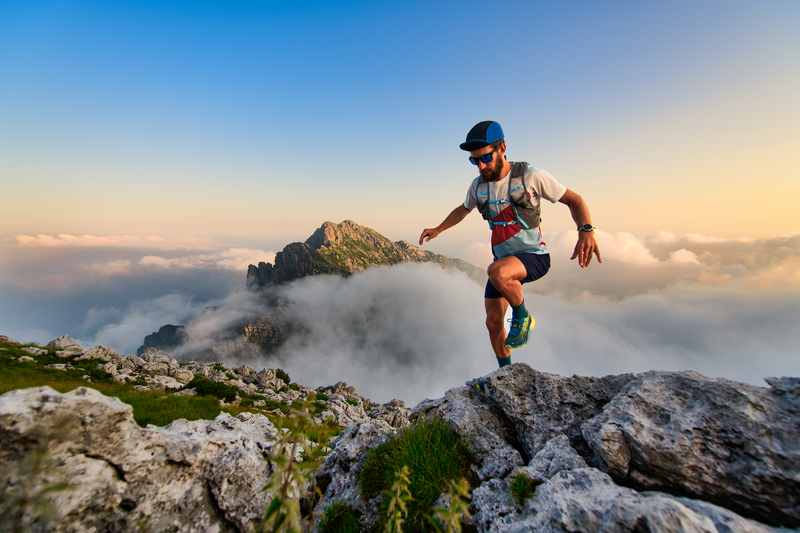
94% of researchers rate our articles as excellent or good
Learn more about the work of our research integrity team to safeguard the quality of each article we publish.
Find out more
ORIGINAL RESEARCH article
Front. Nutr. , 28 November 2022
Sec. Nutrition and Microbes
Volume 9 - 2022 | https://doi.org/10.3389/fnut.2022.950446
This article is part of the Research Topic Food, Nutrition and Microecological Health View all 27 articles
Dietary non-starch polysaccharides and phenolics are usually ingested at the same time. They are both regarded as prebiotics, and they regulate the intestinal microbiota through various mechanisms. Notably, however, reports of their combined or synergistic effects are rare. Arabinoxylan (AX), a polysaccharide, and chlorogenic acid (CA), a polyphenol, are widely consumed, and their effects on the microbiota have previously been discussed. In the present study, they were given to dextran sulfate sodium (DSS)–treated mice, separately and together, and the intestinal microbiota were investigated by high-throughput sequencing. The data showed that CA attenuated body weight loss, colon shortening, and histological damage in DSS-treated mice, while neither AX nor the AX+CA combination exhibited any ameliorating potential. AX+CA had less of a modulating effect on intestinal microbiota profiles than did CA. AX+CA administration increased the relative abundance of Flavonifractor, Coprobacillus, and Clostridium_XlVa, and decreased the abundance of Robinsoniella and Lactobacillus. Compared to AX and CA, AX+CA contributed to a more complicated shift in the biological functions of the intestinal microbiotaAX seemed to weaken the beneficial effects of CA, at least in the present experimental model of DSS-induced colitis. The combined effects and mechanisms of dietary polysaccharides and phenolic compounds on the intestinal microbiota and on overall health still need to be further investigated.
Low intake of health-benefiting foods, such as whole grains, vegetables, and fruits, has been a major dietary risk factor for mortality and disability-adjusted life-years over the past few decades (1). The beneficial effects of whole grains, vegetables, fruits, and other plant-based foods, including their antioxidant and anti-inflammatory properties, as well as the attenuation of metabolic disorders, could come from their functional ingredients, such as non-starch polysaccharides, phenolics, and other bioactive components (2). Arabinoxylan (AX) is the main non-starch polysaccharide in cereal grains, including maize, rye, barley, oats, sorghum, wheat, rice, and other plants. It accounts for between 11 and 26% of wheat bran and between 60% and 70% of the endosperm cell wall and aleurone layer (3). The main chain structure of AX consists of a β-1,4-D-Xylp backbone with L-Araf. AX exhibits many bioactive characteristics, including antioxidant, fermentable, and prebiotic properties, glucose- and lipid-regulating effects, and immune-modulating potentials (4, 5). AX has been described as an efficient immunomodulator for synergistic or complementary cancer treatment (6). Chlorogenic acid (CA), a hydroxycinnamic acid–derived phenolic compound, is widely distributed in many plant foods, including vegetables, fruits, and herbal remedies (7). It is an extensively studied phytochemical that possesses many health-promoting properties, such as antioxidant, anti-inflammatory, antidiabetic, antilipidemic, and antihypertensive activities (8).
Inflammatory bowel disease (IBD) has become a global disease and health burden with increasing incidence. Although its exact aetiopathogenesis remains unclear, it is widely known that the gut microbiota is closely associated with IBD. Some members of the gut microbiome and bacterial metabolites, including Faecalibacterium prausnitzii, Roseburia, short-chain fatty acids, and secondary bile acids, have potentially protective effects against IBD, while others, such as adherent invasive Escherichia coli, Enterococcus faecium, enterotoxigenic Bacteroides fragilis, and Campylobacter concisus, show potentially causative effects (9). Diet also plays an important role in the development and progression of IBD. Epidemiological studies have identified potential dietary risk factors for IBD, such as red meat, processed foods, refined sugar, and saturated fat, and dietary intervention studies have shown promising results, demonstrating that diets rich in fruits, vegetables, whole grains, and seafood are associated with reduced risk (10).
Dietary fermentable polysaccharides and phenolics have modulating effects on the intestinal microbiota, and they are considered prebiotics (11). In in vitro studies of fecal samples, AX exhibited significant effects on the microbiota, including increased abundance of Collinsella, Blautia, and Bifidobacterium, and decreased abundance of Sutterella, Bilophila, and Parabacteroides. It also significantly increased the amount of total and individual short-chain fatty acids (SCFAs) (12). AX has also been shown to contribute to a global shift in the intestinal microbiota profile in overweight or obese adults, promote the growth of operational taxonomic units related to Bifidobacterium longum, Blautia obeum, and Prevotella copri, and increase the concentration of fecal propionate (13). Supplementation of AX promoted the proliferation of fiber-degrading bacteria and the generation of short-chain fatty acids (SCFAs), and decreased the abundance of opportunistic pathogens, in type 2 diabetic mice (14). Phenolic CA has been shown to increase the relative abundance of SCFA-producing bacteria, including Bacteroides, Prevotellaceae UGC-001, and Butyricimonas, in hyperuricemic mice (15). It also promoted the abundance of Bifidobacterium and decreased the content of Escherichia coli in the feces of mice with non-alcoholic fatty liver disease (16).
Dietary bioactive polysaccharides and phenolics both have prebiotic effects on the intestinal microbiota, and they exert regulating potential via distinct mechanisms. However, there has been little research into their combined effects. The present study investigated the effects of AX, CA, and their combination on the intestinal microbiota of mice treated with dextran sulfate sodium (DSS). Our data have the potential to provide novel insights into the synergistic potential of dietary components.
AX (derived from wheat, low viscosity, Lot 160419b) was purchased from Megazyme Ltd. (Bray, Ireland). CA was obtained from Aladdin Biochemical Technology Co., Ltd. (Shanghai, China). Colitis-grade DSS was obtained from MP Biomedicals (Irvine, CA). AIN-93G purified rodent diet was purchased from Jiangsu Xietong Pharmaceutical Bio-engineering Co., Ltd. (Nanjing, China).
The animal experimental protocol was approved by the Ethical Committee of Nanjing Agricultural University Animal Experiment Center (SYXK- <Jiangsu>-2021-0086) according to the National Guidelines for Experimental Animal Welfare. Forty 6-week-old C57BL/6 mice were kept in a specific pathogen-free facility under standard laboratory conditions. After acclimating for 1 week, they were randomly divided into five groups. The feeding and treatment procedure are illustrated in Figure 1A. The mice in the normal control (Ctrl) and DSS-treated (DSS) groups were daily orally gavaged with water. The mice in the AX and CA groups were daily given AX and CA, respectively, at a dose of 200 mg/kg body weight. The mice in the AX+CA group were administered a combination of AX and CA dosed at 200 mg/kg body weight. The mice in the Ctrl group freely drank water. The other mice had free access to DSS solution (1.5%, w/v) for the first 7 days, then the DSS solution was changed to water for the remaining days. After 14 days of treatment, fresh feces were collected, immediately frozen in liquid nitrogen, and stored at −80°C until further analysis.
Figure 1. (A) Experimental design and treatment structure; (B) Changes in body weight over time for each group. The * symbol indicates the body weight of mice in the group was significantly different from those in the Ctrl group after feeding and treatment for 14 days (p < 0.05).
After sacrifice, the distal colon tissues of the mice were immediately fixed with 4% formalin, and they were washed, dehydrated, embedded into paraffin, sliced into 5 μm sections, and transferred onto glass slides. The slides were stained with hematoxylin-eosin reagent (Sigma-Aldrich, St. Louis, MO), and a histological examination was performed, with a focus on crypt architecture, inflammatory cell infiltration, goblet cell depletion, and muscle tissue thickening (17).
The high-throughput amplicon sequencing and gut microbiota analysis were performed by Genesky Biotechnologies Inc. (Shanghai, China) according to a general pipeline. The total DNA of the fecal samples was extracted with the QIAamp Fast DNA Stool Mini Kit (Qiagen, Germany). The resulting DNA was amplified with barcoded specific bacterial primers targeting the variable region 3 (V3) and V4 of the 16S rRNA gene using Primer F (5'-Illumina adapter sequence 1+ CCTACGGGNGGCWGCAG-3') and Primer R (5'-Illumina adapter sequence 2+ GACTACHVGGGTATCTAATCC-3'). The sequencing was performed on an Illumina NovaSeq 6000 platform via the SP-Xp two-end strategy. An amplicon sequence variants (ASVs) table was generated after the raw sequencing data were filtered, denoised, merged, and depleted of chimeras with the QIIME2 and DAD2 plugins (18, 19). Taxonomic assignments of the ASV sequences were conducted by a naive Bayes classifier with a confidence threshold of 0.8; the classifier had been pretrained on the RDP database (version 11.5). The function of the bacterial community was predicted by Phylogenetic Investigation of Communities by Reconstruction of Unobserved States (PICRUSt2) software (20).
The data were expressed as mean ± standard error of the mean, and statistical significance was tested by one-way analysis of variance (ANOVA). A p < 0.05 was considered statistically significant. The difference of microbial taxon was determined by Metastats software and the linear discriminant analysis effect size (LEfSe) package (21, 22).
Body weight is one of the most important objective indicators of nutrition and health status. Body weight changes in the mice were therefore analyzed to assess whether the treatments had any effects on their body condition. Administration of DSS decreased body weight due to colon inflammation. As shown in Figure 1B, at the experimental endpoint, the body weights of the mice in the DSS, AX, and AX+CA groups were still lower than those of the Ctrl group (p < 0.05). However, the body weights of the CA group mice were attenuated, remaining closest to those of the Ctrl group (p > 0.05), suggesting the ameliorating effects of CA on colitis. Unfortunately, the combination of AX and CA did not show satisfying potential with regard to body weight.
The colon lengths of the mice in each group are presented in Figure 2. DSS administration significantly shortened colon length, as compared to those of mice in the Ctrl group (p < 0.05). CA supplementation reversed these losses, ultimately restoring the colon lengths of CA group mice to lengths similar to those of Ctrl group mice (p > 0.05); however, the AX and AX+CA treatments did not show any attenuating potential. As shown by the histological examination (Figure 3), the crypt damage caused by DSS administration was remedied by CA treatment, while AX and AX+CA supplementation had only slight effects on colon crypt structure. This is consistent with the data on body weight and colon length.
The alpha-diversity of the mice's intestinal microbiota was evaluated with the Chao1, Shannon, and Simpson indices (data not shown), but the results did not demonstrate a difference in the diversity of intestinal microbiota across the five groups.
Beta-diversity is widely used to compare the similarity of different ecosystems. To identify possible differences between the intestinal bacterial profiles among the five groups, as well as the changes in gut microbiota derived from the AX and CA interventions, the beta-diversity of the samples was assessed using principal coordinates analysis (PCA) and non-metric multidimensional scaling (NMDS). The results are displayed in Figure 4.
The results derived from different methods exhibited similar tendencies, and the data showed that the intestinal microbial profiles of DSS group mice were significantly different from those in the Ctrl group. Intervention with AX and CA shifted the overall intestinal microbial profiles in DSS-treated mice toward those of the Ctrl group, suggesting their modulating effects on the intestinal microbiota. The results further indicated that the microbial profile of CA group mice was closest that of Ctrl group mice, while AX+CA supplementation had little effect on the beta-diversity of the intestinal microbiota. These results were consistent with the tendencies illustrated by the findings for body weight and colon morphological structure.
The taxonomic compositions of the intestinal microbiota of each group, at both the phylum and genus levels, are displayed in Figure 5. At the phylum level, Firmicutes, Proteobacteria, Bacteroidetes, Verrucomicrobia, and Actinobacteria were the dominant communities. DSS treatment significantly decreased the relative abundance of Verrucomicrobia and Actinobacteria. AX and CA supplementation both increased the relative abundance of Verrucomicrobia and decreased the level of Firmicutes. Notably, the combined administration of AX+CA did not significantly affect the relative abundance of Verrucomicrobia or Actinobacteria. At the genus level, Lactobacillus, Akkermansia, Bacteroides, Escherichia/Shigella, Romboutsia, Enterococcus, Erysipelotrichaceae incertae sedis, Blautia, Parasutterella, Robinsoniella, Turicibacter, Clostridium XVIII, Proteus, Clostridium XI, Enterorhabdus, Bifidobacterium, Barnesiella, Citrobacter, and Streptococcus were the most abundant communities.
Figure 5. Intestinal microbial composition of the mice in each group, at the phylum (A) and genus (B) levels.
As shown in Figure 6, LEfSe was used for discriminant analysis of multilevel species differences. For each group, bar charts were drawn for the highest relative abundance. Bars with different colors represent the different species in different groups with an LDA score > 2. Compared to the DSS group, CA supplementation decreased the relative abundance of Enterococcus and Robinsoniella and increased the relative abundance of Coprobacillus, Clostridium XIVa, Enterorhabdus, Corynebacterium, and Akkermansia at the genus level. AX decreased the relative abundance of Clostridium XIVa and increased the level of Akkermansia and Coprobacillus. The combination of AX+CA decreased the relative abundance of Lactobacillus and Robinsoniella and increased the relative abundance of Coprobacillus, Flavonifractor, and Clostridium XIVa. Interestingly, AX and CA exhibited similar regulating effects on certain communities, such as Coprobacillus, and opposite effects on other communities, such as Clostridium XIVa. In addition, AX+CA supplementation significantly modulated the levels of some communities, including Lactobacillus and Flavonifractor, and they were not significantly affected by solo AX or CA.
Figure 6. LEfSe cladogram plot of the intestinal microbiota of mice in the AX (A), CA (B), and AX+CA (C) groups, compared to those in the DSS group.
As shown in Figure 7, the combination of AX and CA significantly regulated the biological functions of the microbial communities. Compared to those of the DSS group, supplementation with AX or CA alone mainly contributed to changes in carbohydrate and amino acid metabolism. However, the modulation of microbial functions derived from the combination of AX+CA was much more sophisticated. AX+CA supplementation had a significant impact on nitrogen metabolism, carbohydrate metabolism, and cellular components, including amino acids, nucleotide bases, monosaccharides, lipids, and lipopolysaccharide biosynthesis.
Figure 7. Biological functions according to the differences in intestinal microbiota of mice in the AX (A), CA (B), and AX+CA (C) groups, compared to those of the DSS group.
Dietary non-starch polysaccharides and phenolics are usually ingested at the same time. They are both considered prebiotics, and they regulate intestinal microbiota through various mechanisms. The exact mechanisms regarding the modulation of intestinal microbiota by polyphenols remain unclear, though it is believed that they could exert regulating potential through antibacterial effects derived from phenol groups. Phenolic compounds can interact with bacterial development as well as substance and energy metabolism, and interfere with cellular membrane integrity and function. In addition, phenolics could inhibit bacterial biofilm formation and quorum sensing (23). On the other hand, non-digestible carbohydrates can modulate the composition and function of the intestinal microbiota as energy sources for some microbial communities. These microorganisms encode certain glycosidases and are capable of degrading and utilizing the given carbohydrates (24). However, their combined or synergistic effects have rarely been discussed in the literature.
Arabinoxylan, a polysaccharide, and chlorogenic acid, a polyphenol, are widely consumed, and their effects on the microbiota have been described in the literature. In the present study, they were given to DSS-treated mice, separately and together, and the intestinal microbiota were investigated by high-throughput sequencing. The data provided insights into the combined effects of different prebiotics.
AX has been shown to promote the proliferation of Prevotella 9, Megamonas, and Bifidobacterium in fermentation by pig or duck intestinal microbiota (25, 26). It has also been observed to increase Lactobacillus, Bifidobacterium, and Bacteroidetes populations, and reduce Escherichia coli populations, in the colonic digesta of high-fat diet–fed mice (27). AX-containing diets promoted probiotic Lactobacillus and Bifidobacterium populations, compared to a basal control diet without fiber, in piglets (28). Supplementation with AX oligosaccharides promoted the growth of bifidobacteria in the cecum of mice fed on a high-fat diet (29). Previous research into the effects of AX on the intestinal microbiota mainly focuses on Bifidobacterium, Lactobacillus, and Bacteroidetes. These populations are known widely as probiotics; their genomes encode glycoside hydrolase family genes, including xylanases and arabinofuranosidases (3, 30). AX-containing diets have been found to downregulate the gene expression of pro-inflammatory cytokines (tumor necrosis factor [TNF]-α, interleukin [IL]-1β, and IL-6) and the TLRs/MyD88/NF-κB pathway, compared to a basal control diet without fiber, in piglets (28). Wheat bran–derived AX oligosaccharides also reduced metabolic endotoxemia, macrophage infiltration in the adipose tissue, and plasma IL-6 in high-fat diet–fed mice, and improved tight junction proteins (ZO-1 and claudin 3) and gut barrier function (29). A synbiotic composed of AX and L. fermentum HFY06 significantly reversed histopathological changes in the colon and inhibited the activation of the NF-κB signaling pathway and the expression of TNF-α, iNOS, and COX-2, alleviating DSS-induced colitis (31). However, many of the previous in vivo studies of AX were performed in mice fed a basal diet or high-fat diet, and the effects of AX on DSS-treated mice have rarely been reported. It should be noted that the modulation of the intestinal microbiota seen with AX supplementation could be highly individualized (13), and the intestinal microbiota–modulating effects of AX seen in the present study may differ from previous data due to different experimental inducements, feeding conditions, and dietary fiber sources (32).
CA has the potential to regulate the intestinal microbiota under various physiological conditions. CA has been shown to increase the relative abundance of Akkermansia and Bacteroides, and reduce the populations of Erysipelatoclostridium, Faecalibaculum, and Erysipelotrichaceae, in L-carnitine–fed mice (33). It has also been found to increase the population of Lactobacillus and decrease that of Escherichia coli in weaned piglets (34). Pigs fed a CA-containing diet had a higher abundance of Lactobacillus, Prevotella, Anaerovibrio, and Alloprevotella in the cecum (35). CA administration alleviated gut dysbiosis by enhancing the relative abundances of Ruminiclostridium 9, Alloprevotella, and Rikenella in cadmium-treated mice (36). CA also increased the relative abundance of Bacteroides, Prevotellaceae UGC-001, and Butyricimonas, and reversed the purine metabolism and glutamate metabolism functions of the gut microbiota, in hyperuricemic mice, and it inhibited the expression of pro-inflammatory cytokines and activation of the TLR4/MyD88/NF-κB signaling pathway in the kidney (15). It also reduced the growth of Bacteroides and Bacteroides-derived liposaccharides and increased the abundance of Lactobacillus in indomethacin- and DSS-induced inflammation, respectively (37, 38).
CA has been shown to activate the Nrf-2/HO-1 pathway in DSS-treated mice, upregulate the expression of anti-inflammatory cytokines, antioxidant enzymes, and gut-tight junction proteins, and alleviate colitis (39). CA has also been reported to attenuate DSS-induced colitis by promoting the growth of Akkermansia in mice (40). Our data are consistent with these previous results.
The combined effects of phenolics and polysaccharides on colon inflammation have only rarely been described in the literature. It was hypothesized that AX and CA, belonging to two different categories of prebiotics, could synthetically modulate the intestinal microbiota to attenuate DSS-induced colitis. Unfortunately, the combination of AX+CA did not exhibit the expected benefits on colon inflammation, at least in the present mice model of DSS-induced colitis; AX depleted the colitis-ameliorating potential of CA. Compared to the DSS group, AX+CA administration increased the relative abundance of Flavonifractor, Coprobacillus, and Clostridium_XIVa, and decreased the populations of Robinsoniella and Lactobacillus.Gut-derived Flavonifractor species could be involved in degrading flavonoids; oral administration of F. plautii has been shown to promote recovery from acute colitis in mice via suppression of IL-17 (41), attenuation of inflammatory responses in obese adipose tissue (42), and suppression of Th2 immune responses (43). An association between F. plautii and the gut microbiome was identified in patients with colorectal cancer in India (44), and it is regarded as a potential pathogen for cholecystitis (45).
The Eubacterium-like genus Coprobacillus nov. was first isolated and identified from human feces in 2000 (46). Coprobacillus is thought to positively interact with Akkermansia and Blautia; they maintain intestinal stability and confer resistance against colonization with the pathogen Clostridium difficile (47). The abundance of Coprobacillus cateniformis was improved by supplementation of chitosan and positively correlated with serum leptin in high-fat diet–fed mice (48). The abundance of Coprobacillus was found to be decreased in the gut microbiota of patients with acne (49). Notably, C. cateniformis has been reported to cause bacteremia (50). Besides the effects on microbial composition, AX+CA contributed to more complex changes in the biological functions of the intestinal microbiota.
In summary, CA attenuated body weight loss, colon shortening, and histological damage in DSS-treated mice, while AX and the combination of AX+CA did not exhibit any potential to ameliorate these effects. AX+CA had less of a modulating effect on the profile of the intestinal microbiota than did CA. AX+CA administration increased the relative abundance of Flavonifractor, Coprobacillus, and Clostridium XIVa, and decreased the populations of Robinsoniella and Lactobacillus, in DSS-treated mice. Compared to AX or CA alone, AX+CA contributed to a more complicated shift in the biological functions of the intestinal microbiotaAX seemed to weaken the beneficial effects of CA, at least in the present experimental model of DSS-induced colitis. The combined effects and mechanisms of dietary polysaccharides and phenolic compounds on the intestinal microbiota and on overall health still need to be investigated further.
The original contributions presented in the study were included in the article, and further inquiries can be directed to the corresponding author. The raw sequence data generated and presented in the study were deposited in the Genome Sequence Archive in the BIG Data Center Chinese Academy of Sciences (https://ngdc.cncb.ac.cn/gsa/) with Bio Project number PRJCA013252 and accession number CRA008935.
The animal study was reviewed and approved by Ethical Committee of Nanjing Agricultural University Animal Experiment Center.
MX: conception, investigation, formal analysis, writing—original draft, and funding acquisition. XZh and XW: investigation and formal analysis. GC: investigation and project administration. JL: writing—review and editing. XZe and WY: conception, supervision, writing—review, and funding acquisition. All authors contributed to the manuscript and approved the submitted version.
This research was supported by the National Natural Science Foundation of China (32001700), the Natural Science Foundation of Jiangsu Province (BK20221347), and the Priority Academic Program Development of Jiangsu Higher Education Institutions (PAPD).
The authors declare that the research was conducted in the absence of any commercial or financial relationships that could be construed as a potential conflict of interest.
All claims expressed in this article are solely those of the authors and do not necessarily represent those of their affiliated organizations, or those of the publisher, the editors and the reviewers. Any product that may be evaluated in this article, or claim that may be made by its manufacturer, is not guaranteed or endorsed by the publisher.
1. GBD 2017 Diet Collaborators. Health effects of dietary risks in 195 countries, 1990-2017: A systematic analysis for the Global Burden of Disease Study 2017. Lancet. (2019) 393:1958–72. doi: 10.1016/S0140-6736(19)30041-8
2. Zhang K, Sun J, Fan M, Qian H, Ying H, Li Y, et al. Functional ingredients present in whole-grain foods as therapeutic tools to counteract obesity: Effects on brown and white adipose tissues. Trends Food Sci Technol. (2021) 109:513–26. doi: 10.1016/j.tifs.2021.01.055
3. Mendis M, Leclerc E, Simsek S. Arabinoxylans, gut microbiota and immunity. Carbohydr Polym. (2016) 139:159–66. doi: 10.1016/j.carbpol.2015.11.068
4. Wang J, Bai J, Fan M, Li T, Li Y, Qian H, et al. Cereal-derived arabinoxylans: Structural features and structure–activity correlations. Trends Food Sci Technol. (2020) 96:157–65. doi: 10.1016/j.tifs.2019.12.016
5. He HJ, Qiao J, Liu Y, Guo Q, Ou X, Wang X. Isolation, structural, functional, and bioactive properties of cereal arabinoxylan–a critical review. J Agric Food Chem. (2021) 69:15437–57. doi: 10.1021/acs.jafc.1c04506
6. Ooi SL, McMullen D, Golombick T, Nut D, Pak SC. Evidence-based review of BioBran/MGN-3 arabinoxylan compound as a complementary therapy for conventional cancer treatment. Integr Cancer Ther. (2017) 17:165–78. doi: 10.1177/1534735417735379
7. Clifford MN, Kerimi A, Williamson G. Bioavailability and metabolism of chlorogenic acids (acyl-quinic acids) in humans. Compr Rev Food Sci Food Saf. (2020) 19:1299–352. doi: 10.1111/1541-4337.12518
8. Santana-Galvez J, Cisneros-Zevallos L, Jacobo-Velazquez DA. Chlorogenic acid: recent advances on its dual role as a food additive and a nutraceutical against metabolic syndrome. Molecules. (2017) 22:358. doi: 10.3390/molecules22030358
9. Liu S, Zhao W, Lan P, Mou X. The microbiome in inflammatory bowel diseases: from pathogenesis to therapy. Protein Cell. (2021) 12:331–45. doi: 10.1007/s13238-020-00745-3
10. Khalili H, Chan SS, Lochhead P, Ananthakrishnan AN, Hart AR, Chan AT. The role of diet in the aetiopathogenesis of inflammatory bowel disease. Nat Rev Gastroenterol Hepatol. (2018) 15:525–35. doi: 10.1038/s41575-018-0022-9
11. Gibson GR, Hutkins RW, Sanders ME, Prescott SL, Reimer RA, Salminen SJ, et al. The International Scientific Association for Probiotics and Prebiotics (ISAPP) consensus statement on the definition and scope of prebiotics. Nat Rev Gastroenterol Hepatol. (2017) 14:491–502. doi: 10.1038/nrgastro.2017.75
12. Gu I, Lam WS, Marasini D, Brownmiller C, Savary BJ, Lee JA, et al. In vitro fecal fermentation patterns of arabinoxylan from rice bran on fecal microbiota from normal-weight and overweight/obese subjects. Nutrients. (2021) 13:2052. doi: 10.3390/nu13062052
13. Nguyen NK, Deehan EC, Zhang Z, Jin M, Baskota N, Perez-Muñoz ME, et al. Gut microbiota modulation with long-chain corn bran arabinoxylan in adults with overweight and obesity is linked to an individualized temporal increase in fecal propionate. Microbiome. (2020) 8:118. doi: 10.1186/s40168-020-00887-w
14. Nie Q, Hu J, Chen H, Geng F, Nie S. Arabinoxylan ameliorates type 2 diabetes by regulating the gut microbiota and metabolites. Food Chem. (2022) 371:131106. doi: 10.1016/j.foodchem.2021.131106
15. Zhou X, Zhang B, Zhao X, Lin Y, Wang J, Wang X, et al. Chlorogenic acid supplementation ameliorates hyperuricemia, relieves renal inflammation, and modulates intestinal homeostasis. Food Funct. (2021) 12:5637–49. doi: 10.1039/D0FO03199B
16. Shi A, Li T, Zheng Y, Song Y, Wang H, Wang N, et al. Chlorogenic acid improves NAFLD by regulating gut microbiota and GLP-1. Front Pharmacol. (2021) 12:693048. doi: 10.3389/fphar.2021.693048
17. Xie M, Yang J, Zhang J, Sherman HL, Zhang Z, Minter LM, et al. Effects of linoleic acid-rich diet on plasma profiles of eicosanoids and development of colitis in il-10-/- mice. J Agric Food Chem. (2020) 68:7641–7. doi: 10.1021/acs.jafc.0c03024
18. Bolyen E, Rideout JR, Dillon MR, Bokulich NA, Abnet CC, Al-Ghalith GA, et al. Reproducible, interactive, scalable and extensible microbiome data science using QIIME 2. Nat Biotechnol. (2019) 37:852–7. doi: 10.1038/s41587-019-0209-9
19. Callahan BJ, McMurdie PJ, Rosen MJ, Han AW, Johnson AJA, Holmes SP. DADA2: High-resolution sample inference from Illumina amplicon data. Nat Methods. (2016) 13:581–3. doi: 10.1038/nmeth.3869
20. Douglas GM, Maffei VJ, Zaneveld JR, Yurgel SN, Brown JR, Taylor CM, et al. PICRUSt2 for prediction of metagenome functions. Nature Biotechnol. (2020) 38:685–8. doi: 10.1038/s41587-020-0548-6
21. Segata N, Izard J, Waldron L, Gevers D, Miropolsky L, Garrett WS, et al. Metagenomic biomarker discovery and explanation. Genome Biol. (2011) 12:R60. doi: 10.1186/gb-2011-12-6-r60
22. Paulson JN, Pop M, Bravo HC. Metastats: an improved statistical method for analysis of metagenomic data. Genome Biol. (2011) 12:P17. doi: 10.1186/1465-6906-12-S1-P17
23. Espín JC, González-Sarrías A, Tomás-Barberán FA. The gut microbiota: a key factor in the therapeutic effects of (poly)phenols. Biochem Pharmacol. (2017) 139:82–93. doi: 10.1016/j.bcp.2017.04.033
24. Davani-Davari D, Negahdaripour M, Karimzadeh I, Seifan M, Mohkam M, Masoumi SJ, et al. Prebiotics: definition, types, sources, mechanisms, and clinical applications. Foods. (2019) 8:92. doi: 10.3390/foods8030092
25. Bai Y, Zhou X, Li N, Zhao J, Ye H, Zhang S, et al. In vitro fermentation characteristics and fiber-degrading enzyme kinetics of cellulose, arabinoxylan, β-glucan and glucomannan by pig fecal microbiota. Microorganisms. (2021) 9:1071. doi: 10.3390/microorganisms9051071
26. Tian D, Xu X, Peng Q, Wen Z, Zhang Y, Wei C, et al. In vitro fermentation of arabinoxylan from oat (Avena sativa L) by Pekin duck intestinal microbiota. 3 Biotech. (2019) 9:54. doi: 10.1007/s13205-019-1571-5
27. Li S, Sun Y, Hu X, Qin W, Li C, Liu Y, et al. Effect of arabinoxylan on colonic bacterial metabolites and mucosal barrier in high-fat diet-induced rats. Food Sci Nutr. (2019) 7:3052–61. doi: 10.1002/fsn3.1164
28. Chen H, Chen D, Qin W, Liu Y, Che L, Huang Z, et al. Wheat bran components modulate intestinal bacteria and gene expression of barrier function relevant proteins in a piglet model. Int J Food Sci Nutr. (2017) 68:65–72. doi: 10.1080/09637486.2016.1212817
29. Neyrinck AM, Van Hee VF, Piront N, De Backer F, Toussaint O, Cani PD, et al. Wheat-derived arabinoxylan oligosaccharides with prebiotic effect increase satietogenic gut peptides and reduce metabolic endotoxemia in diet-induced obese mice. Nutr Diabetes. (2012) 2:e28. doi: 10.1038/nutd.2011.24
30. Komeno M, Yoshihara Y, Kawasaki J, Nabeshima W, Maeda K, Sasaki Y, et al. Two alpha-L-arabinofuranosidases from Bifidobacterium longum subsp. longum are involved in arabinoxylan utilization. Appl Microbiol Biotechnol. (2022) 106:1957–65. doi: 10.1007/s00253-022-11845-x
31. Li F, Huang H, Zhu F, Zhou X, Yang Z, Zhao XA. Mixture of Lactobacillus fermentum HFY06 and arabinoxylan ameliorates dextran sulfate sodium-induced acute ulcerative colitis in mice. J Inflammation Res. (2021) 14:6575–85. doi: 10.2147/JIR.S344695
32. Paesani C, Degano AL, Salvucci E, Zalosnik MI, Fabi JP, Sciarini LS, et al. Soluble arabinoxylans extracted from soft and hard wheat show a differential prebiotic effect in vitro and in vivo. J Cereal Sci. (2020) 93:102956. doi: 10.1016/j.jcs.2020.102956
33. Zhang X, Shi L, Chen R, Zhao Y, Ren D, Yang X. Chlorogenic acid inhibits trimethylamine-N-oxide formation and remodels intestinal microbiota to alleviate liver dysfunction in high l-carnitine feeding mice. Food Funct. (2021) 12:10500–11. doi: 10.1039/D1FO01778K
34. Zhang Y, Wang Y, Chen D, Yu B, Zheng P, Mao X, et al. Dietary chlorogenic acid supplementation affects gut morphology, antioxidant capacity and intestinal selected bacterial populations in weaned piglets. Food Funct. (2018) 9:4968–78. doi: 10.1039/C8FO01126E
35. Chen J, Yu B, Chen D, Zheng P, Luo Y, Huang Z, et al. Changes of porcine gut microbiota in response to dietary chlorogenic acid supplementation. Appl Microbiol Biotechnol. (2019) 103:8157–68. doi: 10.1007/s00253-019-10025-8
36. Ding Y, Li X, Liu Y, Wang S, Cheng D. Protection mechanisms underlying oral administration of chlorogenic acid against cadmium-induced hepatorenal injury related to regulating intestinal flora balance. J Agric Food Chem. (2021) 69:1675–83. doi: 10.1021/acs.jafc.0c06698
37. Yan Y, Zhou X, Guo K, Zhou F, Yang H. Chlorogenic acid protects against indomethacin-induced inflammation and mucosa damage by decreasing Bacteroides-derived LPS. Front Immunol. (2020) 11:1125. doi: 10.3389/fimmu.2020.01125
38. Zhang P, Jiao H, Wang C, Lin Y, You S. Chlorogenic acid ameliorates colitis and alters colonic microbiota in a mouse model of dextran sulfate sodium-induced colitis. Front Physiol. (2019) 10:325. doi: 10.3389/fphys.2019.00325
39. Wan F, Cai X, Wang M, Chen L, Zhong R, Liu L, et al. Chlorogenic acid supplementation alleviates dextran sulfate sodium (DSS)-induced colitis via inhibiting inflammatory responses and oxidative stress, improving gut barrier integrity and Nrf-2/HO-1 pathway. J Funct Foods. (2021) 87:104808. doi: 10.1016/j.jff.2021.104808
40. Zhang Z, Wu X, Cao S, Cromie M, Shen Y, Feng Y, et al. Chlorogenic acid ameliorates experimental colitis by promoting growth of Akkermansia in mice. Nutrients. (2017) 9:677. doi: 10.3390/nu9070677
41. Mikami A, Ogita T, Namai F, Shigemori S, Sato T, Shimosato T. Oral administration of Flavonifractor plautii, a bacteria increased with green tea consumption, promotes recovery from acute colitis in mice via suppression of IL-17. Front Nutr. (2021) 7:610946. doi: 10.3389/fnut.2020.610946
42. Mikami A, Ogita T, Namai F, Shigemori S, Sato T, Shimosato T. Oral administration of Flavonifractor plautii attenuates inflammatory responses in obese adipose tissue. Mol Biol Rep. (2020) 47:6717–25. doi: 10.1007/s11033-020-05727-6
43. Ogita T, Yamamoto Y, Mikami A, Shigemori S, Sato T, Shimosato T. Oral administration of Flavonifractor plautii strongly suppresses Th2 immune responses in mice. Front Immunol. (2020) 11:379. doi: 10.3389/fimmu.2020.00379
44. Gupta A, Dhakan DB, Maji A, Saxena R, PK VP, Mahajan S, et al. Association of Flavonifractor plautii, a flavonoid-degrading bacterium, with the gut microbiome of colorectal cancer patients in India. mSystems. (2019) 4:e00438–19. doi: 10.1128/mSystems.00438-19
45. Berger FK, Schwab N, Glanemann M, Bohle RM, Gärtner B, Groesdonk HV. Flavonifractor (Eubacterium) plautii bloodstream infection following acute cholecystitis. IDCases. (2018) 14:e00461. doi: 10.1016/j.idcr.2018.e00461
46. Kageyama A, Benno Y. Coprobacillus catenaformis gen. nov, sp nov, a new genus and species isolated from human feces. Microbiol Immunol. (2000) 44:23–8. doi: 10.1111/j.1348-0421.2000.tb01242.x
47. Stein RR, Bucci V, Toussaint NC, Buffie CG, Rätsch G, Pamer EG, et al. Ecological modeling from time-series inference: Insight into dynamics and stability of intestinal microbiota. PLoS Comput Biol. (2013) 9:e1003388. doi: 10.1371/journal.pcbi.1003388
48. Tang D, Wang Y, Kang W, Zhou J, Dong R, Feng Q. Chitosan attenuates obesity by modifying the intestinal microbiota and increasing serum leptin levels in mice. J Funct Foods. (2020) 64:103659. doi: 10.1016/j.jff.2019.103659
49. Yan HM, Zhao HJ, Guo DY, Zhu PQ, Zhang CL, Jiang W. Gut microbiota alterations in moderate to severe acne vulgaris patients. J Dermatol. (2018) 45:1166–71. doi: 10.1111/1346-8138.14586
Keywords: arabinoxylan, chlorogenic acid, intestinal microbiota, dextran sulfate sodium-treated mice, synergistic
Citation: Xie M, Zhang X, Wang X, Chen G, Liu J, Zeng X and Yang W (2022) Effects of arabinoxylan and chlorogenic acid on the intestinal microbiota in dextran sulfate sodium–treated mice. Front. Nutr. 9:950446. doi: 10.3389/fnut.2022.950446
Received: 22 May 2022; Accepted: 27 October 2022;
Published: 28 November 2022.
Edited by:
Guifang Tian, Agricultural University of Hebei, ChinaReviewed by:
Biao Yuan, China Pharmaceutical University, ChinaCopyright © 2022 Xie, Zhang, Wang, Chen, Liu, Zeng and Yang. This is an open-access article distributed under the terms of the Creative Commons Attribution License (CC BY). The use, distribution or reproduction in other forums is permitted, provided the original author(s) and the copyright owner(s) are credited and that the original publication in this journal is cited, in accordance with accepted academic practice. No use, distribution or reproduction is permitted which does not comply with these terms.
*Correspondence: Xiaoxiong Zeng, emVuZ3h4QG5qYXUuZWR1LmNu; Wenjian Yang, bGluZ3dlbnR0QDE2My5jb20=
Disclaimer: All claims expressed in this article are solely those of the authors and do not necessarily represent those of their affiliated organizations, or those of the publisher, the editors and the reviewers. Any product that may be evaluated in this article or claim that may be made by its manufacturer is not guaranteed or endorsed by the publisher.
Research integrity at Frontiers
Learn more about the work of our research integrity team to safeguard the quality of each article we publish.