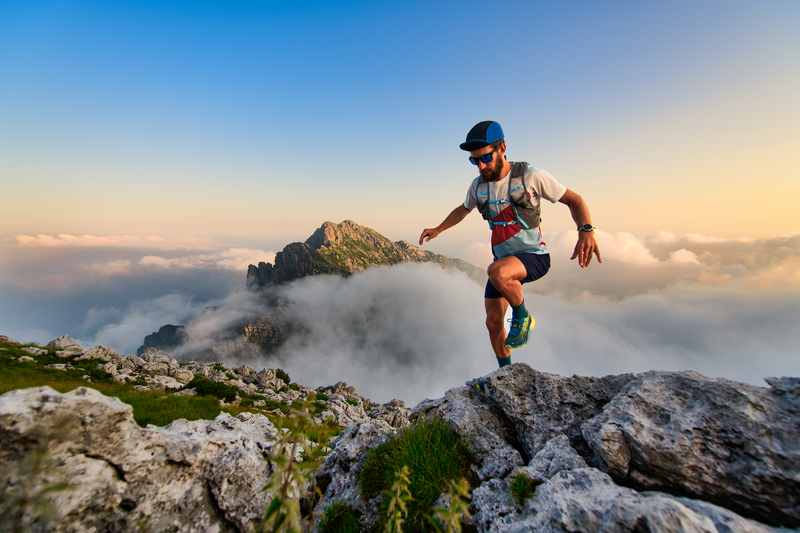
95% of researchers rate our articles as excellent or good
Learn more about the work of our research integrity team to safeguard the quality of each article we publish.
Find out more
ORIGINAL RESEARCH article
Front. Nutr. , 15 September 2022
Sec. Nutrition and Food Science Technology
Volume 9 - 2022 | https://doi.org/10.3389/fnut.2022.989410
Rapeseed cake is a by-product of rapeseed oil separation. The nutritional components of rapeseed cake mainly include a variety of carbohydrates, proteins, and minerals. In order to improve the conversion rate of rapeseed cake, we studied the physicochemical properties, the structure of microbial communities, and the composition of metabolites in rapeseed cake after enzymatic fermentation. The results showed that the addition of enzymatic preparation increased microbial diversity. The relative abundance of Bacillus, Lysinibacillus, Empedobacter, Debaryomyces, Hyphopichia, and Komagataella in enzymatic fermentation was significantly higher than that in natural fermentation. Unlike natural fermentation, microbial diversity during enzymatic fermentation is specific, which improves the efficiency of fermentation. Otherwise, enzymatic fermentation promotes the conversion of macromolecular substances in rapeseed cake, which increases small metabolites, such as fatty acids, organic acids, amino acids and their derivatives. The metabolite enrichment pathway is mostly concentrated in sugar metabolism and fatty acid metabolism. In conclusion, after adding enzymatic preparation, enzymes and microorganisms jointly promote the transformation of macromolecules during the fermentation of rapeseed cake, which laid a good foundation for further utilization of rapeseed cake.
Rapeseed cake is a by-product of rapeseed oil separation. It has a huge output in China. It is mainly composed of protein, wood fiber, and minerals (1). Its crude protein content is 31–35.7%, and the crude protein contains 82% amino acids, with a good balance of essential amino acids and a relatively high content of sulfur-containing amino acids (2). Usually, the rapeseed cake is used as animal feedstuffs or soil fertilizers (3).
Rapeseed cake is a feed component with huge economic potential. However, it contains anti-nutritional compounds, such as glucosinolates, phytic acid, alkaloids, erucic acid, and tannins, which may be harmful to the digestive system of animals (4). Therefore, the direct use of rapeseed cake as animal feed is greatly limited. In order to improve the effective utilization of rapeseed cake, it needs to be fermented. Currently, the results showed that the solid-state fermentation of rapeseed cake by Aspergillus niger was helpful to degrade glucosinolates, improve their nutritional value, and improve their application in animal feed (2). In the process of solid-state fermentation, inoculation of the fungus Mortierella alpina has the potential to improve the nutritional value of rapeseed cake. At the same time, the utilization of linseed oil as a supplement to the fermentation was positive for fungal growth and the production of protein (5). During solid-state fermentation, adding mixed fungi such as Rhyzopus oryzae, Aspergillus ibericus, and Aspergillus niger can obtain more cellulase and xylanase, which can improve bioactive compounds, so as to improve the nutritional value of rapeseed cake and improve animal feed by-products (6).
Rapeseed cake is also a traditional organic fertilizer widely used in crops, vegetables, fruit trees, etc. It has been shown that the application of rapeseed cake fertilizer in rice fields could increase the soil microbial population and improve the rice yield by regulating the nutrient retention and release process (7–9). Mixing rapeseed cake and inorganic fertilizer is beneficial to increasing corn plant biomass and improving crop productivity, soil fertility, and nutrient balance (10, 11). However, as a kind of fertilizer, rapeseed cake is mainly applied directly, which is difficult to give full play to its best fertilizer efficiency, resulting in the shortcomings of high cost and slow fertilizer effect.
In order to improve the utilization of rapeseed cake, the changes in nutrients, microbial community structure, and metabolites of rapeseed cake during natural fermentation and enzymatic fermentation were studied, which provided a theoretical basis for the fermentation and deep utilization of rapeseed cake.
The experiment was conducted in Qingdao, Shandong Province, China (36° 18′ N, 120° 07′ E) in 2021. All the rapeseed cake in this test were purchased from Xincang’s No.1 oil manufacture (Anhui, China). The appearance of the rapeseed cakes was irregular and fragmented, and the color was brown to dark brown, with the aroma of cooked rapeseed. The fermentation barrel is made of high-density polyethylene, with a capacity of 25 L and good sealing performance. The high-efficiency enzymatic preparation is a mixture powder with bacteria and enzymes. Its main components are lipase, hemicellulase, yeast, lactic acid bacteria, amylolytic bacillus, and other digestive bacteria. The number of effective viable bacteria is ≥ 20 billion/g, and it is easy to synthesize digestive enzymes, with total enzyme activity > 10,000 μ/g.
The experiment was divided into two groups: natural fermentation (T1) and enzymatic fermentation (T2), with 3 fermentation barrels in each group. Add 8 kg rapeseed cake and 16 kg distilled water into each fermentation barrel, and the feed water ratio is 1:2. In T2, the high-efficiency enzymatic preparation shall be added in rapeseed cake at a 3‰ ratio based on the total weight of rapeseed cake and water. Then, evenly mixed and sealed. The fermentation process is facultative anaerobic until the fermentation is complete. Random sampling was carried out for each treatment in the four stages: the non-fermented stage (0d, S0), early stage (3d, S1), middle stage (7d, S2), and late stage (14d, S3). Then, each sample with 3 replications was divided into two parts, one part was stored at 4°C to determine its nutritional properties, and the other part was frozen at –80°C to determine its microbial composition and metabolites.
The contents of the free amino acid (AA) and the free fatty acids (FFA) were determined by using Suzhou Grace Biotechnology kits (China) following the manufacturer’s instructions. The total nitrogen (TN) content was analyzed by the Kjeldahl method, total phosphorus (TP) content was analyzed by the AutoAnalyzer system, and the total potassium (TK) content was analyzed by atomic absorption spectrophotometer (12). The content of organic carbon was analyzed by the K2Cr2O7/H2SO4 method (13). According to the method of Ortiz et al., the contents of mineral elements were determined (14). The enzymatic activities of lipase, hemicellulase, α-amylase, phytase, polygalacturonase, and cellulase were determined using microplate assay kits (Suzhou Grace Biotechnology Co., Ltd.).
According to the manufacturer’s protocols, the genomic deoxyribonucleic acid (DNA) of the rapeseed cake was extracted by CTAB method (15–17). Then, the purity and concentration of DNA were detected by agarose gel electrophoresis. A suitable sample of DNA was used in the centrifuge tube, and the sample was diluted with sterile water to 1 ng/μL. Using the diluted genomic DNA as the template. The DNA samples were individually amplified in V3 + V4 hyper variable regions by polymerase chain reaction (PCR) using primers 341F (5′-CCTAYGGGRBGCASCAG- 3′) and 806R (5′-GGACTACNNGGGTATCTAAT- 3′) for 16S rDNA in bacteria, and primers ITS5-1737F (5′- GGAAGTAAAAGTCGTAACAAGG- 3′) and ITS2-2043R (5′- GCTGCGTTCTTCATCGATGC- 3′) for ITS in fungi. Phusion ® High fidelity PCR Master Mix with GC buffer (New England Biolabs, United States) and high-efficiency and high-fidelity enzyme for PCR to ensure amplification efficiency and accuracy. PCR was carried out under the following conditions: 98°C for 30 s, 50°C for 30 s, and 72°C for 30 s for a total of 30 cycles. Then, the resulting PCR product was extracted from a 2% agarose gel and further purified and recycled using the Universal DNA purification kit (TianGen, China).
The purified amplicons were pooled on the Illumina NovaSeq platform (Illumina, San Diego, CA, United States) of equal molecular weight and double-end sequencing according to the standard protocol of MetWare Biotechnology Co., Ltd. (Wuhan, China). The sheared original sequencing sequences were merged by FLASH software (V1.2.7).1 Refer to Qiime (V1.9.1)2 Tags quality control process to filter the quality of the connection sequence. Then the sequences were compared with the reference database using the VSEARCH algorithm3 to detect chimeric sequences, and then the chimeric sequences were removed. The effective sequences were used in the final analysis. Sequences were grouped into operational taxonomic units (OTUs) at 97% sequence identity by using the clustering algorithm UPARSE (V7.0.1001).4 Using the confidence threshold of 70%, the classification of each 16S rRNA gene sequence was analyzed against the Silva database (SILVA138)5 through the Mothur classifier algorithm. Using the confidence threshold of 70%, the classification of each ITS sequence was analyzed against the Unite database (V 8.2)6 through the Blast7 classifier algorithm. Fast multi-sequence alignment was performed using MUSCLE (V3.8.31)8 software, finally, the data of each sample were homogenized.
The total metabolic profiles of the rapeseed cake with fermentation were performed using two independent approaches: UPLC-MS/MS and GC-MS. Metabolome analysis was performed by MetWare Biotechnology Co., Ltd. (Wuhan, China).
For UPLC-MS/MS analysis, extraction procedure and UPLC-MS/MS procedure were performed as described by Fraga et al. (18) and Chen et al. (19). For GC- MS analysis, the frozen samples of rapeseed cake were ground to a powder in liquid nitrogen. 1 g of the powder was transferred immediately to a 20 mL head-space vial (Agilent, Palo Alto, CA, United States), containing NaCl saturated solution, to inhibit any enzyme reaction. The vials were sealed using crimp-top caps with TFE-silicone headspace septa (Agilent, United States). The GC-MS procedure was modified based on previous studies (20). Briefly, the identification and quantification of derivatized samples were carried out using an Agilent Model 8890 GC and a 5977B mass spectrometer (Agilent, United States), equipped with a DB-5MS capillary column (5% phenyl-polymethyl siloxane: 30 m × 0.25 mm × 0.25 μm). Helium was used as the carrier gas at a linear velocity of 1.2 mL/min. The injector temperature was kept at 250°C and the detector at 280°C. The oven temperature was programmed from 40°C (3.5 min), increasing at 10°C/min to 100°C, at 7°C/min to 180°C, at 25°C/min to 280°C, and hold for 5 min. Mass spectra was recorded in electron impact (EI) ionization mode at 70 eV. The quadrupole mass detector, ion source, and transfer line temperatures were set, respectively, at 150°C, 230°C and 280°C. Mass spectra was scanned in the range m/z 50–500 amu at 1 s intervals. Identification of volatile compounds was achieved by comparing the mass spectra with the data system library (MWGC or NIST) and linear retention index.
The HCA (hierarchical cluster analysis) results of samples and metabolites were presented as heatmaps with dendrograms, while Pearson correlation coefficients (PCC) between samples were calculated by the core function in R and presented as only heatmaps. Both HCA and PCC were carried out by R package ComplexHeatmap. For HCA, normalized signal intensities of metabolites (unit variance scaling) are visualized as a color spectrum. Significantly regulated metabolites between groups were determined by VIP ≥ 1 and absolute log2FC (fold change) ≥ 1. VIP values were extracted from OPLS-DA result, which also contains score plots and permutation plots, and were generated using the R package MetaboAnalystR. The data was log transform (log2) and mean centering before OPLS-DA. In order to avoid overfitting, a permutation test (200 permutations) was performed.
Identified metabolites were annotated using the kyoto encyclopedia of genes and genomes (KEGG) Compound database9, and annotated metabolites were then mapped to the KEGG Pathway database.10 Pathways with significantly regulated metabolites mapped to were then fed into MSEA (metabolite sets enrichment analysis), and their significance was determined by the hypergeometric test’s p-values.
Statistical analysis was performed using SPSS 20.0 software (SPSS Inc., Chicago, United States). One-way analysis of variance (ANOVA) and Duncan’s test were used to determine significant differences (p < 0.05) among rapeseed cake nutrition analysis (GraphPad Prism 8.0) and the relative abundance of microbial taxa. Alpha diversity indexes were calculated by Qiime (Version 1.9.1), and the difference between beta diversity index groups was analyzed using R (Version 2.15.3). Spearman correlation coefficient (R2 ≥ 0.64 and p ≤ 0.05) was also used to analyze the correlation between the composition of microbial communities and metabolites in the rhizosphere.
In order to study the nutritional status of rapeseed cake under fermentation, and determine the degree of fermentation in rapeseed cake, the physical and chemical indexes of different stages were analyzed (Table 1). The results showed that the contents of AA and FFA increased gradually during the whole fermentation process. Compared with the S0, the S3 of T1 increased by 88.6 and 75.2% respectively, and the S3 of T2 increased by 93.1% and 2.13 times, respectively. In order to further study the composition of amino acids and fatty acids in rapeseed cake after fermentation, we analyzed the contents of 20 amino acids and fatty acids in four stages. The results showed that the content of methionine in T1 and T2 increased significantly with continuous fermentation, while the contents of tyrosine, valine, and lysine decreased. In S3, there were significant differences in some amino acids between the T1 and T2 (p < 0.05) (Figures 1A,B). With the continuous fermentation, oleic acid (FFA18:1), linoleic acid (FFA18:2), linolenic acid (FFA18:3), palmitic acid (FFA16:0), erucic acid (FFA22:1), eicosapentaenoic acid (FFA20:1) and stearic acid (FFA18:0) increased significantly. In S3, except erucic acid (FFA22:1), other components had extremely significant differences in T1 and T2 (p < 0.01), and the contents in T2 were higher than in T1 (Figures 1C,D).
Figure 1. The content of amino acids (AA) and free fatty acids (FFA). (A) AA content of fermented rapeseed cake in S1. (B) AA content of fermented rapeseed cake in S3. (C) FFA content of fermented rapeseed cake in S1. (D) FFA content of fermented rapeseed cake in S3. *p < 0.05; **p < 0.01; ***p < 0.001.
During the whole fermentation process, the content of OM tended to be stable without obvious change. In S3, the contents of total nitrogen, total phosphorus, and total potassium in T2 were significantly higher than those in T1 (p < 0.05). In addition, the mineral elements were analyzed in the fermented rapeseed cake, and there was no significant difference between enzymatic fermentation and natural fermentation (Supplementary Table 1). It indicated that the enzymatic preparation did not affect the content of trace elements.
To further clarify the changes in microbial community composition in rapeseed cake during fermentation, 16sDNA and ITS of microorganisms in rapeseed cake were sequenced. We used the alpha diversity indexes to judge the richness of microbial communities. During the whole fermentation process, the diversity coverage of bacteria and fungi exceeded 99.9%, indicating that the sequencing data were sufficient and the microbial community was almost covered. For bacteria, the diversity of T1 was significantly higher than that of T2 in S1 and S2 (p < 0.05), while the bacterial diversity of T2 was significantly higher than that of T1 in S3 (p < 0.05). For fungi, the diversity of T2 was higher than that of T1 in S0 and S1 (p < 0.05), while there was no significant difference between the two groups in S3. Chao1 index was used to calculate the abundance of microorganisms. There was no significant difference in abundance between the two groups at all fermentation stages (Table 2).
In order to intuitively study the species with a higher relative abundance, the top 10 phyla and genera were selected with the highest abundance according to the results of species annotation. In the bacterial community, the dominant phyla are Firmicutes, Proteobacteria, Cyanobacteria, and Bacteroidetes (Figure 2A). The dominant genera are Bacillus, Pseudomonas, Enterococcus, Lactobacillus, Weissella, Ralstonia, Acetobacter, Lysinibacillus and Comamonas (Figure 2C). In the fungal community, the dominant phyla are Ascomycota, Basidiomycota, Mucoromycota, Mortierellomycota, and Glomeromycota (Figure 2B). The dominant genera are Diotina, Wickerhamomyces, Mucor, Bipolaris, Debaryomyces, Naganishia, Candida, Cephaliophor, and Lysurus (Figure 2D).
Figure 2. The composition of bacterial and fungal communities in fermented rapeseed cake. (A) The histogram of bacterial composition in fermented rapeseed cake at the phylum level. (B) The histogram of fungal composition in fermented rapeseed cake at the phylum level. (C) The histogram of bacterial composition in fermented rapeseed cake at the genus level. (D) The histogram of fungal composition in fermented rapeseed cake at the genus level.
To further determine the microbial community composition at the genus level of rapeseed cake under different fermentation methods, we screened the bacterial and fungal communities with significant differences in abundance by using the inter-group t-test. For the bacterial community, in S0, the relative abundance of Bacillus in T2 treatment was significantly higher than that in T1 (Figure 3A). In S3, the relative abundance of Bacillus, Lysinibacillus, and Empedobacter in T2 were significantly higher than those in T1 (Figure 3B). In the fungal community, in S0, Diutina, Debaryomyces, and Hyphopichia in T2 were significantly higher than those in T1 (Figure 3C). In S3, Debaryomyces, Hyphopichia, and Komagataella in T2 were significantly higher than those in T1 (Figure 3D).
Figure 3. T-test of bacterial and fungal communities in fermented rapeseed cake. (A) The t-test of bacterial communities in fermented rapeseed cake in S0. (B) The t-test of bacterial communities in fermented rapeseed cake in S3. (C) The t-test of fungal communities in fermented rapeseed cake in S0. (D) The t-test of fungal communities in fermented rapeseed cake in S3.
In order to further analyze the metabolites of rapeseed cake under different fermentation methods, firstly, we identified the metabolites in T1 and T2 by GC-MS and LC-MS. A total of 1,055 metabolites were detected, mainly including organic acids, amino acids and their derivatives, lipids, flavonoids, terpenoids, esters, and aromatics. Secondly, OPLS-DA analysis was performed on the metabolites in four stages to maximize the differentiation between groups (Figure 4). The results showed that T1 and T2 were well separated in different stages, indicating that the metabolites of rapeseed cake changed significantly after adding enzymatic preparation. To further compare the differences of metabolites between T1 and T2, fold_change value (≥ 2, ≤ 0.5) and VIP value (≥ 1) were used to screen the differential metabolites in four stages, and 130, 411, 423 and 402 substances were screened, respectively (Supplementary Tables 2–5). During the whole fermentation process, organic acids, amino acids and their derivatives, lipids, terpenoids, esters, and flavonoids were significantly changed after adding enzymatic preparation (Figure 5A). With continuous fermentation, amino acids and their derivatives, lipids, and organic acids were significantly increased in T2 (Figure 5B).
Figure 4. OPLS-DA analysis of metabolites in fermented rapeseed cake. (A) OPLS-DA analysis of metabolites in fermented rapeseed cake in S0. (B) OPLS-DA analysis of metabolites in fermented rapeseed cake in S1. (C) OPLS-DA analysis of metabolites in fermented rapeseed cake in S2. (D) OPLS-DA analysis of metabolites in fermented rapeseed cake in S3.
Figure 5. (A) Column chart of metabolites in fermented rapeseed cake in four stages. (B) Cluster heat map of metabolites in fermented rapeseed cake in four stages.
During the whole fermentation process, the addition of high-efficiency enzymatic preparation significantly increased the levels of some lipids, mainly including methyl linolenate, (9Z,11E)-13-oxooctadecadecadecadeca-9,11-dienoic acid, 9-hydroxy-10,12,15-octadecaterenoic acid, 1-eicosanol, ricinoleic acid, 9oxo-10E,12Z-octadecadienoic acid, arachidic acid, and crepenynic acid. While methyl palmitate and 1-linoleoylglycerol were significantly decreased. In S2 and S3, 12-hydroxystearic acid, (9Z, 12Z)–(7S, 8S)-dihydroxyadeca-9,12-dienoic acid, (12Z)-9,10-dihydroxyadec-12-enoic acid, hexadecanedioic acid were significantly increased, while 5,8,11,14-pentadecanoamide, 1-monomyristin, and 17-Hydroxylinolenic acid were significantly decreased.
During the whole fermentation process, the addition of high-efficiency enzymatic preparation significantly increased some amino acids, mainly including L-Alanyl-L-Phenylalanine, L-Alanyl-L-Phenylalanine, L-Valyl-L-Leucine, L-Glycyl-L-Isoleucine, N-Glycyl-L-Leucine, L-Phenylalanine and L-Methionine. While L-Methionine Sulfoximine was significantly decreased. In S2 and S3, Hexanoyl-l-glycine, N6-acetyl-l-lysine, L-Isoleucine, L-Norleucine, L-Leucine, N,N-Dimethylglycine, and N-Acetyl-L-Methionine were significantly increased. While L-Proline was significantly decreased.
During the whole fermentation process, the addition of high-efficiency enzymatic preparation significantly increased some organic acids, mainly including: β- Hydroxyisovaleric acid, 2-Hydroxyisohexanoic acid, argininosuccinic acid, ethyl caffeic, trigallic acid, ethyl ferulate, phenethyl caffeic, and 3-Methoxybenzoic acid. While 3,4-Dihydroxyphenylacetic acid, 3-O-Methylgallic acid, 6′-O-Sinapoylsucrose, Gentisic Acid, Salicylic acid, and 4-Hydroxy-3-Methoxybenzaldehyde were significantly decreased. In S2 and S3, 4-Methylpentanoic acid, 6-Aminocaproic acid, L-Citric acid, 5-Acetamidopentanoic acid, 2-Methylsuccinic acid, Dimethylmalonic acid, Rosmarinic acid, Sinapic acid, etc. While Citric acid, (E)-3-(3,4-dihydroxyphenyl) acrylaldehyde, 4-Hydroxybenzoic acid, 6′-O-Feruloyl-D-sucrose, 1,3-O-Diferoyloylglycerol, and Hydroxyphenyllactic acid were significantly decreased.
To further explore the role of different metabolites in different fermentation methods, we enriched the selected compounds by the KEGG pathway. The results showed that, in S0, the differential metabolites were mainly enriched in amino sugar and nucleotide sugar metabolism; biosynthesis of unsaturated fatty acids; neomycin, kanamycin, and gentamicin biosynthesis; ascorbate and aldate metabolism; alpha-Linolenic acid metabolism; galactose metabolism and indole alkaloid biosynthesis (Figure 6A). In S1, the differential metabolites were mainly enriched in starch and sucrose metabolism, galactose metabolism, purine metabolism, glucosinolate biosynthesis, and ABC transporters (Figure 6B). In S2, the differential metabolites were mainly enriched in glucosinolate biosynthesis, 2-Oxocarboxylic acid metabolism, aminoacyl-tRNA biosynthesis, and phenylalanine metabolism (Figure 6C). In S3, the differential metabolites were mainly enriched in glucosinolate biosynthesis, galactose metabolism, aminoacyl tRNA biosynthesis, starch and sucrose metabolism, linoleic acid metabolism, biosynthesis of secondary metabolites, and inositol phosphate metabolism (Figure 6D).
Figure 6. Kyoto encyclopedia of genes and genomes (KEGG) enrichment pathway of different metabolites in fermented rapeseed cake. (A) KEGG enrichment pathway of different metabolites in fermented rapeseed cake in S0. (B) KEGG enrichment pathway of different metabolites in fermented rapeseed cake in S1. (C) KEGG enrichment pathway of different metabolites in fermented rapeseed cake in S2. (D) KEGG enrichment pathway of different metabolites in fermented rapeseed cake in S3.
In order to study the effect of enzymatic addition on fermentation products, we analyzed the correlation between enzymatic activity and differential metabolites. Heatmaps were prepared by using the differential metabolites and enzymatic activities with correlation (R2 ≥ 0.64, p < 0.05) (Figure 7). The results showed that lipase was significantly correlated with lipids, mainly including octanoic acid, methyl linolenate, and 12,13-Epoxy-9-Octadecenoic acid (Figure 7A). Hemicellulase was significantly related to organic acids, mainly including Sinapyl alcohol, 3-Methyl-2-Oxobutanoic acid, L-Cyclopentylglycine, N-Methyl-Trans-4-Hydroxy-L-Proline, γ-Glutamyltyrosine and 4-Methoxyphenylpropionic acid (Figure 7B). And α-amylase, phytase, polygalacturonase, and cellulase were not significantly correlated with metabolites.
Figure 7. Correlation diagram of six enzymes with metabolites in fermented rapeseed cake. (A) Correlation diagram of six enzymes with lipids in S3. (B) Correlation diagram of six enzymes with Organic acids in S3.
In order to study the correlation between differential microbiota and differential metabolites, spearman correlation was used to analyze the relationship between microorganisms and metabolites. The chord diagram was plotted by using the differential metabolites and microorganisms with correlation (R2 ≥ 0.64, p < 0.05) (Figure 8). The results showed that, in S0, the microbial community was significantly correlated with lipids by adding enzymatic preparation. Bacillus was significantly correlated with lipids, mainly including arachidic acid, 12-Oxo phytodienoic acid, 1-Eicosanol, 13 kode; (9Z, 11E)-13-Oxooctadeca-9,11-dienoic acid, myristoleic acid, α-Linolenic acid and palmitoleic acid (Figure 8A). Diutina and Debaryomyces were significantly correlated with 1-Oleoyl-Sn-Glycerol, palmitoleic acid, α-Linolenic acid, and eicosadienoic acid. Debaryomyces and Hyphopichia were significantly correlated with arachidic acid (Figure 8C). In S3, after adding enzymatic preparation, the microbial community was significantly correlated to amino acids and their derivatives. Bacillus, Lysinibacillus and Empedobacter were significantly correlated with L-valine, L-Tyrosine, L-Leucine, L-Tryptophan, L-Phenylalanine, L-Norleucine, L-Isoleucine and some derivatives (Figure 8B). Komagataella and Debaryomyces were significantly correlated to L-Tyrosine, L-Valine, L-Norleucine, L-Isoleucine, L-Leucine, L-Valine, and some derivatives. While Hyphopichia was significantly correlated to successful acid, L-Alanine, and some derivatives (Figure 8D).
Figure 8. Correlation chord diagram of microorganisms and metabolites. (A) Correlation chord diagram of bacteria and metabolites in S0. (B) Correlation chord diagram of bacteria and metabolites in S3. (C) Correlation chord diagram of fungus and metabolites in S0. (D) Correlation chord diagram of fungus and metabolites in S3.
Microbial diversity is of great significance to fermentation and promotes the flavor and stability of fermented products (21–23). According to the previous study, natural fermentation is an effective measure to maximize the role of “native microorganisms” (24). During the fermentation of Chinese liquor grains, the existence of hydrolase promotes the increase of bacterial diversity (25). In this study, it was obvious that the bacterial diversity of natural fermentation was significantly higher than that of enzymatic fermentation in the early and middle stages. While the bacterial diversity of enzymatic fermentation was significantly higher than that of natural fermentation in the late stage. We speculate that the addition of enzymatic preparation introduces a large number of Bacillus to participate in the fermentation process, thus inhibiting the growth of the original microbes and reducing the bacterial diversity of the rapeseed cake with enzymatic fermentation in the early and middle stages. With the progress of fermentation, the existence of hydrolase promotes the increase of bacterial diversity. The fungal diversity of enzymatic fermentation was higher than that of natural fermentation in the non-fermented and early stages. This could be because the addition of enzymatic preparation accelerated the degradation of macromolecules and increased the temperature, providing a more suitable environment for the survival of fungi. With the progress of fermentation, a suitable fermentation microenvironment was established, and the diversity of fungal species tended to be the same.
Previous studies showed that in the process of solid-state fermentation, high concentrations of volatile compounds produced by Bacillus and Empedobacter were significantly related to the volatile aroma of the fermentation products (26–28). Bacillus can reduce the anti-nutritional factors during soybean meal fermentation, which is conducive to improving the quality of fermented products (29). Lysinibacillus can degrade urea produced by solid-state fermentation and reduce toxic substances in fermentation products (30, 31). In this study, although the dominant phyla in natural fermentation and enzymatic fermentation are the same, their abundance is different. The main performance is that the abundance of Firmicutes, Bacteroidetes, and Ascomycota by enzymatic fermentation is significantly higher than that in natural fermentation. After fermentation, the relative abundance of Bacillus, Lysinibacillus, Empedobacter, Debaryomyces, Hyphopichia, and Komagataella in enzymatic fermentation was significantly higher than that in natural fermentation (Figures 3B,D). The results showed that, unlike natural fermentation, microbial diversity during enzyme fermentation is specific, which improves fermentation efficiency.
Through the analysis of KEGG pathway enrichment, it was obviously found that, unlike natural fermentation, the metabolite enrichment pathway in the process of enzymatic fermentation was specific, and mostly concentrated in sugar metabolism and fatty acid metabolism. The composition of metabolites is an important indicator to judge the degree of fermentation (32–34). With the continuous fermentation of rapeseed cake, the metabolites increased gradually. More substances tended to be up-regulated after adding high-efficiency enzymatic preparation, such as fatty acids, organic acids, amino acids and their derivatives. This further shows that the addition of enzymatic preparation helps to promote the fermentation of rapeseed cake, and more organic matter is decomposed into small molecules.
Previous studies have shown that bacteria release lipase during fermentation (35). Lipid hydrolysis can explain the increase of saturated medium chain fatty acids after fermentation, such as palmitic acid, trans-13-octadecenoic acid, linolenic acid, stearic acid, etc. (36). In this study, with continuous fermentation, FFA gradually increased, including oleic acid, linoleic acid, linolenic acid, palmitic acid, and so on. The contents of fatty acids after enzymatic fermentation were much higher than that of natural fermentation. We speculate that one part of FFA comes from the decomposition of lipids by microbial hydrolases, and the other part comes from the synthesis of carbohydrates. It is proved that enzymatic fermentation is effective in improving the formation of fatty acids in rapeseed cake.
Organic acids are the main components of chemical substances and the intermediate products of the metabolic cycle produced by microbial processes (37). In this study, the beneficial organic acids were significantly up-regulated after enzymatic fermentation. We speculate that it is due to the presence of lactic acid bacteria in the fermentation process, which decomposes carbohydrates into more organic acids. In addition, organic acids provide an acidic environment for the fermentation process and a suitable environment for the survival of lactic acid bacteria.
There are large amounts of proteins in rapeseed cake, which can be transformed into amino acids and their derivatives through the action of enzymes and microorganisms. In this study, the contents of amino acids and their derivatives were significantly increased after enzymatic fermentation. On the one hand, under the action of microorganisms and enzymes, proteins or peptides are decomposed into small molecular amino acids and their derivatives; on the other hand, as the precursor of secondary metabolites, amino acids participate in the synthesis of other substances. Amino acids and derivatives participate in cellular immune response and affect plant growth. Several amino acids can be used as precursors for the synthesis of secondary metabolites (38–40). In addition, microbial proteinases released during fermentation also help to decompose complex proteins into their constituent amino acids. Therefore, enzymatic fermentation will further promote the application value of rapeseed cake.
Combining microbiomes and metabolomics, studying the relationship between metabolites and microbial community changes can provide a theoretical basis for the efficient fermentation of rapeseed cake (41, 42). Previous studies have shown that Firmicutes tend to enrich in high carbohydrate species, and can degrade macromolecular organic substances such as sugars and proteins (43). Bacteroidetes occupy a major advantage in many fermentation processes. It can decompose macromolecule compounds, including polysaccharides, fats, and proteins, into small organic acids, including monosaccharides, lower alcohols and amino acids (44, 45). In anaerobic fermentation, Proteobacteria consume volatile fatty acids, propionic acid, and butyric acid (46). In this study, a close relationship between microbial community and metabolites was found during the fermentation of rapeseed cake. The bacterial and fungal communities in fermentation products were strongly associated with metabolites. Spearman correlation chord diagram showed that bacteria and fungi were significantly correlated with lipids in the non-fermented stage. In the late stage of fermentation with enzymatic preparation, Bacillus, Lysinibacillus, Empedobacter, Komagataella, Debaryomyces, and Hyphopichia were significantly associated with organic acids and amino acids.
Hemicellulase and cellulase are important hydrolases, which are mainly used in the saccharification process (47). In this study, after adding enzymatic preparation, the existence of hemicellulase and lipase makes the conversion rate of lipids and acids higher, mainly including octanoic acid, methyl linolenate, 3-methyl-2-oxobutanoic acid, and sinapyl alcohol. The above results indicated that enzymes and microorganisms jointly promoted the conversion and degradation of macromolecules such as proteins, sugars, and fats in rapeseed cake.
In this study, the addition of enzymatic preparation increases microbial diversity. The relative abundance of Bacillus, Lysinibacillus, Empedobacter, Debaryomyces, Hyphopichia, and Komagataella in enzymatic fermentation was significantly higher than that in natural fermentation. Unlike natural fermentation, microbial diversity during enzymatic fermentation is specific, which improves the efficiency of fermentation. Otherwise, enzymatic fermentation promotes the conversion of macromolecular substances of rapeseed cake, which increases small metabolites, such as fatty acids, organic acids, amino acids and their derivatives. The metabolite enrichment pathway is mostly concentrated in sugar metabolism and fatty acid metabolism. In conclusion, after adding enzymatic preparation, enzymes and microorganisms jointly promote the transformation of macromolecules during the fermentation of rapeseed cake, which laid a good foundation for further utilization of rapeseed cake.
The data presented in this study are deposited in the NCBI SRA repository, accession numbers: PRJNA869846 and PRJNA869857.
YSo conducted an experiment, analyzed the data, and wrote the manuscript. SZ and YSh collected the samples. ZD, YW, and KF put forward hypotheses and designed the experiments. LS and HW reviewed the manuscript. YZS, WW, JZ, YM, and XH participated in the experimental design and guided the research. All authors contributed to the article and approved the submission.
This research was funded by the Significant Application Projects of Agriculture Technology Innovation in Shandong Province (no. SD2019ZZ010), the Technology System of Modern Agricultural Industry in Shandong Province (no. SDAIT-19-01), the Special Foundation for Distinguished Taishan Scholar of Shandong Province (no. ts201712057), the Livelihood Project of Qingdao City (no. 21-1-4-ny-2-nsh), and the Project of Agricultural Science and Technology Fund in Shandong Province (nos. 2019LY002, 2019YQ010, and 2019TSLH0802).
The authors declare that the research was conducted in the absence of any commercial or financial relationships that could be construed as a potential conflict of interest.
All claims expressed in this article are solely those of the authors and do not necessarily represent those of their affiliated organizations, or those of the publisher, the editors and the reviewers. Any product that may be evaluated in this article, or claim that may be made by its manufacturer, is not guaranteed or endorsed by the publisher.
The Supplementary Material for this article can be found online at: https://www.frontiersin.org/articles/10.3389/fnut.2022.989410/full#supplementary-material
1. Lomascolo A, Uzan-Boukhris E, Sigoillot JC, Fine F. Rapeseed and sunflower meal: a review on biotechnology status and challenges. Appl Microbiol Biotechnol. (2012) 95:1105–14. doi: 10.1007/s00253-012-4250-6
2. Shi C, He J, Yu J, Yu B, Huang Z, Mao X, et al. Solid state fermentation of rapeseed cake with Aspergillus niger for degrading glucosinolates and upgrading nutritional value. J Anim Sci Biotechnol. (2015) 6:13. doi: 10.1186/s40104-015-0015-2
3. Ramachandran S, Singh SK, Larroche C, Soccol CR, Pandey A. Oil cakes and their biotechnological applications–a review. Bioresour Technol. (2007) 98:2000–9. doi: 10.1016/j.biortech.2006.08.002
4. Konkol D, Szmigiel I, Domzal-Kedzia M, Kulazynski M, Krasowska A, Opalinski S, et al. Biotransformation of rapeseed meal leading to production of polymers, biosurfactants, and fodder. Bioorg Chem. (2019) 93:102865. doi: 10.1016/j.bioorg.2019.03.039
5. Ferreira M, Fernandes H, Peres H, Oliva-Teles A, Belo I, Salgado JM. Bio-enrichment of oilseed cakes by Mortierella alpina under solid-state fermentation. Lwt Food Sci Technol. (2020) 134:109981. doi: 10.1016/j.lwt.2020.109981
6. Sousa D, Salgado JM, Cambra-Lopez M, Dias ACP, Belo I. Degradation of lignocellulosic matrix of oilseed cakes by solid-state fermentation: fungi screening for enzymes production and antioxidants release. J Sci Food Agric. (2022) 102:1550–60. doi: 10.1002/jsfa.11490
7. Teng Q, Hu XF, Chang YY, Luo F, Liu L, Cheng C, et al. Effects of different fertilisers on rice resistance to pests and diseases. Soil Res. (2016) 54:242–53. doi: 10.1071/sr15144
8. Zhang M, Tian YH, Zhao M, Yin B, Zhu ZL. The assessment of nitrate leaching in a rice-wheat rotation system using an improved agronomic practice aimed to increase rice crop yields. Agric Ecosyst Environ. (2017) 241:100–9. doi: 10.1016/j.agee.2017.03.002
9. Zhou TY, Chen L, Wang WL, Xu YJ, Zhang WY, Zhang H, et al. Effects of application of rapeseed cake as organic fertilizer on rice quality at high yield level. J Sci Food Agric. (2022) 102:1832–41. doi: 10.1002/jsfa.11518
10. Karforma J, Ghosh M, Ghosh DC, Mandal S, Ghosh PK. Effect of integrated nutrient management on performance of rainfed fodder maize-rapeseed cropping system. Range Manag Agrofor. (2016) 37:214–21.
11. Raza ST, Wu JP, Ali Z, Anjum R, Bazai NA, Feyissa A, et al. Differential effects of organic amendments on maize biomass and nutrient availability in upland calcareous soil. Atmosphere. (2021) 12:1034. doi: 10.3390/atmos12081034
12. Thomas RL, Sheard RW, Moyer JR. Comparison of conventional and automated procedures for nitrogen, phosphorus, and potassium analysis of plant material using a single digestion. Agron J. (1967) 59:240–3. doi: 10.2134/agronj1967.00021962005900030010x
13. Chen DG, Yu XZ, Song C, Pang XL, Huang J, Li YJ. Effect of pyrolysis temperature on the chemical oxidation stability of bamboo biochar. Bioresour Technol. (2016) 218:1303–6. doi: 10.1016/j.biortech.2016.07.112
14. Ortiz J, Astudillo G, Castro M, Castro C, Astudillo S, Donoso S. 12th IFDC 2017 Special Issue-Seasonal variations in nutrient composition of plant-based foods produced at the Southern highlands of Ecuador. J Food Composit Anal. (2019) 83:103284. doi: 10.1016/j.jfca.2019.103284
15. Nickerent DL. From field to film: rapid sequencing methods for field-collected plant species. Biotechniques. (1994) 16:470–5.
16. Aziz M, Singh S, Anand Kumar P, Bhatnagar R. Expression of protective antigen in transgenic plants: a step towards edible vaccine against anthrax. Biochem Biophys Res Commun. (2002) 299:345–51. doi: 10.1016/s0006-291x(02)02625-6
17. Lutz K, Wang W, Zdepski A, Michael T. Isolation and analysis of high quality nuclear DNA with reduced organellar DNA for plant genome sequencing and resequencing. BMC Biotechnol. (2011) 11:54. doi: 10.1186/1472-6750-11-54
18. Fraga C, Clowers B, Moore R, Zink E. Signature-discovery approach for sample matching of a nerve-agent precursor using liquid chromatography-mass spectrometry, XCMS, and chemometrics. Anal Chem. (2010) 82:4165–73. doi: 10.1021/ac1003568
19. Chen W, Gong L, Guo ZL, Wang WS, Zhang HY, Liu XQ, et al. A novel integrated method for large-scale detection, identification, and quantification of widely targeted metabolites: application in the study of rice metabolomics. Mol Plant. (2013) 6:1769–80. doi: 10.1093/mp/sst080
20. Huang W, Gfeller V, Erb M. Root volatiles in plant-plant interactions II: root volatiles alter root chemistry and plant-herbivore interactions of neighbouring plants. Plant Cell Environ. (2019) 42:1964–73. doi: 10.1111/pce.13534
21. Li H, He RY, Xiong XM, Zhang MC, Yang TY, Jiang ZB, et al. Dynamic diversification of bacterial functional groups in the Baiyunbian liquor stacking fermentation process. Ann Microbiol. (2016) 66:1229–37. doi: 10.1007/s13213-016-1211-9
22. Xin XD, He JG, Li L, Qiu W. Enzymes catalyzing pre-hydrolysis facilitated the anaerobic fermentation of waste activated sludge with acidogenic and microbiological perspectives. Bioresour Technol. (2018) 250:69–78. doi: 10.1016/j.biortech.2017.09.211
23. Ji XA, Yu XW, Wu Q, Xu Y. Initial fungal diversity impacts flavor compounds formation in the spontaneous fermentation of Chinese liquor. Food Res Int. (2022) 155:110995. doi: 10.1016/j.foodres.2022.110995
24. De Filippis F, La Storia A, Blaiotta G. Monitoring the mycobiota during Greco di Tufo and Aglianico wine fermentation by 18S rRNA gene sequencing. Food Microbiol. (2017) 63:117–22. doi: 10.1016/j.fm.2016.11.010
25. Guo JH, Sun LH, Liu XL. Effects of cellulase-producing bacteria on bacterial community structure and diversity during fermentation of Chinese liquor grains. J Institute Brewing. (2017) 123:130–7. doi: 10.1002/jib.390
26. Zhang R, Wu Q, Xu Y. Aroma characteristics of Moutai-flavour liquor produced with Bacillus licheniformis by solid-state fermentation. Lett Appl Microbiol. (2013) 57:11–8. doi: 10.1111/lam.12087
27. Wu HC, Zhang SY, Ma YY, Zhou J, Luo HB, Yang JG. Comparison of microbial communities in the fermentation starter used to brew Xiaoqu liquor. J Institute Brewing. (2017) 123:113–20. doi: 10.1002/jib.388
28. Wei GM, Regenstein JM, Zhou P. The aroma profile and microbiota structure in oil furu, a Chinese fermented soybean curd. Food Res Int. (2021) 147:110473. doi: 10.1016/j.foodres.2021.110473
29. Zheng L, Li D, Li ZL, Kang LN, Jiang YY, Liu XY, et al. Effects of Bacillus fermentation on the protein microstructure and anti-nutritional factors of soybean meal. Lett Appl Microbiol. (2017) 65:520–6. doi: 10.1111/lam.12806
30. Wu Q, Lin JC, Cui KX, Du RB, Zhu Y, Xu Y. Effect of microbial interaction on urea metabolism in Chinese liquor fermentation. J Agric Food Chem. (2017) 65:11133–9. doi: 10.1021/acs.jafc.7b04099
31. Cui KX, Wu Q, Xu Y. Biodegradation of Ethyl carbamate and urea with Lysinibacillus sphaericus MT33 in Chinese liquor fermentation. J Agric Food Chem. (2018) 66:1583–90. doi: 10.1021/acs.jafc.7b05190
32. Park SE, Seo SH, Kim EJ, Na CS, Son HS. Effects of different fermentation temperatures on metabolites of Kimchi. Food Biosci. (2018) 23:100–6. doi: 10.1016/j.fbio.2018.03.009
33. Li JJ, Zhao YY, Qin YQ, Shi HZ. Influence of microbiota and metabolites on the quality of tobacco during fermentation. BMC Microbiol. (2020) 20:356. doi: 10.1186/s12866-020-02035-8
34. Zhou XJ, Liu ZQ, Xie L, Li LY, Zhou WH, Zhao LZ. The correlation mechanism between dominant bacteria and primary metabolites during fermentation of red sour soup. Foods. (2022) 11:341. doi: 10.3390/foods11030341
35. Mahapatra P, Kumari A, Garlapati V, Banerjee R, Nag A. Optimization of process variables for lipase biosynthesis from Rhizopus oligosporus NRRL 5905 using evolutionary operation factorial design technique. Indian J Microbiol. (2010) 50:396–403. doi: 10.1007/s12088-011-0071-z
36. Gupta S, Lee JJL, Chen WN. Analysis of improved nutritional composition of potential functional food (Okara) after probiotic solid-state fermentation. J Agric Food Chem. (2018) 66:5373–81. doi: 10.1021/acs.jafc.8b00971
37. Ricke S. Perspectives on the use of organic acids and short chain fatty acids as antimicrobials. Poultry Sci. (2003) 82:632–9. doi: 10.1093/ps/82.4.632
38. Hildebrandt TM, Nesi AN, Araujo WL, Braun HP. Amino acid catabolism in plants. Mol Plant. (2015) 8:1563–79. doi: 10.1016/j.molp.2015.09.005
39. Dinkeloo K, Boyd S, Pilot G. Update on amino acid transporter functions and on possible amino acid sensing mechanisms in plants. Semin Cell Dev Biol. (2018) 74:105–13. doi: 10.1016/j.semcdb.2017.07.010
40. Li D, Zhang XC, Qu HX, Li L, Mao BZ, Xu YQ, et al. Delaying the biosynthesis of aromatic secondary metabolites in postharvest strawberry fruit exposed to elevated CO2 atmosphere. Food Chem. (2020) 306:125611. doi: 10.1016/j.foodchem.2019.125611
41. Deng YK, Huang D, Han BL, Ning XQ, Yu D, Guo HX, et al. Correlation: between autochthonous microbial diversity and volatile metabolites during the fermentation of nongxiang daqu. Front Microbiol. (2021) 12:688981. doi: 10.3389/fmicb.2021.688981
42. Qiu XY, Yu LL, Wang WY, Yan RM, Zhang ZB, Yang HL, et al. Comparative evaluation of microbiota dynamics and metabolites correlation between spontaneous and inoculated fermentations of nanfeng tangerine wine. Front Microbiol. (2021) 12:649978. doi: 10.3389/fmicb.2021.649978
43. Zhang Q, Cao J, Wu Y, Zhao J, Guo W, Huang W, et al. Shifts of microbial community and metabolic function during food wastes and waste activated sludge co-fermentation in semi-continuous-flow reactors: effects of fermentation substrate and zero-valent iron. Bioresour Technol. (2020) 313:123686. doi: 10.1016/j.biortech.2020.123686
44. Peng BY, Huang SC, Liu TT, Geng AL. Bacterial xylose isomerases from the mammal gut Bacteroidetes cluster function in Saccharomyces cerevisiae for effective xylose fermentation. Microbial Cell Factor. (2015) 14:70. doi: 10.1186/s12934-015-0253-1
45. Brinkmann S, Kurz M, Patras MA, Hartwig C, Marner M, Leis B, et al. Genomic and chemical decryption of the bacteroidetes phylum for its potential to biosynthesize natural products. Microbiol Spectr. (2022) 10:e0247921. doi: 10.1128/spectrum.02479-21
46. Luo JY, Zhu Y, Song AQ, Wang L, Shen C, Gui ZC, et al. Efficient short-chain fatty acids recovery from anaerobic fermentation of wine vinasse and waste activated sludge and the underlying mechanisms. Biochem Eng J. (2019) 145:18–26. doi: 10.1016/j.bej.2019.02.010
Keywords: rapeseed cake, enzymatic fermentation, bacillus, Lysinibacillus, organic acid, amino acid, fatty acid
Citation: Song Y, Sun L, Zhang S, Fan K, Wang H, Shi Y, Shen Y, Wang W, Zhang J, Han X, Mao Y, Wang Y and Ding Z (2022) Enzymes and microorganisms jointly promote the fermentation of rapeseed cake. Front. Nutr. 9:989410. doi: 10.3389/fnut.2022.989410
Received: 12 July 2022; Accepted: 18 August 2022;
Published: 15 September 2022.
Edited by:
Zhaojun Wei, Hefei University of Technology, ChinaReviewed by:
Nattakorn Kuncharoen, Kasetsart University, ThailandCopyright © 2022 Song, Sun, Zhang, Fan, Wang, Shi, Shen, Wang, Zhang, Han, Mao, Wang and Ding. This is an open-access article distributed under the terms of the Creative Commons Attribution License (CC BY). The use, distribution or reproduction in other forums is permitted, provided the original author(s) and the copyright owner(s) are credited and that the original publication in this journal is cited, in accordance with accepted academic practice. No use, distribution or reproduction is permitted which does not comply with these terms.
*Correspondence: Yu Wang, d2FuZ3l1dGVhQDE2My5jb20=; Zhaotang Ding, ZHp0dGVhQDE2My5jb20=
Disclaimer: All claims expressed in this article are solely those of the authors and do not necessarily represent those of their affiliated organizations, or those of the publisher, the editors and the reviewers. Any product that may be evaluated in this article or claim that may be made by its manufacturer is not guaranteed or endorsed by the publisher.
Research integrity at Frontiers
Learn more about the work of our research integrity team to safeguard the quality of each article we publish.