- 1Department of Food Science and Experimental Nutrition, School of Pharmaceutical Science, University of São Paulo, São Paulo, SP, Brazil
- 2Food Research Center (FoRC), CEPID-FAPESP (Research, Innovation and Dissemination Centers, São Paulo Research Foundation), São Paulo, SP, Brazil
- 3Food and Nutrition Research Center (NAPAN), University of São Paulo, São Paulo, SP, Brazil
In the last decades, evidence has indicated the beneficial properties of dietary polyphenols. In vitro and in vivo studies support that the regular intake of these compounds may be a strategy to reduce the risks of some chronic non-communicable diseases. Despite their beneficial properties, they are poorly bioavailable compounds. Thus, the main objective of this review is to explore how nanotechnology improves human health while reducing environmental impacts with the sustainable use of vegetable residues, from extraction to the development of functional foods and supplements. This extensive literature review discusses different studies based on the application of nanotechnology to stabilize polyphenolic compounds and maintain their physical–chemical stability. Food industries commonly generate a significant amount of solid waste. Exploring the bioactive compounds of solid waste has been considered a sustainable strategy in line with emerging global sustainability needs. Nanotechnology can be an efficient tool to overcome the challenge of molecular instability, especially using polysaccharides such as pectin as assembling material. Complex polysaccharides are biomaterials that can be extracted from citrus and apple peels (from the juice industries) and constitute promising wall material stabilizing chemically sensitive compounds. Pectin is an excellent biomaterial to form nanostructures, as it has low toxicity, is biocompatible, and is resistant to human enzymes. The potential extraction of polyphenols and polysaccharides from residues and their inclusion in food supplements may be a possible application to reduce environmental impacts and constitutes an approach for effectively including bioactive compounds in the human diet. Extracting polyphenolics from industrial waste and using nanotechnology may be feasible to add value to food by-products, reduce impacts on nature and preserve the properties of these compounds.
1. Introduction
Polyphenolic compounds in foods, supplements, and pharmaceuticals have been gaining interest due to their health benefits, reducing the risks of developing chronic non-communicable diseases (1–3). These natural compounds from the secondary metabolism of plants occur in a wide range of plant species, and regular consumption is highly encouraged, mainly due to their antioxidant properties (4–6). Extracting polyphenolic compounds from residues of food industries and by-products is a viable option to minimize environmental impacts (7, 8). In addition, including natural antioxidants in functional foods and developing dietetic supplements constitute a public health strategy (9, 10).
Despite being recognized for their functional properties, the technological incorporation of polyphenolic compounds in food or pharmaceutical formulations could be more feasible due to pronounced molecular instability (9, 11). They are sensitive compounds to environmental conditions such as temperature, changes in pH ranges, and luminosity (12, 13). Polyphenolic compounds have limited stability in human gastrointestinal ambient, such as intestinal pH, enzyme action, and intestinal microbiota, reducing the absorption of intact structures and bioavailability and significantly affecting functional activity (14, 15). Thus, the nanoencapsulation of these natural compounds can be an alternative to enable technological inclusion in different food matrices (16).
Natural polysaccharides extracted from fruit peel can be used as resistant biomaterials to form nanostructures to encapsulate chemically unstable compounds such as polyphenols (17). In recent years, complex polysaccharides such as pectins have been studied for this purpose (18, 19). They are low-toxic compounds, biodegradable, biocompatible, and widely applicable in food products. Therefore, polysaccharides extracted from food residues are composed of a sustainable source for elaborating efficient nanosystems to overcome the (20, 21) physical–chemical instability of some bioactive compounds (22, 23). The nanoencapsulation process maintains the integrated structure of stable bioactive compounds that is better biologically utilized than free compounds and enables specialized application in the food and pharmaceutical industries (24).
The scientific literature has reported that polyphenolic compounds have functionalities and bioactivities (anti-aging, anti-inflammatory, antioxidant, and antiproliferative) that effectively promote human health (9). Moreover, there is an emerging global need for sustainable actions for using residues from the food industries (25). Therefore, this review summarizes current knowledge about the properties of polyphenols from by-products and discusses the implications of nanoencapsulation with polysaccharides extracted from by-products to stabilize polyphenols. The basic principles of the full use of food residues as primary sources of phenolic compounds and the advantages of nanoencapsulation techniques applied to protect these phytochemicals, thus creating nano-fortified foods and supplements. Polysaccharides, such as pectins, are biomaterials indicated for this purpose, and together with the main techniques, they will be discussed. The explanation of aspects related to the extraction of compounds from by-products and the inclusion of food products through nanoencapsulation is essential to instigate future research with impacts on the environment and, simultaneously, stimulate the consumption of bioactive for health promotion.
2. The molecular structure and role of polyphenolic compounds for human health
Polyphenolic compounds are extensive and heterogeneous phytochemicals in plant-based foods (26, 27). They are among plant tissues’ most important secondary metabolites, such as flowers, seeds, peels, roots, and edible parts. They are widely distributed in various food sources, such as wine, tea, coffee, cereal grains, and vegetables, such as multiple fruits (28–30).
Polyphenols have a wide diversity of structures, changing their functional properties according to their molecular composition. They are constituted by aromatic rings (benzene) with attached hydroxyl groups, organic acids, sugars (mono-, di-, or oligosaccharides), and acylated sugars that are conjugated to primary phenolics structure with many hydrophilic groups (27). They are composed of two phenyl groups linked by a three-carbon bridge, with different degrees of oxidation and unsaturation of the three-carbon segment, and various sugar units associated in various positions of the polyphenol structure – or associated with organic acids or both – can be attached to the hydroxyl groups of flavonoids (31). Phenolic acids consist of a single phenyl ring, and the molecular structure of these compounds can affect their absorption and, consequently, their functional properties. Despite attributing positive effects to the metabolites, the bioavailability and activity of polyphenolic compounds are directly linked to their intact structure. Some aspects can affect molecular stability, such as the interactions with other food constituents and factors intrinsic to human digestion, such as intestinal pH and microbiota (32, 33). The functional properties (antioxidants) protect cells against oxidative damage. However, these compounds’ biological activity directly depends on the structural and glycosylation patterns (34).
Polyphenolic compounds are present in the human diet, and their regular consumption is highly recommended (34, 35). The sources of dietary polyphenols in nature are vast, and they can be found in various plant-based foods, including cereals, teas, chocolates, vegetables (such as broccoli, onions, and cabbage), and fruits (such as grapes, pears, apples, cherries, and others). In berries, the content can vary from 200–300 mg of polyphenols for each 100 g of total fresh weight (34, 36–38). Dietary polyphenols are divided into subclasses according to their chemical structures, from simple ones (single aromatic ring), such as hydroxycinnamic acids, to complex structures, such as ellagitannins (37). The main classes found in plants are flavonoids, phenolic acids, lignans, and stilbenes. Flavonoids are considered the most abundant polyphenolic compounds in food. The different structures can be subdivided into six main subclasses: flavonols, flavones, flavanones, flavanols, isoflavones, and anthocyanidins (39). The basic structure of flavonoids comprises three aromatic rings linked together and different radicals attached to the primary structure. In addition, the position and number of hydroxyl and glycosylation groups also differ in other flavonoids. Resveratrol, a subclass of stilbenes, and some phenolic acids, such as caffeic acid, chlorogenic acid, and ferulic acid, are some examples of polyphenols (40–43). The chemical structures of dietary polyphenol groups in foods are shown in Figure 1.
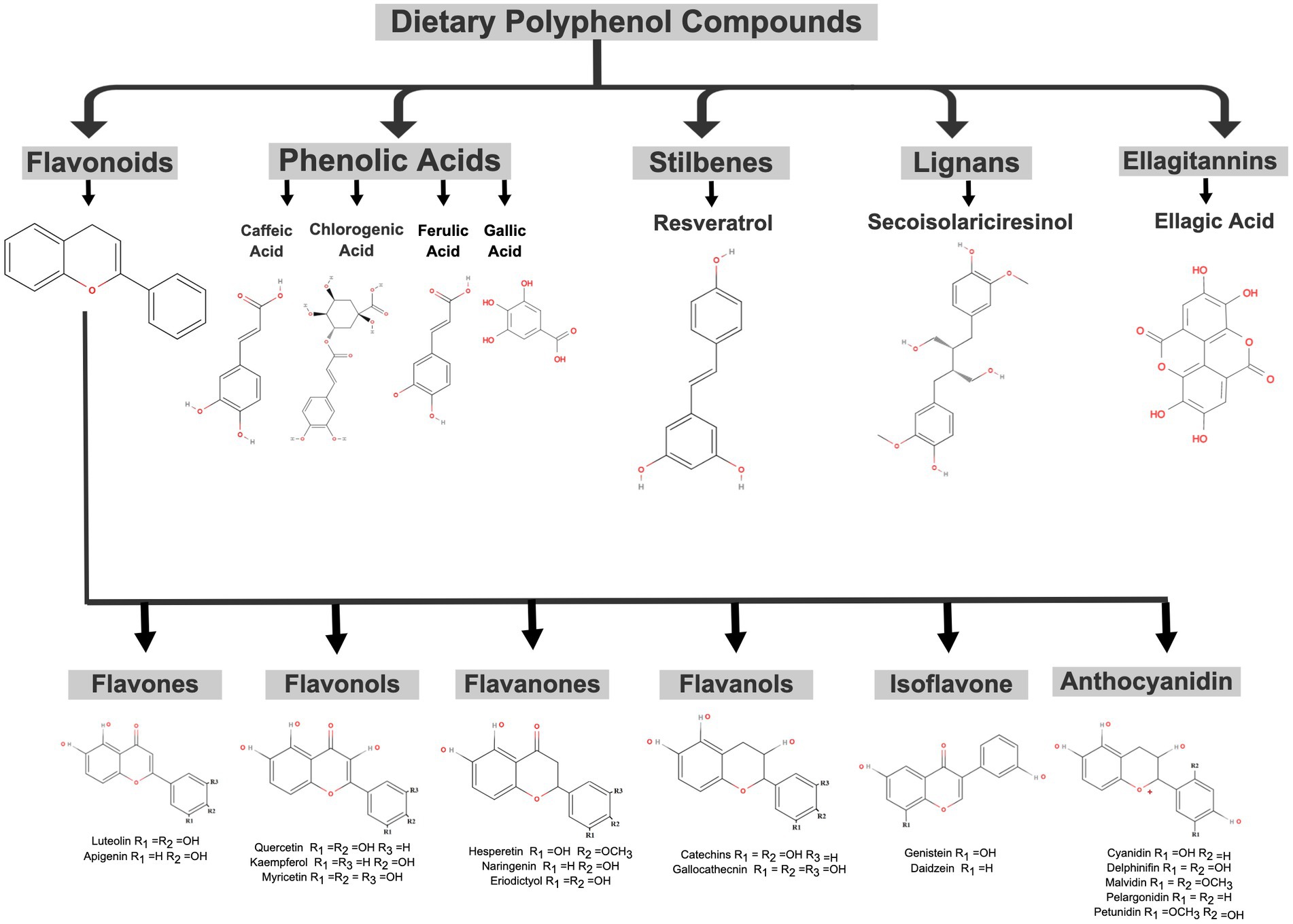
Figure 1. Chemical structures of polyphenolic compounds in foods. The basic structure forms of the main groups are depicted: flavonoids, phenolic acids, stilbenes, lignans, and ellagitannin and their subclasses. The figure was created with MolView (https://molview.org) and Mind the Graph (https://mindthegraph.com) (accessed on 10 April 2023).
Flavonoids are the predominant polyphenols in the human diet, and many foods are sources of these phytochemical classes. Anthocyanins (glycosylated anthocyanidins) are present in foods such as blackberries, strawberries, grapes, red cabbage, and blueberries. They are responsible for various plant species’ blue, purple, pink, and red colors. Quercetin is the main flavonoid in onions, tea, and apples. Citrus fruits are the primary sources of hesperidin, and soy is an essential source of genistein and daidzein. Tea, cocoa, chocolate, and beans are sources of other flavonoids. In turn, flavanones are primarily present in oranges, grapefruits, and lemon; flavonols in onion, broccoli, apple, berries, bean, and red wine and flavones in capsicum pepper (43–48). Recent studies suggest that appropriate combinations of polyphenols (quantity and proportion) can increase their bioactivity (49).
The benefits are mainly due to the antioxidant action – but not the only one – as they are considered a natural reactive oxygen species reducer and can donate hydrogen atoms and electrons (32, 50, 51). They are potent antioxidants and can prevent oxidative damage (reactions mediated by free radicals) of biomolecules such as proteins, nucleic acids, polyunsaturated lipids, and sugars (52, 53). The structure–activity relationship of polyphenolic compounds interferes with the antioxidant activities, such as the number and positions of the hydroxyl group (–OH), the presence of a double bond (C2 = C3), glycosylation and the presence of radicals attached to the primary structure (33, 54, 55). The hydroxyl groups donate hydrogens and electrons to stabilize the radicals. The ring with two hydroxyl groups (–OH) indicates a high antioxidant effect. Also, the number of hydroxyls is related to the increase in the hydrophilicity of the molecule (54). The double bond in molecular structure (C2 = C3) with a 4-carbonyl group provides planarity, electron expansion, and displacement between adjacent rings, altering the dissociation constant of the hydroxyl groups and favoring the radical stabilization, thus increasing the antioxidant activity (36, 54). The presence of glucose also affects the antioxidant activity, while the C-glucoside form demonstrated greater antioxidant capacity when compared to O-glycoside due to planarity, methylation, and electron displacement (54, 56). The mechanisms of antioxidant action can occur through two distinct pathways (57). In the first mechanism, the free radical (R) removes a hydrogen atom from the antioxidant (ArOH) (Scheme 1). The second mechanism involves donating an electron to the free radical, which becomes a cation radical (Scheme 2) (53, 57).
1. R. + ArOH → RH + ARO˙
2. R. + ArOH → RH− + ARO˙ +
Plant proteins derived from residues are considered ideal biomaterials to nanoencapsulate phenolic compounds for protection and controlled delivery (58–61). They can be used alone or combined with polysaccharides (17, 62). Phenolic compounds and proteins can interact and form complexes (through covalent and non-covalent bonds) through hydrophobic, electrostatic, van der Waals forces, and hydrogen bonds. Interaction between polyphenolic compounds and proteins can change the conformation of the protein structure, lead to folding or unfolding, forming insoluble or soluble complexes, affecting their nutritional and functional properties and their bioactivities that could generate beneficial effects on human health (63, 64). On the other hand, molecular interactions between proteins and polyphenolic compounds can favor molecular stability and modify polyphenols’ physical and chemical properties, thus improving intestinal release and absorption (65).
Polyphenolic compounds may synergistically affect cellular signaling to increase the expression of molecules, including receptor proteins, enzymes, cofactors, and regulators. Furthermore, these compounds can also regulate different signaling, such as activating convergent mitochondrial signaling pathways (66–68). Polyphenolic compounds can regulate intracellular signaling pathways, targeting intracellular enzymes, transcription factors, receptors, and other functional proteins regulation (64, 65, 69–71). Due to these mechanisms, polyphenolic compounds have anti-inflammatory action, anti-aging, antibacterial, cardio-protective, neuro-protective, and anticancer effects (5, 49, 72).
Another aspect is the ability of polyphenolic compounds positively impact human health due to systemic effects (73, 74). Polyphenols can induce microbiota homeostasis, maintaining intestinal barrier integrity (75, 76). They can modify the composition of the microbiota by increasing the ratio of beneficial bacteria to pathogenic ones and promoting immunomodulatory and prebiotic effects (77, 78). At the same time, intestinal microbiota can biotransform some of the polyphenols into more active metabolites. The literature has reported that, although not fully elucidated, changes in the microbiota composition affect the neuronal and endocrine systems. The microbiota-gut-brain is an essential neuroendocrine system that bidirectionally regulates the development of some neural disorders. Thus, the positive effect of phenolic compounds in the gut is initially due to the modulation of the local microbiota, extending to systemic impacts (79–81).
Several studies support that consuming these phytochemicals is associated with health benefits. Particularly, anthocyanin consumption has been associated with the prevention of type 2 diabetes mellitus (82), the prevention of metabolic diseases and obesity (32, 83), the cardio-protective potential, the protection of the gastrointestinal tract from high fat diet-induced alterations in redox signaling, barrier integrity and dysbiosis (84), the anticancer effects (85), and the decreased oxidative stress and inflammation (86), and many other positive effects on human health (3).
The biological properties of polyphenols indicate the numerous applications for the chemical, pharmaceutical, and food industries. Developing epidemiological strategies to enrich foods with these compounds may constitute a public health strategy to reduce the risks of developing diseases like the ones mentioned above. In addition, dietary supplements enriched with polyphenols may be an effective way to increase consumption in the population, with favorable long-term results (16, 87, 88).
3. Molecular stability of polyphenolic compounds through nanoencapsulation systems
Molecular instability limits the inclusion of polyphenols in foods and reduces the biological effects due to low absorption. Limited bioaccessibility and bioavailability are challenges to be overcome through innovative technologies (17, 89–92). The combination of polyphenolic compounds with other compounds (biomacromolecules) in nanostructures can protect them from factors intrinsic to human digestion (intestinal pH, action of digestive enzymes, and intestinal bacteria) (11, 16, 93). Thus, the wide use of polyphenols is limited due to their poor stability (maintenance of molecular integrity) under gastrointestinal factors, which significantly impact the effectiveness delivered to the target tissues to perform their biological function (92, 94).
The technological use by the food and pharmaceutical industry is impracticable since, during the processing and storage, these compounds are susceptible to several factors, limiting their application (59, 95). Environmental conditions, such as temperature, light, oxygen, pH, enzymes, and the presence of other food compounds, are considered the main factors that degrade these bioactive compounds. Processing, storage, and digestion conditions can cause chemical and structural changes and instability (96, 97). Also, the temperature in the food industry is essential to preserve microbiologically, and to improve texture and flavor characteristics, impairing the addition of these antioxidants before this step (98). Current research points to nanoencapsulation as a potentially efficient approach for protecting polyphenolic compounds from adverse environments, providing stability to maintain the properties, thus enabling technological use (99–101). In addition, scientific literature has reported a significant loss of properties and functionality of polyphenolic compounds when exposed to environmental factors, processing, and human digestion (89). The high temperature and increase in pH values in the period of storage and food processing by the industry are the main factors responsible for polyphenols degradation (59). Molecules such as anthocyanins are susceptible to the environment and easily degraded, limiting their application as a natural dye. Molecular instability impacts color, decreasing the intensity and affecting other sensory characteristics of the food (102, 103).
When ingested, polyphenolic compounds are extensively degraded and biotransformed by the action of digestive enzymes, intestinal pH, and intestinal bacteria (76). Studies have shown that only small amounts of intact molecules are available for absorption after oral ingestion. In the gastrointestinal tract, degraded polyphenols have less absorption, high excretion, decreasing antioxidant activity, and consequent lower biological activity than intact molecules (104, 105). Many polyphenols reach the colon and are biotransformed by the local microbiota, resulting in different metabolites that can be absorbed or excreted (76, 106). In the intestine, polyphenolic compounds undergo successive phases of biotransformation and degradation. In the large intestine, several reactions occur with polyphenols, such as deglycosylation, dihydroxylation, α- and β-oxidation, dehydrogenation, demethylation, decarboxylation, C-ring fission, and cleavage to lower molecular weight (36, 107). Additionally, the molecular composition, bound radicals, size, charge, hydroxylation, glycosylation, acylation and pigmentation, possible matrix effect, and presence of specific transporters are some factors that influence structural stability, antioxidant capacity and absorption of polyphenols in the human organism (108, 109).
The low bioavailability of these compounds results in decreased bioefficacy and decreased health effects. It hinders technological application, which limits all the health benefits attributed to in vitro and in vivo studies and restricts specialized applications (108, 110). Bioavailability can vary between different polyphenol compounds, but bioavailability is generally low for most of these polyphenols due to molecular instability being the determinant factor. The biotransformation in the gastrointestinal tract is basically due to two main factors. The first is specific to the molecular structure of the phenolic compounds because, according to the form (scaffold), it can facilitate metabolization by the human intestinal enzymes and the intestinal microbiota. The second factor responsible for the low biological use concerns the diversity of the colonization of the intestinal microbiota. Some structural alterations of polyphenols (such as deglycosylation) occur by more generic groups of bacteria, while others arise by specific bacterial genera (111–113).
There are several technologies of nanoencapsulation to maintain molecular stability, reduce the degradation of polyphenols, and develop an efficient delivery system (100, 114, 115). Nano-functional plant-based foods have aroused growing interest from researchers to investigate the mechanisms for metabolic diseases, such as prevention and adjuvant treatment, including the disorders mentioned in item 2 (62, 116). Nanotechnology is considered a new frontier for improving food quality in the food sector, resulting in increased nutritional value and food safety. For the health area, nanoscience corresponds to the targeted delivery of nutrients and bioactive compounds in the body, thus possibly impacting disease decrease (117). In addition to the search for applied technologies to maintain molecular integrity/stability, there has been a need to identify alternative sources for extracting polyphenolic compounds. In recent years, sustainable sources have been explored in line with current demands for environmental preservation and the development of nano-functional foods (118, 119).
4. The use of waste from food industries: possible benefits for human health, and prospecting the reduction of impacts on the environment
Recent research indicates that food systems significantly affect climate change (120). It is estimated that in the subsequent years, the impact on the environment caused by the inefficient use of natural resources, concurrent with the exponential growth of the world population, will result in profound social consequences (118, 121). The production of residues from the food industry significantly impacts the environment, constituting approximately one-tenth of food systems emissions (122, 123). This complex panorama indicates a current urgency for actions aimed at the sustainable use of natural sources, such as reducing waste and optimizing the use of by-products from the food industry.
Food losses and waste are part of the production chain, starting at the harvest, passing through the industrial processing stage, and throughout distribution. According to data from the FAO (United States Food and Agriculture Organization) around the world, approximately 14% of the world’s food – about US$ 680 billion in developed countries and US$ 310 billion in developing countries – is lost annually (124, 125). The agro-food system supply chain produces significant by-products (118). The considerable amount of food discards and biomass generates an accumulation in nature, increasing environmental and economic impacts (120).
Regardless of the considerable progress in industrial residue management in the world in the last decades, the remaining natural by-products still need to be used to their maximum potential, indicating a critical and current problem (25). The need for public policies to reduce food losses in the production chain has recently been discussed, as well as the impact on the sustainable use of environmental resources for the economy of countries and on social issues related to food and nutrition security (124, 126). The United Nations (UN) has been encouraging actions to achieve sustainable development goals, which include specific proposals such as “Zero Hunger,” “Good Health and Well-Being,” “Responsible Production and Consumption,” and “Climate Action.” Innovative strategies that culminate in these objectives must be instigated, mainly those that seek the development of concrete actions for the full use of shared natural resources and direct continuous efforts to reduce food waste while promoting the health of populations (120, 123, 127, 128).
Although several technological approaches aim to reduce the environmental impacts of food industries, some challenges remain open (129, 130). Globally, millions of tons of food waste from vegetables are generated annually, mainly from processing fruits and grains (131). Food sub-products constitute a huge extractive source of nutrients such as vitamins, minerals, and macromolecules (proteins and polysaccharides) (121, 132). In addition, vegetable residues, mainly from fruit peel, can extract non-nutrients, such as bioactive phytochemicals. The wide diversity of these compounds includes several groups, comprehending carotenoids, prebiotics and dietary fibers, pigments, and phenolic compounds – most of them with remarkable beneficial bioactivity (26, 133). Sustainability actions are being planned, such as the rational use of raw food materials and the correct disposal of by-products (121). In this sense, the technological use of food waste can be an alternative to reduce environmental impacts with positive economic consequences (127). In addition, the extraction methods must also be carefully selected, with environmentally friendly techniques, and exclude potentially toxic compounds (119, 134, 135).
Pereira et al. (121) indicated that adding value to the wasted parts of food can be a strategy to mitigate food shortages in the future. The use of peels and seeds, in addition to being nutritionally adequate, can have positive environmental impacts in the long term. The use of by-products from the food industry as alternative sources for the extraction of polyphenolic compounds and the development of supplements can be an action to provide functional products to the population while attributing value to the waste (131). The use of vegetable peels for the characterization and extraction of polyphenols and the destination for the enrichment of products can be a promising option for this purpose (119, 124).
Some of the extracted phenolic compounds (e.g., anthocyanins) can be used as pigments and natural dyes, replacing synthetic ones with vast industrial applications and commercial interest (103). Due to the beneficial health properties already discussed, they can be used as an additive to nano-foods due to their properties, and for the development of new functional products/ingredients and supplements for use as adjuvant treatments in different physiological processes (116, 136, 137), upcycling by-products into biofunctional components (26, 119).
Suleria et al. (26) indicated that fruit peels have diverse phytochemicals, including phenolic compounds. The levels of the bioactive compounds were identified through a comprehensive screening and characterization/quantification by HPLC and LC–MS/MS in different fruit peels. The antioxidant content was attested and the potential use for extracting phenolic compounds in the peel of twenty other fresh and ripe fruits was proposed, such as apple, apricot, avocado, banana, custard apple, dragon fruit, grapefruit, kiwifruit, lime, mango, melon, nectarine, orange, papaya, passionfruit, peach, pear, pineapple, plum, and pomegranate.
The sub-product sources for polyphenolic compounds are varied and widely available and indicate a potential unexplored source for extraction. Table 1 shows some of the residual sources rich in phenolic compounds, and different studies (in vitro and in vivo) are described to indicate the biological effects.
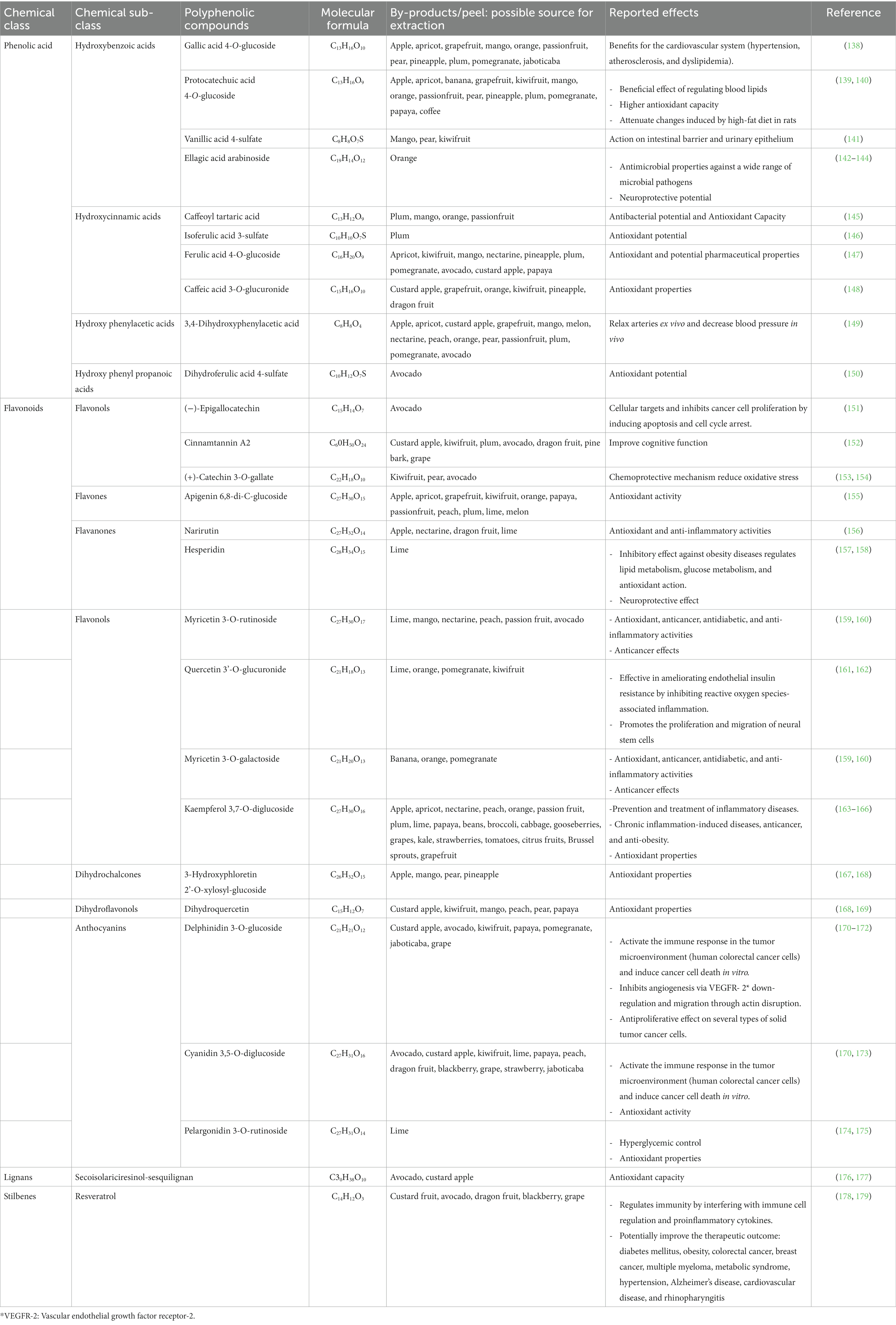
Table 1. According to the group and sub-group, polyphenolic compounds in by-products of the food industry, and the main effects are reported in studies in vitro e in vivo.
Most of the macromolecules extracted in abundance from by-products can be considered sustainable, with high quantities for extraction (118, 131). Non-starch polysaccharides, such as pectins, chitosan, and cellulose, are abundant in industrial food waste and with well-established extraction and isolation methods (134, 180) (Figure 2). These biomaterials can be used to form nanostructures for encapsulating compounds highly sensitive to environmental and biological factors (181).
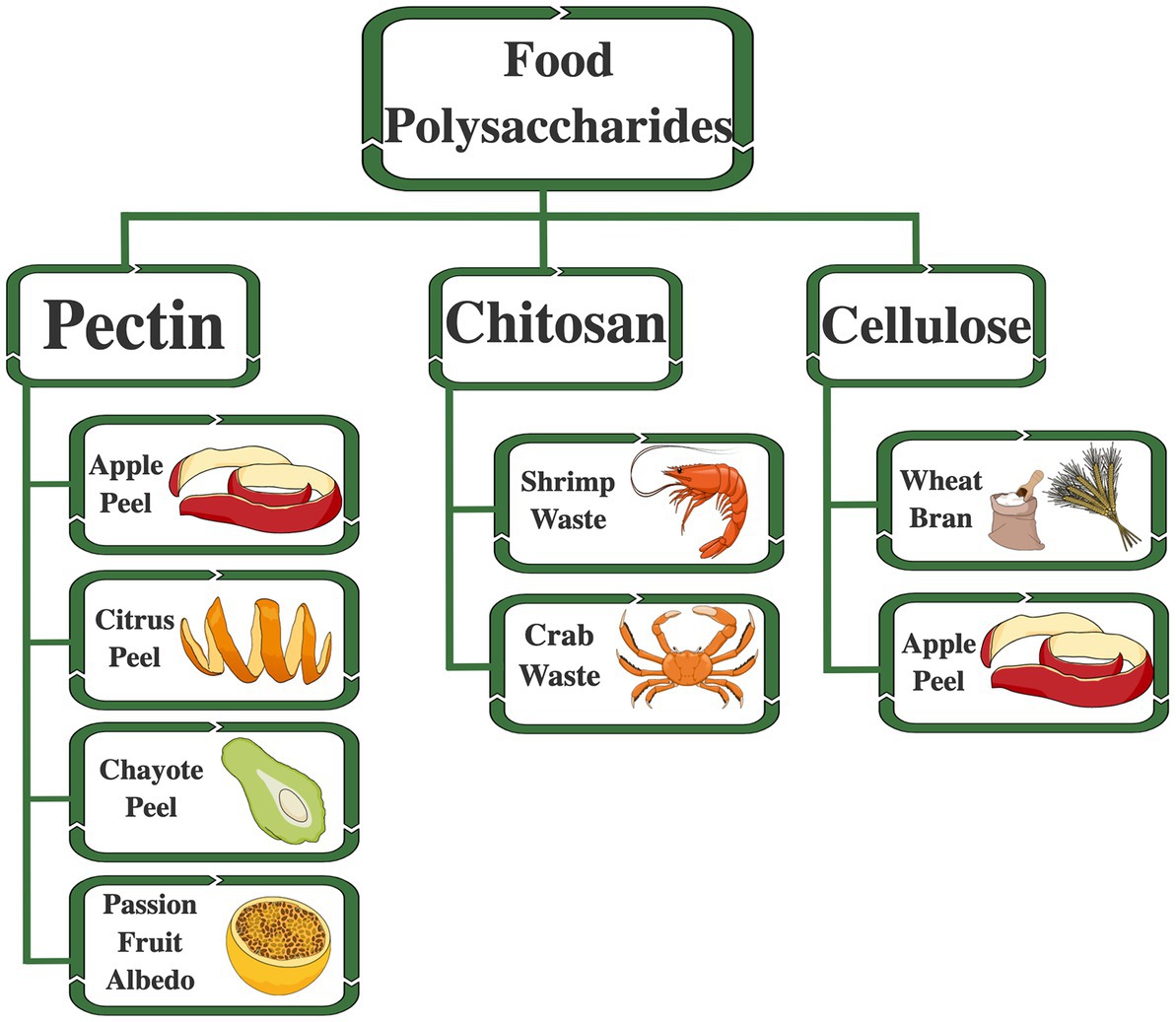
Figure 2. Polysaccharides are found in by-products of the food industry, the primary sources for extraction available. Polysaccharides are considered potential biomaterials to form nanocapsules for the entrapment of chemically unstable compounds. The figure was created by Mind the Graph (https://mindthegraph.com) (accessed on 10 April 2023).
Food polysaccharides are used in food technology applications as physical modifiers and gelling agents for foods and drug formulations. Especially the non-starch polysaccharides are gastro-resistant and are used to compose drug delivery systems (33, 182). The various new functionalities and biological effects are being extensively explored (183), and nanotechnology is a new possibility for applying these biomaterials, such as pectins (62). Pectins are complex polysaccharides of plant origin and are widely distributed in nature, with excellent application in nanotechnology for food and pharmaceutical industries (184).
Pectins are abundant in wasted food because they are constituents of the plant cell wall and form a primary layer that surrounds and supports plant cells. They are in various vegetables, mainly citrus peel and albedo (orange, lemon, lime, and pomelo), apple peel, chayote peel, and passion fruit albedo. All these by-products from fruits are considered adequate sources for the extraction of pectin (Figure 2) (185–187). The industrial processing of citrus fruits to produce juices is one of the primary sources for obtaining pectin, and several agro-industrial residues are being investigated as potential extractive sources. The peel and albedo of remaining fruits are ~50% of the total fruit, with 25 to 30% (dry weight of citrus peel) composed of pectin (134, 188–190). Other alternatives sources can be used to obtain pectins, such as sugar beet and sunflower seed head (191), tomato waste (192), cocoa husks (193), grapefruit peel, pomegranate peel, passion fruit peel, mango peel, banana peel, and kiwi fruit pomace (194–199).
The extraction, purification, and fractionation of pectins from alternative sources can be considered sustainable. The physical, chemical, rheological, and functional properties of pectin indicate the potential for technological application in several segments since pectin is stable in the digestive tract, with excellent mechanical resistance, biodegradability, and biocompatibility (188). Therefore, pectin is a promising biomaterial to compose nanostructure systems to protect chemically unstable compounds, such as phenolic compounds (8, 23, 200). Other characteristics and applicability of nanostructured pectins will be discussed later in this article.
5. Biomaterial for elaborating nanostructure systems for encapsulating polyphenolic compounds from food residues
To increase the economic value of the compounds extracted from losses/residues and by-products of the food industry, phenolic compounds and pectins can be applied in nanotechnology (16). Using vegetable peels to extract these two natural compounds represents the valorization of the agro-food losses and waste from the food industry while constituting a strategy to produce new products with added economic and nutritional value (118). Using nanoencapsulated polyphenols as a natural and potent source of antioxidants in the various segments of the food and pharmaceutical industries is considered an innovative approach (59).
Over the last few decades, various technologies were developed to encapsulate and consequently protect polyphenols, such as microencapsulation (201, 202). Although effective, technology at nanometric scales (sizes <1,000 nm), such as nanoemulsion, nanoliposomes, nanoparticles, and nanogel, is indicated to increase stability, bioaccessibility, controlled delivery, and bioavailability of phenolic compounds with numerous application possibilities (7, 114, 203, 204). Notably, there is a significant difference between the bioavailability of free and nanoencapsulated polyphenols (100, 116).
The nanoencapsulation techniques physically and chemically stabilize the compounds, promoting better absorption of intact forms, thus improving the bioavailability. Nanoencapsulation can also increase storage stability, enabling its use as a food ingredient (16, 100, 115, 205). The nanotechnology process can also enhance some sensorial characteristics, such as the taste and astringency of some polyphenols (16, 115). Moreover, different nanoencapsulation systems can be used to optimize the controlled delivery of polyphenolic compounds (93, 114). Two distinct approaches are reported for the formation of nanostructures: (I) top-down and (II) bottom-up. The top-down approach aims to reduce the size of structured material to a nanometer scale through external disruptive mechanical forces using precise tools/equipment. On the other hand, the bottom-up approach involves using molecules by self-organization/self-assembly (62, 200). Factors such as proportion and concentration between compounds (encapsulated and encapsulating), temperature, pH, agitation, and ionic strength must be carefully controlled to form nanocapsules (206, 207).
Nanoencapsulation means a scientific and technological effort that can reduce food waste by upcycling by-products to generate value-added products, highly specialized and intended for broad application in the food industry (food, health, pharmaceutical, and cosmetic areas). Nanotechnology is an alternative to value this industrial waste and can contribute to global sustainability actions (16, 119). Different materials such as fruit peels as sources of polysaccharides to form nanostructures – for improving the stability of labile compounds – indicates an innovative possibility for introducing new resources, such as expanded technological use of phytochemicals and their controlled release in the specific tissues/organs. The impacts on the use of by-products of the food industry through the application of nanotechnology polysaccharide-based constitute opportunities and innovations (11, 16, 17, 116, 131).
Several scientific investigations are directed toward identifying methods to retain and improve the polyphenolic content and delivery to the human body. The antioxidant activity of polyphenolic compounds extracted from coffee grounds was preserved through encapsulation based on polysaccharides (maltodextrin, gum Arabic, 1:1 ratio) and with techniques such as lyophilization and spray-drying (30). The study done by Kasote et al. (208) reviewed several studies that used different methodologies for stabilizing polyphenolic compounds and their inclusion in cereals. Nanoencapsulation was cited as a promising technology and as one of the options for developing functional foods with bioavailability improvement (16, 59, 116).
Polysaccharides, such as pectin, are important biomolecules to be used as biomaterials for sectors of the food and pharmaceutical industries. These natural polysaccharides’ properties, bioactivities, and versatility can be applied in nanotechnology (209). Furthermore, pectins are found in many renewable sources and food by-products due to their wide distribution in nature (180). Nanoencapsulation based on pectin has attracted attention due to favorable properties, such as large specific surface area, excellent stability, good biocompatibility, improved permeability and retention time of the encapsulated compound, uncomplicated design and preparation, structural flexibility and preferred characteristics of controlled release in the intestine, and delivery to specific physiological sites (210–212). In addition, the nanoencapsulation process contributes to improved antioxidant properties and prebiotic effects (213).
Food polysaccharides can undergo reversible self-assembly and respond to stimuli such as ionotropic gelation. Besides, they interact with other molecules through self-assembly, generating structures on a nanometric scale (214, 215). The process can be performed without adding surfactants, emulsifiers, or other potentially unsafe products for human ingestion and the environment (62, 215). Scientific literature has reported that different approaches and varied techniques are used to prepare nanostructured polysaccharides, proteins, and lipids. They can be used alone or with other food biomacromolecules, forming nanocomplexes to encapsulate chemically unstable compounds (16, 216). For example, some coating materials are indicated for anthocyanin’s protection (24). Natural polysaccharides are indicated due to their high nutritional value and potential functional health properties. In this sense, some studies demonstrated the pectin-based nanoencapsulation of anthocyanins resulting in physical–chemical protection, enabling more excellent stability and color maintenance and aiming at an increase in intestinal absorption (18, 184, 217). Polyphenolic compounds were encapsulated in pectin-based nanoparticles (isolated or combined with proteins) with excellent results and repeatability (205, 218).
As depicted above, food biopolymers (polysaccharides and proteins) are suitable for wall material encapsulation (219). Several studies successfully used pectin to encapsulate phenolic compounds, isolated or combined with other polysaccharides (chitosan and cellulose) and proteins (lysozyme and whey protein) (18, 205, 217, 218, 220, 221). Table 2 shows the most recent studies that used different biomaterials to nanoencapsulation polyphenolic compounds (extracted from other sources), indicating the versatility of combinations between biomacromolecules and different purposes and applications. The various research highlights the main objectives for nanoencapsulation of phenolic compounds, which are maintaining the stability, the color, and the application in food matrices for resistance during the processing. In addition to industrial applicability, increased bioaccessibility, antioxidant capacity, and bioavailability are identified as the biological factors that motivate the development of nanocapsules.
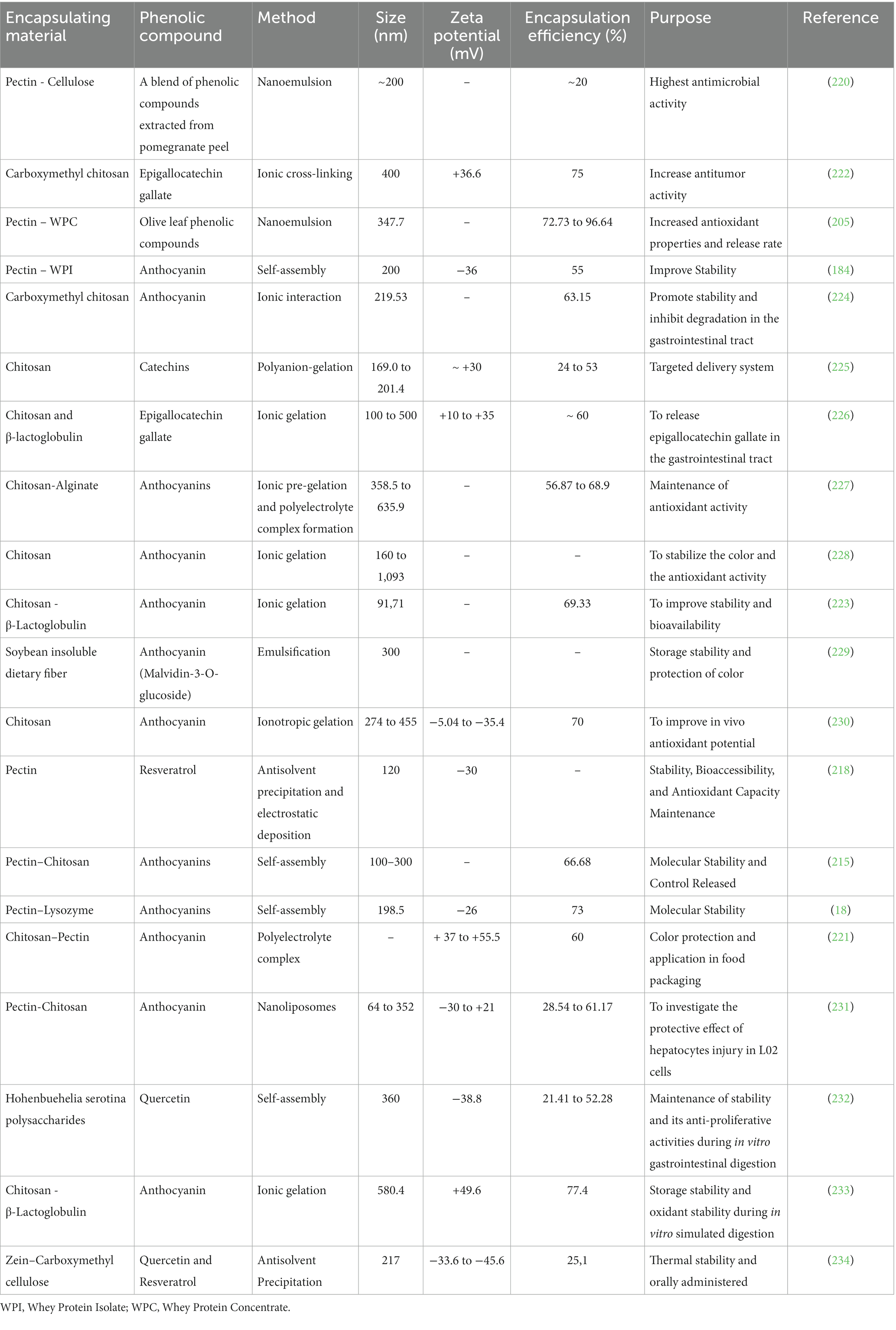
Table 2. Nanoencapsulation of polyphenolic compounds polysaccharide and protein-based nanostructures.
As shown in Table 2, polysaccharides – isolated or bound to proteins – are predominantly used for the nanoencapsulation of anthocyanins, epigallocatechin gallate, catechins, and resveratrol. The nanoencapsulation of these polyphenolic compounds was developed to maintain physicochemical stability and properties such as antimicrobial, antitumor, antioxidant, and antiproliferative activity. Also, the nanoencapsulation process can promote a better intestinal release rate, improve bioaccessibility and bioavailability, inhibit degradation in the gastrointestinal tract, ensure storage stability and color protection, apply in food packaging, promote thermal stability, and enable the oral administration of these compounds.
Some methods are indicated as viable for elaborating polysaccharide-based nanostructures to encapsulate polyphenols. The definitions, advantages, and applicability of the main techniques used to encapsulate polyphenols will be discussed below. (1) Emulsification/Nanoemulsion: this nanoencapsulation process involves mixing two immiscible liquids using an interface agent, such as a surfactant. Among the main advantages is the promotion of adequate solubility and kinetic stability, providing a sufficient matrix for targeted delivery, protection, and stability of polyphenolic compounds, which tend to be spherical droplets. However, using surfactants and co-surfactant can be potentially toxic, which may be one of the disadvantages of this technique (235–237). (2) Ionic gelation: biopolymers with electric charges can interact by different forces when homogenized in an aqueous solution forming nanostructures with different characteristics of isolated initial compounds. The polysaccharide can be dissolved in aqueous acid and added to a polycationic solution. The ionic-gelling polysaccharides can be precipitated to form spherical nanoparticles. The methodology can form stable nanostructures, and the low cost and non-addition of potentially toxic products are advantages of this technique. On the other hand, the disadvantages are the non-uniformity of size, and the release of the encapsulated compound may be limited (200, 238, 239). (3) Ionic cross-linking: formed by complexes of polyelectrolytes and complex coacervates. Nanoparticles are formed through the binding of divalent cations, such as the addition of calcium. Ionic cross-linking is based on the interaction between tripolyphosphate anions and protonated amine groups of polymers. The interaction occurs between two oppositely charged molecules or polyelectrolytes. Polysaccharide, mainly composed of guluronic acid and the mannuronic acid units (alginates), forms ionic complexes with divalent cations like Mg2+, Ca2+, and Ba2+. This technique forms stable molecules without adding toxic products and with reduced diameter sizes (200, 240, 241). (4) Coacervation: This process involves an interaction between two oppositely charged biopolymers forming a complex to protect a bioactive compound. This technique’s use of biodegradable compounds, adequate stability, and high encapsulation efficiency is advantageous. However, the complexity of elaboration and control of all the factors involved limits its application (238, 242, 243). (5) Self-assembly: different biopolymers are homogenized in an aqueous solution interacting by different electrostatic forces. It is low-cost and free of potentially toxic products. Despite this, encapsulation efficiency can be limited (62, 215, 238, 244). (6) Nanoprecipitation: the biopolymer nanoprecipitation process occurs by adding a non-solvent to a polymeric solution in supersaturation, nucleation, growth by condensation, and coagulation. It forms nanoparticles or polymeric aggregates that can adequately encapsulate polyphenolics. Despite having a limited controlled release, this method has low cost and broad applicability (245, 246).
Current challenges, such as increased chronic disease incidence in the population and diverse environmental impacts, instigate the research community for emerging intervention possibilities. There is considerable food waste from industries considered a potent source of nutritional compounds. In addition to the environmental impact of solid accumulation, there are significant economic losses. In this sense, nanotechnology can be an advantageous alternative. The development of functional nano foods could increase the consumption of bioactive compounds – extracted from food waste – generating a protective effect on public health. The use of food waste directs it to a noble destination and generates economic value (247, 248).
6. Nanocarriers based on natural polysaccharides: the promising interaction between pectin and phenolic compounds
Polyphenolic compounds encapsulated into pectin nanocomplexes for physical–chemical stabilization are a breakthrough in allowing several technological applications aiming at human health benefits (249). The functional properties (mainly antioxidant activity), bioaccessibility, and bioavailability of polyphenolic compounds are known to be influenced by compounds linked to their structure (250). Nanoencapsulation is not different, and physical–chemical studies should be done to depict how phenolic compounds are disposed inside the nanostructures to indicate determined applications since polyphenol-carbohydrate interactions can preserve chemical properties and stabilize the molecular structure (251). Moreover, pectin-polyphenolic interactions are influenced by these two compounds’ high structural variability and complexity (252, 253). Considerable scientific evidence indicates that polyphenol-carbohydrate interaction, especially with pectin, can preserve the properties of the polyphenols maintaining their chemical structure, inhibiting the degradation, and preserving the antioxidant action (101). Polyphenolic compounds and complex polysaccharides have a natural affinity and can bind via non-covalent and covalent interactions (107). Due to its characteristics, pectin has been used as a carrier to protect and deliver unstable bioactive compounds (209, 219).
Pectins are a class of polysaccharides composed of long chains of galacturonic acids widely distributed in nature (187, 254). Pectin is formed by heterogeneous non-starch polysaccharide complexes, originating from the structure of the cell walls of plant tissues and being considered an essential dietary fiber in the human diet (255). Pectins are formed by homogalacturonans (HG), type I (RG-I), and type II (RG-II) rhamnogalacturonans, in addition to xylogalacturonans and apiogalacturonans. Homogalacturonans are formed by linear structures of [→ α-1,4-D-galacturonic acid →], with variation in the degree of acetyl- and methyl esterification and may contain xylose (xylogalacturonan) (207, 256). Due to the possibility of ionization of galacturonic acids, pectins have good solubility in water. When galacturonic acid residues are esterified by methyl or acetyl groups, the solubility characteristics are modified according to the degree of methylation (high degree of methoxylation >50%, low degree <50%) (257). These compounds can produce highly viscous gels (depending on the degree of methoxylation and ligands), so they are used as an emulsifier and/or thickener (258). At neutral pH, the carboxylic acid (pKa pH ~3.6) has a net negative charge interacting with cationic molecules (proteins and polysaccharides) to form nanocomplexes (8). Pectins have the property of interacting with certain types of proteins at variable pH, stabilizing the nanostructures formed in acid or neutral dispersions (8, 213).
Due to their resistance to stomachal pH and human enzymes, pectins can protect and control the release of bioactive compounds, as they can reach (in their intact form) distal portions of the intestine, maintaining the functionality of different bioactive compounds nanoencapsulated in their structure (58, 136). In the intestine, pectins are fermented by microorganisms from intestinal microbiota. This fermentation process produces short-chain fatty acids with beneficial systemic effects and provides better absorption of nutrients and bioactive compounds (259). Due to its characteristics, pectin is a biomaterial suitable for forming nanocapsules for physical–chemical protection, increased absorption, and bioavailability of different active compounds. Using polyphenolic compounds as nanocarriers is an innovative alternative for designing new smart foods (23, 219).
Although the mechanism is not fully understood, it is known that polysaccharides, especially dietary fibers, can transport phenolic compounds in the gastrointestinal tract and protect them from the intestinal microbiota. The presence of these compounds in the intestinal lumen causes changes in intestinal bacteria composition through pectin fermentation (108, 260, 261). A recent study by Zhang et al. (261) aimed to evaluate the release and activity of polyphenols bound to soluble dietary fiber (wheat bran) in a simulated in vitro digestion and colonic fermentation system. The authors concluded that there was an influence on the bioaccessibility of fiber-bound polyphenols after colonic fermentation. There was a stimulation of the growth of beneficial bacteria after fermentation, indicating the potential prebiotic effect of the system.
The natural affinity of anthocyanins and polysaccharides was confirmed in a study that analyzed the tendency to bind during the processing of blueberry pomace with different dietary fibers, including pectin. A strong trend for anthocyanin-polysaccharide binding was observed (262, 263). The interaction occurs through electrostatic interaction between hydroxyl groups (OH+) and the carboxylic acids (COOH−) of the polysaccharides, including pectins (264). The different binding between anthocyanin and pectin was investigated in a study by Fernandes et al. (252). The interaction between cyanidin-3-O-glycoside and four citrus pectic fractions was explored through analyzes such as isothermal titration calorimetry, nuclear magnetic resonance, and UV–Visible spectrophotometry. The results indicated that different binding affinities could be correlated with changes in coloration, with the degree of pectin esterification being the primary determinant for complex formation.
Pectin-based nanostructures can be formed through different methods, such as spray drying, emulsion, hydrogel formation, liposomes, and nanocomplexes through electrostatic complexation and molecular self-organization. Pectin at neutral/basic pH predominates negative electrical charge and may interact with other positively charged macromolecules forming stable nanostructures (265, 266). Currently, pectin combined with other compounds (proteins, lipids, and polysaccharides) is considered a highly effective wall material for phenolic compounds (205). Stabilized polyphenolic compounds maintain color, functional properties, and stability in the gastrointestinal system improving their bioavailability (267, 268). Pectin is also considered a nanocarrier of polyphenolic compounds for the controlled release in distal parts of the colon, protecting from microbial processing from intestinal microbiota and promoting the absorption of intact molecules (200, 269).
7. Conclusion and future perspectives
Nanotechnology is a viable option to enhance the use of bioactive molecules from commonly wasted sources, producing a new product with high added value and broad applicability. Polyphenolic compounds benefit human health and protect against chronic non-communicable diseases, such as cardiovascular diseases, obesity, diabetes, and cancers. Using sustainable sources to extract phytochemicals is an effective strategy to reduce the accumulation of waste from the food industries on the environment. Fruit residues are sources of phenolic compounds and pectin. Pectin is an excellent encapsulating biomaterial for phenolic compounds aiming for innovative applications. Pectin-based nanostructures can protect compounds from molecular degradation and enable the development of nano-engineered foods for different purposes and applications. Applied nanotechnology adds economic value to these functional ingredients and reduces the impacts caused by the food industries.
As discussed in the manuscript, it is essential to understand that despite being potentially applicable to nanotechnology in the valorization of industrial waste – positively affecting the circular economy and as a sustainability strategy – studies are strongly supported and necessary to attest to in vivo safety for human consumption. Another relevant factor is the ingested doses. Most of the results were performed in the laboratory using in vitro and in vivo models. Nanocapsules with phenolic compounds may enhance their bioavailability, so they should be carefully evaluated in toxicity studies (in vitro and in vivo) and long-term ingestion. Also, another fundamental point that must be considered is the optimization for production on an industrial scale; from the extraction process to the elaboration of nanostructures, these parameters still need to be explored. This review provided new perspectives for possible research directions in nanoscience and minimizing impacts on nature, as well as supporting information for using different fruit peels rich in polyphenolic compounds to be extracted with potential benefits for human health. Many efforts have been made to mitigate food waste and protect the environment. However, innovative ideas with practical strategies for fully utilizing industrial by-products are urgently needed. Using nanoencapsulation of polyphenolic compounds extracted from by-products characterizes the circularity in food systems, promoting new functionality for products with no commercial value while encouraging the increased consumption of bioactive compounds. Dietary supplements and foods enriched with nanoencapsulated bioactive compounds are considered promising to reduce the risk of developing various diseases. The use of polyphenolic compounds within nanocapsules represents new perspectives on current study gaps and future directions in this field, providing enriched and highly specialized foods for optimal target intestinal release of bioactive compounds. The full use of by-products is achieved through technological incentive policies that support the use of unused parts of plants to be recovered and destined for the full use of their nutritional, techno-functional, and health-enhancing properties, resulting in economic, environmental, and public health benefits. The impact on the valuation of losses/waste and by-products of the food industry through the application of nanotechnology represents opportunities, trends, and innovations (Figure 3).
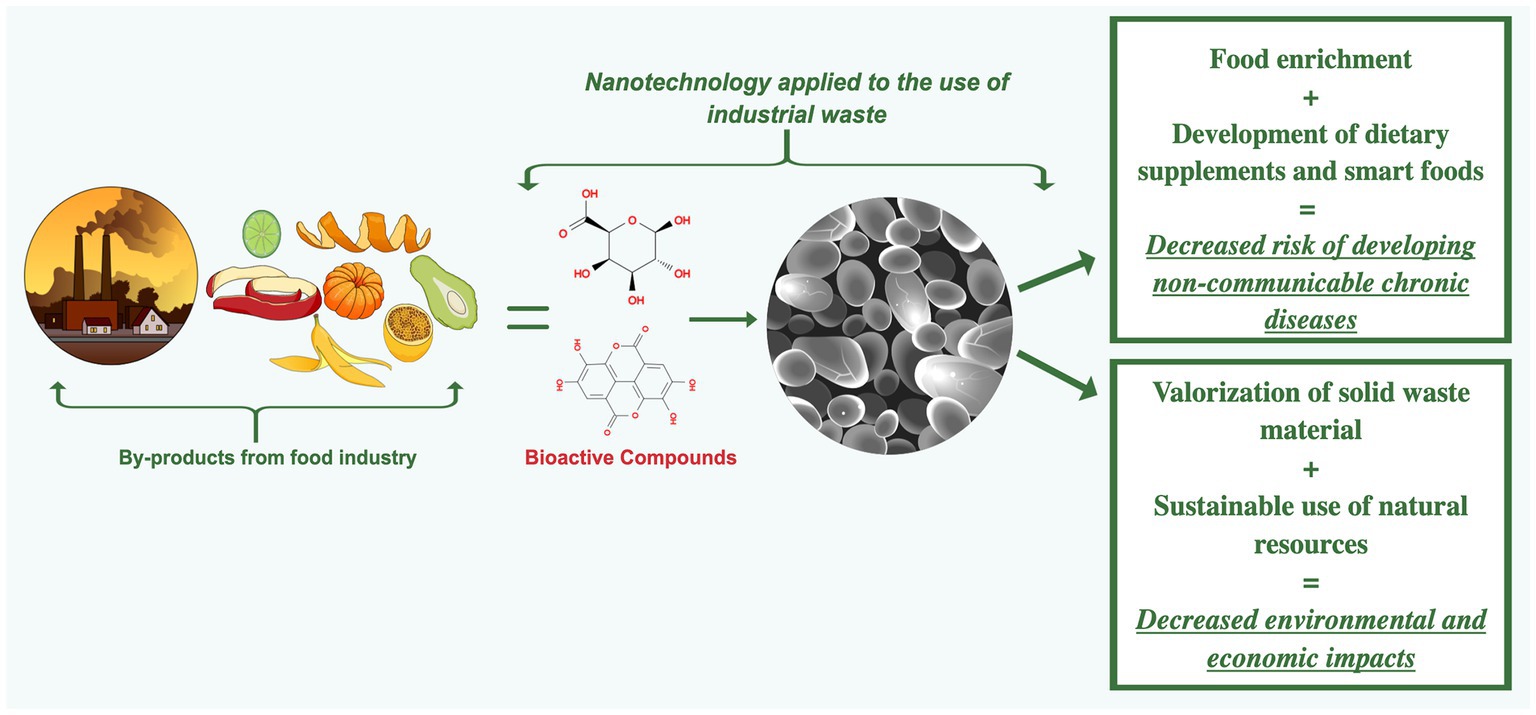
Figure 3. The by-products of the food industry can be used to extract bioactive compounds to be nanoencapsulated with polysaccharides also extracted from by-products. Food enrichment and new dietary supplements can be done to decrease the development of non-communicable chronic diseases, thus increasing the value of waste material and reducing environmental and economic impacts. The figure was created by Mind the Graph (https://mindthegraph.com) (accessed on 10 April 2023).
Author contributions
TR: conceptualization, data curation, and writing – original draft and review and editing. JF: conceptualization, supervision, writing – review and editing, and grant acquisition. All authors have read and agreed to the published version of the manuscript.
Funding
The authors acknowledge The National Council for Scientific and Technological Development (CNPq) for JF productivity scholarship (CNPq Proc. #307842/2022-3). The study was financially supported by grants #2013/07914-8 and #2019/11816-8 from the São Paulo Research Foundation (FAPESP).
Conflict of interest
The authors declare that the research was conducted in the absence of any commercial or financial relationships that could be construed as a potential conflict of interest.
Publisher’s note
All claims expressed in this article are solely those of the authors and do not necessarily represent those of their affiliated organizations, or those of the publisher, the editors and the reviewers. Any product that may be evaluated in this article, or claim that may be made by its manufacturer, is not guaranteed or endorsed by the publisher.
References
1. Polia, F, Pastor-Belda, M, Martínez-Blázquez, A, Horcajada, MN, Tomás-Barberán, FA, and García-Villalba, R. Technological and biotechnological processes to enhance the bioavailability of dietary (poly)phenols in humans. J Agric Food Chem. (2022) 70:2092–107. doi: 10.1021/acs.jafc.1c07198
2. Salazar-Orbea, GL, García-Villalba, R, Bernal, MJ, Hernández, A, Tomás-Barberán, FA, and Sánchez-Siles, LM. Stability of phenolic compounds in apple and strawberry: effect of different processing techniques in industrial set up. Food Chem. (2023) 401:134099. doi: 10.1016/j.foodchem.2022.134099
3. Zhou, L, Xie, M, Yang, F, and Liu, J. Antioxidant activity of high purity blueberry anthocyanins and the effects on human intestinal microbiota. LWT. (2020) 117:108621. doi: 10.1016/j.lwt.2019.108621
4. Khan, N, and Mukhtar, H. Tea polyphenols in promotion of human health. Nutrients. (2019) 11, 1–39. doi: 10.3390/nu11010039
5. Yahfoufi, N, Alsadi, N, Jambi, M, and Matar, C. The immunomodulatory and anti-inflammatory role of polyphenols. Nutrients. (2018) 10:1–23. doi: 10.3390/nu10111618
6. Yan, Z, Zhong, Y, Duan, Y, Chen, Q, and Li, F. Antioxidant mechanism of tea polyphenols and its impact on health benefits. Anim Nutr. (2020) 6:115–23. doi: 10.1016/j.aninu.2020.01.001
7. Hosseini, SF, Ramezanzade, L, and McClements, DJ. Recent advances in nanoencapsulation of hydrophobic marine bioactives: bioavailability, safety, and sensory attributes of nano-fortified functional foods. Trends Food Sci Technol. (2021) 109:322–39. doi: 10.1016/j.tifs.2021.01.045
8. Noreen, A, Nazli, ZIH, Akram, J, Rasul, I, Mansha, A, Yaqoob, N, et al. Pectins functionalized biomaterials; a new viable approach for biomedical applications: a review. Int J Biol Macromol. (2017) 101:254–72. doi: 10.1016/j.ijbiomac.2017.03.029
9. Cory, H, Passarelli, S, Szeto, J, Tamez, M, and Mattei, J. The role of polyphenols in human health and food systems: a mini-review. Front Nutr. (2018) 5:87. doi: 10.3389/fnut.2018.00087
10. Chatterjee, NS, Raghavankutty, M, and Anandan, R. Dietary supplementation of encapsulated anthocyanin loaded-chitosan nanoparticles attenuates hyperlipidemic aberrations in male wistar rats. Carbohydr Polym Technol Appl. (2021) 2:100051. doi: 10.1016/j.carpta.2021.100051
11. Gaber Ahmed, GH, Fernández-González, A, and Díaz García, ME. Nano-encapsulation of grape and apple pomace phenolic extract in chitosan and soy protein via nanoemulsification. Food Hydrocoll. (2020) 108:105806. doi: 10.1016/j.foodhyd.2020.105806
12. Voss, DM, Miyagusuku-Cruzado, G, and Giusti, MM. Thermal stability comparison between 10-catechyl-pyranoanthocyanins and anthocyanins derived from pelargonidin, cyanidin, and malvidin. Food Chem. (2022):134305. doi: 10.1016/j.foodchem.2022.134305
13. Azad, MOK, Adnan, M, Kang, WS, Lim, JD, and Lim, YS. A technical strategy to prolong anthocyanins thermal stability in formulated purple potato (Solanum tuberosum L. cv bora valley) processed by hot-melt extrusion. Int J Food Sci Technol. (2021) 57:6925–34. doi: 10.1111/ijfs.15485
14. Jokioja, J, Yang, B, and Linderborg, KM. Acylated anthocyanins: a review on their bioavailability and effects on postprandial carbohydrate metabolism and inflammation. Compr Rev Food Sci Food Saf. (2021) 20:5570–615. doi: 10.1111/1541-4337.12836
15. Jiao, X, Li, B, Zhang, Q, Gao, N, Zhang, X, and Meng, X. Effect of in vitro-simulated gastrointestinal digestion on the stability and antioxidant activity of blueberry polyphenols and their cellular antioxidant activity towards HepG2 cells. Int J Food Sci Technol. (2018) 53:61–71. doi: 10.1111/ijfs.13516
16. Tahir, A, Shabir Ahmad, R, Imran, M, Ahmad, MH, Kamran Khan, M, Muhammad, N, et al. Recent approaches for utilization of food components as nano-encapsulation: a review. Int J Food Prop. (2021) 24:1074–96. doi: 10.1080/10942912.2021.1953067
17. Lu, X, Chen, J, Guo, Z, Zheng, Y, Rea, MC, Su, H, et al. Using polysaccharides for the enhancement of functionality of foods: a review. Trends Food Sci Technol. (2019) 86:311–27. doi: 10.1016/j.tifs.2019.02.024
18. Osvaldt Rosales, TK, Pessoa da Silva, M, Lourenço, FR, Aymoto Hassimotto, NM, and Fabi, JP. Nanoencapsulation of anthocyanins from blackberry (Rubus spp.) through pectin and lysozyme self-assembling. Food Hydrocoll. (2021) 114:106563. doi: 10.1016/j.foodhyd.2020.106563
19. Silva, MP, Rosales, TKO, De, PLF, and Fabi, JP. Formulation of a new proof-of-concept pectin/lysozyme nanocomplex as potential β- lactose delivery matrix: structure and thermal stability analyses. Food Hydrocoll. (2022) 134:108011. doi: 10.1016/j.foodhyd.2022.108011
20. Song, J, Yu, Y, Chen, M, Ren, Z, Chen, L, Fu, C, et al. Advancement of protein- and polysaccharide-based biopolymers for anthocyanin encapsulation. Front Nutr. (2022) 9:1–11. doi: 10.3389/fnut.2022.938829
21. Esmaili, SK, Ghanbarzadeh, B, Ayaseh, A, Pezeshki, A, and Hosseini, M. Design, fabrication and characterization of pectin-coated gelatin nanoparticles as potential nano-carrier system. J Food Biochem. (2019) 43:1–12. doi: 10.1111/jfbc.12729
22. Shishir, MRI, Karim, N, Gowd, V, Xie, J, Zheng, X, and Chen, W. Pectin-chitosan conjugated nanoliposome as a promising delivery system for neohesperidin: characterization, release behavior, cellular uptake, and antioxidant property. Food Hydrocoll. (2019) 95:432–44. doi: 10.1016/j.foodhyd.2019.04.059
23. Rosales, TKO, and Fabi, JP. Polysaccharides as natural nanoencapsulants for controlled. Smart Nanomater. Bioencapsulat. (2023):23–38. doi: 10.1016/B978-0-323-91229-7.00002-7
24. Rosales, TKO, and Fabi, JP. Nanoencapsulated anthocyanin as a functional ingredient: technological application and future perspectives. Colloids Surf B Biointerfaces. (2022) 218:112707. doi: 10.1016/j.colsurfb.2022.112707
25. Mellinas, C, Ramos, M, and Jim, A. Recent trends in the use of pectin from agro-waste residues as a natural-based biopolymer for food packaging applications. Materials. (2020) 13:673. doi: 10.3390/ma13030673
26. Suleria, HAR, Barrow, CJ, and Dunshea, FR. Screening and characterization of phenolic compounds and their antioxidant capacity in different fruit peels. Foods. (2020) 9:1206. doi: 10.3390/foods9091206
27. Hanhineva, K, Törrönen, R, Bondia-Pons, I, Pekkinen, J, Kolehmainen, M, Mykkänen, H, et al. Impact of dietary polyphenols on carbohydrate metabolism. Int J Mol Sci. (2010) 11:1365–402. doi: 10.3390/ijms11041365
28. Fraga, CG, Croft, KD, Kennedy, DO, and Tomás-Barberán, FA. The effects of polyphenols and other bioactives on human health. Food Funct. (2019) 10:514–28. doi: 10.1039/C8FO01997E
29. Beckman, CH. Phenolic-storing cells: keys to programmed cell death and periderm formation in wilt disease resistance and in general defence responses in plants? Physiol Mol Plant Pathol. (2000) 57:101–10. doi: 10.1006/pmpp.2000.0287
30. Ballesteros, LF, Ramirez, MJ, Orrego, CE, Teixeira, JA, and Mussatto, SI. Encapsulation of antioxidant phenolic compounds extracted from spent coffee grounds by freeze-drying and spray-drying using different coating materials. Food Chem. (2017) 237:623–31. doi: 10.1016/j.foodchem.2017.05.142
31. Jakobek, L. Interactions of polyphenols with carbohydrates, lipids and proteins. Food Chem. (2015) 175:556–67. doi: 10.1016/j.foodchem.2014.12.013
32. Kardum, N, and Glibetic, M. Polyphenols and their interactions with other dietary compounds: implications for human health In:. Advances in food and nutrition research, vol. 84. 1st ed. Toldrá, F. Amsterdam: Elsevier Inc. (2018). 103–44.
33. Dobson, CC, Mottawea, W, Rodrigue, A, Buzati Pereira, BL, Hammami, R, Power, KA, et al. Impact of molecular interactions with phenolic compounds on food polysaccharides functionality In:. Advances in food and nutrition research, vol. 90. 1st ed. Toldrá, F. Amsterdam: Elsevier Inc. (2019). 135–81.
34. Zhang, H, and Tsao, R. Dietary polyphenols, oxidative stress and antioxidant and anti-inflammatory effects. Curr Opin Food Sci. (2016) 8:33–42. doi: 10.1016/j.cofs.2016.02.002
35. Abbaszadeh, H, Keikhaei, B, and Mottaghi, S. A review of molecular mechanisms involved in anticancer and antiangiogenic effects of natural polyphenolic compounds. Phyther Res. (2019) 33:2002–14. doi: 10.1002/ptr.6403
36. Cosme, P, Rodríguez, AB, and Espino, JGM. Plant Phenolics: bioavailability as a key determinant of their potential health-promoting applications. Antioxidants. (2020) 9:1263. doi: 10.3390/antiox9121263
37. Ovaskainen, ML, Törrönen, R, Koponen, JM, Sinkko, H, Hellström, J, Reinivuo, H, et al. Dietary intake and major food sources of polyphenols in Finnish adults. J Nutr. (2008) 138:562–6. doi: 10.1093/jn/138.3.562
38. Amos, R, Atmodjo, M, Huang, C, Gao, Z, Venkat, A, Taujale, R, et al. Polymerization of the backbone of the pectic polysaccharide rhamnogalacturonan I. Nat Plants. (2022) 8:1289–303. doi: 10.1038/s41477-022-01270-3
39. El Gharras, H. Polyphenols: food sources, properties and applications – a review. Int J Food Sci Technol. (2009) 44:2512–8. doi: 10.1111/j.1365-2621.2009.02077.x
40. Panche, AN, Diwan, AD, and Chandra, SR. Flavonoids: an overview. J Nutr Sci. (2016) 5:5. doi: 10.1017/jns.2016.41
41. Pereira, DM, Valentão, P, Pereira, JA, and Andrade, PB. Phenolics: from chemistry to biology. Molecules. (2009) 14:2202–11. doi: 10.3390/molecules14062202
42. Mukherjee, PK. Phyto-pharmaceuticals, nutraceuticals and their evaluation. Qual Control Eval Herb Drugs. (2019):707–22. doi: 10.1016/B978-0-12-813374-3.00020-X
43. Kim, YA, Keogh, JB, and Clifton, PM. Polyphenols and glycémie control. Nutrients. (2016) 8:1. doi: 10.3390/nu8010017
44. Manach, C, Scalbert, A, Morand, C, Rémésy, C, and Jiménez, L. Polyphenols: food sources and bioavailability. Am J Clin Nutr. (2004) 79:727–47. doi: 10.1093/ajcn/79.5.727
45. Joseph, SV, Edirisinghe, I, and Burton-freeman, BM. Berries: anti-inflammatory effects in humans. J Agric Food Chem. (2014) 62:3886–903. doi: 10.1021/jf4044056
46. Zamora-ros, R, Biessy, C, Rothwell, JA, Monge, A, Lajous, M, Scalbert, A, et al. Dietary polyphenol intake and their major food sources in the Mexican Teachers’ Cohort. Br J Nutr. (2018) 120:353–60. doi: 10.1017/S0007114518001381
47. Chaves, JO, de Souza, MC, da Silva, LC, Lachos-Perez, D, Torres-Mayanga, PC, Machado, APF d, et al. Extraction of flavonoids from natural sources using modern techniques. Front Chem. (2020) 8:507887. doi: 10.3389/fchem.2020.507887
48. Paik, SS, Jeong, E, Jung, SW, Ha, TJ, Kang, S, Sim, S, et al. Anthocyanins from the seed coat of black soybean reduce retinal degeneration induced by N-methyl-N-nitrosourea. Exp Eye Res. (2012) 97:55–62. doi: 10.1016/j.exer.2012.02.010
49. Mitra, S, Tareq, AM, Das, R, Bin, ET, Nainu, F, Chakraborty, AJ, et al. Polyphenols: a first evidence in the synergism and bioactivities polyphenols. Food Rev. Int. (2022) 1:1–23. doi: 10.1080/87559129.2022.2026376
50. Mao, H, Wen, Y, Yu, Y, Li, H, Wang, J, and Sun, B. Materials today bio ignored role of polyphenol in boosting reactive oxygen species generation for polyphenol / chemodynamic combination therapy. Mater Today Bio. (2022) 16:100436. doi: 10.1016/j.mtbio.2022.100436
51. Pandey, KB, and Rizvi, SI. Plant polyphenols as dietary antioxidants in human. Oxidative Med Cell Longev. (2009) 2:270–8. doi: 10.4161/oxim.2.5.9498
52. Heleno, SA, Martins, A, João, M, Queiroz, RP, and Ferreira, ICFR. Bioactivity of phenolic acids: metabolites versus parent compounds: a review. Food Chem. (2015) 173:501–13. doi: 10.1016/j.foodchem.2014.10.057
53. Vuolo, MM, Lima, VS, Roberto, M, and Junior, M. Phenolic compounds: structure, classification, and antioxidant power. (2019) 33–50. doi: 10.1016/b978-0-12-814774-0.00002-5
54. Wang, T, Li, Q, and Bi, K. Bioactive flavonoids in medicinal plants: structure, activity and biological fate. Asian J Pharm Sci (2018) 13:12–23. doi: 10.1016/j.ajps.2017.08.004
55. Bendary, E, Francis, RR, Ali, HMG, Sarwat, MI, and El, HS. Antioxidant and structure – activity relationships (SARs) of some phenolic and anilines compounds. Ann Agric Sci. (2013) 58:173–81. doi: 10.1016/j.aoas.2013.07.002
56. Heim, KE, Tagliaferro, AR, and Bobilya, DJ. Flavonoid antioxidants: chemistry, metabolism and structure-activity relationships. J Nutr Biochem. (2002) 13:572–84. doi: 10.1016/s0955-2863(02)00208-5
57. Valko, M, Leibfritz, D, Moncol, J, Cronin, MTD, Mazur, M, and Telser, J. Free radicals and antioxidants in normal physiological functions and human disease. Int J Biochem Cell Biol. (2007) 39:44–84. doi: 10.1016/j.biocel.2006.07.001
58. Bealer, EJ, Onissema-karimu, S, Rivera-galletti, A, Francis, M, Wilkowski, J, and Hu, X. Protein – polysaccharide composite materials: fabrication and applications. Polymers. (2020) 12:464. doi: 10.3390/polym12020464
59. Cao, H, Saroglu, O, Karadag, A, Diaconeasa, Z, Zoccatelli, G, Conte-Junior, CA, et al. Available technologies on improving the stability of polyphenols in food processing. Food Front. (2021) 2:109–39. doi: 10.1002/fft2.65
60. Zimet, P, and Livney, YD. Beta-lactoglobulin and its nanocomplexes with pectin as vehicles for ω-3 polyunsaturated fatty acids. Food Hydrocoll. (2009) 23:1120–6. doi: 10.1016/j.foodhyd.2008.10.008
61. Ju, M, Zhu, G, Huang, G, Shen, X, Zhang, Y, Jiang, L, et al. A novel Pickering emulsion produced using soy protein-anthocyanin complex nanoparticles. Food Hydrocoll. (2020) 99:105329. doi: 10.1016/j.foodhyd.2019.105329
62. Zhang, Q, Zhou, Y, Yue, W, Qin, W, Dong, H, and Vasanthan, T. Nanostructures of protein-polysaccharide complexes or conjugates for encapsulation of bioactive compounds. Trends Food Sci Technol. (2021) 109:169–96. doi: 10.1016/j.tifs.2021.01.026
63. Shahidi, F, and Dissanayaka, CS. Phenolic – protein interactions: insight from in - silico analyses – a review. Food Prod Process Nutr. (2023) 5, 1–21. doi: 10.1186/s43014-022-00121-0
64. Jiang, L, Liu, Y, Li, L, Qi, B, Ju, M, and Xu, Y. Covalent conjugates of anthocyanins to soy protein: Unravelling their structure features and in vitro gastrointestinal digestion fate. Food Res Int. (2019) 120:603–9. doi: 10.1016/j.foodres.2018.11.011
65. Guan, H, Zhang, W, Sun-waterhouse, D, Jiang, Y, Li, F, Geoffrey, I, et al. Phenolic-protein interactions in foods and post ingestion: switches empowering health outcomes. Trends Food Sci. Technol. (2021) 118:71–86. doi: 10.1016/j.tifs.2021.08.033
66. Iskender, H, Dokumacioglu, E, Mazlum, T, and Ince, I. The effect of hesperidin and quercetin on oxidative stress, NF- k B and SIRT1 levels in a STZ-induced experimental diabetes model. Biomed Pharmacother. (2017) 90:500–8. doi: 10.1016/j.biopha.2017.03.102
67. Nichols, M, Zhang, J, Polster, BM, Elustondo, PA, Thirumaran, A, Pavlov, EV, et al. Synergistic neuroprotection by epicatechin and quercetin: activation of convergent mitochondrial signaling pathways. Neuroscience. (2015) 308:75–94. doi: 10.1016/j.neuroscience.2015.09.012
68. Li, Y, He, D, Li, B, Lund, MN, Xing, Y, Wang, Y, et al. Engineering polyphenols with biological functions via polyphenol-protein interactions as additives for functional foods. Trends Food Sci Technol. (2021) 110:470–82. doi: 10.1016/j.tifs.2021.02.009
69. Xiong, R, Wang, X, Wu, J, Tang, Y, Qiu, W, Shen, X, et al. Polyphenols isolated from lychee seed inhibit Alzheimer’s disease-associated Tau through improving insulin resistance via the IRS-1/PI3K/Akt/GSK-3β pathway. J Ethnopharmacol. (2020) 251:112548. doi: 10.1016/j.jep.2020.112548
70. Liu, G, Shi, A, Wang, N, Li, M, He, X, Yin, C, et al. Redox Biology Polyphenolic proanthocyanidin-B2 suppresses proliferation of liver cancer cells and hepatocellular carcinogenesis through directly binding and inhibiting AKT activity. Redox Biol. (2020) 37:101701. doi: 10.1016/j.redox.2020.101701
71. Jia, L, Huang, S, Yin, X, Zan, Y, Guo, Y, and Han, L. Quercetin suppresses the mobility of breast cancer by suppressing glycolysis through Akt-mTOR pathway mediated autophagy induction (2018) 208:123–30. doi: 10.1016/j.lfs.2018.07.027
72. Zhang, Y, Wu, S, Qin, Y, Liu, J, Liu, J, and Wang, Q. Interaction of phenolic acids and their derivatives with human serum albumin: structure – a ffi nity relationships and e ff ects on antioxidant activity (2018) July 2017:1072–80. doi: 10.1016/j.foodchem.2017.07.100
73. Ray, SK, and Mukherjee, S. Evolving interplay between dietary polyphenols and gut microbiota—an emerging importance in healthcare. Front Nutr. (2021) 8:634944. doi: 10.3389/fnut.2021.634944
74. Cardona, F, Andrés-lacueva, C, Tulipani, S, Tinahones, FJ, and Queipo-ortuño, MI. Benefits of polyphenols on gut microbiota and implications in human health. J Nutr Biochem. (2013) 24:1415–22. doi: 10.1016/j.jnutbio.2013.05.001
75. Hidalgo, M, Oruna-Concha, MJ, Kolida, S, Walton, GE, Kallithraka, S, Spencer, JPE, et al. Metabolism of anthocyanins by human gut microflora and their influence on gut bacterial growth. J Agric Food Chem. (2012) 60:3882–90. doi: 10.1021/jf3002153
76. Chen, Y, Li, Q, Zhao, T, Zhang, Z, Mao, G, Feng, W, et al. Biotransformation and metabolism of three mulberry anthocyanin monomers by rat gut microflora. Food Chem. (2017) 237:887–94. doi: 10.1016/j.foodchem.2017.06.054
77. Bi, J, Sepodes, B, and Fernandes, PCB. Polyphenols in health and disease: gut microbiota, bioaccessibility, and bioavailability. Compounds, 3 40–72. doi: 10.3390/compounds3010005
78. Rodríguez-Daza, MC, Daoust, L, Boutkrabt, L, Pilon, G, Varin, T, Dudonné, S, et al. Wild blueberry proanthocyanidins shape distinct gut microbiota profile and influence glucose homeostasis and intestinal phenotypes in high-fat high-sucrose fed mice. Sci Rep. (2020) 10:1–16. doi: 10.1038/s41598-020-58863-1
79. Berding, K, Vlckova, K, Marx, W, Schellekens, H, Stanton, C, Clarke, G, et al. Diet and the microbiota – gut – brain axis: sowing the seeds of good mental health. Adv Nutr. (2021) 12:1239–85. doi: 10.1093/advances/nmaa181
80. Martin, CR, Osadchiy, V, Kalani, A, and Mayer, EA. The brain-gut-microbiome axis. Cell Mol Gastroenterol Hepatol. (2018) 6:133–48. doi: 10.1016/j.jcmgh.2018.04.003
81. Sterling, KG, Dodd, GK, Alhamdi, S, Asimenios, PG, Dagda, RK, De, MKL, et al. Mucosal immunity and the gut-microbiota-brain-axis in neuroimmune disease. Int J Mol Sci. (2022) 23:13328. doi: 10.3390/ijms232113328
82. Damián-medina, K, Milenkovic, D, Salinas-moreno, Y, Corral-jara, KF, Figueroa-yáñez, L, Marino-marmolejo, E, et al. Anthocyanin-rich extract from black beans exerts anti-diabetic effects in rats through a multi-genomic mode of action in adipose tissue. Front. Nutr. (2022) 9:1019259. doi: 10.3389/fnut.2022.1019259
83. Nistor, M, Pop, R, Daescu, A, Pintea, A, and Socaciu, C. Anthocyanins as key phytochemicals acting for the prevention of metabolic diseases: an overview. Molecules. (2022) 27:4254. doi: 10.3390/molecules27134254
84. Cremonini, E, Daveri, E, Mastaloudis, A, Adamo, AM, Mills, D, Kalanetra, K, et al. Anthocyanins protect the gastrointestinal tract from high fat diet-induced alterations in redox signaling, barrier integrity and dysbiosis. Redox Biol. (2019) 26:101269. doi: 10.1016/j.redox.2019.101269
85. Mok, JW, Chang, DJ, and Joo, CK. Antiapoptotic effects of anthocyanin from the seed coat of black soybean against oxidative damage of human lens epithelial cell induced by H2O2. Curr Eye Res. (2014) 39:1090–8. doi: 10.3109/02713683.2014.903497
86. Speer, H, D’Cunha, NM, Alexopoulos, NI, McKune, AJ, and Naumovski, N. Anthocyanins and human health—a focus on oxidative stress, inflammation and disease. Antioxidants. (2020) 9. doi: 10.3390/antiox9050366
87. Jafari, SM, and McClements, DJ. Nanotechnology approaches for increasing nutrient bioavailability In:. Advances in food and nutrition research, vol. 81. 1st ed. ed. Toldrá, F. Amsterdam: Elsevier Inc. (2017). 1–30.
88. Carnauba, RA, Sarti, FM, Hassimotto, NMA, and Lajolo, FM. Assessment of dietary intake of bioactive food compounds according to income level in the Brazilian population. Br J Nutr. (2021) 127:1232–9. doi: 10.1017/S0007114521001987
89. Victoria-Campos, CI, De, O-PJ, Rocha-Guzmán, NE, Gallegos-Infante, JA, Failla, ML, Pérez-Martínez, JD, et al. Gastrointestinal metabolism and bioaccessibility of selected anthocyanins isolated from commonly consumed fruits. Food Chem. (2022) 383:132451. doi: 10.1016/j.foodchem.2022.132451
90. Tian, L, Tan, Y, Chen, G, Wang, G, Sun, J, Ou, S, et al. Metabolism of anthocyanins and consequent effects on the gut microbiota. Crit Rev Food Sci Nutr. (2019) 59:982–91. doi: 10.1080/10408398.2018.1533517
91. Salarbashi, D, Bazeli, J, and Rad, EF. An update on the new achievements in the nanocapsulation of anthocyanins. Nanomedicine J. (2020) 7:87–97. doi: 10.22038/NMJ.2020.07.001
92. Grgić, J, Šelo, G, Planinić, M, Tišma, M, and Bucić-Kojić, A. Role of the encapsulation in bioavailability of phenolic compounds. Antioxidants. (2020) 9:1–36. doi: 10.3390/antiox9100923
93. Rosales, O, Mariko, N, Hassimotto, A, and Lajolo, FM. Nanotechnology as a tool to mitigate the effects of intestinal microbiota on metabolization of anthocyanins. Antioxidants. (2022) 11:506. doi: 10.3390/antiox11030506
94. Garavand, F, Jalai-jivan, M, Assadpour, E, and Mahdi, S. Encapsulation of phenolic compounds within nano/microemulsion systems: a review. Food Chem. (2021) 364:130376. doi: 10.1016/j.foodchem.2021.130376
95. Klimczak, I, Małecka, M, Szlachta, M, and Gliszczyńska-Świgło, A. Effect of storage on the content of polyphenols, vitamin C and the antioxidant activity of orange juices. J Food Compos Anal. (2007) 20:313–22. doi: 10.1016/j.jfca.2006.02.012
96. Hurtado, NH, Morales, AL, González-Miret, ML, Escudero-Gilete, ML, and Heredia, FJ. Colour, pH stability and antioxidant activity of anthocyanin rutinosides isolated from tamarillo fruit (Solanum betaceum Cav.). Food Chem. (2009) 117:88–93. doi: 10.1016/j.foodchem.2009.03.081
97. Suzery, M, Nudin, B, Nurwahyu Bima, D, and Cahyono, B. Effects of temperature and heating time on degradation and antioxidant activity of anthocyanin from Roselle petals (Hibiscus sabdariffa L.). Int J Sci Technol Manag. (2020) 1:288–38. doi: 10.46729/ijstm.v1i4.78
98. Zhao, C, Liu, Y, Lai, S, Cao, H, Guan, Y, San Cheang, W, et al. Effects of domestic cooking process on the chemical and biological properties of dietary phytochemicals. Trends Food Sci Technol. (2019) 85:55–66. doi: 10.1016/j.tifs.2019.01.004
99. Rashwan, AK, Younes, H, Karim, N, Taha, E, Mozafari, MR, Tawfeuk, HZ, et al. Health properties of bioactive food compounds-loaded micro and nano-encapsulation systems: a review. SVU Int J Agric Sci. (2022) 4:178–203. doi: 10.21608/SVUIJAS.2022.134530.12081
100. Akbari-Alavijeh, S, Shaddel, R, and Jafari, SM. Encapsulation of food bioactives and nutraceuticals by various chitosan-based nanocarriers. Food Hydrocoll. (2020) 105:105774. doi: 10.1016/j.foodhyd.2020.105774
101. Jiao, A, Jin, Z, and Qiu, C. Polyphenols as plant-based Nutraceuticals: health effects, encapsulation, Nano-Delivery, and application. Foods. (2022) 11:2189. doi: 10.3390/foods11152189
102. Braga, ARC, Murador, DC, de Souza Mesquita, LM, and de Rosso, VV. Bioavailability of anthocyanins: gaps in knowledge, challenges and future research. J Food Compos Anal. (2018) 68:31–40. doi: 10.1016/j.jfca.2017.07.031
103. Mattioli, R, Francioso, A, Mosca, L, and Silva, P. Anthocyanins: a comprehensive review of their chemical properties and health effects on cardiovascular and neurodegenerative diseases. Molecules. (2020) 25:3809. doi: 10.3390/molecules25173809
104. Karaś, M, Jakubczyk, A, Szymanowska, U, Złotek, U, and Zielińska, E. Digestion and bioavailability of bioactive phytochemicals. Int J Food Sci Technol. (2017) 52:291–305. doi: 10.1111/ijfs.13323
105. Toydemir, G, Boyacioglu, D, Capanoglu, E, Van Der Meer, IM, Tomassen, MMM, Hall, RD, et al. Investigating the transport dynamics of anthocyanins from unprocessed fruit and processed fruit juice from sour cherry (Prunus cerasus L.) across intestinal epithelial cells. J Agric Food Chem. (2013) 61:11434–41. doi: 10.1021/jf4032519
106. Catalkaya, G, Venema, K, Lucini, L, Rocchetti, G, Delmas, D, Daglia, M, et al. Interaction of dietary polyphenols and gut microbiota: microbial metabolism of polyphenols, influence on the gut microbiota, and implications on host health. Food Front. (2020) 1:109–33. doi: 10.1002/fft2.25
107. Rocchetti, G, Barba, FJ, Gregorio, RP, Lorenzo, JM, Oliveira, PG, Prieto, MA, et al. Functional implications of bound phenolic compounds and phenolics – food interaction: a review. Compr Rev Food Sci Food Saf. (2022) 21:811–42. doi: 10.1111/1541-4337.12921
108. Kamiloglu, S, Tomas, M, Ozdal, T, and Capanoglu, E. Effect of food matrix on the content and bioavailability of flavonoids. Trends Food Sci Technol. (2021) 117:15–33. doi: 10.1016/j.tifs.2020.10.030
109. Ozdal, T, Sela, DA, Xiao, J, Boyacioglu, D, Chen, F, and Capanoglu, E. The reciprocal interactions between polyphenols and gut microbiota and effects on bioaccessibility. Nutrients. (2016) 8:1–36. doi: 10.3390/nu8020078
110. Enaru, B, Drețcanu, G, Pop, TD, Stǎnilǎ, A, and Diaconeasa, Z. Anthocyanins: factors affecting their stability and degradation. Antioxidants. (2021) 10:1967. doi: 10.3390/antiox10121967
111. Ashley, D, Marasini, D, Brownmiller, C, Lee, JA, Carbonero, F, and Lee, S. Impact of grain sorghum polyphenols on microbiota of normal weight and overweight/obese subjects during in vitro fecal fermentation. Nutrients. (2019) 11:217. doi: 10.3390/nu11020217
112. Singh, AK, Cabral, C, Kumar, R, Ganguly, R, and Pandey, AK. Beneficial effects of dietary polyphenols on gut microbiota and strategies to improve delivery efficiency. Nutrients. 2019, 11:2216. doi: 10.3390/nu11092216
113. Murota, K, Nakamura, Y, and Uehara, M. Flavonoid metabolism: the interaction of metabolites and gut microbiota. Biosci Biotechnol Biochem. (2018) 82:1–11. doi: 10.1080/09168451.2018.1444467
114. Bao, C, Jiang, P, Chai, J, Jiang, Y, Li, D, Bao, W, et al. The delivery of sensitive food bioactive ingredients: absorption mechanisms, influencing factors, encapsulation techniques and evaluation models. Food Res Int. (2019) 120:130–40. doi: 10.1016/j.foodres.2019.02.024
115. Sharif, N, Khoshnoudi-Nia, S, and Jafari, SM. Nano/microencapsulation of anthocyanins; a systematic review and meta-analysis. Food Res Int. (2020) 132:109077. doi: 10.1016/j.foodres.2020.109077
116. Faridi Esfanjani, A, Assadpour, E, and Jafari, SM. Improving the bioavailability of phenolic compounds by loading them within lipid-based nanocarriers. Trends Food Sci Technol. (2018) 76:56–66. doi: 10.1016/j.tifs.2018.04.002
117. Aguilar-Pérez, KM, Ruiz-Pulido, G, Medina, DI, Parra-Saldivar, R, and Iqbal, HMN. Insight of nanotechnological processing for nano-fortified functional foods and nutraceutical—opportunities, challenges, and future scope in food for better health. Crit Rev Food Sci Nutr. (2021) 1: 1–18. doi: 10.1080/10408398.2021.2004994
118. Berenguer, CV, Andrade, C, Pereira, JAM, and Perestrelo, R. Current challenges in the sustainable valorisation of agri-food wastes: a review. Processes. (2023) 11, 1–20. doi: 10.3390/pr11010020
119. Brito, TBN, Ferreira, MSL, and Fai, AEC. Utilization of agricultural by-products: bioactive properties and technological applications. Food Rev Int. (2022) 38:1305–29. doi: 10.1080/87559129.2020.1804930
120. Udenigwe, CC. From waste to wealth: practical considerations for food waste and byproduct upcycling to biofunctional components. ACS Physical Chemistry Au. (2022) 3:15–16. doi: 10.1021/acsfoodscitech.2c00379
121. Pereira, JAM, Berenguer, CV, CFP, A, and Câmara, JS. Unveiling the bioactive potential of fresh fruit and vegetable waste in human health from a consumer perspective. Appl Sci. (2022) 12:2747. doi: 10.3390/app12052747
122. Anusha, S, Anwar, S, Mohammed, B, Ahmad, G, and Mousavi, A. The potential of apricot seed and oil as functional food: composition, biological properties, health benefits & safety. Food Biosci. (2023) 51:102336. doi: 10.1016/j.fbio.2022.102336
123. Cecilia, JA, García-Sancho, C, Maireles-Torres, PJ, and Luque, R. Industrial food waste valorization: A general overview In: J Schmidt, editor. Biorefinery. Berlin: Springer (2019)
124. FAO. FAO key facts on food loss and waste you should know!. (2022). Available at: http://www.fao.org/save-food/%0Aresources/keyfindings/en/
125. Gustavsson, J., Cederberg, C., and Sonesson, U. Global food losses and food waste. (2011). Available at: http://www.fao.org/fileadmin/user_upload/suistainability/pdf/Global_Food_Losses_and_Food_Waste.pdf
126. Murray, A, Skene, K, Haynes, K, Murray, A, Skene, K, and Haynes, K. The circular economy: an interdisciplinary exploration of the concept and application in a global context. J Bus Ethics. (2017) 140:369–80. doi: 10.1007/s10551-015-2693-2
127. Read, QD, Brown, S, Cuéllar, AD, Finn, SM, Gephart, JA, Marston, LT, et al. Science of the Total Environment Assessing the environmental impacts of halving food loss and waste along the food supply chain. Sci Total Environ. (2020) 712:136255. doi: 10.1016/j.scitotenv.2019.136255
128. Resolution Adopted by the General Assembly (2015). Transforming Our World: The 2030 Agenda for Sustainable Development. 2015 16301:1–35. http://www.un.org/ga/search/view_doc.asp?symbol=A/RES/70/1&Lang=E
129. Delgado, AN. Our environment: everything is natural on earth, but … editorial piece on current and future soil and environmental research. Processes. (2023) 11:10–3. doi: 10.3390/pr11010006
130. Henz, GP. Postharvest losses of perishables in Brazil: what do we know so far? Hortic Bras. (2017) 35:6–13. doi: 10.1590/S0102-053620170102
131. Saini, A, Panesar, PS, and Bera, MB. Valorization of fruits and vegetables waste through green extraction of bioactive compounds and their nanoemulsions – based delivery system. Bioresour Bioprocess. (2019) 1:1–13. doi: 10.1186/s40643-019-0261-9
132. Jiménez-Moreno, N, Esparza, I, Bimbela, F, Gandía, LM, and Ancín-Azpilicueta, C. Valorization of selected fruit and vegetable wastes as bioactive compounds: opportunities and challenges. Crit Rev Environ Sci Technol. (2020) 50:2061–108. doi: 10.1080/10643389.2019.1694819
133. Petkova, D, Mihaylova, D, and Desseva, I. Microencapsulation in food industry – an overview. BIO Web Conf. (2022) 45:02005. doi: 10.1051/bioconf/20224502005
134. Marenda, FRB, Mattioda, F, Demiate, IM, de Francisco, A, de Oliveira Petkowicz, CL, Canteri, MHG, et al. Advances in studies using vegetable wastes to obtain pectic substances: a review. J Polym Environ. (2019) 27:549–60. doi: 10.1007/s10924-018-1355-8
135. Câmara, JS, Albuquerque, BR, Aguiar, J, Corrêa, RCG, Gonçalves, JL, Granato, D, et al. Food bioactive compounds and emerging techniques for their extraction: polyphenols as a case study. Foods. (2020) 10:37. doi: 10.3390/foods10010037
136. Feng, J, Wu, Y, Zhang, L, Li, Y, Liu, S, Wang, H, et al. Enhanced chemical stability, intestinal absorption, and intracellular antioxidant activity of cyanidin-3-O-glucoside by composite nanogel encapsulation. J Agric Food Chem. (2019) 67:10432–47. doi: 10.1021/acs.jafc.9b04778
137. Shen, Y, Zhang, N, Tian, J, Xin, G, Liu, L, Sun, X, et al. Advanced approaches for improving bioavailability and controlled release of anthocyanins. J Control Release. (2022) 341:285–99. doi: 10.1016/j.jconrel.2021.11.031
138. Juurlink, BHJ, Azouz, HJ, Aldalati, AMZ, Altinawi, BMH, and Ganguly, P. Hydroxybenzoic acid isomers and the cardiovascular system. Nutr J. (2014) 13:63. doi: 10.1186/1475-2891-13-63
139. Quiroz-figueroa, FR, and Monribot-villanueva, JL. Proteometabolomic analysis reveals molecular features associated with grain size and antioxidant properties amongst chickpea (Cicer arietinum L.). Seeds Genotypes. (2022) 11:1850. doi: 10.3390/antiox11101850
140. Grzelak-Błaszczyk, K, Milala, J, Kołodziejczyk, K, Sójka, M, Czarnecki, A, Kosmala, M, et al. Protocatechuic acid and quercetin glucosides in onions attenuate changes induced by high fat diet in rats. Food Funct. (2020) 11:3585–97. doi: 10.1039/c9fo02633a
141. Faggian, M, Bernab, G, Valente, M, Francescato, S, Baratto, G, Brun, P, et al. Characterization of PACs profile and bioactivity of a novel nutraceutical combining cranberry extracts with different PAC-A oligomers, D-mannose and ascorbic acid: an in vivo/ex vivo evaluation of dual mechanism of action on intestinal barrier and urinary epithelium. Food Res Int. (2021) 149:110649. doi: 10.1016/j.foodres.2021.110649
142. Chambers, SA, Gaddy, JA, and Townsend, SD. Synthetic ellagic acid glycosides inhibit early stage adhesion of Streptococcus agalactiae biofilms as observed by scanning electron microscopy. Chemistry. (2020) 26:9923–8. doi: 10.1002/chem.202000354
143. Gupta, A, Singh, AK, Kumar, R, Jamieson, S, Pandey, AK, and Bishayee, A. Neuroprotective potential of ellagic acid: a critical review. Adv Nutr. (2021) 13:1211–38. doi: 10.1093/advances/nmab007
144. Artamonova, EA, and Abdullatypov, AV. Ellagic acid derivatives: possible drugs against metapneumoviru?. Preprints.org (2022) 2022100055. doi: 10.20944/preprints202210.0055.v1
145. Mar, L, Sierra-rivera, CA, Cobos-puc, LE, Silva-belmares, SY, and Alberto, J. Antibacterial potential by rupture membrane and antioxidant capacity of purified phenolic fractions of Persea americana leaf extract. Antibiotics. (2021) 10:508. doi: 10.3390/antibiotics10050508
146. Zhong, B, Robinson, NA, Warner, RD, Barrow, CJ, Dunshea, FR, and Suleria, HAR. LC-ESI-QTOF-MS/MS characterization of seaweed Phenolics and their antioxidant potential. Mar Drugs. (2020) 18:331. doi: 10.3390/md18060331
147. Li, B, He, X, Zhang, S, Chang, S, and He, B. Efficient synthesis of 4-O-D-glucopyranosylferulic acid from ferulic acid by whole cells harboring glycosyltransferase GTBP1. Biochem Eng J. (2018) 130:99–103. doi: 10.1021/acs.jafc.9b01856
148. Piazzon, A, Vrhovsek, U, Masuero, D, Mattivi, F, Mandoj, F, and Nardini, M. Antioxidant activity of phenolic acids and their metabolites: synthesis and antioxidant properties of the sulfate derivatives of ferulic and caffeic acids and of the acyl glucuronide of ferulic acid. J Agric Food Chem. (2012, 2012) 60:12312–23. doi: 10.1021/jf304076z
149. Pourová, J, Najmanová, I, Vopr, M, Migkos, T, Pila, V, Applová, L, et al. Two flavonoid metabolites, 3,4-dihydroxyphenylacetic acid and 4-methylcatechol, relax arteries ex vivo and decrease blood pressure in vivo. Vascul Pharmacol. (2018) 111:36–43. doi: 10.1016/j.vph.2018.08.008
150. Saleem, H, Zengin, G, Locatelli, M, Mollica, A, and Ahmad, I. In vitro biological propensities and chemical profiling of Euphorbia milii Des Moul (Euphorbiaceae): a novel source for bioactive agents. Industr. Crops Products. (2019) 130:9–15. doi: 10.1016/j.indcrop.2018.12.062
151. Aggarwal, V, Singh, H, Tania, M, Srivastava, S, Ritzer, EE, Pandey, A, et al. Molecular mechanisms of action of epigallocatechin gallate in cancer: recent trends and advancement. Semin Cancer Biol. (2022) 80:256–75.
152. Fujii, Y, Suzuki, K, Adachi, T, Taira, S, and Osakabe, N. Corticotropin releasing hormone is significantly upregulated in the mouse paraventricular nucleus following a single oral dose of cinnamtannin A2 as an (−) epicatechin tetramer. J Clin Biochem Nutr. (2019) 65:29–33.
153. Jagtap, S, Meganathan, K, Wagh, V, Winkler, J, Hescheler, J, and Sachinidis, A. Chemoprotective mechanism of the natural compounds, epigallocate- chin-3-O-gallate, quercetin and curcumin against cancer and cardiovascular diseases. Curr Med Chem. (2009) 16:1451–62. doi: 10.2174/092986709787909578
154. Kaihatsu, K, Yamabe, M, and Ebara, Y. Antiviral mechanism of action of epigallocatechin-3-O-gallate and its fatty acid esters. Molecules. (2018) 23:2475. doi: 10.3390/molecules23102475
155. Vieira, FN, Lourenço, S, Fidalgo, LG, Santos, SAO, Silvestre, AJD, Jerónimo, E, et al. Long-term effect on bioactive components and antioxidant activity of thermal and high-pressure pasteurization of orange juice. Molecules. (2018) 23:2706. doi: 10.3390/molecules23102706
156. Mitra, S, Subha, M, Mahtab, T, Das, R, Islam, F, Anjum, J, et al. Prospective multifunctional roles and pharmacological potential of dietary flavonoid narirutin. Biomed. Pharmacother. (2022) 150:112932. doi: 10.1016/j.biopha.2022.112932
157. Xiong, H. Hesperidin: a therapeutic agent for obesity. Drug Des Devel Ther. (2019) 13:3855–66. doi: 10.2147/DDDT.S227499
158. Hajialyani, M, Farzaei, MH, Nabavi, SM, and Uriarte, E. Hesperidin as a neuroprotective agent: a review of animal and clinical evidence. Molecules. (2019) 24:648. doi: 10.3390/molecules24030648
159. Semwal, DK, Semwal, RB, Combrinck, S, and Viljoen, A. Myricetin: a dietary molecule with diverse biological activities. Nutrients. (2016) 8:90. doi: 10.3390/nu8020090
160. Pandima, K, Rajavel, T, Habtemariam, S, Fazel, S, and Mohammad, S. Molecular mechanisms underlying anticancer effects of myricetin. Life Sci. (2015) 142:19–25. doi: 10.1016/j.lfs.2015.10.004
161. Guo, X, Zhang, D, Gao, X, Parry, J, Liu, K, and Liu, B. Quercetin and quercetin-3-O-glucuronide are equally effective in ameliorating endothelial insulin resistance through inhibition of reactive oxygen species-associated inflammation. Mol Nutr Food Res. (2013):1037–45. doi: 10.1002/mnfr.201200569
162. Baral, S, Pariyar, R, Kim, J, Lee, H, and Seo, J. Quercetin-3-O-glucuronide promotes the proliferation and migration of neural stem cells. Neurobiol Aging. (2017) 52:39–52. doi: 10.1016/j.neurobiolaging.2016.12.024
163. Pandima, K, Sheeja, D, Fazel, S, Sureda, A, Xiao, J, Mohammad, S, et al. Kaempferol and inflammation: from chemistry to medicine (2015) 99:1–10. doi: 10.1016/j.phrs.2015.05.002
164. Ren, JIE, Lu, Y, Qian, Y, Chen, B, Wu, TAO, and Ji, G. Recent progress regarding kaempferol for the treatment of various diseases (review). Exp Ther Med. (2019) 18:2759–76. doi: 10.3892/etm.2019.7886
165. Herrera-Balandrano, DD, Chai, Z, Beta, T, Feng, J, and Huang, W. Blueberry anthocyanins: an updated review on approaches to enhancing their bioavailability. Trends Food Sci Technol. (2021) 118:808–21. doi: 10.1016/j.tifs.2021.11.006
166. Herrera-Rocha, KM, Rocha-Guzmán, NE, Gallegos-Infante, JA, González-Laredo, RF, Larrosa-Pérez, M, and Moreno-Jiménez, MR. Phenolic acids and flavonoids in acetonic extract from quince (Cydonia oblonga mill.). Molecules. (2022) 27:2462. doi: 10.3390/molecules27082462
167. Wang, Z, Barrow, CJ, and Dunshea, FR. A comparative investigation on phenolic composition, characterization and antioxidant potentials of five different Australian grown pear varieties. Antioxidants. (2021) 10:151. doi: 10.3390/antiox10020151
168. Zapata-campos, CC, García-martínez, JE, Salinas-chavira, J, and Ascacio-valdés, JA. Chemical composition and nutritional value of leaves and pods of Leucaena leucocephala, prosopis laevigata and Acacia farnesiana in a xerophilous shrubland. Emir J Food Agric. (2020) 32:723–30. doi: 10.9755/ejfa.2020.v32.i10.2148
169. Szczepa, J, and De, VU. Changes in the polyphenolic profile and oxidoreductases activity under static and multi-pulsed high pressure processing of cloudy apple juice. Food Chem. (2022) 384:132439. doi: 10.1016/j.foodchem.2022.132439
170. Mazewski, C, Kim, MS, and de Mejia, EG. Anthocyanins, delphinidin-3-O-glucoside and cyanidin-3-O-glucoside, inhibit immune checkpoints in human colorectal cancer cells in vitro and in silico. Sci Rep. (2019) 9:11560. doi: 10.1038/s41598-019-47903-0
171. Viegas, O, Faria, MA, Sousa, JB, Vojtek, M, Gonçalves-monteiro, S, Suliburska, J, et al. Delphinidin-3-O-glucoside inhibits angiogenesis via VEGFR2 downregulation and migration through actin disruption (2019) 54:393–402.
172. León-gonzález, AJ, Sharif, T, and Kayali, A. Delphinidin-3-O-glucoside and delphinidin-3-O-rutinoside mediate the redox- sensitive caspase 3-related pro-apoptotic effect of blackcurrant juice on leukaemia Jurkat cells (2015) 17:847–56. doi: 10.1016/j.jff.2015.06.043
173. Legua, P, Forner-Giner, MA, Nuncio-Jáuregui, N, and Hernández, F. Polyphenolic compounds, anthocyanins and antioxidant activity of nineteen pomegranate fruits: a rich source of bioactive compounds. J Funct Foods. (2016) 23:628–36. doi: 10.1016/j.jff.2016.01.043
174. Xu, Y, Xie, L, Xie, J, Liu, Y, and Chen, W. Pelargonidin-3-O-rutinoside as a novel α-glucosidase inhibitor for improving postprandial hyperglycemia. Chem Commun. (2019) 55:39–42. doi: 10.1039/C8CC07985D
175. Gowd, V, Karim, N, Xie, L, Rezaul, M, Shishir, I, Xu, Y, et al. In vitro study of bioaccessibility, antioxidant, and α -glucosidase inhibitory effect of pelargonidin-3-O-glucoside after interacting with beta-lactoglobulin and chitosan/pectin. Int J Biol Macromol. (2020) 154:380–9.
176. Rocchetti, G, Chiodelli, G, Giuberti, G, Masoero, F, Trevisan, M, and Lucini, L. Evaluation of phenolic profile and antioxidant capacity in gluten-free flours. Food Chem. (2017) 228:367–73. doi: 10.1016/j.foodchem.2017.01.142
177. Buenrostro-figueroa, JJ, Velázquez, M, Flores-ortega, O, and Ascacio-valdés, JA. Solid state fermentation of fig (Ficus carica L.) by-products using fungi to obtain phenolic compounds with antioxidant activity and qualitative evaluation of phenolics obtained. Proc Biochem. (2017) 62:16–23. doi: 10.1016/j.procbio.2017.07.016
178. Malaguarnera, L. Influence of resveratrol on the immune response. Nutrients. (2019) 11:946. doi: 10.3390/nu11050946
179. Singh, AP, and Kaschula, CH. Health benefits of resveratrol: evidence from clinical studies. Med Res Rev. 2019, 39:1851–91. doi: 10.1002/med.21565
180. Nasrollahzadeh, M, Sajjadi, M, Iravani, S, and Varma, RS. Starch, cellulose, pectin, gum, alginate, chitin and chitosan derived (nano)materials for sustainable water treatment: a review. Carbohydr Polym. (2021) 251:116986. doi: 10.1016/j.carbpol.2020.116986
181. Assadpour, E, and Jafari, SM. An overview of biopolymer nanostructures for encapsulation of food ingredients In:. Biopolymer nanostructures for food encapsulation purposes. ed. Jafari, S Amsterdam: Elsevier Inc (2019). 1–35.
182. Li, D, Xu, F, and Li, J. Chapter 4 – Pectin-based micro- and nanomaterials in drug delivery. in Micro and Nano technologies, eds. S. Jana, and S. Jana. (2022) 97–125.
183. Rehman, A, Mahdi, S, Tong, Q, Riaz, T, Assadpour, E, Muhammad, R, et al. Drug nanodelivery systems based on natural polysaccharides against different diseases. Adv Colloid Interface Sci. (2020) 284:102251. doi: 10.1016/j.cis.2020.102251
184. Arroyo-Maya, IJ, and McClements, DJ. Biopolymer nanoparticles as potential delivery systems for anthocyanins: fabrication and properties. Food Res Int. (2015) 69:1–8. doi: 10.1016/j.foodres.2014.12.005
185. Reichembach, LH, Lúcia, C, and Petkowicz, DO. Food hydrocolloids Pectins from alternative sources and uses beyond sweets and jellies: an overview. Food Hydrocoll. (2021) 118:106824. doi: 10.1016/j.foodhyd.2021.106824
186. Sabater, C, Villamiel, M, and Montilla, A. Food hydrocolloids integral use of pectin-rich by-products in a biorefinery context: a holistic approach. Food Hydrocoll. (2022) 128:107564. doi: 10.1016/j.foodhyd.2022.107564
187. Roy, MC, Alam, M, Saeid, A, Das, BC, Mia, MB, Rahman, MA, et al. Extraction and characterization of pectin from pomelo peel and its impact on nutritional properties of carrot jam during storage. J Food Process Preserv. (2018) 42:e13411. doi: 10.1111/jfpp.13411
188. Dranca, F, and Oroian, M. Extraction, Puri fi cation and characterization of pectin from alternative sources with potential technological applications. Food Res Int. (2018) 113:327–50. doi: 10.1016/j.foodres.2018.06.065
189. Carvalho, R, Soares, SD, Martins, MG, Alves, C, Otávio, J, Silva, C, et al. Bioactive, technological-functional potential and morphological structures of passion fruit albedo (Passiflora edulis). Food Sci. Technol. (2022) 2061:1–10. doi: 10.1590/fst.22222
190. Wang, L, Xu, H, Yuan, F, Fan, R, and Gao, Y. Preparation and physicochemical properties of soluble dietary fiber from orange peel assisted by steam explosion and dilute acid soaking. Food Chem. (2015) 185:90–8. doi: 10.1016/j.foodchem.2015.03.112
191. Adetunji, LR, Adekunle, A, Orsat, V, and Raghavan, V. Advances in the pectin production process using novel extraction techniques: a review. Food Hydrocoll. (2017) 62:239–50. doi: 10.1016/j.foodhyd.2016.08.015
192. Dent, M. Ultrasound assisted extraction and characterization of pectin from tomato waste. Food Chem. (2016) 198:93–100. doi: 10.1016/j.foodchem.2015.11.095
193. Mollea, C, Chiampo, F, and Conti, R. Food chemistry extraction and characterization of pectins from cocoa husks: a preliminary study. Food Chem. (2008) 107:1353–6. doi: 10.1016/j.foodchem.2007.09.006
194. Xu, Y, Zhang, L, Bailina, Y, Ge, Z, Ding, T, Ye, X, et al. Effects of ultrasound and/or heating on the extraction of pectin from grapefruit peel. J. Food Eng. (2014) 126:72–81. doi: 10.1016/j.jfoodeng.2013.11.004
195. Moorthy, IG, Maran, JP, Surya, SM, Naganyashree, S, and Shivamathi, CS. Response surface optimization of ultrasound assisted extraction of pectin from pomegranate peel. Int J Biol Macromol. (2015) 72:1323–8. doi: 10.1016/j.ijbiomac.2014.10.037
196. Kulkarni, SG, and Vijayanand, P. Effect of extraction conditions on the quality characteristics of pectin from passion fruit peel (Passiflora edulis f. flavicarpa L.). LWT – Food Sci Technol. (2010) 43:1026–31.
197. Berardini, N, Kno, M, Schieber, A, and Carle, R. Utilization of mango peels as a source of pectin and polyphenolics. Innovat Food Sci Emerg Technol. (2005) 6:442–52.
198. Gopi, D, Kanimozhi, K, Bhuvaneshwari, N, Indira, J, and Kavitha, L. Novel banana peel pectin mediated green route for the synthesis of hydroxyapatite nanoparticles and their spectral characterization. Spectrochim Acta A Mol Biomol Spectrosc. (2014) 118:589–97. doi: 10.1016/j.saa.2013.09.034
199. Yuliarti, O, Goh, KKT, Matia-merino, L, Mawson, J, and Brennan, C. Extraction and characterisation of pomace pectin from gold kiwifruit (Actinidia chinensis). Food Chem. (2015) 187:290–6. doi: 10.1016/j.foodchem.2015.03.148
200. Dogan Ergin, A, Bayindir, ZS, Ozcelikay, AT, and Yuksel, N. A novel delivery system for enhancing bioavailability of S-adenosyl-L-methionine: pectin nanoparticles-in-microparticles and their in vitro - in vivo evaluation. J Drug Deliv Sci Technol. (2021) 61:102096. doi: 10.1016/j.jddst.2020.102096
201. Fang, Z, and Bhandari, B. Encapsulation of polyphenols – a review. Trends Food Sci Technol. (2010) 21:510–23. doi: 10.1016/j.tifs.2010.08.003
202. Marisa Ribeiro, A, Estevinho, BN, and Rocha, F. Microencapsulation of polyphenols – the specific case of the microencapsulation of Sambucus Nigra L. extracts – a review. Trends Food Sci Technol. (2020) 105:454–67. doi: 10.1016/j.tifs.2019.03.011
203. Katouzian, I, and Jafari, SM. Nano-encapsulation as a promising approach for targeted delivery and controlled release of vitamins. Trends Food Sci Technol. (2016) 53:34–48. doi: 10.1016/j.tifs.2016.05.002
204. Shishir, MRI, Xie, L, Sun, C, Zheng, X, and Chen, W. Advances in micro and nano-encapsulation of bioactive compounds using biopolymer and lipid-based transporters. Trends Food Sci Technol. (2018) 78:34–60. doi: 10.1016/j.tifs.2018.05.018
205. Mohammadi, A, Jafari, SM, Assadpour, E, and Faridi, EA. Nano-encapsulation of olive leaf phenolic compounds through WPC-pectin complexes and evaluating their release rate. Int J Biol Macromol. (2016) 82:816–22. doi: 10.1016/j.ijbiomac.2015.10.025
206. Antonov, YA, Celus, M, Kyomugasho, C, Hendrickx, M, Moldenaers, P, and Cardinaels, R. Complexation of pectins varying in overall charge with lysozyme in aqueous buffered solutions. Food Hydrocoll. (2019) 94:268–78. doi: 10.1016/j.foodhyd.2019.02.049
207. Antonov, YA, Zhuravleva, IL, Celus, M, Kyomugasho, C, Hendrickx, M, Moldenaers, P, et al. Effect of overall charge and local charge density of pectin on the structure and thermal stability of lysozyme. J Therm Anal Calorim. (2021) 147:6271–86. doi: 10.1007/s10973-021-10954-5
208. Kasote, D, RNT, J, KJD, S, Itagi, H, Roy, P, Kohli, A, et al. Food processing technologies to develop functional foods with enriched bioactive phenolic compounds in cereals. Front Plant Sci. (2021) 12:771276. doi: 10.3389/fpls.2021.771276
209. Rosales, TKO, and Fabi, JP. Pectin-based nanoencapsulation strategy to improve the bioavailability of bioactive compounds. Int J Biol Macromol. (2023) 229:11–21. doi: 10.1016/j.ijbiomac.2022.12.292
210. Mcclements, DJ, Decker, EA, and Park, Y. Structural design principles for delivery of bioactive components in nutraceuticals and functional foods. Crit Rev Food Sci Nutr. (2009) 49:577–606. doi: 10.1080/10408390902841529
211. Ahmad, A, Gulraiz, Y, Ilyas, S, and Bashir, S. Polysaccharide based nano materials: health implications. Food Hydrocoll Health. (2022) 2:100075. doi: 10.1016/j.fhfh.2022.100075
212. Carrasco-Sandoval, J, Aranda-Bustos, M, Henríquez-Aedo, K, López-Rubio, A, and Fabra, MJ. Bioaccessibility of different types of phenolic compounds co-encapsulated in alginate/chitosan-coated zein nanoparticles. LWT. (2021) 149:112024. doi: 10.1016/j.lwt.2021.112024
213. Tian, S, Xue, X, Wang, X, and Chen, Z. Preparation of starch-based functional food nano-microcapsule delivery system and its controlled release characteristics. Front Nutr. (2022) 9:982370. doi: 10.3389/fnut.2022.982370
214. Li, X-L, Xie, Q-T, Liu, W-J, Xu, B-C, and Zhang, B. Self-assembled pea protein isolate nanoparticles with various sizes: explore the formation mechanism. J Agric Food Chem. (2021) 69:9905–14. doi: 10.1021/acs.jafc.1c02105
215. Zhao, X, Zhang, X, Tie, S, Hou, S, Wang, H, Song, Y, et al. Food hydrocolloids facile synthesis of nano-nanocarriers from chitosan and pectin with improved stability and biocompatibility for anthocyanins delivery: an in vitro and in vivo study. Food Hydrocoll. (2020) 109:106114. doi: 10.1016/j.foodhyd.2020.106114
216. Gonçalves, RFS, Martins, JT, Duarte, CMM, Vicente, AA, and Pinheiro, AC. Advances in nutraceutical delivery systems: from formulation design for bioavailability enhancement to efficacy and safety evaluation. Trends Food Sci Technol. (2018) 78:270–91. doi: 10.1016/j.tifs.2018.06.011
217. Zhao, X, Zhang, X, Tie, S, Hou, S, Wang, H, Song, Y, et al. Facile synthesis of nano-nanocarriers from chitosan and pectin with improved stability and biocompatibility for anthocyanins delivery: an in vitro and in vivo study. Food Hydrocoll. (2020) 109:106114. doi: 10.1016/j.foodhyd.2020.106114
218. Huang, X, Liu, Y, Zou, Y, Liang, X, Peng, Y, McClements, DJ, et al. Encapsulation of resveratrol in zein/pectin core-shell nanoparticles: stability, bioaccessibility, and antioxidant capacity after simulated gastrointestinal digestion. Food Hydrocoll. (2019) 93:261–9. doi: 10.1016/j.foodhyd.2019.02.039
219. Rehman, A, Ahmad, T, Aadil, RM, Spotti, MJ, Bakry, AM, Khan, IM, et al. Pectin polymers as wall materials for the nano-encapsulation of bioactive compounds. Trends Food Sci Technol. (2019) 90:35–46. doi: 10.1016/j.tifs.2019.05.015
220. Hassan, E, Fadel, S, Abou-elseoud, W, and Mahmoud, M. Cellulose nanofibers/pectin/pomegranate extract nanocomposite as antibacterial and antioxidant films and coating for paper. Polymers. (2022) 14:4605. doi: 10.3390/polym14214605
221. Borges, V, Maciel, V, Yoshida, CMP, and Teixeira, T. Chitosan/pectin polyelectrolyte complex as a pH indicator. Carbohydr Polym. (2015) 132:537–45. doi: 10.1016/j.carbpol.2015.06.047
222. Liang, J, Cao, L, Zhang, L, and Wan, X. Preparation, characterization, and in vitro antitumor activity of folate conjugated chitosan coated EGCG nanoparticles. Food Sci Biotechnol. (2014) 23:569–75. doi: 10.1007/s10068-014-0078-4
223. Ge, J, Yue, X, Wang, S, Chi, J, Liang, J, Sun, Y, et al. Nanocomplexes composed of chitosan derivatives and β-Lactoglobulin as a carrier for anthocyanins: preparation, stability and bioavailability in vitro. Food Res Int. (2019) 116:336–45. doi: 10.1016/j.foodres.2018.08.045
224. He, B, Ge, J, Yue, P, Yue, XY, Fu, R, Liang, J, et al. Loading of anthocyanins on chitosan nanoparticles influences anthocyanin degradation in gastrointestinal fluids and stability in a beverage. Food Chem. (2017) 221:1671–7. doi: 10.1016/j.foodchem.2016.10.120
225. Ing, BHU, An, CHP, Un, YIS, Ou, ZHH, Ong, HYE, and Ing, BHU. Optimization of fabrication parameters to produce chitosan – tripolyphosphate nanoparticles for delivery of tea catechins. J Agric Food Chem. (2008) 56:7451–8. doi: 10.1021/jf801111c
226. Liang, J, Yan, H, Yang, HJ, Kim, HW, Wan, X, Lee, J, et al. Synthesis and controlled-release properties of chitosan/β-Lactoglobulin nanoparticles as carriers for oral administration of epigallocatechin gallate. Food Sci Biotechnol. (2016) 25:1583–90. doi: 10.1007/s10068-016-0244-y
227. Bulatao, RM, Samin, JPA, Salazar, JR, and Monserate, JJ. Encapsulation of anthocyanins from black rice (Oryza Sativa L.) bran extract using chitosan-alginate nanoparticles. J Food Res. (2017) 6:40. doi: 10.5539/jfr.v6n3p40
228. Ko, A, Lee, JS, Sop Nam, H, and Gyu, LH. Stabilization of black soybean anthocyanin by chitosan nanoencapsulation and copigmentation. J Food Biochem. (2017) 41, 5264–5271. doi: 10.1111/jfbc.12316
229. He, Y, Chen, D, Liu, Y, Sun, X, Guo, W, An, L, et al. Protective effect and mechanism of soybean insoluble dietary fiber on the color stability of malvidin-3- O-glucoside. Foods. (2022) 11:1474. doi: 10.3390/foods11101474
230. Chatterjee, NS, Dara, PK, Perumcherry Raman, S, Vijayan, DK, Sadasivam, J, Mathew, S, et al. Nanoencapsulation in low-molecular-weight chitosan improves in vivo antioxidant potential of black carrot anthocyanin. J Sci Food Agric. (2021) 101:5264–71. doi: 10.1002/jsfa.11175
231. Karim, N, Rezaul, M, Shishir, I, Li, Y, Zineb, OY, Mo, J, et al. Pelargonidin- 3 - O -Glucoside encapsulated pectin-chitosan-nanoliposomes recovers Palmitic acid-induced hepatocytes injury. Antioxidants (2022) 11:623. doi: 10.3390/antiox11040623
232. Li, X, Zhou, P, Luo, Z, Feng, R, and Wang, L. Hohenbuehelia serotina polysaccharides self-assembled nanoparticles for delivery of quercetin and their anti-proliferative activities during gastrointestinal digestion in vitro. Int J Biol Macromol. (2022) 203:244–55.
233. Chen, C, Li, Z, Wang, C, Liu, S, Wang, Y, Zhang, M, et al. Stability and antioxidant activity of chitosan/β-Lactoglobulin on anthocyanins from Aronia melanocarpa. LWT – Food Sci Technol. (2023) 173:114335. doi: 10.1016/j.lwt.2022.114335
234. Yang, Z, Julian, D, Peng, X, Xu, Z, Meng, M, Chen, L, et al. Fabrication of zein – carboxymethyl cellulose nanoparticles for co-delivery of quercetin and resveratrol. J Food Eng. (2023) 341:111322. doi: 10.3390/ma15248711
235. Akhavan, S, Assadpour, E, Katouzian, I, and Jafari, SM. Lipid nano scale cargos for the protection and delivery of food bioactive ingredients and nutraceuticals. Trends Food Sci Technol. (2018) 74:132–46. doi: 10.1016/j.tifs.2018.02.001
236. Garavand, F, Jalai-jivan, M, Assadpour, E, and Mahdi, S. Encapsulation of phenolic compounds within nano/microemulsion systems: a review. Food Chem. (2021) 364:130376. doi: 10.1016/j.foodchem.2021.130376
237. Cao, N, Tang, X, Gao, RJ, Kong, L, Zhang, J, Qin, W, et al. Galectin-3 participates in PASMC migration and proliferation by interacting with TGF-β1. Life Sci. (2021) 274:119347. doi: 10.1016/j.lfs.2021.119347
238. Antonov, YA, Celus, M, Kyomugasho, C, Hendrickx, M, Moldenaers, P, and Cardinaels, R. Complexation of pectins varying in overall charge with lysozyme in aqueous buffered solutions. Food Hydrocoll. (2019) 94:268–78. doi: 10.1016/j.foodhyd.2019.02.049
239. Ahirrao, SP, Gide, PS, Shrivastav, B, and Sharma, P. Ionotropic gelation: a promising cross linking technique for hydrogels. Res Rev J Pharm Nanotechnol. (2014) 2:1–6. doi: 10.3390/pharmaceutics15010108
240. Prabaharan, M, and Mano, JF. Chitosan-based particles as controlled drug delivery systems. Drug Deliv J Deliv Target Ther Agents. (2005) 12:41–57. doi: 10.1080/10717540590889781
241. Andersen, T, Auk-emblem, P, and Dornish, M. 3D cell culture in alginate hydrogels. Microarrays. (2015) 4:133–61. doi: 10.3390/microarrays4020133
242. Ban, E, and Kim, A. Coacervates: recent developments as nanostructure delivery platforms for therapeutic biomolecules. Int J Pharm. (2022) 624:122058. doi: 10.1016/j.ijpharm.2022.122058
243. Singh, AN, and Yethiraj, A. Driving force for the complexation of charged polypeptides. J Phys Chem B. (2020) 124:1285–92. doi: 10.1021/acs.jpcb.9b09553
244. Gummel, J, Boué, F, Demé, B, and Cousin, F. Charge stoichiometry inside polyelectrolyte-protein complexes: a direct SANS measurement for the PSSNa-lysozyme system. J Phys Chem B. (2006) 110:24837–46. doi: 10.1021/jp064383k
245. Chen, S, McClements, DJ, Jian, L, Han, Y, Dai, L, Mao, L, et al. Core-shell biopolymer nanoparticles for co-delivery of curcumin and piperine: sequential electrostatic deposition of hyaluronic acid and chitosan shells on the Zein Core. ACS Appl Mater Interfaces. (2019) 11:38103–15. doi: 10.1021/acsami.9b11782
246. Martínez Rivas, CJ, Tarhini, M, Badri, W, Miladi, K, Greige-Gerges, H, Nazari, QA, et al. Nanoprecipitation process: from encapsulation to drug delivery. Int J Pharm. (2017) 532:66–81. doi: 10.1016/j.ijpharm.2017.08.064
247. Nile, SH, Baskar, V, Selvaraj, D, Nile, A, Xiao, J, and Kai, G. Nanotechnologies in food science: applications, recent trends, and future perspectives. Nano-Micro Lett. (2020) 12:45. doi: 10.1007/s40820-020-0383-9
248. Otchere, E, Mckay, BM, English, MM, and Aryee, ANA. Current trends in nano-delivery systems for functional foods: a systematic review. PeerJ. (2023) 11:e14980. doi: 10.7717/peerj.14980
249. Tan, C, Huang, M, Wang, J, and Sun, B. Biopolyelectrolyte complex (bioPEC)-based carriers for anthocyanin delivery. Food Hydrocoll Heal. (2021) 1:100037. doi: 10.1016/j.fhfh.2021.100037
250. Ait, Y, Gunenc, A, Bendali, F, and Hosseinian, F. LWT - food science and technology simulated gastrointestinal digestion and in vitro colonic fermentation of carob polyphenols: bioaccessibility and bioactivity. LWT – Food Sci Technol. (2020) 117:108623. doi: 10.1016/j.lwt.2019.108623
251. Wu, CL, Chen, QH, Li, XY, Hui, SJ, He, S, Liu, J, et al. Formation and characterisation of food protein–polysaccharide thermal complex particles: effects of pH, temperature and polysaccharide type. Int J Food Sci Technol. (2020) 55:1368–74. doi: 10.1111/ijfs.14416
252. Fernandes, A, Oliveira, J, Fonseca, F, Ferreira-da-Silva, F, Mateus, N, Vincken, JP, et al. Molecular binding between anthocyanins and pectic polysaccharides – unveiling the role of pectic polysaccharides structure. Food Hydrocoll. (2020) 102:105625. doi: 10.1016/j.foodhyd.2019.105625
253. Fernandes, JM, Madalena, DA, Vicente, AA, and Pinheiro, AC. Influence of the addition of different ingredients on the bioaccessibility of glucose released from rice during dynamic in vitro gastrointestinal digestion. Int J Food Sci Nutr. (2021) 72:45–56. doi: 10.1080/09637486.2020.1763926
254. Amos, R, Atmodjo, M, Huang, C, Gao, Z, Venkat, A, Taujale, R, et al. Polymerization of the backbone of the pectic polysaccharide rhamnogalacturonan I. Nat Plants. (2022) 8:1289–303. doi: 10.1038/s41477-022-01270-3
255. Maxwell, EG, Colquhoun, IJ, Chau, HK, Hotchkiss, AT, Waldron, KW, Morris, VJ, et al. Modified sugar beet pectin induces apoptosis of colon cancer cells via an interaction with the neutral sugar side-chains. Carbohydr Polym. (2016) 136:923–9. doi: 10.1016/j.carbpol.2015.09.063
256. Mohnen, D. Pectin structure and biosynthesis. Curr Opin Plant Biol. (2008) 11:266–77. doi: 10.1016/j.pbi.2008.03.006
257. Sriamornsak, P. Application of pectin in oral drug delivery. Drug Deliv. (2011) 8:1009–23. doi: 10.1517/17425247.2011.584867
258. Viebke, C, Al-Assaf, S, and Phillips, GO. Food hydrocolloids and health claims. Bioact Carbohydrates Diet Fibre. (2014) 4:101–14. doi: 10.1016/j.bcdf.2014.06.006
259. Prado, SBR d, Castro-Alves, VC, Ferreira, GF, and Fabi, JP. Ingestion of non-digestible carbohydrates from plant-source foods and decreased risk of colorectal cancer: a review on the biological effects and the mechanisms of action. Front Nutr. (2019) 6:72. doi: 10.3389/fnut.2019.00072
260. Tomas, M. Effect of dietary fiber addition on the content and in vitro bioaccessibility of antioxidants in red raspberry puree. Food Chem. (2022) 375:131897. doi: 10.1016/j.foodchem.2021.131897
261. Zhang, L, Wu, T, Zhang, Y, Chen, Y, Ge, X, Sui, W, et al. Release of bound polyphenols from wheat bran soluble dietary fiber during simulated gastrointestinal digestion and colonic fermentation in vitro. Food Chem. (2023) 402:134111. doi: 10.1016/j.foodchem.2022.134111
262. Dao, A, Phan, T, Flanagan, BM, Arcy, BRD, and Gidley, MJ. Binding selectivity of dietary polyphenols to different plant cell wall components: quantification and mechanism. Food Chem. (2017) 233:216–27. doi: 10.1016/j.foodchem.2017.04.115
263. Simon, S, Hotchkiss, AT, Chau, HK, Strahan, GD, Nu, A, White, AK, et al. Structure and composition of blueberry fiber pectin and xyloglucan that bind anthocyanins during fruit puree processing. Food Hydrocoll. (2021) 116:106572. doi: 10.1016/j.foodhyd.2020.106572
264. Fernandes, A, Ivanova, G, Brás, NF, Mateus, N, Ramos, MJ, Rangel, M, et al. Structural characterization of inclusion complexes between cyanidin-3-O-glucoside and β-cyclodextrin. Carbohydr Polym. (2014) 102:269–77. doi: 10.1016/j.carbpol.2013.11.037
265. Sadeghi, R, Mehryar, L, Karimi, M, and Kokini, J. Nanocapsule formation by individual biopolymer nanoparticles In: SM Jafari, editor. Nanoencapsulation technologies for the food and nutraceutical industries. Amsterdam: Elsevier Inc (2017). 404–46.
266. Walia, N, Dasgupta, N, Ranjan, S, Ramalingam, C, and Gandhi, M. Methods for nanoemulsion and nanoencapsulation of food bioactives. Environ Chem Lett. (2019) 17:1471–83. doi: 10.1007/s10311-019-00886-w
267. Koh, J, Xu, Z, and Wicker, L. Binding kinetics of blueberry pectin-anthocyanins and stabilization by non-covalent interactions. Food Hydrocoll. (2020) 99:105354. doi: 10.1016/j.foodhyd.2019.105354
268. Koh, J, Xu, Z, and Wicker, L. Blueberry pectin and increased anthocyanins stability under in vitro digestion. Food Chem. (2020) 302:125343. doi: 10.1016/j.foodchem.2019.125343
Keywords: antioxidants, bioactive compounds, dietary polyphenols, food industry, dietary supplements, polysaccharides, nanoencapsulation, sustainability
Citation: Rosales TKO and Fabi JP (2023) Valorization of polyphenolic compounds from food industry by-products for application in polysaccharide-based nanoparticles. Front. Nutr. 10:1144677. doi: 10.3389/fnut.2023.1144677
Edited by:
Ana Beltrán Sanahuja, University of Alicante, SpainReviewed by:
Jorge Sánchez-Burgos, Instituto Tecnológico de Tepic, MexicoYong-Quan Xu, Chinese Academy of Agricultural Sciences, China
Copyright © 2023 Rosales and Fabi. This is an open-access article distributed under the terms of the Creative Commons Attribution License (CC BY). The use, distribution or reproduction in other forums is permitted, provided the original author(s) and the copyright owner(s) are credited and that the original publication in this journal is cited, in accordance with accepted academic practice. No use, distribution or reproduction is permitted which does not comply with these terms.
*Correspondence: João Paulo Fabi, anBmYWJpQHVzcC5icg==