- 1Medical and Scientific Affairs, Reckitt/Mead Johnson Nutrition Institute, Evansville, IN, United States
- 2Medical and Scientific Affairs, Reckitt/Mead Johnson Nutrition Institute, Nijmegen, Netherlands
- 3NIZO Food Research BV, Ede, Netherlands
- 4Department of Paediatrics, University of Cambridge, Cambridge, United Kingdom
- 5MRC Epidemiology Unit, Wellcome Trust-MRC Institute of Metabolic Science, University of Cambridge, Cambridge, United Kingdom
- 6Wellcome Trust-MRC Institute of Metabolic Science, University of Cambridge, Cambridge, United Kingdom
Background: Infant gut microbiota composition is influenced by various factors early in life. Here, we investigate associations between infant gut microbiome development, infant age, breastfeeding duration, and human milk oligosaccharides (HMO) composition in breastmilk.
Methods: A total of 94 mother-infant pairs were recruited as part of the Cambridge Baby Growth and Breastfeeding Study (CBGS-BF) (Cambridge, UK). Infant stool samples (n = 337) were collected at 2 week, 6 week, 3 month, and 6 month of age. The 16S rRNA V3-V4 rRNA region was sequenced using MiSeq Illumina to determine microbiota composition and diversity. Mother’s hindmilk samples were collected at birth, 2 week, 6 week, 3 month, and 6 month postpartum. Concentrations of five neutral [2′FL, 3′FL, lacto-N-fucopentaose 1 (LNFP1), LNnT, LNT] and two acidic (3′SL, and 6′SL) HMOs were measured in all milk samples using High-Performance Anion-Exchange Chromatography with Pulsed Amperometric Detection (HPAEC-PAD). We explored the associations between infant gut microbiome parameters and age, duration of exclusive breastfeeding (EBF), and levels of individual HMOs.
Results: Bifidobacterium was the most abundant genus in infant stool at all-time points, irrespective of breastfeeding duration, with an overall mean relative abundance of 70%. The relative abundance of B. bifidum in stool from infants who were breastfed for longer than 6 months was significantly higher compared to the infant breastfed up to 3 months (p = 0.0285). Alpha-diversity (both Shannon and ASV-level Richness) of infant gut microbiota showed a biphasic change with infant age, decreasing from 2 weeks until 3 months and then increasing until 6 months of age. Bifidobacterium relative abundance was associated with higher concentrations of 2′FL and LNFP1 in breastmilk across all time-points (p = 0.049 and 0.017, respectively), with trends toward a higher abundance of B. longum species. No significant association with Bifidobacterium was found for breastmilk LNnT, 3′SL, and 6′SL levels.
Conclusion: Our study is in line with previous data demonstrating that EBF duration in the first months of life impacts infant gut microbiota composition. The observed links between specific HMOs in breastmilk and bacteria in infant stool provide evidence of how mother’s milk affects infant microbiome development.
Key message and impact
Our study demonstrates that exclusive breastfeeding during the first months of life significantly impacts infant gut microbiota composition. In a cohort of healthy mother-infant pairs in the UK we observed a trend of decreased microbial diversity in infant fecal microbiota during the first 3 months of age. It was followed by an increased diversity at 3 months of age, which coincides with prominent changes in human milk oligosaccharides (HMO) concentrations in corresponding breastmilk samples from respective mothers during the first 3 months. In our study, select bifidobacteria levels in infant stool were higher in infants who were exclusively breastfed longer (over 6 months) compared to infants breastfed for a shorter period of time (less than 3 months). These associations between levels of specific HMOs in breastmilk and bacteria in infant stool provide important insights into how mother’s milk affects infant microbiome development.
Introduction
Gut microbiota plays a critical role in influencing infant growth, body composition, and later life health via modulating gastrointestinal, nervous, and immune systems, as well as energy metabolism and fat deposition (1). Infant gut microbiota develops and evolves in the first 1,000 days of life and would attain adult-like composition by the age of 3 years (2). In addition to Bifidobacterium spp., anaerobic bacteria such as Bacteroides spp. and Clostridium spp. have also been identified as colonizers of infant gut during the first 6 months of life and are known to have various effects on infant development and maturation (3).
Infant feeding has been established as one of the major factors shaping he development of gut microbiota (4). Gut microbiome and fecal metabolites of breastfed infants change during lactation and are influenced by breast milk components (5); breastmilk promotes the selective proliferation of a characteristic microbiota. Bacterial families in exclusively breastfed infants have a higher occupancy of Bifidobacteriaceae and a lower presence of Enterococcaceae and Enterobacteriaceae than those in formula-fed infants (6). However, bacterial genera such as Bifidobacterium spp. fluctuate dramatically in exclusively breastfed infants (7, 8), which may be partly explained by mother’s variation in breastmilk (BM) composition.
Human milk oligosaccharides (HMOs) support bifidobacteria-predominant gut microbiota in breastfed infants (9). HMOs represent the third most abundant solid human milk component after lipid and lactose, even higher than protein (10). With a concentration of 5–10 g/L, HMOs make BM distinct from the milk of other farm animals, including cows, whose oligosaccharide concentrations are 100–1,000-fold lower (10). HMOs composition in BM depends on several factors, including the maternal secretor (FUT2) genotype. Fucosylated lactoses such as 2′-fucosyllactose (2′FL), sialylated lactoses such as 3′-sialyllactose (3′SL) and 6′SL, and oligosaccharides with lacto-N-biose structure such as lacto-N-fucopentaose (LNFP) 1 are some of the most abundant HMOs in BM.
HMOs act as selective prebiotics for the gut microbiota (11), and associations between HMOs and infant gut microbiome development have been demonstrated. Bifidobacterium longum subsp. infantis (B. infantis) is the most well-known species that is particularly well-suited to colonize the infant gut (12), attributed to its unique ability to consume a large variety of HMOs. As evidenced in a clinical study (13), the administration of B. infantis may lead to successful colonization in the gut, whereby its highly selective and acidic fermentation of HMOs increases the production of lactate and acetate and subsequently reduces intestinal pH as well as counteracts gut dysbiosis [reviewed previously (14)]. This potentially includes an important role in the development and maturation of the immune system. Although HMOs are also consumed by other bacteria (e.g., Bacteroidaceae), in general, Bifidobacteriaceae is the only bacterial family that can substantially convert HMOs to acidic end products that affect stool pH (15). Previously, Bai et al. reported changes in HMOs in the milk of Chinese mothers with different secretor statuses during 6 months of lactation. Those researchers observed the correlations between fucosylated HMOs (e.g., 2′FL) and Bifidobacterium spp. in infant stool during early lactation (16). The impact of milk composition on infant stool microbiome was also suggested by Pace and colleagues, who focused on HMO analyses in different geographical locations (17). Finally, Borewicz and colleagues investigated the associations between concentrations of selected HMOs and infant stool microbiota in 24 mother-infant pairs at 2, 6, and 12 weeks of age. Microbiota composition was associated with mode of delivery and breastmilk LNFP1, 3′SL, and Lacto-N-hexaose (LNH) at different time points (18). Despite accumulating knowledge on the effect of HMOs on the infant gut microbiome [summarized elegantly in a recent review by Masi and Stewart (19)], clinical and cohort studies confirming associations between HMOs levels and infant gut microbiota composition in infant-mother cohorts are still scarce.
In the present analysis, we focused on investigating the associations between early gut microbiota development and composition with infant age, exclusive breastfeeding (EBF) duration as well as individual HMOs by analyzing breastmilk and stool samples during the first 6 months of lactation from 94 mother-infant pairs of the Cambridge Baby Growth and Breastfeeding Study (CBGS-BF) (Cambridge, UK).
Materials and methods
Study design and participants
This study is part of the CBGS-BF, a UK-based prospective observational infant cohort (20). The CBGS-BF is an extension of the original Cambridge Baby Growth Study (21), aiming to investigate determinants of infant growth and body composition. All infants recruited to this cohort were singletons and vaginally born at term from healthy mothers with normal pre-pregnancy BMI and without any significant comorbidities (n = 94) (20). All infants received EBF for at least 6 weeks. Infant stool samples were collected at 2 week, 6 week, 3 month, and 6 month (Figure 1). Mother milk samples were collected at birth, 2 weeks, 6 weeks, 3 month, and 6 month postpartum. The study was approved by the Cambridge Local Research Ethics Committee, and all mothers gave written informed consent.
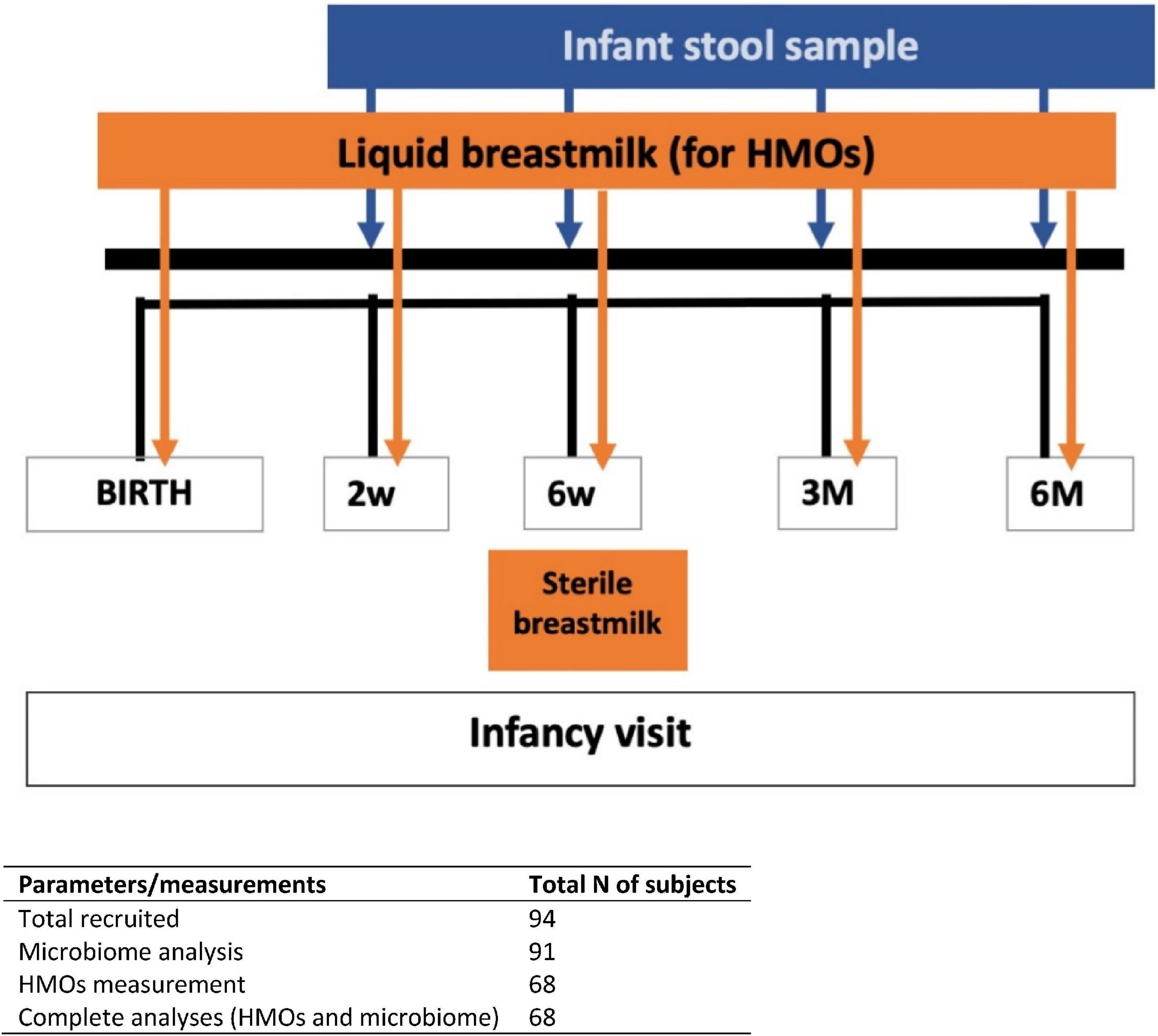
Figure 1. Timeline for the entire study duration. Fecal and breast milk samples were collected based on specified days (infant’s age) as stated for each visit.
Feeding modules
For statistical analysis, infants were categorized into three feeding groups based on the duration of EBF:
1. Less than 3 months (< 3 month EBF), these infants received EBF for at least 6 weeks.
2. 3–6 months (3–6 month EBF).
3. More than 6 months (> 6 month EBF).
Human milk oligosaccharides analysis
Levels of selected HMOs in maternal breast milk samples were quantitatively determined using high-performance anion-exchange chromatography as reported by Durham et al. (22). The analyzed HMOs included: 2′FL, 3′FL, LNFP1, LNnT, LNT, 3′SL, and 6′SL. For generalized linear mixed models (gLMM) “low” or “high” HMO levels were defined as lower or higher than median levels at each time point).
Infant gut microbiome analysis
Detailed methodology describing DNA extraction from stool samples is provided in the Supplementary material. Briefly, 250 mg of fecal sample in S.T.A. R. Buffer (Roche, Indianapolis, IN, USA) were lysed with glass beads. After centrifugation, the supernatant was kept on ice. Purification of DNA was performed on the automated Maxwell instrument (Promega, Madison, WI, USA) by applying the Maxwell 16 Tissue LEV Total RNA Purification Kit (Promega) following the manufacturer’s protocol.
PCR amplification of 16S rRNA gene in infant stool DNA, library preparation and sequencing
After DNA extraction, PCR amplification and DNA sequencing of the V3-V4 region of the bacterial 16S rRNA gene was performed in an Illumina MiSeq instrument (Illumina, San Diego, CA, USA). Detailed methodology describing PCR amplification and sequencing is provided in the Supplementary material.
Data analysis
A detailed description of analytical methods is provided in the Supplementary material. Shannon index and ASV (amplicon sequence variant)-level richness were calculated using the vegan v2.5-7 R package (23). Faith’s phylogenetic diversity was calculated using the picante v1.8.2 R package. Weighted Unifrac distances were calculated using the phyloseq UniFrac function. PERMANOVA analyses were performed on the fecal microbiome weighted Unifrac distance matrixes using the adonis function of the vegan R package with 10,000 permutations to estimate the proportion of beta-diversity explained by time point, feeding module, or their interaction, For exclusively breastfed infants only, the effect of individual HMOs—2′FL, 3′FL, LNT, LNnT, LNFP1, 3′SL, and 6′SL—on microbial composition were assessed using PERMANOVA models of weighted UNIFRAC distances at each time point.
Beta-diversity was visualized using Principal Coordinate Analysis (PCoA) performed with the ape v5.5 R package (24) on weighted Unifrac distances.
Generalized linear mixed models (gLMM) were fitted using glmmTMB v1.7.22. Multiple comparison adjustment was performed using the False Discovery Rate (FDR) controlling procedure of Benjamini-Hochberg to limit the FDR to 5% (25). The DHARMa, performance and parameters R packages were used for model diagnostics. All statistical modeling and visualizations were performed in R v4.1.1 using the tidyverse v1.3.1 package. The metacoder v0.3.5 R package was used to create heat tree visualizations. The effect of the individual infant was modeled as a random effect, and an offset term was added to account for differences in library size (i.e., sequencing depth) between the samples.
Results
Data was collected from 91 infants and 68 mothers. Infant sex (35 females, 56 males), parity (1 for 35 infants, 2 for 47 infants, 3 for 7 infants, and 4 for 2 infants), and FUT2 secretory status (56 producers, 12 non- or low producers) were included in the analysis as covariates.
Infants included in the analysis were classified based on EBF duration: < 3 months EBF (n = 13), 3–6 months EBF (n = 51), and > 6 months EBF (n = 27; see Feeding Modules above).
Infant stool microbiome composition and diversity over time and per feeding module
From 16S rRNA gene sequencing, a total of 1,037 ASVs were detected in the infant stool samples. The fifteen most abundant taxa per feeding module and infant age are shown at genus level (Supplementary Figure 1A) and species level (Supplementary Figure 1B). Irrespective of breastfeeding duration, the genus Bifidobacterium was the most abundant taxon at 2 weeks of infant age (∼50% of infant gut composition) and increased further to ∼75% of total microbiome composition at 6 months of age (Figure 2; p < 0.001). Bifidobacterium bifidum abundance was significantly associated with EBF duration (higher in infants EBF > 6 month than EBF < 3 month; p = 0.0285). In addition, the abundance of Enterococcus durans/faecalis/faecium was lower in both longer EBF groups compared to infants EBF < 3 month (Supplementary Figure 1B). Changes in Bifidobacterium relative abundance from 2 weeks to 6 months are shown in Figure 3, with levels increasing from 2 weeks to 3 month for all three EBF groups. Thereafter, the levels of Bifidobacterium decreased between 3 and 6 month among infants who remained EBF (EBF 3–6 and > 6 month).
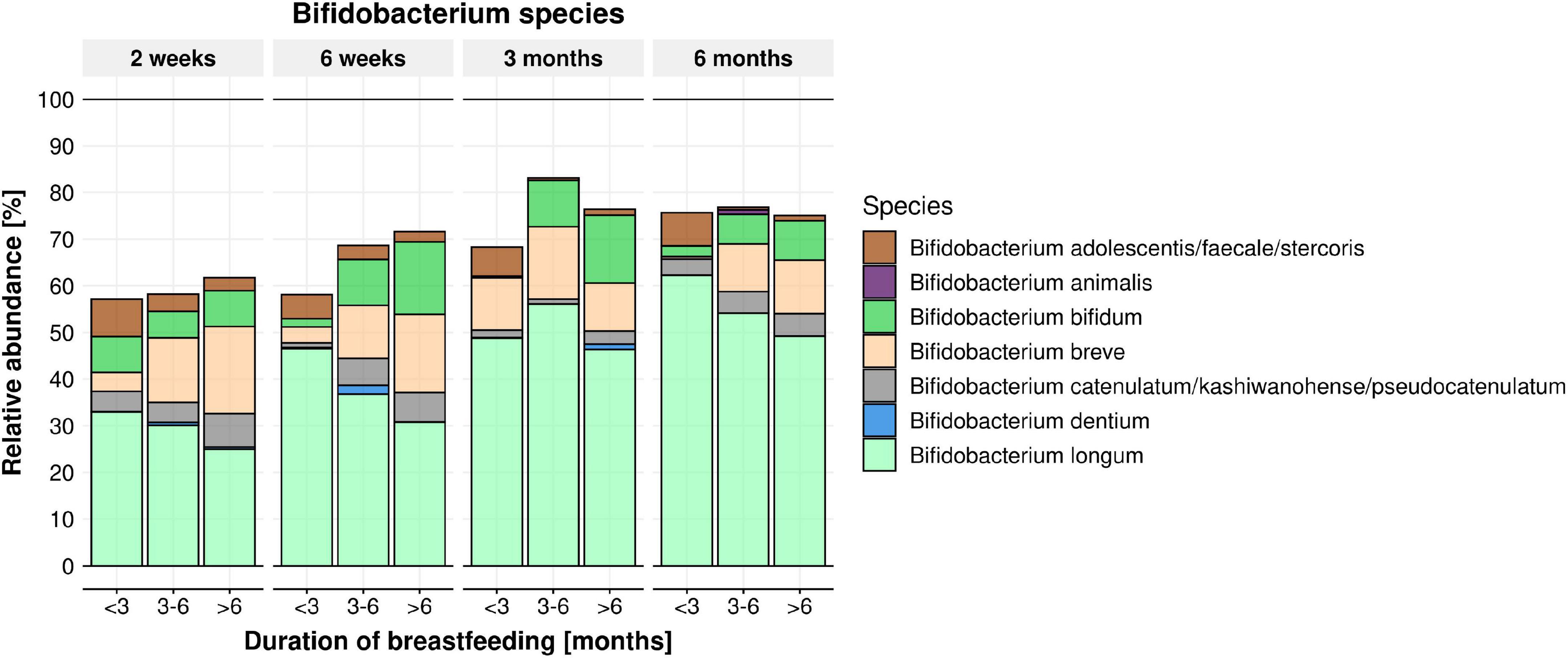
Figure 2. Mean relative abundance of Bifidobacterium species per feeding module (breastfeeding duration < 3, 3–6, > 6 months) across time (infant age 2 weeks, 6 weeks, 3 months, 6 months). B. bifidum abundance was significantly impacted by feeding modules (higher in infants breastfed > 6 month. compared to infants breastfed < 3 month; within the 3–6 months’ time frame) (p = 0.0285).
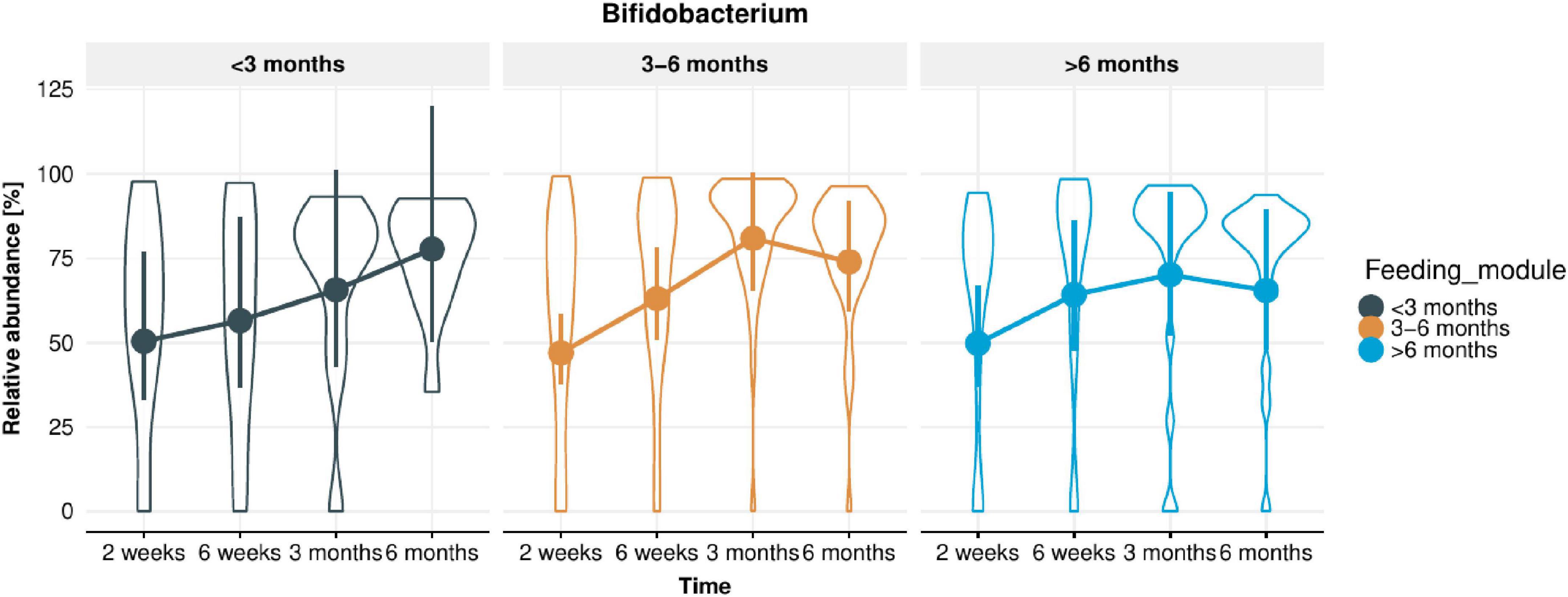
Figure 3. Change in Bifidobacterium relative abundance in infant stool microbiota from 2 weeks to 6 months of infant age in each of the feeding modules (breastfeeding duration < 3, 3–6, > 6 months). Overall, Bifidobacterium relative abundance increased significantly over time (p < 0.001).
The heat tree shown in Figure 4 provides a visual representation of the global changes in the infant fecal microbiome from age 2 weeks to 6 months. B. adolescentis/faecale/stercosis and B. catenulatum/kashiwanohense/pseudocatenulatum were higher at 6 months while other bifidobacteria (e.g., B. bifidum, B. longum, and B. breve) were lower.
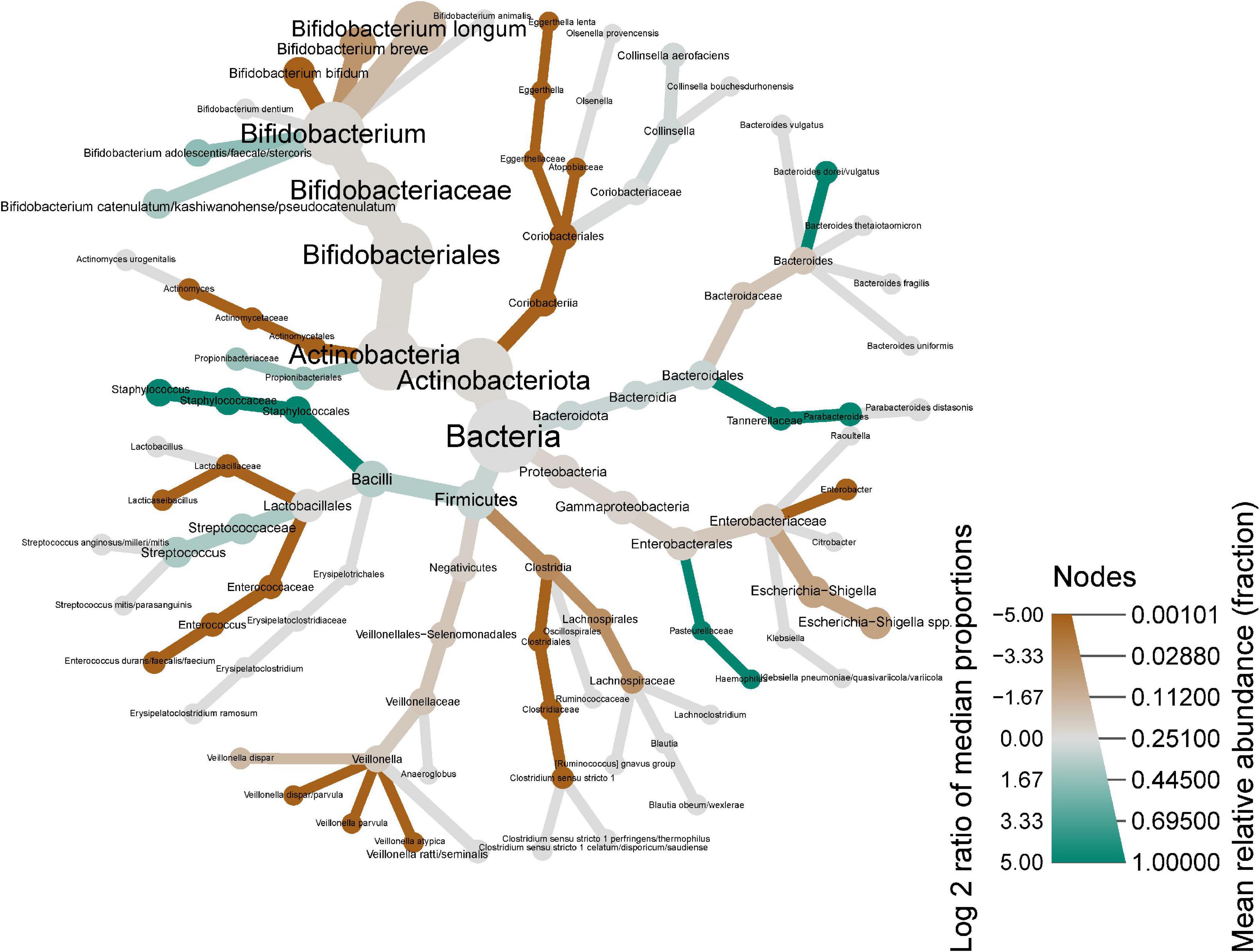
Figure 4. Heat tree showing shifts in infant stool microbiome composition between 2 weeks and 6 months of infant age. Nodes represent each taxonomic rank from kingdom (bacteria, center) species (tips of each branch). Node color indicates the log2 of the median proportions of each taxa at the two time points (i.e., green shows an increase, red shows a decrease), while the node size represents the respective taxon’s mean relative abundance.
The impact of EBF duration on stool microbiome diversity was also evaluated. A longitudinal trend of decreased alpha-diversity (both Shannon and ASV-level Richness) was observed from 2 weeks until 3 months of infant age (except for Richness in infants EBF < 3 month), followed by an increase at 6 months of age (Figure 5A). Regardless of EBF duration, Shannon index decreased by 0.13 units from 2 to 6 weeks (p = 0.0068), and a further 0.14 units from 6 weeks to 3 months (p = 0.0022), but then increased by 0.15 units from 3 to 6 months (p = 0.00026). Richness decreased by 2.97 ASVs from 2 to 6 weeks (p = 0.0022), was unchanged from 6 weeks to 3 months (p = 0.50), then increased by 8.48 ASVs from 3 to 6 months (p = 2.7 × 10–8). Moreover, Richness was significantly lower in longer EBF groups. This difference was significant around 12 ASVs in the 6 weeks to 3 months period (p = 0.01) and around 9 ASVs in the 3–6 months period (p = 0.058; Figure 5B). At 6 weeks, the estimated number of ASVs for infants in the < 3 month. breastfed group was 61 ASVs, while the estimate for the 3–6 months breastfed groups was 49 ASVs (lower for the > 6 month group). At 6 months, the estimated number of ASVs for infants in the < 3 month. breastfed group was around 70 ASVs, while the estimate for the longer breastfed groups was around 61 ASVs.
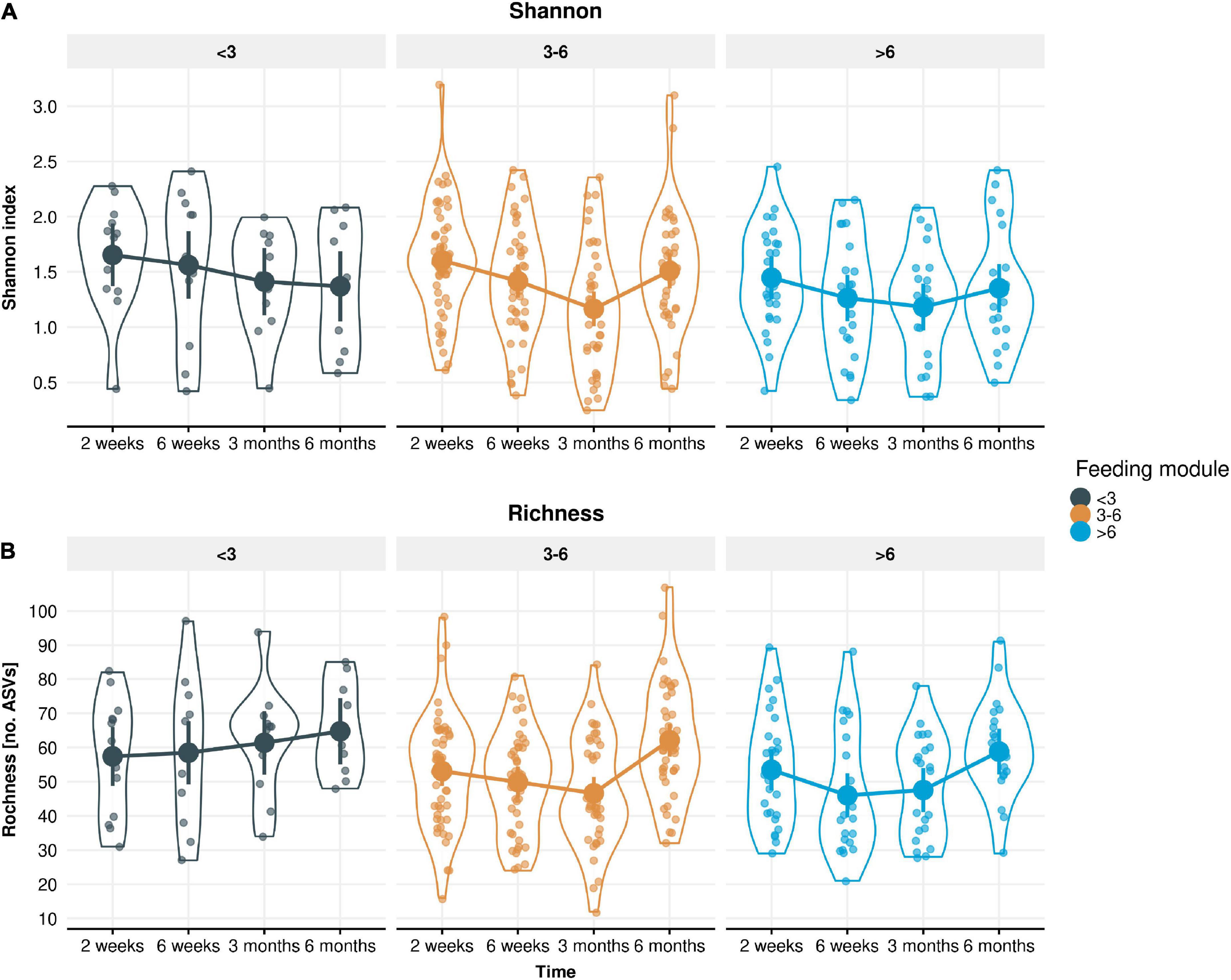
Figure 5. Measured alpha-diversity values displayed by (A) Shannon index and (B) Richness. LMM group estimates with 95% confidence intervals across the four time points (infant age 2 weeks, 6 weeks, 3 months, 6 months) stratified by the three feeding modules (breastfeeding duration < 3, 3–6, > 6 months). Richness was significantly lower in longer breastfed infant groups (p = 0.01 in the 6 weeks to 3 months period and p = 0.058 in the 3–6 months period).
Beta diversity analysis of the fecal microbiome showed that most of the variance in the weighted Unifrac (quantitative) distances was influenced by inter-individual differences (infants) (R2 = 0.556, p < 0.001) and time point (R2 = 0.06, p < 0.001). Figure 6 shows the Principal Component Analysis plot illustrating the intra-individual variance (after removing inter-individual variance) and demonstrates the shift in microbiota composition over time.
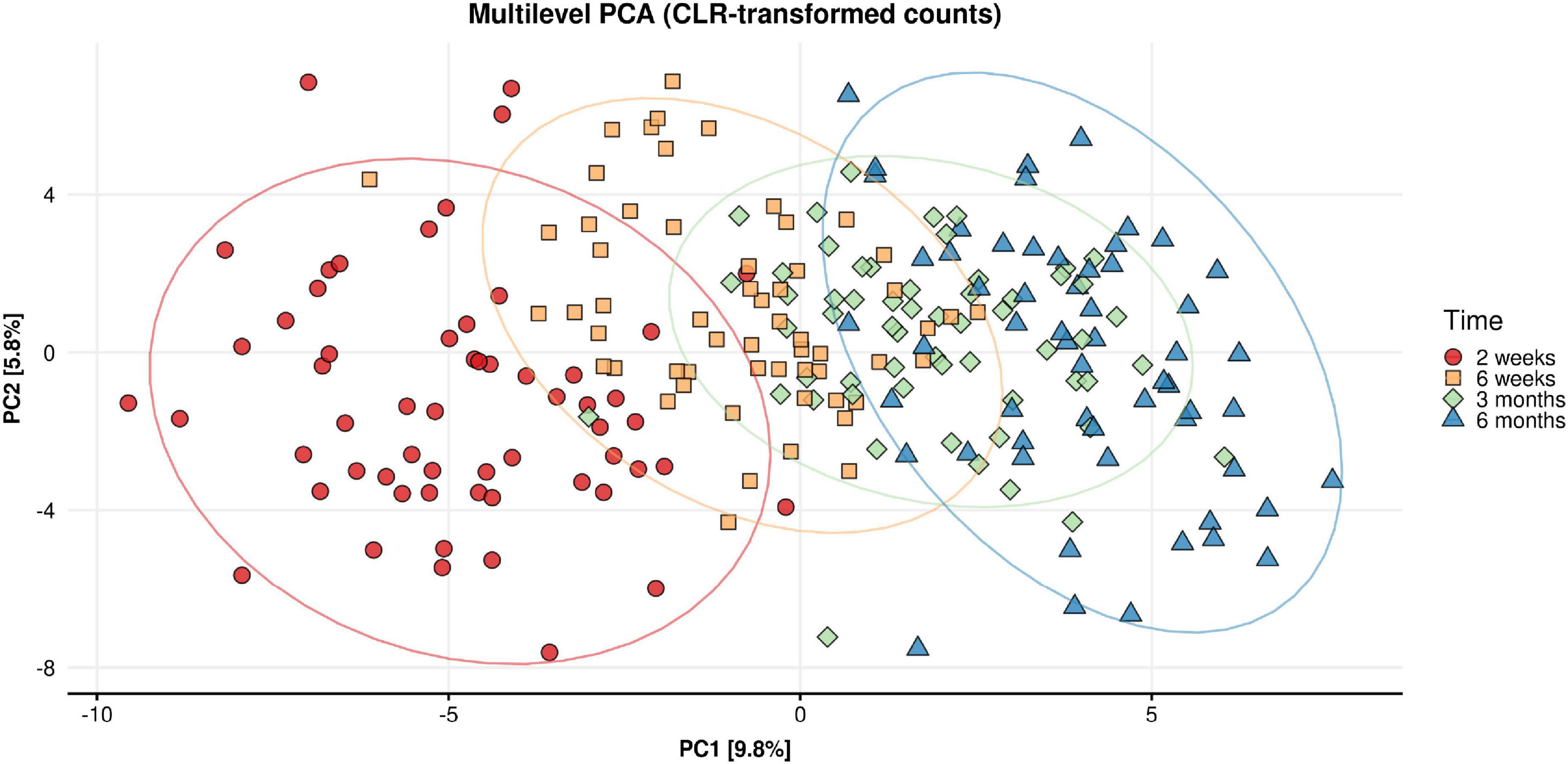
Figure 6. Multilevel principal component analysis plot of CLR-transformed microbial counts for infant stool microbiota composition across time (infant age 2 week, 6 weeks, 3 months, 6 months). Only intra-individual variance is decomposed and shown. Inter-individual variance (i.e., between infant differences) is removed.
Associations between HMOs levels in breastmilk and abundance of Bifidobacterium in infant gut
Associations between levels of individual HMOs and Bifidobacterium spp. relative abundance in infant gut microbiota were examined using gLMMs. Only samples collected while infants were exclusively breastfed were included in these models (n = 91 at 2 weeks, n = 79 at 6 weeks, n = 63 at 3 months, and n = 21 at 6 months). Over time (across all four time points), higher levels of 2′FL and LNFP1 in breastmilk (p = 0.049 and 0.017, respectively) were associated with higher Bifidobacterium relative abundance in infant stool (Figure 7), while a higher LNT level was associated with a lower relative abundance of Bifidobacterium (p = 0.029, Supplementary Figure 4A). LNnT, 3SL, and 6SL levels were not associated with Bifidobacterium relative abundance (Supplementary Figures 4B–D). Regarding Bifidobacterium species, we observed a trend toward higher B. longum levels with higher LNFP1 levels (p = 0.078). Associations between levels of individual HMOs and relative abundance of other bacterial groups described to metabolize HMOs were either not significant (Bacteroides; Supplementary Figure 5) or only present in ≤ 2 infant samples and therefore no statistical modeling was possible (Akkermansia, Roseburia, or Eubacterium).
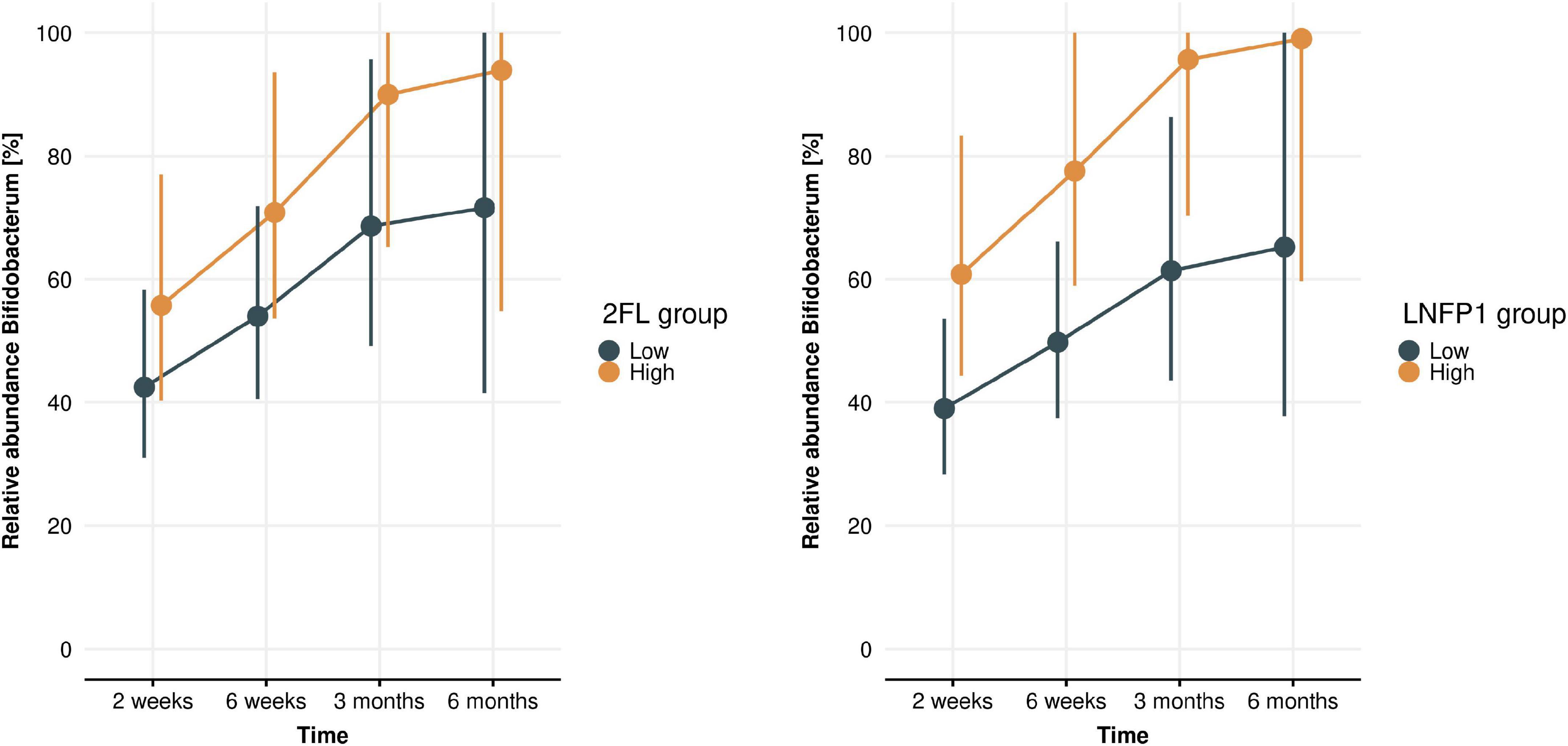
Figure 7. Bifidobacterium levels across time (infant age 2 weeks, 6 weeks, 3 months, 6 months) in high and low 2′FL (left) and LNFP1 (right) groups. Plots show gLMM estimates (95% confidence intervals) of Bifidobacterium relative abundance in infants with either low or high HMO levels (where “low” or “high” is defined as lower or higher than median levels at each time point). 2FL (p = 0.049) and LNFP1 (p = 0.017) were significantly associated with a higher Bifidobacterium relative abundance.
Discussion
Breast milk is ideally the nutritional source for infants since it contains various bioactive components, such as HMOs and antibacterial properties. However, relationships between human milk components, infant gut microbiome, and fecal metabolites throughout lactation remain largely unclear.
This analysis is part of the CBGS-BF recently described by Olga et al. (20). Durham and colleagues reported varying patterns of individual HMO concentrations longitudinally in the same cohort and concluded that the most prominent temporal changes occurred during the first 3 months (22). The present study aimed to analyze infant gut microbiota composition during the first 6 months of life and associations with EBF duration overall, as well as levels of HMOs in breastmilk that could potentially drive changes in microbial communities.
Our study has demonstrated that the duration of EBF significantly affected the infant gut microbiota during the first 6 months of life. We chose to visualize changes in microbiota data for each EBF feeding group separately, even at 2 and 6 weeks (before discontinuation of breastfeeding). This reflects the longitudinal data analysis that was performed. Another reason to separate the EBF feeding groups at 2 and 6 weeks is because infant characteristics (i.e., slower weight gain associated with reduced milk intake were seen in the group who stopped EBF earliest) in the EBF feeding groups already differ before discontinuation of EBF [previously published studies with the same cohort (20, 26)]. There were, however, no significant differences in alpha diversity or Bifidobacterium species between feeding groups at 2 weeks (data not shown).”
It has been reported that the gut microbiome of formula-fed infants is more diverse but less stable compared to breast-fed infants (27). In that study, alpha diversity was lower in breast-fed infants compared to formula-fed infants during the first 3 months after birth but increased significantly at 6 months of age. It has been suggested that higher bacterial diversity in formula-fed infants leads to a shift toward an adult-like microbiome at earlier ages (28). Lower diversity in infants receiving human milk is likely due to the clear dominance of Bifidobacterium, which preferentially utilize HMOs as substrates for growth. Komatsu and colleagues indicated that the gut microbiome of breastfed infants fluctuated after month 3 postpartum, even though milk components did not change (5). This likely indicates more efficient metabolism of breast milk components by bifidobacteria. Accordingly, it has been reported that after 3 months of age, the functional maturation of the infant GI tract progresses rapidly, and fecal properties change dramatically (29). In our study, Bifidobacterium was the most abundant genus in infant stool at all-time points and irrespective of EBF duration, with an overall mean relative abundance of 70%. Our data shows that levels of Bifidobacterium increased until 3 months of age for all infants but decreased during the period from 3 to 6 months of age in infants who were exclusively breastfed beyond 3 months. On the species level, infants EBF for > 6 months showed a higher relative abundance of B. bifidum compared to infants EBF for < 3 months. Analyzing the same cohort, Durham and colleagues reported varying longitudinal patterns of individual HMO concentrations and concluded that the most prominent temporal changes occur during the first 3 months (22). In line with the fluctuation of the dominant bifidobacteria, we here describe a biphasic longitudinal trend of initially decreasing alpha-diversity (both Shannon and ASV-level Richness) from 2 weeks until 3 months of infant age followed by an increase until 6 months of age. In addition, richness was lower in infants who were longer EBF.
In our study, levels of selected HMOs in breastmilk were significantly correlated with higher relative abundance of bifidobacteria in infant stool. In particular, levels of 2′FL and LNFP1 were positively correlated with higher bifidobacteria levels, from 2 weeks to 6 months, indicating that bioactive milk components, such as HMOs, contribute to shaping infant gut microbiota composition in exclusively breastfed infants. Another study recently reported positive correlations between HMOs (3′FL and 3′SL) and B. breve in infant stool (5). Here, we observed a trend toward higher abundance of B. longum in infant stool associated with higher levels of LNFP1 in breastmilk. We could not discriminate between subspecies B. longum subsp. longum and B. longum subsp. infantis, but the trend between LNFP1 levels and B. longum can likely be attributed most to B. longum subsp. infantis. B. longum subsp. infantis is equipped with fucosidases that are required to assimilate fucosylated HMOs, while B. longum subsp. longum generally is not (30). However, no associations were observed between Bifidobacterium (spp.) and LNnT, 3′SL, or 6′SL levels. The sialidases, required to utilize 3′SL and 6′SL (31), are also commonly detected in B. longum subsp. infantis, but not in B. longum subsp. longum (30, 32). Nonetheless, specific Bifidobacterium species that thrive on specific HMOs would particularly benefit from higher levels of these HMOs in breastmilk, resulting in higher relative abundance. HMO metabolism by Bifidobacterium species has been studied mechanistically, in particular using in vitro bacterial cultures allowing in depth analyses of specific bacterial strains or consortia and bacterial genes responsible for HMO utilization patterns (33, 34). However, evidence from clinical and cohort studies are limited so far to confirm associations between levels of specific HMOs in breastmilk and abundance of bacterial species in infant gut microbiota. To our knowledge, our present study is one of only few to explore natural variation in HMO levels and its impact on infant gut microbiota composition (35).
Our results provide direct evidence that the infant gut microbiome is dynamically associated with levels of bioactive milk components during a certain lactation period. Since gut microbiota in this sensitive time window in early life plays an important role in the infant’s physiology and development of the immune system (36), these insights are important when aiming to support the best start in life and ultimately optimal health outcomes at later ages.
Study limitations
In this study, the microbiome profiling methodology (16S rRNA gene sequencing) did not allow to distinguish subspecies of B. longum, therefore we cannot determine the impact of select bioactives on B. infantis spp. Next, only a select panel of seven HMOs was analyzed due to lack of reliable standards at time of analysis. On top of the current analysis, it would be of interest to analyze the effects of actual HMO intake instead of breastmilk levels on infant gut microbiota composition. Breastmilk intake volume was only assessed at limited timepoints in the current study. Therefore, analysis on HMO intake would have been underpowered and was not performed.
Conclusion
Our study demonstrates that EBF duration during the first months of life impacts infant gut microbiota composition. Bifidobacterium was the most abundant genus in infant stool at all-time points and irrespective of breastfeeding duration, with an overall mean relative abundance of 70%. B. bifidum levels were higher in infants who were breastfed longer (over 6 months) compared to infants breastfed for a shorter period of time (less than 3 months). In addition, richness was significantly lower in the longer breastfed infant groups across time points. Links between specific HMOs in breastmilk and bacteria in infant stool as demonstrated in this study provide evidence for how components present in mother’s milk affect infant microbiome development.
Data availability statement
The raw sequencing data presented in this study are deposited in the European Nucleotide Archive accession number PRJEB50418.
Ethics statement
The study was conducted according to the guidelines of the Declaration of Helsinki and approved by the National Research Ethics Service Cambridgeshire 2 Research Ethics Committee (IRAS No 67546, REC No 11/EE/0068).
Author contributions
GG, JD, MC, LO, KO, and DD conceived and designed the study. AP and GK conducted the microbiome analysis. MC drafted the manuscript. All authors interpreted the data, contributed to manuscripts drafts, reviewed, and revised the manuscript for critical intellectual content, and approved the final version to be published.
Funding
This research was supported by the National Institute for Health Research/Wellcome Trust Clinical Research Facility at Cambridge University Hospitals NHS Foundation Trust and the NIHR Cambridge Comprehensive Biomedical Research Centre. KO was supported by the Medical Research Council (Unit programme: MC_UU_00006/2).
Acknowledgments
We thank study site staff for their cooperation. The participation of parents and infants in this study is greatly appreciated. We would like to thank all NIZO laboratory personnel, Dr. Sierra Durham (UC Davis) and Prof. Daniela Barile (UC Davis) for their contributions to the HMO analysis described in this manuscript. We acknowledge the CBGS research nurses Suzanne Smith, Anne-Marie Wardell, and Karen Forbes, all the families who contributed to the study, the staff at the National Institute for Health Research (NIHR) Cambridge/Wellcome Trust Clinical Research Facility, the NIHR Cambridge Comprehensive Biomedical Research Centre, the midwives at the Rosie Maternity Hospital, Cambridge, UK, and Karen Whitehead and Dianne Wingate at the Department of Paediatrics, University of Cambridge, for laboratory support.
Conflict of interest
The authors declare that this study received funding from Reckitt/Mead Johnson Nutrition. The CBGS BF was funded by a research project award by Reckitt/Mead Johnson Nutrition. The funder advised in the study design, data collection, data discussions and preparation of the manuscript. At the time of study execution MC, JD, and GG were employed by Reckitt/Mead Johnson Nutrition.
AP and GK were employed by the NIZO Food Research BV.
The remaining authors declare that the research was conducted in the absence of any commercial or financial relationships that could be construed as a potential conflict of interest.
Publisher’s note
All claims expressed in this article are solely those of the authors and do not necessarily represent those of their affiliated organizations, or those of the publisher, the editors and the reviewers. Any product that may be evaluated in this article, or claim that may be made by its manufacturer, is not guaranteed or endorsed by the publisher.
Supplementary material
The Supplementary Material for this article can be found online at: https://www.frontiersin.org/articles/10.3389/fnut.2023.1003032/full#supplementary-material
References
1. Borre YE, O’Keeffe GW, Clarke G, Stanton C, Dinan TG, Cryan JF. Microbiota and neurodevelopmental windows: implications for brain disorders. Trends Mol Med. (2014) 20:509–18. doi: 10.1016/j.molmed.2014.05.002
2. Derrien M, Alvarez AS, de Vos WM. The gut microbiota in the first decade of life. Trends Microbiol. (2019) 27:997–1010.
3. Robertson RC, Manges AR, Finlay BB, Prendergast AJ. The human microbiome and child growth-first 1000 days and beyond. Trends Microbiol. (2019) 27:131–47.
4. Song SJ, Dominguez-Bello MG, Knight R. How delivery mode and feeding can shape the bacterial community in the infant gut. Cmaj. (2013) 185:373–4.
5. Komatsu Y, Kumakura D, Seto N, Izumi H, Takeda Y, Ohnishi Y, et al. Dynamic associations of milk components with the infant gut microbiome and fecal metabolites in a mother–infant model by microbiome, NMR metabolomic, and time-series clustering analyses. Front Nutr. (2022) 8:813690. doi: 10.3389/fnut.2021.813690
6. Lee SA, Lim JY, Kim B-S, Cho SJ, Kim NY, Kim OB, et al. Comparison of the gut microbiota profile in breast-fed and formula-fed Korean infants using pyrosequencing. Nutr Res Pract. (2015) 9:242–8. doi: 10.4162/nrp.2015.9.3.242
7. Nagpal R, Tsuji H, Takahashi T, Nomoto K, Kawashima K, Nagata S, et al. Ontogenesis of the gut microbiota composition in healthy, full-term, vaginally born and breast-fed infants over the first 3 years of life: a quantitative bird’s-eye view. Front Microbiol. (2017) 8:1388. doi: 10.3389/fmicb.2017.01388
8. Simeoni U, Berger B, Junick J, Blaut M, Pecquet S, Rezzonico E, et al. Gut microbiota analysis reveals a marked shift to bifidobacteria by a starter infant formula containing a synbiotic of bovine milk-derived oligosaccharides and Bifidobacterium animalis subsp. lactis CNCM I-3446. Environ Microbiol. (2016) 18:2185–95. doi: 10.1111/1462-2920.13144
9. Le Doare K, Holder B, Bassett A, Pannaraj PS. Mother’s milk: a purposeful contribution to the development of the infant microbiota and immunity. Front Immunol. (2018) 9:361. doi: 10.3389/fimmu.2018.00361
10. Bode L. Human milk oligosaccharides: every baby needs a sugar mama. Glycobiology. (2012) 22:1147–62. doi: 10.1093/glycob/cws074
11. Chichlowski M, German JB, Lebrilla CB, Mills DA. The influence of milk oligosaccharides on microbiota of infants: opportunities for formulas. Annu Rev Food Sci Technol. (2011) 2:331–51.
12. Underwood MA, German JB, Lebrilla CB, Mills DA. Bifidobacterium longum subspecies infantis: champion colonizer of the infant gut. Pediatr Res. (2015) 77:229–35. doi: 10.1038/pr.2014.156
13. Frese SA, Hutton AA, Contreras LN, Shaw CA, Palumbo MC, Casaburi G, et al. Persistence of supplemented Bifidobacterium longum subsp. infantis EVC001 in breastfed infants. mSphere. (2017) 2:e501–17. doi: 10.1128/mSphere.00501-17
14. Chichlowski M, Shah N, Wampler JL, Wu SS, Vanderhoof JA. Bifidobacterium longum Subspecies infantis (B. infantis) in pediatric nutrition: current state of knowledge. Nutrients. (2020) 12:1581. doi: 10.3390/nu12061581
15. Henrick BM, Hutton AA, Palumbo MC, Casaburi G, Mitchell RD, Underwood MA, et al. Elevated fecal pH indicates a profound change in the breastfed infant gut microbiome due to reduction of bifidobacterium over the past century. mSphere. (2018) 3:e00041-18. doi: 10.1128/mSphere.00041-18
16. Bai Y, Tao J, Zhou J, Fan Q, Liu M, Hu Y, et al. Fucosylated human milk oligosaccharides and N-Glycans in the milk of Chinese mothers regulate the gut microbiome of their breast-fed infants during different lactation stages. mSystems. (2018) 3:e00206-18. doi: 10.1128/mSystems.00206-18
17. Pace RM, Williams JE, Robertson B, Lackey KA, Meehan CL, Price WJ, et al. Variation in human milk composition is related to differences in milk and infant fecal microbial communities. Microorganisms. (2021) 9:1153.
18. Borewicz K, Gu F, Saccenti E, Hechler C, Beijers R, de Weerth C, et al. The association between breastmilk oligosaccharides and faecal microbiota in healthy breastfed infants at two, six, and twelve weeks of age. Sci Rep. (2020) 10:4270. doi: 10.1038/s41598-020-61024-z
19. Masi AC, Stewart CJ. Untangling human milk oligosaccharides and infant gut microbiome. iScience. (2021) 25:103542–103542. doi: 10.1016/j.isci.2021.103542
20. Olga L, Petry CJ, van Diepen JA, Prentice PM, Hughes IA, Vervoort J, et al. Extensive study of breast milk and infant growth: protocol of the cambridge baby growth and breastfeeding study (CBGS-BF). Nutrients. (2021) 13:2879. doi: 10.3390/nu13082879
21. Prentice P, Acerini CL, Eleftheriou A, Hughes IA, Ong KK, Dunger DB. Cohort profile: the Cambridge Baby Growth Study (CBGS). Int J Epidemiol. (2016) 45:35a–35g.
22. Durham SD, Robinson RC, Olga L, Ong KK, Chichlowski M, Dunger DB, et al. A one-year study of human milk oligosaccharide profiles in the milk of healthy UK mothers and their relationship to maternal FUT2 genotype. Glycobiology. (2021) 31:1254–67. doi: 10.1093/glycob/cwab057
23. Dixon P. VEGAN, a package of R functions for community ecology. J Vegetat Sci. (2003) 14:927–30.
24. Paradis E, Schliep K. ape 5.0: an environment for modern phylogenetics and evolutionary analyses in R. Bioinformatics. (2019) 35:526–8. doi: 10.1093/bioinformatics/bty633
25. Benjamini Y, Hochberg Y. Controlling the false discovery rate: a practical and powerful approach to multiple testing. J R Stat Soc. (1995) 57:289–300.
26. Olga L, Vervoort J, van Diepen JA, Gross G, Petry CJ, Prentice PM, et al. Associations between breast milk intake volume, macronutrient intake, and infant growth in a longitudinal birth cohort: the Cambridge Baby Growth and Breastfeeding Study (CBGS-BF). Br J Nutr. (2022). [Online ahead of print]. doi: 10.1017/S0007114522003178
27. Ma J, Li Z, Zhang W, Zhang C, Zhang Y, Mei H, et al. Comparison of gut microbiota in exclusively breast-fed and formula-fed babies: a study of 91 term infants. Sci Rep. (2020) 10:15792. doi: 10.1038/s41598-020-72635-x
28. Roger LC, Costabile A, Holland DT, Hoyles L, McCartney AL. Examination of faecal Bifidobacterium populations in breast- and formula-fed infants during the first 18 months of life. Microbiology. (2010) 156:3329–41. doi: 10.1099/mic.0.043224-0
29. Commare CE, Tappenden KA. Development of the infant intestine: implications for nutrition support. Nutr Clin Pract. (2007) 22:159–73.
30. Sakanaka M, Gotoh A, Yoshida K, Odamaki T, Koguchi H, Xiao J-Z, et al. Varied pathways of infant gut-associated Bifidobacterium to assimilate human milk oligosaccharides: prevalence of the gene set and its correlation with bifidobacteria-rich microbiota formation. Nutrients. (2020) 12:71. doi: 10.3390/nu12010071
31. Kiyohara M, Tanigawa K, Chaiwangsri T, Katayama T, Ashida H, Yamamoto K. An exo-α-sialidase from bifidobacteria involved in the degradation of sialyloligosaccharides in human milk and intestinal glycoconjugates. Glycobiology. (2010) 21:437–47. doi: 10.1093/glycob/cwq175
32. Sela DA, Chapman J, Adeuya A, Kim JH, Chen F, Whitehead TR, et al. The genome sequence of Bifidobacterium longum subsp. infantis reveals adaptations for milk utilization within the infant microbiome. Proc Natl Acad Sci USA. (2008) 105:18964–9.
33. Walsh C, Lane JA, van Sinderen D, Hickey RM. Human milk oligosaccharide-sharing by a consortium of infant derived Bifidobacterium species. Sci Rep. (2022) 12:4143. doi: 10.1038/s41598-022-07904-y
34. Shani G, Hoeflinger JL, Heiss BE, Masarweh CF, Larke JA, Jensen NM, et al. Fucosylated human milk oligosaccharide foraging within the species Bifidobacterium pseudocatenulatum is driven by glycosyl hydrolase content and specificity. Appl Environ Microbiol. (2022) 88:e0170721. doi: 10.1128/AEM.01707-21
35. Cheema AS, Trevenen ML, Turlach BA, Furst AJ, Roman AS, Bode L, et al. Exclusively breastfed infant microbiota develops over time and is associated with human milk oligosaccharide intakes. Int J Mol Sci. (2022) 23:2804. doi: 10.3390/ijms23052804
Keywords: microbiome, human milk oligosaccharides (HMO), bifidobacteria, fucosyllactose, clinical
Citation: Chichlowski M, van Diepen JA, Prodan A, Olga L, Ong KK, Kortman GAM, Dunger DB and Gross G (2023) Early development of infant gut microbiota in relation to breastfeeding and human milk oligosaccharides. Front. Nutr. 10:1003032. doi: 10.3389/fnut.2023.1003032
Received: 25 July 2022; Accepted: 09 January 2023;
Published: 09 March 2023.
Edited by:
Defu Ma, Peking University, ChinaReviewed by:
Ana Griselda Binetti, CONICET Instituto de Lactología Industrial (INLAIN), ArgentinaKieuske Yoshida, Morinaga Milk Industry Co., Ltd., Japan
Takahiro Matsuki, Yakult Central Institute for Microbiological Research, Japan
Copyright © 2023 Chichlowski, van Diepen, Prodan, Olga, Ong, Kortman, Dunger and Gross. This is an open-access article distributed under the terms of the Creative Commons Attribution License (CC BY). The use, distribution or reproduction in other forums is permitted, provided the original author(s) and the copyright owner(s) are credited and that the original publication in this journal is cited, in accordance with accepted academic practice. No use, distribution or reproduction is permitted which does not comply with these terms.
*Correspondence: Janna A. van Diepen, SmFubmEudmFuRGllcGVuQHJlY2tpdHQuY29t
†Deceased