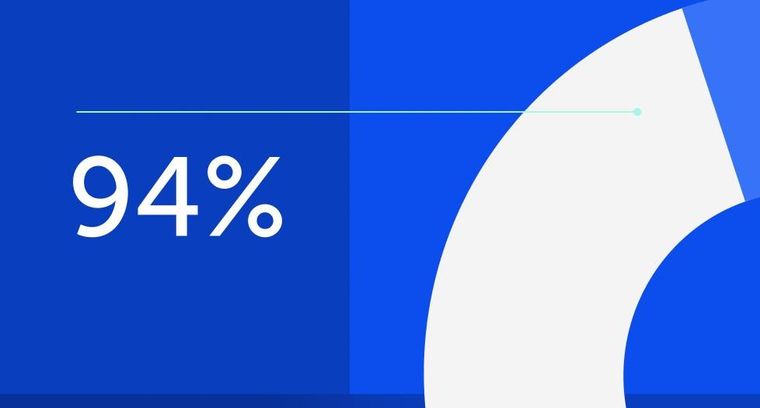
94% of researchers rate our articles as excellent or good
Learn more about the work of our research integrity team to safeguard the quality of each article we publish.
Find out more
REVIEW article
Front. Nutr., 04 August 2022
Sec. Food Chemistry
Volume 9 - 2022 | https://doi.org/10.3389/fnut.2022.951935
This article is part of the Research TopicGlobal Excellence in Food ChemistryView all 21 articles
In the past few years, phytochemicals from natural products have gotten the boundless praise in treating cancer. The promising role of cruciferous vegetables and active components contained in these vegetables, such as isothiocyanates, indole-3-carbinol, and isothiocyanates, has been widely researched in experimental in vitro and in vivo carcinogenesis models. The chemopreventive agents produced from the cruciferous vegetables were recurrently proven to affect carcinogenesis throughout the onset and developmental phases of cancer formation. Likewise, findings from clinical investigations and epidemiological research supported this statement. The anticancer activities of these functional foods bioactive compounds are closely related to their ability to upregulate p53 and its related target genes, e.g., p21. As the “guardian of the genome,” the p53 family (p53, p63, and p73) plays a pivotal role in preventing the cancer progression associated with DNA damage. This review discusses the functional foods bioactive compounds derived from several cruciferous vegetables and their use in altering the tumor-suppressive effect of p53 proteins. The association between the mutation of p53 and the incidence of gastrointestinal malignancies (gastric, small intestine, colon, liver, and pancreatic cancers) is also discussed. This review contains crucial information about the use of cruciferous vegetables in the treatment of gastrointestinal tract malignancies.
Our cells are always exposed to a wide array of cellular stresses, such as ionizing radiation, oncogenes, and oxidative stress. Following the exposure of these stresses, genomic aberration and instability may occur which could lead to cancer development. Therefore, a proper and delicate mechanism is required for detecting any DNA damage and protecting cells from malignancies. It has been known that the role of the p53 family (p53, p63, and p73 proteins) in suppressing the development of cancerous cells is indispensable. More than 50% of human cancers are closely linked to the mutation or deletion of p53 which indicates the pivotal role of this transcription factor (1, 2). Once cellular stressors, such as ionizing radiation, are exposed to cells, p53 is activated. This activation could lead to cell cycle arrest and apoptosis. While the former is aimed to repair the damage occurring in DNA due to the stressors exposure, the latter is activated if the damage cannot be repaired.
Of several cancers, gastrointestinal-related cancers, such as colorectal cancer, have been attracting big interest as their malignancies are associated with a high figure of death. In the United States, colorectal cancer-related death is placed in second place. From ~148,000 cases of colorectal cancer in the United States in 2020, the death was estimated at the rate of almost 35% (3). Surprisingly, from this figure, ~18,000 cases were detected in individuals under 50 years old (3).
The efforts conducted to discover and develop novel anticancer candidates from various sources, including from cruciferous vegetables, are still ongoing. These vegetables are a member of Brassicaceae (Cruciferae). It has been known that consumption of cruciferous vegetables, such as broccoli and cauliflower, is correlated with the lower incidence of chronic diseases, including cancer (4, 5). In addition to their nutritive contents (e.g., proteins, carbohydrates, vitamins, and minerals) (6), these vegetables also contain a number of metabolites that can promote health e.g., glucosinolates, isothiocyanates, methyl cysteine sulfoxide, terpenes, anthocyanins, and flavonoids (7–10). Some studies have reported the anticarcinogenic activity of several metabolites found in cruciferous vegetables. For example, glucosinolates, a secondary metabolite mainly found in cruciferous plants, display activity in preventing the development and progression of gastrointestinal cancers as well as in treating these cancers (11). To date, the existence of more than 130 glucosinolates has been reported which can be classified into three major structural groups i.e., aliphatic, indole, and aromatic glucosinolates (12). Biologically, the parent glucosinolate possesses negligible activity in preventing cancer progression. However, once it is converted into its derivatives, such as isothiocyanates, thiocyanates, nitriles, and indoles, the biological activity becomes potent. This conversion is mediated by the action of myrosinases during the mechanical digestion (e.g., chewing and cutting) of the vegetables (13). The anticancer activity of the cruciferous metabolites might be associated with several proposed mechanisms of action. It has been suggested that isothiocyanates and indoles play a pivotal role in regulating cell growth and cell cycle arrest which is essential in repairing the damaged DNA so that this prevents DNA alteration. The pro-apoptotic activity of these metabolites has also been reported. Other putative mechanisms include their activity in modulating oxidative stress and preventing the occurrence of angiogenesis (7). Anticancer activities of these metabolites are closely linked to their ability in upregulating p53 and its related target genes, e.g., p21 (14–16).
This review summarizes the metabolites obtained from various cruciferous vegetables and their potential uses in modifying the tumor-suppressive action of p53 proteins. Further, the correlation between the incidence of gastrointestinal cancers (gastric, small intestine, colon, liver, and pancreatic cancers) and the mutation of p53 is also described. This review provides information that is important for those who are interested in investigating further utilization of cruciferous vegetables in tackling cancers developed in the gastrointestinal system.
Cruciferous vegetables, often called Brassicaceae or mustard family, are commonly consumed globally in the human diet (17, 18). Brassica genus is the most popular among the Brassicaceae family and consists of 37 different species (19). Brassica oleracea are the principal Brassica vegetable species including cabbages, broccoli, brussels sprouts, kale, cauliflower, and others. Their cultivars are classified into seven main groups based on morphology and developmental forms: B. oleracea “capitata group” (cabbages), alboglabra group (Chinese broccoli or Kai-lan), acephala group (kale and collard greens), botrytis group (broccoflower, cauliflower, and Romanesco broccoli,), italica group (broccoli), gongylodes group (kohlrabi), and gemmifera group (Brussels sprouts) (17). Brassica rapa includes turnip, Asian greens, bok choy, Japanese mustard spinach, mizuna, rapini and napa cabbage are also consumed (Nikolov, 2019). Other species of Brassica are Brassica hirta (white mustard), Nasturtium officinale (watercress), Amoracia rusticana (horseradish), Eruca vesicaria (arugula), Lepidium sativum (garden cress), Raphanus sativus (radish), and Wasabia japonica (wasabi) (17). Cruciferous vegetables are high in nutrients and secondary metabolites such as sulfur-containing compounds, phenolic compounds, carotenoids, and others, known to have beneficial health properties.
Cruciferous vegetables have high macronutrients and micronutrients (20). Macronutrients are the nutritive components that provide energy and are required to maintain body functions. The macronutrients of different Brassica vegetables is shown in Table 1. Water is the main component of these vegetables, with ranges 89–92%, while the fiber and fat content are relatively low (6, 8). Brussels sprouts possess high carbohydrates compared to other vegetables (21).
Moreover, cruciferous vegetables are also rich of micronutrients (Table 2) such as minerals (calcium, potassium, magnesium, iron, phosphorus, sulfur, chlorine, sodium, zinc, and selenium) and vitamins (thiamine, riboflavin, niacin, folate, and tocopherol) (22). These vegetables contain calcium in the range of 22–150 mg/100 g. Kale is an important mineral source especially potassium, magnesium, calcium, and iron. On the other hand, kale also contains a high level of vitamin C and folates (19, 23). Broccoli and brussels sprouts comprise potentially useful amounts of nutritionally important minerals. In a fresh state, these vegetables also consist of catalase, peroxidase, and superoxide dismutase enzymes. However, the nutritional constituent of Brassica vegetables depends on diversity, growth environment, time of harvest, processing, and cooking conditions (11).
Among the bioactive compounds in brassica vegetables, sulfur-containing compounds such as glucosinolates are the major constituents (24). Glucosinolates are found in these vegetables in high quantities of 1,500–2,000 μg/g, especially in broccoli, brussels sprouts, and cabbage (19). Glucosinolates are responsible for their spicy taste and pungent odor (25). These compounds are water-soluble anions with the basic structure consisting of a β-D-glucopyranose moiety and β-thioglucoside N-hydroxysulfate with a variable side chain derived from amino acids (26). The glucosinolates are biosynthesized in brassica plants by major three steps of naturally occurring chemical reactions namely, side chain modification, side chain elongation and glucone biosynthesis (8). A small change in the side chain determines its classification, such as aliphatic (e.g., glucobrassicanapin, glucoalyssin, glucoraphanin, glucocapparin, dehydroerucin, glucoerucin, epi-progoitrin, glucoiberin, glucoerysolin, glucolepidin, progoitrin, and sinigrin), aromatic aryl (e.g., glucobarberin, glucotropaeolin, gluconasturtiin, glucosinalbin, and glucosibarin), and aromatic indoles (neoglucobrassicin, glucobrassicin, 4-methoxyglucobrassicin, and 4-hydroxyglucobrassicin) (27, 28). The hydrolysis pathways of glucosinolate can be seen in Figure 1.
Glucosinolates are chemically and thermally stable, but not biologically active until they are hydrolyzed (Figure 1) by the β-thioglucosidase or myrosinase enzymes. This enzyme is released after damage in the plant by chewing or processing such as cutting, chopping, and mixing (19). Upon the plant cells are injured, the thioglucosidic bond breaks down, β-thioglucoside yields a β-D-glucose molecule and thiohydroximate-O-sulfonate (an unstable aglycone) (29). Then, some breakdown products are formed depending on the pH level and other conditions (30). Hydrolysis of glucosinolates can also occur by gut microbiota action (25, 31). Both glucosinolates and their breakdown products play a large role in the health benefits of cruciferous vegetables. The more common breakdown products (Figure 1) include isothiocyanates (ITC), thiocyanate, amines, epithionitriles, indolic alcohols, nitriles, and oxazolidinethions (Figure 2) (12). The type and concentration of glucosinolates depends on cultivar, cultivation site, genotype, growth conditions, storage conditions, plant stage, preparation and cooking techniques (32). For example in cooking methods, glucosinolate levels were maximized in steaming compared to microwaving, boiling, and pressure cooking (33). Moreover, Casajús et al. found that the content of aliphatic glucosinolates decreased after storing broccoli in the darkness (34).
In a study by Hanschen et al., the formation of glucosinolates and their hydrolysis products of the five Brassica oleracea varieties including broccoli, cauliflower, and white, red, as well as savoy cabbages were determined. They reported that broccoli and red cabbage contains highest level of 4-(methylsulfinyl) butyl glucosinolate (glucoraphanin), whereas cauliflower, white cabbage and savoy cabbage mainly rich in 2-propenyl (sinigrin) and 3-(methylsulfinyl) propyl glucosinolate (glucoiberin). The sprouts of white cabbage is a rich source of the hydrolysis products such as epithionitriles or nitriles with 1-cyano-2,3-epithio propane with up to 5.7 μmol/g fresh weight. They also figured out that mini vegetable heads contained the highest concentrations of isothiocyanate (35). Similarly, Bhandari et al. evaluated the profiles of nine Brassica crops (baemuchae, broccoli, cabbage, Chinese cabbage, cauliflower, kale, leaf mustard, pakchoi, and radish) in various tissues: seeds, sprouts, mature roots, and shoots. Their results showed that total glucosinolate levels in most Brassica crops were highest in seeds and lowest in shoots. Aliphatic, indole, and aromatic glucosinolates were highest in the seeds, shoots, and roots tissues in most of the crops, respectively. The highest total glucosinolate levels were observed in seed and sprout of broccoli (110.76 and 162.19 μmol/g), whereas radish exhibited the lowest total glucosinolate levels across all tissues examined. On the other hand, leaf mustard showed the highest total concentration of glucosinolate in shoots (61.76 μmol/g) and roots (73.61 μmol/g) (36).
Carotenoids are highly pigmented constituents (red, yellow, or orange) and also responsible for the appearance of the cruciferous vegetables (37, 38). These phytochemicals are classified as symmetrical tetraterpenes that possess a C40 backbone structure with conjugated double bonds. A wide range of carotenoids are observed in these vegetables including lutein (Figure 3) and β-carotene as the predominant ones, followed by α-carotene, γ-carotene, β-cryptoxanthin, and lycopene presenting an antioxidant activity (39). Other carotenoids such as zeaxanthin, cryptoxanthin, neoxanthin, and violaxanthin have also been found in variable amounts (21, 40, 41).
Among the cruciferous vegetables, kale is considered the richest source of carotenoids, exceeding cabbage in about 40 times (18, 42). The main carotenoids present in kale are zeaxanthin and lutein, but important differences were identified among several cultivars of kale (41, 43, 44). Similarly to glucosinolates, the carotenoid accumulations in the brassica family are regulated by the developmental stage, environment, and tissue type (45). Tocopherols and tocotrienols (vitamin E) are lipid-soluble compounds present in cruciferous vegetables (39). Regarding the main tocopherols, α-tocopherol has been reported, but γ-tocopherols and δ-tocopherols are also detected (46, 47).
Phenolic compounds are ubiquitously distributed bioactive compounds found in most plant tissues, including cruciferous vegetables (10, 48). These compounds are characterized by having at least one aromatic ring and one or more hydroxyl groups bonded directly to the former, showing diversity of structures (49). Their categorized based on the number and arrangement of carbon atoms in flavonoids (flavones, flavonols, flavanones, flavan-3-ols, isoflavones, and anthocyanidins) and non-flavonoids (phenolic acids, hydroxycinnamates, and stilbenes) (48, 50–52). Cruciferous vegetables possess antioxidant activity attributes to the high contents of phenolic compounds (53, 54). The most important phenolic constituents (Figure 4) present in cruciferous vegetables are the hydroxycinnamic acids and the flavonoids such as kaempferol, isorhamnetin, quercetin, and rutin (11, 18).
The profile of phenolic compounds vary significantly among species. In studies carried out by Upadhyay et al., red cabbage presented high contents of rutin (102.14 μg/g of fresh weight) compared to green cabbage (1.34 μg/g of fresh weight) and cauliflower (39.07 μg/g of fresh weight). The sinapic acid content of red cabbage, broccoli, cauliflower, and green cabbage are 30.20, 7.37, 4.66, and 3.52 μg/g of fresh weight, respectively. They also detected that broccoli had a high content of gentisic acid (83.17 μg/g of fresh weight), followed by cauliflower and green cabbage with values of 35.50 and 2.50 μg/g of fresh weight, respectively (55). Furthermore, Li et al. identified 74 phenolic compounds of 12 different cruciferous vegetables, including 58 flavonoids and 16 hydroxycinnamic acids and their derivatives using ultra-high performance liquid chromatography-quadrupole time-of-flight mass spectrometry (UHPLC-Q-TOF-MS/MS). Among the flavonoids, the main compounds found were kaempferol, isorhamnetin, and glycosylated quercetin. The main hydroxycinnamic acids were caffeic, ferulic, sinapic, and p-coumaric acids. Emphasizing that the cauliflower and cabbage presented high contents of flavonoids (5.70 mg/g dry weight) and hydroxycinnamic acids (46.02 mg/g of dry weight) (48, 56, 57).
Another group of phenolic compounds frequently detected in Brassicaceae vegetables is anthocyanins (18). They are responsible for pigmentations of red cabbage and purple cauliflower. The common anthocyanins in Brassica crops are cyanidin, delphinidin, pelargonidin, petunidin, peonidin, and malvidin (21, 58). Broccoli sprouts and red cabbage contain mainly cyanidin glucosides derivatives (59). Red radish contains mainly cyanidin and peonidin anthocyanins acylated with aromatic acids (60).
Phytosterols are other compounds found in cruciferous vegetables (21). They are steroid alcohols with a molecular nucleus of 17 carbon atoms and a characteristic three-dimensional arrangement of four rings. Brassica napus L., known as rapeseed, is the rich source of phytosterols among cruciferous vegetables, with yields of up to 9.79 g/kg oil (18, 61). In addition, Brassica juncea is also the most abundant natural source of phytosterols (62).
Brassicaceae family containing phytoalexins possess an indolic ring with C3 substitutions with N and S atoms (63, 64). Phytoalexins are produced from brassinin, which confers a unique structure among other vegetables (64). They have been identified in Brassica juncea, Brassica napus, Brassica oleracea, Sinapis alba, Raphanus sativus, and Wasabi japonica (18, 65). Furthermore, fatty acids such as palmitic, stearic, oleic, linoleic, linolenic, eicosenoic, erucic, arachidic, arachidonic, and behenic acid also present in the oil extracted from Brassica crops (66).
As the “guardian of the genome,” the p53 family (p53, p63, and p73) plays a critical role in preventing the development of cancers associated with DNA damage (67). Following the activation of the p53 family by a number of cellular stress (e.g., oncogenes, oxygen deficiency in the blood, radiation, and oxidative stress), several mechanisms are activated as the mitigating system toward the affected DNA i.e., cell cycle arrest, apoptosis, and senescence (68). Given the crucial role of p53 in suppressing cancer development, mutations causing dysfunctionality of p53 put the body in danger. Mutations occurring in the p53 family have been found in more than half of human cancers (1). Of this figure, p53 family-related mutations are detected in more than 50% of gastrointestinal cancer cases (69). It has been demonstrated that most p53 mutations are identified as missense mutations with DNA-binding domain (DBD) as the main site for the mutations (67).
The three members of the p53 family are encoded by p53, p63, and p73 genes located in different chromosomes i.e., 17p13, 3q27-29, and 1p36, respectively (69). Nevertheless, they share the high identity of amino acid sequence in the main three structural domains namely transactivation (TAD), DBD, and oligomerization domains (OD) (68). The former domain is responsible for regulating the transcription activity of p53 by providing the binding sites for either positive or negative regulators of p53. Further, as the central domain, the DBD is important for binding to response elements of various target genes. Finally, the latter domain acts as the main site for DNA alternative splicing and post-translational modification. In the normal physiological condition, the expression of p53 in the cell is very low. Upon the exposure of the aforementioned cellular stress to the cells, p53 upregulates the expression of its main negative regulator namely murine/human double minute 2 (MDM2) which provides a negative feedback mechanism to maintain the minute levels of p53 in normal cells (68).
As stated above, activation of p53 could induce apoptosis through either the intrinsic or extrinsic pathways. While the former pathway involves the role of mitochondria, the latter apoptotic pathway is induced by death receptors (DRs) (70). Molecularly, the intrinsic pathway of apoptosis is initiated by the upregulation of several B-cell lymphoma-2 (Bcl-2) pro-apoptotic proteins (e.g., Bax, Bak, Noxa, and PUMA) and downregulation of Bcl-2 pro-survival proteins (e.g., Bcl-2, Bcl-w, and Bcl-XL) (71). Upon cellular stress, Bax and Bak experience oligomerization leading to the release of cytochrome c from the intermembrane space of mitochondria to the cytosol (72). As a response to the release of cytochrome c, oligomerization of the apoptotic protease activating factor-1 (APAF-1) occurs followed by the formation of apoptosome complex. This complex recruits and activates pro-caspase-9, an initiator caspase of the intrinsic apoptotic pathway, which is subsequently followed by the induction of several executioner caspases, mainly caspase-3 (73).
The extrinsic pathway of apoptosis involves the role of the superfamily of tumor necrosis factor receptors (TNFRs), also known as DRs, such as TNFR1, DR3, DR6, CD4 (TRAIL-R1), CD5 (TRAIL-R2), and CD95 (67, 74, 75). These receptors are characterized by the presence of the “death domain” in their structure located in the cytoplasmic region. Upon binding of the appropriate ligand to the DRs, several adaptor proteins are activated followed by the recruitment of the caspase-8 and caspase-10 known as the initiator caspases. Eventually, this cascade activates caspase-3 resulting in the occurrence of apoptotic events (74).
Another mechanism exerted by p53 as a response to cellular stress is the activation of cell cycle arrest. This mechanism acts as the checkpoint in which the cell is evaluated, checked, and repaired if there is damage, before moving to the other phases in the cell cycle. It has been known that the cell cycle is delicately regulated by cyclins. These proteins exert their actions on controlling the cell cycle through their interaction with another protein belonging to the cyclin-dependent kinases (CDKs) family (68). The activation of these proteins is essential for driving the cell cycle. Conversely, the inhibition of the CDKs could stop the cell cycle so that no cellular duplication and division occurs which is important in blocking the progression of cancer cells. At this point, the involvement of p21 is crucial as this protein could inhibit the CDKs. It has been demonstrated that p53 could induce the activation of p21 following cellular stress exposure. In addition to p21, other target genes of p53 are involved in facilitating cell cycle arrest. Some of those genes are 14-3-3σ, GADD45, and retinoblastoma protein (Rb) (67).
Senescence is a form of cell cycle arrest that can be induced upon exposure to various stimuli either exposed endogenously or exogenously (76). The senescent cells can be characterized by their bigger size, a significant abundance of nucleoli and vacuoles compared to the normal cells (77). Cellular senescence could also be induced following the activation of p53 through several related target genes e.g., p21, p16-Rb, and BTG2 (67, 76, 78). Compared to the previously described p53-related mechanisms, apoptosis and cell cycle arrest, senescence also plays a pivotal role in preventing the progression of cancerous cells.
In addition to these three main mechanisms of p53, other functions of p53 have also been deciphered. Those functions include p53 involvement in preventing cancer migration and metastasis, angiogenesis, cellular metabolism, oxidative stress, drug resistance, inducing autophagy, and promoting genomic stabilization (67, 79–84). Taken together, all of these mechanisms are crucial for preventing the genome from being cancerous.
As stated above, p53 mutations are found as the most mutated gene in many types of cancers, including cancers developed in the gastrointestinal tract, such as colorectal cancer (85, 86). p53 mutations can be detected in 34% of colon cancers occurring in the proximal area and in 45% of those occurring in the distal area of the colon (87). In addition to p53 genes, a number of other genes also experience mutations in colorectal cancer. However, a study conducted by Wood and co-workers indicated four other commonly mutated genes i.e., KRAS, APC, PIK3CA, and FBXW7 (86).
Furthermore, p53 mutations in colorectal cancer mainly occur in exon 5 to exon 8 in the DBD (p53 has 11 exons and 10 introns) (88). Throughout these vulnerable exons, several codons have been identified as the preferred sites of mutations (Table 3). For example, in exon 5, a missense mutation in codon 175 causes the failure to produce arginine. As a result, the codon is translated into histidine. This mutation occurs as codon CGC is changed into CAC. Another example is exhibited by missense alteration occurring in codon 282 in exon 8 where codon CGG (arginine) is changed into TGG (tryptophan) (68). Some other codons that are commonly vulnerable to mutations are codons 245, 248, and 273.
Table 3. Several common codons that are vulnerable to cellular stress leading to missense mutations (68).
These data emerge other interesting questions. For example, why these exons and codons become the preferred sites of p53 mutations and why specific amino acid (e.g., arginine) seems to have higher vulnerability over the other amino acids (68). It has been demonstrated that p53 mutations are closely associated with several events leading to the malignancies. p53 mutations have a clear association with the emergence and development of cancer. Lopez et al. found that p53 mutations can be detected in more than 50% of colorectal cancer occurring sporadically (89). Not only, colorectal cancer, p53 mutations are also identified in high percentage in other types of gastrointestinal cancers. It is estimated that p53 mutations are involved in up to 77% of stomach cancer (90).
In addition, as in the other cancers, p53 mutations also show a close correlation with the progression and invasiveness of gastrointestinal cancer. One example is given by Russo et al. found that p53 mutations were closely linked to the invasiveness of colorectal cancer which is related to its ability to reach lymphatic and blood circulation providing a great chance to be metastatic to the other parts of the body (87).
Most importantly, p53 mutation could diminish the effectiveness of chemotherapeutic agents (e.g., doxorubicin, temozolomide, tamoxifen, and gemcitabine) (80). Several mechanisms have been proposed to explain the emergence of this drug resistance (91, 92). p53 mutation could inhibit the entry of the chemotherapeutics so that the intracellular level of the drugs is inefficient in exerting their anticarcinogenic activities. Another proposed mechanism is related to the overexpression of ABC transporters, such as ABCB1 (p-glycoprotein) in cancerous cells, making the efflux of the drugs occurs extensively. The increased drug metabolism is also observed in p53 mutation which eventually causes extensive inactivation of the chemotherapeutics (91). Another study found that this effect was associated with the failure of the mutant p53 in upregulating PUMA expression (93). Although this study was only focused on colon cancer, this finding might correlate with the other types of cancer and provide a new insight for tackling mutant p53-related chemoresistance.
Molecularly, the involvement of the Wnt signaling pathway and epithelial-mesenchymal transition (EMT) in mediating pro-carcinogenic effects of the mutated p53 have attracted much interest. It has been found that proliferation, invasiveness, and development of colorectal cancer are facilitated by the upregulation of Wnt and EMT genes (94, 95). In the normal states, p53, in collaboration with microRNA-34, acts as a suppressor of the Wnt signaling pathway (94). A more recent study carried out in vivo investigating gastric cancer suggested that dysfunctionality of the wild-type p53 was accompanied by induction of Wnt and EMT. Also, this study found that loss of p53 function was negatively correlated with cyclooxygenase-2 (COX-2) levels indicating the role of inflammation in the progression of gastrointestinal cancer (96). In sum, the inhibition of these pathways (Wnt, EMT, and COX-2) might be useful in the effort of developing new chemotherapeutic agents.
Several drug candidates have been developed with promising potency in tackling the pro-carcinogenic effects of the mutated p53, especially in gastrointestinal cancers. The candidates working in inhibiting MDM2 activity may be the most attractive approach (97). As previously stated, MDM2 is the negative regulator of p53. It binds to the N-terminal of p53 initiating ubiquitination of the protein resulting in p53 degradation. It means that the excessive activity of MDM2 could lead to the significant inhibition of p53. As a result, this affects the tumor-suppressive function of p53. Conversely, inhibition of MDM2 could stabilize p53 protein (98).
Recently, several candidates with anti-MDM2 activities have been found and developed. Of those, MI-43 and nutlins are the most potent candidates. It has been reported that the former candidate has the ability to antagonize the action of MDM2 on p53. As a result, MI-43 could induce p53 accumulation leading to the activation of its target genes, such as p21, Noxa, and PUMA (99). A study reported that MI-43 could induce cell cycle arrest and apoptosis in colon cancer (100). Nutlins also have the ability to stabilize p53 by inhibiting MDM2 activity. Among the other Nutlins, Nutlin-3 activity in antagonizing MDM2 has been reported in the literature. In colorectal cancer, Nutlin-3 binds to the MDM2 pocket so that it disturbs the interaction between MDM2 and p53. As a result, p53 is stabilized and its downstream target genes are activated. However, it is noteworthy that Nutlin-3 has a great affinity only in MDM2, but not in the other members of the MDM family, such as MDMX. Consequently, cancer cells expressing abundant MDMX cannot be an appropriate target for Nutlin-3 (101).
Another MDM2 inhibitor with potential use in suppressing the growth of colon cancer is RITA (reactivation of p53 and induction of tumor cell apoptosis). Unlike the other MDM2 inhibitors that have been described above, this candidate binds directly to p53 instead of MDM2. This binding leads to the conformational change of p53 resulting in the interference with MDM2-p53 interaction (102).
As stated previously, in addition to p53, the p53 family has two other proteins, p63 and p73. It has been demonstrated that targeting these members of the p53 family could be a promising strategy in developing anticancer drugs. For example, a derivative of ellipticine (a plant alkaloid), NSC176327, could kill the cancerous cells of colorectal cancer which is independent of p53 status. Also, this derivative could activate p73 and the target genes of the p53 family (e.g., p21 and DR5). Further, the loss of the p73 function plays a significant role in the emergence of chemoresistance (47).
Reactivation of mutant p53 is another strategy developed in the effort of seeking anticancer drugs. PRIMA-1 (p53-reactivation and induction of massive apoptosis-1) is an example of drugs utilizing this strategy (103). Reactivation of the mutated p53 by PRIMA-1 is linked to its covalent binding in DBD resulting in the restoration of a certain sequence in the core domain (104). Eventually, activation of p53 target genes in the cancer cells occurs. A more recent study reported the use of polysaccharides isolated from Ganoderma lucidum as an agent for restoration of the suppressive action of the mutated p53 in colorectal cancer (105).
Many biological activities, such as DNA repair, cell cycle arrest, and apoptosis, depend on the tumor suppressor p53 (106). Approximately 50% of all malignancies in humans have p53 mutations (106). The majority of p53 mutations occur in the central core DNA-binding domain (DBD), which substantially impairs p53's ability to bind DNA and prevent tumor growth. Mutant p53 reduces the DNA-damage response and increases the resistance of tumor cells to drug-induced apoptosis (107). Additionally, there is evidence that suggests mutant p53 causes cancer by gaining function by transactivating genes related to growth or by silencing particular target genes (107). Increased tumorigenicity in nude mice and improved soft agar plating efficiency are both results of mutant p53 overexpression in deficient cells (107). In addition, p53 point mutation-carrying animals develop tumors and spread them more frequently than p53-deficient mice (108). Li-Fraumeni Syndrome, a germ-line p53 mutation, dramatically raises the chance of developing cancer in humans (108). Therefore, eliminating mutant p53 may present a viable strategy for cancer therapy and prevention. Cruciferous vegetables compounds have the capacity to arrest the cell cycle or induce apoptosis in cells by activating p53. For instance, carotene promotes the production of p53, p21, and BAX in cancer cells, which are all tumor suppressors (109). Activation of p53 and its targets p21, BAX, and RPRM led to the observation of the induction of cell cycle arrest when combined with bixin and canthaxanthin (110, 111). Apoptosis was seen in breast and bladder cancer cells after treatment with beta-carotene, phenethyl isothiocyanate, and allicin (112–114). The activation of BAX, Bcls, SAS, PERP, and LRDD resulted in this apoptosis, which was dependent on the protein p53. Natural compounds may be used to target these signaling pathways, ultimately halting the growth of cancer. For instance, astaxanthin, lycopene, and isothiocyanates, individually, have been found to induce p53-mediated apoptosis. The ERK, PI3K/Akt, and p21WAF1 pathways were activated in order to do this (115–117). Pharmacologically, therefore, MDM2 inhibition has become a promising mechanism utilized by a drug to exert its anticancer activity (118). Growth factors also play a crucial role in the development of cancer, including insulin-like growth factor (IGF), PDGF, EGF, tumor growth factor (TGF), FGF, and colony-stimulating factor (CSF). These substances stimulate signaling pathways, which in turn promote increased cell proliferation, block apoptosis, and allow cancer cells to invade healthy cells. Numerous downstream signaling pathways, including PI3K-AKT, Ras-MAPK, and others, are activated as a result of growth factor receptor activation (119). Additionally, it has been shown that isothiocyanates interact with the bladder epithelium and activate the cytoprotective enzymes GST and NQO1, which are known to detoxify carcinogens. Curiously, NQ01 has also been demonstrated to stabilize the p53 tumor suppressor. Additionally, it was shown that the bladder is one of the most receptive tissues to the activation of these enzymes by broccoli sprout extracts, suggesting that it may be particularly useful for guarding the bladder against the development of cancer (108). On the other side, angiogenesis makes cancer cells more resistant to the effects of chemotherapy and radiation while also playing a crucial role in the development, spread, and starts of cancer. In HUVEC, capillary tubes' ability to survive, migrate, and develop was diminished by both quercetin and benzyl isothiocyanate (120, 121). They also have success reducing the expression of angiogenic and metastatic markers. Additionally, it has been shown in a number of malignancies that methylation of the promoter regions of tumor-suppressor genes silences those genes (122). In addition, methylation of certain genes has been linked to resistance to radiation and chemotherapy (119). The suppression and reversal of the DNA methylation process may be one of the mechanisms by which natural substances exert their chemopreventive impact. In order to repair damaged DNA, it has been suggested that fucoxanthin and sulforaphane may inhibit and reverse DNA methylation, increase histone acetylation, and modify the structure of chromatin (123, 124). In summary, Crusiferous vegetable chemicals led to an increase in the tumor suppressor p53 protein level and transcriptional activity, which was accompanied by an increase in the levels of p21WAF1 and Bax, two of p53's transcriptional targets. Despite the fact that p21 is increased during p53-mediated G1 arrest, this occurrence does not lead to p53-induced apoptosis. The Bax/Bcl-2 ratio was altered by the p53-dependent increase in Bax expression, which also coincided with the activation of caspases 9 and 3 and PARP cleavage (109). Bax siRNA transfection of cells reversed these effects and prevented death, but it had no impact on the buildup of G1 cells. In conclusion, we suggest that p53-dependent pathways play a major role in cruciferous vegetable compound-mediated growth arrest and apoptosis. The area of the current investigation was to determine the function of p53 in cancer prevention and to clarify how it contributes to cell cycle arrest and apoptosis caused by Cruciferous vegetables compounds.
Gastrointestinal (GIT) cancers, including those of the esophagus, stomach and colon, are closely associated with lifestyle factors, particularly diet (125). Dietary variables are thought to be responsible for one-third of all cancer deaths. A diet high in cruciferous vegetables like brussels sprouts and broccoli is linked to a lower risk of several cancers of gastro-intestinal tract (GIT). It has been found that cruciferous vegetables protect against GIT cancers more effectively than a diet rich in fruits and vegetables. p53 is a transcription factor that inhibits tumor growth and suppresses tumor growth. This protein regulates a wide range of physiological processes, including cell signaling, DNA damage response, genomic integrity, cell cycle regulation, and apoptosis. To stop cancerous or damaged cells from multiplying, p53 activates genes such as p21WAF1 and Bax, which in turn activate the apoptotic pathway. A lack of p21, a regulator of cell division, is caused by a failure of p53 to bind DNA, and lead to unchecked cell proliferation resulting in tumors (126). The tumor suppresses p53 during cancer cell growth (either silenced or mutated). In order to control cancer cell development, angiogenesis, and cancer cell migration, p53 must be expressed in cancer cells (127).
Isothiocyanates (ITCs), a hydrolysis product of glucosinolates present in cruciferous vegetables, are chemoprotective compounds synthesized by the enzyme myrosinase. Gastric cancer may benefit from the use of potential ITCs such as PEITC (phenethyl isothiocyanate), BITC (benzyl isothiocyanate), and SFN (sulforaphane) (128, 129). An anticancer possibility for gastric cancer has been examined using the nontoxic indole derivative 3,3'-Diindolylmethane (DIM) from cruciferous vegetables (130). It was observed that DIM inhibits gastric cancer growth in vitro and in vivo, depending on the dose, by Ye et al. (131). By stimulating the Hippo signaling system, DIM slowed the growth of gastric cancers in a xenograft mouse model, according to Li et al. Reduced synthesis of CDKs (cyclin-dependent kinases) 2, 4 and 6, as well as cyclin D1, allowed for G1 cell cycle arrest while increasing levels of p53 protein (132). Studies on laboratory animals have shown that carotenoids (lycopene, lutein, and β-carotene) prevent the formation and growth of chemotherapeutically produced gastric tumors (133, 134). There was an investigation into the effects of oral lycopene supplements in ferrets by Liu et al., who found that the p53 gene, its target genes (p21Waf1/Cip1 and Bax-1), and gastric mucosal cell proliferation and apoptosis were all affected by lycopene supplementation (135). Bax (Bcl-2 family member) and P21waf1/cip1 (CDK inhibitor) are both critical for apoptosis and G1 cell cycle arrest, respectively. p21waf1/cip1 and Bax-1 act together as mediators for the enhancement of p53-dependent necrosis (136). There was a 9-week experiment in which ferrets were exposed to cigarette smoke and given either modest or high dosages of lycopene supplementation. Ferrets given lycopene alone had significantly more lycopene in their gastrointestinal mucosa than ferrets given lycopene, and exposed to smoke, a finding that was dose-related (Figure 5). Lycopene dramatically reduced both the smoke-induced total p53 and phosphorylated p53 levels in ferrets that had recently been exposed to smoke, regardless of the level of total p53 and phosphorylated p53. In ferrets exposed to smoke alone, cell death indicators such p21 (Waf1/Cip1), Bax-1, and cleaved caspase 3 were significantly reduced, whereas lycopene dose-dependently mitigated the effects of smoke on these same markers as well as cyclin D1 and PCNA (Table 4) (135). Reactive oxygen species (ROS)-induced phosphorylation of p53 at serine 15 improves p53 accumulation and activation to prevent stomach cancer (159). It is possible to induce apoptosis in gastric cancer cells by activating p53 and upregulating the transcription of key target genes such as p21 (which is involved in cell cycle arrest) and pro-apoptotic BH3-only Bcl-2 family proteins, such as Bad, Noxa, BH3 interacting domain death agonist (Bid), and Bcl-2-like protein 11 (Bim; activator of BH3). Anti-apoptotic proteins like Bcl-2 and Bcl-2xL can be rendered inactive by the BH3 protein, a BH3-only sensitizer. There is a competition for the binding of BH3-only proteins like Bax and Bak, which results in their displacement from anti-apoptotic proteins like Bax and Bak (160).
Figure 5. Functional foods bioactive compounds from cruciferous vegetables targeting p53 Family in gastrointestinal tract and associated cancers.
Table 4. Functional ingredients from cruciferous vegetables in gastrointestinal tract and associated cancers.
The presence of ITCs, particularly BITC, has been linked to a lower risk of small intestine cancers or cancer consequences in people who eat cruciferous vegetables. Cruciferous vegetables may contain ITCs that may be useful in the treatment of small intestinal malignancies, including BITC, PEITC, and SFN (128, 129). Thioredoxin reductase (TR), a selenoprotein that lowers thioredoxin and controls cell development by providing the reducing power for p53 and the redox cycling of endogenous antioxidants including vitamin C, lipoic acid, and niacin, can be made more active by ITCs and carotenoids present in cruciferous vegetables (Figure 4) (141). Apoptosis of cancer cells can be induced by increasing the expression and stability of the cell-killing protein p53, as well as PEITC and sulforaphane's role in p53 stabilization and nuclear localization (141, 142). Jang et al. and Shin et al. reported that carotene can promote apoptosis in intestinal cellular epithelium by boosting p53 and lowering Bcl-2, which is anti-apoptotic (Figure 5) (133, 161). Kim et al. conducted an in vitro investigation to evaluate the mechanism of astaxanthin's anticancer effects on small intestine carcinoma cell lines. Astaxanthin slowed down the growth of small intestinal cancer cells. The phosphorylation of extracellular signal-regulated kinase (ERK) was suppressed, and the expression of p53 was shown to rise as a result (Table 4) (117).
Oncogenes including K-ras and adenomatous polyposis coli (APC), as well as tumor suppressor genes like Smad4 and p53, have a critical role in colon cancer formation (162, 163). PI3K/AKT/mTOR pathway and p53 pathway abnormalities are the most prevalent anomalies in most colon cancer cells (164, 165). Blocking the phosphatidylinositol-3-kinases (PI3K/AKT) pathways while activating the p53 pathway; slows the growth of cancer cells. These pathways affect glucose metabolism, apoptosis, cell proliferation, and migration (166). ITCs present in cruciferous vegetables such as BITC, PEITC and sulforaphane have antimetastatic properties against colon cancer. These ITCs block the (PI3K)/AKT-dependent survival pathway of colon cancer cell lines, while stimulating the p53 pathway. In addition, they increase apoptosis-related proteins due to activation of p53-family genes, while decreasing metastasis-related proteins. Because of all these reasons, BITC, PEITC and sulforaphane were capable of ameliorating the inflammation associated with colon cancer (116, 167). In HT29 colon cancer cells, BITC and PEITC have been demonstrated to have anti-metastatic and anti-inflammatory effects against colon cancer, and it slowed the migration of colon cancer cells through the activation of p53 pathway (147, 168, 169). Sulforaphane (SFN)-induced acetylation of a DNA repair protein causes DNA damage in colon cancer cells (Figure 5) (123). The p53-stimulated apoptosis of colon cancer cells is induced by activation of detoxifying enzymes, the release of cytochrome c, and the stimulation of poly(ADP-ribose) polymerase (PARP) proteolysis (170–172). Apoptosis occurs as a result of cell cycle arrest (173). Using human colorectal cancer cells as a model, Myzak et al., found that sulforaphane decreased histone deacetylase (HDAC) activity while simultaneously increasing histone acetylation (174). HDAC inhibition has been linked to an increase in the transcription of p53 gene, which is a tumor-suppressor gene. Lycopene and canthaxanthin are two carotenoids that inhibited colon carcinoma cell proliferation by altering the cell cycle (175). As a result of cell cycle arrest due to activation of p53 pathway, astaxanthin was demonstrated to reduce cell proliferation and trigger death in cancer cells (Table 4) (117). The study by Palozza et al., found that Haematococcus pluvialis reduced cyclin D1 levels in colon cancer cells while increasing p53 and p21WAF-1/CIP1 levels in the cells (117). As a result of these findings, it can be concluded that the G1-phase cell cycle is blocked. In addition, β-carotene extracted from cruciferous vegetable has been demonstrated to increase Bax and P53 levels in malignant colon cells while decreasing Bcl-2 levels (149, 176).
Anti-apoptotic genes overexpressed and tumor suppressor genes mutated, such as p53, are the first signs of tumor formation in the liver and pancreatic (158, 177). Several carotenoids in cruciferous vegetables, including bixin, β-cryptoxanthin, lutein, lycopene, astaxanthin, and fucoxanthin, have been examined for their function in triggering apoptosis in hepatic and pancreatic cancer cells through ROS generation. When the anti-apoptotic Bcl-2 and xL proteins, as well as proteins associated with B-cell lymphoma 2 (Bcl-2), are inhibited and pro-apoptotic proteins like p21, p27, and p53 are activated, hepato-pancreatic cancer cells might undergo apoptosis (149, 178). Dietary lutein raised the mRNA expression of proapoptotic genes p53 and Bax, decreased the expression of antiapoptotic gene Bcl-2, and increased the Bax:Bcl-2 ratio in hepato-pancreatic cancers (Figure 5). Activation of the p53 tumor suppressor gene causes apoptosis, DNA damage tolerance, and DNA repair (Table 4) (179). Further studies have demonstrated that the cruciferous vegetable isothiocyanates stimulate the GST and NQO1 enzymes renowned for their ability to remove carcinogens from the cells of the hepatic and pancreatic epithelial tissue. NQ01 appears to stabilize the tumor suppressor p53, according to certain research results. Liver and pancreas are the tissues susceptible to the stimulation of these enzymes by broccoli sprout extracts, which may be particularly effective in protecting these organs against the onset of cancer (119, 180).
The ability of cruciferous bioactive chemicals to suppress cancers in experimental animals has been documented in numerous studies. At the same time, the research studies conducted on human beings are very less. Isothiocyanates, indole-3-carbinol, and phytoalexins are among the bioactive chemicals in cruciferous vegetables studied for prevention of cancer of gastro-intestinal tract and other associated cancers (181). Cell cycle, apoptosis, genomic integrity, and DNA repair in human beings are all controlled by the tumor suppressor gene p53. Angiogenesis inhibition, cell cycle inhibition, and genetic stability come from the activation of particular genes by activated p53 gene (19). In addition, active p53 can operate as both a transactivator and a transrepressor at the same time. Activating p53 by the bioactive chemicals present in cruciferous vegetables may cause cell cycle arrest or death in cancerous cells of gastro-intestinal tract (129, 182). Carotenoids, for example, activates p53 and its targets p21 and Bax in gastric and colon cancer cells (183). Cell cycle arrest and apoptosis were both induced by lutein, which also activated the p53 gene's targets, including p21, Bax and PUMA (p53-upregulated modulator of apoptosis). Using ITCs, human gastric and colon cancer cells were able to apoptosis through the p53-dependent BAX induction. Apoptosis and ROS (reactive oxygen species) generation are two ways that ITCs limit tumor growth in body tissues of human beings. As ITCs and β-carotene activates p53's mitochondrial translocation, it has been shown to incite cell death through p53-mediated mechanisms. There are two closely related proteins in mammalian cells that when stimulated can cause apoptosis, similar to p53 protein, which are known as p63 and p73 (149). Indole-3-carbinol and phytoalexins have been found to induce apoptosis and cell cycle arrest in HT-29 human colon cancer cells (8). DNA damage checkpoints are essential for genome integrity because they stop the progression of the cell cycle when DNA damage or incomplete replication occurs, and the arrest of the G1 checkpoint occurs in mammalian cells because p53 protein mediates the action (182). Li et al. investigated the effects of indole-3-carbinol extracted from cruciferous vegetables on p53 protein induction. Indole-3-carbinol therapy enhanced the p53 protein level in human gastric cancer cell lines, causing cell cycle arrest in the G1 phase (132). By modifying the amounts of proteins that control cell cycle progression, indole-3-carbinol prevented human gastric cancer cells from entering the G1 phase. Glucosinolate compounds present in seeds of cruciferous vegetables, such as sinigrin, have been demonstrated to dramatically suppress hepatotumor cell proliferation when administered through the p53 pathway. Sinigrin, a major glucosinolate in cruciferous vegetable seeds, has been shown to suppress the growth of human liver cancer cells in a way that is dependent on the p53 signaling pathway (184, 185). More research studies should be conducted in the near future to attain further more development with regard to clinical applications of bioactive compounds available from cruciferous vegetables in human beings.
A synopsis of the cancer-preventive potential of numerous Brassicaceae family members has been reported in this review. Even though there is a strong correlation between the prevention of carcinogenesis and consumption of cruciferous vegetables, it must be emphasized that many more studies are needed to fully understand the influence of these functional foods bioactive compounds on the human body. The upcoming experiments must specifically address the questions of bioavailability, stability, transport, and metabolism. These substances may have synergistic effects. It needs to be confirmed in further studies. In terms of the cancer-preventive characteristics of phytochemicals included in these veggies, the additional effects of typical food preparation techniques constitute another component that has yet to be fully investigated. Numerous studies aided in the acceptance of dietary agents as cancer treatment options. Due to their anti-tumorigenic and anti-proliferative competences, cruciferous vegetables are abundant with many functional bioactive compounds that have noteworthy inhibitory effects on different pathways of cancer cells. These veggies are advantageous because they are precursors to glucosinolates, which are precursors to isothiocyanates like sulforaphane and indoles like indole-3-carbinol. HDAC and DNMT overexpression, as well as miRNA misexpression, are common features of most malignancies. I3C, SFN, and I3C are regulators and inhibitors of these processes, and their usage causes malignant cell lines to seem more normal and healthier. With the inclusion of SFN and I3C, an increase in programmed cell death, as well as substantial reductions in uncontrolled cell proliferation, have been observed. Studies showed that functional bioactive compounds from cruciferous vegetables are potential candidates to fight cancer. Future research will likely focus on determining the epigenetic events influenced by the bioactive components of cruciferous vegetables and their importance in not just cancer prevention but also a variety of other biological systems.
SM, DC, and TE conceptualized and designed the manuscript, participating in drafting the article and/or acquisition of data, and/or analysis and interpretation of data. SM, BZ, RD, SSM, AM, MS, and FN participating in drafting the article and prepared the figures and tables. SM, TE, MK, AI, and JS-G wrote, edited, and revised the manuscript critically. TE and JS-G revised the final written. All authors critically revised the manuscript concerning intellectual content and approved the final manuscript.
The authors express their gratitude to Research Center of Advanced Materials, King Khalid University, Saudi Arabia, for support (award number KKU/RCAMS/22). Funding for open access charge: Universidade de Vigo/CISUG.
The authors declare that the research was conducted in the absence of any commercial or financial relationships that could be construed as a potential conflict of interest.
All claims expressed in this article are solely those of the authors and do not necessarily represent those of their affiliated organizations, or those of the publisher, the editors and the reviewers. Any product that may be evaluated in this article, or claim that may be made by its manufacturer, is not guaranteed or endorsed by the publisher.
1. Ozaki T, Nakagawara A. Role of p53 in cell death and human cancers. Cancers. (2011) 3:994–1013. doi: 10.3390/cancers3010994
2. Mitra S, Lami MS, Ghosh A, Das R, Tallei TE, Islam F, et al. Hormonal therapy for gynecological cancers: how far has science progressed toward clinical applications? Cancers. (2022) 14:759. doi: 10.3390/cancers14030759
3. Siegel RL, Miller KD, Goding Sauer A, Fedewa SA, Butterly LF, Anderson JC, et al. Colorectal cancer statistics, 2020. CA Cancer J Clin. (2020) 70:145–64. doi: 10.3322/caac.21601
4. Morrison MEW, Joseph JM, McCann SE, Tang L, Almohanna HM, Moysich KB. Cruciferous vegetable consumption and stomach cancer: a case-control study. Nutr Cancer. (2020) 72:52–61. doi: 10.1080/01635581.2019.1615100
5. Mitra S, Anjum J, Muni M, Das R, Rauf A, Islam F, et al. Exploring the journey of emodin as a potential neuroprotective agent: novel therapeutic insights with molecular mechanism of action. Biomed Pharmacother. (2022) 149:112877. doi: 10.1016/j.biopha.2022.112877
6. Mitra S, Paul S, Roy S, Sutradhar H, Emran T, Nainu F, et al. Exploring the immune-boosting functions of vitamins and minerals as nutritional food bioactive compounds: a comprehensive review. Molecules. (2022) 27:20555. doi: 10.3390/molecules27020555
7. Esteve M. Mechanisms underlying biological effects of cruciferous glucosinolate-derived isothiocyanates/indoles: a focus on metabolic syndrome. Front Nutr. (2020) 7:111. doi: 10.3389/fnut.2020.00111
8. Manchali S, Chidambara Murthy KN, Patil BS. Crucial facts about health benefits of popular cruciferous vegetables. J Funct Foods. (2012) 4:94–106. doi: 10.1016/j.jff.2011.08.004
9. Mitra S, Lami MS, Uddin TM, Das R, Islam F, Anjum J, et al. Prospective multifunctional roles and pharmacological potential of dietary flavonoid narirutin. Biomed Pharmacother. (2022) 150:112932. doi: 10.1016/j.biopha.2022.112932
10. Mitra S, Tareq AM, Das R, Emran T, Nainu F, Chakraborty AJ, et al. Polyphenols: a first evidence in the synergism and bioactivities. Food Rev Int. (2022) 2022:1–23. doi: 10.1080/87559129.2022.2026376
11. Melim C, Lauro MR, Pires IM, Oliveira PJ, Cabral C. The role of glucosinolates from cruciferous vegetables (Brassicaceae) in gastrointestinal cancers: from prevention to therapeutics. Pharmaceutics. (2022) 14:10190. doi: 10.3390/pharmaceutics14010190
12. Connolly EL, Sim M, Travica N, Marx W, Beasy G, Lynch GS, et al. Glucosinolates from cruciferous vegetables and their potential role in chronic disease: investigating the preclinical and clinical evidence. Front Pharmacol. (2021) 12:767975. doi: 10.3389/fphar.2021.767975
13. Kissen R, Rossiter JT, Bones AM. The “mustard oil bomb”: Not so easy to assemble?! Localization, expression and distribution of the components of the myrosinase enzyme system. Phytochem Rev. (2009) 8:69–86. doi: 10.1007/s11101-008-9109-1
14. Fimognari C, Nüsse M, Berti F, Iori R, Cantelli-Forti G, Hrelia P. Cyclin D3 and p53 mediate sulforaphane-induced cell cycle delay and apoptosis in non-transformed human T lymphocytes. Cell Mol Life Sci. (2002) 59:2004–12. doi: 10.1007/PL00012523
15. Fimognari C, Nüsse M, Cesari R, Iori R, Cantelli-Forti G, Hrelia P. Growth inhibition, cell-cycle arrest and apoptosis in human T-cell leukemia by the isothiocyanate sulforaphane. Carcinogenesis. (2002) 23:581–6. doi: 10.1093/carcin/23.4.581
16. Matsui TA, Murata H, Sakabe T, Sowa Y, Horie N, Nakanishi R, et al. Sulforaphane induces cell cycle arrest and apoptosis in murine osteosarcoma cells in vitro and inhibits tumor growth in vivo. Oncol Rep. (2007) 18:1263–8. doi: 10.3892/or.18.5.1263
17. Šamec D, Salopek-Sondi B. Cruciferous (brassicaceae) vegetables. Nonvitamin Nonmineral Nutr Suppl. (2018) 8:195–202. doi: 10.1016/B978-0-12-812491-8.00027-8
18. Ramirez D, Abellán-Victorio A, Beretta V, Camargo A, Moreno DA. Functional ingredients from brassicaceae species: overview and perspectives. Int J Mol Sci. (2020) 21:61998. doi: 10.3390/ijms21061998
20. Tiwari JN, Tiwari RN, Kim KS. Zero-dimensional, one-dimensional, two-dimensional and three-dimensional nanostructured materials for advanced electrochemical energy devices. Prog Mater Sci. (2012) 57:724–803. doi: 10.1016/j.pmatsci.2011.08.003
21. Favela-González KM, Hernández-Almanza AY, De la Fuente-Salcido NM. The value of bioactive compounds of cruciferous vegetables (Brassica) as antimicrobials and antioxidants: a review. J Food Biochem. (2020) 44:13414. doi: 10.1111/jfbc.13414
22. Kmiecik W, Lisiewska Z, Korus A. Retention of mineral constituents in frozen brassicas depending on the method of preliminary processing of the raw material and preparation of frozen products for consumption. Eur Food Res Technol. (2007) 224:573–9. doi: 10.1007/s00217-006-0337-6
23. Cartea ME, Francisco M, Soengas P, Velasco P. Phenolic compounds in Brassica vegetables. Molecules. (2011) 16:251–80. doi: 10.3390/molecules16010251
24. Miekus N, Marszałek K, Podlacha M, Iqbal A, Puchalski C, Swiergiel AH. Health benefits of plant-derived sulfur and organosulfur compounds. Molecules. (2020) 25:173804. doi: 10.3390/molecules25173804
25. Barba FJ, Nikmaram N, Roohinejad S, Khelfa A, Zhu Z, Koubaa M. Bioavailability of glucosinolates and their breakdown products: impact of processing. Front Nutr. (2016) 3:24. doi: 10.3389/fnut.2016.00024
26. Abbaoui B, Lucas CR, Riedl KM, Clinton SK, Mortazavi A. Cruciferous vegetables, isothiocyanates, and bladder cancer prevention. Mol Nutr Food Res. (2018) 62:79. doi: 10.1002/mnfr.201800079
27. Melrose J. The glucosinolates: a sulphur glucoside family of mustard anti-tumour and antimicrobial phytochemicals of potential therapeutic application. Biomedicines. (2019) 7:v2. doi: 10.20944/preprints201906.0042.v2
28. Wang M, Zhang Y, Leng C, Li X, Wang P, Gu Z, et al. Glucosinolates metabolism and redox state of rocket (Eruca sativa Mill) during germination. J Food Process Preserv. (2019) 43:14019. doi: 10.1111/jfpp.14019
29. BlaŽević I, Montaut S, Burčul F, Olsen CE, Burow M, Rollin P, et al. Glucosinolate structural diversity, identification, chemical synthesis and metabolism in plants. Phytochemistry. (2020) 169:112100. doi: 10.1016/j.phytochem.2019.112100
30. Bell L, Yahya HN, Oloyede OO, Methven L, Wagstaff C. Changes in rocket salad phytochemicals within the commercial supply chain: glucosinolates, isothiocyanates, amino acids and bacterial load increase significantly after processing. Food Chem. (2017) 221:521–34. doi: 10.1016/j.foodchem.2016.11.154
31. Luang-In V, Narbad A, Nueno-Palop C, Mithen R, Bennett M, Rossiter JT. The metabolism of methylsulfinylalkyl- and methylthioalkyl-glucosinolates by a selection of human gut bacteria. Mol Nutr Food Res. (2014) 58:875–83. doi: 10.1002/mnfr.201300377
32. Maina S, Misinzo G, Bakari G, Kim HY. Human, animal and plant health benefits of glucosinolates and strategies for enhanced bioactivity: a systematic review. Molecules. (2020) 25:163682. doi: 10.3390/molecules25163682
33. Shakour ZT, Shehab NG, Gomaa AS, Wessjohann LA, Farag MA. Metabolic and biotransformation effects on dietary glucosinolates, their bioavailability, catabolism and biological effects in different organisms. Biotechnol Adv. (2022) 54:107784. doi: 10.1016/j.biotechadv.2021.107784
34. Casajús V, Demkura P, Civello P, Gómez Lobato M, Martínez G. Harvesting at different time-points of day affects glucosinolate metabolism during postharvest storage of broccoli. Food Res Int. (2020) 136:109529. doi: 10.1016/j.foodres.2020.109529
35. Hanschen FS, Schreiner M. Isothiocyanates, nitriles, and epithionitriles from glucosinolates are affected by genotype and developmental stage in Brassica oleracea varieties. Front Plant Sci. (2017) 8:1095. doi: 10.3389/fpls.2017.01095
36. Bhandari SR, Jo JS, Lee JG. Comparison of glucosinolate profiles in different tissues of nine brassica crops. Molecules. (2015) 20:15827–41. doi: 10.3390/molecules200915827
37. Flakelar CL, Prenzler PD, Luckett DJ, Howitt JA, Doran G. A rapid method for the simultaneous quantification of the major tocopherols, carotenoids, free and esterified sterols in canola (Brassica napus) oil using normal phase liquid chromatography. Food Chem. (2017) 214:147–55. doi: 10.1016/j.foodchem.2016.07.059
38. Bahbah EI, Ghozy S, Attia MS, Negida A, Emran T, Mitra S, et al. Molecular mechanisms of astaxanthin as a potential neurotherapeutic agent. Mar Drugs. (2021) 19:40201. doi: 10.3390/md19040201
39. Lee HW, Zhang H, Liang X, Ong CN. Simultaneous determination of carotenoids, tocopherols and phylloquinone in 12 Brassicaceae vegetables. LWT. (2020) 130:109649. doi: 10.1016/j.lwt.2020.109649
40. Björkman M, Klingen I, Birch ANE, Bones AM, Bruce TJA, Johansen TJ, et al. Phytochemicals of Brassicaceae in plant protection and human health - influences of climate, environment and agronomic practice. Phytochemistry. (2011) 72:538–56. doi: 10.1016/j.phytochem.2011.01.014
41. Mitra S, Rauf A, Tareq AM, Jahan S, Emran T, Shahriar TG, et al. Potential health benefits of carotenoid lutein: an updated review. Food Chem Toxicol. (2021) 154:112328. doi: 10.1016/j.fct.2021.112328
42. Nilsson J, Olsson K, Engqvist G, Ekvall J, Olsson M, Nyman M, et al. Variation in the content of glucosinolates, hydroxycinnamic acids, carotenoids, total antioxidant capacity and low-molecular-weight carbohydrates in Brassica vegetables. J Sci Food Agric. (2006) 86:528–38. doi: 10.1002/jsfa.2355
43. Walsh RP, Bartlett H, Eperjesi F. Variation in carotenoid content of kale and other vegetables: a review of pre- and post-harvest effects. J Agric Food Chem. (2015) 63:9677–82. doi: 10.1021/acs.jafc.5b03691
44. Mageney V, Baldermann S, Albach DC. Intraspecific variation in carotenoids of Brassica oleracea var. sabellica. J Agric Food Chem. (2016) 64:3251–7. doi: 10.1021/acs.jafc.6b00268
45. Frede K, Schreiner M, Baldermann S. Light quality-induced changes of carotenoid composition in pak choi Brassica rapa ssp. Chinensis. J Photochem Photobiol B Biol. (2019) 193:18–30. doi: 10.1016/j.jphotobiol.2019.02.001
46. Mène-Saffrané L. Vitamin E biosynthesis and its regulation in plants. Antioxidants. (2018) 7:10002. doi: 10.3390/antiox7010002
47. Lu D, Yang Y, Li Y, Sun C. Analysis of tocopherols and tocotrienols in pharmaceuticals and foods: a critical review. Curr Pharm Anal. (2014) 11:66–78. doi: 10.2174/1573412910666140630170055
48. Li Z, Lee HW, Liang X, Liang D, Wang Q, Huang D, et al. Profiling of phenolic compounds and antioxidant activity of 12 cruciferous vegetables. Molecules. (2018) 23:51139. doi: 10.3390/molecules23051139
49. Cheynier V. Phenolic compounds: from plants to foods. Phytochem Rev. (2012) 11:153–77. doi: 10.1007/s11101-012-9242-8
50. Baenas N, Gómez-Jodar I, Moreno DA, García-Viguera C, Periago PM. Broccoli and radish sprouts are safe and rich in bioactive phytochemicals. Postharvest Biol Technol. (2017) 127:60–7. doi: 10.1016/j.postharvbio.2017.01.010
51. Vergun OM, Rakhmetov DB, Shymanska OV, Fishchenko V, Ivanisova E, Brindza J. Leaves extracts of selected crops. Plant Introd. (2019) 4:82–8. doi: 10.5281/zenodo.3566626
52. Islam MR, Islam F, Nafady MH, Akter M, Mitra S, Das R, et al. Natural small molecules in breast cancer treatment: understandings from a therapeutic viewpoint. Molecules. (2022) 27:72165. doi: 10.3390/molecules27072165
53. Avato P, Argentieri MP. Brassicaceae: a rich source of health improving phytochemicals. Phytochem Rev. (2015) 14:1019–33. doi: 10.1007/s11101-015-9414-4
54. Raiola A, Errico A, Petruk G, Monti DM, Barone A, Rigano MM. Bioactive compounds in brassicaceae vegetables with a role in the prevention of chronic diseases. Molecules. (2018) 23:10015. doi: 10.3390/molecules23010015
55. Upadhyay R, Sehwag S, Singh SP. Antioxidant activity and polyphenol content of Brassica oleracea varieties. Int J Veg Sci. (2016) 22:353–63. doi: 10.1080/19315260.2015.1048403
56. Mitra S, Islam F, Das R, Urmee H, Akter A, Idris AM, et al. Pharmacological potential of Avicennia alba leaf extract: an experimental analysis focusing on antidiabetic, anti-inflammatory, analgesic, and antidiarrheal activity. Biomed Res Int. (2022) 2022:1–10. doi: 10.1155/2022/7624189
57. Islam F, Mitra S, Nafady MH, Rahman MT, Tirth V, Akter A, et al. Neuropharmacological and antidiabetic potential of Lannea coromandelica (Houtt) merr leaves extract: an experimental analysis evidence-based complement. Altern Med. (2022) 2022:6144733. doi: 10.1155/2022/6144733
58. Rodríguez-García C, Sánchez-Quesada C, Toledo E, Delgado-Rodríguez M, Gaforio JJ. Naturally lignan-rich foods: a dietary tool for health promotion? Molecules. (2019) 24:50917. doi: 10.3390/molecules24050917
59. Moreno DA, Pérez-Balibrea S, Ferreres F, Gil-Izquierdo Á, García-Viguera C. Acylated anthocyanins in broccoli sprouts. Food Chem. (2010) 123:358–63. doi: 10.1016/j.foodchem.2010.04.044
60. Matera R, Gabbanini S, De Nicola GR, Iori R, Petrillo G, Valgimigli L. Identification and analysis of isothiocyanates and new acylated anthocyanins in the juice of Raphanus sativus cv. Sango sprouts. Food Chem. (2012) 133:563–72. doi: 10.1016/j.foodchem.2012.01.050
61. Gül MK, Seker M. Comparative analysis of phytosterol components from rapeseed (Brassica napus L) and olive (Olea europaea L) varieties. Eur J Lipid Sci Technol. (2006) 108:759–65. doi: 10.1002/ejlt.200600085
62. Sharma A, Rai PK, Prasad S. GC–MS detection and determination of major volatile compounds in Brassica juncea L. leaves and seeds. Microchem J. (2018) 138:488–93. doi: 10.1016/j.microc.2018.01.015
63. Ahuja I, Kissen R, Bones AM. Phytoalexins in defense against pathogens. Trends Plant Sci. (2012) 17:73–90. doi: 10.1016/j.tplants.2011.11.002
64. Klein AP, Sattely ES. Biosynthesis of cabbage phytoalexins from indole glucosinolate. Proc Natl Acad Sci USA. (2017) 114:1910–5. doi: 10.1073/pnas.1615625114
65. Pedras MSC, Okanga FI, Zaharia IL, Khan AQ. Phytoalexins from crucifers: synthesis, biosynthesis, and biotransformation. Phytochemistry. (2000) 53:161–76. doi: 10.1016/S0031-9422(99)00494-X
66. Cartea E, De Haro-Bailón A, Padilla G, Obregón-Cano S, Del Rio-Celestino M, Ordás A. Seed oil quality of Brassica napus and Brassica rapa germplasm from Northwestern Spain. Foods. (2019) 8:80292. doi: 10.3390/foods8080292
67. Pflaum J, Schlosser S, Müller M. P53 family and cellular stress responses in cancer. Front Oncol. (2014) 4:285. doi: 10.3389/fonc.2014.00285
68. Li XL, Zhou J, Chen ZR, Chng WJ. P53 mutations in colorectal cancer- molecular pathogenesis and pharmacological reactivation. World J Gastroenterol. (2015) 21:84–93. doi: 10.3748/wjg.v21.i1.84
69. Soussi T. The p53 tumor suppressor gene: From molecular biology to clinical investigation. Ann N Y Acad Sci. (2000) 910:121–39. doi: 10.1111/j.1749-6632.2000.tb06705.x
70. Ryan KM, Phillips AC, Vousden KH. Regulation and function of the p53 tumor suppressor protein. Curr Opin Cell Biol. (2001) 13:332–7. doi: 10.1016/S0955-0674(00)00216-7
71. Elkholi R, Floros K V, Chipuk JE. The role of BH3-only proteins in tumor cell development, signaling, and treatment. Genes Cancer. (2011) 2:523–37. doi: 10.1177/1947601911417177
72. Chipuk JE, Moldoveanu T, Llambi F, Parsons MJ, Green DR. The BCL-2 family reunion. Mol Cell. (2010) 37:299–310. doi: 10.1016/j.molcel.2010.01.025
73. Shakeri R, Kheirollahi A, Davoodi J. Apaf-1: regulation and function in cell death. Biochimie. (2017) 135:111–25. doi: 10.1016/j.biochi.2017.02.001
74. Guicciardi ME, Gores GJ. Life and death by death receptors. FASEB J. (2009) 23:1625–37. doi: 10.1096/fj.08-111005
75. Lavrik IN. Systems biology of death receptor networks: live and let die. Cell Death Dis. (2014) 5:160. doi: 10.1038/cddis.2014.160
76. Kumari R, Jat P. Mechanisms of cellular senescence: cell cycle arrest and senescence associated secretory phenotype. Front Cell Dev Biol. (2021) 9:645593. doi: 10.3389/fcell.2021.645593
77. Campisi J, D'Adda Di Fagagna F. Cellular senescence: when bad things happen to good cells. Nat Rev Mol Cell Biol. (2007) 8:729–40. doi: 10.1038/nrm2233
78. Mallette FA, Ferbeyre G. The DNA damage signaling pathway connects oncogenic stress to cellular senescence. Cell Cycle. (2007) 6:1831–6. doi: 10.4161/cc.6.15.4516
79. Beyfuss K, Hood DA. A systematic review of p53 regulation of oxidative stress in skeletal muscle. Redox Rep. (2018) 23:100–17. doi: 10.1080/13510002.2017.1416773
80. Hientz K, Mohr A, Bhakta-Guha D, Efferth T. The role of p53 in cancer drug resistance and targeted chemotherapy. Oncotarget. (2017) 8:8921–46. doi: 10.18632/oncotarget.13475
81. Teodoro JG, Evans SK, Green MR. Inhibition of tumor angiogenesis by p53: a new role for the guardian of the genome. J Mol Med. (2007) 85:1175–86. doi: 10.1007/s00109-007-0221-2
82. Yeo CQX, Alexander I, Lin Z, Lim S, Aning OA, Kumar R, et al. P53 maintains genomic stability by preventing interference between transcription and replication. Cell Rep. (2016) 15:132–46. doi: 10.1016/j.celrep.2016.03.011
83. Rauf A, Abu-Izneid T, Khalil AA, Imran M, Shah ZA, Bin Emran T, et al. Berberine as a potential anticancer agent: a comprehensive review. Molecules. (2021) 26:237368. doi: 10.3390/molecules26237368
84. Mitra S, Sarker J, Mojumder A, Shibbir TB, Das R, Emran T, et al. Genome editing and cancer: how far has research moved forward on CRISPR/Cas9? Biomed Pharmacother. (2022) 150:113011. doi: 10.1016/j.biopha.2022.113011
85. Kandoth C, McLellan MD, Vandin F, Ye K, Niu B, Lu C, et al. Mutational landscape and significance across 12 major cancer types. Nature. (2013) 502:333–9. doi: 10.1038/nature12634
86. Wood LD, Parsons DW, Jones S, Lin J, Sjöblom T, Leary RJ, et al. The genomic landscapes of human breast and colorectal cancers. Science. (2007) 318:1108–13. doi: 10.1126/science.1145720
87. Russo A, Bazan V, Iacopetta B, Kerr D, Soussi T, Gebbia N. The TP53 colorectal cancer international collaborative study on the prognostic and predictive significance of p53 mutation: influence of tumor site, type of mutation, and adjuvant treatment. J Clin Oncol. (2005) 23:7518–28. doi: 10.1200/JCO.2005.00.471
88. Saha MN, Qiu L, Chang H. Targeting p53 by small molecules in hematological malignancies. J Hematol Oncol. (2013) 6:23. doi: 10.1186/1756-8722-6-23
89. López I, Oliveira LL, Tucci P, Álvarez-Valín FA, Coudry R, Marín M. Different mutation profiles associated to P53 accumulation in colorectal cancer. Gene. (2012) 499:81–7. doi: 10.1016/j.gene.2012.02.011
90. Fenoglio-Preiser CM, Wang J, Stemmermann GN, Noffsinger A. TP53 and gastric carcinoma: a review. Hum Mutat. (2003) 21:258–70. doi: 10.1002/humu.10180
91. Cao X, Hou J, An Q, Assaraf YG, Wang X. Towards the overcoming of anticancer drug resistance mediated by p53 mutations. Drug Resist Updat. (2020) 49:100671. doi: 10.1016/j.drup.2019.100671
92. Rahman MM, Islam F, Afsana Mim S, Khan MS, Islam MR, Haque MA, et al. Multifunctional therapeutic approach of nanomedicines against inflammation in cancer and aging. J Nanomater. (2022) 2022:4217529. doi: 10.1155/2022/4217529
93. Huang Y, Liu N, Liu J, Liu Y, Zhang C, Long S, et al. Mutant p53 drives cancer chemotherapy resistance due to loss of function on activating transcription of PUMA. Cell Cycle. (2019) 18:3442–55. doi: 10.1080/15384101.2019.1688951
94. Kim NH, Kim HS, Kim NG, Lee I, Choi HS, Li XY, et al. p53 and microRNA-34 are suppressors of canonical Wnt signaling. Sci Signal. (2011) 4:2001744. doi: 10.1126/scisignal.2001744
95. Kim NH, Cha YH, Kang SE, Lee Y, Lee I, Cha SY, et al. p53 regulates nuclear GSK-3 levels through miR-34-mediated Axin2 suppression in colorectal cancer cells. Cell Cycle. (2013) 12:1578–87. doi: 10.4161/cc.24739
96. Ohtsuka J, Oshima H, Ezawa I, Abe R, Oshima M, Ohki R. Functional loss of p53 cooperates with the in vivo microenvironment to promote malignant progression of gastric cancers. Sci Rep. (2018) 8:1. doi: 10.1038/s41598-018-20572-1
97. Shen HG, Maki C. Pharmacologic activation of p53 by small-molecule MDM2 antagonists. Curr Pharm Des. (2011) 17:560–8. doi: 10.2174/138161211795222603
98. Micel LN, Tentler JJ, Smith PG, Eckhardt SG. Role of ubiquitin ligases and the proteasome in oncogenesis: novel targets for anticancer therapies. J Clin Oncol. (2013) 31:1231–8. doi: 10.1200/JCO.2012.44.0958
99. Sun SH, Zheng M, Ding K, Wang S, Sun Y. A small molecule that disrupts Mdm2-p53 binding activates p53, induces apoptosis and sensitizes lung cancer cells to chemotherapy. Cancer Biol Ther. (2008) 7:845–52. doi: 10.4161/cbt.7.6.5841
100. Shangary S, Ding K, Qiu S, Nikolovska-Coleska Z, Bauer JA, Liu M, et al. Reactivation of p53 by a specific MDM2 antagonist (MI-43) leads to p21-mediated cell cycle arrest and selective cell death in colon cancer. Mol Cancer Ther. (2008) 7:1533–42. doi: 10.1158/1535-7163.MCT-08-0140
101. Patton JT, Mayo LD, Singhi AD, Gudkov A V, Stark GR, Jackson MW. Levels of HdmX expression dictate the sensitivity of normal and transformed cells to Nutlin-3. Cancer Res. (2006) 66:3169–76. doi: 10.1158/0008-5472.CAN-05-3832
102. Issaeva N, Bozko P, Enge M, Protopopova M, Verhoef LGGC, Masucci M, et al. Small molecule RITA binds to p53, blocks p53-HDM-2 interaction and activates p53 function in tumors. Nat Med. (2004) 10:1321–8. doi: 10.1038/nm1146
103. Bykov VJN, Issaeva N, Shilov A, Hultcrantz M, Pugacheva E, Chumakov P, et al. Restoration of the tumor suppressor function to mutant p53 by a low-molecular-weight compound. Nat Med. (2002) 8:282–8. doi: 10.1038/nm0302-282
104. Lambert JMR, Gorzov P, Veprintsev DB, Söderqvist M, Segerbäck D, Bergman J, et al. PRIMA-1 reactivates mutant p53 by covalent binding to the core domain. Cancer Cell. (2009) 15:376–88. doi: 10.1016/j.ccr.2009.03.003
105. Jiang D, Wang L, Zhao T, Zhang Z, Zhang R, Jin J, et al. Restoration of the tumor-suppressor function to mutant p53 by Ganoderma lucidum polysaccharides in colorectal cancer cells. Oncol Rep. (2017) 37:594–600. doi: 10.3892/or.2016.5246
106. Hussain SP, Harris CC. Molecular epidemiology of human cancer: contribution of mutation spectra studies of tumor suppressor genes. Cancer Res. (1998) 58:4023–37.
107. Lorne J, HofsethNewton AC, Perwez Hussain P, Harris CC. P53: 25 years after its discovery. Trends Pharmacol Sci. (2004) 25:177–81. doi: 10.1016/j.tips.2004.02.009
108. Rao VA, Terzian T, Valentin-Vega YA, El-Naggar AK, Iwakuma T, Suh YA, et al. Gain of function of a p53 hot spot mutation in a mouse model of Li-Fraumeni syndrome. Cell. (2004) 119:861–72. doi: 10.1016/j.cell.2004.11.006
109. Hastak K, Agarwal MK, Mukhtar H, Agarwal ML. Ablation of either p21 or Bax prevents p53-dependent apoptosis induced by green tea polyphenol epigallocatechin-3-gallate. FASEB J. (2005) 19:1–19. doi: 10.1096/fj.04-2226fje
110. Kumar Y, Phaniendra A, Periyasamy L. Bixin triggers apoptosis of human Hep3B hepatocellular carcinoma cells: an insight to molecular and in silico approach. Nutr Cancer. (2018) 70:971–83. doi: 10.1080/01635581.2018.1490445
111. Qiu Y, Li C, Zhang B, Gu Y. Bixin prevents colorectal cancer development through AMPK-activated endoplasmic reticulum stress. Biomed Res Int. (2022) 2022:9329151. doi: 10.1155/2022/9329151
112. Yuan A. Allicin induces apoptosis in gastric cancer cells through activation of both extrinsic and intrinsic pathways. Oncol Rep. (2010) 24:1021. doi: 10.3892/or_00001021
113. Persson C, Sasazuki S, Inoue M, Kurahashi N, Iwasaki M, Miura T, et al. Plasma levels of carotenoids, retinol and tocopherol and the risk of gastric cancer in Japan: a nested case-control study. Carcinogenesis. (2008) 29:1042–8. doi: 10.1093/carcin/bgn072
114. Yang G, Gao YT, Shu XO, Cai Q, Li GL, Li HL, et al. Isothiocyanate exposure, glutathione S-transferase polymorphisms, and colorectal cancer risk. Am J Clin Nutr. (2010) 91:704–11. doi: 10.3945/ajcn.2009.28683
115. Tang FY, Shih CJ, Cheng LH, Ho HJ, Chen HJ. Lycopene inhibits growth of human colon cancer cells via suppression of the Akt signaling pathway. Mol Nutr Food Res. (2008) 52:646–54. doi: 10.1002/mnfr.200700272
116. Yang Q, Miyagawa M, Liu X, Zhu B, Munemasa S, Nakamura T, et al. Methyl-β-cyclodextrin potentiates the BITC-induced anti-cancer effect through modulation of the Akt phosphorylation in human colorectal cancer cells. Biosci Biotechnol Biochem. (2018) 82:2158–67. doi: 10.1080/09168451.2018.1514249
117. Kim JH, Park JJ, Lee BJ, Joo MK, Chun HJ, Lee SW, et al. Astaxanthin inhibits proliferation of human gastric cancer cell lines by interrupting cell cycle progression. Gut Liver. (2016) 10:369–74. doi: 10.5009/gnl15208
118. Vassilev LT. MDM2 inhibitors for cancer therapy. Trends Mol Med. (2007) 13:23–31. doi: 10.1016/j.molmed.2006.11.002
119. Gullett NP, Ruhul Amin ARM, Bayraktar S, Pezzuto JM, Shin DM, Khuri FR, et al. Cancer prevention with natural compounds. Semin Oncol. (2010) 37:258–81. doi: 10.1053/j.seminoncol.2010.06.014
120. Ho CC, Lai KC, Hsu SC, Kuo CL, Ma CY, Lin ML, et al. Benzyl isothiocyanate (BITC) inhibits migration and invasion of human gastric cancer AGS cells via suppressing ERK signal pathways. Hum Exp Toxicol. (2011) 30:296–306. doi: 10.1177/0960327110371991
121. Ekström AM, Serafini M, Nyrén O, Wolk A, Bosetti C, Bellocco R. Dietary quercetin intake and risk of gastric cancer: results from a population-based study in Sweden. Ann Oncol. (2011) 22:438–43. doi: 10.1093/annonc/mdq390
122. Poke F, Qadi A, Holloway A. Reversing aberrant methylation patterns in cancer. Curr Med Chem. (2010) 17:1246–54. doi: 10.2174/092986710790936329
123. Rajendran P, Kidane AI, Yu TW, Dashwood WM, Bisson WH, Löhr CV, et al. Turnover, CtIP acetylation and dysregulated DNA damage signaling in colon cancer cells treated with sulforaphane and related dietary isothiocyanates. Epigenetics. (2013) 8:612–23. doi: 10.4161/epi.24710
124. Murase W, Kamakura Y, Kawakami S, Yasuda A, Wagatsuma M, Kubota A, et al. Fucoxanthin prevents pancreatic tumorigenesis in c57bl/6j mice that received allogenic and orthotopic transplants of cancer cells. Int J Mol Sci. (2021) 22:413620. doi: 10.3390/ijms222413620
125. Chung MY, Lim TG, Lee KW. Molecular mechanisms of chemopreventive phytochemicals against gastroenterological cancer development. World J Gastroenterol. (2013) 19:984–93. doi: 10.3748/wjg.v19.i7.984
126. Aggarwal BB, Shishodia S. Molecular targets of dietary agents for prevention and therapy of cancer. Biochem Pharmacol. (2006) 71:1397–421. doi: 10.1016/j.bcp.2006.02.009
127. Soundararajan P, Kim JS. Anti-carcinogenic glucosinolates in cruciferous vegetables and their antagonistic effects on prevention of cancers. Molecules. (2018) 23:112983. doi: 10.3390/molecules23112983
128. Yamada H, Shinmura K, Okudela K, Goto M, Suzuki M, Kuriki K, et al. Identification and characterization of a novel germ line p53 mutation in familial gastric cancer in the Japanese population. Carcinogenesis. (2007) 28:2013–8. doi: 10.1093/carcin/bgm175
129. Ranjan A, Ramachandran S, Gupta N, Kaushik I, Wright S, Srivastava S, et al. Role of phytochemicals in cancer prevention. Int J Mol Sci. (2019) 20:204981. doi: 10.3390/ijms20204981
130. Hintze KJ, Keck AS, Finley JW, Jeffery EH. Induction of hepatic thioredoxin reductase activity by sulforaphane, both in Hepa1c1c7 cells and in male Fisher 344 rats. J Nutr Biochem. (2003) 14:173–9. doi: 10.1016/S0955-2863(02)00282-6
131. Ye Y, Fang Y, Xu W, Wang Q, Zhou J, Lu R. 3,3′-Diindolylmethane induces anti-human gastric cancer cells by the miR-30e-ATG5 modulating autophagy. Biochem Pharmacol. (2016) 115:77–84. doi: 10.1016/j.bcp.2016.06.018
132. Li XJ, Park ES, Park MH, Kim SM. 3,3'-Diindolylmethane suppresses the growth of gastric cancer cells via activation of the Hippo signaling pathway. Oncol Rep. (2013) 30:2419–26. doi: 10.3892/or.2013.2717
133. Shin J, Song MH, Oh JW, Keum YS, Saini RK. Pro-oxidant actions of carotenoids in triggering apoptosis of cancer cells: a review of emerging evidence. Antioxidants. (2020) 9:1–17. doi: 10.3390/antiox9060532
134. Liu C, Russell RM. Nutrition and gastric cancer risk: an update. Nutr Rev. (2008) 66:237–49. doi: 10.1111/j.1753-4887.2008.00029.x
135. Liu C, Russell RM, Wang XD. Lycopene supplementation prevents smoke-induced changes in p53, p53 phosphorylation, cell proliferation, and apoptosis in the gastric mucosa of ferrets. J Nutr. (2006) 136:106–11. doi: 10.1093/jn/136.1.106
136. El-Deiry WS. Regulation of p53 downstream genes. Semin Cancer Biol. (1998) 8:345–57. doi: 10.1006/scbi.1998.0097
137. Cai XZ, Wang J, Li XD, Wang GL, Liu FN, Cheng MS, et al. Curcumin suppresses proliferation and invasion in human gastric cancer cells by downregulation of PAK1 activity and cyclin D1 expression. Cancer Biol Ther. (2009) 8:1360–8. doi: 10.4161/cbt.8.14.8720
138. Moy KA, Yuan JM, Chung FL, Wang XL, Van Den Berg D, Wang R, et al. Isothiocyanates, glutathione S-transferase M1 and T1 polymorphisms and gastric cancer risk: a prospective study of men in Shanghai, China. Int J Cancer. (2009) 125:2652–9. doi: 10.1002/ijc.24583
139. Ge M, Zhang L, Cao L, Xie C, Li X, Li Y, et al. Sulforaphane inhibits gastric cancer stem cells via suppressing sonic hedgehog pathway. Int J Food Sci Nutr. (2019) 70:570–8. doi: 10.1080/09637486.2018.1545012
140. Peng W, Zhou Z, Zhong Y, Sun Y, Wang Y, Zhu Z, et al. Plasma activity of thioredoxin reductase as a novel biomarker in gastric cancer. Sci Rep. (2019) 9:6. doi: 10.1038/s41598-019-55641-6
141. McKenzie RC, Arthur JR, Beckett GJ. Selenium and the regulation of cell signaling, growth, and survival: molecular and mechanistic aspects. Antioxidants Redox Signal. (2002) 4:339–51. doi: 10.1089/152308602753666398
142. Wang Y, Wu H, Dong N, Su X, Duan M, Wei Y, et al. Sulforaphane induces S-phase arrest and apoptosis via p53-dependent manner in gastric cancer cells. Sci Rep. (2021) 11:2. doi: 10.1038/s41598-021-81815-2
143. Shen G, Xu C, Hu R, Jain MR, Gopalkrishnan A, Nair S, et al. Modulation of nuclear factor E2-related factor 2-mediated gene expression in mice liver and small intestine by cancer chemopreventive agent curcumin. Mol Cancer Ther. (2006) 5:39–51. doi: 10.1158/1535-7163.MCT-05-0293
144. Murakami A, Ashida H, Terao J. Multitargeted cancer prevention by quercetin. Cancer Lett. (2008) 269:315–25. doi: 10.1016/j.canlet.2008.03.046
145. Sugie S, Okamoto K, Okumura A, Tanaka T, Mori H. Inhibitory effects of benzyl thiocyanate and benzyl isothiocyanate on methylazoxymethanol acetate-induced intestinal carcinogenesis in rats. Carcinogenesis. (1994) 15:1555–60. doi: 10.1093/carcin/15.8.1555
146. Hu R, Khor TO, Shen G, Jeong WS, Hebbar V, Chen C, et al. Cancer chemoprevention of intestinal polyposis in ApcMin/+ mice by sulforaphane, a natural product derived from cruciferous vegetable. Carcinogenesis. (2006) 27:2038–46. doi: 10.1093/carcin/bgl049
147. Hu R, Kim BR, Chen C, Hebbar V, Kong ANT. The roles of JNK and apoptotic signaling pathways in PEITC-mediated responses in human HT-29 colon adenocarcinoma cells. Carcinogenesis. (2003) 24:1361–7. doi: 10.1093/carcin/bgg092
148. Gamet-Payrastre L, Lumeau S, Gasc N, Cassar G, Rollin P, Tulliez J. Selective cytostatic and cytotoxic effects of glucosinolates hydrolysis products on human colon cancer cells in vitro. Anticancer Drugs. (1998) 9:141–8. doi: 10.1097/00001813-199802000-00005
149. Al-Ishaq RK, Overy AJ, Büsselberg D. Phytochemicals and gastrointestinal cancer: cellular mechanisms and effects to change cancer progression. Biomolecules. (2020) 10:10105. doi: 10.3390/biom10010105
150. Palozza P, Torelli C, Boninsegna A, Simone R, Catalano A, Mele MC, et al. Growth-inhibitory effects of the astaxanthin-rich alga Haematococcus pluvialis in human colon cancer cells. Cancer Lett. (2009) 283:108–17. doi: 10.1016/j.canlet.2009.03.031
151. Millán CS, Soldevilla B, Martín P, Gil-Calderón B, Compte M, Pérez-Sacristán B, et al. β-Cryptoxanthin synergistically enhances the antitumoral activity of oxaliplatin through ΔNP73 negative regulation in colon cancer. Clin Cancer Res. (2015) 21:4398–409. doi: 10.1158/1078-0432.CCR-14-2027
152. Liu ZJ, Xu W, Han J, Liu QY, Gao LF, Wang XH, et al. Quercetin induces apoptosis and enhances gemcitabine therapeutic efficacy against gemcitabine-resistant cancer cells. [[i]]Anticancer Drugs[[/i]] (2020)
153. Shehzad A, Lee J, Lee YS. Curcumin in various cancers. BioFactors. (2013) 39:56–68. doi: 10.1002/biof.1068
154. Mózsik G, Rumi G, Dömötör A, Figler M, Gasztonyi B, Papp E, et al. Involvement of serum retinoids and Leiden mutation in patients with esophageal, gastric, liver, pancreatic, and colorectal cancers in Hungary. World J Gastroenterol. (2005) 11:7646–50. doi: 10.3748/wjg.v11.i48.7646
155. Seren S, Lieberman R, Bayraktar UD, Heath E, Sahin K, Andic F, et al. Lycopene in cancer prevention and treatment. Am J Ther. (2008) 15:66–81. doi: 10.1097/MJT.0b013e31804c7120
156. Faraone I, Sinisgalli C, Ostuni A, Armentano MF, Carmosino M, Milella L, et al. Astaxanthin anticancer effects are mediated through multiple molecular mechanisms: a systematic review. Pharmacol Res. (2020) 155:104689. doi: 10.1016/j.phrs.2020.104689
157. Pham NA, Jacobberger JW, Schimmer AD, Cao P, Gronda M, Hedley DW. The dietary isothiocyanate sulforaphane targets pathways of apoptosis, cell cycle arrest, and oxidative stress in human pancreatic cancer cells and inhibits tumor growth in severe combined immunodeficient mice. Mol Cancer Ther. (2004) 3:1239–48. doi: 10.1158/1535-7163.1239.3.10
158. Boreddy SR, Pramanik KC, Srivastava SK. Pancreatic tumor suppression by benzyl isothiocyanate is associated with inhibition of PI3K/AKT/FOXO pathway. Clin Cancer Res. (2011) 17:1784–95. doi: 10.1158/1078-0432.CCR-10-1891
159. Xie S, Wang Q, Wu H, Cogswell J, Lu L, Jhanwar-Uniyal M, et al. Reactive oxygen species-induced phosphorylation of p53 on serine 20 is mediated in part by polo-like kinase-3. J Biol Chem. (2001) 276:36194–9. doi: 10.1074/jbc.M104157200
160. Kale J, Osterlund EJ, Andrews DW. BCL-2 family proteins: changing partners in the dance towards death. Cell Death Differ. (2018) 25:65–80. doi: 10.1038/cdd.2017.186
161. Jang SH, Lim JW, Kim H. Mechanism of β-carotene-induced apoptosis of gastric cancer cells: involvement of ataxia-telangiectasia-mutated. Ann N Y Acad Sci. (2009) 1171:156–62. doi: 10.1111/j.1749-6632.2009.04711.x
162. Tomasetti C, Marchionni L, Nowak MA, Parmigiani G, Vogelstein B. Only three driver gene mutations are required for the development of lung and colorectal cancers. Proc Natl Acad Sci USA. (2015) 112:118–23. doi: 10.1073/pnas.1421839112
163. Rauf A, Ali Shariati M, Imran M, Bashir K, Ali Khan S, Mitra S. Comprehensive review on naringenin and naringin polyphenols as a potent anticancer agent. Environ Sci Pollut Res Int. (2022) 29:31025–41. doi: 10.1007/s11356-022-18754-6
164. LoPiccolo J, Blumenthal GM, Bernstein WB, Dennis PA. Targeting the PI3K/Akt/mTOR pathway: effective combinations and clinical considerations. Drug Resist Updat. (2008) 11:32–50. doi: 10.1016/j.drup.2007.11.003
165. Tse G, Eslick GD. Cruciferous vegetables and risk of colorectal neoplasms: a systematic review and meta-analysis. Nutr Cancer. (2014) 66:128–39. doi: 10.1080/01635581.2014.852686
166. Zhu M, Li W, Dong X, Chen Y, Lu Y, Lin B, et al. Benzyl-isothiocyanate induces apoptosis and inhibits migration and invasion of hepatocellular carcinoma cells in vitro. J Cancer. (2017) 8:240–8. doi: 10.7150/jca.16402
167. Lai KC, Huang ANC, Hsu SC, Kuo CL, Yang JS, Wu SH, et al. Benzyl Lsothiocyanate (BITC) inhibits migration and invasion of human colon cancer HT29 cells by inhibiting matrix metalloproteinase-2/-9 and urokinase plasminogen (uPA) through PKC and MAPK signaling pathway. J Agric Food Chem. (2010) 58:2935–42. doi: 10.1021/jf9036694
168. Lai KC, Hsu SC, Kuo CL, Ip SW, Yang JS, Hsu YM, et al. Phenethyl isothiocyanate inhibited tumor migration and invasion via suppressing multiple signal transduction pathways in human colon cancer HT29 cells. J Agric Food Chem. (2010) 58:11148–55. doi: 10.1021/jf102384n
169. Liu Y, Dey M. Dietary phenethyl isothiocyanate protects mice from colitis associated colon cancer. Int J Mol Sci. (2017) 18:91908. doi: 10.3390/ijms18091908
170. Parnaud G, Li PF, Cassar G, Rouimi P, Tulliez J, Combaret L, et al. Mechanism of sulforaphane-induced cell cycle arrest and apoptosis in human colon cancer cells. Nutr Cancer. (2004) 48:198–206. doi: 10.1207/s15327914nc4802_10
171. Jakubíková J, Sedlák J, Mithen R, Bao Y. Role of PI3K/Akt and MEK/ERK signaling pathways in sulforaphane- and erucin-induced phase II enzymes and MRP2 transcription, G2/M arrest and cell death in Caco-2 cells. Biochem Pharmacol. (2005) 69:1543–52. doi: 10.1016/j.bcp.2005.03.015
172. Martin SL, Kala R, Tollefsbol TO. Mechanisms for the inhibition of colon cancer cells by sulforaphane through epigenetic modulation of MicroRNA-21 and human telomerase reverse transcriptase (hTERT) down-regulation. Curr Cancer Drug Targets. (2017) 18:97–106. doi: 10.2174/1568009617666170206104032
173. Okonkwo A, Mitra J, Johnson GS Li L, Dashwood WM, Hegde ML, Yue C, et al. Heterocyclic analogs of sulforaphane trigger DNA damage and impede DNA repair in colon cancer cells: interplay of HATs and HDACs. Mol Nutr Food Res. (2018) 62:800228. doi: 10.1002/mnfr.201800228
174. Myzak MC, Karplus PA, Chung FL, Dashwood RH. A novel mechanism of chemoprotection by sulforaphane: inhibition of histone deacetylase. Cancer Res. (2004) 64:5767–74. doi: 10.1158/0008-5472.CAN-04-1326
175. Kim SM. Cellular and molecular mechanisms of 3,3'-diindolylmethane in gastrointestinal cancer. Int J Mol Sci. (2016) 17:711550. doi: 10.3390/ijms17071155
176. Pan JH, Abernathy B, Kim YJ, Lee JH, Kim JH, Shin EC, et al. Cruciferous vegetables and colorectal cancer prevention through microRNA regulation: a review. Crit Rev Food Sci Nutr. (2018) 58:2026–38. doi: 10.1080/10408398.2017.1300134
177. Kountouras J, Zavos C, Chatzopoulos D. Apoptotic and anti-angiogenic strategies in liver and gastrointestinal malignancies. J Surg Oncol. (2005) 90:249–59. doi: 10.1002/jso.20254
178. Arathi BP, Sowmya PRR, Kuriakose GC, Vijay K, Baskaran V, Jayabaskaran C, et al. Enhanced cytotoxic and apoptosis inducing activity of lycopene oxidation products in different cancer cell lines. Food Chem Toxicol. (2016) 97:265–76. doi: 10.1016/j.fct.2016.09.016
179. Bolhassani A, Khavari A, Bathaie SZ. Saffron and natural carotenoids: biochemical activities and anti-tumor effects. Biochim Biophys Acta Rev Cancer. (2014) 1845:20–30. doi: 10.1016/j.bbcan.2013.11.001
180. Zhou Y, Li Y, Zhou T, Zheng J, Li S, Li H. Dietary natural products for prevention and treatment of liver cancer. Nutrients. (2016) 8:30156. doi: 10.3390/nu8030156
181. Blanchet A, Bourgmayer A, Kurtz JE, Mellitzer G, Gaiddon C. Isoforms of the p53 family and gastric cancer: a ménage à trois for an unfinished affair. Cancers. (2021) 13:1–45. doi: 10.3390/cancers13040916
182. Kunst C, Haderer M, Heckel S, Schlosser S, Müller M. The p53 family in hepatocellular carcinoma. Transl Cancer Res. (2016) 5:632–8. doi: 10.21037/tcr.2016.11.79
183. Nakayama M, Oshima M. Mutant p53 in colon cancer. J Mol Cell Biol. (2019) 11:267–76. doi: 10.1093/jmcb/mjy075
184. Jie M, Cheung WM, Yu V, Zhou Y, Tong PH, Ho JWS. Anti-proliferative activities of sinigrin on carcinogen-induced hepatotoxicity in rats. PLoS ONE. (2014) 9:110145. doi: 10.1371/journal.pone.0110145
Keywords: cruciferous vegetables, foods bioactive compounds, gastrointestinal cancer, p53 family, apoptosis
Citation: Mitra S, Emran TB, Chandran D, Zidan BMRM, Das R, Mamada SS, Masyita A, Salampe M, Nainu F, Khandaker MU, Idris AM and Simal-Gandara J (2022) Cruciferous vegetables as a treasure of functional foods bioactive compounds: Targeting p53 family in gastrointestinal tract and associated cancers. Front. Nutr. 9:951935. doi: 10.3389/fnut.2022.951935
Received: 24 May 2022; Accepted: 12 July 2022;
Published: 04 August 2022.
Edited by:
Marcello Iriti, University of Milan, ItalyReviewed by:
Junling Shi, Northwestern Polytechnical University, ChinaCopyright © 2022 Mitra, Emran, Chandran, Zidan, Das, Mamada, Masyita, Salampe, Nainu, Khandaker, Idris and Simal-Gandara. This is an open-access article distributed under the terms of the Creative Commons Attribution License (CC BY). The use, distribution or reproduction in other forums is permitted, provided the original author(s) and the copyright owner(s) are credited and that the original publication in this journal is cited, in accordance with accepted academic practice. No use, distribution or reproduction is permitted which does not comply with these terms.
*Correspondence: Talha Bin Emran, dGFsaGFibWJAYmdjdHViLmFjLmJk; Abubakr M. Idris, YWJ1YmFrcmlkcmlzQGhvdG1haWwuY29t; Jesus Simal-Gandara, anNpbWFsQHV2aWdvLmVz
Disclaimer: All claims expressed in this article are solely those of the authors and do not necessarily represent those of their affiliated organizations, or those of the publisher, the editors and the reviewers. Any product that may be evaluated in this article or claim that may be made by its manufacturer is not guaranteed or endorsed by the publisher.
Research integrity at Frontiers
Learn more about the work of our research integrity team to safeguard the quality of each article we publish.