- 1Center for Mitochondria and Healthy Aging, College of Life Science, Yantai University, Yantai, Shandong, China
- 2Beijing Key Laboratory of Functional Food from Plant Resources, College of Food Science and Nutritional Engineering, China Agricultural University, Beijing, China
- 3School of Food Science and Technology, Jiangnan University, Wuxi, Jiangsu, China
Introduction: Bioactive peptides based on foodstuffs are of particular interest as carriers for calcium delivery due to their safety and high activity. The phosphorylated peptide has been shown to enhance calcium absorption and bone formation.
Method: A novel complex of peptide phosphorylation modification derived from soybean protein was introduced, and the mechanism, stability, and osteogenic differentiation bioactivity of the peptide with or without calcium were studied.
Result: The calcium-binding capacity of phosphorylated soy peptide (SPP) reached 50.24 ± 0.20 mg/g. The result of computer stimulation and vibration spectrum showed that SPP could chelate with calcium by the phosphoric acid group, carboxyl oxygen of C-terminal Glu, Asp, and Arg, and phosphoric acid group of Ser on the SPP at a stoichiometric ratio of 1:1, resulting in the formation of the complex of ligand and peptide. Thermal stability showed that chelation enhanced peptide stability compared with SPP alone. Additionally, in vitro results showed that SPP-Ca could facilitate osteogenic proliferation and differentiation ability.
Discussion: SPP may function as a promising alternative to current therapeutic agents for bone loss.
1. Introduction
Calcium is an essential element that is crucial for the maintenance of bone health. The deficiency of calcium leads to rickets in children and osteoporosis in adults (1, 2). Numerous calcium supplementation on the market emerged, but many questions remain for various calcium salts with stability and absorption. Ion calcium supplements are easily influenced by phytates, oxalate, and non-fermentable fiber of food and may induce the formation of insoluble calcium salt precipitation under intestinal conditions (3). Thus, calcium-binding peptides from foodstuff became a new source of calcium-enriched nutrients with the potential to overcome the limitations of simple calcium intake.
Bioactive peptides normally contain 2 to 20 amino acid residues and are abundant in hydrophobic amino acids (4). These bioactive motifs exhibited properties such as antihypertensive, antimicrobial, and excellent health effects as antioxidants (5–7). It has been reported that bioactive peptides could chelate with calcium ions to form stable complexes with superior absorptivity based on mineral binding functions (8, 9). Nowadays, various calcium-binding peptides have been obtained from food resources, including phosvitin hydrolysates, porcine plasma protein hydrolysates, and wheat germ protein hydrolysates (10–12). Among them, casein phosphor-peptides have been reported to be desired mineral carriers that can strengthen elemental mineral absorption, mostly chelated with calcium through the negatively charged phosphate group (13).
In our preliminary experiments, DEDEQIPSHPPR dodecapeptide from soy yogurt was confirmed to possess 36.64 ± 0.04 mg/g calcium-chelating capacity (14). Previous studies found that the calcium chelation capacity is closely related to their molecular mass, amino acid compositions, sequences, and spatial conformation (15, 16). The carboxyl groups of Asp and Glu, the δ-N in the imidazole ring of His, and the ε-amino nitrogen of Lys are considered to play a critical role in calcium binding (17). Among them, His mainly depends on the accumulation of cyclic side chains, and nitrogen atoms on the imidazole ring can also be used as hydrogen bond donors and acceptors with calcium ions under different conditions to improve calcium-binding ability (18). However, our study showed that the binding ability of His is obviously lower than that of Asp and Glu (19). Additionally, the primary structure of SPP contains serine residues with free hydroxyl groups, which are sites for the modification with the phosphate group, thus contributing to calcium-binding capacity (20). Luo et al. (21) also confirmed that factors such as phosphate residue and amino acid composition on serine and molecular structure affect the calcium absorption activity and mechanism of CPP. Therefore, we tried to phosphorylate soybean peptides to improve calcium-binding capacity.
Taking all of these into account, the main purpose of this study was to prepare phosphorylated soy peptides calcium complex, to explore its calcium-binding mechanism by computational docking and spectroscopic methods, to investigate the thermal stability and the ability to promote osteogenic proliferation and differentiation in vitro. The study could provide comprehensive descriptions of developing new food therapy options to prevent calcium deficiency.
2. Materials and methods
2.1. Materials
Polypeptide SPP isolated from soy yogurt with a purity of 98% was synthesized by Nanjing Peptide Industry Biotechnology co., ltd. Bile salts, trypsin, and pepsin were products of Sigma–Aldrich (St. Louis, MO). All commercial reagents were of analytical grade.
2.2. Molecular dynamic simulation
The Desmond program was performed to study high-performance molecular dynamic simulation properties for SPP and calcium. The initial SPP structure was parameterized in Maestro 11.8 with Epik protonation, following conducting energy minimization at pH 7.0. Then, the solvent system was developed using the system builder platform under SPC mode building an explicit solvation cube with a 10Å × 10Å × 10Å margin added 0.75 mol/L Ca2+. Meanwhile, the cube was injected with Cl− as a counter ion to equalize the charge on the OPLS_3E force field. The peptide calcium complex system was initially balanced for 100 nanoseconds (ns) using an NVT ensemble. The simulation process is as follows: step 1, Brownian dynamics NVT, T = 10 K, small time steps, restraining on heavy solute atoms, 100 ps; step 2: NVT, T = 10 K, small time steps, controlling of heavy solute atoms, 12 ps; step 3: NPT, T = 10 K, restraining on heavy solute atoms, 12 ps; step 4: NPT, restricting on solute heavy particles, 12 ps; step 5: NPT, no restrictions, 24 ps; step 6: NPT, no restraints, 100 ns. Subsequently, the system stability was determined by analyzing the MD simulation results of 100 ns, including calculating root mean square deviation (RMSD), root mean square equation (RMSF), the radius of gyration (Rg), and the number of hydrogen bonds (H-bonds).
2.3. Calcium-binding capacity analysis
After synthesis, 3 mg/mL SPP and 5 mM CaCl2 were mixed in 20 mM sodium phosphate buffer solution (pH = 7.8) maintained at 40°C for 30 min through a shaker water bath, followed by dialysis to remove other elements and centrifugation (4,000 g, 4°C, 20 min). We collected supernation to determine calcium content inductively coupled plasma-atomic emission spectrometry (ELAN DRC II, PerkinElmer, Waltham, United States).
2.4. Fabrication of peptide–calcium chelate
Peptide–calcium complex preparation was performed following the method of Cai with slight modification (16). In brief, the stock solution of SPP (10 mg/mL) was mixed with CaCl2 at a ratio of 2:1. The pH was adjusted to 8.0 using 1 M NaOH dropwise. After that, the mixture of SPP/CaCl2 was shaken at 37°C at 140 rpm in a water bath for 20 min, and absolute ethanol (90 mL) was added to remove the free calcium. The final solution was, then, placed in a dialysis bag for 48 h and centrifuged for 10 min at 10,000 g. The collected precipitation was lyophilized in a vacuum desiccator (ALPHA1-2LDplus, Marin Christ, Osterode, Germany), and thus, the peptide–calcium chelate was prepared for subsequent experiments.
2.5. Isothermal titration calorimetry (ITC)
Isothermal titration calorimetry was tested for the characterization of the thermodynamic parameters of SPP binding with calcium. Both the peptide and the SPP-Ca chelate of SPP were dissolved in a Tris-HCl buffer solution. The sample was degassed followed by filtration through a 0.22 μm filter. 2.5 mM of DEDEQIPSHPPR was loaded into the ITC cell, and then, the CaCl2 solution (50 mM) was loaded into the ITC syringe. During measurements, 20 drops were injected into the sample cell. The titration parameter was configured to inject CaCl2 every 5 min with volumes of 2.5 μL. Nano Analyze software (TA Instrument-Waters LLC, New Castle, Delaware) was applied for evaluating the raw data based on data fitting.
2.6. Analysis of binding sites of calcium on SPP
2.6.1. Ultraviolet spectroscopy
The ultraviolet absorption spectra of SPP and SPP-Ca were determined utilizing an ultraviolet spectrophotometer (UV-1200, Xiaofen Instrument Co. Ltd., Guangzhou, China). A stock solution of the peptide was prepared (0.02 mg/mL, pH = 8.0), and then 0.2, 0.4, 0.6, and 0.8 mM of CaCl2 were constantly shaking at 50°C for 1 h, and the measurement was conducted over the wavelength ranged from 190 to 400 nm.
2.6.2. Fourier transform infrared spectroscopy
Infrared spectra of samples were obtained by an infrared spectrophotometer (IS50, Thermo Nicolet Co., Waltham, MA) within a scope of 4,000 to 400 cm−1. Pellets were created by mixing the sample with KBr and pressing them to form disks. Circular Dichroism Spectroscopy.
Phosphorylated soy peptide (SPP) and SPP-Ca chelate were dissolved in distilled water, making a final concentration of 0.5 mg/mL. The CD spectra were searched by a Chirascan-plus CD spectrometer (Applied Photophysics, Surrey, UK). All experiments were taken over from 200 to 260 nm with programmed 0.5 nm intervals at 25°C.
2.7. Thermal stability analysis of peptide–calcium chelate in vivo
A thermogravimetric/differential scanning calorimeter (STA449C, Netzsch, Germany) was used to investigate the thermal decomposition of SPP-Ca chelate. Approximately 5 mg of lyophilized sample was weighed and heated from 40 to 600°C with a heating rate of 20°C/min and Nitrogen flow rate of 30 mL/min.
2.8. Cell proliferation assay
The influence of the SPP-Ca chelate on MC3T3-E1 cells promoting osteoblast proliferation was quantified using an MTT assay proposed by Wang et al. (22). In brief, MC3T3-E1 cells at a density of 1 × 104 cells/well were grown in 96-well tissue culture plates for 24 h. The medium was replaced by SPP at concentrations of 0, 0.7, 7, and 70 μM and SPP-Ca at a concentration of 70 μM and incubation for 72 h. For survival assay, the cells were treated with 10 μL MTT solution (5 mg/mL in PBS), and the plate was kept in the incubator for 3 h. After that, 150 μL DMSO was added into each well followed by shaken 96-well plates for 10 min. Finally, the optical density was determined at 570 nm by a microplate reader (Molecular Devices, San Jose, CA, United States).
2.9. ALP activity assay
Alkaline phosphatase (ALP) activity is a recognized osteogenic differentiation marker of MC3T3-E1 cells. Cells were grown in a complete medium at a density of 1 × 104 cells/well and cultured for 48 h. Then, the medium was changed to a differentiation medium. After 4 days of incubation, fresh serum containing various concentrations of SPP (0.7, 7, and 70 μM) and SPP-Ca (70 μM) was added and kept for 24 h at 37°C. Next, the cells were rinsed with PBS buffer, lysed with 70 μL of ice-cold lysis buffer which was supplemented with PMSF per well, and broken down using a cell disruptor (BiLon 92-II, Beijing, China). The ALP activity and protein concentration were tested using an Alkaline Phosphatase Assay Kit at OD405 and a BCA Protein Assay Kit at OD562 nm.
2.10. Statistical analysis
Each experiment was carried out in triplicate, and data were presented as the mean ± SD. The results were subjected to one-way analysis of variance (ANOVA) by the program SPSS Statistics 26.0 (SPSS Institute, Chicago, IL, United States), and the significant statistical differences were set at p < 0.05.
3. Results
3.1. Molecular dynamic simulation of SPP-Ca
Molecular docking was used to visualize the binding site and patterns between the small molecule ligand and the receptor (23). In this study, molecular docking technology was carried out to predict bound conformations of binding for Ca2+ to SPP at a molecular level. Materials Studio 6.0 software package was performed to calculate the docking results accurately. The structure of peptides was optimized on a model based on the density functional theory. The molecular dynamics simulation snapshot (0, 20, 50, and 100 ns) of SPP-calcium chelate is shown in Figure 1A. The RMSD presented a significant tendency to be stable ranging from 2 to 6 Å with the increasing of snapshot. As expected, SPP has undergone a marked conformational change, with various number of contiguous ion binding sites ranging from 0 to 5. For 0 ns, calcium ions were uniformly distributed in the energy minimization system. Subsequently, the number of binding sites gradually increased and reached a stable value of 5 after 100 ns (Figure 1B). Many studies revealed that the presence of negatively charged acidic amino groups was responsible for the covalent bonding of metal ions (4, 12), which was also supported by Figure 1C. In addition, phosphate groups are also involved in chelation. Thus, the result demonstrated the possibility of SPP binding to calcium ions.
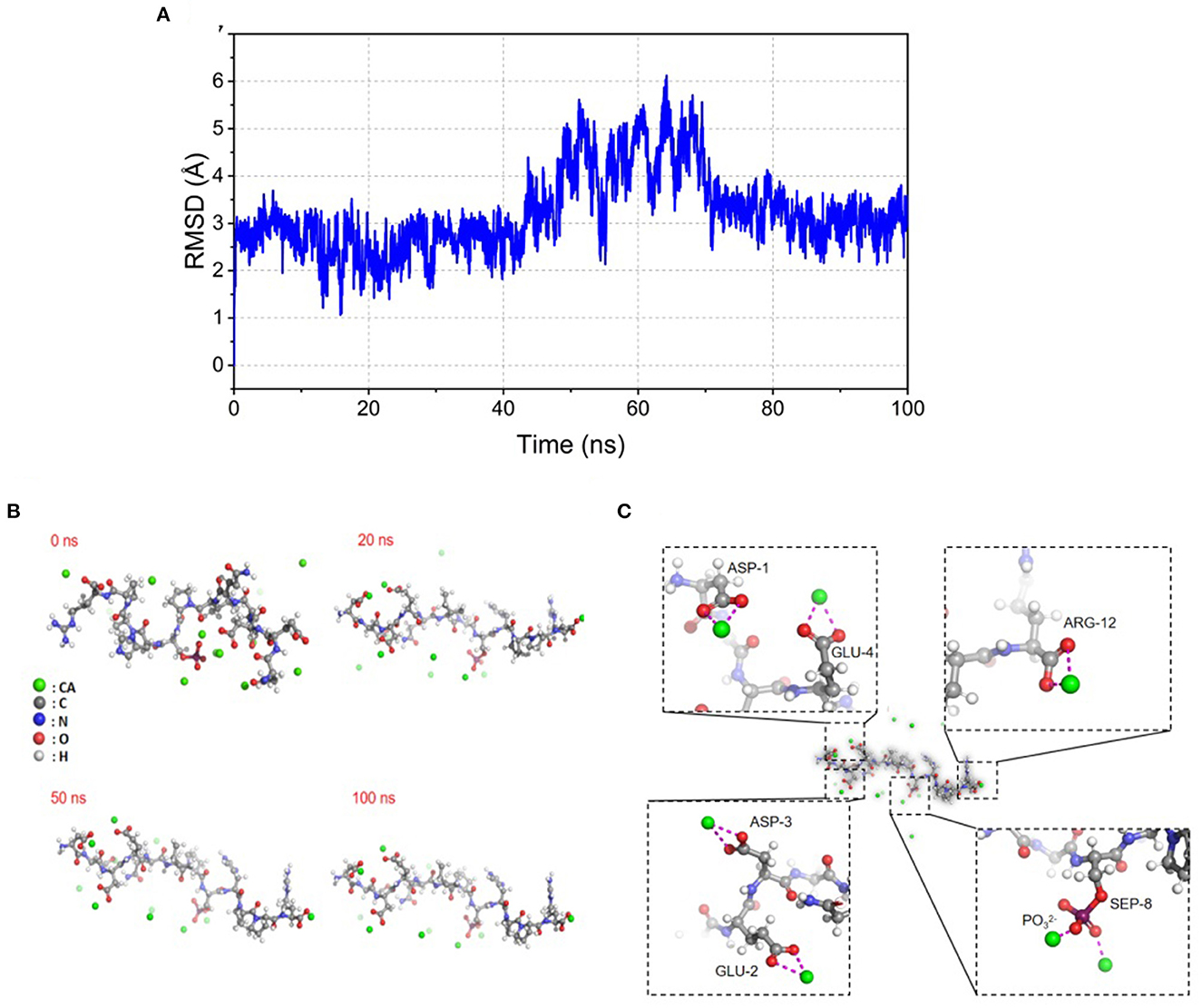
Figure 1. Illustration of molecular dynamic analysis simulation for SPP-Ca. (A) RMSD stability analysis, changes of RMSD of SPPs with simulated time during molecular docking; (B) snapshots of molecular dynamics simulation of SPP-calcium chelate at (0, 20, 50, and 100 ns), the green ball represents Ca2+, the gray ball represents carbon atom on amino acid, the blue ball represents nitrogen atom on amino acid, the red ball represents oxygen atom on amino acid, and the white ball represents hydrogen atom on amino acid; (C) molecular dynamic analysis simulation chelation of SPP-Ca complex based on density functional theory calculation in the Materials Studio 6.0 software package at 100 ns.
3.2. Determination of the binding stoichiometry and binding constant with SPP-calcium complex
3.2.1. Analysis of the calcium-binding activity of the SPP
In our study, SPP was confirmed to possess a calcium-binding capacity of 50.24 ± 0.20 mg/g. Moreover, the molecular weight of SPP was 750.65 Da determined by LC-MS, as shown in the Supplementary Figure 1, supporting prior research that the peptide with low molecular weight demonstrates an enhanced affinity to calcium (24, 25). The high proportion of acidic amino acids in SPP also has been reported to contribute to calcium chelation (26, 27). Therefore, SPP could act as an effective carrier in delivering calcium.
3.2.2. Determination of the binding stoichiometry and binding constant
Isothermal titration calorimetry (ITC) is a powerful technique for understanding the interaction of ligand-binding peptide (28). The thermodynamic parameters showed that binding to calcium generated negative changes in enthalpy and entropy values (ΔH = −2.224 kJ/mol, ΔG = −24.02 kJ/mol), which demonstrated that the reaction occurs spontaneously and is an exothermic process (Figure 2). In addition, the exothermic reaction yielded by the formation of a coordination bond between Ca2+ and phosvitin exerted a negative effect on ΔH. Meanwhile, the high absolute value of ΔS (66.40 J/mol·K) revealed that the association process was electrostatic interaction-driven. The estimated stoichiometric ratio (n) was 1.577, manifesting that the stoichiometric ratio of peptide and calcium was 1:1.
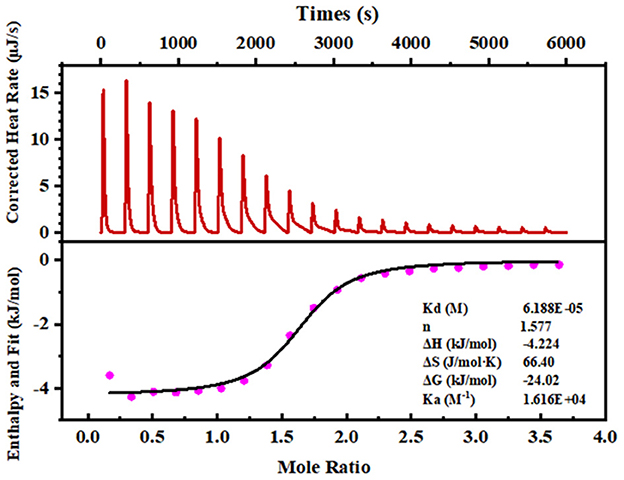
Figure 2. ITC analyses of the SPP reacting with calcium ions. The upper panel exhibits a representative calorimetric titration curve. CaCl2 (50 mM) was titrated into 2.5 mM of the peptide solution at 25°C. The lower panel shows the integrated areas corresponding to each titration, plotted as a function of the Ca2+/peptide molar ratio. The solid line represents the best curve fit obtained by using an independent binding site model.
3.3. Mechanism of SPP-Ca chelation by spectroscopy
3.3.1. Ultraviolet spectroscopy analysis
Ultraviolet-visible (UV) spectroscopy is recognized as an excellent method to analyze peptide conformational changes induced by the binding of calcium. The peptide–calcium complex formation involving organic ligands and metal ions could produce different UV spectra because of the transfer or disappearance of the original absorbance peaks or the emergence of new absorption peaks (15). Figure 3A shows that SPP has a specific absorption peak at ~200 nm, representing the characteristics of n → π* in the amide bond. After cooperating with calcium, it can be observed that the absorption band intensity of the amide bond increased significantly from 1.227 to 1.338 in the magnified UV spectra image, suggesting a hyperchromic effect. The transformation may be due to polarization changes generated by auxochrome groups (–OH, –NH2) and chromophore groups (–C = O, –COOH) (29). Hence, it could be concluded that the nitrogen atom of –NH and –NH2, the oxygen atom of –C = O and –COOH, and the phosphate group of SPP might be involved in calcium binding.
3.3.2. FTIR analysis
The FTIR analysis could be utilized to observe characteristic absorption peak variations in position, peak number and intensity of amides and carboxylates, and further to reflect the interaction between ligand and metal ion (30). With this in mind, we attempted to explore changes induced by chelation in SPP by FTIR analysis.
As presented in Figure 3B, the dominant spectral peaks are contributed by the amide I (1,700–1,600 cm−1) ascribed to C = O bonds stretching and amide II groups (1,600–1,500 cm−1) and assigned to the folding of N–H bonds and stretching of C–N bonds, respectively (31, 32). In the spectra of SPP-Ca, the band at 1,660.64 and 1,542.11 cm−1 shifted to 1,656.16 and 1,572.18 cm−1, with decreased intensity. These results indicated that the amino groups and carboxylate groups of peptides might be involved in the covalent binding reaction with calcium ions (33). Furthermore, the wave numbers at 1,450.13, 1,203.22, and 1,071.28 cm−1 shifted to 1,447.44, 1,319.69, and 1,101.95 cm−1, respectively, when bound with calcium, it might be attributed to the carbonyl oxygen contribute to the calcium chelate activity. Similar observations in the spectra were found in schizochytrium protein hydrolysates bound with calcium (16). The absorption peak of 3,346.21 cm−1 moved to the higher frequency of 3,384.49 cm−1 in the chelation procedure, indicating that hydrogen bonds were replaced with N–Ca bonds (34). In addition, the absorption band at 995.09 cm−1 corresponding to P-O-C stretching vibrations reduced to 990.22 cm−1 after chelation, which was attributed to the combination of P–O with calcium (35).
The results confirmed that the carboxyl oxygen group, amino group, and phosphoric acid group might be involved in the chelate reaction of SPP and calcium ions.
3.3.3. Conformation analysis
Circular dichroism (CD) spectra are a classical method for fast determination of peptide secondary structure. Figure 3C shows that the presence of calcium induces different conformations. One negative peak emerged at ~196 nm in the original peptide, which demonstrated random coil conformation (36). After chelating, the secondary structure of SPP changed as follows: the α-helix, β-turn, and random coil conformation contents decreased, whereas the percentage of β-sheet increased.
3.4. Thermal stability analysis of SPP-Ca
The thermostability changes between SPP and SPP-Ca chelate are presented in Figure 4. The thermal decomposition reaction of SPP underwent 76.35% weight loss throughout the process, and the Td value of SPP corresponding to the three stages was 69.05, 196.73, 292.17, 360.48, and 417.30°C, respectively. These existed endothermic peaks were probably due to the C–N bond groups in SPP (37). Whereas, for SPP-Ca, some prominent shift endothermic peaks were observed at 72.50, 219.16, 331.77, 395.83, and 446.94°C accompanied by greater weight loss.
3.5. Effect of SPP and SPP-Ca complexes on proliferation and osteogenic differentiation in MC3T3-E1
As shown in Figure 5A, the MTT assay indicated that SPP dose-dependently increase the cell viability of MC3T3-E1 cells. The greatest facilitating proliferation osteoblast activity was observed in 70 μM SPP reaching 143.52% with no obvious cytotoxic effect. Interestingly, SPP-Ca achieved stronger activity to promote the proliferation osteoblast at an equal concentration (Figure 5B). Then, we further investigated the biological effects of SPP-Ca on differentiation. The ALP activity reached 0.014, 0.015, 0.018, and 0.020 U/mg at 0.7, 7, and 70 μM SPP treatment, respectively (Figure 5C). In summary, 70 μM SPP significantly increased the osteogenic differentiation of MC3T3-E1. Therefore, the SPP-Ca group used a fixed concentration in the further experiment. Treatment with 70 μM SPP-Ca resulted in a significant increase in the ALP activity of MC3T3-E1 cells when compared with the control group.
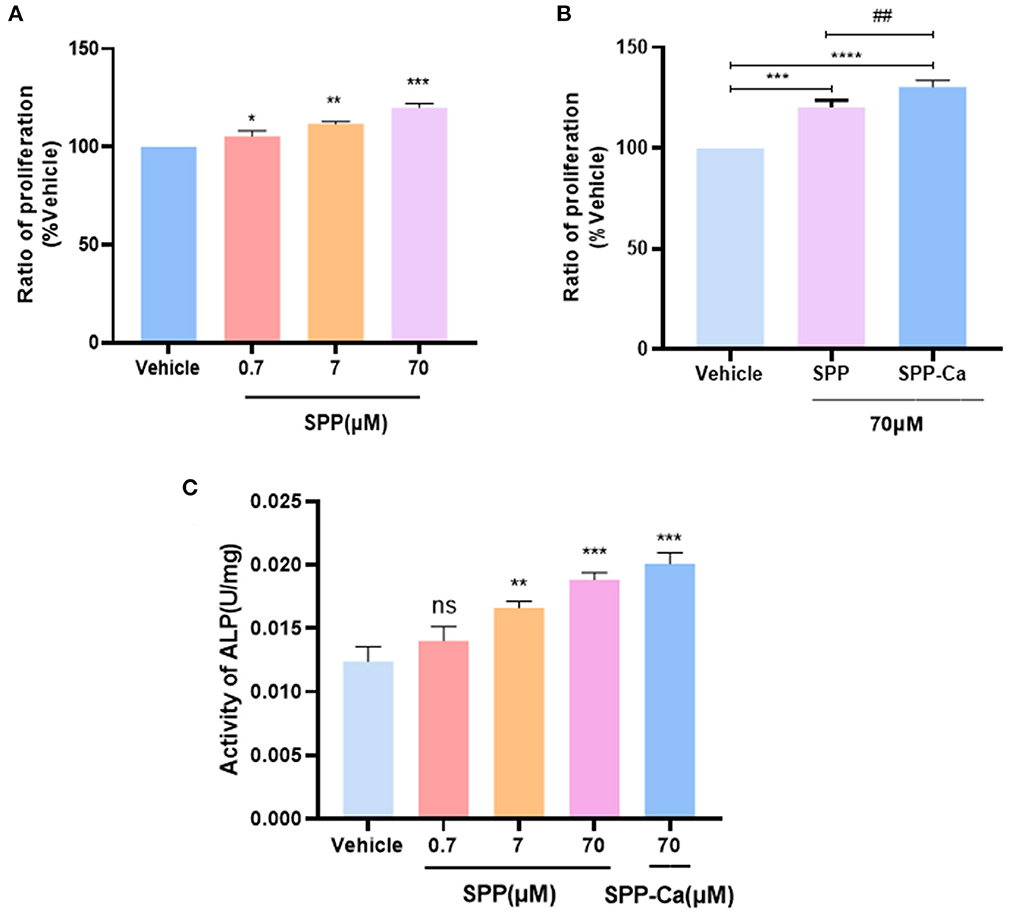
Figure 5. Proliferation of osteoblast response to SPP (A) and SPP-Ca (B). MC3T3-E1 was treated with different concentrations (0.7, 7, and 70 μM) of SPP and SPP-Ca (70 μM) for 72h. Changes in the ALP activity of MC3T3-E1 treated with different concentrations of SPP and SPP-Ca (C). n = 5. Data are presented as means ± SEMs and analyzed by one-way ANOVA followed by Tukey's multiple comparison test. *p < 0.05, **p < 0.01, and ***p < 0.001 vs. vehicle. ##Indicated significant differences, p < 0.05.
4. Discussion
The motivation for this study stemmed from a previous study on soy peptide (DEDEQIPSHPPR) stimulating osteoblast differentiation (22). Interestingly, current published literature highlighted the crucial role of phosphorylated modification in calcium binding or further absorption, offering theoretical support for the efficacy and feasibility of the modification that we performed. In this study, phosphorylated soy peptide SPP (DEDEQIPPHPPR) was innovatively synthesized and employed as materials, and the possible interaction mechanism between the SPP and calcium, stability of SPP-calcium complexes, and their promoting osteogenesis activity were comprehensively investigated.
The chemically synthesized SPP with a sequence of Asp-Glu-Asp-Glu-Gln-Ile-Pro-Ser-O--His-Pro-Pro-Arg (DEDEQIPPHPPR, 1,499.43) had a calcium-binding activity of 50.24 ± 0.20 mg/g. Generally, it is well established that the binding ability of calcium ions for peptides not only depends on the length and net charge of peptides but also on specific amino acid groups and amino acid composition (16, 38). The functional calcium-binding site in peptide bond contains an O atom of the carbonyl group and an N atom of the amino group or imino group. Liu et al. (26) suggested that wheat germ protein-derived calcium-binding peptides are rich in Glu, Asp, and Arg. Bao et al. (27) pointed out that the calcium-binding site of polypeptide might be carboxyl groups of Asp and Glu. Consequently, both the Asp and Glu residues in the SPP might contribute to chelation with calcium ions. Though phosphorylation of serine residues might provide suitable binding sites for positively charged metals. However, previous studies have shown that phosvitin phosphopeptides (39), herring egg phosphopeptides (40), and CPPs (41) were 468 ± 152.80, 90.08 ± 2.02, and 60.17 mg/g, respectively, much higher than that of the SPP isolated from soy yogurt. When calcium content reached a high level, the steric hindrance of phosphate groups might be strengthened, which could not be conducive to the chelation of SPP with amino groups and carboxyl groups, so similar calcium-binding ability cannot be achieved.
Molecule docking results showed that the SPP possesses certain calcium-binding abilities (Figure 1). Generally speaking, the combination between macromolecules and small ligands is driven by non-covalent bonds involving hydrogen bonding, van der Waals forces, hydrophobic interactions, and electrostatic attractions, which are often determined by thermodynamic parameter values (42). Through ITC measurement, it can be concluded that chelation produced an exothermic reaction of 4.224 kJ/mol. In addition, the exothermic reaction yielded by the formation of a coordination bond between Ca2+ and phosvitin exerted a negative effect on ΔH. Similar results were also observed in the binding of calcium to VHS(p)S(p) and VLPVPQK (43, 44). When ΔH < 0 and Δ s > 0, the electrostatic forces dominated. Moreover, the estimated stoichiometric ratio of SPP and calcium was 1:1, which also occurred in EDLALLEK peptide that was reported by Sun where the binding of EDLALLEK peptide to Ca2+ occurred mostly through the carboxyl oxygen atom of Glu and Asp at a ratio of 1:1 (17).
To clarify the interaction patterns between SPP and calcium, a variety of spectral analyses have been used. Both UV and FTIR spectra results indicated that there was a complex of SPP and calcium via the carboxyl oxygen group, amino group, and phosphoric acid group. Corresponding conformational changes are shown in Figure 3C. The increase in β-turn under the presence of Ca2+ greatly docks of peptide in the hydrophobic cavity and ultimately promote the transport of Ca-chelated form across the plasma membrane, indicating that the chelation reaction between SPP and calcium produced a much more ordered and compact structure (45, 46). Considering previous research, chelation does change the structure of SPP, and it will affect thermal stability. Thus, additional study is required to confirm this possibility.
Compared with SPP, the chemical bonds breaking of SPP-Ca required higher energy-generating alterations in thermal denaturation temperature. Chelation enhanced the thermal stability of SPP which gives unique advantages in the applications in the functional food field. Many studies also have reported that the application of naturally bioactive peptide calcium chelates generally positively regulating the proliferation and differentiation of osteoblasts, thus preventing bone loss (47, 48). Cell proliferation plays a vital role in the first stage of the osteoblast phenotype (49). We also found that SPP-Ca exhibited biological effects on promoting osteogenesis in MC3T3-E1. In our study, SPP-Ca with concentrations ranging from 0.7 to 70 μM was added for 72 h. MTT assay indicated that SPP-Ca showed a concentration-related stimulation in response to increase cell viability. Similar results were also obtained for the binding of calcium to bovine collagen peptides and chum salmon skin gelatin hydrolysates (50, 51). Moreover, ALP activity was the most widely recognized biochemical marker for osteoblastic activity (52). It was worth noting that the ALP activity of peptide increased slightly in the presence of calcium. Taken together, SPP-Ca treatment positively regulated osteoblast proliferation and differentiation.
5. Conclusion
In conclusion, a specific soy yogurt peptide with calcium-chelating ability was chemically synthesized with phosphorylation modification, and its binding mechanism was investigated. The results showed that SPP chelated with calcium mainly through carboxyl oxygen of Glu, Asp, and Arg at a stoichiometric ratio of 1:1 and phosphoric acid group, resulting in the formation of a stable complex. SPP-Ca chelate exhibited superior effects in promoting osteoblast proliferation and differentiation than peptide alone in vitro assays. Additionally, the peptide can be simply and directly synthesized, which is conducive to future large-scale production of SPP-Ca as a calcium supplement. However, further research is also required to identify the therapeutic effects of SPP-Ca against bone diseases.
Data availability statement
The original contributions presented in the study are included in the article/Supplementary material, further inquiries can be directed to the corresponding authors.
Author contributions
XK and ZX were responsible for conceptualizing and designing this study, validation, data curation, and writing—original draft preparation. YC, MD, and ZZ played an important role in software and formal analysis. BX and ZW participated in investigation and visualization. YC, TY, and JG contributed to resources, writing—review and editing, supervision, project administration, and funding acquisition. All authors have read and agreed to the published version of the manuscript.
Funding
This study was supported by the National Natural Science Foundation of China (31871450) and the Natural Science Foundation of Shandong (ZR2022MC217). This study was also partly supported by the Center for Mitochondria and Healthy Aging, College of Life Sciences, Yantai University.
Conflict of interest
The authors declare that the research was conducted in the absence of any commercial or financial relationships that could be construed as a potential conflict of interest.
Publisher's note
All claims expressed in this article are solely those of the authors and do not necessarily represent those of their affiliated organizations, or those of the publisher, the editors and the reviewers. Any product that may be evaluated in this article, or claim that may be made by its manufacturer, is not guaranteed or endorsed by the publisher.
Supplementary material
The Supplementary Material for this article can be found online at: https://www.frontiersin.org/articles/10.3389/fnut.2023.1129548/full#supplementary-material
Supplementary Figure 1. Identifications of SPP [DEDEQIPS(P)HPPR] by analytical reversed-phase HPLC (A) and HPLC-MS (B).
References
1. Fischer V, Haffner-Luntzer M, Amling M, Ignatius A. Calcium and vitamin D in bone fracture healing and post-traumatic bone turnover. Eur Cell Mater. (2018) 35:365–85. doi: 10.22203/eCM.v035a25
2. Guo L, Harnedy PA, Li B, Hou H, Zhang Z, Zhao X, et al. Food protein-derived chelating peptides: Biofunctional ingredients for dietary mineral bioavailability enhancement. Trends Food Sci Technol. (2014) 37:92–105. doi: 10.1016/j.tifs.2014.02.007
3. Amalraj A, Pius A. Bioavailability of calcium and its absorption inhibitors in raw and cooked green leafy vegetables commonly consumed in India–an in vitro study. Food Chem. (2015) 170:430–6. doi: 10.1016/j.foodchem.2014.08.031
4. Moller NP, Scholz-Ahrens KE, Roos N, Schrezenmeir J. Bioactive peptides and proteins from foods: indication for health effects. Eur J Nutr. (2008) 47:171–82. doi: 10.1007/s00394-008-0710-2
5. Chakrabarti S, Jahandideh F, Wu J. Food-derived bioactive peptides on inflammation and oxidative stress. Biomed Res Int. (2014) 2014:608979. doi: 10.1155/2014/608979
6. Ji H, Zhao W, Yu Z. Interaction mechanism of three egg protein derived ACE inhibitory tri-peptides and DPPC membrane using FS, FTIR, and DSC studies. Food Chem X. (2022) 15:100366. doi: 10.1016/j.fochx.2022.100366
7. Luo Y, Song Y. Mechanism of antimicrobial peptides: antimicrobial, anti-inflammatory and antibiofilm activities. Int J Mol Sci. (2021) 22:11401. doi: 10.3390/ijms222111401
8. Kheeree N, Kuptawach K, Puthong S, Sangtanoo P, Srimongkol P, Boonserm P, et al. Discovery of calcium-binding peptides derived from defatted lemon basil seeds with enhanced calcium uptake in human intestinal epithelial cells, Caco-2. Scientific Rep. (2022) 12:4565. doi: 10.1038/s41598-022-08380-0
9. Platel K, Srinivasan K. Bioavailability of micronutrients from plant foods: an update. Crit Rev Food Sci Nutr. (2016) 56:1608–19. doi: 10.1080/10408398.2013.781011
10. Zhang X, Jia Q, Li M, Liu H, Wang Q, Wu Y, et al. Isolation of a novel calcium-binding peptide from phosvitin hydrolysates and the study of its calcium chelation mechanism. Food Res Int. (2021) 141:110169. doi: 10.1016/j.foodres.2021.110169
11. Lee SH. Isolation of a Calcium-binding Peptide from Enzymatic Hydrolysates of Porcine Blood Plasma Protein. J Korean Soc Appl Biologic Chemistr. (2009) 52:290–4. doi: 10.3839/jksabc.2009.051
12. Wang L, Ding Y, Zhang X, Li Y, Wang R, Luo X, et al. Isolation of a novel calcium-binding peptide from wheat germ protein hydrolysates and the prediction for its mechanism of combination. Food Chem. (2018) 239:416–26. doi: 10.1016/j.foodchem.2017.06.090
13. Huang H, Li B, Liu Z, Wu H, Mu X, Zeng M. Purification of a novel oligophosphopeptide with high calcium binding activity from carp egg hydrolysate. Food Sci Technol Res. (2014) 20:799–807. doi: 10.3136/fstr.20.799
14. Gan J, Xiao Z, Wang K, Kong X, Du M, Wang Z, et al. Isolation, characterization, and molecular docking analyses of novel calcium-chelating peptide from soy yogurt and the study of its calcium chelation mechanism. J Sci Food Agric. (2023) 103:2939–48. doi: 10.1002/jsfa.12370
15. Sun N, Cui P, Jin Z, Wu H, Wang Y, Lin S. Contributions of molecular size, charge distribution, and specific amino acids to the iron-binding capacity of sea cucumber (Stichopus japonicus) ovum hydrolysates. Food Chem. (2017) 230:627–36. doi: 10.1016/j.foodchem.2017.03.077
16. Cai X, Lin J, Wang S. Novel peptide with specific calcium-binding capacity from schizochytrium sp. protein hydrolysates and calcium bioavailability in Caco-2 Cells. Mar Drugs. (2016) 15:3. doi: 10.3390/md15010003
17. Sun N, Jin Z, Li D, Yin H, Lin S. An exploration of the calcium-binding mode of egg white peptide, asp-his-thr-lys-glu, and in vitro calcium absorption studies of peptide-calcium complex. J Agric Food Chem. (2017) 65:9782–9. doi: 10.1021/acs.jafc.7b03705
18. Schmiedekamp A, Nanda V. Metal-activated histidine carbon donor hydrogen bonds contribute to metalloprotein folding and function. J Inorg Biochem. (2009) 103:1054–60. doi: 10.1016/j.jinorgbio.2009.04.017
19. Gan J, Kong X, Xiao ZQ, Chen YH, Du MD, Wang Y, et al. Calcium binding mechanism of soybean peptide with histidine alteration by molecular docking analysis and spectroscopic methods. Foods. (2022) 11:3290. doi: 10.3390/foods11203290
20. Zong H, Peng L, Zhang S, Lin Y, Feng F. Effects of molecular structure on the calcium-binding properties of phosphopeptides. Euro Food Res Technol. (2012) 235:811–6. doi: 10.1007/s00217-012-1809-5
21. Luo M, Xiao J, Sun S, Cui F, Liu G, Li W, et al. Deciphering calcium-binding behaviors of casein phosphopeptides by experimental approaches and molecular simulation. Food Funct. (2020) 11:5284–92. doi: 10.1039/D0FO00844C
22. Wang K, Kong X, Du M, Yu W, Wang Z, Xu B, et al. Novel Soy Peptide CBP: stimulation of osteoblast differentiation via TbetaRI-p38-MAPK-Depending RUNX2 Activation. Nutrients. (2022) 14:1940. doi: 10.3390/nu14091940
23. Xia QD, Xun Y, Lu JL, Lu YC, Yang YY, Zhou P, et al. Network pharmacology and molecular docking analyses on Lianhua Qingwen capsule indicate Akt1 is a potential target to treat and prevent COVID-19. Cell Prolif. (2020) 53:e12949. doi: 10.1111/cpr.12949
24. Sun N, Cui P, Lin S, Yu C, Tang Y, Wei Y, et al. Characterization of sea cucumber (stichopus japonicus) ovum hydrolysates: calcium chelation, solubility and absorption into intestinal epithelial cells. J Sci Food Agric. (2017) 97:4604–11. doi: 10.1002/jsfa.8330
25. Wang Z, Zhai X, Fang J, Wu H, Cheng Y, Gao Y, et al. Peptide-calcium chelate from antler (cervus elaphus) bone enhances calcium absorption in intestinal caco-2 cells and d-gal-induced aging mouse model. Nutrients. (2022) 14:3738. doi: 10.3390/nu14183738
26. Liu FR, Wang L, Wang R, Chen ZX. Calcium-binding capacity of wheat germ protein hydrolysate and characterization of Peptide-calcium complex. J Agric Food Chem. (2013) 61:7537–44. doi: 10.1021/jf401868z
27. Bao Z, Zhang P, Sun N, Lin S. Elucidating the calcium-binding site, absorption activities, and thermal stability of egg white peptide-calcium chelate. Foods. (2021) 10:2565. doi: 10.3390/foods10112565
28. Le VH, Buscaglia R, Chaires JB, Lewis EA. Modeling complex equilibria in isothermal titration calorimetry experiments: thermodynamic parameters estimation for a three-binding-site model. Anal Biochem. (2013) 434:233–41. doi: 10.1016/j.ab.2012.11.030
29. Armas A, Sonois V, Mothes E, Mazarguil H, Faller P. Zinc(II) binds to the neuroprotective peptide humanin. J Inorg Biochem. (2006) 100:1672–8. doi: 10.1016/j.jinorgbio.2006.06.002
30. Peng Z, Hou H, Zhang K, Li B. Effect of calcium-binding peptide from Pacific cod (Gadus macrocephalus) bone on calcium bioavailability in rats. Food Chemistry. (2017) 221:373–8. doi: 10.1016/j.foodchem.2016.10.078
31. Zhao L, Huang S, Cai X, Hong J, Wang S. A specific peptide with calcium chelating capacity isolated from whey protein hydrolysate. J Funct Foods. (2014) 10:46–53. doi: 10.1016/j.jff.2014.05.013
32. Nara M, Morii H, Tanokura M. Coordination to divalent cations by calcium-binding proteins studied by FTIR spectroscopy. Biochim Biophys Acta. (2013) 1828:2319–27. doi: 10.1016/j.bbamem.2012.11.025
33. Wang X, Li K, Yang XD, Wang LL, Shen RF. Complexation of Al(III) with reduced glutathione in acidic aqueous solutions. J Inorg Biochem. (2009) 103:657–65. doi: 10.1016/j.jinorgbio.2008.11.007
34. Huang SL, Zhao LN, Cai X, Wang SY, Huang YF, Hong J, et al. Purification and characterization of a glutamic acid-containing peptide with calcium-binding capacity from whey protein hydrolysate. J Dairy Res. (2015) 82:29–35. doi: 10.1017/S0022029914000715
35. Barth A, Bezlyepkina N. P-O bond destabilization accelerates phosphoenzyme hydrolysis of sarcoplasmic reticulum Ca2+ -ATPase. J Biol Chem. (2004) 279:51888–96. doi: 10.1074/jbc.M410867200
36. Cui P, Lin S, Han W, Jiang P, Zhu B, Sun N. Calcium delivery system assembled by a nanostructured peptide derived from the sea cucumber ovum. J Agric Food Chem. (2019) 67:12283–92. doi: 10.1021/acs.jafc.9b04522
37. Wang X, Zhou J, Tong PS, Mao XY. Zinc-binding capacity of yak casein hydrolysate and the zinc-releasing characteristics of casein hydrolysate-zinc complexes. J Dairy Sci. (2011) 94:2731–40. doi: 10.3168/jds.2010-3900
38. Lv Y, Liu Q, Bao X, Tang W, Yang B, Guo S. Identification and characteristics of iron-chelating peptides from soybean protein hydrolysates using IMAC-Fe3+. J Agric Food Chem. (2009) 57:4593–7. doi: 10.1021/jf9000204
39. Sun N, Wang Y, Bao Z, Cui P, Wang S, Lin S. Calcium binding to herring egg phosphopeptides: binding characteristics, conformational structure and intermolecular forces. Food Chem. (2020) 310:125867. doi: 10.1016/j.foodchem.2019.125867
40. Anastasiou E, Lorentz KO, Stein GJ, Mitchell PD. Prehistoric schistosomiasis parasite found in the Middle East. Lancet Infect Dis. (2014) 14:553–4. doi: 10.1016/S1473-3099(14)70794-7
41. Si K, Gong T, Ding S, Liu H, Shi S, Tu J, et al. Binding mechanism and bioavailability of a novel phosvitin phosphopeptide (Glu-Asp-Asp-pSer-pSer) calcium complex. Food Chem. (2023) 404:134567. doi: 10.1016/j.foodchem.2022.134567
42. Du X, Li Y, Xia YL, Ai SM, Liang J, Sang P, et al. Insights into protein-ligand interactions: mechanisms, models, and methods. Int J Mol Sci. (2016) 17:144. doi: 10.3390/ijms17020144
43. Hou T, Wang C, Ma Z, Shi W, Weiwei L, He H. Desalted duck egg white peptides: promotion of calcium uptake and structure characterization. J Agric Food Chem. (2015) 63:8170–6. doi: 10.1021/acs.jafc.5b03097
44. Liao W, Liu S, Liu X, Duan S, Xiao S, Yang Z, et al. The purification, identification and bioactivity study of a novel calcium-binding peptide from casein hydrolysate. Food Funct. (2019) 10:7724–32. doi: 10.1039/C9FO01383K
45. Baldwin Graham S, Bailey Michael F, Shehan BP, Sims I, Norton Raymond S. Tyrosine modification enhances metal-ion binding. Biochem J. (2008) 416:77–84. doi: 10.1042/BJ20081059
46. Cui P, Sun N, Jiang P, Wang D, Lin S. Optimized condition for preparing sea cucumber ovum hydrolysate-calcium complex and its structural analysis. Int J Food Sci Technol. (2017) 52:1914–22. doi: 10.1111/ijfs.13468
47. Huang W, Lao L, Deng Y, Li Z, Liao W, Duan S, et al. Preparation, characterization, and osteogenic activity mechanism of casein phosphopeptide-calcium chelate. Front Nutr. (2022) 9:960228. doi: 10.3389/fnut.2022.960228
48. He JL, Guo H, Zhang M, Wang M, Sun LP, Zhuang YL. Purification and characterization of a novel calcium-binding heptapeptide from the hydrolysate of tilapia bone with its osteogenic activity. Foods. (2022) 11:9813. doi: 10.3390/foods11030468
49. Mizukoshi M, Kaku M, Thant L, Kitami K, Arai M, Saito I, et al. In vivo cell proliferation analysis and cell-tracing reveal the global cellular dynamics of periodontal ligament cells under mechanical-loading. Sci Rep. (2021) 11:9813. doi: 10.1038/s41598-021-89156-w
50. Liu J, Zhang B, Song S, Ma M, Si S, Wang Y, et al. Bovine collagen peptides compounds promote the proliferation and differentiation of MC3T3-E1 pre-osteoblasts. PLoS One. (2014) 9:e99920. doi: 10.1371/journal.pone.0099920
51. Fu Y, Zhao X-H. In vitro responses of hFOB1.19 cells toward chum salmon (Oncorhynchus keta) skin gelatin hydrolysates in cell proliferation, cycle progression and apoptosis. Journal of Functional Foods. (2013) 5:279–88. doi: 10.1016/j.jff.2012.10.017
Keywords: phosphorylation, peptide-calcium chelate, characterization, thermal stability, osteogenic differentiation, calcium supplement
Citation: Kong X, Xiao Z, Chen Y, Du M, Zhang Z, Wang Z, Xu B, Cheng Y, Yu T and Gan J (2023) Calcium-binding properties, stability, and osteogenic ability of phosphorylated soy peptide-calcium chelate. Front. Nutr. 10:1129548. doi: 10.3389/fnut.2023.1129548
Received: 22 December 2022; Accepted: 29 March 2023;
Published: 21 April 2023.
Edited by:
Yang Li, Northeast Agricultural University, ChinaReviewed by:
Zhipeng Yu, Hainan University, ChinaShanggui Deng, Zhejiang Ocean University, China
Xixi Cai, Fuzhou University, China
Copyright © 2023 Kong, Xiao, Chen, Du, Zhang, Wang, Xu, Cheng, Yu and Gan. This is an open-access article distributed under the terms of the Creative Commons Attribution License (CC BY). The use, distribution or reproduction in other forums is permitted, provided the original author(s) and the copyright owner(s) are credited and that the original publication in this journal is cited, in accordance with accepted academic practice. No use, distribution or reproduction is permitted which does not comply with these terms.
*Correspondence: Tianying Yu, dHl5dSYjeDAwMDQwO3l0dS5lZHUuY24=; Jing Gan, Z2FuamluZyYjeDAwMDQwO3l0dS5lZHUuY24=