- 1Laboratorio de Investigacao Medica 12, Faculdade de Medicina, Universidade de São Paulo, São Paulo, Brazil
- 2Laboratorio de Investigacao Medica 12, Hospital das Clinicas HCFMUSP, Faculdade de Medicina, Universidade de São Paulo, São Paulo, Brazil
Acute kidney injury (AKI) alters renal hemodynamics, leading to tubular injury, activating pathways of inflammation, proliferation, and cell death. The initial damage caused to renal tissue after an ischemia/reperfusion (I/R) injury exerts an important role in the pathogenesis of the course of AKI, as well as in the predisposition to chronic kidney disease. Vitamin D deficiency has been considered a risk factor for kidney disease and it is associated with tubulointerstitial damage, contributing to the progression of kidney disease. Obesity is directly related to diabetes mellitus and hypertension, the main metabolic disorders responsible for the progression of kidney disease. Furthermore, the expansion of adipose tissue is described as an important factor for increased secretion of pro-inflammatory cytokines and their respective influence on the progression of kidney disease. We aimed to investigate the influence of vitamin D deficiency and obesity on the progression of renal disease in a murine model of renal I/R. Male Wistar rats underwent renal I/R surgery on day 45 and followed until day 90 of the protocol. We allocated the animals to four groups according to each diet received: standard (SD), vitamin D-depleted (VDD), high fat (HFD), or high fat vitamin D-depleted (HFDV). At the end of 90 days, we observed almost undetectable levels of vitamin D in the VDD and HFDV groups. In addition, HFD and HFDV groups presented alterations in the anthropometric and metabolic profile. The combination of vitamin D deficiency and obesity contributed to alterations of functional and hemodynamic parameters observed in the HFDV group. Moreover, this combination favored the exacerbation of the inflammatory process and the renal expression of extracellular matrix proteins and phenotypic alteration markers, resulting in an enlargement of the tubulointerstitial compartment. All these changes were associated with an increased renal expression of transforming growth factor β and reduced expression of the vitamin D receptor. Our results show that the synergistic effect of obesity and vitamin D deficiency exacerbated the hemodynamic and morphological changes present in the evolution of renal disease induced by I/R.
Introduction
Over the last few decades, there has been a growing interest in risk factors related to the progression of kidney disease. This fact is due to the high prevalence of chronic kidney disease (CKD) and its high costs, as well as the risk of progression to end-stage renal disease (1–3). In addition to advanced age, gender, and family history, there are other traditional and common risk factors related to the progression of kidney disease, including diabetes mellitus (DM), hypertension, obesity, and cardiovascular diseases (CVD) (3–5). Furthermore, non-traditional risk factors such as hypovitaminosis D has been considered as an aggravating feature regarding the evolution of kidney disease (2, 6–9). It is well known that kidney diseases are accompanied by decreased levels of vitamin D (9, 10), which impair the crucial role of the kidney in vitamin D metabolism. As a consequence, hypovitaminosis D disarranges the regulation of numerous physiological activities, including the renoprotection performed by that hormone (9, 10).
An increasing number of studies have been demonstrating a relation between hypovitaminosis D and anthropometric status (11–15). In 2015, a meta-analysis showed that the prevalence of vitamin D deficiency in obese and overweight individuals was, respectively, 35 and 24% higher when compared to lean subjects (16). This association between body mass index (BMI) and hypovitaminosis D is also described in sunny countries. Unger et al. observed a high prevalence of hypovitaminosis D in healthy Brazilian adults and showed a negative association between serum levels of vitamin D and BMI (17). Corroborating those data, Bolland et al. suggested a possible link involving the sequestration and deposition of vitamin D in the adipose tissue and the lower sun exposure by choice and lifestyle (17, 18). Moreover, it has been described that hypovitaminosis D in obesity occurs independently of factors, such as age, ethnicity, gender, and sun exposure (16, 19). In the 1970s, Rosenstreich et al. experimentally showed that adipose tissue had a greater storage capacity for the different forms of vitamin D compared to other organs and tissues (20). In 2000, Wortsman et al. demonstrated a lower bioavailability of vitamin D in obese patients compared to eutrophic individuals after acute ingestion of ergocalciferol or phototherapy session (21).
It is acknowledged that adipose tissue is not just a fat reservoir, but a dynamic tissue involved in the production of adipokines, including leptin, adiponectin, tumor necrosis factor α (TNF-α), chemotactic protein for monocytes 1 (MCP-1), transforming growth factor β (TGF-β), angiotensin II (Ang II), and endothelin-1 (22, 23). This endocrine action of adipose tissue generates oxidative stress, activates the renin-angiotensin-aldosterone system (RAAS), and promotes insulin resistance with subsequent abnormal production/accumulation of lipids (23–25). In addition, obesity has been linked to inflammation and is considered a risk factor for a decline in renal function (25, 26). Based on the information regarding the low levels of vitamin D in the course of renal diseases and the impaired bioavailability of this hormone in obese individuals, we aimed to study the influence of vitamin D deficiency and obesity in rats submitted to renal ischemia-reperfusion injury (IRI).
Materials and methods
Animals
Male Wistar rats (Rattus novergicus), weighing 180–200 g, were provided by the animal facility from the Institute of Biomedical Sciences–University of São Paulo. During the 90-day protocol, we kept our animals at a controlled temperature (23 ± 1°C) with a light/dark cycle of 12/12 h. All the experiments followed our institutional guidelines and were approved by the local Research Ethics Committee (CEUA, registration 1438/2020).
Diets
We used four different types of diet in this experimental protocol: (1) standard diet (20% protein, 70% carbohydrates, and 10% lipids); (2) standard diet depleted in vitamin D (20% protein, 70% carbohydrates, 10% lipids, and vitamin D-free); (3) high-fat diet (20% protein, 35% carbohydrates and 45% lipids); and (4) high-fat diet depleted in vitamin D (20% protein, 35% carbohydrates, 45% lipids, and vitamin D-free)—purchased from PragSoluções Biociências (Jaú, São Paulo, Brazil). The animals were placed in cages according to their diet, with free access to water.
Experimental protocol
We allocated the rats to four groups according to each type of diet: Standard (SD, n = 8), fed the standard diet for 90 days; Vitamin D deficient (VDD, n = 9), fed the vitamin D-free diet for 90 days; High-fat (HFD, n = 10), fed the high-fat diet for 90 days; and High-fat vitamin D deficient (HFDV, n = 10), fed the high-fat vitamin D-free diet for 90 days. On day 45, all the rats were anesthetized with 2,2,2-tribromoethanol [250 mg/Kg body weight (BW)]. Subsequently, a suprapubic incision was made for induction of renal IRI by clamping both renal arteries for 45 min, followed by reperfusion.
Analysis of urine samples
Before the clearance studies, all the rats were placed in individual metabolic cages, on a 12/12-h light/dark cycle, with free access to drinking water. We collected 24-h urine to assess urinary output and then centrifuged the samples to remove suspended material. We evaluated urinary protein excretion by colorimetric assay (Labtest Diagnóstica, Minas Gerais, Brazil).
Anthropometry
On day 90, we evaluated anthropometric measurements in the animals under anesthesia just before the inulin clearance experiment. We used a sterile non-extensible measuring tape to assess: body length (cm), from the nostrils to the beginning of the tail (nose-to-anus); abdominal circumference (cm), taking the largest zone of the abdomen as the reference; and thoracic circumference (cm), immediately behind the foreleg (27, 28). We determined the BMI by dividing the body weight (g) by the body length squared (cm2) (29).
Inulin clearance and hemodynamic studies
On day 90, we anesthetized the animals with sodium thiopental (50 mg/Kg BW) and then we cannulated the trachea with a PE-240 catheter for spontaneous breathing. The jugular vein was cannulated with a PE-60 catheter for infusion of inulin and fluids. To monitor mean arterial pressure (MAP) and collect blood samples, the right femoral artery was catheterized with a PE-50 catheter. We assessed MAP with a data acquisition system (MP100; Biopac Systems, Santa Barbara, CA). To collect urine samples, we cannulated the bladder with a PE-240 catheter by suprapubic incision. After the surgical procedure, a loading dose of inulin (100 mg/Kg BW diluted in 1 mL of 0.9% saline) was administered through the jugular vein. A constant infusion of inulin (10 mg/Kg BW) was started and continued at 0.04 mL/min throughout the whole experiment. We collected three urine samples at 30-min intervals. Blood samples were obtained at the beginning and at the end of the experiment. Inulin clearance values represent the mean of three periods. Plasma and urinary inulin were determined by the anthrone method, and the glomerular filtration rate (GFR) data were expressed as mL/min/100 g BW. To measure renal blood flow (RBF), we made a median incision and dissected the left renal pedicle for isolating the renal artery. An ultrasonic flow probe was placed around the exposed renal artery, and RBF was measured (mL/min) with an ultrasonic flow meter (T402; Transonic Systems, Bethesda, MD). We divided blood pressure by RBF to calculate renal vascular resistance (RVR, mmHg/mL/min).
Biochemical parameters
We collected blood samples after the clearance studies to assess plasma levels of 25-hydroxyvitamin D [25(OH)D], parathormone (PTH), aldosterone, Ang II, total cholesterol (cholesterol), triglycerides, glucose, leptin, phosphate (PP), and calcium (PCa). We assessed 25(OH)D, PTH, aldosterone, Ang II, and leptin by enzyme-linked immunosorbent assay (ELISA) using commercial kits: 25-hydroxyvitamin D (ALPCO, Salem, NH, USA); Rat Intact PTH (Immutopics, Inc., San Clemente, CA, USA); Aldosterone (Enzo Life Sciences, Farmingdale, NY, USA); Rat angiotensin II (Elabscience, Houston, TX, USA); Leptin (EMD Millipore, St. Louis, MO, USA). We measured PCa, PP, and glucose by colorimetric assay (Labtest Diagnóstica, Minas Gerais, Brazil). Plasma levels of cholesterol and triglycerides were determined by specific electrodes (ABL800Flex—Radiometer, Brønshøj, Denmark).
Tissue samples preparation
After the blood samples collection, we perfused the kidneys with a phosphate-buffered solution (PBS, pH 7.4). We froze the right kidneys in liquid nitrogen and stored them at –80°C for western blotting, ELISA, and real-time quantitative polymerase chain reaction (qPCR). The left kidneys were removed and a fragment of the renal tissue was fixed in methacarn solution (60% methanol, 30% chloroform, 10% glacial acetic acid) for 24 h and replaced by 70% alcohol thereafter. The kidney blocks were embedded in paraffin and cut into 4-μm sections for histological and immunohistochemical (IHC) studies.
Total protein isolation
Kidney samples were homogenized in ice-cold isolation solution (200 mM mannitol, 80 mM HEPES, and 41 mM KOH, pH 7.5) containing a protease inhibitor cocktail (Sigma Chemical Company, St. Louis, MO, USA) with a homogenizer (Tissue Master TM125, Omni International, Kennesaw, GA, USA). Homogenates were centrifuged at 4,000 × rpm for 30 min at 4°C to remove nuclei and cell debris. Supernatants were isolated, and protein was quantified by Bradford assay (Bio-Rad Laboratories, Hercules, CA, USA).
Western blot assay
For western blot analysis, 100 μg of total kidney protein was separated on SDS-polyacrylamide minigels by electrophoresis (30). After a transfer by electroelution to PVDF membranes (GE Healthcare Limited, Little Chalfont, UK), blots were blocked for 1 h with 5% non-fat milk in a Tris-buffered saline solution. Blots were then incubated overnight with a primary antibody for anti-VDR (1:500; Santa Cruz Biotechnology, Santa Cruz, CA, USA). The labeling was visualized with a horseradish peroxidase-conjugated secondary antibody (anti-mouse, 1:2,000; Sigma Chemical, St. Louis, MO, USA) and enhanced chemiluminescence detection (GE Healthcare Limited, Little Chalfont, UK). Kidney protein levels were further analyzed with a gel documentation system (Alliance 4.2; Uvitec, Cambridge, UK) and the software Image J for Windows (Image J-NIH Image). We used densitometry to quantitatively analyze the protein levels, normalizing the bands to β-actin expression (anti-β-actin, Sigma Chemical, St. Louis, MO, USA).
Enzyme-linked immunosorbent assay in renal tissue
We assessed collagen type 3 (Col-3), Ang II, and MCP-1 in renal tissue by ELISA using commercial kits (Elabscience, Houston, TX, USA). The detection system and the quantification followed the protocols described by the manufacturer. The absorbances were obtained using the Epoch/2 device (Biotek Instruments, Winooski, VE, USA).
Light microscopy
Four-μm histological sections of kidney tissue were stained with Masson’s trichrome and examined under light microscopy. We quantified the fractional interstitial area (FIA) by analyzing tubulointerstitial involvement and glomerular tuft area as well. For histomorphometry, the images obtained using microscopy were captured on a computer screen via an image analyzer software (ZEN, Carl Zeiss, Munich, Germany). For FIA evaluation, we analyzed 30–40 grid fields (0.09 mm2 each) per kidney cortex. The interstitial areas were manually demarcated, and the proportion of the field was determined after excluding the glomeruli. For the glomerular area, we calculated the arithmetic mean after analyzing approximately 80 glomeruli per kidney section. The glomerular tuft area (μm2) was manually circulated and automatically calculated by the software. We minimized bias during the morphometric analysis by keeping the observer blinded to the treatment groups.
Immunohistochemical analysis
Immunohistochemistry was performed on 4-μm-thick paraffinized kidney sections mounted on 2% silane-coated glass slides. We used the following antibodies: mouse monoclonal to CD68 (ED1, 1:100; BioRad, Hercules, CA, USA); rabbit polyclonal to mannose receptor (CD206, 1:2,000; Abcam, Cambridge, MA, USA); mouse monoclonal to CD3 (1:50; Dako, Glostrup, Denmark); mouse monoclonal to α-smooth muscle actin (α-SMA, 1:200; Millipore, Billerica, MA, USA); rabbit monoclonal to fibronectin (1:400; Abcam, Cambridge, MA, USA); mouse monoclonal to vimentin (1:100; Dako, Glostrup, Denmark); rabbit polyclonal to TGF-β1 (1:100; Santa Cruz Biotechnology, Santa Cruz, CA, USA); mouse monoclonal to proliferating nuclear cell antigen (PCNA, 1:50; Dako, Glostrup, Denmark); and mouse monoclonal to JG12, direct against to aminopeptidase P (1:100; Santa Cruz Biotechnology, Santa Cruz, CA, USA). We subjected the kidney tissue sections to IHC reaction according to the protocol for each primary antibody. Reaction products were detected by anti-rabbit or mouse EnVision+ System™ and the color reaction was developed in 3,3-diaminobenzidine (Dako North America, Carpinteria, CA, USA). Counterstaining was with Harris’ hematoxylin. We analyzed 30–40 renal cortex fields (0.09 mm2) to evaluate the immunoreactions. The volume ratios of positive areas of renal tissue (%), determined by the color limit, were obtained by ZEN image analyzer software (Carl Zeiss, Munich, Germany) on a computer coupled to a microscope (Carl Zeiss Axioskop 40) and a digital camera (2, 31). To minimize bias during the IHC analysis, the observer was blinded to the treatment groups.
Gene expression
We performed real-time qPCR in frozen adipose tissue assessing VDR gene (Rn00690616_m1). Firstly, we extracted and prepared total RNA by centrifugation technique using the commercial kit SV Total RNA Isolation System (Promega Corporation, Madison, WI, USA). Next, we determined the quantity and quality of RNA by Nanodrop™. We used 1 ul of RNA to prepare the cDNA following the manufacturer’s instructions of the GoScript Reverse Transcription System (Promega Corporation, Madison, WI, USA) and quantified again by Nanodrop™. We performed real-time PCR in 2 μl of cDNA (50 ng) using GoTaq Probe qPCR Master Mix (Promega Corporation, Madison, WI, USA) and TaqMan on Step One Plus (both from Applied Biosystems, Foster City, CA, USA). We evaluated relative gene expression with the 2–ΔΔCt method (32) using glyceraldehyde 3-phosphate dehydrogenase (GAPDH) as the housekeeping gene (Rn01775763_g1).
Statistical analysis
All data were expressed as mean ± SEM (standard error of the mean). Differences among groups were analyzed with GraphPad Prism 5.0 software (GraphPad Software, La Jolla, CA) by one-way analysis of variance followed by the Student–Newman–Keuls test. Values of p < 0.05 were considered statistically significant.
Results
Anthropometric parameters
We evaluated anthropometric data on day 90 of the experimental protocol. As expected, we observed a significant difference (p < 0.001) regarding the body weight of rats fed high-fat diets (HFD and HFDV) when compared to rats fed a standard diet (SD) or vitamin D-free diet (VDD) (Table 1). In addition, the body weight gain profile from HFD and HFDV groups reflected on the differences observed concerning the assessment of BMI (g/cm2), AC (cm), and TC (cm), as shown in Table 1. These results demonstrate that high-fat diets were effective in the experimental development of obesity. We did not find any difference in the length (cm) of the animals, characterizing a homogeneous and adequate growth in relation to the age and period studied (Table 1).
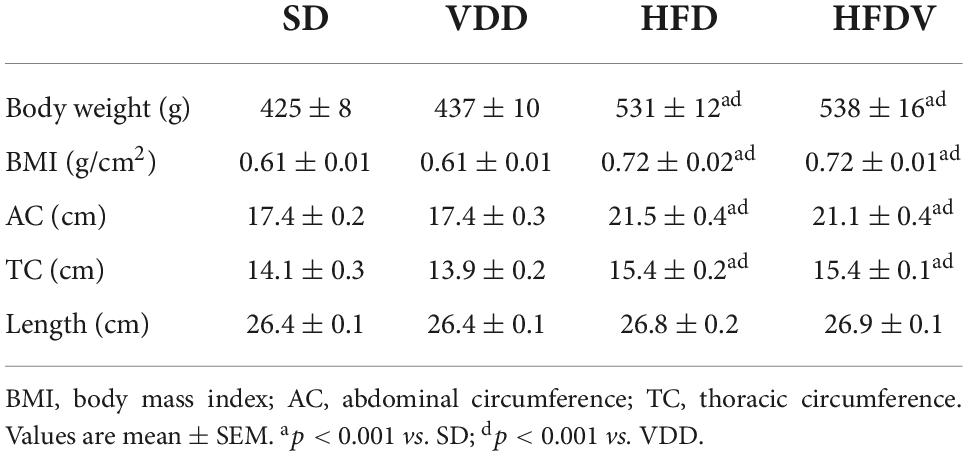
Table 1. Anthropometric parameters evaluated after the 90-day protocol in rats submitted to renal ischemia-reperfusion insult on day 45 treated with standard diet (SD), vitamin D-free diet (VDD), high-fat diet (HFD), or high-fat vitamin D-free diet (HFDV).
Vitamin D, parathormone, and metabolic profile
We evaluated plasma concentration of 25(OH)D at 45 and 90 days of the protocol. We observed significantly lower levels (p < 0.001) of that hormone on day 45 in the animals that received vitamin D-depleted diets (VDD and HFDV) when compared to SD and HFD animals. These results confirm that those animals were deficient in vitamin D at the time they were submitted to renal IRI. As expected, we found almost undetectable levels of vitamin D in VDD and HFDV groups on day 90 (Figure 1). In addition, we noted that the HFD group presented sufficient but lower levels of vitamin D compared to the SD group (Figure 1). Although without significant differences among the groups, we observed an evident upward tendency in plasma levels of PTH (pg/mL) on day 90 in the vitamin D deficient groups (VDD and HFDV) in relation to SD and HFD groups (Table 2). We did not find any difference regarding plasma phosphate and calcium levels (data not shown).
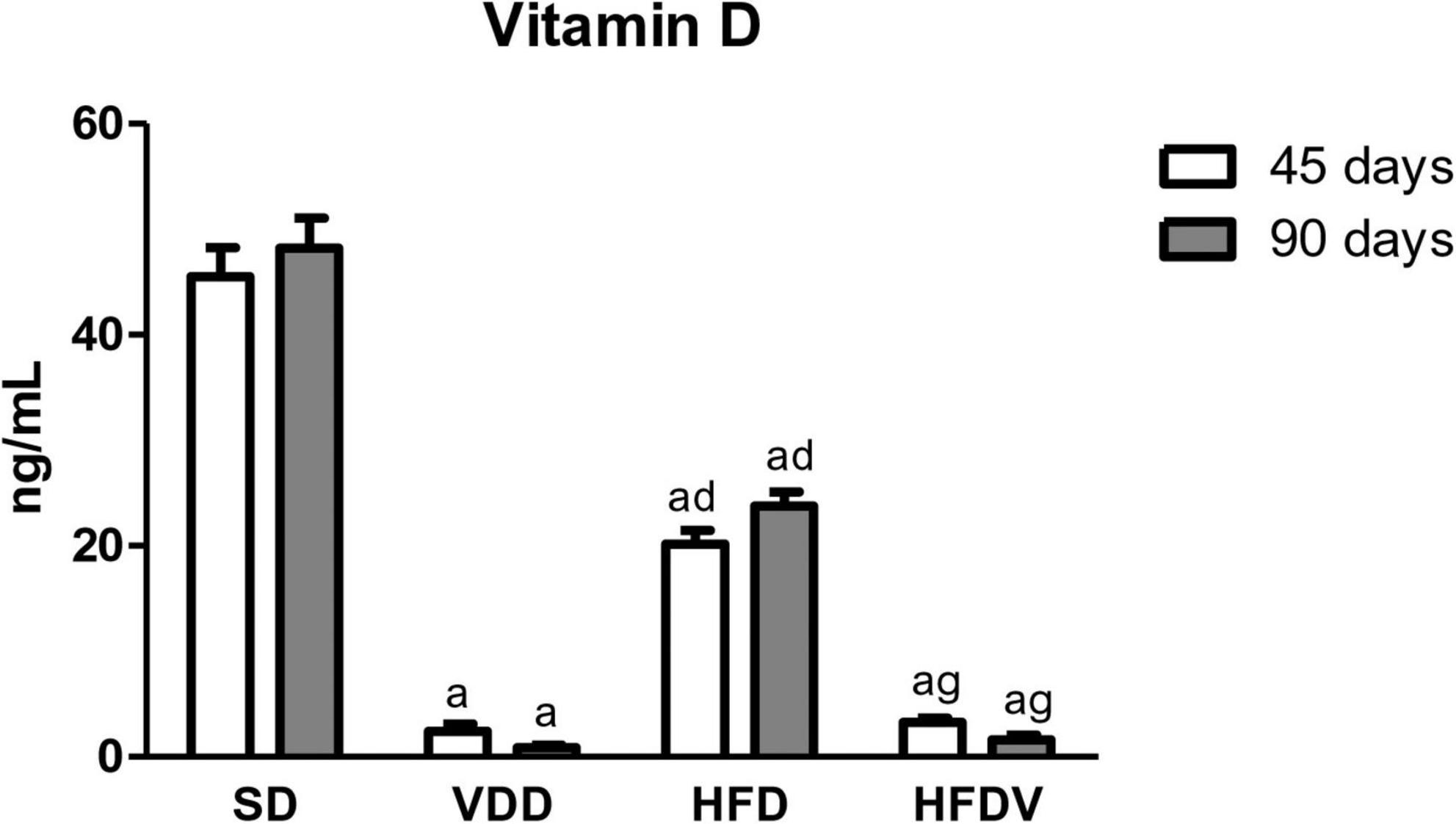
Figure 1. Plasma 25(OH)D levels evaluated at 45 and 90 days of protocol in rats submitted to renal ischemia-reperfusion insult on day 45 treated with standard diet (SD), vitamin D-free diet (VDD), high-fat diet (HFD), or high-fat vitamin D-free diet (HFDV). Data are mean ± SEM. ap < 0.001 vs. SD; dp < 0.001 vs. VDD; gp < 0.001 vs. HFD.
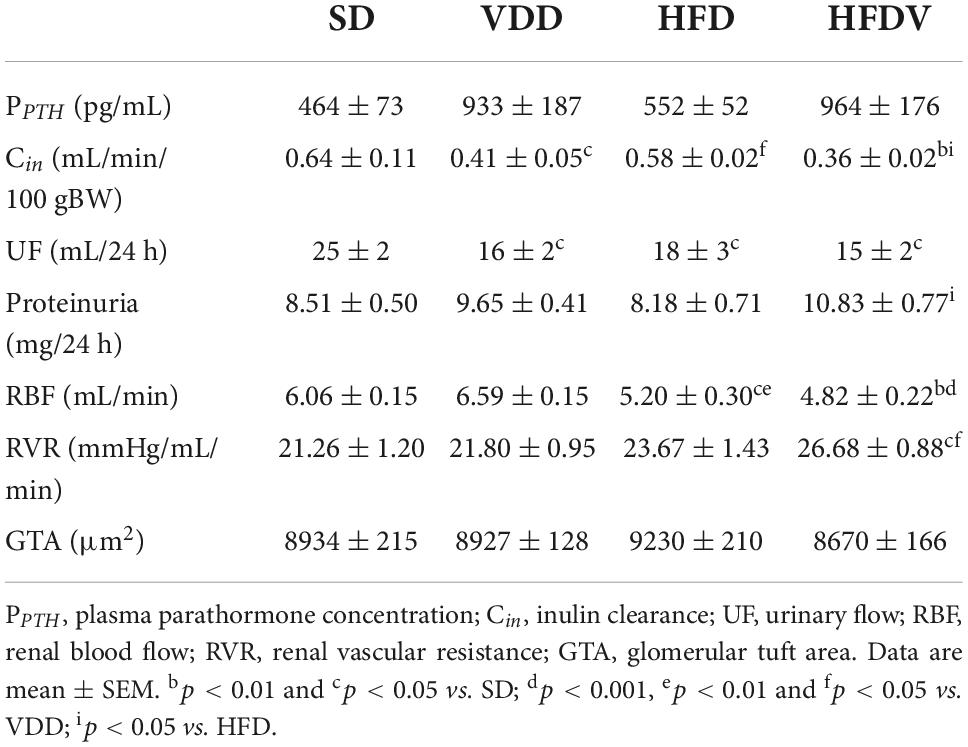
Table 2. Renal function, biochemical parameters, and glomerular tuft area evaluated after the 90-day protocol in rats submitted to renal ischemia-reperfusion insult on day 45 treated with standard diet (SD), vitamin D-free diet (VDD), high-fat diet (HFD), or high-fat vitamin D-free diet (HFDV).
The analysis regarding lipid profile allowed us to observe isolated and synergistic actions of both high-fat and vitamin D-free diets on cholesterol and triglyceride levels. The evaluation of cholesterol levels (mg/dL) on day 90 showed a slight upward tendency in the VDD group in relation to the SD group. However, HFD group presented a significant increase concerning cholesterol levels compared to SD (p < 0.001) and VDD (p < 0.05) groups (Figure 2A). Furthermore, our results show that the vitamin D-free diet promoted a significant increase (p < 0.05) in triglyceride levels (mg/dL) in the VDD group compared to the SD group. We also observed higher and more significant triglyceride levels in the HFD group compared to the SD (p < 0.001) and VDD (p < 0.01) groups. Of note, the HFDV group presented higher levels of cholesterol and triglycerides in relation to all the other groups, demonstrating an evident imbalance of the lipid profile in vitamin D deficiency associated with obesity (Figure 2B). In addition, the results of fasting blood glucose (mg/dL) showed an upward tendency for this parameter in the VDD and HFD groups in relation to the SD group (Figure 2C). The animals from the HFDV group showed a noteworthy and significant increase in fasting blood glucose compared to all the other groups (Figure 2C). As expected, we observed a significant increase (p < 0.001) in plasma leptin levels (ng/mL) in the HFD and HFDV groups when compared to the SD and VDD groups (Figure 2D). This alteration was even more remarkable in the HFDV group, with a significant increase in comparison to all the other groups.
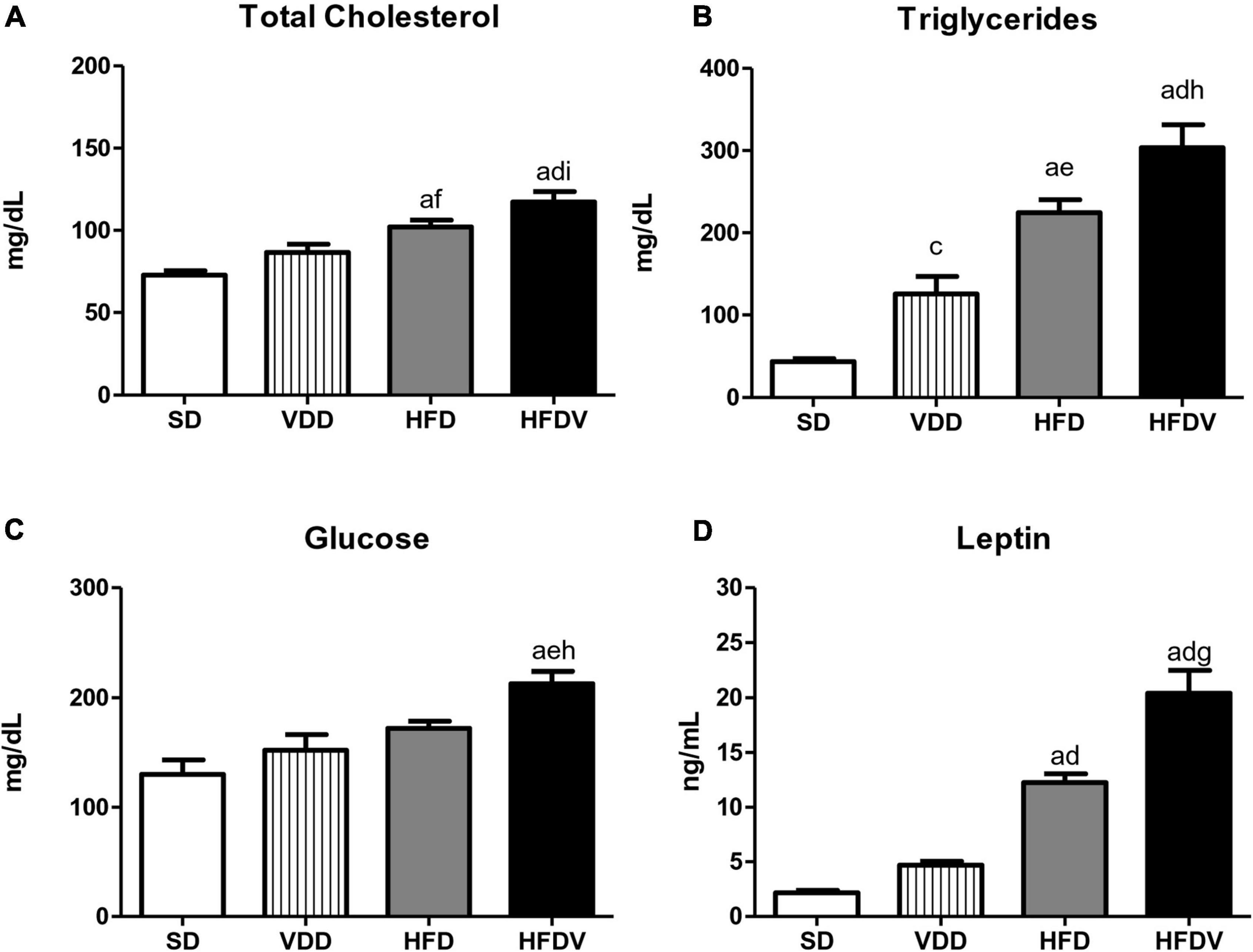
Figure 2. Plasma concentrations of (A) total cholesterol, (B) triglycerides, (C) glucose, and (D) leptin evaluated after the 90-day protocol in rats submitted to renal ischemia-reperfusion insult on day 45 treated with standard diet (SD), vitamin D-free diet (VDD), high-fat diet (HFD), or high-fat vitamin D-free diet (HFDV). Data are mean ± SEM. ap < 0.001 and cp < 0.05 vs. SD; dp < 0.001, ep < 0.01 and fp < 0.05 vs. VDD; gp < 0.001, hp < 0.01 and ip < 0.05 vs. HFD.
Renal function and hemodynamic analysis
Our inulin clearance studies showed that the vitamin D-free diet associated or not with the high-fat diet modified the renal function. We observed a lower GFR (mL/min/100 g BW) in the VDD group (p < 0.05) compared to the SD group. This alteration was more evident in the HFDV group, which presented a lower GFR in comparison to the SD (p < 0.01) and HFD (p < 0.05) groups (Table 2). Regarding the urinary flow (mL/24 h), we found a lower urinary output (p < 0.05) in the VDD, HFD, and HFDV groups compared to the SD group (Table 2). In addition, we observed a slight upward tendency in proteinuria from VDD group in relation to SD and HFD groups and a significant increase (p < 0.05) of this parameter in the HFDV group compared to the HFD group (Table 2).
Our VDD, HFD and HFDV groups presented a higher MAP (mmHg) than the SD group. Supporting this data, we noticed a similar profile regarding plasma Ang II and aldosterone levels observed in the VDD, HFD, and HFDV groups in comparison to the SD group. Corroborating those findings, our results regarding the evaluation of renal expression of Ang II (pg/μg) showed an upward tendency in the amount of this polypeptide in the VDD, HFD, and HFDV groups in relation to the SD group. It is important to highlight that those alterations were more evident in the HFDV group (Figure 3).
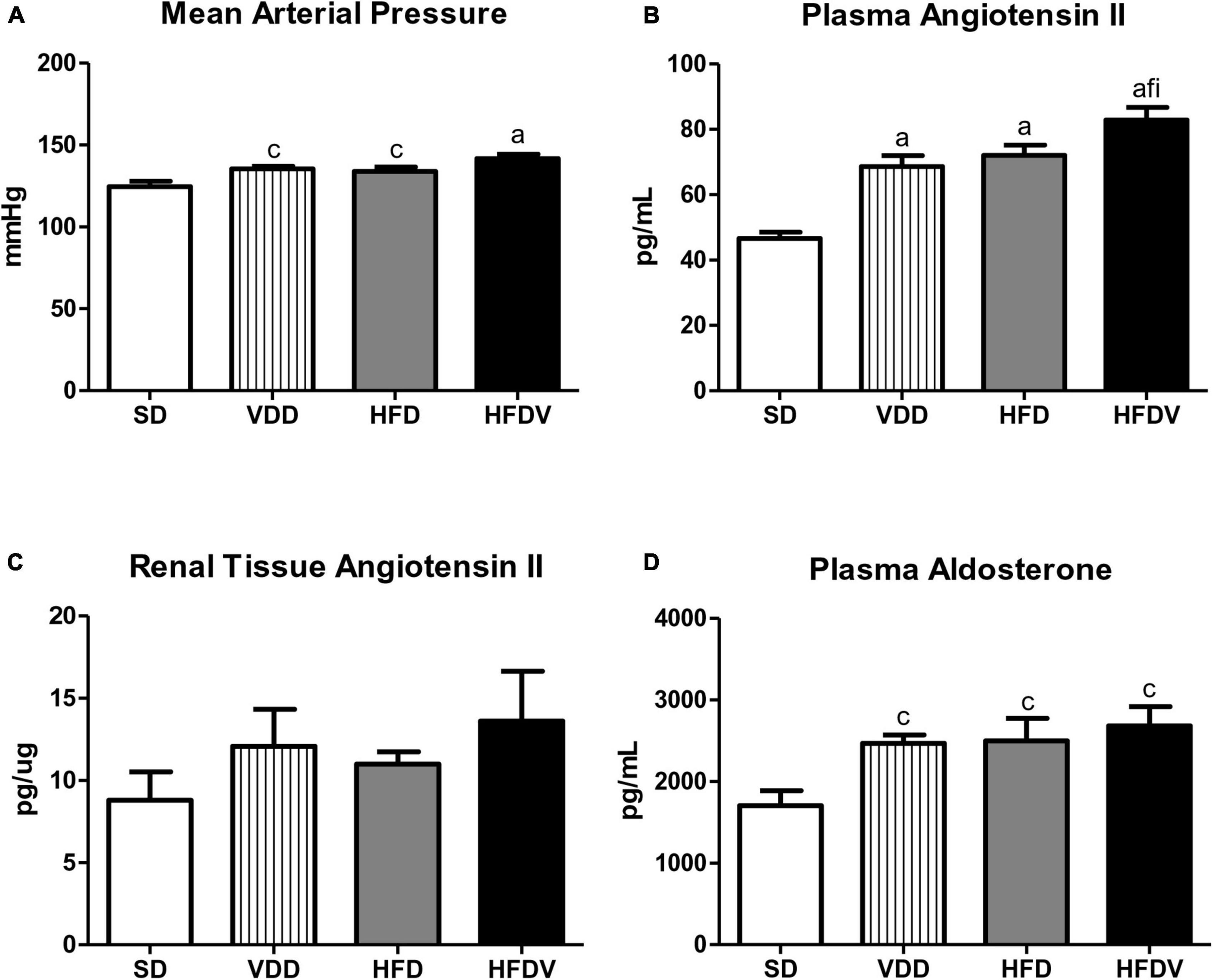
Figure 3. (A) Mean arterial pressure (MAP), (B) plasma angiotensin II concentration, (C) quantitative amount of angiotensin II in renal tissue, and (D) plasma aldosterone concentration evaluated after the 90-day protocol in rats submitted to renal ischemia-reperfusion insult on day 45 treated with standard diet (SD), vitamin D-free diet (VDD), high-fat diet (HFD), or high-fat vitamin D-free diet (HFDV). Data are mean ± SEM. ap < 0.001 and cp < 0.05 vs. SD; fp < 0.05 vs. VDD; ip < 0.05 vs. HFD.
We also observed the influence of high-fat diets regarding RBF and RVR. HFD and HFDV groups presented a lower RBF (mL/min) than SD and VDD groups (Table 2). In addition, the HFD group showed a slight upward tendency in the RVR (mmHg/mL/min), while the HFDV group presented a significant increase (p < 0.05) of this parameter in relation to SD and VDD groups (Table 2).
Vitamin D receptor expression and inflammation
We evaluated VDR protein expression in kidney tissue and VDR gene expression in the adipose tissue at the end of the 90-day protocol. The renal protein expression of VDR (%) was lower (p < 0.001) in the groups of animals that received the vitamin D-free diets (VDD and HFDV) when compared to the SD group (Figures 4A,B). Even with vitamin D deficiency, we observed a higher renal expression (p < 0.01) of VDR in the HFDV group than in the VDD group (Figures 4A,B). In addition, our results regarding real-time qPCR showed a downward tendency in VDR gene expression (ΔΔCt) in the adipose tissue from HFD and HFDV groups in relation to the SD group. Also, we noticed a significantly lower (p < 0.001) gene expression of VDR in the VDD group when compared to the SD group (Figure 4C).
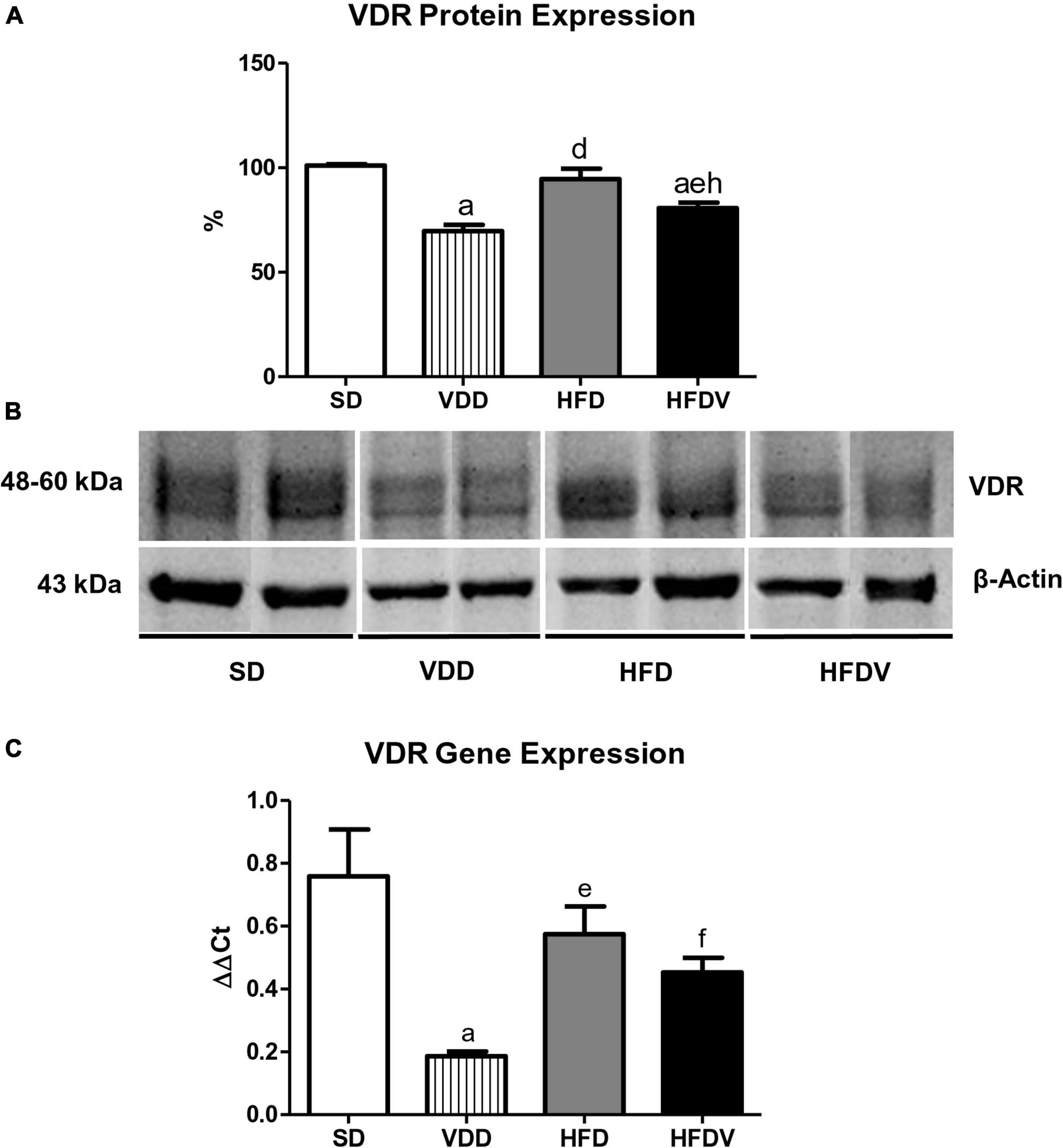
Figure 4. Semiquantitative immunoblotting for renal vitamin D receptor (VDR) protein expression and RT qPCR for adipose tissue VDR gene expression evaluated after the 90-day protocol in rats submitted to renal ischemia-reperfusion insult on day 45 treated with standard diet (SD), vitamin D-free diet (VDD), high-fat diet (HFD), or high-fat vitamin D-free diet (HFDV). (A) Densitometric analysis of samples from an SD, VDD, HFD, and HFDV rat. (B) Representative immunoblots reacted with anti-VDR revealing a 51 kDa band. (C) Bar graph of VDR gene expression values. Data are mean ± SEM. ap < 0.001 vs. SD; dp < 0.001, ep < 0.01 and fp < 0.05 vs. VDD; hp < 0.01 vs. HFD.
It is well known that vitamin D and adipose tissue are closely related to inflammation status. Based on that, we firstly evaluated the renal amount of MCP-1 (ng/μg protein) by ELISA and the renal expression of CD3+ and CD68+ cells (T cells and macrophages, respectively) by IHC studies (%). As shown in Figure 5A, we found a higher MCP-1 amount (p < 0.001) in the renal tissue from VDD and HFD compared to the SD group. This alteration was more evident in the HFDV group, which showed a significant increase in the renal amount of MCP-1 when compared to all the other groups (Figure 5A). Similarly, Figures 5B,C show a higher renal expression of CD3+ cells (p < 0.001) in the HFDV compared to all the other groups. Our next step was focused on the macrophage infiltration in the renal cortex. By using an anti-CD68 antibody we immunolocalized the M1 and M2 macrophages, also known to express the glycoprotein ED1 on their lysosomal membrane (33). As illustrated in Figures 6A,D, we observed a higher expression of CD68+ cells (p < 0.05) in the renal cortex from the VDD, HFD, and HFDV groups when compared to the SD group. Furthermore, for knowledge and differentiation between the macrophage subtypes, we evaluated the proportion of CD206+ cells (M2 macrophages) in relation to the whole amount of macrophages stained with CD68 (M1+M2 macrophages, Figure 6C). CD206 is also known as mannose receptor, which is an exclusive marker for M2 macrophages (1, 34). Although without significance among the groups, we observed a downward tendency in the expression of CD206+ cells in the VDD, HFD, and HFDV groups in relation to the SD group (Figures 6B,E), reinforcing the role of vitamin D and adipose tissue on the modulation of renal inflammation.
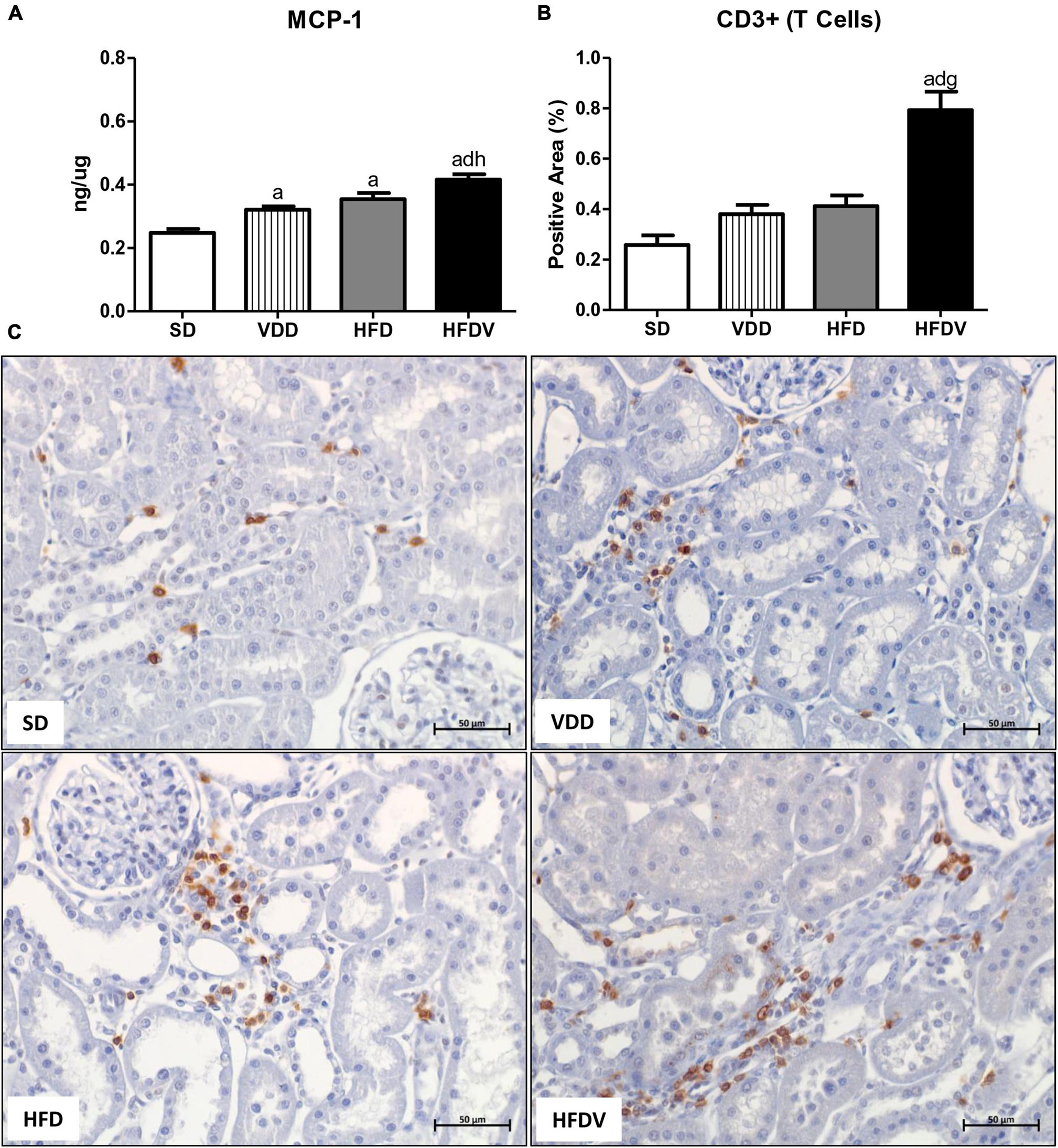
Figure 5. Quantitative amount of monocyte chemotactic protein 1 (MCP-1)—ELISA and immunohistochemical analysis for CD3+ cells (T cells) expression in renal tissue evaluated after the 90-day protocol in rats submitted to renal ischemia-reperfusion insult on day 45 treated with standard diet (SD), vitamin D-free diet (VDD), high-fat diet (HFD), or high-fat vitamin D-free diet (HFDV). (A) Bar graphs of MCP-1 quantification and (B) CD3+ cells expression values. (C) Representative photomicrographs of immunostaining for CD3+ cells in the renal cortex from an SD, VDD, HFD, and HFDV rat (×400). Data are mean ± SEM. ap < 0.001 vs. SD; dp < 0.001 vs. VDD; gp < 0.001 and hp < 0.01 vs. HFD.
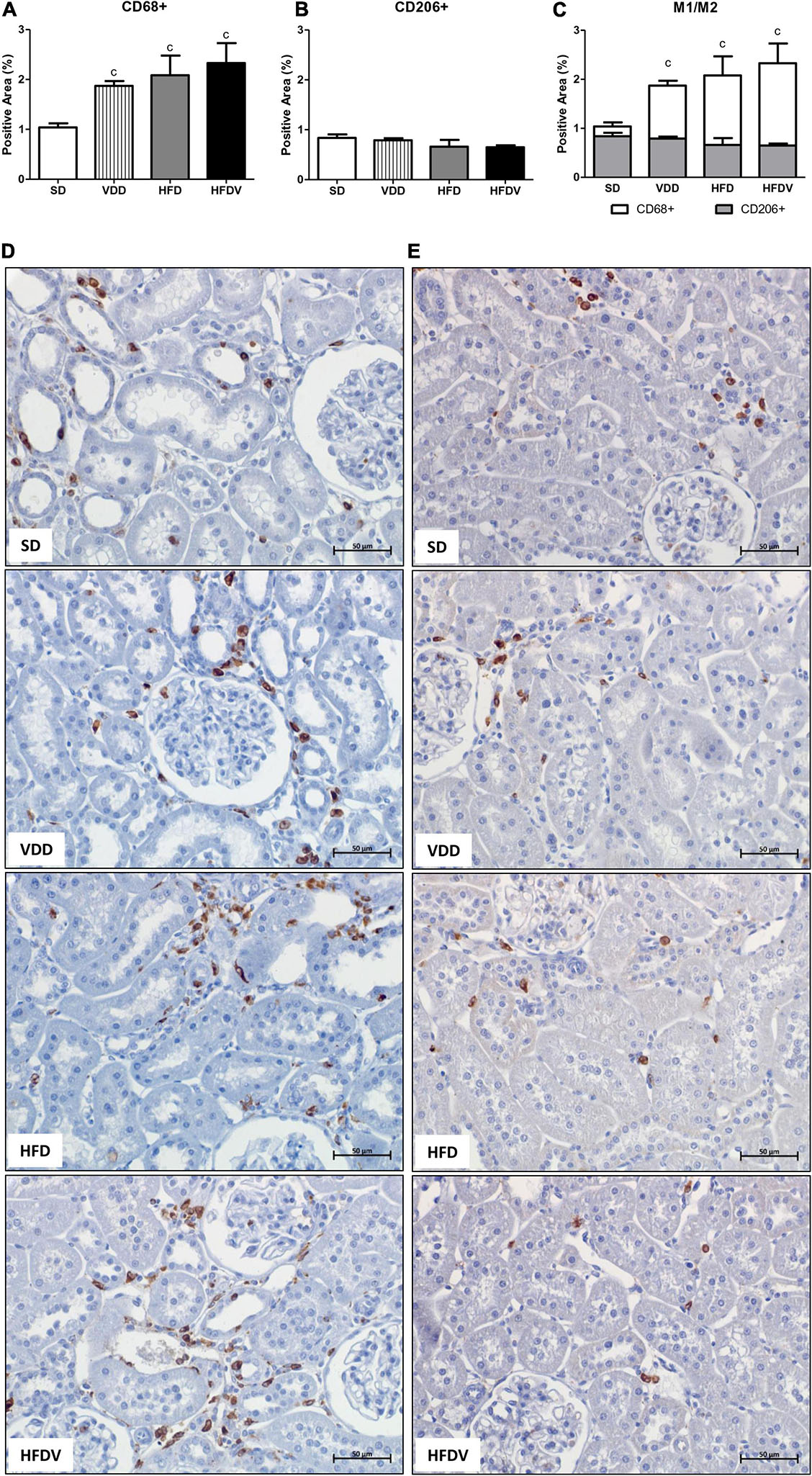
Figure 6. Immunohistochemical analysis for CD68+ cells (M1 + M2 macrophages) and CD206+ cells (M2 macrophages) expression in the renal cortex evaluated after the 90-day protocol in rats submitted to renal ischemia-reperfusion insult on day 45 treated with standard diet (SD), vitamin D-free diet (VDD), high-fat diet (HFD), or high-fat vitamin D-free diet (HFDV). (A) Bar graph of CD68+ cells expression values. (B) Bar graph of CD206+ cells expression values. (C) Bar graph regarding the proportion of CD206+ cells in relation to the amount of CD68+ cells. Representative photomicrographs of immunostaining for CD68+ (D) and CD206+ (E) cells in the renal cortex from an SD, VDD, HFD, and HFDV rat (×400). Data are mean ± SEM. cp < 0.05 vs. SD.
Synergistic effect of vitamin D deficiency and adipose tissue on the renal expression of transforming growth factor β1 and extracellular matrix proteins
High-fat diets and hypovitaminosis D seem to be associated with the susceptibility to renal fibrosis formation (RFF) through an increased expression of TGF-β and extracellular matrix (ECM) components (1, 2, 35–37). In this study, we could observe the isolated influence of vitamin D deficiency and obesity, which contributed to a higher renal expression of TGF-β1 (%) in the VDD and HFD groups than in the SD group (p < 0.01). Simultaneously, we noticed a synergistic influence of both risk factors, which promoted a significant increase (p < 0.001) in the expression of TGF-β1 in the HFDV group compared to all the other groups (Figure 7).
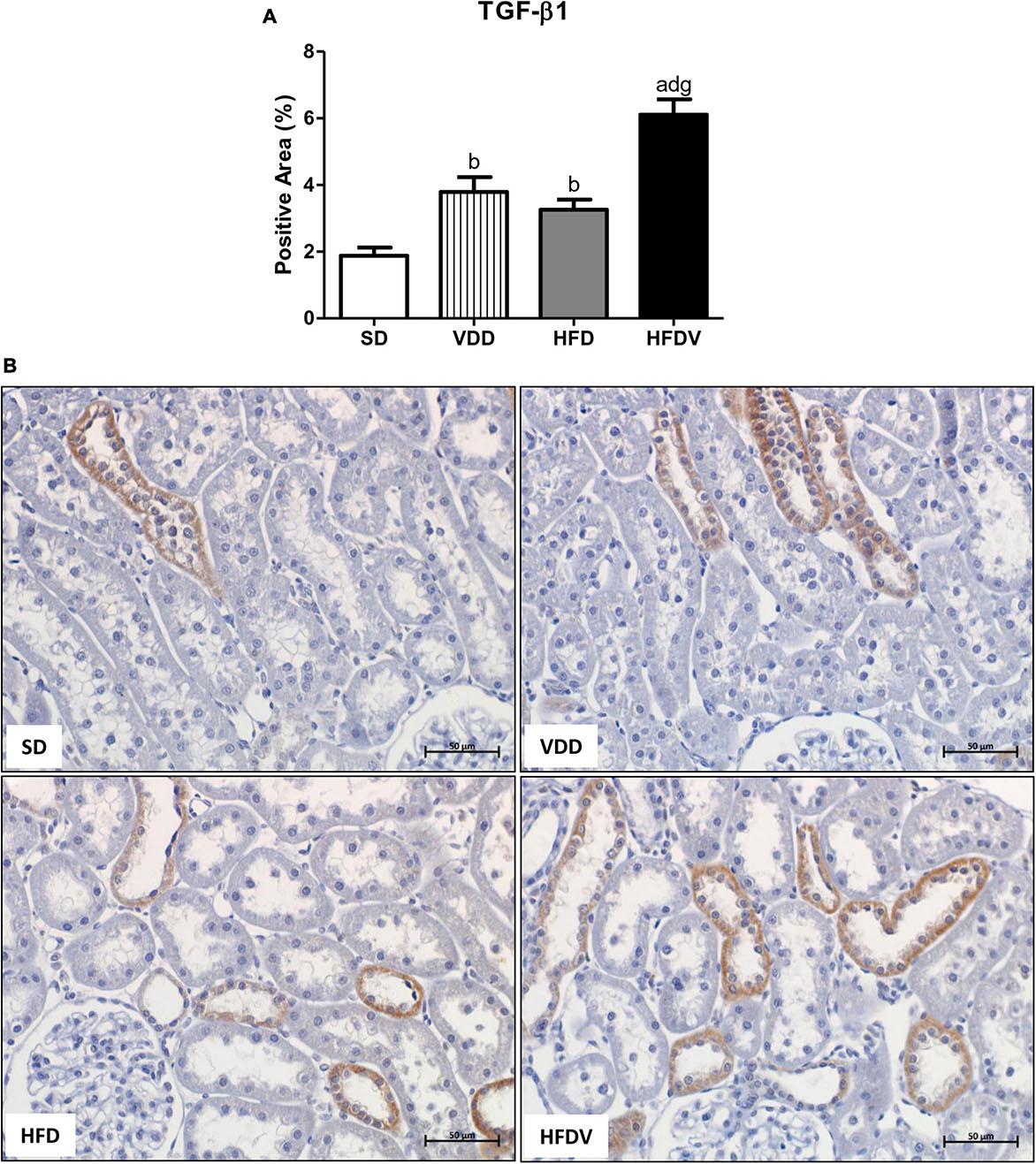
Figure 7. Immunohistochemical analysis for transforming growth factor β1 (TGF-β1) expression in the renal cortex evaluated after the 90-day protocol in rats submitted to renal ischemia-reperfusion insult on day 45 treated with standard diet (SD), vitamin D-free diet (VDD), high-fat diet (HFD) or high-fat vitamin D-free diet (HFDV). (A) Bar graph of TGF-β1 expression values. (B) Representative photomicrographs of immunostaining for TGF-β1 in the renal cortex from an SD, VDD, HFD, and HFDV rat (×400). Data are mean ± SEM. ap < 0.001 and bp < 0.01 vs. SD; dp < 0.001 vs. VDD; gp < 0.001 vs. HFD.
To assess the production and secretion of ECM components generated from fibroblast activation, we investigated the renal expression of two ECM proteins, including Col-3 and fibronectin. First, our results showed a slight upward tendency in the renal amount of Col-3 (ng/μg) and a higher expression of fibronectin (%) in the VDD group in relation to the SD group. Meanwhile, the HFD group presented a higher renal expression of Col-3 and fibronectin than the SD group. Of note, those alterations were even more pronounced in the HFDV group. This group not only presented a higher amount of Col-3 in relation to SD and VDD groups, but also a significant increase in fibronectin expression compared to SD and HFD groups (Figure 8).
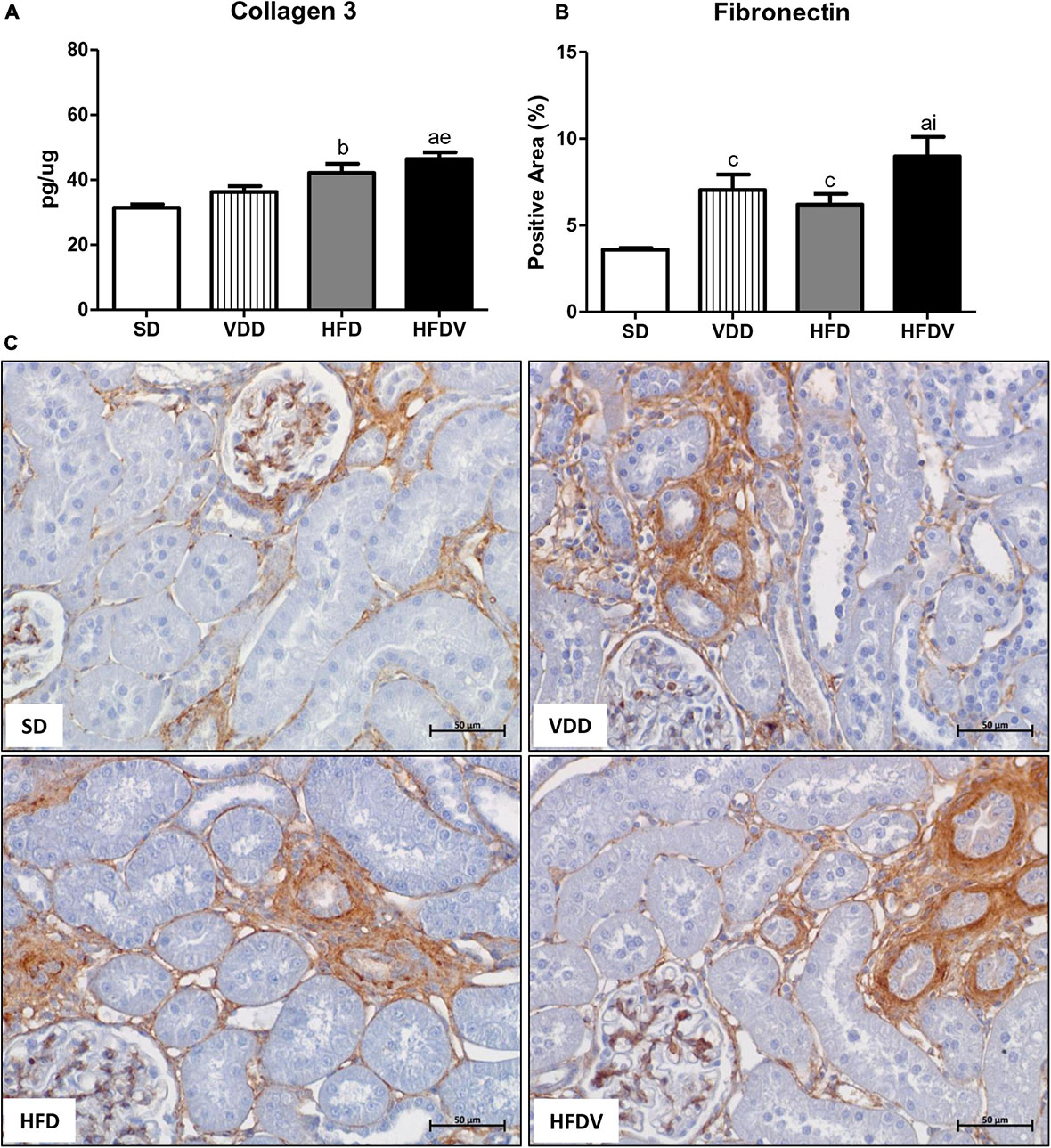
Figure 8. Quantitative amount of collagen 3—ELISA and immunohistochemical analysis for fibronectin expression in the kidney tissue evaluated after the 90-day protocol in rats submitted to renal ischemia-reperfusion insult on day 45 treated with standard diet (SD), vitamin D-free diet (VDD), high-fat diet (HFD), or high-fat vitamin D-free diet (HFDV). Bar graphs of (A) collagen 3 and (B) fibronectin expression values. (C) Representative photomicrographs of immunostaining for fibronectin in the renal cortex from an SD, VDD, HFD, and HFDV rat (400×). Data are mean ± SEM. ap < 0.001, bp < 0.01 and cp < 0.05 vs. SD; ep < 0.01 vs. VDD; ip < 0.05 vs. HFD.
Obesity and vitamin D deficiency increase the phenotypic change of renal cells
We studied the presence of markers of phenotypic change which included the renal expression of α-smooth muscle actin (α-SMA) and vimentin. We observed a mild upward tendency in the renal expression of α-SMA and a significant increase (p < 0.05) in vimentin expression in the VDD group in relation to the SD group. Simultaneously, we noticed a higher α-SMA expression (p < 0.05) and a slight upward tendency in vimentin expression in the HFD group compared to the SD group. Regarding the HFDV group, we found a higher vimentin expression than the SD group and a significant increase in renal expression of α-SMA compared to all the other groups (Figures 9, 10). Based on our results, it is plausible to suggest that the synergistic effect of vitamin D deficiency and obesity increased the phenotypical change of renal cells.
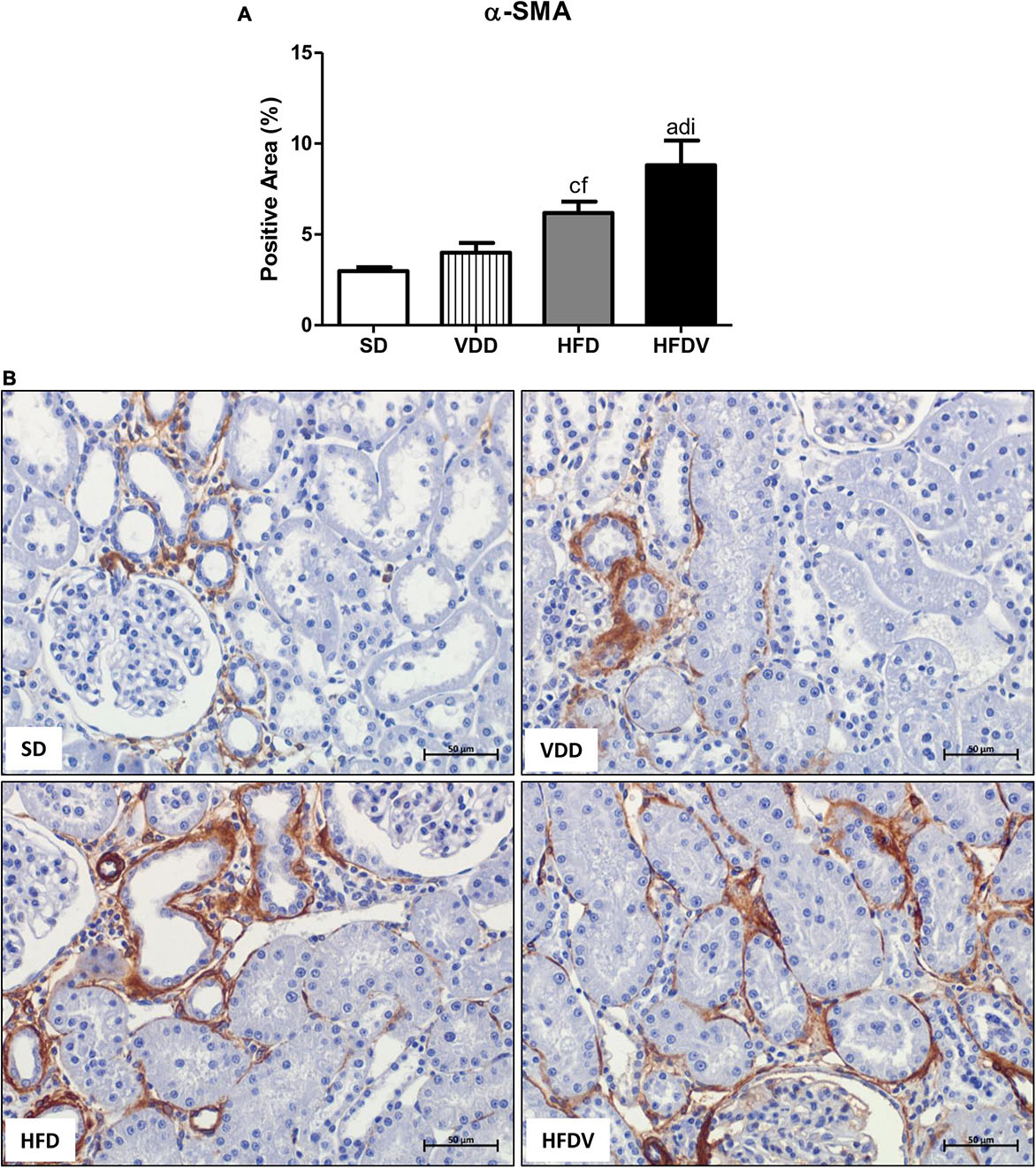
Figure 9. Immunohistochemical analysis for α-smooth muscle actin (α-SMA) expression in the renal cortex evaluated after the 90-day protocol in rats submitted to renal ischemia-reperfusion insult on day 45 treated with standard diet (SD), vitamin D-free diet (VDD), high-fat diet (HFD) or high-fat vitamin D-free diet (HFDV). (A) Bar graph of α-SMA expression values. (B) Representative photomicrographs of immunostaining for α-SMA in the renal cortex from an SD, VDD, HFD, and HFDV rat (400×). Data are mean ± SEM. ap < 0.001 and cp < 0.05 vs. SD; dp < 0.001 and fp < 0.05 vs. VDD; ip < 0.05 vs. HFD.
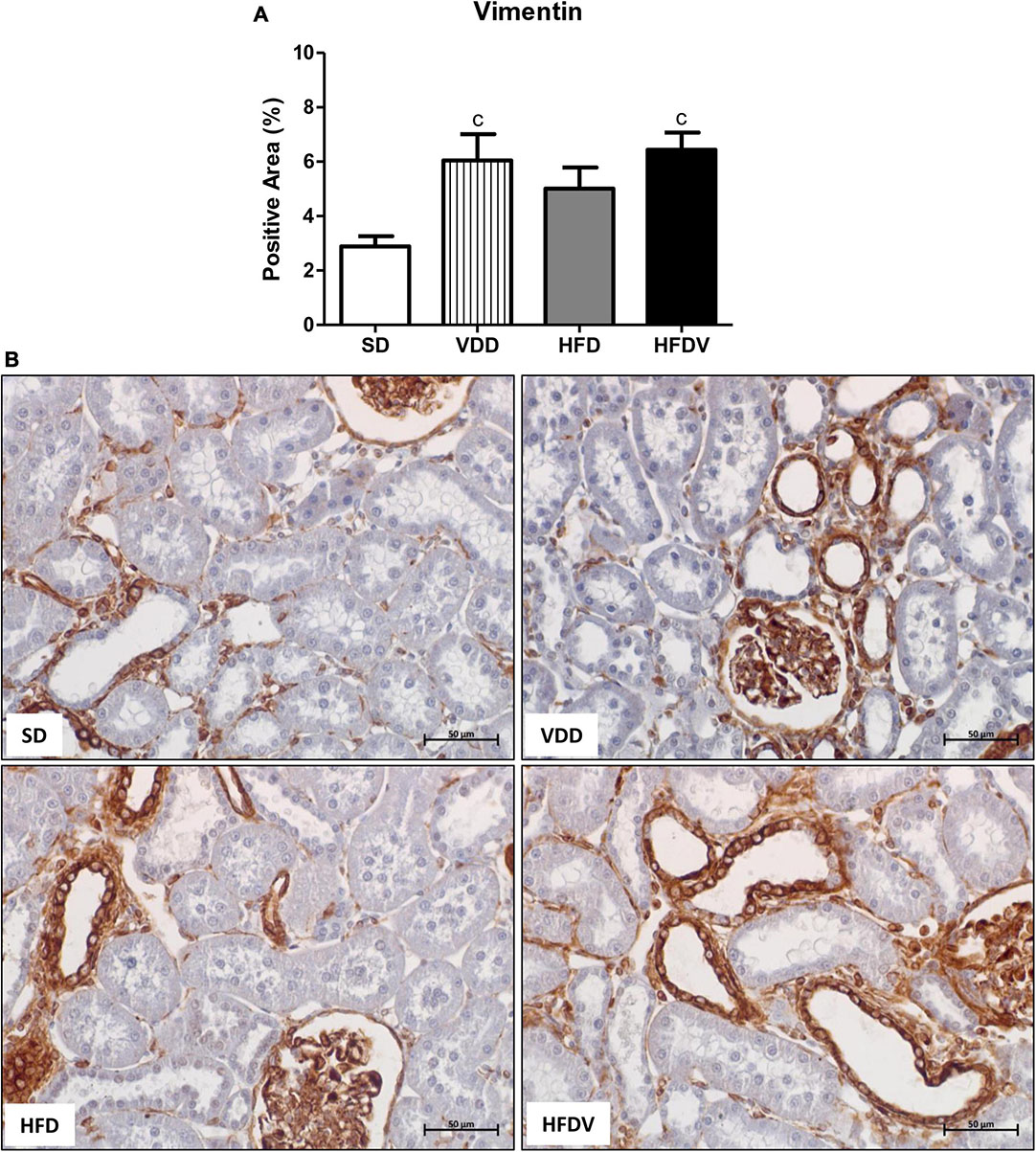
Figure 10. Immunohistochemical analysis for vimentin expression in the renal cortex evaluated after the 90-day protocol in rats submitted to renal ischemia-reperfusion insult on day 45 treated with standard diet (SD), vitamin D-free diet (VDD), high-fat diet (HFD) or high-fat vitamin D-free diet (HFDV). (A) Bar graph of vimentin expression values. (B) Representative photomicrographs of immunostaining for vimentin in the renal cortex from an SD, VDD, HFD, and HFDV rat (400×). Data are mean ± SEM. cp < 0.05 vs. SD.
Adipose tissue and vitamin D roles on the cell proliferation, glomerular vascular endothelium, and tubulointerstitial alterations
The process of epithelial–mesenchymal transition (EMT) promotes greater cell division, allows cells to acquire a secretory phenotype, and contributes to greater deposition of ECM components. After our studies regarding ECM proteins and markers of phenotypical alterations, we proposed to investigate the proliferation of renal cells as well as the integrity of the glomerular vascular endothelium. Initially, we assessed the cell proliferation by evaluating the immunostaining for PCNA in the renal cortex. We verified a higher renal expression of PCNA (%) in the HFDV group (p < 0.05) compared to all the other groups (Figure 11). As a marker of glomerular vascular endothelium, we performed IHC studies using an antibody against JG12. Our data revealed a lower JG12 staining (p < 0.001) per glomerular tuft area (%) in the VDD and HFD groups compared to the SD group. This alteration was even more evident in the HFDV group, which showed a remarkable reduction in the expression of JG12 in comparison to all the other groups (Figure 12). We found no differences among the groups concerning the glomerular tuft area used to correct the expression of JG12 (Table 2).
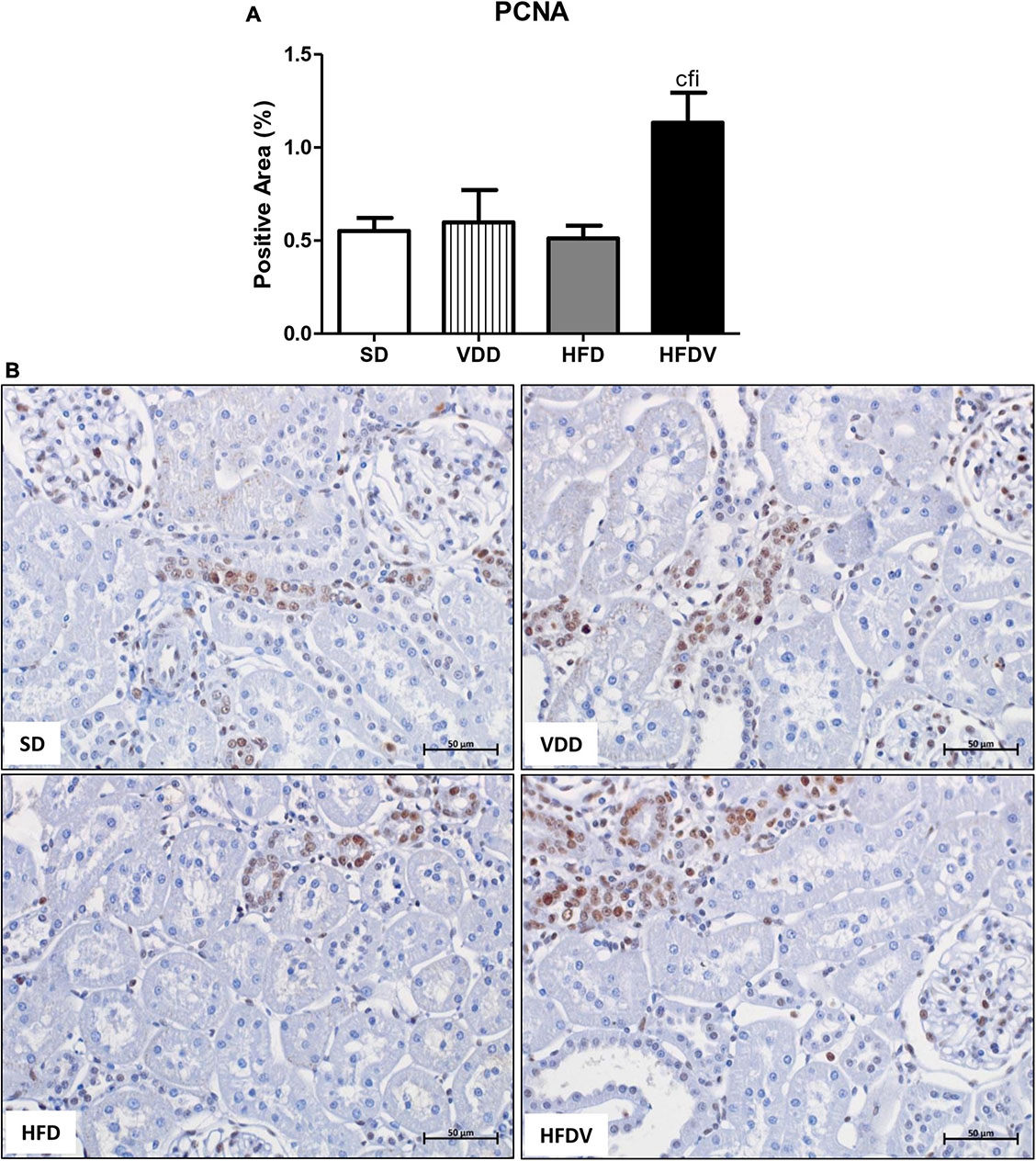
Figure 11. Immunohistochemical analysis for proliferating cell nuclear antigen (PCNA) expression in the renal cortex evaluated after the 90-day protocol in rats submitted to renal ischemia-reperfusion insult on day 45 treated with standard diet (SD), vitamin D-free diet (VDD), high-fat diet (HFD), or high-fat vitamin D-free diet (HFDV). (A) Bar graph of PCNA expression values. (B) Representative photomicrographs of immunostaining for PCNA in the renal cortex from an SD, VDD, HFD, and HFDV rat (×400). Data are mean ± SEM. cp < 0.05 vs. SD; fp < 0.05 vs. VDD; ip < 0.05 vs. HFD.
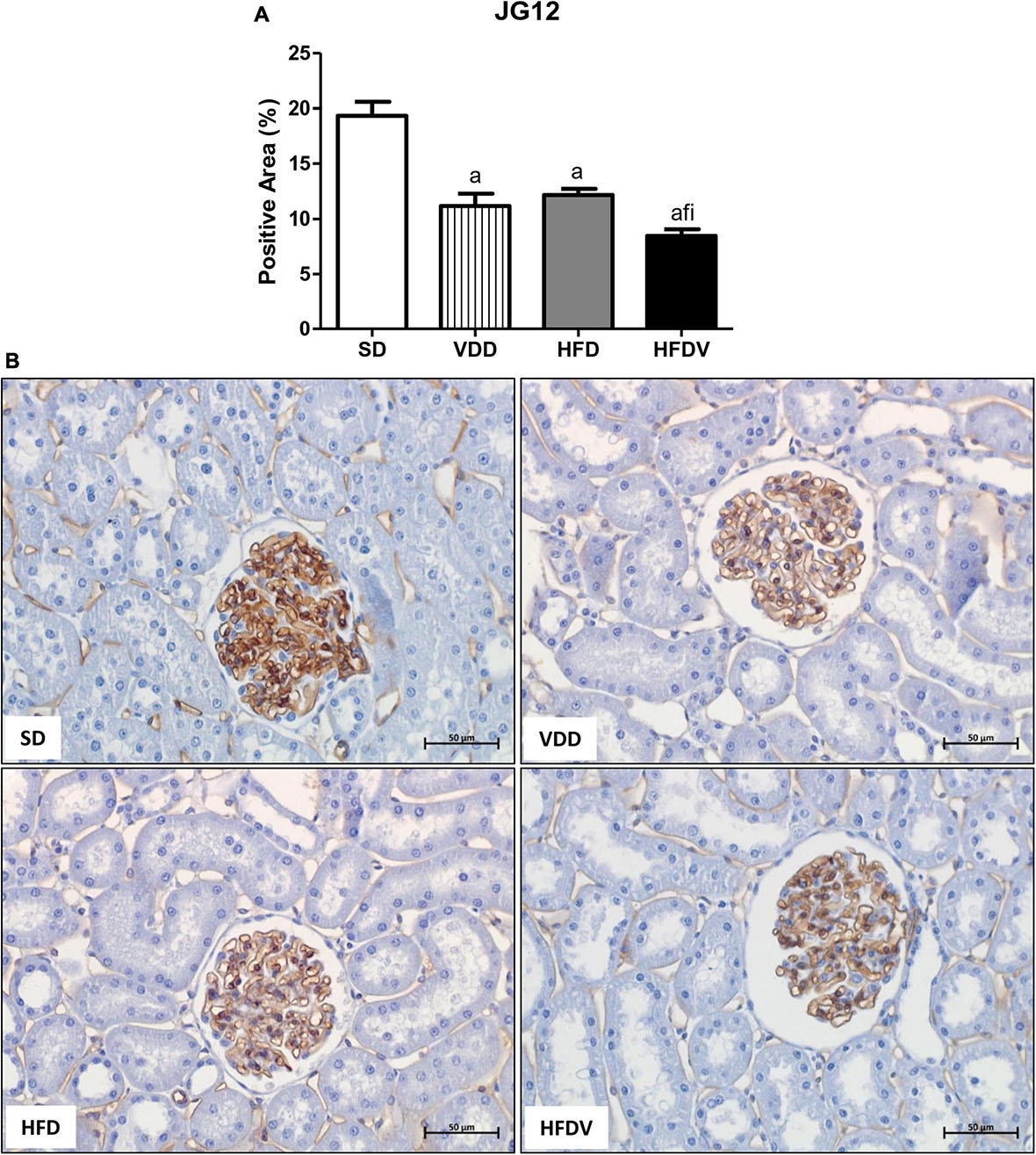
Figure 12. Immunohistochemical analysis for aminopeptidase P (JG12) expression by glomerular tuft area in the renal tissue evaluated after the 90-day protocol in rats submitted to renal ischemia-reperfusion insult on day 45 treated with standard diet (SD), vitamin D-free diet (VDD), high-fat diet (HFD) or high-fat vitamin D-free diet (HFDV). (A) Bar graph of JG12 expression values. (B) Representative photomicrographs of immunostaining for JG12 in the renal cortex from an SD, VDD, HFD, and HFDV rat (×400). Data are mean ± SEM. ap < 0.001 vs. SD; fp < 0.05 vs. VDD; ip < 0.05 vs. HFD.
Finally, we evaluated the tubulointerstitial involvement based on our dataset which included the results obtained from the experiments regarding the inflammatory infiltrate, RFF, and consequent interstitial expansion. By histomorphometry, we evaluated the FIA in the renal cortex. The histomorphometric studies revealed an upward tendency regarding FIA in the VDD group in relation to the SD group. In addition, we observed a larger FIA (p < 0.05) in the HFD group than in the SD group. Of note, the HFDV group showed a more evident increase (p < 0.001) in the FIA compared to all the other groups (Figure 13), which indicates a synergistic role of vitamin D and adipose tissue on the alterations of the tubulointerstitial compartment.
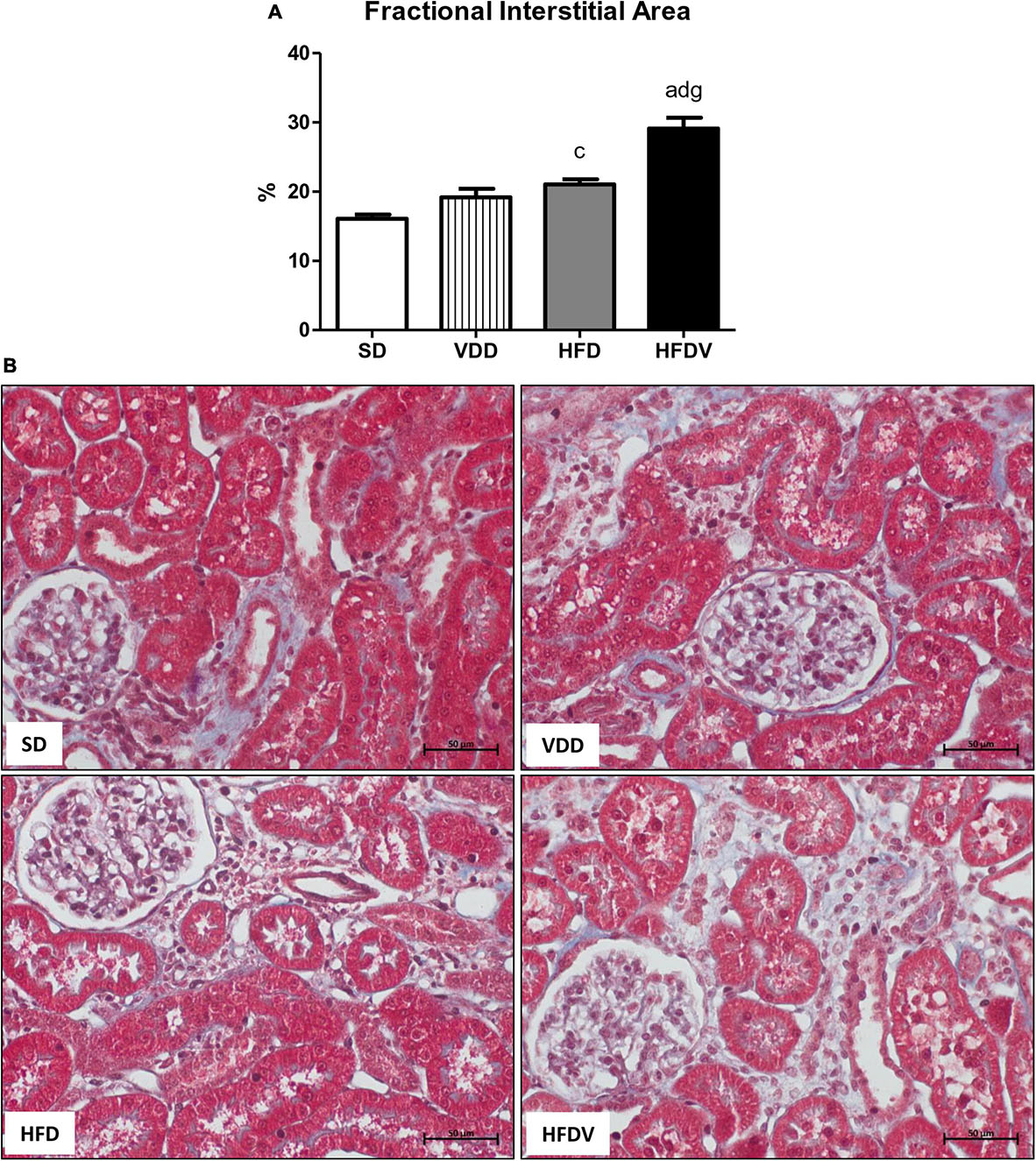
Figure 13. Fractional interstitial area (FIA) of the renal cortex evaluated after the 90-day protocol in rats submitted to renal ischemia-reperfusion insult on day 45 treated with standard diet (SD), vitamin D-free diet (VDD), high-fat diet (HFD) or high-fat vitamin D-free diet (HFDV). (A) Bar graph of FIA values. (B) Masson’s trichrome representative photomicrographs of renal histological changes from an SD, VDD, HFD, and HFDV rat (×400). Data are mean ± SEM. ap < 0.001 and cp < 0.05 vs. SD; dp < 0.001 vs. VDD; gp < 0.001 vs. HFD.
Discussion
Our results show that the animals fed a high-fat vitamin D-free diet and submitted to renal IRI presented almost undetectable levels of vitamin D and changes in the anthropometric and metabolic profile. The combination of vitamin D deficiency and obesity modified functional and hemodynamic parameters. Furthermore, we observed an increase in proteinuria, renal expression of MCP-1, infiltration of inflammatory cells, ECM proteins, and phenotypical markers in the HFDV. This group also presented a greater cell proliferation, impairment of the glomerular vascular endothelium, and expansion of the tubulointerstitial compartment. All those alterations were associated with a higher expression of TGF-β1 and a lower expression of VDR in the renal tissue from the HFDV group.
Plasma levels of vitamin D represent the sum of biological production from diet and sun exposure (38). It is important to state that our animals were kept without sun exposure and received diets depleted or not in vitamin D. The vitamin D-free diet groups (VDD and HFDV) had almost undetectable plasma levels of vitamin D at the end of the 90-day protocol, confirming the efficiency of the experimental model of vitamin D deficiency (2, 6, 8). Plasma levels of vitamin D have been inversely associated with obesity, insulin resistance, and type 2 diabetes (12, 15, 39). In addition to the HFDV rats, we observed lower plasma levels of vitamin D as well as a downward tendency regarding the renal expression of VDR in the HFD rats. Our results are consistent with the hypothesis of a lower and inadequate bioavailability of vitamin D in obesity (12, 15, 39).
Usually considered a storage organ for vitamin D, adipose tissue also seems to influence endocrine and paracrine actions, modulating the expression of enzymes responsible for the formation, activation, and degradation of vitamin D (12, 40, 41). Besides the renal protein expression, we evaluated VDR gene expression in the adipose tissue. Our VDD group showed a lower VDR gene expression, which primarily followed the deficient plasma levels of vitamin D. In 2015, Nguyen et al. described the association between plasma vitamin D levels and VDR gene expression in adipose tissue (39). However, we did not observe this relationship in our HFDV rats. Even receiving a vitamin D-depleted diet, HFDV rats showed a higher renal expression of VDR and a marked gene expression of this receptor in adipose tissue compared to VDD rats. In addition, our groups that received the high-fat diets (HFD and HFDV) showed a downward tendency in the VDR gene expression in adipose tissue in relation to the SD group. Wamberg et al. also observed a reduced VDR gene expression in the subcutaneous adipose tissue of obese women in comparison to lean individuals. On the other hand, these authors demonstrated that there was a 33% higher expression of VDR in the visceral adipose tissue of obese women compared to lean women (41). Nevertheless, our results are not enough to understand the physiological actions of vitamin D on adipose tissue associated with the degree of obesity and future studies are needed to explain this relationship.
Hypercaloric and high-fat diets are described in the literature as being effective for the experimental induction of obesity in rodents (42–44). As a confirmation of the effectiveness of our experimental model, the anthropometric measurements used as markers of obesity demonstrated that HFD and HFDV rats presented higher body weight and BMI as well as a significant increase in their abdominal and thoracic circumferences.
Hyperlipidemia is commonly related to a high intake of diets rich in fatty acids and obesity (45, 46). It is described that the abnormal deposition of fat in adipose tissue and in other organs, such as the liver and kidneys, can be considered an important risk in the follow-up of pathologies, including CKD (45, 47, 48). The presence of dyslipidemia is reported in all stages of CKD, with impairment of the glomerular filtration barrier, tubular damage, and proteinuria (47). Our HFD and HFDV rats showed higher plasma levels of cholesterol and triglycerides than SD and VDD rats. Bhandari et al. also showed the adverse effects of obesity induced by a high-fat diet. Corroborating our data, these authors demonstrated alterations in the lipid profile of rodents, such as the presence of high levels of total cholesterol, triglycerides, and LDL, followed by low levels of HDL (43).
The relationship between lipid profile and vitamin D is also demonstrated in the literature (6, 49, 50). It has been reported that vitamin D and cholesterol share the same biosynthesis pathway, as they have 7-dehydroxycholesterol as a common precursor (46). In the present study, the vitamin D-deficient rats, particularly HFDV rats, had higher levels of cholesterol associated with remarkable triglyceride levels compared to SD animals. Vitamin D plays important roles in the regulation and absorption of calcium, thus reducing the absorption of fatty acids and exerting an influence on plasma cholesterol levels (51). In addition, low levels of vitamin D promote plasma PTH elevation. High levels of PTH increase lipogenesis, and bone remodeling, and reduce lipolytic activity, thus influencing lipid metabolism (46, 48, 51). As expected, our VDD and HFDV groups presented elevated PTH levels in relation to SD and HFD groups, demonstrating the negative feedback caused by vitamin D deficiency.
Hyperglycemia is a typical sign of both reduced gluconeogenesis in the liver and reduced glucose uptake in skeletal muscle, liver, and adipose tissue, which feature the onset of insulin resistance (50, 52). In addition to overweight, increased visceral adipose tissue represents an important association with insulin resistance and changes in adipose tissue functionality (53). Vitamin D deficiency is also related to inadequate insulin secretion, altered blood glucose levels, and type 2 diabetes (54–57). One of the mechanisms by which hypovitaminosis D contributes to the installation of insulin resistance is via the regulation of intracellular calcium in pancreatic β-cells (50, 58). In the present study, we found an upward tendency in fasting plasma glucose in VDD and HDF groups. Simultaneously, the association between obesity and vitamin D deficiency promoted a higher glycemia in the HFDV group in comparison to all the other groups. Thereby our dataset regarding the metabolic parameters (cholesterol, triglycerides, and fasting blood glucose) allowed us to observe the influence of obesity and vitamin D deficiency, alone or in association, on the installation of metabolic syndrome, especially in the HFDV animals.
Adipose tissue is not only recognized for its capacity for energy storage and lipid mobilization, but also as an adipokine-secreting endocrine organ (22, 53). It is known that adipocytes, one of the main cell types of the adipose tissue, secrete a variety of factors and hormones, such as TNF-α, IL-6, PAI-1, MCP-1, adiponectin, resistin, and leptin (22, 23, 59). Physiologically, leptin plays an important role in the control of energy homeostasis and maintenance of body weight, with circulating levels proportional to food intake and body energy reserve from the suppression or activation of specific neurotransmitters (60, 61). The condition called hyperleptinemia in obesity induces leptin resistance, which is similar to insulin resistance in type 2 diabetes (61). In the present study, our HFD and HFDV groups showed higher plasma leptin levels than the SD group. Corroborating our results, previous studies also showed an increase in serum leptin levels in experimental models of obesity induced by high-fat diets (62–64). In addition to being directly related to the amount of adipose tissue, leptin concentration seems to be also regulated by serum vitamin D levels (65, 66). Conversely, leptin appears to have an inhibitory effect on the conversion of 25(OH)D to 1,25(OH)2D3, by inhibiting 1-α-hydroxylase in renal and adipose tissue (66, 67). Those findings are in agreement with our data regarding the notorious high plasma leptin levels observed in the HFDV rats. Thus, our results allow us to infer that the synergistic effect of vitamin D deficiency and obesity could explain the significant increase in plasma leptin levels observed in the HFDV group.
It is well known that CKD, even in the early stages, is accompanied by a progressive decline in GFR and low levels of vitamin D (1, 7, 8, 10, 68). In 2011, de Boer et al. suggested that vitamin D deficiency may be a risk factor for the decline in GFR, especially when associated with diabetes and hypertension (69). Our VDD rats presented a lower GFR compared to SD and HFD rats, confirming previous results from our group regarding the role of vitamin D deficiency on the impairment of the renal function (1, 7, 8). In addition, obesity and dyslipidemia are described as aggravating factors in the progression of kidney disease, promoting damage to the glomerular filtration barrier and the subsequent presence of proteinuria (25, 47, 70, 71). Although not significant, the HFDV group presented a decrease of ∼13% in GFR in relation to VDD group and a greater proteinuria than the HFD group. Associated with these results, the HFDV group also presented a lower glomerular expression of JG12 compared to all the other groups. Corroborating these results, previous studies from our laboratory demonstrated a decreased renal JG12 expression in vitamin D-deficient rats in renal IRI and 5/6 nephrectomy model (7, 8). Since JG12 is an aminopeptidase responsible for anchoring the cells in the cell membrane, it is plausible to infer that the lower expression of this protein might have contributed to the impaired renal function observed in the HFDV group. Taken together, those results suggest that either isolated or combined effects of obesity and vitamin D deficiency may impair renal function.
It is common knowledge that the RAAS is responsible for maintaining vascular resistance and extracellular fluid homeostasis (72). The negative endocrine regulation of the RAAS by vitamin D is demonstrated in the literature mainly by an inverse relation of this hormone levels and the expression of renin (72, 73). This impairment of systemic blood pressure control generated by hypovitaminosis D has also been demonstrated in previous studies from our group, which associated changes in the activation of the RAAS with alterations in endothelium and renal vasculature (1, 2, 7, 72–74). Furthermore, the association between obesity and increased MAP has been suggested as an important link in kidney injury (5). In the present study, we found a higher MAP as well as increased plasma levels of Ang II and aldosterone in the VDD, HFD, and HFDV groups, suggesting a greater activation of the RAAS. The main mechanisms involved in the elevation of MAP levels in the course of obesity are described as: (1) activation of the sympathetic nervous system by increasing intra-abdominal pressure and higher levels of leptin; (2) RAAS activation due to increased secretion of inflammatory cytokines such as TNF-α, IL-6, resistin, and leptin by adipocytes; (3) increased aldosterone levels by leptin stimulation to the adrenal gland, increasing Na+ retention and plasma volume expansion; and (4) reduced adiponectin expression, which appears to be one of the causes of increased inflammation in obesity (5, 26, 75, 76). Our data showed that the association between obesity and vitamin D deficiency potentiated hemodynamic impairment. Collectively, changes in MAP and plasma levels of Ang II/aldosterone as well as in RBF and RVR support the hemodynamic disturbance observed in the HFDV group.
Inflammatory cells including macrophages and T cells play a key role in tissue homeostasis and immune responses, especially in the course of kidney diseases (7, 77). Moreover, an exacerbated inflammatory response is usually associated with a growing RFF (7, 77). This process involves several steps, including the stimulation of cell division and the production of chemokines to recruit cells to the site of injury (78). In addition to a higher renal expression of MCP-1 and CD68+ cells, our HFD group presented an upward tendency regarding the expression of CD3+ cells in relation to the SD group, which demonstrates a possible influence of adipose tissue on the inflammatory response. Corroborating our results, Decleves et al. also observed increased renal and urinary expression of MCP-1 in mice fed a high-fat diet (35). In the present study, we also observed similar results concerning the renal expression of MCP-1, CD3+, and CD68+ cells in the VDD group, reinforcing the immunomodulatory effect of vitamin D (1, 7, 77). Of note, we found a higher renal expression of MCP-1, CD3+, and CD68+ cells in the HFDV group compared to all the other groups, demonstrating a synergistic effect of adipose tissue and vitamin D deficiency regarding the inflammatory process.
Macrophage activation and function are heterogeneous and regulated by the microenvironment and stage of tissue injury, reflecting in different phenotypes (1, 77, 79). In general, macrophages are classified into two subtypes: (a) M1 macrophages, classically activated and considered pro-inflammatory due to their ability to produce and release pro-inflammatory cytokines, such as IL-1, IL-6, IL-12, IL- 23, and TNF-α; and (b) M2 macrophages, which are known to have anti-inflammatory and immunomodulatory functions (79, 80). In the present study, we observed a higher renal expression of CD68+ cells (M1+M2 macrophages) in the VDD, HFD, and HFDV groups compared to the SD group. In addition, we noted an upward tendency concerning the expression of those cells in the HFDV group. Although not significant, we noticed a lower renal expression of CD206+ cells (M2 macrophages) in relation to the total amount of CD68+ cells in the HFDV group. It is reported that the balance between M1/M2 macrophages is related to the renal microenvironment and may influence the progression of renal disease (1, 77, 80). In previous studies, we demonstrated that vitamin D deficiency contributed to the extension of the active state of inflammation, reinforcing the role of vitamin D in the modulation of inflammatory cells (1, 7, 77). Concurrently, macrophage infiltration is correlated with the degree of obesity, mainly with the M1 phenotype (79). The inflammatory cytokines produced by M1 macrophages neutralize the insulin-sensitizing actions of the hormones adiponectin and leptin, which eventually lead to insulin resistance (79). In contrast, macrophages in lean subjects express high levels of M2-specific genes, such as IL-10 and Arg-1 (79). Thus, our results show that the association between obesity and vitamin D deficiency contributed to an exacerbation of the inflammatory process observed in the HFDV group, which had higher expression of MCP-1 and CD3+ cells and a lower proportion of CD206+ cells in relation to the CD68+ cells.
As previously reported, AKI can result in incomplete tissue repair, persistent tubulointerstitial inflammation, fibroblast proliferation, and excessive deposition of ECM components (81). Furthermore, in response to kidney injury, cells that are normally stably differentiated to promote homeostasis can dedifferentiate into a new phenotype and redirect tissue repair in a process known as EMT (78). High-fat diets seem to be associated with susceptibility to RFF through increased expression of TGF-β and ECM components (36, 82). Furthermore, studies have shown that vitamin D can suppress the expression of TGF-β and its respective receptor and inhibit the EMT process, cell proliferation, and apoptosis as well (1, 2, 37). Corroborating those findings, we observed a higher renal expression of TGF-β1 in the VDD and HFD groups compared to the SD group. Importantly, the HFDV group presented a higher expression of TGF-β1 than all the other groups, which was associated with higher amounts of Col-3 and fibronectin in the renal tissue. These results suggest the interaction between obesity and vitamin D deficiency on the renal TGF-β1 expression and ECM production.
As aforementioned, EMT process promotes greater cell division, allows cells to acquire a secretory phenotype, and contributes to greater deposition of ECM components (78, 83). In 2012, Xiong et al. reported that the low expression of VDR in CKD would be involved in the relationship between inflammation and EMT (84). In the present study, we observed a higher renal expression of PCNA, α-SMA, and vimentin in the renal cortex from the HFDV group. Previous studies from our laboratory demonstrated the influence of vitamin D deficiency on increased phenotypic change (2) and cell proliferation (8). Corroborating our results regarding obesity, Coimbra et al. also observed a higher expression of vimentin in the renal tissue of obese Zucker rats (85). Furthermore, Amaral et al. showed a higher PCNA expression in the renal cortex of ovariectomized rats in obesity induced by a high-fat diet (86). Thus, similar to our data regarding ECM markers, our results suggest that the synergistic effect of obesity and vitamin D deficiency exacerbated cell proliferation and phenotype alteration of renal tubule cells in the HFDV group.
Fibrosis and inflammation are hallmarks in the course of kidney disease, in which unresolved kidney inflammation becomes an important driving force for the RFF (1, 87, 88). Some studies have been demonstrating that sufficient levels of vitamin D exert a protective effect in preserving cell integrity. Moreover, those reports show that a close relationship among vitamin D levels, VDR expression, and TGF-β could be involved in inflammation and EMT process (2, 37, 84). Previous data from our group showed that vitamin D deficiency caused a lower renal VDR expression and a higher renal TGF-β1 expression in rats submitted to renal IRI (2) and 5/6 nephrectomy (7, 77). Concomitantly, adipose tissue is recognized as an endocrine organ involved in the production and action of adipokines, including leptin, TNF-α, MCP-1, and TGF-β (23, 70, 89). In the present study, along with the increased expression of TGF-β1 and leptin as well as the decreased expression of the VDR, the HFDV group presented an exacerbated inflammatory infiltrate, greater cell proliferation, and phenotypic alteration of renal tubular cells. Taken together, these changes reflected a significant enlargement of the FIA in the HFDV group. Those results demonstrated a plausible synergistic effect of increased production and secretion of adipokines by adipose tissue and an impaired renoprotective action attributed to hypovitaminosis D. Hence, our data demonstrated that obesity associated with vitamin D deficiency led to a potentiation of the expression of inflammatory and pro-fibrotic factors in the progression following AKI induced by renal IRI.
Our results allow us to conclude that the association between vitamin D deficiency and obesity in the renal ischemia/reperfusion model modified functional, hemodynamic, and metabolic parameters and contributed to a greater expression of inflammatory and pro-fibrotic factors related to the progression of renal disease.
Data availability statement
The original contributions presented in this study are included in the article/supplementary material, further inquiries can be directed to the corresponding author.
Ethics statement
The animal study was reviewed and approved by Research Ethics Committee of Faculty of Medicine, University of São Paulo (CEUA, registration 1438/2020).
Author contributions
DB, DC, MN, MS, AB, and RV: performed the experiments. DB, DC, AB, and RV: analyzed the data and contributed to the writing of the manuscript. All authors conceived and designed the experiments, reviewed the manuscript, and approved the submitted version.
Funding
This work was supported by Fundação de Amparo à Pesquisa do Estado de São Paulo (FAPESP), grant nos. 2018/04930-6, 2018/12297-1, and 2019/20840-0.
Conflict of interest
The authors declare that the research was conducted in the absence of any commercial or financial relationships that could be construed as a potential conflict of interest.
Publisher’s note
All claims expressed in this article are solely those of the authors and do not necessarily represent those of their affiliated organizations, or those of the publisher, the editors and the reviewers. Any product that may be evaluated in this article, or claim that may be made by its manufacturer, is not guaranteed or endorsed by the publisher.
References
1. Dos Santos MS, Canale D, Bernardo DRD, Shimizu MHM, Seguro AC, Volpini RA, et al. The restoration of vitamin D levels slows the progression of renal ischemic injury in rats previously deficient in vitamin D. Front Med. (2021) 8:625647. doi: 10.3389/fmed.2021.625647
2. Goncalves JG, de Braganca AC, Canale D, Shimizu MH, Sanches TR, Moyses RM, et al. Vitamin D deficiency aggravates chronic kidney disease progression after ischemic acute kidney injury. PLoS One. (2014) 9:e107228. doi: 10.1371/journal.pone.0107228
3. Yuste C, Barraca D, Aragoncillo-Sauco I, Vega-Martinez A, Abad S, Verdalles-Guzman U, et al. Factors related with the progression of chronic kidney disease. Nefrologia. (2013) 33:685–91. doi: 10.3265/Nefrologia.pre2013.May.11900
4. Jha V, Garcia-Garcia G, Iseki K, Li Z, Naicker S, Plattner B, et al. Chronic kidney disease: global dimension and perspectives. Lancet. (2013) 382:260–72. doi: 10.1016/S0140-6736(13)60687-X
5. Ruster C, Wolf G. The role of the renin-angiotensin-aldosterone system in obesity-related renal diseases. Semin Nephrol. (2013) 33:44–53. doi: 10.1016/j.semnephrol.2012.12.002
6. Canale D, de Braganca AC, Goncalves JG, Shimizu MH, Sanches TR, Andrade L, et al. Vitamin D deficiency aggravates nephrotoxicity, hypertension and dyslipidemia caused by Tenofovir: role of oxidative stress and renin-angiotensin system. PLoS One. (2014) 9:e103055. doi: 10.1371/journal.pone.0103055
7. de Braganca AC, Canale D, Goncalves JG, Shimizu MHM, Seguro AC, Volpini RA. Vitamin D deficiency aggravates the renal features of moderate chronic kidney disease in 5/6 nephrectomized rats. Front Med. (2018) 5:282. doi: 10.3389/fmed.2018.00282
8. de Braganca AC, Volpini RA, Canale D, Goncalves JG, Shimizu MH, Sanches TR, et al. Vitamin D deficiency aggravates ischemic acute kidney injury in rats. Physiol Rep. (2015) 3:e12331. doi: 10.14814/phy2.12331
9. Dusso AS. Kidney disease and vitamin D levels: 25-hydroxyvitamin D, 1,25-dihydroxyvitamin D, and Vdr activation. Kidney Int Suppl. (2011) 1:136–41. doi: 10.1038/kisup.2011.30
10. Dusso A, Gonzalez EA, Martin KJ. Vitamin D in chronic kidney disease. Best Pract Res Clin Endocrinol Metab. (2011) 25:647–55. doi: 10.1016/j.beem.2011.05.005
11. Cipriani C, Pepe J, Piemonte S, Colangelo L, Cilli M, Minisola S. Vitamin D and its relationship with obesity and muscle. Int J Endocrinol. (2014) 2014:841248. doi: 10.1155/2014/841248
12. Clemente-Postigo M, Munoz-Garach A, Serrano M, Garrido-Sanchez L, Bernal-Lopez MR, Fernandez-Garcia D, et al. Serum 25-hydroxyvitamin D and adipose tissue vitamin D receptor gene expression: relationship with obesity and type 2 diabetes. J Clin Endocrinol Metab. (2015) 100:E591–5. doi: 10.1210/jc.2014-3016
13. Minambres I, Sanchez-Hernandez J, Sanchez-Quesada JL, Rodriguez J, de Leiva A, Perez A. The association of hypovitaminosis D with the metabolic syndrome is independent of the degree of obesity. ISRN Endocrinol. (2012) 2012:691803. doi: 10.5402/2012/691803
14. Karampela I, Sakelliou A, Vallianou N, Christodoulatos GS, Magkos F, Dalamaga M. Vitamin D and obesity: current evidence and controversies. Curr Obes Rep. (2021) 10:162–80. doi: 10.1007/s13679-021-00433-1
15. Snijder MB, van Dam RM, Visser M, Deeg DJ, Dekker JM, Bouter LM, et al. Adiposity in relation to vitamin d status and parathyroid hormone levels: a population-based study in older men and women. J Clin Endocrinol Metab. (2005) 90:4119–23. doi: 10.1210/jc.2005-0216
16. Pereira-Santos M, Costa PR, Assis AM, Santos CA, Santos DB. Obesity and vitamin D deficiency: a systematic review and meta-analysis. Obes Rev. (2015) 16:341–9. doi: 10.1111/obr.12239
17. Unger MD, Cuppari L, Titan SM, Magalhaes MC, Sassaki AL, dos Reis LM, et al. Vitamin D status in a sunny country: where has the sun gone? Clin Nutr. (2010) 29:784–8. doi: 10.1016/j.clnu.2010.06.009
18. Bolland MJ, Grey AB, Ames RW, Mason BH, Horne AM, Gamble GD, et al. The effects of seasonal variation of 25-hydroxyvitamin D and fat mass on a diagnosis of vitamin D sufficiency. Am J Clin Nutr. (2007) 86:959–64. doi: 10.1093/ajcn/86.4.959
19. Saneei P, Salehi-Abargouei A, Esmaillzadeh A. Serum 25-hydroxy vitamin D levels in relation to body mass index: a systematic review and meta-analysis. Obes Rev. (2013) 14:393–404. doi: 10.1111/obr.12016
20. Rosenstreich SJ, Rich C, Volwiler W. Deposition in and release of vitamin D3 from body fat: evidence for a storage site in the rat. J Clin Investig. (1971) 50:679–87. doi: 10.1172/JCI106538
21. Wortsman J, Matsuoka LY, Chen TC, Lu Z, Holick MF. Decreased bioavailability of vitamin D in obesity. Am J Clin Nutr. (2000) 72:690–3. doi: 10.1093/ajcn/72.3.690
22. Miricescu D, Balan DG, Tulin A, Stiru O, Vacaroiu IA, Mihai DA, et al. Impact of adipose tissue in chronic kidney disease development (review). Exp Ther Med. (2021) 21:539. doi: 10.3892/etm.2021.9969
23. Silva Junior GB, Bentes AC, Daher EF, Matos SM. Obesity and kidney disease. J Bras Nefrol. (2017) 39:65–9. doi: 10.5935/0101-2800.20170011
24. Koch VH. The effects of obesity on kidney function: a challenge for nephrologists. J Bras Nefrol. (2019) 41:162–5. doi: 10.1590/2175-8239-JBN-2019-0064
25. Kovesdy CP, Furth SL, Zoccali C, World Kidney Day Steering Committee. Obesity and kidney disease: hidden consequences of the epidemic. Blood Purif. (2017) 43:346–54. doi: 10.1159/000458481
26. Lakkis JI, Weir MR. Obesity and kidney disease. Prog Cardiovasc Dis. (2018) 61:157–67. doi: 10.1016/j.pcad.2018.07.005
27. Angeloco N, Rodrigues L, Leme IA, Lataro RC, Jordao AA. Bioelectrical impedance analysis and anthropometry for the determination of body composition in rats: effects of high-fat and high-sucrose diets. Rev Nutr. (2012) 25:331–9. doi: 10.1590/S1415-52732012000300003
28. Gerbaix M, Metz L, Ringot E, Courteix D. Visceral fat mass determination in rodent: validation of dual-energy X-ray absorptiometry and anthropometric techniques in fat and lean rats. Lipids Health Dis. (2010) 9:140. doi: 10.1186/1476-511X-9-140
29. Novelli EL, Diniz YS, Galhardi CM, Ebaid GM, Rodrigues HG, Mani F, et al. Anthropometrical parameters and markers of obesity in rats. Lab Anim. (2007) 41:111–9. doi: 10.1258/002367707779399518
30. Burnette WN. “Western Blotting”: electrophoretic transfer of proteins from sodium dodecyl sulfate–polyacrylamide gels to unmodified nitrocellulose and radiographic detection with antibody and radioiodinated protein A. Anal Biochem. (1981) 112:195–203. doi: 10.1016/0003-2697(81)90281-5
31. Lancas T, Kasahara DI, Gross JL, Pires-Neto RC, Deheinzelin D, Mauad T, et al. Cholinergic hyperresponsiveness of peripheral lung parenchyma in chronic obstructive pulmonary disease. Respiration. (2011) 82:177–84. doi: 10.1159/000326897
32. Livak KJ, Schmittgen TD. Analysis of relative gene expression data using real-time quantitative Pcr and the 2(–delta delta C(T)) method. Methods. (2001) 25:402–8. doi: 10.1006/meth.2001.1262
33. Volpini RA, Costa RS, da Silva CG, Coimbra TM. Inhibition of nuclear factor-Kappab activation attenuates tubulointerstitial nephritis induced by gentamicin. Nephron Physiol. (2004) 98:97–106. doi: 10.1159/000081558
34. Huen SC, Cantley LG. Macrophages in renal injury and repair. Annu Rev Physiol. (2017) 79:449–69. doi: 10.1146/annurev-physiol-022516-034219
35. Decleves AE, Mathew AV, Cunard R, Sharma K. Ampk mediates the initiation of kidney disease induced by a high-fat diet. J Am Soc Nephrol. (2011) 22:1846–55. doi: 10.1681/ASN.2011010026
36. Decleves AE, Sharma K. Obesity and kidney disease: differential effects of obesity on adipose tissue and kidney inflammation and fibrosis. Curr Opin Nephrol Hypertens. (2015) 24:28–36. doi: 10.1097/MNH.0000000000000087
37. Tan X, Li Y, Liu Y. Paricalcitol attenuates renal interstitial fibrosis in obstructive nephropathy. J Am Soc Nephrol. (2006) 17:3382–93. doi: 10.1681/ASN.2006050520
38. Holick MF. Vitamin D status: measurement, interpretation, and clinical application. Ann Epidemiol. (2009) 19:73–8. doi: 10.1016/j.annepidem.2007.12.001
39. Nguyen VT, Li X, Elli EF, Ayloo SM, Castellanos KJ, Fantuzzi G, et al. Vitamin D, inflammation, and relations to insulin resistance in premenopausal women with morbid obesity. Obesity. (2015) 23:1591–7. doi: 10.1002/oby.21131
40. Nimitphong H, Guo W, Holick MF, Fried SK, Lee MJ. Vitamin D Inhibits adipokine production and inflammatory signaling through the vitamin D receptor in human adipocytes. Obesity. (2021) 29:562–8. doi: 10.1002/oby.23109
41. Wamberg L, Christiansen T, Paulsen SK, Fisker S, Rask P, Rejnmark L, et al. Expression of vitamin D-metabolizing enzymes in human adipose tissue – the effect of obesity and diet-induced weight loss. Int J Obes. (2013) 37:651–7. doi: 10.1038/ijo.2012.112
42. Akiyama T, Tachibana I, Shirohara H, Watanabe N, Otsuki M. High-fat hypercaloric diet induces obesity, glucose intolerance and hyperlipidemia in normal adult male wistar rat. Diabetes Res Clin Pract. (1996) 31:27–35. doi: 10.1016/0168-8227(96)01205-3
43. Bhandari U, Kumar V, Khanna N, Panda BP. The effect of high-fat diet-induced obesity on cardiovascular toxicity in wistar albino rats. Hum Exp Toxicol. (2011) 30:1313–21. doi: 10.1177/0960327110389499
44. Woods SC, Seeley RJ, Rushing PA, D’Alessio D, Tso PA. Controlled high-fat diet induces an obese syndrome in rats. J Nutr. (2003) 133:1081–7. doi: 10.1093/jn/133.4.1081
45. Gai Z, Wang T, Visentin M, Kullak-Ublick GA, Fu X, Wang Z. Lipid accumulation and chronic kidney disease. Nutrients. (2019) 11:722. doi: 10.3390/nu11040722
46. Vekic J, Zeljkovic A, Stefanovic A, Jelic-Ivanovic Z, Spasojevic-Kalimanovska V. Obesity and dyslipidemia. Metab Clin Exp. (2019) 92:71–81. doi: 10.1016/j.metabol.2018.11.005
47. Pei K, Gui T, Li C, Zhang Q, Feng H, Li Y, et al. Recent progress on lipid intake and chronic kidney disease. Biomed Res Int. (2020) 2020:3680397. doi: 10.1155/2020/3680397
48. Yang P, Xiao Y, Luo X, Zhao Y, Zhao L, Wang Y, et al. Inflammatory stress promotes the development of obesity-related chronic kidney disease via Cd36 in mice. J Lipid Res. (2017) 58:1417–27. doi: 10.1194/jlr.M076216
49. Lupton JR, Faridi KF, Martin SS, Sharma S, Kulkarni K, Jones SR, et al. Deficient serum 25-hydroxyvitamin D is associated with an atherogenic lipid profile: the very large database of lipids (Vldl-3) study. J Clin Lipidol. (2016) 10:72–81.e1. doi: 10.1016/j.jacl.2015.09.006
50. Szymczak-Pajor I, Drzewoski J, Sliwinska A. The molecular mechanisms by which vitamin D prevents insulin resistance and associated disorders. Int J Mol Sci. (2020) 21:6644. doi: 10.3390/ijms21186644
51. Kim MR, Jeong SJ. Relationship between vitamin D level and lipid profile in non-obese children. Metabolites. (2019) 9:125. doi: 10.3390/metabo9070125
52. Petersen MC, Shulman GI. Mechanisms of insulin action and insulin resistance. Physiol Rev. (2018) 98:2133–223. doi: 10.1152/physrev.00063.2017
53. Despres JP, Lemieux I. Abdominal obesity and metabolic syndrome. Nature. (2006) 444:881–7. doi: 10.1038/nature05488
54. Devaraj S, Jialal G, Cook T, Siegel D, Jialal I. Low vitamin D levels in northern American adults with the metabolic syndrome. Horm Metab Res. (2011) 43:72–4. doi: 10.1055/s-0030-1268485
55. Hossein-nezhad A, Holick MF. Vitamin D for health: a global perspective. Mayo Clin Proc. (2013) 88:720–55. doi: 10.1016/j.mayocp.2013.05.011
56. Park S, Kim DS, Kang S. Vitamin D deficiency impairs glucose-stimulated insulin secretion and increases insulin resistance by reducing Ppar-gamma expression in nonobese type 2 diabetic rats. J Nutr Biochem. (2016) 27:257–65. doi: 10.1016/j.jnutbio.2015.09.013
57. Yang K, Liu J, Fu S, Tang X, Ma L, Sun W, et al. Vitamin D status and correlation with glucose and lipid metabolism in Gansu Province, China. Diabetes Metab Syndr Obes Targets Ther. (2020) 13:1555–63. doi: 10.2147/DMSO.S249049
58. Yaribeygi H, Maleki M, Sathyapalan T, Iranpanah H, Orafai HM, Jamialahmadi T, et al. The molecular mechanisms by which vitamin D improve glucose homeostasis: a mechanistic review. Life Sci. (2020) 244:117305. doi: 10.1016/j.lfs.2020.117305
59. Trayhurn P, Bing C, Wood IS. Adipose tissue and adipokines–energy regulation from the human perspective. J Nutr. (2006) 136(7 Suppl.):1935S–9S. doi: 10.1093/jn/136.7.1935S
60. Adamczak M, Wiecek A. The adipose tissue as an endocrine organ. Semin Nephrol. (2013) 33:2–13. doi: 10.1016/j.semnephrol.2012.12.008
61. Mendoza-Herrera K, Florio AA, Moore M, Marrero A, Tamez M, Bhupathiraju SN, et al. The leptin system and diet: a mini review of the current evidence. Front Endocrinol. (2021) 12:749050. doi: 10.3389/fendo.2021.749050
62. de Git KC, Adan RA. Leptin resistance in diet-induced obesity: the role of hypothalamic inflammation. Obes Rev. (2015) 16:207–24. doi: 10.1111/obr.12243
63. Lin L, Martin R, Schaffhauser AO, York DA. Acute changes in the response to peripheral leptin with alteration in the diet composition. Am J Physiol Regul Integr Comp Physiol. (2001) 280:R504–9. doi: 10.1152/ajpregu.2001.280.2.R504
64. Wong KE, Kong J, Zhang W, Szeto FL, Ye H, Deb DK, et al. Targeted expression of human vitamin D receptor in adipocytes decreases energy expenditure and induces obesity in mice. J Biol Chem. (2011) 286:33804–10. doi: 10.1074/jbc.M111.257568
65. Menendez C, Lage M, Peino R, Baldelli R, Concheiro P, Dieguez C, et al. Retinoic acid and vitamin D(3) powerfully inhibit in vitro leptin secretion by human adipose tissue. J Endocrinol. (2001) 170:425–31. doi: 10.1677/joe.0.1700425
66. Nobre JL, Lisboa PC, Carvalho JC, Martins MR, Vargas S, Barja-Fidalgo C, et al. Leptin blocks the inhibitory effect of vitamin D on adipogenesis and cell proliferation in 3t3-L1 adipocytes. Gen Comp Endocrinol. (2018) 266:1–8. doi: 10.1016/j.ygcen.2018.01.014
67. Tsuji K, Maeda T, Kawane T, Matsunuma A, Horiuchi N. Leptin stimulates fibroblast growth factor 23 expression in bone and suppresses renal 1alpha,25-dihydroxyvitamin D3 synthesis in leptin-deficient mice. J Bone Miner Res. (2010) 25:1711–23. doi: 10.1002/jbmr.65
68. Urena-Torres P, Metzger M, Haymann JP, Karras A, Boffa JJ, Flamant M, et al. Association of kidney function, vitamin D deficiency, and circulating markers of mineral and bone disorders in Ckd. Am J Kidney Dis. (2011) 58:544–53. doi: 10.1053/j.ajkd.2011.04.029
69. de Boer IH, Katz R, Chonchol M, Ix JH, Sarnak MJ, Shlipak MG, et al. Serum 25-hydroxyvitamin D and change in estimated glomerular filtration rate. Clin J Am Soc Nephrol. (2011) 6:2141–9. doi: 10.2215/CJN.02640311
70. Kopple JD, Feroze U. The effect of obesity on chronic kidney disease. J Ren Nutr. (2011) 21:66–71. doi: 10.1053/j.jrn.2010.10.009
71. Lu JL, Kalantar-Zadeh K, Ma JZ, Quarles LD, Kovesdy CP. Association of body mass index with outcomes in patients with Ckd. J Am Soc Nephrol. (2014) 25:2088–96. doi: 10.1681/ASN.2013070754
72. Li YC, Kong J, Wei M, Chen ZF, Liu SQ, Cao LP. 1,25-dihydroxyvitamin D(3) is a negative endocrine regulator of the renin-angiotensin system. J Clin Invest. (2002) 110:229–38. doi: 10.1172/JCI15219
73. Petchey WG, Johnson DW, Isbel NM. Shining D’ light on chronic kidney disease: mechanisms that may underpin the cardiovascular benefit of vitamin D. Nephrology (Carlton). (2011) 16:351–67. doi: 10.1111/j.1440-1797.2011.01450.x
74. de Braganca AC, Volpini RA, Mehrotra P, Andrade L, Basile DP. Vitamin D deficiency contributes to vascular damage in sustained ischemic acute kidney injury. Physiol Rep. (2016) 4:e12829. doi: 10.14814/phy2.12829
75. Packer M. Leptin-aldosterone-neprilysin axis: identification of its distinctive role in the pathogenesis of the three phenotypes of heart failure in people with obesity. Circulation. (2018) 137:1614–31. doi: 10.1161/CIRCULATIONAHA.117.032474
76. Thethi T, Kamiyama M, Kobori H. The link between the renin-angiotensin-aldosterone system and renal injury in obesity and the metabolic syndrome. Curr Hypertens Rep. (2012) 14:160–9. doi: 10.1007/s11906-012-0245-z
77. Goncalves JG, Canale D, de Braganca AC, Seguro AC, Shimizu MHM, Volpini RA. The blockade of tace-dependent Egf receptor activation by losartan-Erlotinib combination attenuates renal fibrosis formation in 5/6-nephrectomized rats under vitamin D deficiency. Front Med. (2020) 7:609158. doi: 10.3389/fmed.2020.609158
78. Schnaper HW. The tubulointerstitial pathophysiology of progressive kidney disease. Adv Chron Kidney Dis. (2017) 24:107–16. doi: 10.1053/j.ackd.2016.11.011
79. Shapouri-Moghaddam A, Mohammadian S, Vazini H, Taghadosi M, Esmaeili SA, Mardani F, et al. Macrophage plasticity, polarization, and function in health and disease. J Cell Physiol. (2018) 233:6425–40. doi: 10.1002/jcp.26429
80. Guiteras R, Flaquer M, Cruzado JM. Macrophage in chronic kidney disease. Clin Kidney J. (2016) 9:765–71. doi: 10.1093/ckj/sfw096
81. Bonventre JV, Yang L. Cellular pathophysiology of ischemic acute kidney injury. J Clin Investig. (2011) 121:4210–21. doi: 10.1172/JCI45161
82. Henegar JR, Bigler SA, Henegar LK, Tyagi SC, Hall JE. Functional and structural changes in the kidney in the early stages of obesity. J Am Soc Nephrol. (2001) 12:1211–7. doi: 10.1681/ASN.V1261211
83. Ferenbach DA, Bonventre JV. Mechanisms of maladaptive repair after AKI leading to accelerated kidney ageing and Ckd. Nat Rev Nephrol. (2015) 11:264–76. doi: 10.1038/nrneph.2015.3
84. Xiong M, Gong J, Liu Y, Xiang R, Tan X. Loss of vitamin D receptor in chronic kidney disease: a potential mechanism linking inflammation to epithelial-to-mesenchymal transition. Am J Physiol Renal Physiol. (2012) 303:F1107–15.
85. Coimbra TM, Janssen U, Grone HJ, Ostendorf T, Kunter U, Schmidt H, et al. Early events leading to renal injury in obese zucker (fatty) rats with type ii diabetes. Kidney Int. (2000) 57:167–82. doi: 10.1046/j.1523-1755.2000.00836.x
86. Amaral LS, Silva JA, Trindade TM, Ribas WB, Macedo CL, Coimbra TM, et al. Renal changes in the early stages of diet-induced obesity in ovariectomized rats. Physiol Res. (2014) 63:723–32. doi: 10.33549/physiolres.932619
87. Gu YY, Liu XS, Huang XR, Yu XQ, Lan HY. Diverse role of Tgf-beta in kidney disease. Front Cell Dev Biol. (2020) 8:123. doi: 10.3389/fcell.2020.00123
88. Lucisano S, Buemi M, Passantino A, Aloisi C, Cernaro V, Santoro D. New insights on the role of vitamin D in the progression of renal damage. Kidney Blood Press Res. (2013) 37:667–78. doi: 10.1159/000355747
Keywords: chronic kidney disease, acute kidney injury, obesity, adipose tissue, vitamin D deficiency, inflammation, renal fibrosis
Citation: Bernardo DRD, Canale D, Nascimento MM, Shimizu MHM, Seguro AC, de Bragança AC and Volpini RA (2022) The association between obesity and vitamin D deficiency modifies the progression of kidney disease after ischemia/reperfusion injury. Front. Nutr. 9:952028. doi: 10.3389/fnut.2022.952028
Received: 24 May 2022; Accepted: 13 October 2022;
Published: 17 November 2022.
Edited by:
Marija Djekic Ivankovic, McGill University, CanadaReviewed by:
Fernando Almeida-Souza, State University of Maranhão, BrazilLida Tartaglione, Umberto I Hospital, Italy
Copyright © 2022 Bernardo, Canale, Nascimento, Shimizu, Seguro, de Bragança and Volpini. This is an open-access article distributed under the terms of the Creative Commons Attribution License (CC BY). The use, distribution or reproduction in other forums is permitted, provided the original author(s) and the copyright owner(s) are credited and that the original publication in this journal is cited, in accordance with accepted academic practice. No use, distribution or reproduction is permitted which does not comply with these terms.
*Correspondence: Rildo Aparecido Volpini, cmlsZG8udm9scGluaUBoYy5mbS51c3AuYnI=