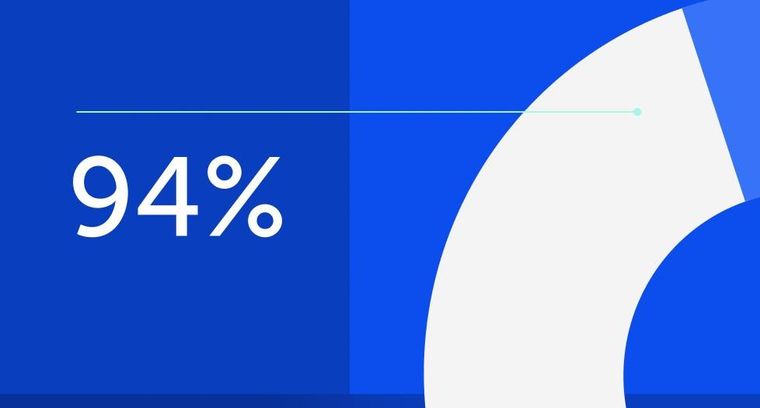
94% of researchers rate our articles as excellent or good
Learn more about the work of our research integrity team to safeguard the quality of each article we publish.
Find out more
ORIGINAL RESEARCH article
Front. Nutr., 17 August 2022
Sec. Nutrition and Metabolism
Volume 9 - 2022 | https://doi.org/10.3389/fnut.2022.987956
Background: 7,8-dihydroxyflavone (DHF) is a naturally occurring flavonoid found in Godmania, Tridax, and Primula species that confers protection against high-fat diet (HFD) induced metabolic pathologies selectively in female mice. We have previously reported that this metabolic protection is associated with early and stable remodeling of the intestinal microbiome, evident in female but not male DHF-supplemented mice. Early changes in the gut microbiome in female DHF-fed mice were highly predictive of subsequent metabolic protection, suggesting a causative association between the gut microbiome and the metabolic effects of DHF.
Objective: To investigate a causal association between the gut microbiome and the metabolic effects of DHF using a model of antibiotic-induced gut microbiome ablation.
Materials and methods: Age-matched male and female C57Bl6/J mice were given ad libitum access to HFD and drinking water containing vehicle or DHF for 12 weeks. For antibiotic (Abx) treatment, female mice were given drinking water containing a cocktail of antibiotics for 2 weeks prior to HFD feeding and throughout the feeding period. Metabolic phenotyping consisted of longitudinal assessments of body weights, body composition, food, and water intake, as well as measurement of energy expenditure, glucose tolerance, and plasma and hepatic lipids. Protein markers mediating the cellular effects of DHF were assessed in brown adipose tissue (BAT) and skeletal muscle.
Results: Metabolic protection conferred by DHF in female HFD-fed mice was only apparent in the presence of an intact gut microbiome. Abx-treated mice were not protected from HFD-induced obesity by DHF administration. Further, tissue activation of the tropomyosin-related kinase receptor B (TrkB) receptor, which has been attributed to the biological activity of DHF, was lost upon gut microbiome ablation, indicating a requirement for microbial “activation” of DHF for its systemic effects. In addition, we report for the first time that DHF supplementation significantly activates TrkB in BAT of female, but not male, mice uncovering a novel target tissue of DHF. DHF supplementation also increased uncoupling protein 1 (UCP1) and AMP-activated protein kinase (AMPK) protein in BAT, consistent with protection from diet-induced obesity.
Conclusion: These results establish for the first time a requirement for the gut microbiome in mediating the metabolic effects of DHF in female mice and uncover a novel target tissue that may mediate these sexually-dimorphic protective effects.
Dietary flavonoids are non-nutritive bioactive compounds associated with reduced risk for developing numerous pathologies, including obesity, and associated chronic diseases, such as cardiovascular disease, type 2 diabetes, and gastrointestinal disorders (1–4). Flavonoids are poorly absorbed in the upper GI tract, and thus spend a considerable amount of time in the lower GI tract and colon, where their interactions with and biotransformation by gut microbiota (5, 6) are thought to mediate some of their subsequent systemic effects (7).
The composition and diversity of the gut microbiome is influenced by diverse factors such as diet, age, antibiotic use, birth mode, and exercise (8–14). Recent human and animal studies have also highlighted biological sex as a modulator of the gut microbiome and thus as a significant factor influencing disease progression (15–24). For example, sexual dimorphism in the intestinal microbiome appears at the onset of puberty and is thus thought to be driven by the influence of the sex hormones (25, 26). Sexually divergent changes in the gut microbiome have been proposed to predispose males to development of metabolic diseases including diabetes, cardiovascular diseases while conferring protection in pre-menopausal females (27–30). These host–microbiome interactions are thus critical factors modulating metabolic health, but our understanding of how particular dietary components interact with the gut microbiome in the context of biological sex, thereby influencing sexual dimorphism in disease development, is limited.
7,8-Dihydroxyflavone (DHF), is a naturally occurring flavonoid found in several plants, including Tridax procumbens, Godmania aesculifolia, and Primula tree leaves (31–33). DHF is a mimetic of brain-derived neurotrophic factor (BDNF), and like BDNF, it exerts its cellular actions through activation of tropomyosin-related kinase receptor B (TrkB) (32, 34). DHF supplementation has been explored as a therapeutic strategy against neurodegeneration, cognitive decline, and depression (32, 35–37). Recently, we and others have shown that oral DHF supplementation can also protect against high-fat diet (HFD) induced obesity, adiposity, and glucose intolerance (38, 39). Interestingly, this protection was only observed in female but not in male mice, where DHF paradoxically exacerbated lipid accumulation and adipose tissue inflammation. Metabolic protection in female mice was associated with early and stable changes in the composition of the gut microbiome that were highly predictive of subsequent protection against weight gain in female mice.
Given the association between gut microbial changes and body weight, we explored the question of whether the gut microbiome may play a causal role in the metabolic effects attributed to oral DHF. Using a model of antibiotic-mediated gut microbiome ablation, we report here that an intact intestinal microbiome is required to mediate both the cellular signaling and metabolic protection elicited by oral DHF in female mice.
Age-matched male and female C57Bl6/J mice were used in all studies. 13-week old mice were given ad libitum access to a HFD (Research Diets D12451; 45% kcals fat, 20% kcals protein, 35% kcals carbohydrate; 5.8% fiber; 4.7 kcal/g metabolizable energy) and drinking water containing vehicle or 1 mg/mL DHF for 12-weeks. Water preparation for the conventional non-antibiotic treated group was as reported previously (38). For antibiotic (Abx) treatment, 11-week old female mice were given drinking water containing a cocktail of Abx including ampicillin (1 g/L), neomycin (1 g/L), metronidazole (1 g/L) (all from Sigma, St. Louis, MO, United States) and vancomycin (VWR Radnor, PA, United States) (0.5 g/L) in 1% sucrose and 0.17% DMSO in PBS for 2 weeks prior to HFD feeding. Bacterial ablation was confirmed as described below. Following this, mice were randomly assigned to Abx-control or Abx-DHF groups and received Abx throughout the 12-week HFD-feeding.
Body weights and food intake were measured weekly, and water intake was measured twice a week (38). Body composition was determined by NMR (Echo MRI, Columbus, OH, United States), at the start and end of HFD-feeding (38). The breeding and care of the animals were in accordance with the protocols approved by the Animal Care and Use Committee of Rutgers University, New Brunswick, New Jersey. For in vivo procedures, all efforts were made to minimize discomfort and suffering, in accordance with approved animal care protocols. Following 12 weeks of feeding, mice were euthanized by isoflurane overdose, followed by cardiac exsanguination. Tissues were rapidly collected, snap-frozen in liquid nitrogen, and stored at −80°C until further analyses.
Fresh fecal pellets were collected before and after 2 weeks of Abx-treatment and after 1, 6, and 12 weeks of HFD-feeding and stored at −80°C until further analysis. Fecal bacterial DNA was isolated using QIAamp DNA stool mini kit (QIAGEN, Germantown, MD, United States) following manufacturer’s instructions. Total fecal 16S rRNA was quantified by qPCR using forward primer 785F: 5′-GGA TTA GAT ACC CTG GTA and reverse primer 907R: 5′-CCG TCA ATT CCT TTR AGT TT, spanning the V5 region of 16S rRNA gene (40). A 16S rRNA plasmid DNA with known copy number was used to generate a standard curve to quantify bacterial DNA in samples, as previously described (40).
Energy expenditure was measured by indirect calorimetry (Oxymax, Columbus Instruments, Columbus, OH) after 8 weeks of feeding, as previously described (41). Briefly, oxygen consumption (VO2) and carbon dioxide production (VCO2) were determined in individually housed animals with ad libitum access to HFD and water; both DHF and Abx were maintained in drinking water of respective groups during the calorimetry studies. Animals were acclimatized to Oxymax chambers for 24 h, and VO2 and VCO2 were recorded for eight consecutive 12-h light and 12-h dark cycles. Metabolic variables were recorded every minute, with a room air reference taken following each cycle of measurements.
Oral glucose tolerance test (GTT) was performed after 10 weeks of HFD-feeding. Briefly, mice were fasted for 4 h, followed by oral gavage of 20% dextrose at a dose of 1 g/kg body weight. Blood was collected from the tail at 0, 20, 40, 90, and 120 min for assessment of plasma glucose using an Accu-Chek glucometer (Accu-Chek Performa, Roche Diagnostics, IN, United States).
Plasma free cholesterol was measured using the Wako Free Cholesterol E Microtiter kit, as per manufacturer’s instructions (FUJIFILM Healthcare, Lexington, MA, United States). Hepatic lipids were extracted by a modified Folch method and separated by thin-layer chromatography (TLC), as previously described (42). Briefly, 20 mg liver tissue was homogenized in chloroform:methanol (2:1), followed by phase separation by the addition of acidified saline and centrifugation. The organic phase was collected, dried under a nitrogen stream, reconstituted in chloroform:methanol (2:1), spotted onto Silica Gel 60 chromatography plates, and developed in heptane:isopropyl ether:acetic acid (60:40:3) until the solvent front reached 1 cm from the top of the plate. Plates were dried, dipped in 10% copper sulfate in 10% phosphoric acid, and charred at 110°C for 30 min.
Protein samples were prepared by homogenizing tissues in HEPES lysis buffer (50 mM pH 7.4 HEPES, 150 mM NaCl, 10 mM Na-pyrophosphate, 2 mM EDTA, 1% NP-40, 10% Glycerol) containing 1x protease and phosphatase inhibitor cocktail (Sigma-Aldrich). Equal amounts of protein were separated by SDS-PAGE, transferred on PVDF membranes, and immunoblotted with primary antibodies. Primary antibodies including PGC-1α (Novus Biologicals, Littleton, CO, United States), VDAC (Pierce Biotechnology, Waltham, MA, United States), UCP1, COX-IV (Abcam, Cambridge, United Kingdom), and Total TrkB, P-TrkB, PAMPK, Total AMPK, HSP60, SIRT1, and GAPDH (all from Cell Signaling Technology, Danvers, MA, United States) were used at manufacturer recommended dilutions. Following incubation with HRP- or near-IR dye-conjugated secondary antibodies, membranes were visualized using enhanced chemiluminescence or near-IR imaging on an Azure c600 imaging system (Azure Biosystems, United States) and quantified using ImageStudio Lite.
All data are expressed as mean ± SEM. Statistical analysis are carried out using student’s t- test for two group comparisons or, for multi-group comparisons using one-way ANOVA, followed by post hoc analysis (Bonferroni) in Graph Pad Prism (version 9.3.1 for Windows, GraphPad Software, La Jolla, CA, United States). p-values < 0.05 were considered significant.
We previously reported that mice supplemented with oral DHF for 12 weeks accumulated differential amounts of body weight and fat mass on a high-fat diet (HFD), dependent on sex. Female mice gained significantly less weight and adiposity when supplemented with oral DHF, but male mice were resistant to this metabolic protection afforded by DHF supplementation. We also reported that DHF-induced early and stable remodeling of the gut microbiome in female but not male mice, corresponding with metabolic protection or lack thereof, upon DHF supplementation (38).
To examine a causal role for the gut microbiome in mediating the metabolic effects of DHF in females, we generated an experimental model of gut microbiome ablation by treatment with a cocktail of broad-spectrum antibiotics (Abx) for 2 weeks prior to DHF supplementation and HFD-feeding. Similar protocols have been previously reported to stably and consistently ablate the gut microbial community (43–46). Germ-free mice were not utilized in order to avoid the developmental and immune defects associated with this model (47). Two weeks of Abx-treatment did not result in any body weight changes. Gut microbiome ablation was confirmed by comparing fecal bacterial load at baseline (Week-2) and after 2 weeks of Abx-treatment (Week 0) to a standard curve generated with a 16S rRNA plasmid DNA with known copy number (40). Oral Abx-supplementation decreased the fecal 16S rRNA copy number by >3 log10 fold, with similar reductions maintained throughout the course of the study.
Following this, all conventionally raised i.e., which did not receive Abx-treatment and Abx-treated mice received HFD and were segregated into groups receiving vehicle or DHF, with or without oral Abx, for an additional 12 weeks. As we have previously reported (38), DHF treatment reduced weight gain in female mice that were conventionally raised (Figure 1A). Abx-treatment alone has been demonstrated to attenuate weight gain (48–52), independent of changes in food and water intake and solely dependent on absence of gut microbiome. However, Abx-treatment alone did not attenuate HFD-induced weight gain to the same extent as DHF supplementation (Figure 1A). Furthermore, DHF treatment in Abx-treated animals did not have any further impact on body weights (Figure 1A). Similarly, DHF treatment reduced fat mass in conventional mice but had no effect on fat or lean mass in Abx-treated animals (Figures 1B,C).
Figure 1. Gut microbiome ablation prevents improvement in metabolism by DHF. (A–C) Conventionally raised and Abx-treated females were maintained on a high-fat diet (HFD), supplemented with or without oral 7,8-DHF (1 mg/mL) for 12 weeks. Body weights were measured weekly, and initial and terminal body compositions were measured by NMR. (D,E) Food and water intake were measured weekly and biweekly, respectively. (F) Cecum weights were measured at the end of 12 weeks. Data were analyzed by one way ANOVA followed by Bonferroni post hoc comparison. Groups with different letters are significantly different from each other. *p < 0.05 for Control vs. DHF. Data are expressed as average ± SEM (n = 8–9).
Food intake was similar across all groups throughout the treatment period (Figure 1D). A significant reduction in water intake was observed with Abx-treatment (Figure 1E), which may be attributable to taste aversion due to antibiotics. This resulted in a daily DHF intake of 0.26 ± 0.01 mg/d/g BW in conventional females and 0.16 ± 0.01 mg/d/g BW in Abx-treated animals. It is important to note that the lower daily dose was still over 2-3-fold higher than a previously reported low-dose of DHF that conferred protection against weight gain in female mice (37, 51). Thus, the lack of protection from weight gain in the Abx-DHF group is not attributable to reduced DHF intake but rather to the concurrent Abx administration. These data indicate that reductions in HFD-induced weight gain due to oral DHF require an intact gut microbiome.
Gut microbiome ablation has been previously reported to significantly increase cecal weights (52–54). These results were recapitulated in the current study, and DHF did not induce any additional effects on cecal weights (Figure 1F).
Plasma cholesterol was significantly reduced by DHF in the conventional group (Figure 2A). Abx-treatment alone did not reduce plasma cholesterol levels. Importantly, DHF treatment in the Abx-group had no impact on plasma cholesterol, unlike in the conventional group (Figure 2A). Hepatic lipid accumulation was measured by thin-layer chromatography and revealed a significant reduction in levels of diacylglycerols (DAG), triacylglycerols (TAG), and cholesterol esters (CE) in DHF-treated females (Figures 2B,C). However, Abx-DHF animals had no significant reductions in hepatic lipids, relative to Abx-controls. Taken together, these data indicate that reductions in plasma and hepatic lipids by DHF administration require an intact gut microbiome.
Figure 2. Gut microbiome ablation prevents improvement in lipid metabolism by DHF. (A) Plasma free cholesterol was measured at the end of 12 weeks of HFD-feeding in conventional and microbiome-ablated groups. Data are expressed as average ± SEM (n = 8–9). (B) Hepatic lipids were separated by thin layer chromatography (TLC). CE, cholesterol esters; DAG, diacylglycerols; FFA, free fatty acids; PL, phospholipids; TG, triglycerides. (C) Hepatic lipid quantification was done by ImageJ software. Data were analyzed by one-way ANOVA followed by Bonferroni post hoc comparison. p-values < 0.05 were considered significant. Groups with different letters are significantly different from each other. Data are expressed as average ± SEM (n = 3).
To examine the mechanisms of protection from HFD-induced obesity in DHF-fed females, energy expenditure was measured by indirect calorimetry after 8 weeks of HFD-feeding. Significant increases in O2 consumption and CO2 respiration were observed in conventionally-raised female DHF-fed mice (Figures 3A,B). Similar increases were not apparent in Abx-treated DHF-fed females (Figures 3C,D). Similarly, regression of energy expenditure against body weight indicated a steeper regression slope in DHF-fed females, relative to control females (Figure 3E; slope: 0.0070 for Control vs. 0.0179 for DHF females) in the conventionally-raised mice, although this difference was not statistically significant. However, this same trend was not observed for DHF-fed females following Abx-treatment (Figure 3F; slope: 0.0011 for Abx-Control vs. 0.0035 for Abx-DHF). We interpret these data to collectively indicate a modest increase in energy expenditure upon DHF supplementation, which is lost in Abx-treated mice. These data are consistent with the protection from weight gain observed in DHF-fed females and lack of protection upon Abx-treatment (Figure 1A) and point to an important role for the gut microbiome in mediating DHF-induced alterations in energy balance.
Figure 3. Effect of DHF on energy expenditure and glucose tolerance in conventional and gut microbiome ablated females. (A,C) O2 consumption, (B,D) CO2 respiration, and (E,F) average cumulative energy expenditure were measured via indirect calorimetry over a 4-day period after 8 weeks of HFD-feeding. (G–I) Glucose tolerance was measured and area under the curve (AUC) was calculated after 10 weeks of HFD-feeding. Data are expressed as average ± SEM (n = 8–9). *p < 0.05 vs. sex-matched controls. Statistical analysis for indirect calorimetry data (A–F) was performed by analysis of covariance using web-based analysis tool CalR. Data in panels (G–I) were analyzed by one-way ANOVA followed by Bonferroni post hoc comparison. Significant differences are identified by * or different letters.
Consistent with obesity resistance, DHF supplementation of conventional females ameliorated glucose tolerance relative to control females (Figure 3G). Abx-treatment resulted in lower glucose area under the curve (AUC) relative to conventional controls, possibly due to the reduced weight gain in these mice. However, DHF treatment did not improve glucose tolerance in Abx-treated mice, consistent with body weight changes and further implicating a role for the gut microbiome in mediating the improvements in glucose tolerance upon DHF supplementation (Figures 3H,I).
7,8-dihydroxyflavone is an activator of tropomyosin-related kinase receptor B (TrkB) which is activated by phosphorylation at discrete tyrosine residues. We measured activation of TrkB by DHF in both male and female mice (32, 34). A previous study reported that oral DHF increased skeletal TrkB phosphorylation in female mice, without any report on the effects of DHF in males (39). Apart from the brain, peripheral expression of TrkB was reported to be greatest in brown and white adipose tissues, with very low expression reported in liver or skeletal muscle (53). Since brown adipose tissue (BAT) plays a critical role in maintaining energy homeostasis, we focused our current investigations on this tissue. Given the prior report on the role of DHF in activating energy expenditure in skeletal muscle, we also investigated the impact of Abx and DHF in gastrocnemius tissue.
In BAT, we observed that DHF induced TrkB-phosphorylation in female, but not male mice (Figures 4A,B). Further, phosphorylation and activation of AMP-activated protein kinase (AMPK), one of the downstream targets of TrkB, was also elevated in female but not male mice, consistent with sexually-dimorphic TrkB activation in these tissues (Figures 4A,B). In addition, expression of deacetylase, Sirtuin 1 (SIRT1), and its downstream target Peroxisome proliferator activated receptor-gamma coactivator (PGC-1a), were also increased in BAT of DHF-fed female, but not male mice, indicating increased BAT mitochondrial content and metabolism in these animals (Figure 4C,D). Consistent with activation of SIRT1-PGC-1a signaling, DHF supplementation also significantly increased expression of uncoupling protein-1 (UCP1) in BAT of female but not male mice (Figures 4C,D). Given the role of PGC-1α in modulating mitochondrial mass, we measured expression of mitochondrial content markers including COX-IV, VDAC and HSP60. No differences in mitochondrial content were noted (Figures 4C,D).
Figure 4. DHF activates TrkB and UCP1 in BAT of females. (A,B) Expression and quantification of TrkB and AMPK activation in BAT. (C,D) Expression and quantification of mitochondrial content markers in brown adipose tissue (BAT). Statistical analyses were carried out using student’s t-test. p-values < 0.05 were considered significant. Data are expressed as average ± SEM (n = 5).
Similar to BAT, activation of TrkB was significantly increased in gastrocnemius of female, but not male, DHF-fed mice (Figures 5A,B). While AMPK phosphorylation was significantly elevated in BAT of female DHF-fed mice, only a trend for elevation was observed in female gastrocnemius (Figure 5B). Expression of PGC-1α and SIRT1 were also slightly elevated in gastrocnemius of female mice, but again not to the same extent as observed in BAT (Figure 5D). Since a previous study reported a role for DHF in activating UCP1 in skeletal muscle (39), we measured expression of UCP1 in gastrocnemius, despite its low expression in this tissue. We observed a slight but significant increase in UCP1 expression in gastrocnemius of DHF-fed female mice, but not male mice (Figures 5C,D). Again, this 1.5-fold elevation of UCP1 protein expression in gastrocnemius was not as great as the 3-fold elevation observed in BAT, where the role of UCP1 in modulating energy homeostasis is more clearly established. Similar to BAT, no significant changes in mitochondrial content were evident in gastrocnemius of male or female DHF-fed mice (Figures 5C,D).
Figure 5. 7,8-DHF activates TrkB and UCP1 in gastrocnemius muscle of females. (A,B) Expression and quantification of TrkB and AMPK activation in gastrocnemius muscle. (C,D) Expression and quantification of mitochondrial content markers in gastrocnemius muscle. Statistical analyses were carried out using student’s t-test. p-values < 0.05 were considered significant. Data are expressed as average ± SEM (n = 5). *p < 0.05 vs. sex-and treatment-matched controls.
Taken together, these data indicate clear increases in TrkB activation, UCP1 induction, and activation of the SIRT1-PGC-1α -AMPK axis in BAT and skeletal muscle of DHF-fed female but not male mice, consistent with the sexually-dimorphic effects of DHF on energy homeostasis and consequently on HFD-induced weight gain.
We next examined these markers in Abx-treated females. Interestingly, upon Abx-treatment, no induction of any of these markers was apparent in either BAT (Figures 6A,B) or gastrocnemius (Figures 7A,B) of female mice. Phosphorylation of TrkB and AMPK, and expression of UCP1, SIRT1, and PGC-1a were static across control and DHF-treated mice upon Abx-treatment. These data clearly indicate, for the first time, that an intact gut microbiome is necessary for induction of TrkB as well as for downstream cellular signaling by DHF.
Figure 6. Gut microbiome ablation prevents induction of UCP1 and TrkB activation by DHF in BAT of females. (A) Expression and quantification of TrkB and AMPK activation in BAT. (B) Expression and quantification of mitochondrial content markers in brown adipose tissue (BAT). Statistical analyses were carried out using student’s t-test. p-values < 0.05 were considered significant. Data are expressed as average ± SEM (n = 5).
Figure 7. Gut microbiome ablation prevents UCP1 and TrkB activation by DHF in gastrocnemius tissue of females. (A) Expression and quantification of TrkB and AMPK activation in gastrocnemius tissue. (B) Expression and quantification of mitochondrial content markers in gastrocnemius tissue. Statistical analyses were carried out using student’s t-test. p-values < 0.05 were considered significant. Data are expressed as average ± SEM (n = 5). * p < 0.05 vs. sex-and treatment-matched controls.
7,8-dihydroxyflavone is a mimetic of brain-derived neurotrophic factor (BDNF) (31–33, 53–57). Like BDNF, DHF induces dimerization of the tropomyosin-related kinase receptor B (TrkB), followed by activation of downstream signaling molecules, including AKT and ERK (31–33, 39, 53–55, 57–60). However, compared to BDNF, DHF has a much longer half-life of approximately 4-8 h in vivo in a primate model (56), compared to just 10 minutes for BDNF (61). This, combined with its shelf-stability and smaller molecular size, make DHF an attractive candidate for clinical applications and oral therapies (31–33, 56, 59, 61–63). Given its actions as a TrkB agonist, many studies regarding DHF have investigated its potential central effects and demonstrated promising results for its use as an antidepressant and neuroprotective factor (34, 36, 58, 64–66) despite known peripheral expression of TrkB receptors in metabolically active tissues (67–69). Furthermore, the prior literature regarding the use of DHF for attenuation of depression and cognitive decline has been carried out almost exclusively in male animals (34, 36, 58, 64–66).
Dietary flavonoids represent a diverse range of polyphenolic compounds that are found in many commonly consumed fruits and vegetables, as well as herbs and grains. Growing evidence suggests that these non-nutritive bioactive components of our diets impart important protective effects against a diverse range of pathologies, including cardiometabolic diseases, specific cancers, and neurocognitive function (5, 70–72). A large proportion of ingested flavonoids are not absorbed in the small intestine but rather reach the colon, where their interaction with colonic bacteria can alter their absorption and excretion rates and give rise to various metabolites that modulate risk for obesity and metabolic disease (73–80). Although some limited reports have suggested sex-differences in flavonoid metabolism, such as via glucuronidation, sexually-dimorphic effects of flavonoids on health outcomes have not been systematically investigated (5, 70–72). The lack of such reports may simply stem from the routine reliance on male animals in most studies but serve to seriously limit our understanding of how males and females may respond differentially to therapies intended to modulate the intestinal microbiome. The intestinal microbiome is now understood to be an important determinant of body weight and metabolic health (5, 81–95). In this regard, it is increasingly appreciated that dietary flavonoids, by virtue of their low absorption in the small intestine and greater time spent in the colon, exert important effects on the host intestinal microbiome (5).
We and others have previously reported that oral DHF administration protects female but not male mice from HFD-induced obesity (38, 39). We also reported that paradoxically, DHF supplementation exacerbates some of the metabolic pathologies associated with HFD-feeding in male mice, including increasing hepatic lipid accumulation and adipose tissue inflammation (38). In our previous study, we discovered a novel role for DHF in modulating the gut microbiome of female, but not male, mice at an early time point, prior to any divergence in body weights between DHF-treated and control groups. These stable early changes significantly reduced the relative abundance of pathogenic Desulfovibrionaceae ASV_1 and Rikenella ASV_41 in females but not males (96–99). Desulfovibrionceae are sulfate reducing and lipopolysaccahride (LPS) producing bacteria promoting inflammation and obesity (100, 101). In addition, members of Rikenella are also associated with obesity and gut epithelial injury (96, 102). More importantly, these changes in the gut microbiome were highly predictive of subsequent protection from HFD-induced weight gain in female mice, while no such association was observed in male DHF-supplemented animals (38). Given these previous associations between the gut microbiome and the metabolic effects of oral DHF supplementation, the current study was designed to determine whether the gut microbiome is causally linked to the effects of DHF. Using a model of Abx-mediated microbiome ablation, we demonstrate here that an intact gut microbiome is required to mediate the body weight lowering effects of DHF in female mice. While DHF-supplementation reduced body weight and hepatic lipid accumulation in female mice, concurrent Abx-treatment abolished these favorable metabolic effects of DHF. Further, we identified that DHF supplementation induces TrkB activation, AMPK phosphorylation, and induction of SIRT1-PGC-1α and UCP1 in BAT of female mice. Notably, these cellular effects of DHF were absent in male mice, that were not protected from obesity by DHF supplementation. We also report that the effects of DHF supplementation are more apparent in BAT than in skeletal muscle, where more modest effects of DHF supplementation were observed. To our knowledge, these are the first reports of activation of TrkB signaling in BAT by DHF. Given the relatively higher expression of TrkB receptors in BAT than in skeletal muscle (53), we propose that the actions of DHF in this tissue play an important role in mediating the sexually-dimorphic metabolic effects of DHF. Further, we also demonstrate that DHF does not induce any changes in TrkB or associated signaling in BAT or muscle of Abx-treated animals. This lack of cellular changes after Abx-treatment is consistent with the lack of protection from weight gain in this cohort, and thus mechanistically links the gut microbiome to both TrkB signaling in BAT and muscle and the whole-body metabolic effects of DHF.
Peripheral expression of TrkB is highest in BAT in comparison to other metabolically active tissues like liver and skeletal muscle (53). DHF mimics the physiological functions of BDNF by binding and activating its receptor TrkB (53, 59, 60). Although BDNF is known to activate UCP1 and enhance thermogenesis in BAT (103–106), the impact of DHF on BAT was not previously known. Our studies indicate that in addition to TrkB activation, DHF supplementation also impacts AMPK phosphorylation and induction of UCP1, in a gut microbiome-dependent and sexually-dimorphic manner. Other studies have pointed to a potential role for flavonoids in increasing thermogenesis by activating AMPK and downstream mediators including SIRT1 and PGC-1α (107, 108). In our studies, sex- and microbiome-dependent activation of UCP1 expression was associated with improved metabolism in DHF-fed females, suggesting that increased thermogenesis may play a role in preventing obesity and associated metabolic diseases. These effects were observed in skeletal muscle, as well as, to a greater magnitude, in BAT. In this regard, it is important to note that BAT, and skeletal muscle are functionally linked (109) and originate from a common Myf5-expressing precursor cell, distinct from other adipose depots including visceral and subcutaneous fat depots (110). Our observations of sexually-dimorphic activation of TrkB signaling in BAT and gastrocnemius upon DHF supplementation highlight a role for these metabolically-active tissues in mediating the metabolic protection afforded by oral DHF, in a gut microbiome-dependent manner.
The metabolic effects of DHF were recently reported to be impacted by interactions between estrogen receptor-α (ERα) and the TrkB receptor in female mice (111). Specifically, DHF stimulated transactivation of ERα at Serine and Tyrosine residues, while TrkB signaling was reported to mediate ligand-independent activation of ERα. Gut microbiome-mediated alterations in ERα signaling may be an attractive area of future investigations into the mechanism driving the sexually-dimorphic effects of DHF on metabolism. In particular, estrogen has been shown to reduce the incidence of metabolic syndrome via alterations of the gut microbiome (112–114). Female and 17β-estradiol-treated male and ovariectomized mice were found to have gut microbiome profiles associated with a lower susceptibility to metabolic endotoxemia and low-grade chronic inflammation, and metabolic syndrome in response to an obesogenic western-diet in comparison to male and ovariectomized control mice. Further, fecal microbiota-transplants from control male mice to female mice transferred the metabolic syndrome phenotype to female mice, while Abx-treatment of males led to loss of the metabolic syndrome phenotype (114). Further, 17β-Estradiol supplementation has been shown to reduce incidence of colorectal cancer, due to alterations in gut microbiome (112). Together, the above findings may warrant exploration of a possible estrogen-mediated link between gut microbial changes elicited by oral DHF and its protective metabolic effects in female mice.
Understanding sexual dimorphism in both gut microbiome composition, as well as response to dietary polyphenols is of great translational relevance. For instance, clinical studies frequently report greater levels of weight loss in male subjects upon dietary and lifestyle interventions relative to female subjects (115–117). Indeed, similar results were observed in placebo-treated subjects in a recent report that examined the combined impact of probiotic therapy with caloric restriction on weight loss. However, upon supplementation with an oral probiotic, weight loss was significantly enhanced in female but not male subjects (118), lending support to the idea that the gut microbiome may differentially impact health in males vs. females. Further studies that examine sexually-dimorphic responses to gut microbiome manipulation will therefore be crucial for the development of personalized nutrition strategies for combating metabolic diseases.
The original contributions presented in this study are included in the article/supplementary material, further inquiries can be directed to the corresponding author.
The animal study was reviewed and approved by Animal Care and Use Committee of Rutgers University, New Brunswick, NJ, United States.
PS and HS conceptualized and designed the experiment and conducted data analysis. PS, CS, SP, and HY conducted the animal experiments. PS, SP, and HS prepared the manuscript. HS acquired funding and supervised the study. All authors have read and approved the final version of the manuscript.
This work was supported by NIH grant DK100640 and a NIH-Rutgers Botanical Dietary Supplements Research Center (BDSRC) Pilot grant, both to HS and Rutgers Center for Lipid Research Pilot funding to PS.
We thank Martin Blaser for providing 16S rRNA plasmid for quantification of fecal bacterial load, members of the Sampath Lab for helpful discussions, and Ms. Nivi D’Souza for technical assistance.
The authors declare that the research was conducted in the absence of any commercial or financial relationships that could be construed as a potential conflict of interest.
All claims expressed in this article are solely those of the authors and do not necessarily represent those of their affiliated organizations, or those of the publisher, the editors and the reviewers. Any product that may be evaluated in this article, or claim that may be made by its manufacturer, is not guaranteed or endorsed by the publisher.
1. Xu H, Luo J, Huang J, Wen Q. Flavonoids intake and risk of type 2 diabetes mellitus: a meta-analysis of prospective cohort studies. Medicine. (2018) 97:e0686. doi: 10.1097/MD.0000000000010686
2. Knekt P, Kumpulainen J, Järvinen R, Rissanen H, Heliövaara M, Reunanen A, et al. Flavonoid intake and risk of chronic diseases. Am J Clin Nutr. (2002) 76:560–8. doi: 10.1093/ajcn/76.3.560
3. Martin DA, Bolling BW. A review of the efficacy of dietary polyphenols in experimental models of inflammatory bowel diseases. Food Funct. (2015) 6:1773–86. doi: 10.1039/C5FO00202H
4. Pei R, Liu X, Bolling B. Flavonoids and gut health. Curr Opin Biotechnol. (2020) 61:153–9. doi: 10.1016/j.copbio.2019.12.018
5. Cassidy A, Minihane AM. The role of metabolism (and the microbiome) in defining the clinical efficacy of dietary flavonoids. Am J Clin Nutr. (2017) 105:10–22. doi: 10.3945/ajcn.116.136051
6. Kawabata K, Yoshioka Y, Terao J. Role of intestinal microbiota in the bioavailability and physiological functions of dietary polyphenols. Molecules. (2019) 24:370. doi: 10.3390/molecules24020370
7. Porras D, Nistal E, Martínez-Flórez S, Olcoz JL, Jover R, Jorquera F, et al. Functional interactions between gut microbiota transplantation, quercetin, and high-fat diet determine non-alcoholic fatty liver disease development in germ-free mice. Mol Nutr Food Res. (2019) 63:e1800930. doi: 10.1002/mnfr.201800930
8. Bokulich NA, Chung J, Battaglia T, Henderson N, Jay M, Li H, et al. Antibiotics, birth mode, and diet shape microbiome maturation during early life. Sci Transl Med. (2016) 8:343ra82. doi: 10.1126/scitranslmed.aad7121
9. Dominguez-Bello MG, Costello EK, Contreras M, Magris M, Hidalgo G, Fierer N, et al. Delivery mode shapes the acquisition and structure of the initial microbiota across multiple body habitats in newborns. Proc Natl Acad Sci USA. (2010) 107:11971–5. doi: 10.1073/pnas.1002601107
10. Leite G, Pimentel M, Barlow GM, Chang C, Hosseini A, Wang J, et al. Age and the aging process significantly alter the small bowel microbiome. Cell Rep. (2021) 36:109765. doi: 10.1016/j.celrep.2021.109765
11. Mika A, Van Treuren W, González A, Herrera JJ, Knight R, Fleshner M. Exercise is more effective at altering gut microbial composition and producing stable changes in lean mass in juvenile versus adult male F344 rats. PLoS One. (2015) 10:e0125889. doi: 10.1371/journal.pone.0125889
12. Simon H, Vartanian V, Wong MH, Nakabeppu Y, Sharma P, Lloyd RS, et al. OGG1 deficiency alters the intestinal microbiome and increases intestinal inflammation in a mouse model. PLoS One. (2020) 15:e0227501. doi: 10.1371/journal.pone.0227501
13. Hasan N, Yang H. Factors affecting the composition of the gut microbiota, and its modulation. PeerJ. (2019) 7:e7502. doi: 10.7717/peerj.7502
14. Wen L, Duffy A. Factors influencing the gut microbiota, inflammation, and type 2 diabetes. J Nutr. (2017) 147:1468s–75s. doi: 10.3945/jn.116.240754
15. Valeri F, Endres K. How biological sex of the host shapes its gut microbiota. Front Neuroendocrinol. (2021) 61:100912. doi: 10.1016/j.yfrne.2021.100912
16. Bridgewater LC, Zhang C, Wu Y, Hu W, Zhang Q, Wang J, et al. Gender-based differences in host behavior and gut microbiota composition in response to high fat diet and stress in a mouse model. Sci Rep. (2017) 7:10776. doi: 10.1038/s41598-017-11069-4
17. Fransen F, van Beek AA, Borghuis T, Meijer B, Hugenholtz F, van der Gaast-de Jongh C, et al. The impact of gut microbiota on gender-specific differences in immunity. Front Immunol. (2017) 8:754. doi: 10.3389/fimmu.2017.00754
18. Kim YS, Unno T, Kim BY, Park MS. Sex Differences in Gut Microbiota. World J Mens Health. (2020) 38:48–60. doi: 10.5534/wjmh.190009
19. Org E, Mehrabian M, Parks BW, Shipkova P, Liu X, Drake TA, et al. Sex differences and hormonal effects on gut microbiota composition in mice. Gut Microbes. (2016) 7:313–22. doi: 10.1080/19490976.2016.1203502
20. Bolnick DI, Snowberg LK, Hirsch PE, Lauber CL, Org E, Parks B, et al. Individual diet has sex-dependent effects on vertebrate gut microbiota. Nat Commun. (2014) 5:4500. doi: 10.1038/ncomms5500
21. Haro C, Rangel-Zúñiga OA, Alcalá-Díaz JF, Gómez-Delgado F, Pérez-Martínez P, Delgado-Lista J, et al. Intestinal microbiota is influenced by gender and body mass index. PLoS One. (2016) 11:e0154090. doi: 10.1371/journal.pone.0154090
22. de la Cuesta-Zuluaga J, Kelley ST, Chen Y, Escobar JS, Mueller NT, Ley RE, et al. Age- and sex-dependent patterns of gut microbial diversity in human adults. mSystems. (2019) 4:e00261–19. doi: 10.1128/mSystems.00261-19
23. Mueller S, Saunier K, Hanisch C, Norin E, Alm L, Midtvedt T, et al. Differences in fecal microbiota in different European study populations in relation to age, gender, and country: a cross-sectional study. Appl Environ Microbiol. (2006) 72:1027–33. doi: 10.1128/AEM.72.2.1027-1033.2006
24. Dominianni C, Sinha R, Goedert JJ, Pei Z, Yang L, Hayes RB, et al. Sex, body mass index, and dietary fiber intake influence the human gut microbiome. PLoS One. (2015) 10:e0124599. doi: 10.1371/journal.pone.0124599
25. Markle JG, Frank DN, Mortin-Toth S, Robertson CE, Feazel LM, Rolle-Kampczyk U, et al. Sex differences in the gut microbiome drive hormone-dependent regulation of autoimmunity. Science. (2013) 339:1084–8. doi: 10.1126/science.1233521
26. Yurkovetskiy L, Burrows M, Khan AA, Graham L, Volchkov P, Becker L, et al. Gender bias in autoimmunity is influenced by microbiota. Immunity. (2013) 39:400–12. doi: 10.1016/j.immuni.2013.08.013
27. Kautzky-Willer A, Harreiter J, Pacini G. Sex and Gender Differences in Risk, Pathophysiology and Complications of Type 2 Diabetes Mellitus. Endocr Rev. (2016) 37:278–316. doi: 10.1210/er.2015-1137
28. Gao A, Su J, Liu R, Zhao S, Li W, Xu X, et al. Sexual dimorphism in glucose metabolism is shaped by androgen-driven gut microbiome. Nat Commun. (2021) 12:7080. doi: 10.1038/s41467-021-27187-7
29. Ahmed S, Spence JD. Sex differences in the intestinal microbiome: interactions with risk factors for atherosclerosis and cardiovascular disease. Biol Sex Differ. (2021) 12:35. doi: 10.1186/s13293-021-00378-z
30. Cross TL, Kasahara K, Rey FE. Sexual dimorphism of cardiometabolic dysfunction: gut microbiome in the play? Mol Metab. (2018) 15:70–81. doi: 10.1016/j.molmet.2018.05.016
31. Du X, Hill RA. 7,8-Dihydroxyflavone as a pro-neurotrophic treatment for neurodevelopmental disorders. Neurochem Int. (2015) 89:170–80. doi: 10.1016/j.neuint.2015.07.021
32. Liu C, Chan CB, Ye K. 7,8-dihydroxyflavone, a small molecular TrkB agonist, is useful for treating various BDNF-implicated human disorders. Transl Neurodegener. (2016) 5:2. doi: 10.1186/s40035-015-0048-7
33. Wurzelmann M, Romeika J, Sun D. Therapeutic potential of brain-derived neurotrophic factor (BDNF) and a small molecular mimics of BDNF for traumatic brain injury. Neural Regen Res. (2017) 12:7–12. doi: 10.4103/1673-5374.198964
34. Jang SW, Liu X, Yepes M, Shepherd KR, Miller GW, Liu Y, et al. A selective TrkB agonist with potent neurotrophic activities by 7,8-dihydroxyflavone. Proc Natl Acad Sci USA. (2010) 107:2687–92. doi: 10.1073/pnas.0913572107
35. Tan Y, Nie S, Zhu W, Liu F, Guo H, Chu J, et al. 7,8-Dihydroxyflavone ameliorates cognitive impairment by inhibiting expression of tau pathology in ApoE-knockout mice. Front Aging Neurosci. (2016) 8:287. doi: 10.3389/fnagi.2016.00287
36. Zhang Z, Liu X, Schroeder JP, Chan CB, Song M, Yu SP, et al. 7,8-dihydroxyflavone prevents synaptic loss and memory deficits in a mouse model of Alzheimer’s disease. Neuropsychopharmacology. (2014) 39:638–50. doi: 10.1038/npp.2013.243
37. Zhang MW, Zhang SF, Li ZH, Han F. 7,8-Dihydroxyflavone reverses the depressive symptoms in mouse chronic mild stress. Neurosci Lett. (2016) 635:33–8. doi: 10.1016/j.neulet.2016.10.035
38. Sharma P, Wu G, Kumaraswamy D, Burchat N, Ye H, Gong Y, et al. Sex-dependent effects of 7,8-dihydroxyflavone on metabolic health are associated with alterations in the host gut microbiome. Nutrients. (2021) 13:637. doi: 10.3390/nu13020637
39. Chan CB, Tse MC, Liu X, Zhang S, Schmidt R, Otten R, et al. Activation of muscular TrkB by its small molecular agonist 7,8-dihydroxyflavone sex-dependently regulates energy metabolism in diet-induced obese mice. Chem Biol. (2015) 22:355–68. doi: 10.1016/j.chembiol.2015.02.003
40. Ruiz VE, Battaglia T, Kurtz ZD, Bijnens L, Ou A, Engstrand I, et al. A single early-in-life macrolide course has lasting effects on murine microbial network topology and immunity. Nat Commun. (2017) 8:518. doi: 10.1038/s41467-017-00531-6
41. Burchat N, Sharma P, Ye H, Komakula SSB, Dobrzyn A, Vartanian V, et al. Maternal transmission of human OGG1 protects mice against genetically- and diet-induced obesity through increased tissue mitochondrial content. Front Cell Dev Biol. (2021) 9:718962. doi: 10.3389/fcell.2021.718962
42. Sampath H, Flowers MT, Liu X, Paton CM, Sullivan R, Chu K, et al. Skin-specific deletion of stearoyl-CoA desaturase-1 alters skin lipid composition and protects mice from high fat diet-induced obesity. J Biol Chem. (2009) 284:19961–73. doi: 10.1074/jbc.M109.014225
43. Zou J, Chassaing B, Singh V, Pellizzon M, Ricci M, Fythe MD, et al. Fiber-mediated nourishment of gut microbiota protects against diet-induced obesity by restoring IL-22-mediated colonic health. Cell Host Microbe. (2018) 23:41–53.e4. doi: 10.1016/j.chom.2017.11.003
44. Baldridge MT, Nice TJ, McCune BT, Yokoyama CC, Kambal A, Wheadon M, et al. Commensal microbes and interferon-λ determine persistence of enteric murine norovirus infection. Science. (2015) 347:266–9. doi: 10.1126/science.1258025
45. Zeng SL, Li SZ, Xiao PT, Cai YY, Chu C, Chen BZ, et al. Citrus polymethoxyflavones attenuate metabolic syndrome by regulating gut microbiome and amino acid metabolism. Sci Adv. (2020) 6:eaax6208. doi: 10.1126/sciadv.aax6208
46. Ji Y, Sun S, Goodrich JK, Kim H, Poole AC, Duhamel GE, et al. Diet-induced alterations in gut microflora contribute to lethal pulmonary damage in TLR2/TLR4-deficient mice. Cell Rep. (2014) 8:137–49. doi: 10.1016/j.celrep.2014.05.040
47. Kennedy EA, King KY, Baldridge MT. Mouse microbiota models: comparing germ-free mice and antibiotics treatment as tools for modifying gut bacteria. Front Physiol. (2018) 9:1534. doi: 10.3389/fphys.2018.01534
48. Miao Z, Cheng R, Zhang Y, Liang H, Jiang F, Shen X, et al. Antibiotics can cause weight loss by impairing gut microbiota in mice and the potent benefits of lactobacilli. Biosci Biotechnol Biochem. (2020) 84:411–20. doi: 10.1080/09168451.2019.1676696
49. Yao H, Fan C, Lu Y, Fan X, Xia L, Li P, et al. Alteration of gut microbiota affects expression of adiponectin and resistin through modifying DNA methylation in high-fat diet-induced obese mice. Genes Nutr. (2020) 15:12. doi: 10.1186/s12263-020-00671-3
50. Bongers KS, McDonald RA, Winner KM, Falkowski NR, Brown CA, Baker JM, et al. Antibiotics cause metabolic changes in mice primarily through microbiome modulation rather than behavioral changes. PLoS One. (2022) 17:e0265023. doi: 10.1371/journal.pone.0265023
51. Goulding DR, Myers PH, Dickerson AB, Comins MM, Wiltshire RA, Blankenship-Paris TL. Comparative efficacy of two types of antibiotic mixtures in gut flora depletion in female C57BL/6 Mice. Comp Med. (2021) 71:203–9. doi: 10.30802/AALAS-CM-21-000023
52. Reikvam DH, Erofeev A, Sandvik A, Grcic V, Jahnsen FL, Gaustad P, et al. Depletion of murine intestinal microbiota: effects on gut mucosa and epithelial gene expression. PLoS One. (2011) 6:e17996. doi: 10.1371/journal.pone.0017996
53. Nakagomi A, Okada S, Yokoyama M, Yoshida Y, Shimizu I, Miki T, et al. Role of the central nervous system and adipose tissue BDNF/TrkB axes in metabolic regulation. NPJ Aging Mech Dis. (2015) 1:15009. doi: 10.1038/npjamd.2015.9
54. Briana DD, Malamitsi-Puchner A. Developmental origins of adult health and disease: the metabolic role of BDNF from early life to adulthood. Metabolism. (2018) 81:45–51. doi: 10.1016/j.metabol.2017.11.019
55. Genzer Y, Dadon M, Burg C, Chapnik N, Froy O. Effect of dietary fat and the circadian clock on the expression of brain-derived neurotrophic factor (BDNF). Mol Cell Endocrinol. (2016) 430:49–55. doi: 10.1016/j.mce.2016.04.015
56. He J, Xiang Z, Zhu X, Ai Z, Shen J, Huang T, et al. Neuroprotective effects of 7, 8-dihydroxyflavone on midbrain dopaminergic neurons in MPP(+)-treated monkeys. Sci Rep. (2016) 6:34339. doi: 10.1038/srep34339
57. Liao GY, Bouyer K, Kamitakahara A, Sahibzada N, Wang CH, Rutlin M, et al. Brain-derived neurotrophic factor is required for axonal growth of selective groups of neurons in the arcuate nucleus. Mol Metab. (2015) 4:471–82. doi: 10.1016/j.molmet.2015.03.003
58. Liu X, Qi Q, Xiao G, Li J, Luo HR, Ye K. O-methylated metabolite of 7,8-dihydroxyflavone activates TrkB receptor and displays antidepressant activity. Pharmacology. (2013) 91:185–200. doi: 10.1159/000346920
59. Stagni F, Giacomini A, Guidi S, Emili M, Uguagliati B, Salvalai ME, et al. A flavonoid agonist of the TrkB receptor for BDNF improves hippocampal neurogenesis and hippocampus-dependent memory in the Ts65Dn mouse model of DS. Exp Neurol. (2017) 298:79–96. doi: 10.1016/j.expneurol.2017.08.018
60. Gao L, Tian M, Zhao HY, Xu QQ, Huang YM, Si QC, et al. TrkB activation by 7, 8-dihydroxyflavone increases synapse AMPA subunits and ameliorates spatial memory deficits in a mouse model of Alzheimer’s disease. J Neurochem. (2016) 136:620–36. doi: 10.1111/jnc.13432
61. Sakane T, Pardridge WM. Carboxyl-directed pegylation of brain-derived neurotrophic factor markedly reduces systemic clearance with minimal loss of biologic activity. Pharm Res. (1997) 14:1085–91.
62. Wang X, Romine JL, Gao X, Chen J. Aging impairs dendrite morphogenesis of newborn neurons and is rescued by 7, 8-dihydroxyflavone. Aging Cell. (2017) 16:304–11. doi: 10.1111/acel.12553
63. Zeng Y, Wang X, Wang Q, Liu S, Hu X, McClintock SM. Small molecules activating TrkB receptor for treating a variety of CNS disorders. CNS Neurol Disord Drug Targets. (2013) 12:1066–77. doi: 10.2174/18715273113129990089
64. Liu X, Chan CB, Qi Q, Xiao G, Luo HR, He X, et al. Optimization of a small tropomyosin-related kinase B (TrkB) agonist 7,8-dihydroxyflavone active in mouse models of depression. J Med Chem. (2012) 55:8524–37. doi: 10.1021/jm301099x
65. Agrawal R, Noble E, Tyagi E, Zhuang Y, Ying Z, Gomez-Pinilla F. Flavonoid derivative 7,8-DHF attenuates TBI pathology via TrkB activation. Biochim Biophys Acta. (2015) 1852:862–72. doi: 10.1016/j.bbadis.2015.01.018
66. Jiang M, Peng Q, Liu X, Jin J, Hou Z, Zhang J, et al. Small-molecule TrkB receptor agonists improve motor function and extend survival in a mouse model of Huntington’s disease. Hum Mol Genet. (2013) 22:2462–70. doi: 10.1093/hmg/ddt098
67. García-Suárez O, González-Martínez T, Perez-Perez M, Germana A, Blanco-Gélaz MA, Monjil DF, et al. Expression of the neurotrophin receptor TrkB in the mouse liver. Anat Embryol. (2006) 211:465–73. doi: 10.1007/s00429-006-0098-9
68. Personius KE, Parker SD. TrkB expression at the neuromuscular junction is reduced during aging. Muscle Nerve. (2013) 47:532–8. doi: 10.1002/mus.23616
69. Shibayama E, Koizumi H. Cellular localization of the Trk neurotrophin receptor family in human non-neuronal tissues. Am J Pathol. (1996) 148:1807–18.
70. Fu ZD, Csanaky IL, Klaassen CD. Effects of aging on mRNA profiles for drug-metabolizing enzymes and transporters in livers of male and female mice. Drug Metab Dispos. (2012) 40:1216–25. doi: 10.1124/dmd.111.044461
71. Bolling BW, Court MH, Blumberg JB, Chen CY. The kinetic basis for age-associated changes in quercetin and genistein glucuronidation by rat liver microsomes. J Nutr Biochem. (2010) 21:498–503. doi: 10.1016/j.jnutbio.2009.02.006
72. Kawase A, Ito A, Yamada A, Iwaki M. Age-related changes in mRNA levels of hepatic transporters, cytochrome P450 and UDP-glucuronosyltransferase in female rats. Eur J Drug Metab Pharmacokinet. (2015) 40:239–44. doi: 10.1007/s13318-014-0208-7
73. Gil-Cardoso K, Ginés I, Pinent M, Ardévol A, Blay M, Terra X. Effects of flavonoids on intestinal inflammation, barrier integrity and changes in gut microbiota during diet-induced obesity. Nutr Res Rev. (2016) 29:234–48. doi: 10.1017/S0954422416000159
74. Jin T, Song Z, Weng J, Fantus IG. Curcumin and other dietary polyphenols: potential mechanisms of metabolic actions and therapy for diabetes and obesity. Am J Physiol Endocrinol Metab. (2018) 314:E201–5. doi: 10.1152/ajpendo.00285.2017
75. Pan MH, Wu JC, Ho CT, Lai CS. Antiobesity molecular mechanisms of action: resveratrol and pterostilbene. Biofactors. (2018) 44:50–60. doi: 10.1002/biof.1409
76. Springer M, Moco S. Resveratrol and its human metabolites-effects on metabolic health and obesity. Nutrients. (2019) 11:143. doi: 10.3390/nu11010143
77. Carrera-Quintanar L, López Roa RI, Quintero-Fabián S, Sánchez-Sánchez MA, Vizmanos B, Ortuño-Sahagún D. Phytochemicals that influence gut microbiota as prophylactics and for the treatment of obesity and inflammatory diseases. Mediators Inflamm. (2018) 2018:9734845. doi: 10.1155/2018/9734845
78. Kuhn P, Kalariya HM, Poulev A, Ribnicky DM, Jaja-Chimedza A, Roopchand DE, et al. Grape polyphenols reduce gut-localized reactive oxygen species associated with the development of metabolic syndrome in mice. PLoS One. (2018) 13:e0198716. doi: 10.1371/journal.pone.0198716
79. Cheng DM, Roopchand DE, Poulev A, Kuhn P, Armas I, Johnson WD, et al. High phenolics Rutgers Scarlet Lettuce improves glucose metabolism in high fat diet-induced obese mice. Mol Nutr Food Res. (2016) 60:2367–78. doi: 10.1002/mnfr.201600290
80. Graf BL, Zhang L, Corradini MG, Kuhn P, Newman SS, Salbaum JM, et al. Physicochemical differences between malanga (Xanthosoma sagittifolium) and potato (Solanum tuberosum) tubers are associated with differential effects on the gut microbiome. J Funct Foods. (2018) 45:268–76. doi: 10.1016/j.jff.2018.04.032
81. Lam YY, Zhang C, Zhao L. Causality in dietary interventions-building a case for gut microbiota. Genome Med. (2018) 10:62. doi: 10.1186/s13073-018-0573-y
82. Costea PI, Hildebrand F, Arumugam M, Bäckhed F, Blaser MJ, Bushman FD, et al. Enterotypes in the landscape of gut microbial community composition. Nat Microbiol. (2018) 3:8–16. doi: 10.1038/s41564-017-0072-8
83. Ursell LK, Haiser HJ, Van Treuren W, Garg N, Reddivari L, Vanamala J, et al. The intestinal metabolome: an intersection between microbiota and host. Gastroenterology. (2014) 146:1470–6. doi: 10.1053/j.gastro.2014.03.001
84. Caverly LJ, Carmody LA, Haig SJ, Kotlarz N, Kalikin LM, Raskin L, et al. Culture-independent identification of nontuberculous mycobacteria in cystic fibrosis respiratory samples. PLoS One. (2016) 11:e0153876. doi: 10.1371/journal.pone.0153876
85. Singh RK, Chang HW, Yan D, Lee KM, Ucmak D, Wong K, et al. Influence of diet on the gut microbiome and implications for human health. J Transl Med. (2017) 15:73. doi: 10.1186/s12967-017-1175-y
86. Carmody RN, Turnbaugh PJ. Host-microbial interactions in the metabolism of therapeutic and diet-derived xenobiotics. J Clin Invest. (2014) 124:4173–81. doi: 10.1172/JCI72335
87. Greenblum S, Turnbaugh PJ, Borenstein E. Metagenomic systems biology of the human gut microbiome reveals topological shifts associated with obesity and inflammatory bowel disease. Proc Natl Acad Sci USA. (2012) 109:594–9. doi: 10.1073/pnas.1116053109
88. Wang R, Li H, Yang X, Xue X, Deng L, Shen J, et al. Genetically obese human gut microbiota induces liver steatosis in germ-free mice fed on normal diet. Front Microbiol. (2018) 9:1602. doi: 10.3389/fmicb.2018.01602
89. Jumpertz R, Le DS, Turnbaugh PJ, Trinidad C, Bogardus C, Gordon JI, et al. Energy-balance studies reveal associations between gut microbes, caloric load, and nutrient absorption in humans. Am J Clin Nutr. (2011) 94:58–65. doi: 10.3945/ajcn.110.010132
90. Turnbaugh PJ. Microbes and diet-induced obesity: fast, cheap, and out of control. Cell Host Microbe. (2017) 21:278–81. doi: 10.1016/j.chom.2017.02.021
91. Cani PD, Jordan BF. Gut microbiota-mediated inflammation in obesity: a link with gastrointestinal cancer. Nat Rev Gastroenterol Hepatol. (2018) 15:671–82. doi: 10.1038/s41575-018-0025-6
92. Cani PD. Targeting gut microbiota with a complex mix of dietary fibers improves metabolic diseases. Kidney Int. (2019) 95:14–6. doi: 10.1016/j.kint.2018.11.012
93. Van Hul M, Cani PD. Targeting carbohydrates and polyphenols for a healthy microbiome and healthy weight. Curr Nutr Rep. (2019) 8:307–16. doi: 10.1007/s13668-019-00281-5
94. Stanhope KL, Goran MI, Bosy-Westphal A, King JC, Schmidt LA, Schwarz JM, et al. Pathways and mechanisms linking dietary components to cardiometabolic disease: thinking beyond calories. Obes Rev. (2018) 19:1205–35. doi: 10.1111/obr.12699
95. Chagwedera DN, Ang QY, Bisanz JE, Leong YA, Ganeshan K, Cai J, et al. Nutrient sensing in CD11c cells alters the gut microbiota to regulate food intake and body mass. Cell Metab. (2019) 30:364–73.e7. doi: 10.1016/j.cmet.2019.05.002
96. Crittenden S, Goepp M, Pollock J, Robb CT, Smyth DJ, Zhou Y, et al. Prostaglandin E(2) promotes intestinal inflammation via inhibiting microbiota-dependent regulatory T cells. Sci Adv. (2021) 7:eabd7954. doi: 10.1101/2020.07.12.199513
97. Palmas V, Pisanu S, Madau V, Casula E, Deledda A, Cusano R, et al. Gut microbiota markers associated with obesity and overweight in Italian adults. Sci Rep. (2021) 11:5532. doi: 10.1038/s41598-021-84928-w
98. Rowan F, Docherty NG, Murphy M, Murphy B, Calvin Coffey J, O’Connell PR. Desulfovibrio bacterial species are increased in ulcerative colitis. Dis Colon Rectum. (2010) 53:1530–6. doi: 10.1007/DCR.0b013e3181f1e620
99. Shen L, Liu L, Ji HF. Regulative effects of curcumin spice administration on gut microbiota and its pharmacological implications. Food Nutr Res. (2017) 61:1361780. doi: 10.1080/16546628.2017.1361780
100. Loubinoux J, Bronowicki JP, Pereira IA, Mougenel JL, Faou AE. Sulfate-reducing bacteria in human feces and their association with inflammatory bowel diseases. FEMS Microbiol Ecol. (2002) 40:107–12. doi: 10.1111/j.1574-6941.2002.tb00942.x
101. Petersen C, Bell R, Klag KA, Lee SH, Soto R, Ghazaryan A, et al. T cell-mediated regulation of the microbiota protects against obesity. Science. (2019) 365:eaat9351. doi: 10.1126/science.aat9351
102. Su H, Wang WJ, Zheng GD, Yin ZP, Li JE, Chen LL, et al. The anti-obesity and gut microbiota modulating effects of taxifolin in C57BL/6J mice fed with a high-fat diet. J Sci Food Agric. (2022) 102:1598–608. doi: 10.1002/jsfa.11496
103. Yamanaka M, Tsuchida A, Nakagawa T, Nonomura T, Ono-Kishino M, Sugaru E, et al. Brain-derived neurotrophic factor enhances glucose utilization in peripheral tissues of diabetic mice. Diabetes Obes Metab. (2007) 9:59–64. doi: 10.1111/j.1463-1326.2006.00572.x
104. Zhu Q, Liu X, Glazier BJ, Krolick KN, Yang S, He J, et al. Differential sympathetic activation of adipose tissues by brain-derived neurotrophic factor. Biomolecules. (2019) 9:452. doi: 10.3390/biom9090452
105. Tsuchida A, Nonomura T, Ono-Kishino M, Nakagawa T, Taiji M, Noguchi H. Acute effects of brain-derived neurotrophic factor on energy expenditure in obese diabetic mice. Int J Obes Relat Metab Disord. (2001) 25:1286–93. doi: 10.1038/sj.ijo.0801678
106. Wang C, Bomberg E, Billington C, Levine A, Kotz CM. Brain-derived neurotrophic factor in the hypothalamic paraventricular nucleus increases energy expenditure by elevating metabolic rate. Am J Physiol Regul Integr Comp Physiol. (2007) 293:R992–1002. doi: 10.1152/ajpregu.00516.2006
107. Kang HW, Lee SG, Otieno D, Ha K. Flavonoids, potential bioactive compounds, and non-shivering thermogenesis. Nutrients. (2018) 10:1168. doi: 10.3390/nu10091168
108. Mele L, Bidault G, Mena P, Crozier A, Brighenti F, Vidal-Puig A, et al. Dietary (Poly)phenols, brown adipose tissue activation, and energy expenditure: a narrative review. Adv Nutr. (2017) 8:694–704. doi: 10.3945/an.117.015792
109. Kong X, Yao T, Zhou P, Kazak L, Tenen D, Lyubetskaya A, et al. Brown adipose tissue controls skeletal muscle function via the secretion of Myostatin. Cell Metab. (2018) 28:631–43.e3. doi: 10.1016/j.cmet.2018.07.004
110. Seale P, Bjork B, Yang W, Kajimura S, Chin S, Kuang S, et al. PRDM16 controls a brown fat/skeletal muscle switch. Nature. (2008) 454:961–7. doi: 10.1038/nature07182
111. Zhao Z, Xue F, Gu Y, Han J, Jia Y, Ye K, et al. Crosstalk between the muscular estrogen receptor α and BDNF/TrkB signaling alleviates metabolic syndrome via 7,8-dihydroxyflavone in female mice. Mol Metab. (2021) 45:101149. doi: 10.1016/j.molmet.2020.101149
112. Song CH, Kim N, Nam RH, Choi SI, Lee HN, Surh YJ. 17β-Estradiol supplementation changes gut microbiota diversity in intact and colorectal cancer-induced ICR male mice. Sci Rep. (2020) 10:12283. doi: 10.1038/s41598-020-69112-w
113. Baker JM, Al-Nakkash L, Herbst-Kralovetz MM. Estrogen-gut microbiome axis: physiological and clinical implications. Maturitas. (2017) 103:45–53. doi: 10.1016/j.maturitas.2017.06.025
114. Kaliannan K, Robertson RC, Murphy K, Stanton C, Kang C, Wang B, et al. Estrogen-mediated gut microbiome alterations influence sexual dimorphism in metabolic syndrome in mice. Microbiome. (2018) 6:205. doi: 10.1186/s40168-018-0587-0
115. Doucet E, Imbeault P, Alméras N, Tremblay A. Physical activity and low-fat diet: is it enough to maintain weight stability in the reduced-obese individual following weight loss by drug therapy and energy restriction? Obes Res. (1999) 7:323–33. doi: 10.1002/j.1550-8528.1999.tb00415.x
116. Pérusse-Lachance E, Brassard P, Chaput JP, Drapeau V, Teasdale N, Sénécal C, et al. Sex differences in the effects of mental work and moderate-intensity physical activity on energy intake in young adults. ISRN Nutr. (2013) 2013:723250. doi: 10.5402/2013/723250
117. Tremblay A, Despres J-P, Leblanc C, Bouchard C. Sex dimorphism in fat loss in response to exercise-training. J Obes Weight Regul. (1984) 3:193–203.
Keywords: DHF, microbiome, TrkB, brown adipose tissue, sex effects
Citation: Sharma P, Silva C, Pfreundschuh S, Ye H and Sampath H (2022) Metabolic protection by the dietary flavonoid 7,8-dihydroxyflavone requires an intact gut microbiome. Front. Nutr. 9:987956. doi: 10.3389/fnut.2022.987956
Received: 06 July 2022; Accepted: 29 July 2022;
Published: 17 August 2022.
Edited by:
Anandh Babu Pon Velayutham, The University of Utah, United StatesReviewed by:
Hongwei Si, Tennessee State University, United StatesCopyright © 2022 Sharma, Silva, Pfreundschuh, Ye and Sampath. This is an open-access article distributed under the terms of the Creative Commons Attribution License (CC BY). The use, distribution or reproduction in other forums is permitted, provided the original author(s) and the copyright owner(s) are credited and that the original publication in this journal is cited, in accordance with accepted academic practice. No use, distribution or reproduction is permitted which does not comply with these terms.
*Correspondence: Harini Sampath, aGFyaW5pLnNhbXBhdGhAcnV0Z2Vycy5lZHU=
Disclaimer: All claims expressed in this article are solely those of the authors and do not necessarily represent those of their affiliated organizations, or those of the publisher, the editors and the reviewers. Any product that may be evaluated in this article or claim that may be made by its manufacturer is not guaranteed or endorsed by the publisher.
Research integrity at Frontiers
Learn more about the work of our research integrity team to safeguard the quality of each article we publish.