- 1Department of Soil and Food Science, University of Bari Aldo Moro, Bari, Italy
- 2Institute of Sciences of Food Production, National Research Council of Italy, Bari, Italy
- 3Neuroscience Institute, National Research Council of Italy, Padua, Italy
Propelled by an ever-growing awareness about the importance of following dietary recommendations meeting specific biological requirements linked to a person health status, interest in personalized nutrition is on the rise. Soilless biofortification of vegetables has opened the door to the potential for adapting vegetable production to specific dietary requirements. The evolution of vegetables biofortification toward tailored food is examined focusing on some specific categories of people in a context of personalized nutrition instead to simple describe developments in vegetables biofortification with reference to the single element or compound not adequately present in the daily diet. The concepts of bioavailability and bioaccessibility as a useful support tool for the precision biofortification were detailed. Key prospects for challenges ahead aiming to combine product quality and sustainable are also highlighted. Hydroponically cultivation of vegetables with low potassium content may be effective to obtain tailored leafy and fruit vegetable products for people with impaired kidney function. Simultaneous biofortification of calcium, silicon, and boron in the same vegetable to obtain vegetable products useful for bone health deserve further attention. The right dosage of the lithium in the nutrient solution appears essential to obtain tailored vegetables able to positively influence mental health in groups of people susceptible to mental illness. Modulate nitrogen fertilization may reduce or enhance nitrate in vegetables to obtain tailored products, respectively, for children and athletes. Future research are needed to produce nickel-free vegetable products for individuals sensitized to nickel. The multidisciplinary approach toward tailored foods is a winning one and must increasingly include a synergy between agronomic, biological, and medical skills.
Introduction
According to some investigators (1), Personalized Nutrition (PN) “tailors dietary recommendations to specific biological requirements on the basis of a person’s health status and goals.” PN could be also described as a “field that leverages human individuality to drive nutrition strategies that prevent, manage and treat disease and optimize health” (2). At the same time, the term “personalization” can be interpreted both as “individualization” and “categorization,” since the two meanings can indeed coexist. In fact, although individuals can be considered unique, some can be regarded as similar enough to make up a category (3).
Generalizable nutrition recommendations can guide public policy independently of one-on-one nutrition counseling sessions. For example, the well-known Mediterranean Diet (MD) is a dietary model characterized by a high intake of vegetables and fruits leading to a reduction in blood pressure, insulin resistance, and inflammatory markers thus contributing to healthy lifestyles and practically eliminating inadequate ones (4, 5).
Not only the MD, but many other dietary patterns for human health and wellbeing have highlighted the positive role of large quantities of fresh fruits and vegetables in the daily diet (6). Nevertheless, indicating generally to eat standard portions of fruits and vegetables results inadequate to address human individuality (2). Therefore, it is important that studies in this field identify specific dietary patterns producing the most favorable impacts on health and wellbeing in similar groups of people. For example, allergen-free diets, such as nickel-free one can be tailored for specific group of people (7, 8). Adopting these types of diets can nevertheless entail limiting or eliminating multiple food products, resulting in a decrease in the quality of life. Therefore, a diet that includes vegetables with a lower nickel content than their common counterpart could be very useful for people affected by nickel hypersensitivity, since it would avoid a decrease in the quality of life.
At the same time people affected by some diseases or with a health status requiring higher quantities of some nutrients with respect to standard Dietary Reference Values (DRV) need to follow specific dietary guidelines. For example, the malabsorption of folic acid is a common complication of celiac disease (9). Thus, the average daily intake of folate in celiac patients is often lower with respect to that in the general population (10). Therefore, eating vegetables high in folate content could help to supplement the diet and improve the health of celiac patients. Biofortification is usually defined as the practice of deliberately increasing or decreasing the content of an essential micronutrient (i.e., vitamins and minerals) in plants (11, 12). Independently of definitions, the primary aim of biofortification is to improve the nutritional quality of fruits and vegetables for a healthier diet. Biofortification differs from conventional fortification since it is applied to crops during their growth phase. Fortification, instead, refers to the practice of adding micronutrients to food products during the processing phase of food production (13). Biofortification is a process that can be applied to fresh, uncooked vegetables. Increasing research activities have been directed toward the biofortification of vegetables in the last few years (Figure 1). Moreover, some mineral-enriched vegetables are already present on the market (14, 15). But although biofortified vegetables are becoming more and more popular, there are only limited data regarding their potential role in personalized nutrition. In the light of these considerations, this review aims to provide up-to-date information regarding biofortified vegetables for specific categories of people whose health could be enhanced by personalized nutrition plans.
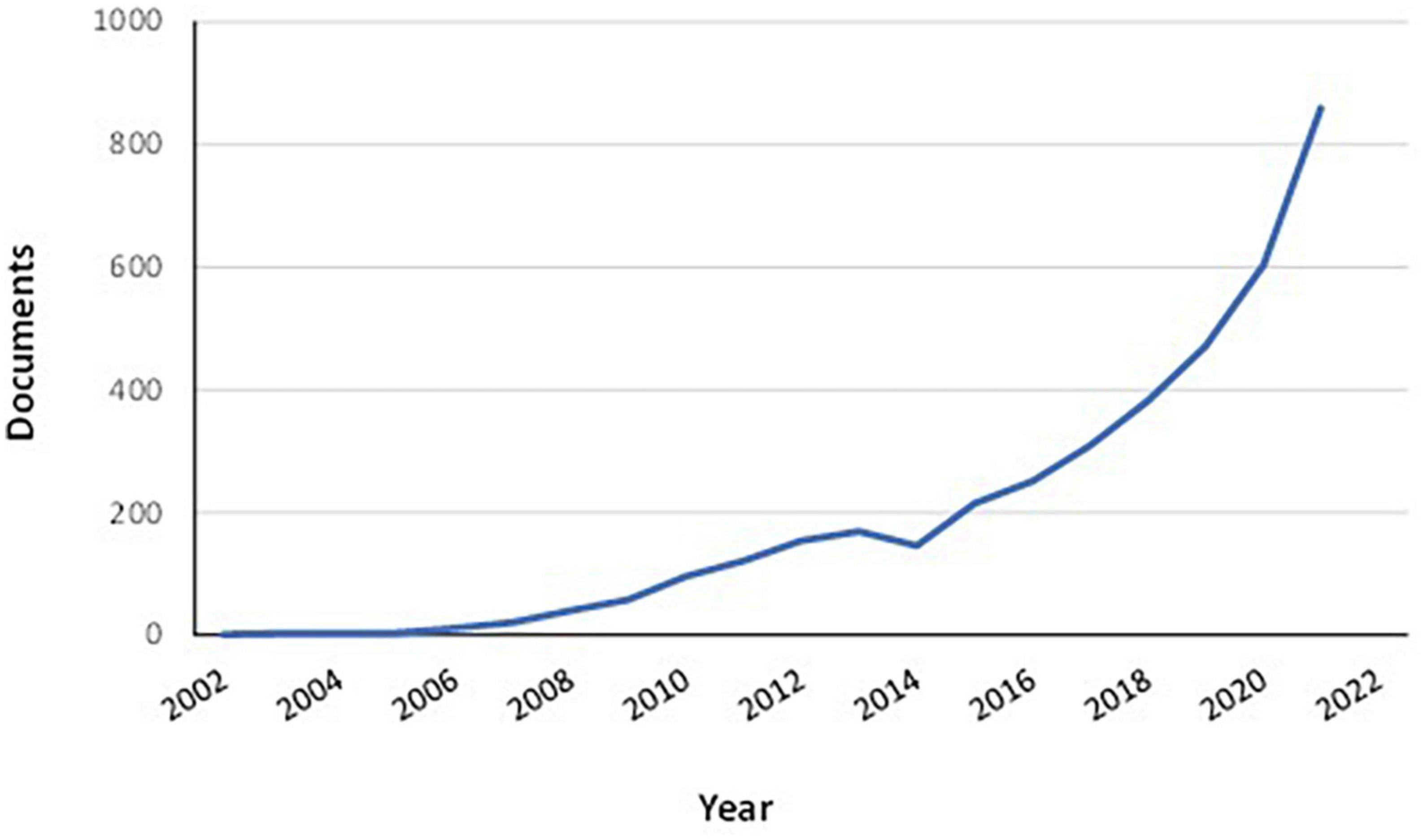
Figure 1. Documents regarding vegetable biofortification published from 2002 to 2021. Documents by type: article (69.3%); review (18.7%); book chapter (8.1%); conference paper (2.2%); book (1.0%); other (0.7%). Data retrieved from Scopus® database (on 15 February 2022) using “biofortification” AND “vegetables” as key search terms.
From vegetable biofortification to tailored food
Although biofortification strategies can include agronomic practices, conventional plant breeding and genetic engineering methods, the first is considered the most promising because it is the least expensive and requires only simple tools and techniques to modify the content of specific compounds in plants (16).
Agronomic practices can be considered the “starting point” of biofortification strategies in light of the fact as they were first used in the “Finland case.” In fact, since 1984 agricultural fertilizers in Finland have been supplemented with sodium selenate in an attempt to improve the nutritional quality of local foodstuffs known to be exceptionally low in selenium. This agronomic strategy affecting several crops and producing higher selenium concentrations in different food items has been proving effective since 1985, and, in fact, the selenium intake in the Finn population has increased significantly (17). We must nevertheless bear in mind that prolonged fertilization application using an enriched fertilizer may modify the soil chemical characteristics and may have a potentially negative environmental impact. It goes without saying that more agronomic practices for improving the soil’s health and the nutritional value of crops need to be identified.
When soilless cultivation systems are used, the soil is replaced by a substrate; plants are grown in liquid culture and are fed through a nutrient solution containing all needed elements (Figure 2). Soilless cultivation is considered an advanced, environmentally friendly agriculture practice for enhancing the quality of fresh vegetables (18).
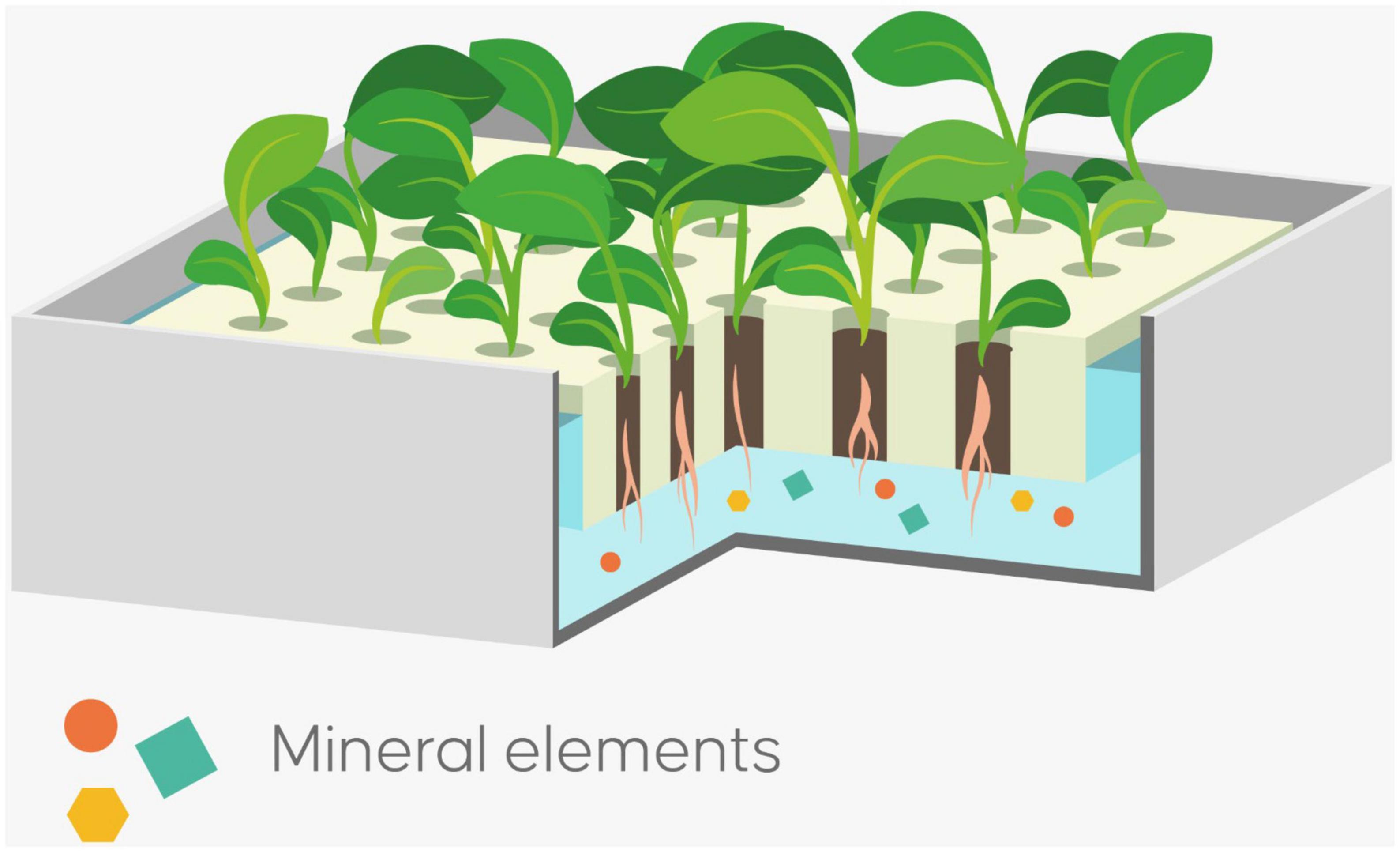
Figure 2. Floating hydroponic system; plants are grown in liquid culture and are fed through a nutrient solution with macro and micro-nutrient essential for plant growth.
In fact, although soilless cultivation systems have been developed primarily to address the challenge of excessive soil pathogens, it is nonetheless true that they also favor optimal control of plant growth, high productivity, and an efficient use of water and fertilizers (19). Furthermore, soilless systems represent an opportunity to modulate the nutrient solution precisely and efficaciously in the effort to improve both its organoleptic and bioactive quality traits (20). Soilless cultivation systems are being used for all of these reasons to monitor nutrient content and to enhance the quality of fresh vegetables. In fact, the constant exposure of the roots to the nutrient solution without soil interaction can maximize their uptake, translocation, and accumulation in the vegetable edible parts (20). At the same time, these novel cultivation systems, especially hydroponic ones, enhance the nutrient content of some vegetables, and thus promote the production of biofortified vegetables for personalized nutrition (21, 22).
The idea of personalized nutrition is based on the widely accepted concept of medically tailored meal programs that are prescribed to patients with specific diseases, such as diabetes (23). The idea of using some foods with specific nutritional traits for a personalized nutrition can be considered advantageous from both clinical and quality of life viewpoints (24). The soilless production of biofortified vegetables could thus represent an important strategy for directly obtaining tailored fresh vegetables without any processing steps in between.
Biofortified vegetable products for specific categories of people
This section presents current up-to-date knowledge about the use of soilless systems to produce biofortified vegetables focusing on three categories of individuals who might benefit the most from their distinctive peculiarities: (a) patients with impaired kidney function; (b) individuals diagnosed with or at risk of osteopenia/osteoporosis; (c) persons suffering from mental illnesses.
(a) Impaired kidney function
Although potassium (K) is an essential nutrient of the human body, groups of people with reduced renal potassium excretion are sensitive to the intake of K recommended by the World Health Organization (at least 3,510 mg day–1 in the adult population) (25). The first group on this list is that of subjects with chronic kidney disease (CKD). Together with other so-called “lifestyle-related diseases,” the condition represents a global problem (26). It is estimated that about 10% of the worldwide population is affected by CKD, and millions die each year because they do not have access to affordable treatments. As opposed to that of the healthy population, the K intake for people affected by CKD is generally restricted to 1,500 mg day–1 (27) in the effort to avoid adverse effects on heart function due to disturbances in plasma potassium concentrations, commonly known as hyperkalemia (28). It is important to note that in addition to patients with CKD, individuals taking medicines such as mineralocorticoid receptor antagonists affecting the renal excretion of potassium, need a lower K intake with respect to the healthy population (28).
Since vegetables contain high concentrations of potassium (29), individuals with impaired kidney function are generally told to avoid raw vegetables and to eat only small quantities of cooked ones given that K content is reduced by leaching and boiling (30). While it is true that boiling vegetables reduces their K content, it should also be remember other important compounds for human health, such as hydrophilic vitamins, are also lost (31). Another important consideration is that people who are accustomed to frequently eating raw vegetables will almost certainly find it difficult to change their eating habits. Clearly, people with impaired kidney function would benefit if vegetables containing lower K content become available. Experimental results regarding vegetables production with low K content are summarized in Table 1.
Some investigators used a hydroponic system to produce micro chicory and micro spinach without K or with 29.1 mg of K L–1 (24). They reported that 100 g of low K content microgreens provided only 7.7–8.6% of the K daily intake recommended for people affected by CKD, while the same serving size of their mature counterparts provided approximately a fourfold higher K intake (24).
A hydroponic floating system of spinach and Swiss chard using a nutrient solution with 50 mg of K L–1 led to a significant decrease in K in baby-leaves for both (on average 27 and 39%, respectively, for spinach and Swiss chard) with respect to the K concentration (200 mg L–1) usually used to grow baby leaf vegetables in hydroponic conditions (21). Since both of these leafy greens are commonly considered potassium-rich vegetables, these experimental results confirm that the commercial production of baby-leaves of spinach and Swiss chard is possible.
When the K content in the nutrient solution was reduced from 156 to 0, the decrease in K in hydroponically grown melons was between 20 and 40% (32). Likewise, melon plants grown with 50% of the required K, produced fruits with a K content about 53% lower with respect to a standard solution of 156 mg of K L–1, excluding other differences among the cultivars (33). These results highlight that 100 g of low-K content melons would provide about 9.3–14.7% of the K daily intake recommended for people affected by CKD, while the same serving size of conventionally grown melons would provide an approximate threefold higher intake of K (32, 33).
The hydroponic production of tomatoes using a nutrient solution of 39 mg of K L–1 and completely removing K from the nutrient solution after anthesis led to a cultivar-dependent decrease of K (between 40 and 60%) in the tomatoes with respect to using a standard solution containing 156 mg of K L–1 (34). These low-K content tomatoes would provide about 6.3–9.1% of the K daily intake recommended for people affected by CKD, while the same serving size of conventionally grown tomatoes would provide approximately a threefold higher K intake (34).
Overall, these experimental results demonstrate that hydroponic production technologies to produce vegetables with a low potassium content can obtain low-K products for people with impaired kidney function. Reducing K content in fruit appears nevertheless to be a more complex process as the growing cycle is longer. A transition from the vegetative to the reproductive phase is also involved and it is difficult to decide the right time to drastically reduce or entirely remove K from the nutrient solution without negatively affecting yield and/or the quality of the vegetable products. In fact, in Japan a standard solution of 156 mg L–1 of K, which was used in the studies described above, is widely utilized for the hydroponic cultivation of tomatoes and melons (33, 34). In other countries, however, 150 mg L–1 is already considered a low K concentration for tomatoes grown hydroponically, while quantities of 300 and 450 mg of K L–1 are considered, respectively, as medium and high (35).
(b) Bone health and osteoporosis
The human skeletal system is a complex organ in constant equilibrium with the rest of the body. In addition to providing structural support for the body, bone is the major reservoir for many minerals and compounds essential for maintaining a healthy pH balance (36). Bone health is the resultant of bone mass, bone architecture, and body mechanics. Illnesses like osteoporosis, characterized by low bone mass and microarchitectural deterioration of the bone tissue lead to decreased bone strength and increased risk of low-energy fractures, or so-called fragility fractures (37). Bone Mineral Density (BMD) is the measure that is commonly used to quantify bone health. A lower BMD value indicates an increased risk of osteoporosis or fractures. Osteoporotic fractures are a major cause of morbidity and disability in the elderly population and, in the case of hip fractures, can lead to premature death. It is important to remember nevertheless that although the deterioration of the body during the aging process renders the older adult particularly susceptible to poor bone health, osteoporosis should not be considered a disease exclusively pertaining to elderly individuals as it globally affects millions of men and women of all ages and ethnicities (38, 39).
Bone mass is influenced by factors such as sex, hormones, as well as genetic and environmental variables and last, but not least, nutrition. From a nutritional viewpoint, it is well known that the optimal intake of calcium (Ca) and vitamins D and K are important factors in the primary as well as secondary prevention of osteoporosis (38). It is also well known that the daily recommended intake of Ca for individuals between 19 and 50 is 1,000 mg, while it is 1,200 mg for those over 50 (40). Silicon is another element that has been associated with promoting bone formation and increasing BMD in men and premenopausal women (41, 42). It would seem that an intake between 10 and 25 mg per day is able to improve bone health (43, 44). Furthermore, some data indicate that between 1.0 and 3.0 mg per day of Boron (B) has beneficial effects on bone health (43–45). It is important to remember nevertheless, that there is no single food or nutrient capable of ensuring bone health on its own. Instead, a balanced diet with appropriate quantities of fruits and vegetables containing vitamins, minerals, and alkalinizing substrates is thought to be the best approach (38, 39). In the light of these remarks, eating vegetables with a high content of Ca, Si, and B appears to be an efficacious way of promoting bone health particularly in people who are susceptible to osteoporosis. Examples of biofortified vegetables with Ca, Si, and B are reported in Table 2.
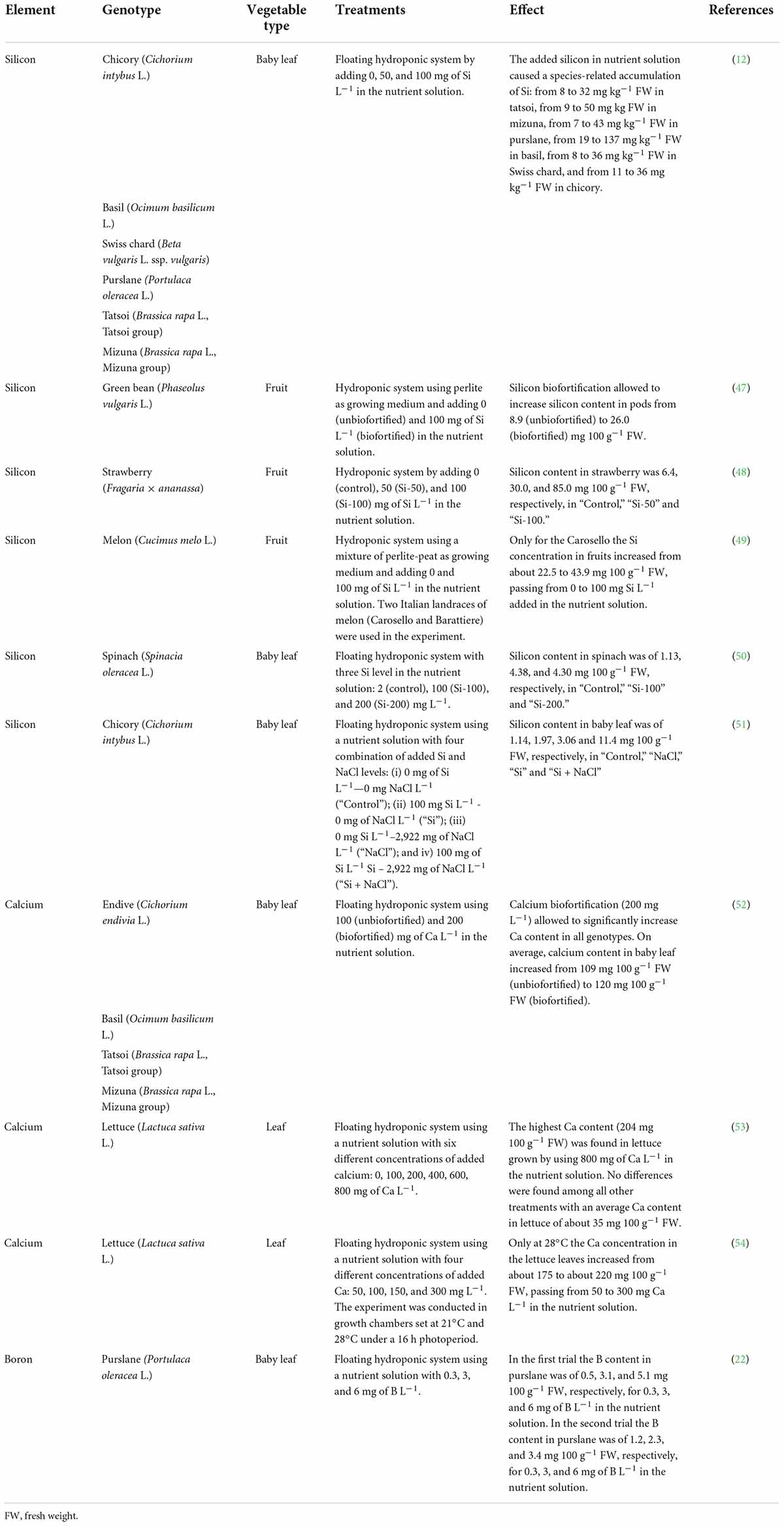
Table 2. Biofortified vegetables with calcium, silicon and boron indicated to people for whom it is desirable to promote bone health.
Silicon in the range of 50–100 mg L–1 in the nutrient solution was used to biofortify a series of baby-leafy vegetables (chicory, basil, purslane, Swiss chard, tatsoi, and mizuna). The biofortified vegetables showed, on average, more bioaccessible Si with respect to the unbiofortified ones (12). It was found that 100 g of these biofortified vegetables could provide approximately 13, 20, 17, 55, 14, and 14% of silicon intake (25 mg/day), respectively, for tatsoi, mizuna, purslane, basil, Swiss chard and chicory. All of these vegetables can be eaten raw or cooked; silicon-biofortified-basil can also be used to make a pesto sauce (46), a particularly healthy dish for persons with risk factors for osteoporosis.
Silicon-enriched green beans through soilless cultivation has produced a Si concentration about 192% higher than that in controls (47). Therefore, the full daily intake of Si (25 mg Si per day) could be satisfied by consuming about 96 g of biofortified green beans. Some investigators reported that the Si content of biofortified pods is higher than that in unbiofortified ones even after cooking, regardless of the cooking method used. Furthermore, Si bioaccessibility in the cooked pods was more than tripled following biofortification (47).
In a study evaluating silicon biofortification of strawberries, the Si content was increased with respect to that in controls, obtaining 3.7–12.2-fold higher values by using, respectively, 50 and 100 mg of Si L–1 in the nutrient solution (48). These results suggest that it is possible to satisfy the full daily intake of Si (25 mg of Si/day) by consuming 83 or 29 g of biofortified strawberries, respectively, for plants grown by adding 50 and 100 mg of Si L–1 in the nutrient solution.
Carosello, an Italian melon consumed ripe or immature, has been biofortified by adding 100 mg of Si L–1 in the nutrient solution (49). Some investigators reported identifying a Si concentration in these landraces about 95% higher than that in plants grown using a nutrient solution without added Si. These results suggest that it is possible to satisfy the full daily intake of silicon (25 mg/day) by consuming only 57 g of biofortified Carosello fruits.
Quantities higher than 100 mg of Si L–1 were added to the nutrient solution in a study evaluating the Si biofortification of baby leaf spinach (50). The investigators reported a Si content about 288% higher in spinach grown using 100 mg Si L–1 in the nutrient solution with respect to that in the controls (2 mg of Si L–1); no further increase in Si content in the spinach was found when addition in the nutrient solution went from 100 to 200 mg of Si L–1 (50). According to that study, 100 g of biofortified spinach provided 17% of the Si intake (25 mg per day), independently of the quantity of Si (100 or 200 mg L–1) added to the nutrient solution.
Other investigators (51) found that the biofortification of chicory plants with Si in combination with NaCl supplementation enhanced the Si tissue enrichment more with respect to Si biofortification alone. Moreover, bioaccessible Si in chicory under “Si + NaCl” treatment was found to be the highest (51). According to these results 100 g of chicory grown using a nutrient solution enriched with Si in combination with NaCl could supply about 46% of the Si intake (25 mg per day). It is important to remember that eating 100 g of silicon-biofortified chicory under salinity stress conditions would mean consuming 190 mg of Na, a value that could be considered negligible as far as the recommended limits are concerned (51).
As far as Ca is concerned, some investigators (52) found that the average Ca content increased by 9.5% in four types of baby leaves (endive, basil, tatsoi, and mizuna) when the addition in the nutrient solution went from 100 to 200 mg of Ca L–1. Moreover, Ca bioaccessibility ranged from 25% (basil) to 40% (endive), and the biofortified vegetables showed more bioaccessible Ca. On average, the consumption of 100 g of Ca biofortified baby leaf vegetables would provide an intake of 119 mg of Ca, equivalent to 10–12% of the daily intake.
Adding 800 mg of Ca L–1 to the nutrient solution led to a Ca concentration in lettuce about fivefold higher with respect to that for other treatments (53). It is interesting to note that 100 g of lettuce biofortified using 800 mg of Ca L–1 in the nutrient solution can supply about 20% of the Ca daily intake; the same serving size of lettuce after lower quantities of Ca biofortification supplies only 3.5% of the Ca daily intake.
Another study aiming to evaluate the increase in calcium content in lettuce leaves grown at different temperatures uncovered that the Ca concentration increased by about 26% rising from 50 to 300 mg of Ca L–1 in the nutrient solution, but only at 28°C (54). In fact, the same Ca concentration used at 21°C did not affect the Ca content in the lettuce (54). The results of this study indicate that 100 g of Ca biofortified lettuce can supply about 18–22% of the recommended daily intake.
When they evaluated boron-biofortification in purslane, a wild edible plant, some investigators found that the B content was increased with respect to that in the controls, obtaining 1.8–10.7-fold higher values by using, respectively, 3 and 6 mg L–1 of B in the nutrient solution. The average daily intake of B (2 mg) could thus be satisfied by consuming between 48 and 75 or 48 g of biofortified purslane (22).
Overall, these experimental results reviewed in the present manuscript show that the hydroponic cultivation of biofortified vegetables for Ca, Si, and B could be an effective way to obtain vegetable products promoting bone health. It should also be remembered that a serving size of less than 100 g of biofortified fruit or vegetables could satisfy the full recommended daily intake of Si, while a serving size of 100 g of leafy vegetables could supply only a part, generally between 13 and 55%. The Ca biofortification of vegetables would mean that 100 g of a vegetable could contain 20% of the recommended daily intake. As far as B biofortification is concerned, consuming a serving size of vegetables less than 100 g would satisfy its recommended daily intake.
Experimental results also demonstrated that progressively increasing amounts of a mineral elements in a nutrient solution does not necessarily correspond to the increase of this element in the edible part of vegetables. Moreover, it should also be remembered that a salt must be introduced into the nutrient solution to increase the content of a specific cation (such as Ca). As a consequence, even the content of undesirable anions, such as nitrate and chloride, may be higher in biofortified vegetables.
From a nutritional point of view, if a vegetable can be enriched with Ca, Si, and B simultaneously this would facilitate the production of bone-healthy vegetables in particular for individuals at risk of osteopenia/osteoporosis.
(c) Mental illnesses
Mood disorders, including bipolar disorder, represent an important category of mental illnesses, whose prevalence is generally increasing in developed countries. Lithium (Li) compounds seem to be among the most promising and effective drugs used to treat this disorder, in particular with regard to bipolar affective disorder (55). There has also been some evidence that Li may be useful in preventing neurodegenerative diseases such as Alzheimer’s disease (56) in treating depression, and in stabilizing moods due to its antimanic, antisocial, and prophylactic properties (57).
Lithium, a naturally occurring metal in the earth’s crust, is used in the form of carbonate (Li2CO3) as a treatment for psychiatric disorders. Between 600 and 1,200 mg Li2CO3 per day, containing 113–226 mg of elemental Li (58), are the usual doses prescribed for these types of disorders. It should in any case be remembered that there is some evidence that although lithium is not officially considered a micronutrient, a daily intake of 1,000 μg Li for a 70-kg adult (14.3 μg kg–1 body weight) could effectively prevent mood disorders and reduce impulsiveness and nervousness especially in subjects at risk (59). According to these studies, lithium-enriched foods could help to stabilize moods in those subjects. The main sources of Li in the diet are nuts, cereals, fish, and vegetables, but their percentages are negligible in many geographic regions (59).
In a study aiming to evaluate the Li intake in foods served to students, some Authors (60) found that the Li amount supplied daily via the diet was 10.7 μg, an intake that can be considered low with respect to the proposed amount of 1,000 μg Li per day. Thus, vegetables enriched with lithium could have a positive effect on the mental health of individuals susceptible to mental illness. Some vegetables biofortified with lithium are reported in Table 3.
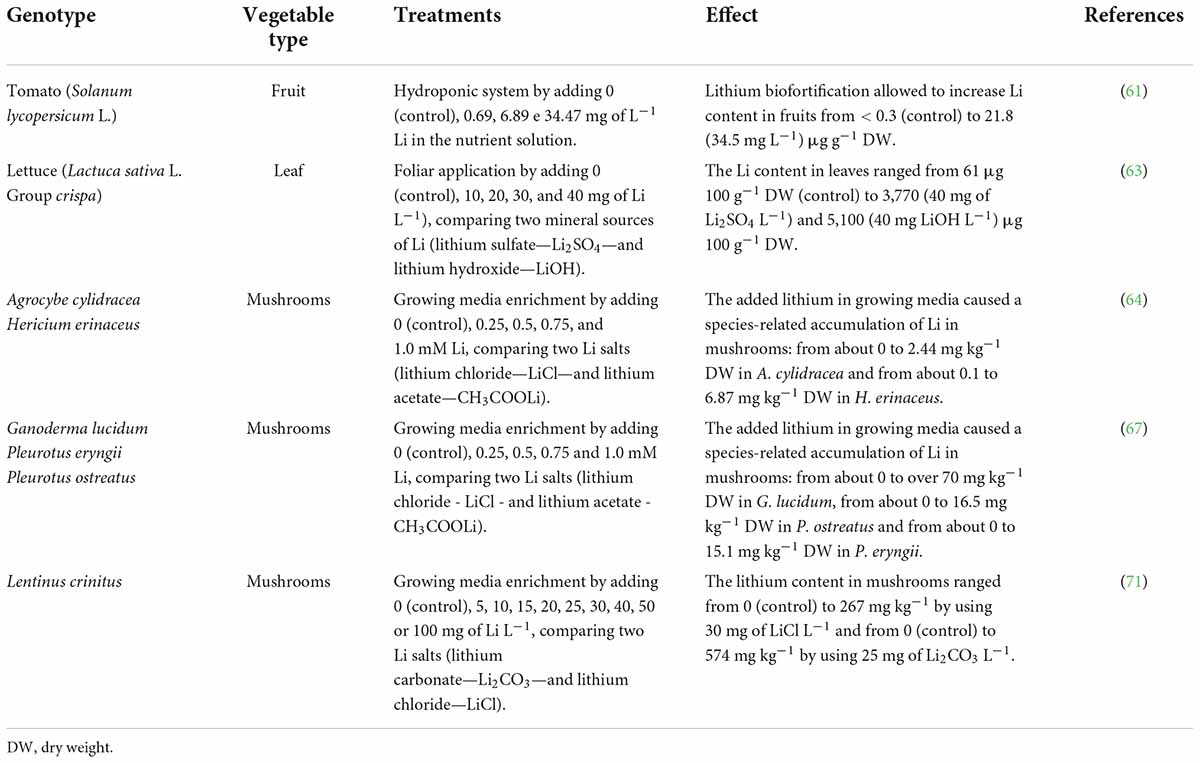
Table 3. Lithium biofortified vegetable products indicated to people for whom it is desirable to promote mental health.
A study carried out some time ago evaluated Li concentrations in tomatoes, which was increased with respect to that in controls, obtaining a value 70-fold higher by using 34.5 mg of Li L–1 in the nutrient solution (61). The investigators reported a content of 21.8 μg of Li g–1 of dry weight, but they did not indicate the dry weight values. Thus, hypothesizing an average dry weight of 5.5 g 100 g fresh weight (62), a serving size (100 g) of lithium-biofortified tomatoes can supply about 12% of the recommended daily intake (1,000 μg Li per day), while the same serving size of unbiofortified tomatoes can supply less than 0.2% of the daily intake.
Another study aiming to evaluate the increase in Li content of lettuce grown using five different foliar spray concentrations (0—control, 10, 20, 30, and 40 mg of Li L–1) and to compare two mineral Li sources (lithium sulfate—Li2SO4—and lithium hydroxide—LiOH) (63) reported that the Li concentration in the lettuce leaves was directly proportional to the concentrations of this element sprayed on the leaves regardless of the chemical form used. The Li concentration, which was low in the control plants (61 μg 100 g–1 dry weight), resulted 84-fold higher when 40 mg of LiOH L–1 was used and 61-fold higher when 40 mg of Li2SO4 L–1 was used, although for both sources the highest Li levels caused about a 15% reduction in plant height with respect to that in the controls (63). Nevertheless, considering an average dry weight of 9.5 g 100 g, a serving size (100 g) of Li biofortified lettuce can supply up to 484 μg of Li (using 40 mg of LiOH L–1), which is about 50% of the Li daily intake.
Several studies have been carried out evaluating the biofortification of mushrooms with Li as a means to increase the daily intake of the mineral. Lithium chloride (LiCl) and lithium acetate (CH3COOLi) at concentrations of 0 (control) 0.25, 0.5, 0.75, and 1.0 mM were used to enrich the substrate in which Agrocybe cylindracea (known as poplar mushroom, velver pioppin or Yanagi-matsutake) and Hericium erinaceus (traditionally called lion’s mane, bearded tooth, satyr’s beard, bearded hedgehog or pom pom) (64) were grown. Although the authors of the study did not indicate the mushrooms’ dry weight, they reported that a concentration of 1.0 mM determinated a Li content in the mushrooms hundreds of times higher than that in the controls. One study reported an average dry weight of 9.3 g 100 g–1 fresh weight for A. cylindracea (65) and 11.4 g 100 g–1 fresh weight H. erinaceus (66). Thus, a serving size (100 g) of biofortified A. cylindracea and H. erinaceus would supply, respectively, up to 2.3 and 7.8% of the lithium daily intake (64).
Other Authors used LiCl and CH3COOLi at concentrations of 0 (control) 0.25, 0.5, 0.75, and 1.0 mM to enrich the cultivation substrate in which Ganoderma lucidum (known as Reishi mushroom), Pleurotus eryngii (also known as king trumpet mushroom) and P. ostreatus (also known as oyster mushroom, “hiratake,” “shimeji,” or “houbitak) (67). Similarly to the previous study, these Authors found an increase in Li hundreds of times higher with respect to that in the control when 1.0 mM was used; again, even in this study the authors did not report the dry weight of the mushrooms. An average dry weight of 11.8, 8.7, and 10.8 g 100 g–1 fresh weight, respectively, for G. lucidum. P. eryngii and P. ostreatus has been reported by other authors (68–70). It would seem then that a serving size (100 g) of biofortified G. lucidum, P. eryngii and P. ostreatus would supply, respectively, up to 83, 13.1, and 17.8% of the recommended Li daily intake (67).
By using LiCl and lithium carbonate (Li2CO3) at concentrations of 0 (control), 5, 10, 15, 20, 25, 30, 40, 50, or 100 mg L–1 to enrich the substrate for growing Lentinus crinitus, other Authors obtained biofortified mushrooms containing up to 26.7 and 57.4 mg Li 100 g–1 dry weight, respectively, with 30 mg of LiCl L–1 and 25 mg of Li2CO3 L–1 (71). The Li concentrations in the mushrooms were higher than those reported by the previous studies. In this case the authors did not provide data concerning the dry weight of the mushrooms and there is no data in the literature specifying the dry weight of L. crinitus. We can assume that the average dry weight is about 11 g 100 g–1 fresh weight given the weight of a mushroom of the same genus, namely L. edodes (also known as “shiitake” mushroom) (72). Based on these assumptions, the daily intake of lithium can be satisfied by consuming about 34 g of L. crinitus biofortified using lithium chloride or about 18 g of mushrooms biofortified using lithium carbonate (71).
Overall, the experimental results show that plants can be biofortified with Li by adding an enriched nutrient solution to the culture substrates or via foliar applications. They also demonstrate that progressively increasing quantities of Li in the nutrient solution corresponds to increasing quantities of the element in the edible part of the vegetables (61, 63, 67, 71). The appropriate dosage of Li in the nutrient solution to positively affect the mental health of people susceptible to mental illness is an important consideration. It would seem that a too low Li level in the nutrient solution translates into vegetable products which supply low daily intakes of Li per serving size. On the other hand, too high Li levels in the nutrient solution should be avoided, since it cannot be excluded that vegetable products with high concentrations may be harmful to human health.
Bioavailability and bioaccessibility: A useful support tool for the precision biofortification
Whatever the biofortification approach used, obtaining biofortified vegetables represents only the first step toward achieving tailored food for personalized nutrition. The next step is determining whether the increase or reduction in a specific nutrient in the edible parts of the plant changes its bioaccessibility and bioavailability parameters. The evaluation of the benefits and/or risks associated with absorbing a particular element from biofortified vegetables must, in fact, take into consideration their bioaccessibility and bioavailability (Figure 3), which refer to the processes involved in extracting mineral elements and absorbing them; food components do not in fact exactly correspond to their functional value.
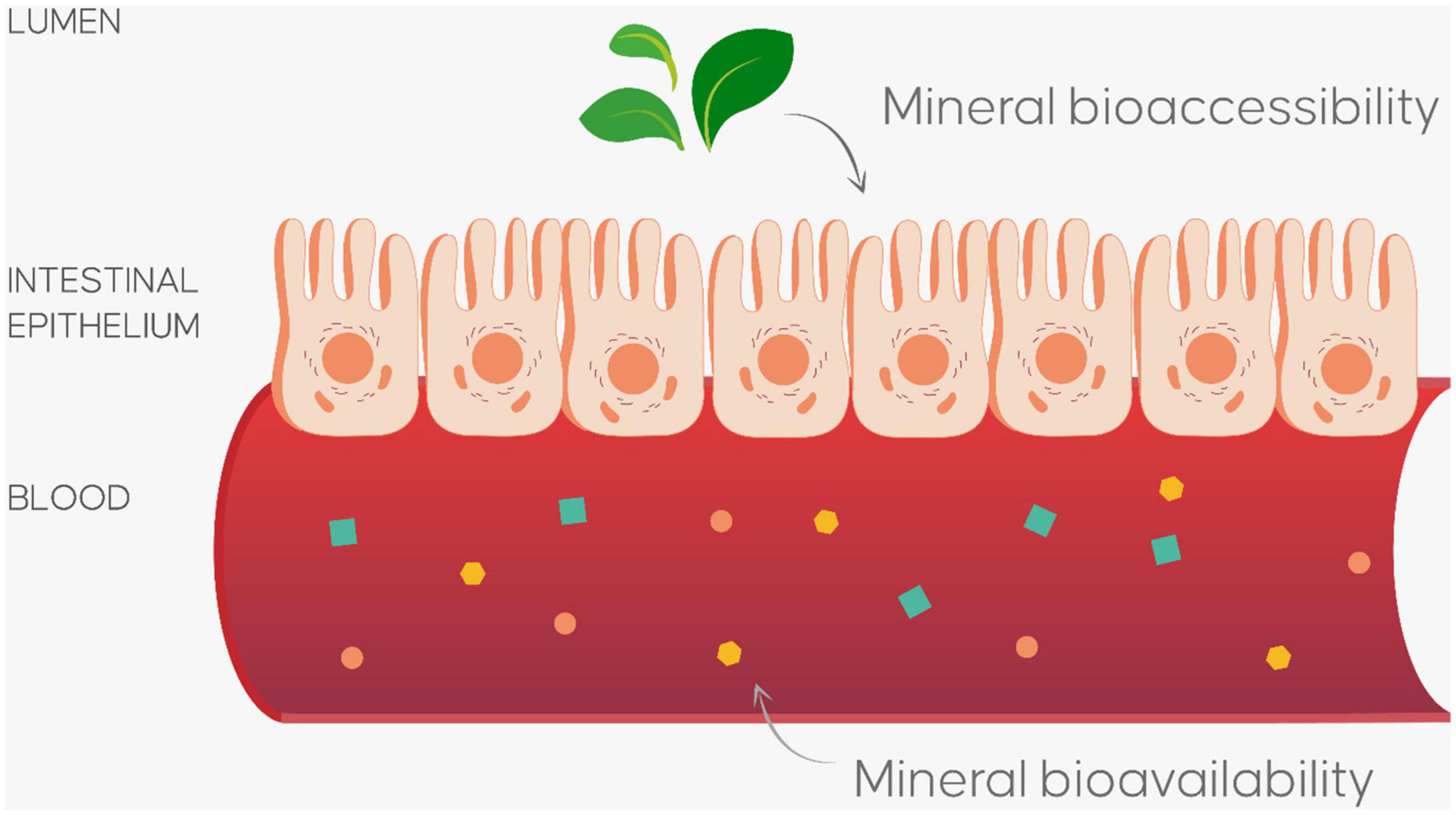
Figure 3. In vitro model for evaluate bioaccessibility (percentage of nutrient release from food matrix during gastro-intestinal digestion process) and bioavailability (percentage of nutrient adsorbed in intestinal tract after gastro-intestinal digestion process) of nutrients and/or bioactive compounds.
When plant foods are consumed, the nutrients (organic and inorganic) and bioactive compounds are released from the plant tissue and modified into absorbable units which can subsequently be absorbed by the epithelial cells in the gastrointestinal tract and transported to their respective target tissues (73) through the blood (74). But not all nutrients in the edible parts of a food can carry out a biological activity. Some in vitro models have been developed to simulate the physiological conditions of the human gastrointestinal digestion. The biochemical, physiological and dynamic conditions of the gastrointestinal system (the mouth, stomach, and intestines) such as temperature, mechanical forces, pH, the concentration of some enzymes (pepsin, pancreatin, and lipase) and the presence of bile (75) have been artificially reproduced in the gastrointestinal digestion model. The in vitro digestion model provides information about the amount of nutrients released from the food matrix (basically its bioaccessibility), their biochemical transformation and chemical degradation, the nutrient-nutrient and nutrient-antinutrient interactions and the effect of the matrix and food processing (75).
The release of nutrients (the bioaccessible fraction) in the intestinal tract during the digestive process of plant materials depends on a variety of factors, such as the species and the type of plant materials, the localization of the nutrient, and it is affected by a host of variables such as the concentrations of other nutrients and/or anti-nutritional compounds, the cooking method used, if and how the food has been processed, and the interaction of other nutrients. The concentration of the nutrient in the edible parts is important, but even the biofortification process can modify the release of nutrients, as has been reported by a number of studies regarding different mineral elements (12, 21, 47, 51, 52, 76).
The gastrointestinal digestion model has been used to identify the biofortified species or treatments (agronomic and/or food processing) that are able to release high quantities of silicon (12), Ca (52), zinc (76, 77), selenium (78), iodine (79), iron (80) in the intestinal tract. Thus, bioaccessibility assessment methodologies can be used to select the species, cultivars and/or genotypes that are able to release high quantities of nutrients during the digestion process in order to maximize the health effects of the biofortification process.
The bioavailability fraction can be used to evaluate bioavailability via an established bioassay (81); this parameter has been defined as the quantity of nutrient/s adsorbed, in the intestinal tract during the gastrointestinal digestive process (82). In general, mineral bioavailability needs to be evaluated via in vivo human studies. Some in vitro models have been proposed as an alternative to using animal models to evaluate mineral bioavailability to estimate nutritional efficiency (bioefficacy) and to determine the potential health effects of the biofortification process (79, 83–85). Currently, there are several in vitro models capable of simulating the intestinal mucosa with phenotypic characteristics comparable to in vivo conditions. The most widely used cell model is represented by the Caco-2 with applications in the study of active transport of mineral nutrients (84, 86).
Only a few studies, aiming to evaluate biofortified vegetables have been conducted to assess bioaccessibility and bioavailability as part of the biofortification process. In general, these studies used a multidisciplinary workflow based on an evaluation of the efficiency of the biofortification process from a nutritional point of view, taking into consideration bioaccessibility, bioavailability and biological activity as well as agronomic efficiency (12, 80, 84, 86, 87). The approach is summarized in Figure 4. Bioavailability and bioaccessibility assessment provides nutritional and biological information regarding biofortified products generally not furnished by agronomic biofortification studies. In addition, it can be used to improve the development of biofortified foods with regard to the choice of the species or of the type of food processing to use to produce plant foods that satisfy consumer needs (76, 80, 86, 88).
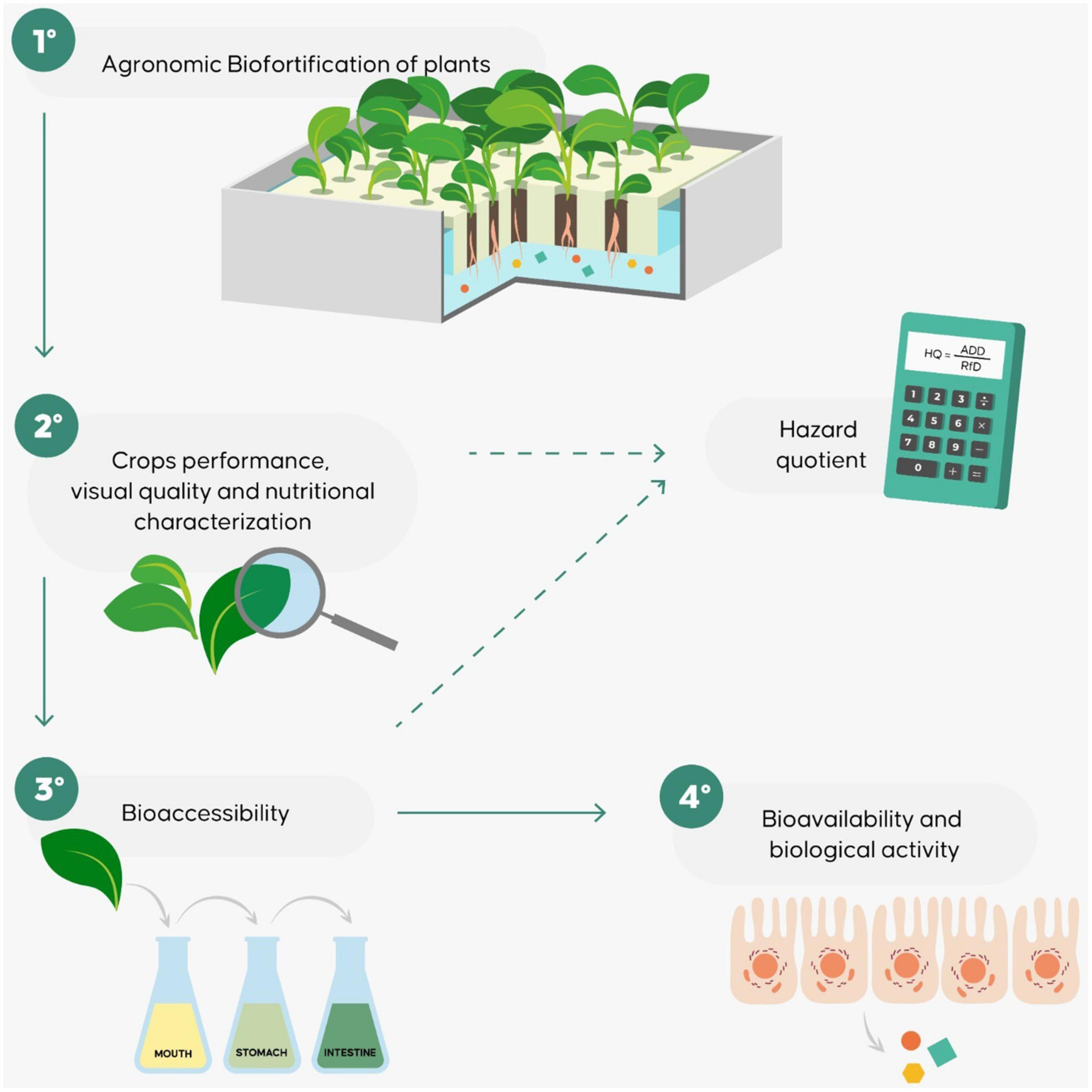
Figure 4. The workflow proposed to evaluate the agronomic, nutritional, and bioefficiency of biofortified products.
Ongoing trends and the challenges ahead
In addition to the three specific categories of individuals discussed above, other groups may be interested in switching over to the consumption of soilless produced vegetables containing higher quantities of essential micronutrients/compounds and low concentrations of undesired elements or compounds.
Nickel (Ni) is an ubiquitous trace element and the commonest cause of metal allergy. Individuals sensitized to Ni through dermal contact and who have allergic contact dermatitis (some have estimated to that it affects up to 15% of women, but it is an undiagnosed entity) develop hand eczema from oral, as well as dermal, exposure to Ni salts. Oral intakes of Ni as low as approximately 500 μg per day have been reported to aggravate hand eczema in Ni sensitized subjects (89). Nickel in soil and water is taken up by living organisms, plants and animals that are food sources for humans; it is therefore present in most of the constituents of a normal diet. The Ni content in fruits and vegetables is on average fourfold higher with respect to food of animal origin (90). At the same time, Ni content in vegetable food products can vary widely, depending on the Ni content in both soil (ranging between 5 and 500 μg g–1) and water (between 5 and 100 μg L–1). For these reasons, the Ni content in individual foods appears to vary widely depending on a number of variables (90). But independently of the Ni content of the soil, some vegetable products, such as legumes, whole wheat, and cocoa and derivates are known to have a high Ni content. With regard to other types of vegetable food products it is difficult to define what is “high in nickel” because different thresholds have been used by many authors and institutions. In fact, the threshold can range from 0.5 to 0.03 mg kg–1. Using the latter threshold, a host of vegetable products including tomatoes and carrots should be considered high in Ni (90). More reliable data about the Ni content in foods are therefore needed. Currently, some hydroponic companies are producing certified vegetables that comply with the requirements of the “Product Technical Specification” regulating the vegetable supply chain and guaranteeing that nickel is absent in food products (91, 92). For the time being there are no studies in the literature regarding the soilless production of vegetables with undetected (verified analytically) nickel. Future studies should thus aim to develop soilless vegetable production methods experimenting with the growing media, irrigation water, fertilizers and cultivars in order to reduce the uptake of Ni and restrict its translocation to the edible parts of the plant and therefore to produce practically Ni-free vegetables for individuals sensitized to Ni.
Nitrate (NO3), which is a naturally occurring form of nitrogen, is an integral part of the nitrogen cycle in the environment. Approximately 80% of the nitrates in the daily diet come from the consumption of vegetables, mainly through green-leaf vegetables (93). For the most part NO3-accumulating vegetables belong to the Brassicaceae (rocket, radish, mustard), Chenopodiaceae (beetroot, Swiss chard, spinach) and Amarantaceae families. The Asteraceae (lettuce) and Apiaceae (celery, parsley) include species that are characterized by a high content of NO3 (94). Nitrate per se is relatively non-toxic; nevertheless once ingested NO3 is converted to nitric oxide. It can react with hemoglobin (oxyHb) to form methaemoglobin (metHb), which may impair oxygen delivery to human tissue causing methaemoglobinaemia, or blue baby syndrome. Infants are more susceptible to a syndrome characterized by clinical symptoms such as the blue discoloration of the skin due to the presence of deoxygenated blood and asphyxia. This occurs because young infants have less of the reductase needed to reconvert the metHb back to oxyHb and have low NO3-reducing activity due to low gastric acidity (94). Given these considerations, the European Food Safety Authority (EFSA) released a statement on possible public health risks for infants and young children linked to the presence of nitrates in leafy vegetables, and established that the maximum NO3 concentration for baby foods is 200 mg kg–1 fresh weight, including vegetables (95). Some of the strategies that can be used to reduce the NO3 content in vegetables grown using hydroponic systems are: (i) removing part or all of the nitrate nitrogen from the nutrient solution a few days before harvesting; (ii) using nutrient solutions with NO3-N and NH4-N rather than nitrate nitrogen only (96); (iii) growing vegetables under high light intensity conditions (97).
Beyond the possible acute health effects of nitrates in infants and young children who consume spinach and lettuce, it should be remembered that NO3 supplementation enhances nitric oxide (NO) bioavailability via the NO3–nitrite–NO pathway, which is involved in several physiological processes that could potentially improve skeletal muscle function. In fact, there is evidence that dietary NO3 supplementation has ergogenic effects during endurance and sprint-type exercises and others types of physical activities such as weightlifting (93, 98). The limited number of studies as well as the diversity of the results published impede us from drawing any clear conclusions about NO3 supplementation in athletes. As far as endurance sports are concerned, the dose necessary for a significant effect continues to be unclear since in some trials acute doses of 12 mmol of NO3 were used while in others smaller doses (up to 6 mmol day–1 of NO3) were utilized (93). Despite the differences in the supplementation dosage (from 32.5 mg NO3 to 6.4 mmol of NO3) used in the trials and in the periods of supplementation (from acute to chronic over 6 days), the limited data existing in the literature suggest that dietary nitrate supplementation could potentially enhance weightlifting performance (98). Further research should attempt to analyze the ergogenic effect of nitrate supplementation on athletes as well as to study the optimal sources and the most suitable species and doses of nitrates and the best hydroponic systems to produce nitrate-enriched tailored vegetables. The challenges ahead include those of identifying the categories of people who would most benefit from vegetable biofortification and determining the sustainability of the production processes. According to the “Farm to Fork” strategy, which is at the heart of the European Green Deal, food systems cannot be resilient to crises if they are not sustainable. Our food systems need therefore to be set on a sustainable path which will also create new opportunities for operators in the food value chain (99). While it may seem counterintuitive, high−tech soilless cultivation systems and organic agriculture have several converging points in view of a sustainable use of the planet’s natural resources (100). In the future those working in the sector should aim to verify that soilless cultivation systems have a low environmental impact and that biofortified vegetables are high quality products.
Conclusion
Enhancing the precision biofortification processes through soilless systems and understanding the aspects related to bioaccessibility and bioavailability of a particular element from a biofortified vegetables are launching horticultural science into the era of personalized nutrition. Consequently, it is clear that the multidisciplinary approach toward tailored foods is a winning one and must increasingly include a synergy between agronomic, biological and medical skills. Therefore, for further goals vegetable biofortification trials could be joined to clinical studies for assessing the potential additional benefits of the emerging biofortified vegetables for specific categories of people.
Author contributions
MR, MD’I, and FS contributed to the study concept and design of the manuscript, tables and figure preparation, and edition. MR, MD’I, FS, and SM critically reviewed the article. MR and MD’I contributed to the acquisition and analysis of data and drafted the manuscript. All authors gave final approval for all aspects of the work, agreed to be fully accountable for ensuring the integrity and accuracy of the work, and read and approved the final manuscript.
Funding
This work was supported by CNR project NUTR-AGE (FOE-2019, DSB.AD004.271) and PON project «R&I» 2014–2020—“ALIFUN—Development of functional foods for the innovation of Italian traditional food products” funded by the Italian Ministry of University and Research (MUR) under the PON Agrifood Program (ARS01_00783).
Acknowledgments
Appreciation is expressed to Linda Inverso Moretti for her assistance in editing the manuscript and Alessia Mennuni for illustrations.
Conflict of interest
The authors declare that the research was conducted in the absence of any commercial or financial relationships that could be construed as a potential conflict of interest.
Publisher’s note
All claims expressed in this article are solely those of the authors and do not necessarily represent those of their affiliated organizations, or those of the publisher, the editors and the reviewers. Any product that may be evaluated in this article, or claim that may be made by its manufacturer, is not guaranteed or endorsed by the publisher.
References
1. van Ommen B, van den Broek T, de Hoogh I, van Erk M, van Someren E, Rouhani-Rankouhi T, et al. Systems biology of personalized nutrition. Nutr Rev. (2017) 75:579–99. doi: 10.1093/nutrit/nux029
2. Bush CL, Blumberg JB, El-Sohemy A, Minich DM, Ordovás JM, Reed DG, et al. Toward the definition of personalized nutrition: a proposal by the American Nutrition Association. J Am Coll Nutr. (2020) 39:5–15. doi: 10.1080/07315724.2019.1685332
3. Penders B, Horstman K, Saris WHM, Vos R. From individuals to groups: a review of the meaning of “personalized” in nutrigenomics. Trends Food Sci Technol. (2007) 18:333–8. doi: 10.1016/j.tifs.2007.02.004
4. Estruch R, Ros E, Salas-Salvadó J, Covas M-I, Corella D, Arós F, et al. Primary prevention of cardiovascular disease with a Mediterranean diet supplemented with extra-virgin olive oil or nuts. N Engl J Med. (2018) 378:e34. doi: 10.1056/NEJMoa1800389
5. Renna M, Montesano FF, Serio F, Gonnella M. The Mediterranean diet between traditional foods and human health through culinary examples. In: Galanakis CM editor. Gastronomy and Food Science. Cambridge, MA: Academic Press (2021). p. 75–99. doi: 10.1016/b978-0-12-820057-5.00005-4
6. Wang L, Sikand G, Wong ND. Nutrition, diet quality, and cardiovascular health. In: M Malavolta, E Mocchegiani editors. Molecular Basis of Nutrition and Aging. A Volume in the Molecular Nutrition Series. Cambridge, MA: Academic Press (2016). p. 315–27.
7. Hochwallner H, Schulmeister U, Swoboda I, Spitzauer S, Valenta R. Cow’s milk allergy: from allergens to new forms of diagnosis, therapy and prevention. Methods. (2014) 66:22–33. doi: 10.1016/j.ymeth.2013.08.005
8. Rostami K, Bold J, Parr A, Johnson MW. Gluten-free diet indications, safety, quality, labels, and challenges. Nutrients. (2017) 9:10–4. doi: 10.3390/nu9080846
9. Halfdanarson TR, Litzow MR, Murray JA. Hematologic manifestations of celiac disease. Blood. (2007) 109:412–21. doi: 10.1182/blood-2006-07-031104
10. Martin J, Geisel T, Maresch C, Krieger K, Stein J. Inadequate nutrient intake in patients with celiac disease: results from a German dietary survey. Digestion. (2013) 87:240–6. doi: 10.1159/000348850
11. Bouis HE, Welch RM. Biofortification-a sustainable agricultural strategy for reducing micronutrient malnutrition in the global south. Crop Sci. (2010) 50:S20–32. doi: 10.2135/cropsci2009.09.0531
12. D’Imperio M, Renna M, Cardinali A, Buttaro D, Santamaria P, Serio F. Silicon biofortification of leafy vegetables and its bioaccessibility in the edible parts. J Sci Food Agric. (2016) 96:751–6. doi: 10.1002/jsfa.7142
13. Gerardi C, Albano C, Calabriso N, Carluccio MA, Durante M, Mita G, et al. Techno-functional properties of tomato puree fortified with anthocyanin pigments. Food Chem. (2018) 240:1184–92. doi: 10.1016/j.foodchem.2017.08.057
14. Marks & Spencer.Selenium Enriched Brussels Sprouts. (2022). Available online at: http://www.pjstirling.com/high-health.html (accessed February 15, 2022).
15. Pizzoli.Iodine Enriched Potato. (2022). Available online at: https://www.pizzoli.it/site/en/products-category/id-318-lin-iod+igrave.html (accessed February 15, 2022).
16. Buturi CV, Mauro RP, Fogliano V, Leonardi C, Giuffrida F. Mineral biofortification of vegetables as a tool to improve human diet. Foods. (2021) 10:223. doi: 10.3390/foods10020223
17. Aro A, Alfthan G, Varo P. Effects of supplementation of fertilizers on human selenium status in Finland. Analyst. (1995) 120:841–3. doi: 10.1039/AN9952000841
18. Sambo P, Nicoletto C, Giro A, Pii Y, Valentinuzzi F, Mimmo T, et al. Hydroponic solutions for soilless production systems: issues and opportunities in a smart agriculture perspective. Front Plant Sci. (2019) 10:923. doi: 10.3389/fpls.2019.00923
19. Montesano FF, van Iersel MW, Boari F, Cantore V, D’Amato G, Parente A. Sensor-based irrigation management of soilless basil using a new smart irrigation system: effects of set-point on plant physiological responses and crop performance. Agric Water Manag. (2018) 203:20–9. doi: 10.1016/j.agwat.2018.02.019
20. Rouphael Y, Kyriacou MC. Enhancing quality of fresh vegetables through salinity eustress and biofortification applications facilitated by soilless cultivation. Front Plant Sci. (2018) 9:1254. doi: 10.3389/fpls.2018.01254
21. D’Imperio M, Montesano FF, Renna M, Parente A, Logrieco AF, Serio F. Hydroponic production of reduced-potassium swiss chard and spinach: a feasible agronomic approach to tailoring vegetables for chronic kidney disease patients. Agronomy. (2019) 9:627. doi: 10.3390/agronomy9100627
22. D’Imperio M, Parente A, Montesano FF, Renna M, Logrieco AF, Serio F. Boron biofortification of Portulaca oleracea L. through soilless cultivation for a new tailored crop. Agronomy. (2020) 10:999. doi: 10.3390/agronomy10070999
23. Rabaut LJ. Medically tailored meals as a prescription for treatment of food-insecure type 2 diabetics. J Patient Centered Res Rev. (2019) 6:179–83. doi: 10.17294/2330-0698.1693
24. Renna M, Castellino M, Leoni B, Paradiso VM, Santamaria P. Microgreens production with low potassium content for patients with impaired kidney function. Nutrients. (2018) 10:675. doi: 10.3390/nu10060675
25. World Health Organization.Guideline: Potassium Intake for Adults and Children. Geneva: World Health Organization (2012).
27. Committee for Revision of Diet Therapy Guideline. Dietary recommendations for chronic kidney disease. Nihon Jinzo Gakkai Shi. (2007) 49:871–8.
28. Putcha N, Allon M. Management of hyperkalemia in dialysis patients. Semin Dial. (2007) 20:431–9. doi: 10.1111/j.1525-139X.2007.00312.x
29. Renna M, Cocozza C, Gonnella M, Abdelrahman H, Santamaria P. Elemental characterization of wild edible plants from countryside and urban areas. Food Chem. (2015) 177:29–36. doi: 10.1016/J.FOODCHEM.2014.12.069
30. Burrowes JD, Ramer NJ. Changes in potassium content of different potato varieties after cooking. J Ren Nutr. (2008) 18:530–4. doi: 10.1053/j.jrn.2008.08.005
31. Martínez-Hernández GB, Artés-Hernández F, Colares-Souza F, Gómez PA, García-Gómez P, Artés F. Innovative cooking techniques for improving the overall quality of a kailan-hybrid broccoli. Food Bioprocess Technol. (2013) 6:2135–49. doi: 10.1007/s11947-012-0871-0
32. Asao T, Asaduzzaman M, Mondal MF, Tokura M, Adachi F, Ueno M, et al. Impact of reduced potassium nitrate concentrations in nutrient solution on the growth, yield and fruit quality of melon in hydroponics. Sci Hortic. (2013) 164:221–31. doi: 10.1016/J.SCIENTA.2013.09.045
33. Asaduzzaman M, Talukder MR, Tanaka H, Ueno M, Kawaguchi M, Yano S, et al. Production of low-potassium content melon through hydroponic nutrient management using perlite substrate. Front Plant Sci. (2018) 9:1382. doi: 10.3389/fpls.2018.01382
34. Tsukagoshi S, Hamano E, Hohjo M, Ikegami F. Hydroponic production of low-potassium tomato fruit for dialysis patients. Int J Veg Sci. (2016) 22:451–60. doi: 10.1080/19315260.2015.1076921
35. Serio F, Leo L, Parente A, Santamaria P. Potassium nutrition increases the lycopene content of tomato fruit. J Hortic Sci Biotechnol. (2007) 82:941–5. doi: 10.1016/j.sjbs.2013.03.002
36. Barzel US, Massey LK. Excess dietary protein adversely affect bone. Issues Opin Nutr. (1998) 128:1051–3.
37. Øyen J, Gjesdal CG, Brudvik C, Hove LM, Apalset EM, Gulseth HC, et al. Low-energy distal radius fractures in middle-aged and elderly men and women—the burden of osteoporosis and fracture risk. Osteoporos Int. (2010) 21:1257–67. doi: 10.1007/s00198-009-1068-x
38. Prentice A. Diet, nutrition and the prevention of osteoporosis. Public Health Nutr. (2004) 7:227–43. doi: 10.1079/PHN2003590
39. Stránský M, Ryšavá L. Nutrition as prevention and treatment of osteoporosis. Physiol Res. (2009) 58:S7–11.
40. Committee to Review Dietary Reference Intakes for Vitamin D and Calcium.Dietary Reference Intakes for Calcium and Vitamin D. Washington, DC: The National Academies Press (2011). p. 662.
41. Macdonald HM, Hardcastle AC, Jugdaohsingh R, Fraser WD, Reid DM, Powell JJ. Dietary silicon interacts with oestrogen to in fl uence bone health: evidence from the aberdeen prospective osteoporosis screening study. Bone. (2012) 50:681–7. doi: 10.1016/j.bone.2011.11.020
42. Reffitt DM, Ogston N, Jugdaohsingh R, Cheung HFJ, Evans BAJ, Thompson RPH, et al. Orthosilicic acid stimulates collagen type 1 synthesis and osteoblastic differentiation in human osteoblast-like cells in vitro. Bone. (2003) 32:127–35. doi: 10.1016/S8756-3282(02)00950-X
43. Hunt CD. Dietary boron: progress in establishing essential roles in human physiology. J Trace Elem Med Biol. (2012) 26:157–60. doi: 10.1016/j.jtemb.2012.03.014
44. Nielsen FH. Is boron nutritionally relevant? Nutr Rev. (2008) 66:183–91. doi: 10.1111/j.1753-4887.2008.00023.x
45. Nielsen FH. Boron in Aging and Longevity. Cham: Springer (2018). p. 163–77. doi: 10.1007/978-3-030-03742-0_6
46. Masino F, Foca G, Ulrici A, Arru L, Antonelli A. A chemometric study of pesto sauce appearance and of its relation to pigment concentration. J Sci Food Agric. (2008) 88:1335–43. doi: 10.1002/jsfa.3221
47. Montesano FF, D’Imperio M, Parente A, Cardinali A, Renna M, Serio F. Green bean biofortification for Si through soilless cultivation: plant response and Si bioaccessibility in pods. Sci Rep. (2016) 6:1–9. doi: 10.1038/srep31662
48. Valentinuzzi F, Cologna K, Pii Y, Mimmo T, Cesco S. Assessment of silicon biofortification and its effect on the content of bioactive compounds in strawberry (Fragaria × ananassa ‘Elsanta’) fruits. Acta Hortic. (2018) 1217:307–12. doi: 10.17660/ActaHortic.2018.1217.38
49. Buttaro D, Bonasia A, Minuto A, Serio F, Santamaria P. Effect of silicon in the nutrient solution on the incidence of powdery mildew and quality traits in carosello and barattiere (Cucumis melo L.) grown in a soilless system. J Hortic Sci Biotechnol. (2009) 84:300–4. doi: 10.1080/14620316.2009.11512521
50. D’Imperio M, Buttaro D, Santamaria P, Serio F. Biofortificazione in silicio di spinacio allevato in floating system. In: S Tartarini, L Dondini editors. XII Giornate Scientifiche SOI. Bologna: Università degli Studi di Bologna (2018). p. 91.
51. D’Imperio M, Montesano FF, Renna M, Leoni B, Buttaro D, Parente A, et al. NaCl stress enhances silicon tissue enrichment of hydroponic “baby leaf” chicory under biofortification process. Sci Hortic. (2018) 235:258–63. doi: 10.1016/j.scienta.2018.03.001
52. D’Imperio M, Renna M, Cardinali A, Buttaro D, Serio F, Santamaria P. Calcium biofortification and bioaccessibility in soilless “baby leaf” vegetable production. Food Chem. (2016) 213:149–56. doi: 10.1016/j.foodchem.2016.06.071
53. Borghesi E, Carmassi G, Uguccioni MC, Vernieri P, Malorgio F. Effects of calcium and salinity stress on quality of lettuce in soilless culture. J Plant Nutr. (2013) 36:677–90. doi: 10.1080/01904167.2012.721909
54. Neeser C, Savidov N, Driedger D. Production of hydroponically grown calcium fortified lettuce. Acta Hortic. (2007) 744:317–22. doi: 10.17660/ActaHortic.2007.744.33
55. Lloyd LC, Giaroli G, Taylor D, Tracy DK. Bipolar depression: clinically missed, pharmacologically mismanaged. Ther Adv Psychopharmacol. (2011) 1:153–62. doi: 10.1177/2045125311420752
56. Yeh HL, Tsai SJ. Lithium may be useful in the prevention of Alzheimer’s disease in individuals at risk of presenile familial Alzheimer’s disease. Med Hypotheses. (2008) 71:948–51. doi: 10.1016/j.mehy.2008.03.049
57. Diniz BS, Machado-Vieira R, Forlenza OV. Lithium and neuroprotection: translational evidence and implications for the treatment of neuropsychiatric disorders. Neuropsychiatr Dis Treat. (2013) 9:493–500. doi: 10.2147/NDT.S33086
58. Young W. Review of lithium effects on brain and blood. Cell Transplant. (2009) 18:951–75. doi: 10.3727/096368909X471251
59. Szklarska D, Rzymski P. Is lithium a micronutrient? From biological activity and epidemiological observation to food fortification. Biol Trace Elem Res. (2019) 189:18–27. doi: 10.1007/s12011-018-1455-2
60. Długaszek M, Kłos A, Bertrandt J. Podaż litu w całodziennych racjach pokarmowych studentów. Probl Hig Epidemiol. (2012) 93:867–70.
61. Patel PM, Wallace A, Alexander GV. Transfer values to fruits of Li in tomato and Cd in busm beans. J Plant Nutr. (1980) 2:87–91. doi: 10.1080/01904168009362741
62. USDA.Tomatoes, Red, Ripe, Raw, Year Round Average. (2019). Available online at: https://fdc.nal.usda.gov/fdc-app.html#/food-details/170457/nutrients (accessed June 3, 2021).
63. da Silva RR, de Faria AJG, do Alexandrino GC, Ribeiro EA, dos Santos ACM, Deusdara TT, et al. Enrichment of lithium in lettuce plants through agronomic biofortification. J Plant Nutr. (2019) 42:2102–13. doi: 10.1080/01904167.2019.1648671
64. Rzymski P, Niedzielski P, Siwulski M, Mleczek M, Budzyńska S, Gąsecka M, et al. Lithium biofortification of medicinal mushrooms Agrocybe cylindracea and Hericium erinaceus. J Food Sci Technol. (2017) 54:2387–93. doi: 10.1007/s13197-017-2679-4
65. Mau JL, Tseng YH. Nonvolatile taste components of three strains of Agrocybe cylindracea. J Agric Food Chem. (1998) 46:2071–4. doi: 10.1021/jf971016k
66. USDA.Mushroom, Lion’s Mane. (2021). Available online at: https://fdc.nal.usda.gov/fdc-app.html#/food-details/1750344/nutrients (accessed June 7, 2021).
67. Mleczek M, Siwulski M, Rzymski P, Budzyńska S, Gąsecka M, Kalaè P, et al. Cultivation of mushrooms for production of food biofortified with lithium. Eur Food Res Technol. (2017) 243:1097–104. doi: 10.1007/s00217-016-2823-9
68. Jeznabadi EK, Jafarpour M, Eghbalsaied S. King oyster mushroom production using various sources of agricultural wastes in Iran. Int J Recycl Org Waste Agric. (2016) 5:17–24. doi: 10.1007/s40093-015-0113-3
69. Kurd-Anjaraki S, Ramezan D, Ramezani S, Samzadeh-Kermani A, Pirnia M, Shahi BY. Potential of waste reduction of agro-biomasses through Reishi medicinal mushroom (Ganoderma lucidum) production using different substrates and techniques. Acta Ecol Sin. (2021). 42:90–101. doi: 10.1016/j.chnaes.2021.04.010
70. USDA.Mushroom, Oyster. (2021). Available online at: https://fdc.nal.usda.gov/fdc-app.html#/food-details/1750345/nutrients (accessed June 7, 2021).
71. Faria MGI, Avelino KV, do Valle JS, da Silva GJ, Gonçalves AC, Dragunski DC, et al. Lithium bioaccumulation in Lentinus crinitus mycelial biomass as a potential functional food. Chemosphere. (2019) 235:538–42. doi: 10.1016/j.chemosphere.2019.06.218
72. USDA.Mushrooms, Shiitake. (2021). Available online at: https://fdc.nal.usda.gov/fdc-app.html#/food-details/1750346/nutrients (accessed June 16, 2021).
73. Boland M, Golding M, Harjinder S editors. Food Structures, Digestion and Health. Cambridge, MA: Academic Press (2014). doi: 10.1016/C2012-0-02243-0
74. Powell JJ, Jugdaohsingh R, Thompson RPH. The regulation of mineral absorption in the gastrointestinal tract. Proc Nutr Soc. (1999) 58:147–53. doi: 10.1079/PNS19990020
75. Fernández-García E, Carvajal-Lérida I, Pérez-Gálvez A. In vitro bioaccessibility assessment as a prediction tool of nutritional efficiency. Nutr Res. (2009) 29:751–60. doi: 10.1016/j.nutres.2009.09.016
76. D’Imperio M, Montesano FF, Santovito E, Parente A, Serio F. Mineral composition and bioaccessibility in rocket and purslane after Zn biofortification process. Foods. (2022) 11:484. doi: 10.3390/foods11030484
77. Zou T, Xu N, Hu G, Pang J, Xu H. Biofortification of soybean sprouts with zinc and bioaccessibility of zinc in the sprouts. J Sci Food Agric. (2014) 94:3053–60. doi: 10.1002/jsfa.6658
78. Dong Z, Liu Y, Dong G, Wu H. Effect of boiling and frying on the selenium content, speciation, and in vitro bioaccessibility of selenium-biofortified potato (Solanum tuberosum L.). Food Chem. (2021) 348:129150. doi: 10.1016/j.foodchem.2021.129150
79. Li R, Li DW, Yan AL, Hong CL, Liu HP, Pan LH, et al. The bioaccessibility of iodine in the biofortified vegetables throughout cooking and simulated digestion. J Food Sci Technol. (2018) 55:366–75. doi: 10.1007/s13197-017-2946-4
80. Cunha Coelho R, Fernandes Barsotti RC, França Maltez H, Alves C, Júnior L, De Sousa Barbosa H. Expanding information on the bioaccessibility and bioavailability of iron and zinc in biofortified cowpea seeds. Food Chem. (2021) 347:129027. doi: 10.1016/j.foodchem.2021.129027
81. Glahn RP, Lee OA, Yeung A, Goldman MI, Miller DD. Caco-2 cell ferritin formation predicts nonradiolabeled food iron availability in an in vitro digestion/Caco-2 cell culture model. J Nutr. (1998) 128:1555–61. doi: 10.1093/jn/128.9.1555
82. Thakur N, Raigond P, Singh Y, Mishra T, Singh B, Kumar Lal M, et al. Recent updates on bioaccessibility of phytonutrients. Trends Food Sci Technol. (2020) 97:366–80. doi: 10.1016/j.tifs.2020.01.019
83. Wei Y, Shohag MJI, Yang X. Biofortification and bioavailability of rice grain zinc as affected by different forms of foliar zinc fertilization. PLoS One. (2012) 7:e45428. doi: 10.1371/journal.pone.0045428
84. D’Imperio M, Brunetti G, Gigante I, Serio F, Santamaria P, Cardinali A, et al. Integrated in vitro approaches to assess the bioaccessibility and bioavailability of silicon-biofortified leafy vegetables and preliminary effects on bone. Vitr Cell Dev Biol Anim. (2017) 53:217–24. doi: 10.1007/s11626-016-0100-7
85. Gupta PK, Balyan HS, Sharma S, Kumar R. Biofortification and bioavailability of Zn, Fe and Se in wheat: present status and future prospects. Theor Appl Genet. (2021) 134:1–35. doi: 10.1007/s00122-020-03709-7
86. Koronowicz AA, Kopeć A, Master A, Smoleń S, Piątkowska E, Bieżanowska-Kopeć R, et al. Transcriptome profiling of Caco-2 cancer cell line following treatment with extracts from iodine-biofortified lettuce (Lactuca sativa L.). PLoS One. (2016) 11:e0147336. doi: 10.1371/journal.pone.0147336
87. Delaqua D, Carnier R, Cadore S, Sanches VL, Severiano Berton R, Corbi CA, et al. In vitro bioaccessibility and bioavailability of selenium in agronomic biofortified wheat. J Food Compos Anal. (2022) 105:104253. doi: 10.1016/j.jfca.2021.104253
88. Rein MJ, Renouf M, Cruz-Hernandez C, Actis-Goretta L, Thakkar SK, da Silva Pinto M. Bioavailability of bioactive food compounds: a challenging journey to bioefficacy. Br J Clin Pharmacol. (2013) 75:588–602. doi: 10.1111/j.1365-2125.2012.04425.x
89. European Food Safety Authority.Tolerable Upper Intake Levels for Vitamins and Minerals. (2006). Available online at: http://www.efsa.europa.eu/sites/default/files/efsa_rep/blobserver_assets/ndatolerableuil.pdf (accessed May 3, 2018).
90. Pizzutelli S. Systemic nickel hypersensitivity and diet: myth or reality? Eur Ann Allergy Clin Immunol. (2011) 43:5–18.
91. Lapietra Company.Nickel-Free Product Certificate. (2019). Available online at: https://fratellilapietra.it/wp-content/uploads/2021/05/nickel-free.pdf (accessed June 17, 2021).
93. Calvo JL, Alorda-Capo F, Pareja-Galeano H, Jiménez SL. Influence of nitrate supplementation on endurance cyclic sports performance: a systematic review. Nutrients. (2020) 12:1796. doi: 10.3390/nu12061796
94. Santamaria P. Nitrate in vegetables: toxicity, content, intake and EC regulation. J Sci Food Agric. (2006) 86:10–7. doi: 10.1002/jsfa.2351
95. European Commission. COMMISSION REGULATION (EU) No 1258/2011 of 2 December 2011 amending Regulation (EC) No 1881/2006 as regards maximum levels for nitrates in foodstuffs. Off J Eur Union. (2011) L 320:15–7. doi: 10.2903/j.efsa.2010.1935
96. Santamaria P, Gonnella M, Elia A, Parente A, Serio F. Ways of reducing rocket salad nitrate content. Acta Hortic. (2001) 548:529–36. doi: 10.17660/ActaHortic.2001.548.64
97. Buttaro D, Renna M, Gerardi C, Blando F, Santamaria P, Serio F. Soilless production of wild rocket as affected by greenhouse coverage with photovoltaic modules. Acta Sci Pol Cultus. (2016) 15:129–42.
98. San Juan AF, Dominguez R, Lago-Rodríguez Á, Montoya JJ, Tan R, Bailey SJ. Effects of dietary nitrate supplementation on weightlifting exercise performance in healthy adults: a systematic review. Nutrients. (2020) 12:1–16. doi: 10.3390/nu12082227
99. European Commission.Farm to Fork Strategy. (2022). Available online at: https://ec.europa.eu/food/horizontal-topics/farm-fork-strategy_en (accessed March 2, 2022).
Keywords: in vitro digestion model, bioavailability, bone health, impaired kidney function, mental illnesses, modulated nutrition
Citation: Renna M, D’Imperio M, Maggi S and Serio F (2022) Soilless biofortification, bioaccessibility, and bioavailability: Signposts on the path to personalized nutrition. Front. Nutr. 9:966018. doi: 10.3389/fnut.2022.966018
Received: 10 June 2022; Accepted: 20 September 2022;
Published: 04 October 2022.
Edited by:
Prisco Piscitelli, Istituto Scientifico Biomedico Euro Mediterraneo (ISBEM), ItalyReviewed by:
Bahar Yildiz Kutman, Gebze Technical University, TurkeyAnna Bonasia, University of Foggia, Italy
Dunyi Liu, Southwest University, China
Copyright © 2022 Renna, D’Imperio, Maggi and Serio. This is an open-access article distributed under the terms of the Creative Commons Attribution License (CC BY). The use, distribution or reproduction in other forums is permitted, provided the original author(s) and the copyright owner(s) are credited and that the original publication in this journal is cited, in accordance with accepted academic practice. No use, distribution or reproduction is permitted which does not comply with these terms.
*Correspondence: Massimiliano D’Imperio, bWFzc2ltaWxpYW5vLmRpbXBlcmlvQGlzcGEuY25yLml0