–11. doi: 10.1523/JNEUROSCI.0609-15.2015
116. Wisor, JP, Rempe, MJ, Schmidt, MA, Moore, ME, and Clegern, WC. Sleep slow-wave activity regulates cerebral glycolytic metabolism. Cereb Cortex. (2013) 23:1978–87. doi: 10.1093/CERCOR/BHS189
117. Keene, AC, Duboué, ER, McDonald, DM, Dus, M, Suh, GSB, Waddell, S, et al. Clock and cycle limit starvation-induced sleep loss in Drosophila. Curr Biol. (2010) 20:1209–15. doi: 10.1016/j.cub.2010.05.029
118. Hua, R, Wang, X, Chen, X, Wang, X, Huang, P, Li, P, et al. Calretinin neurons in the midline thalamus modulate starvation-induced arousal. Curr Biol. (2018) 28:3948–3959.e4. doi: 10.1016/j.cub.2018.11.020
119. Linford, NJ, Ro, J, Chung, BY, and Pletcher, SD. Gustatory and metabolic perception of nutrient stress in Drosophila. Proc Natl Acad Sci U S A. (2015) 112:2587–92. doi: 10.1073/PNAS.1401501112/SUPPL_FILE/PNAS.201401501SI.PDF
120. Yang, Z, Yu, Y, Zhang, V, Tian, Y, Qi, W, and Wang, L. Octopamine mediates starvation-induced hyperactivity in adult Drosophila. Proc Natl Acad Sci U S A. (2015) 112:5219–24. doi: 10.1073/PNAS.1417838112
121. Hasegawa, T, Tomita, J, Hashimoto, R, Ueno, T, Kume, S, and Kume, K. Sweetness induces sleep through gustatory signalling independent of nutritional value in a starved fruit fly. Sci Rep. (2017) 7:14355. doi: 10.1038/s41598-017-14608-1
122. Catterson, JH, Knowles-Barley, S, James, K, Heck, MMS, Harmar, AJ, and Hartley, PS. Dietary modulation of Drosophila sleep-wake behaviour. PLoS One. (2010) 5:e12062. doi: 10.1371/journal.pone.0012062
123. Linford, NJ, Chan, TP, and Pletcher, SD. Re-patterning sleep architecture in Drosophila through gustatory perception and nutritional quality. PLoS Genet. (2012) 8:e1002668. doi: 10.1371/JOURNAL.PGEN.1002668
124. Lee, SH, and Kim, EY. Short-term maintenance on a high-sucrose diet alleviates aging-induced sleep fragmentation in drosophila. Anim Cells Syst. (2021) 25:377–86. doi: 10.1080/19768354.2021.1997801
125. Gaeta, G, and Wilson, DA. Reciprocal relationships between sleep and smell. Front Neural Circuits. (2022) 16:1076354. doi: 10.3389/fncir.2022.1076354
126. Garrison, JL, and Knight, ZA. Linking smell to metabolism and aging. Science. (2017) 358:718–9. doi: 10.1126/science.aao5474
127. Crossland, RF, Balasa, A, Ramakrishnan, R, Mahadevan, SK, Fiorotto, ML, and van den Veyver, IB. Chronic maternal low-protein diet in mice affects anxiety, night-time energy expenditure and sleep patterns, but not circadian rhythm in male offspring. PLoS One. (2017) 12:e0170127. doi: 10.1371/journal.pone.0170127
128. Rajaratnam, SMW, Middleton, B, Stone, BM, Arendt, J, and Dijk, DJ. Melatonin advances the circadian timing of EEG sleep and directly facilitates sleep without altering its duration in extended sleep opportunities in humans. J Physiol. (2004) 561:339–51. doi: 10.1113/JPHYSIOL.2004.073742
129. Minet-Ringuet, J, Le Ruyet, PM, Tomé, D, and Even, PC. A tryptophan-rich protein diet efficiently restores sleep after food deprivation in the rat. Behav Brain Res. (2004) 152:335–40. doi: 10.1016/J.BBR.2003.10.018
130. Silber, BY, and Schmitt, JAJ. Effects of tryptophan loading on human cognition, mood, and sleep. Neurosci Biobehav Rev. (2010) 34:387–407. doi: 10.1016/J.NEUBIOREV.2009.08.005
131. Jenkins, JB, Omori, T, Guan, Z, Vgontzas, AN, Bixler, EO, and Fang, J. Sleep is increased in mice with obesity induced by high-fat food. Physiol Behav. (2006) 87:255–62. doi: 10.1016/j.physbeh.2005.10.010
132. Panagiotou, M, and Deboer, T. Chronic high-caloric diet accentuates age-induced sleep alterations in mice. Behav Brain Res. (2019) 362:131–9. doi: 10.1016/J.BBR.2019.01.017
133. Tafti, M, Petit, B, Chollet, D, Neidhart, E, de Bilbao, F, Kiss, JZ, et al. Deficiency in short-chain fatty acid beta-oxidation affects theta oscillations during sleep. Nat Genet. (2003) 34:320–5. doi: 10.1038/NG1174
134. Heinrichsen, ET, Zhang, H, Robinson, JE, Ngo, J, Diop, S, Bodmer, R, et al. Metabolic and transcriptional response to a high-fat diet in Drosophila melanogaster. Mol Metab. (2014) 3:42–54. doi: 10.1016/j.molmet.2013.10.003
135. Pamboro, ELS, Brown, EB, and Keene, AC. Dietary fatty acids promote sleep through a taste-independent mechanism. Genes Brain Behav. (2020) 19:e12629. doi: 10.1111/GBB.12629
136. DeLany, JP, Windhauser, MM, Champagne, CM, and Bray, GA. Differential oxidation of individual dietary fatty acids in humans. Am J Clin Nutr. (2000) 72:905–11. doi: 10.1093/ajcn/72.4.905
137. St-Onge, M-P, Roberts, A, Shechter, A, and Choudhury, AR. Fiber and saturated fat are associated with sleep arousals and slow wave sleep. J Clin Sleep Med. (2016) 12:19–24. doi: 10.5664/jcsm.5384
138. Tanaka, E, Yatsuya, H, Uemura, M, Murata, C, Otsuka, R, Toyoshima, H, et al. Associations of protein, fat, and carbohydrate intakes with insomnia symptoms among middle-aged Japanese workers. J Epidemiol. (2013) 23:132–8. doi: 10.2188/jea.JE20120101
139. Katagiri, R, Asakura, K, Kobayashi, S, Suga, H, and Sasaki, S, the Three‐generation Study of Women on Diets and Health Study Group. Low intake of vegetables, high intake of confectionary, and unhealthy eating habits are associated with poor sleep quality among middle-aged female Japanese workers. J Occup Health. (2014) 56:359–68. doi: 10.1539/joh.14-0051-OA
140. Komada, Y, Narisawa, H, Ueda, F, Saito, H, Sakaguchi, H, Mitarai, M, et al. Relationship between self-reported dietary nutrient intake and self-reported sleep duration among Japanese adults. Nutrients. (2017) 9:1–10. doi: 10.3390/nu9020134
141. St-Onge, M-P, Mikic, A, and Pietrolungo, CE. Effects of diet on sleep quality. Am Soc Nut. (2016) 7:938–49. doi: 10.3945/an.116.012336.SOL
142. Zadeh, SS, and Begum, K. Comparison of nutrient intake by sleep status in selected adults in Mysore, India. Nutr Res Pract. (2011) 5:230–5. doi: 10.4162/nrp.2011.5.3.230
143. Santana, AA, Pimentel, GD, Romualdo, M, Oyama, LM, Santos, RVT, Pinho, RA, et al. Sleep duration in elderly obese patients correlated negatively with intake fatty. Lipids Health Dis. (2012) 11:1–6. doi: 10.1186/1476-511X-11-99
144. Grandner, MA, Jackson, N, Gerstner, JR, and Knutson, KL. Dietary nutrients associated with short and long sleep duration. Data from a nationally representative sample. Appetite. (2013) 64:71–80. doi: 10.1016/j.appet.2013.01.004
145. Yamaguchi, M, Uemura, H, Katsuura-Kamano, S, Nakamoto, M, Hiyoshi, M, Takami, H, et al. Relationship of dietary factors and habits with sleep-wake regularity. Asia Pac J Clin Nutr. (2013) 22:457–65. doi: 10.6133/apjcn.2013.22.3.01
146. Zhou, J, Kim, JE, Armstrong, CLH, Chen, N, and Campbell, WW. Higher-protein diets improve indexes of sleep in energy-restricted overweight and obese adults: results from 2 randomized controlled trials. Am J Clin Nutr. (2016) 103:766–74. doi: 10.3945/ajcn.115.124669
147. Wilson, K, St-Onge, MP, and Tasali, E. Diet composition and objectively assessed sleep quality: a narrative review. J Acad Nutr Diet. (2022) 122:1182–95. doi: 10.1016/j.jand.2022.01.007
148. Hepsomali, P, and Groeger, JA. Diet, sleep, and mental health: insights from the Uk biobank study. Nutrients. (2021) 13:1–19. doi: 10.3390/nu13082573
149. Hur, S, Oh, B, Kim, H, and Kwon, O. Associations of diet quality and sleep quality with obesity. Nutrients. (2021) 13:1–9. doi: 10.3390/nu13093181
150. Godos, J, Grosso, G, Castellano, S, Galvano, F, Caraci, F, and Ferri, R. Association between diet and sleep quality: a systematic review. Sleep Med Rev. (2021) 57:1–11. doi: 10.1016/j.smrv.2021.101430
151. Howatson, G, Bell, PG, Tallent, J, Middleton, B, McHugh, MP, and Ellis, J. Effect of tart cherry juice (Prunus cerasus) on melatonin levels and enhanced sleep quality. Eur J Nutr. (2012) 51:909–16. doi: 10.1007/s00394-011-0263-7
152. Pigeon, WR, Carr, M, Gorman, C, and Perlis, ML. Effects of a tart cherry juice beverage on the sleep of older adults with insomnia: a pilot study. J Med Food. (2010) 13:579–83. doi: 10.1089/jmf.2009.0096
153. Piekarska, M, Pszczółka, M, Parol, D, Szewczyk, P, Śliż, D, and Mamcarz, A. Sleeping disorders in healthy individuals with different dietary patterns and bmi, questionnaire assessment. Int J Environ Res Public Health. (2021) 18:1–11. doi: 10.3390/ijerph182312285
154. Scoditti, E., Tumolo, M. R., and Garbarino, S., “Mediterranean diet on sleep: A health Alliance,” (2022).
155. Godos, J, Ferri, R, Caraci, F, Cosentino, FII, Castellano, S, Galvano, F, et al. Adherence to the Mediterranean diet is associated with better sleep quality in Italian adults. Nutrients. (2019) 11:1–15. doi: 10.3390/nu11050976
156. Sutanto, CN, Wang, MX, Tan, D, and Kim, JE. Association of sleep quality and macronutrient distribution: a systematic review and meta-regression. Nutrients. (2020) 12:1–21. doi: 10.3390/nu12010126
157. Mehedint, M. G., and Gulledge, A., (2014). “Nutritional impact on cognitive development,” In Reference module in biomedical sciences, Elsevier, doi: 10.1016/B978-0-12-801238-3.00237-3.
158. Ferguson, C, Aisbett, B, Lastella, M, Roberts, S, and Condo, D. Evening whey protein intake, rich in tryptophan, and sleep in elite male Australian rules football players on training and nontraining days. Int J Sport Nutr Exerc Metab. (2021) 32:82–8. doi: 10.1123/ijsnem.2021-0145
159. Falkenberg, E, Aisbett, B, Lastella, M, Roberts, S, and Condo, D. Nutrient intake, meal timing and sleep in elite male Australian football players. J Sci Med Sport. (2021) 24:7–12. doi: 10.1016/j.jsams.2020.06.011
160. Martínez-Rodríguez, A, Rubio-Arias, JÁ, Ramos-Campo, DJ, Reche-García, C, Leyva-Vela, B, and Nadal-Nicolás, Y. Psychological and sleep effects of tryptophan and magnesium-enriched Mediterranean diet in women with fibromyalgia. Int J Environ Res Public Health. (2020) 17:1–12. doi: 10.3390/ijerph17072227
161. Lindseth, G, Lindseth, P, and Thompson, M. Nutritional effects on sleep. West J Nurs Res. (2013) 35:497–513. doi: 10.1177/0193945911416379
162. Wurtman, R. J., Wurtman, J. J., Regan, M. M., Mcdermott, J. M., Tsay, R. H., and Breu, J. J., (2003). “Effects of normal meals rich in carbohydrates or proteins on plasmatryptophan and tyrosine ratios,” [Online]. Available at: https://academic.oup.com/ajcn/article/77/1/128/4689642
163. Yajima, K, et al. Effects of nutrient composition of dinner on sleep architecture and energy metabolism during sleep. J Nutr Sci Vitaminol (Tokyo). (2014) 60:114–21. doi: 10.3177/JNSV.60.114
164. Condo, D, Lastella, M, Aisbett, B, Stevens, A, and Roberts, S. Sleep duration and quality are associated with nutrient intake in elite female athletes. J Sci Med Sport. (2022) 25:345–50. doi: 10.1016/j.jsams.2021.11.045
165. Afaghi, A, O’Connor, H, and Chow, CM. Acute effects of the very low carbohydrate diet on sleep indices. Nutr Neurosci. (2008) 11:146–54. doi: 10.1179/147683008X301540
166. Castro, AI, Gomez-Arbelaez, D, Crujeiras, A, Granero, R, Aguera, Z, Jimenez-Murcia, S, et al. Effect of a very low-calorie ketogenic diet on food and alcohol cravings, physical and sexual activity, sleep disturbances, and quality of life in obese patients. Nutrients. (2018) 10:1–19. doi: 10.3390/nu10101348
167. Rontoyanni, VG, Baic, S, and Cooper, AR. Association between nocturnal sleep duration, body fatness, and dietary intake in Greek women. Nutrition. (2007) 23:773–7. doi: 10.1016/J.NUT.2007.07.005
168. Lindseth, G., and Murray, A. Dietary macronutrients and sleep. West J Nurs Res. (2016) 38. doi: 10.1177/019394591664
169. Luo, J, Ge, H, Sun, J, Hao, K, Yao, W, and Zhang, D. Associations of dietary ω-3, ω-6 fatty acids consumption with sleep disorders and sleep duration among adults. Nutrients. (2021) 13:1–12. doi: 10.3390/nu13051475
170. Dai, Y, Liu, J, Dai, Y, and Liu, J. Omega-3 long-chain polyunsaturated fatty acid and sleep: a systematic review and meta-analysis of randomized controlled trials and longitudinal studies. Nut Rev. (2021) 79:847–68. doi: 10.1093/nutrit/nuaa103
171. Binks, H, Vincent, GE, Gupta, C, Irwin, C, and Khalesi, S. Effects of diet on sleep: a narrative review. Nutrients. (2020) 12:1–18. doi: 10.3390/nu12040936
172. Chaput, JP. Sleep patterns, diet quality and energy balance. Physiol Behav. (2014) 134:86–91. doi: 10.1016/j.physbeh.2013.09.006
173. Wilkinson, MJ, Manoogian, ENC, Zadourian, A, Lo, H, Fakhouri, S, Shoghi, A, et al. Ten-hour time-restricted eating reduces weight, blood pressure, and Atherogenic lipids in patients with metabolic syndrome. Cell Metab. (2020) 31:92–104.e5. doi: 10.1016/j.cmet.2019.11.004
174. McStay, M, Gabel, K, Cienfuegos, S, Ezpeleta, M, Lin, S, and Varady, KA. Intermittent fasting and sleep: a review of human trials. Nutrients. (2021) 13:1–12. doi: 10.3390/nu13103489
175. Zhang, D, Tong, X, Arthurs, B, Guha, A, Rui, L, Kamath, A, et al. Liver clock protein BMAL1 promotes de novo lipogenesis through insulin-mTORC2-AKT signaling. J Biol Chem. (2014) 289:25925–35. doi: 10.1074/jbc.M114.567628
176. Kang, H, Park, YK, and Lee, JY. Nicotinamide riboside, an NAD+ precursor, attenuates inflammation and oxidative stress by activating sirtuin 1 in alcohol-stimulated macrophages. Lab Invest. (2021) 101:1225–37. doi: 10.1038/s41374-021-00599-1
177. Xu, J, Jackson, CW, Khoury, N, Escobar, I, and Perez-Pinzon, MA. Brain SIRT1 mediates metabolic homeostasis and neuroprotection. Front Endocrinol. (2018) 9:702. doi: 10.3389/fendo.2018.00702
178. Lee, Y, and Kim, EK. AMP-activated protein kinase as a key molecular link between metabolism and clockwork. Exp Mol Med. (2013) 45. doi: 10.1038/emm.2013.65
179. Montaigne, D, Butruille, L, and Staels, B. PPAR control of metabolism and cardiovascular functions. Nat Rev Cardiol. (2021) 18:809–23. doi: 10.1038/s41569-021-00569-6
180. Kim, JA. Cooperative instruction of signaling and metabolic pathways on the epigenetic landscape. Mol Cell. (2018) 41:264–70. doi: 10.14348/molcells.2018.0076
181. Poulsen, LLC, Siersbæk, M, and Mandrup, S. PPARs: fatty acid sensors controlling metabolism. Semin Cell Dev Biol. (2012) 23:631–39. doi: 10.1016/j.semcdb.2012.01.003
182. Anggreini, P, Kuncoro, H, Sumiwi, SA, and Levita, J. Role of the AMPK/SIRT1 pathway in non-alcoholic fatty liver disease (review). Mol Med Rep. (2023) 27. doi: 10.3892/mmr.2022.12922
183. Viollet, B, Guigas, B, Leclerc, J, Hébrard, S, Lantier, L, Mounier, R, et al. AMP-activated protein kinase in the regulation of hepatic energy metabolism: from physiology to therapeutic perspectives. Acta Physiol. (2009) 196:81–98. doi: 10.1111/j.1748-1716.2009.01970.x
184. Purushotham, A., Schug, T. T., and Li, X. SIRT1 performs a balancing act on the tight-rope toward longevity (2009).
185. Balsalobre, A, Marcacci, L, and Schibler, U. Multiple signaling pathways elicit circadian gene expression incultured Rat-1 fibroblasts. Curr Biol. (2000) 10:1291–4.
186. Hirota, T., Okano, T., Kokame, K., Shirotani-Ikejima, H., Toshiyuki, M., and Fukada, Y., “Glucose Down-regulates Per1 and Per2 mRNA levels and induces circadian gene expression in cultured Rat-1 fibroblasts* □ S,” (2002).
187. Canaple, L., et al., “Reciprocal regulation of brain and muscle Arnt-like protein 1 and peroxisome proliferator-activated receptor defines a novel positive feedback loop in the rodent liver circadian clock,” (2006).
Keywords: sleep, macronutrients, circadian process, homeostatic process, process C, process S, clock, protein
Citation: Gangitano E, Baxter M, Voronkov M, Lenzi A, Gnessi L and Ray D (2023) The interplay between macronutrients and sleep: focus on circadian and homeostatic processes. Front. Nutr. 10:1166699. doi: 10.3389/fnut.2023.1166699
Edited by:
Reza Rastmanesh, American Physical Society, United StatesReviewed by:
Matthew Thimgan, Missouri University of Science and Technology, United StatesFrédéric Gachon, The University of Queensland, Australia
Copyright © 2023 Gangitano, Baxter, Voronkov, Lenzi, Gnessi and Ray. This is an open-access article distributed under the terms of the Creative Commons Attribution License (CC BY). The use, distribution or reproduction in other forums is permitted, provided the original author(s) and the copyright owner(s) are credited and that the original publication in this journal is cited, in accordance with accepted academic practice. No use, distribution or reproduction is permitted which does not comply with these terms.
*Correspondence: Elena Gangitano, ZWxlbmEuZ2FuZ2l0YW5vQHVuaXJvbWExLml0