- 1Department of Maternal and Child Health, School of Public Health, China Medical University, Shenyang, China
- 2The Second People’s Hospital of Jiashan, Jiaxing, China
- 3Xinzhou District Center for Disease Control and Prevention, Wuhan, China
- 4The First Division of Clinical Medicine, China Medical University, Shenyang, China
- 5Department of Dermatology, First Hospital of China Medical University, Shenyang, China
- 6Key Laboratory of Immunodermatology, Ministry of Education and NHC, National Joint Engineering Research Center for Theranostics of Immunological Skin Diseases, Shenyang, China
- 7Department of Pediatrics, First Hospital of China Medical University, Shenyang, China
Exercise is considered as a favorable measure to prevent and treat childhood obesity. However, the underlying mechanisms of exercise-induced beneficial effects and the difference between obese and non-obese individuals are largely unclear. Recently, miR-27a is recognized as a central upstream regulator of proliferator-activated receptor γ (PPAR-γ) in contributing to various physiological and pathological processes. This study aims to explore the possible cause of exercise affecting white adipose tissue (WAT) browning and reversing skeletal muscle insulin resistance in obese/non-obese immature bodies. For simulating the process of childhood obesity, juvenile mice were fed with a basal diet or high-fat diet (HFD) and took 1 or 2 h swimming exercise simultaneously for 10 weeks. The obese animal model was induced by the HFD. We found that exercise hindered HFD-induced body fat development in growing mice. Exercise modified glucolipid metabolism parameters differently in the obese/non-obese groups, and the changes of the 2 h exercise mice were not consistent with the 1 h exercise mice. The level of serum exosomal miR-27a in the non-exercise obese group was increased obviously, which was reduced in the exercise obese groups. Results from bioinformatics analysis and dual-luciferase reporter assay showed that miR-27a targeted PPAR-γ. Exercise stimulated WAT browning; however, the response of obese WAT lagged behind normal WAT. In the HFD-fed mice, 2 h exercise activated the IRS-1/Akt/GLUT-4 signaling pathway in the skeletal muscles. In summary, our findings confirmed that exercise-induced beneficial effects are associated with exercise duration, and the response of obese and non-obese bodies is different. Exosomal miR-27a might be a crucial node for the process of exercise-induced browning of WAT and improving skeletal muscle insulin sensitivity.
Introduction
Childhood obesity has become one of the most serious global public health challenges in the 21st century, which is an independent disease and can lead to various health impairments, such as insulin resistance, dysglycemia, hypertension, fatty liver, and psychosocial complications (1, 2). Irregular or excessive fat accumulation is distinguished in children with obesity. Imbalance between energy intake and expenditure is the root cause (3).
Described as simple, economical, safe, and feasible characteristic, exercise has been considered as a favorable measure to prevent and treat overweight/obesity in children and adolescents. Although the mechanisms of exercise-induced beneficial effects are not fully understood, convincing conclusions have been drawn that exercise can promote the browning effect of white adipose tissue (WAT) (4), improve insulin sensitivity, and regulate metabolism of glucose and lipids in obese bodies (5). However, the molecular mechanisms of WAT browning and reversing insulin resistance are less clear. Moreover, investigation is lacking on the difference between obese individuals and non-obese individuals in the responsiveness to exercise-mediated browning of WAT (6).
The mechanisms on exercise-mediated beneficial effects remain to be portrayed in-depth. Exosomes emerge as a type of extracellular vesicles carrying a large number of biological information molecules (e.g., protein, DNA, RNA, mRNA, and miRNA), which act in regulating intercellular communication and contribute to various physiological and pathological processes (7). A growing body of evidence indicates that circulating miRNA levels are good biomarkers for quantifying the physiological impacts of dietary or lifestyle intervention studies (8). In addition, miRNA expression in circulation varies widely between subjects who exercise or those who do not exercise (9). People have recognized that miRNA plays an important role in regulating energy homeostasis and WAT browning, affecting glucose and lipid metabolisms and insulin sensitivity (10). Among them, miR-27a exhibits a prominent role. miR-27a is highly expressed in adipose tissue (11). In a cold exposure study in mice, miR-27a was downregulated in subcutaneous WAT; meanwhile, researchers also showed its direct effect on PPAR-γ during brown adipogenesis of primary preadipocytes in vitro. The results pointed to miR-27a as a central upstream regulator of the transcriptional network involved in brown adipogenesis after cold exposure (12). Adipocyte-derived extracellular miR-27a was proposed as a messenger between adipose tissue and other tissues (13). Yu Y et al. showed that the serum miR-27a level correlated positively with obesity and insulin resistance in obese children, and adipocyte-derived exosomal miR-27a could result in insulin resistance in skeletal muscles by targeting PPAR-γ (14).
Skeletal muscles serve as an important exercise and energy metabolism organ. The resistance state in skeletal muscles can significantly affect systemic glucose homeostasis and insulin sensitivity (15). It is thought that skeletal muscle insulin resistance even precedes hepatic insulin resistance in humans with metabolic syndrome (16). In addition, miRNA expression in circulation varies widely between subjects who exercise or do not exercise (9).
Thus, the present study aims to explore the possible cause of exercise affecting WAT browning and skeletal muscle insulin resistance from the perspective of exosomal miRNA. Juvenile mice were fed with a high-fat diet or basal diet and took voluntary exercise at the same time to simulate the process of childhood obesity. The responses in obese and non-obese groups were compared, as well as exercise and non-exercise groups; particularly, the alteration of exosomal miR-27a and PPAR-γ was evaluated in vivo.
Materials and Methods
Animals
In total, 60 first-weaned C57BL/6 male mice were purchased from Beijing Huafukang Biotechnology Co., Ltd. (experimental animal production license number: SCXK (Beijing) 2019-0008). After 1 week of adaptive feeding, the mice were randomly divided into two dietary groups (n = 30/group) and fed with either a basal diet (13% fat) or high-fat diet (60% fat, D12492, Research Diets, China). Mice in each dietary group were then randomized into quiet group (untrained), 1 h exercise group, and 2 h exercise group. Consequently, six groups (n = 10/group) were analyzed during this study: basal quiet group (CON-S), basal 1 h exercise group (CON-PA1), basal 2 h exercise group (CON-PA2), high-fat quiet group (HFD-S), high-fat 1 h exercise group (HFD-PA1), and high-fat 2 h exercise group (HFD-PA2). Dietary and exercise interventions were delivered simultaneously for 10 weeks. All mice were housed in standard cages, and their food intake was recorded weekly.
Exercise Protocol
Exercise intervention was carried out through non-weight-bearing swimming training. The exercise program was based on the mouse swimming exercise load standard in “Resource Book for the Design of Animal Exercise Protocols” published by the American Physiological Society in 2006 and referred to the relevant literature (17). CON-PA1 and HFD-PA1 groups swam 2 × 30 min every day with a 5 min interval; CON-PA2 and HFD-PA2 groups swam 4 × 30 min every day with a 5 min interval. In the 1st week, all mice in the exercise groups performed adaptive training. Intensity was 20 min/day in the first 3 days and then increased gradually every day. From the 2nd week, the training mice swam for 60 min or 120 min per day, respectively, for 5 days, with a 2 day interval for 10 weeks. Swimming training was carried out in a swimming tank of 120 × 80 × 80 cm, and the water temperature was controlled at 30 ± 2°C.
Body Composition Measurements and Tissue Collection
Fat and lean masses were measured by using a Bruker Minispec LF50 (Bruker, Germany). After the mice were anesthetized with 3% sodium pentobarbital, one eyeball was enucleated, and blood was collected. The serum was centrifuged at 1356 × g for 15 min at 4°C. After the mice were killed, the subcutaneous and visceral (epididymal and perirenal) WAT and quadriceps muscle were isolated. Fat coefficients were calculated, as previously described (18).
Insulin Tolerance Tests
The blood from the tail vein of the mice was dropped on the blood glucose test strip and measured by using a blood glucose meter. For the OGTT, after fasting for 12 h, the mice were given glucose (2 g/kg) by intragastric administration, and the blood glucose values were measured at 0, 15, 30, 60, 90, and 120 min. For the ITT, after fasting for 2 h, the mice were injected with insulin (1 U/kg), and the blood glucose values of the mice were measured at 0, 15, 30, 45, and 60 min (19). All values were recorded to draw curves, and the area under the curve (AUC) and ITT-AUC were calculated.
Serum Preparation and Parameter Tests
Serum triglyceride (TG), total cholesterol (TC), low-density lipoprotein cholesterol (LDL-C), and high-density lipoprotein cholesterol (HDL-C) levels were measured using specific reagent kits (Nanjing Jiancheng, China). Insulin was measured by ELISA (Cusabio, Wuhan, China). HOMA-IR was calculated as the fasting insulin level (mU/L) × fasting blood glucose level (mmol/L)/22.5.
Histology
White adipose tissue and skeletal muscle tissue were fixed with 4% paraformaldehyde for 24-48 h. Paraffin sections were stained with hematoxylin and eosin (H&E). Panoramic image acquisition was performed using a scanner, and Image-Pro Plus 6.0 software was used for analysis.
Isolation of Serum Exosomes
Serum exosomes were isolated according to the instruction of Ribo™ exosome isolation reagent (Ribo, Guangzhou, China).
Transmission Electron Microscopy to Identify Exosomes
The exosome PBS suspension was taken on the sample-carrying copper plate and allowed it to stand for 4 min; excess liquid was wiped off with a filter paper; negative staining with phosphotungstic acid was performed for 2 min; a filter paper was used to absorb excess water; its size and shape were observed under a transmission electron microscope; and pictures were collected.
Gene Expression
Total RNA was extracted with TRIzol (TaKaRa, Japan). RNA purity and concentrations were determined with a Nanodrop 2000 spectrophotometer (Thermo, United States). The concentrations generally ranged from 500 to 700 ng/ul. The A260/280 absorbance ratio of 1.8:2.1 was used to assess the purity of RNA and DNA preparations. Totally, 2 μg RNA was extracted for DNA digestion and reverse transcription. Gene expression was analyzed by TB Green real-time quantitative PCR (qPCR) (TaKaRa, Japan). PCR amplification was performed using a QuantStudio6 Flex Real-time Quantitative PCR system (Applied Biosystems, United States) as follows: 95°C for 30 s, one cycle; 95°C for 5 s, 60°C for 34 s, 40 cycles; 95°C for 15 s, 60°C for 1 min, and 95°C for 15 s, one cycle. The level of mRNAs and miRNAs was normalized to the levels of β-actin or U6 exanimated in each sample using the 2–ΔΔCT method. All primer sequences are listed in Table 1.
Dual-Luciferase Reporter Assay
The sequences of 3′-UTR of PPAR-γ were chemically synthesized and introduced into the luciferase reporter vector to construct the wild-type (WT) luciferase reporter plasmids, and the seed regions of miR-27a-3p in the 3′-UTR of PPAR-γ were mutated to construct mutant (Mut) luciferase reporter plasmids. 293T cells were co-transfected with luciferase reporter plasmids and miRNA mimics or inhibitors. After 48 h, the cells were collected, and dual-luciferase activity was measured using the Dual-Luciferase Reporter Assay (Ribo, Guangzhou, China) according to the manufacturer’s instructions.
Western Blot Analysis
For the analysis, 20 μg soluble protein was isolated with 7.5/10% SDS-PAGE gel and transferred to a polyvinylidene fluoride membrane, enclosed with 5% skim milk. The protein was incubated overnight with a primary antibody at 4°C and then incubated with a secondary antibody at room temperature for 1 h. The target protein was normalized according to the β-actin protein content.
Statistical Analyses
Statistical analyses were performed by SPSS software. The data are expressed as mean ± SD. Student’s t-test was used for comparison between the two groups, and one-way ANOVA or two-factor ANOVA was used for comparison between three or more groups. P-values < 0.05 were considered statistically significant.
Results
Exercise Hindered High-Fat Diet-Induced Body Fat Development in Growing Mice
To observe the anti-obesity effect of exercise, juvenile mice were fed with a basal diet (CON) or high-fat diet (HFD) and took swimming exercise simultaneously for 10 weeks. Compared with the CON-S group, the HFD-S group mice were obese, which had higher body weight, more body weight gain, a higher body fat ratio, and a visceral fat coefficient (Figures 1A,B,D). Exercise training significantly reduced the effect of body weight gain of HFD feeding (Figures 1A,B). Energy intake of exercise mice decreased compared with non-exercise mice (Figure 1C). The total body fat mass reduced in HFD-PA1 and HFD-PA2 groups, but the body fat mass percentage changes were not obvious in these exercise groups. The effects of exercise on lean body mass were not obvious in the two dietary treatments (Table 2).
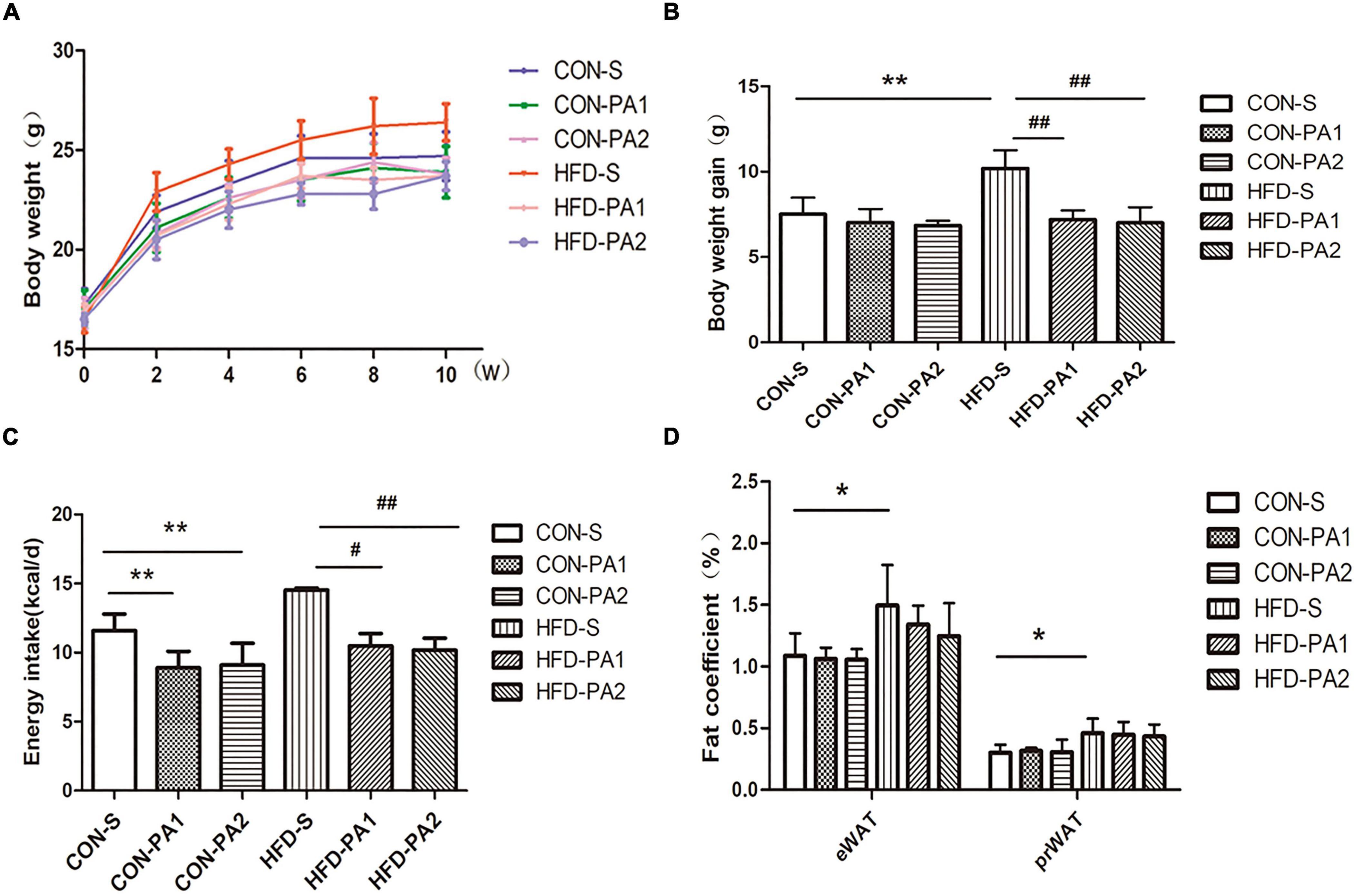
Figure 1. Effects of exercise on body weight, body weight gain, energy intake, and visceral fat in juvenile mice. (A) Bodyweight change. (B) Comparison of body weight gain. (C) Comparison of energy intake. (D) Comparison of epididymal and perirenal fat coefficients. Compared with the CON-S group, *P < 0.05 and **P < 0.01; compared with the HFD-S group, #P < 0.05 and ##P < 0.01.
In addition, obvious morphological changes were seen in both CON and HFD exercise mice. Compared with the CON-S group, the adipocyte sizes of exercise mice (CON-PA1 and CON-PA2 groups) were smaller, while the cross-sectional area of skeletal muscle fibers in the HFD-PA2 group was significantly larger. Compared with the HFD-S group, the adipocyte sizes of exercise mice (HFD-PA1 and HFD-PA2 groups) were smaller, while the cross-sectional area of skeletal muscle fibers in the HFD-PA2 group was significantly larger (Figures 2I–IV).
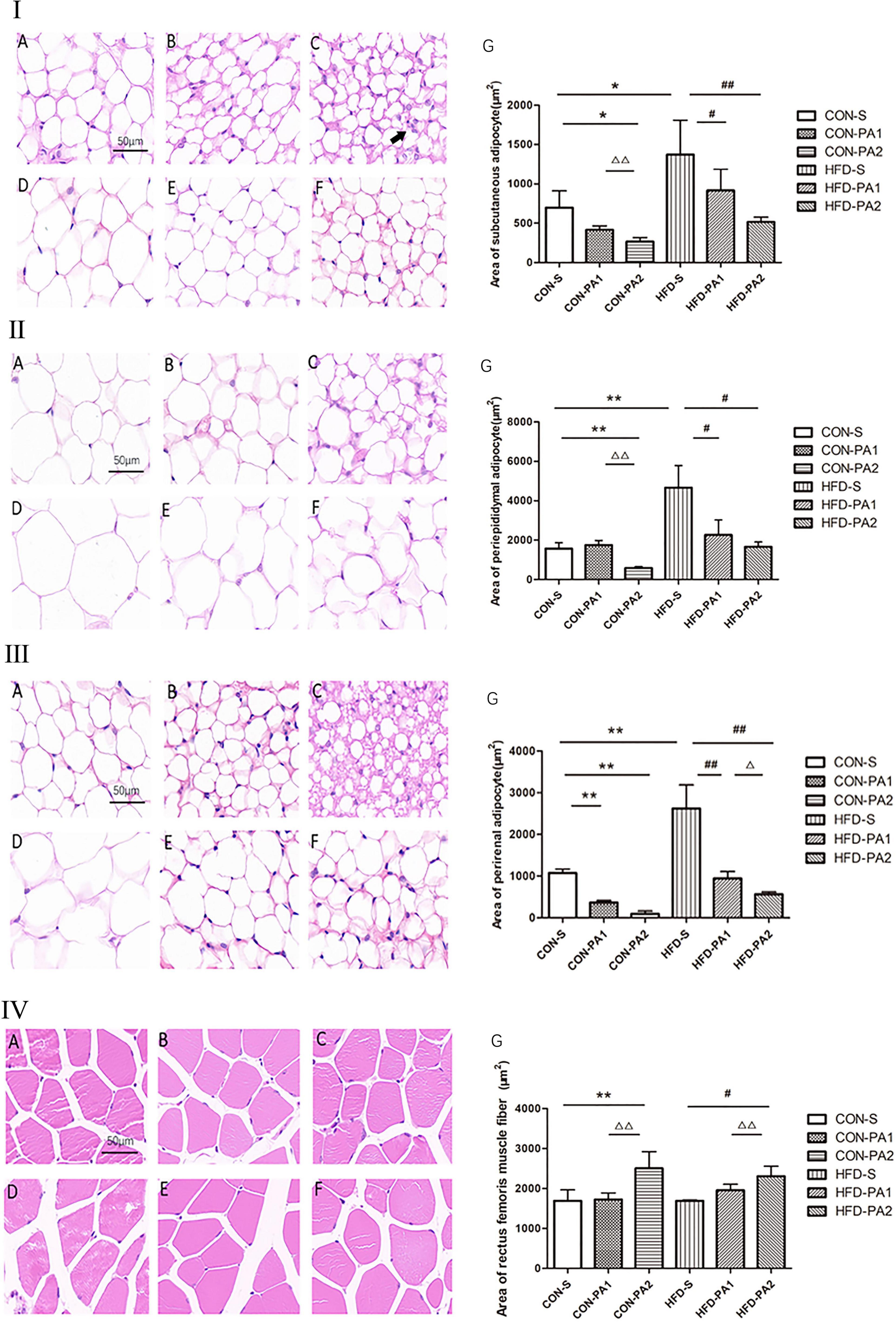
Figure 2. HE staining analysis of adipose tissue and skeletal muscles of mice. (I) Subcutaneous adipocytes. (II) Epididymal adipocytes. (III) Perirenal adipocytes. (IV) Skeletal muscle cells. (A) CON-S group. (B) CON-PA1 group. (C) CON-PA2 group. (D) HFD-S group. (E) HFD-PA1 group. (F) HFD-PA2 group. (G) Area comparison of adipocytes and skeletal muscle cells in each group. Compared with the CON-S group, *P < 0.05 and **P < 0.01; compared with the HFD-S group, #P < 0.05 and ##P < 0.01; comparison between exercise groups (CON-PA1 vs. CON-PA2, HFD-PA1 vs. HFD-PA2), ΔP < 0.05 and ΔΔP < 0.01.
Exercise Modified Glucolipid Metabolism Parameters of Obese/Non-obese Growing Mice
To investigate the therapeutic effect of exercise on metabolic disorders, serum biochemical parameters related to lipid and glucose metabolisms were measured. Compared with the CON-S group, TG, TCHO, LDL-C, FPG, FINS concentrations, and HOMA-IR of the obese HFD-S group were significantly increased. In basal diet-fed groups, 2 h exercise significantly reduced the levels of TCHO and LDL-C and increased the level of HDL-C. In HFD-fed groups, 2 h exercise significantly reduced the levels of TG and TCHO and increased the level of HDL-C; 1 and 2 h exercise significantly reduced the levels of FPG, FINS, and HOMA-IR (Table 3). Since insulin resistance is highly positively correlated with the progression of obesity, OGTT and ITT were completed for evaluating whether exercise could improve the insulin sensitivity of obese mice. The results showed the OGTT-AUC and ITT-AUC were decreased in the HFD-PA1 and HFD-PA2 groups compared with HFD-S group (Figure 3).
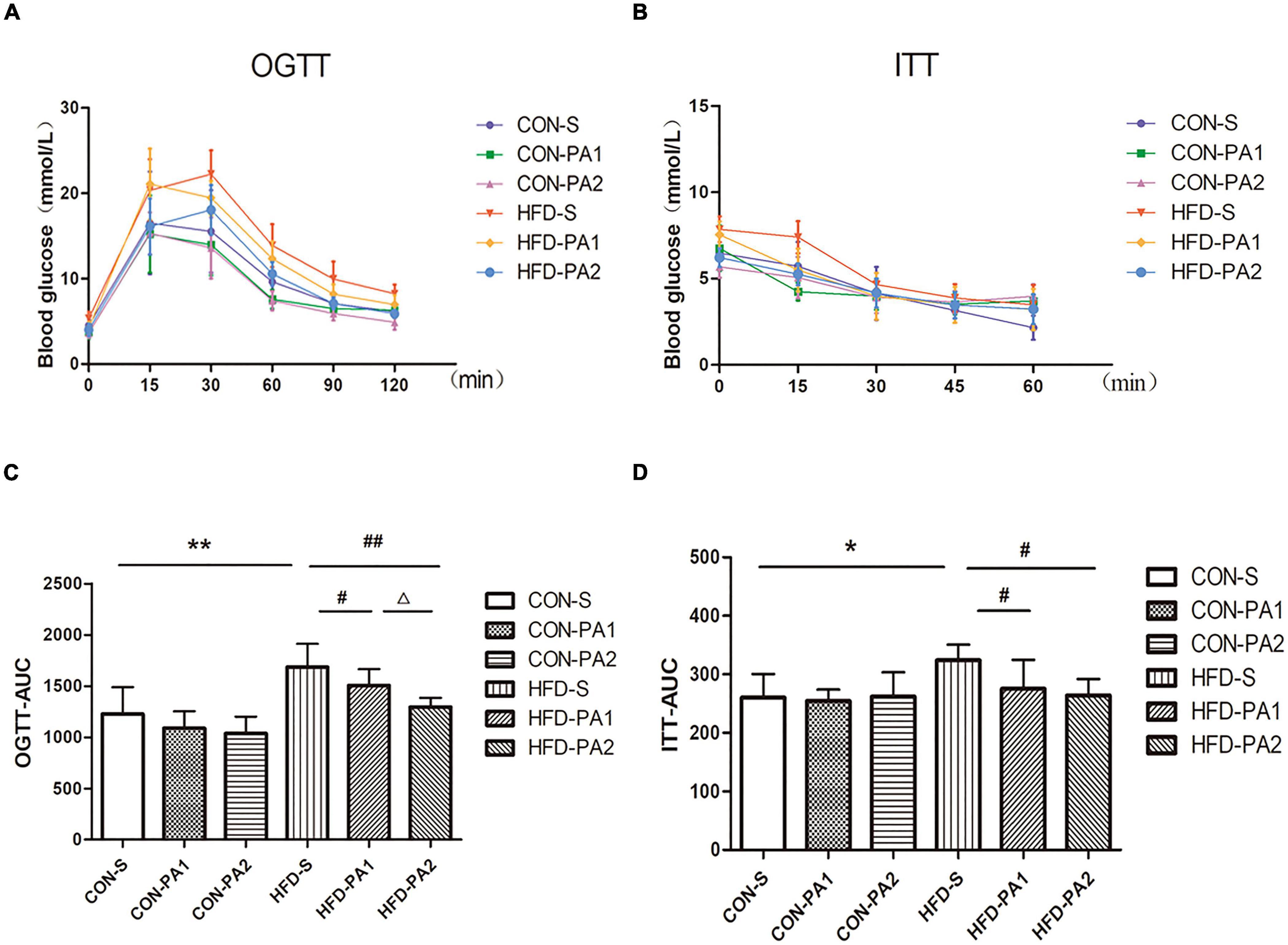
Figure 3. Glucose change curves of OGTT and ITT. (A,C) OGTT in mice after fasting for 12 h. Histograms represent the areas under the glucose curve. (B,D) ITT in mice after fasting for 2 h. Histograms represent the areas under the glucose curve. Compared with the CON-S group, *P < 0.05 and **P < 0.01; compared with the HFD-S group, #P < 0.05 and ##P < 0.01; comparison between exercise groups (CON-PA1 vs. CON-PA2 and HFD-PA1 vs. HFD-PA2), ΔP < 0.05 and ΔΔP < 0.01.
Exercise Reduced Serum Exosomal miR-27a Level in Obese Growing Mice and the Prediction Target Gene
Exosomes contain a large number of miRNAs. It has been reported that exercise leads to changes in the expression of miR-27a in vivo (20). Exosomes were extracted from mouse serum by using kits, and the marker proteins (CD9 and CD63) were detected by Western blot experiments (Figures 4A,B). We also observed that the expression of exosomal miR-27a in obese mice was increased significantly, while that was reduced significantly in exercise mice with HFD (Figure 4C). We predicted PPAR-γ as a potential target downstream of miR-27a by using TargetScan (Figure 4D). Luciferase reports have verified that the reported fluorescence of M PPARG MUT increased significantly compared with M PPAR-γ WT after co-transformation with MMU miR-27a-3p. These results suggest that MMU miR-27a-3p is likely to interact significantly with this site on the predicted M PPARG 3′UTR (Figures 4E,F).
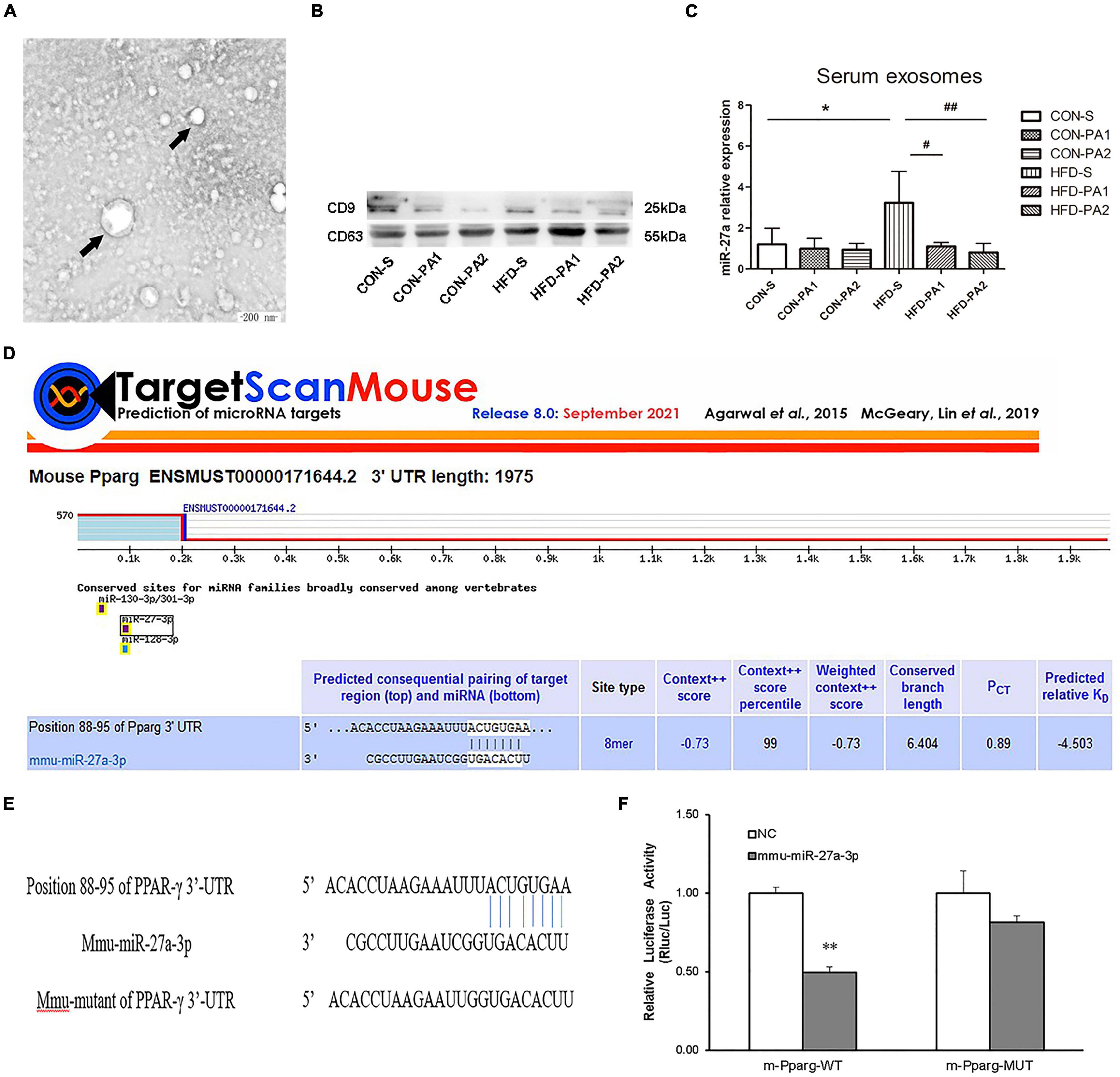
Figure 4. Serum exosome miR-27a level and target gene prediction. (A) TEM demonstrated that exosomes secreted by mice were round vesicles (bars = 200 nm). (B) Western blot detected the protein expressions of CD9 and CD63 in exosomes. (C) Expressions of exosomes miR-27a were measured by qRT-PCR. (D) Prediction of the target gene of miR-27a. (E) Schematic of the miR-27abinding site to PPAR-γ 3′-UTR. (F) Luciferase reporter assay indicated the binding of miR-27a with PPAR-γ. Compared with the CON-S group, *P < 0.05 and **P < 0.01; compared with the HFD-S group, #P < 0.05 and ##P < 0.01; comparison between exercise groups (CON-PA1 vs. CON-PA2, HFD-PA1 vs. HFD-PA2).
Exercise Stimulated White Adipose Tissue Browning
The browning of adipocytes plays a positive role in improving obesity, and subcutaneous WAT is the sensitive site. Results showed that obese mice’s expression of UCP1 was significantly reduced in the HFD-S group compared with the CON-S group. Exercise could upregulate the expression of UCP1, and the comparison between HFD-PA2 and HFD-S was significant, as well as CON-PA2 versus CON-S.
To investigate the effect of exercise on the browning of WAT, the expression levels of adipose tissue brown-related proteins and genes were also examined (21). We found that the expression levels of three main transcriptional regulators of classical brown adipose tissue (BAT) development, namely, PPAR-γ, PR domain containing 16 (PRDM16), and peroxisome proliferator-activated receptor γ coactivator 1α (PGC-1α), were upregulated in the exercise mice, regardless consuming a basal diet or HFD (Figure 5). Meanwhile, we observed a small number of cells similar to brown adipocytes in subcutaneous WAT of mice in the CON-PA2 group. These cells were small in size, their nucleus was located in the center, and there were many small fat droplets around them (Figures 2I–III).
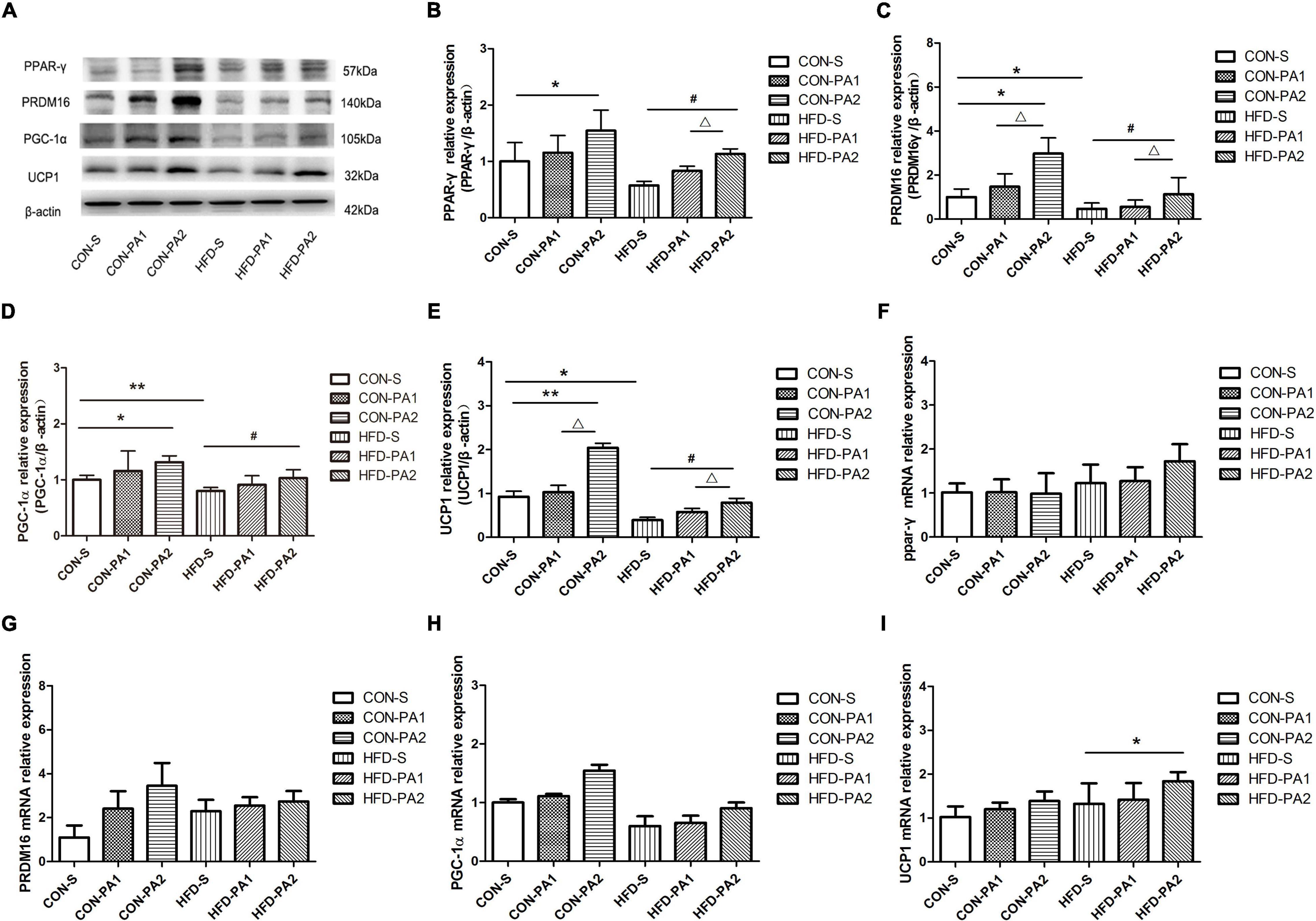
Figure 5. Expression levels of browning-related proteins and genes in mice WAT. (A–E) Western blot analysis of protein PPAR-γ, PRDM16, PGC-1α, and UCP1 in subcutaneous adipose tissues. (F–I) Relative expression analysis results of PRDM16, PPAR-γ, PGC-1α, and UCP1 genes, respectively. Compared with the CON-S group, *P < 0.05 and **P < 0.01; compared with the HFD-S group, #P < 0.05 and ##P < 0.01; comparison between exercise groups (CON-PA1 vs. CON-PA2, HFD-PA1 vs. HFD-PA2), ΔP < 0.05 and ΔΔP < 0.01.
Exercise Activated IRS-1/Akt/GLUT-4 Signaling Pathway in Skeletal Muscle of Obese Growing Mice
Skeletal muscle insulin resistance is mainly characterized by impaired insulin-dependent glucose uptake. In this research, WB and qPCR experiments were carried out to evaluate the expressions of PPAR-γ and key molecules for insulin-dependent glucose uptake. We found that expressions of PPAR-γ and glucose transporter type 4 (GLUT-4) were decreased in the HFD-S group compared with the CON-S group. However, their expressions were upregulated by exercise in the HFD-PA2 group compared with the HFD-S group. Key molecules and their phosphorylation in the insulin signaling pathway were measured. The phosphorylation of insulin receptor substrate-1 (IRS-1) on Ser307 was enhanced, and phosphorylation of protein kinase B (AKT) was inhibited in the HFD-S group compared with the CON-S group. However, swimming exercise significantly decreased the expression of p-IRS-1 and increased the expression of p-AKT in the HFD-PA2 group (Figure 6A–K).
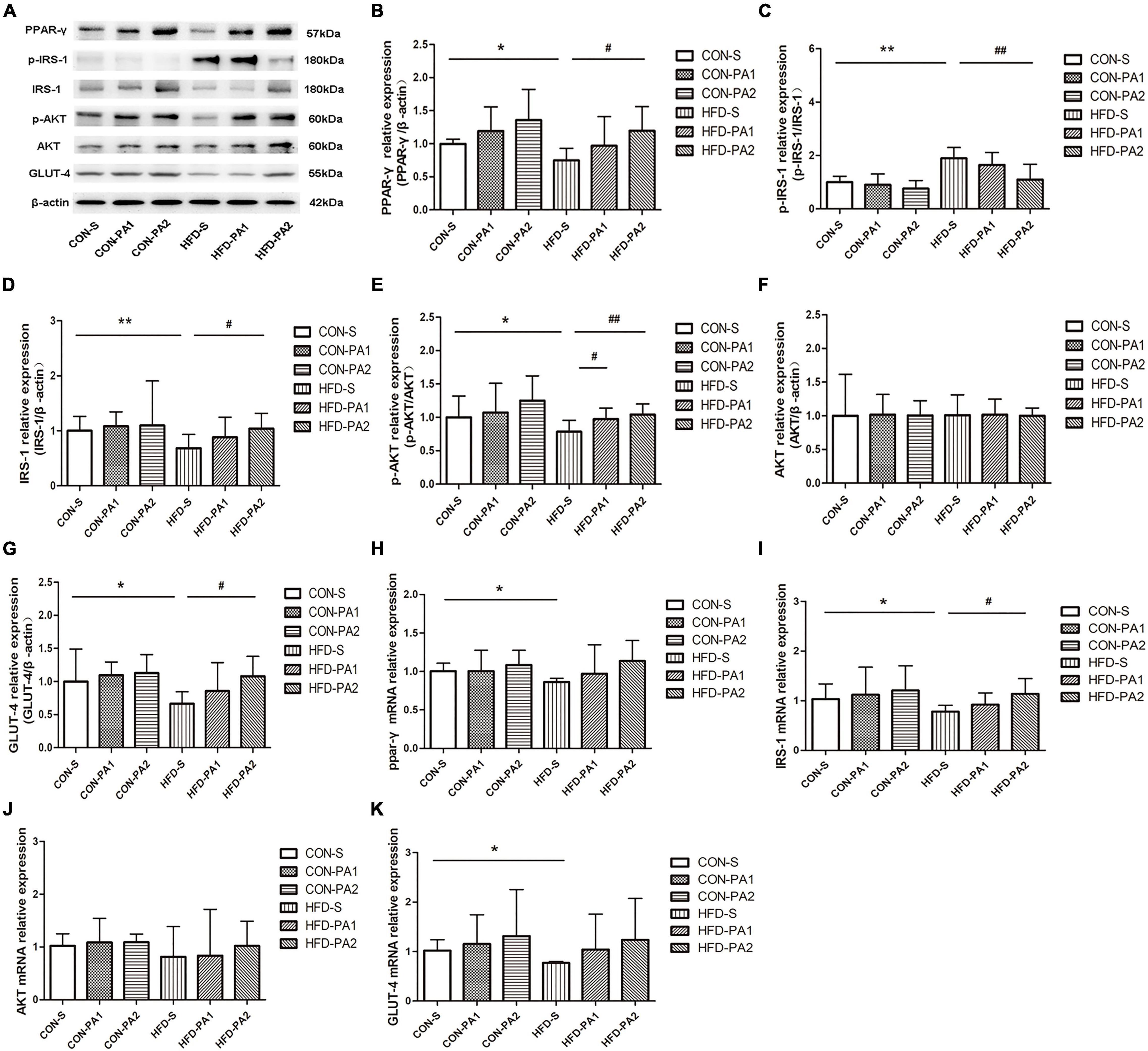
Figure 6. Expressions of glucose uptake-related proteins in skeletal muscles of mice. (A–G) Western blot analysis of proteins PPAR-γ, p-IRS-1, IRS-1, p-AKT, AKT, and GLUT-4 in skeletal muscles of mice. Compared with the CON-S group, *P < 0.05 and **P < 0.01; compared with the HFD-S group, #P < 0.05 and ##P < 0.01; comparison between exercise groups (CON-PA1 vs. CON-PA2, HFD-PA1 vs. HFD-PA2).
Discussion
In recent years, the incidence of overweight and obesity among children and adolescents has increased rapidly, which has become a prominent public health problem in the world (1). Overnutrition and insufficient exercise are the primary causes. Exercise intervention has been proved to be an effective way to control weight and treat childhood obesity (22). Swimming is often used in investigating the effect of exercise on adipose tissue and skeletal muscles (23, 24). In the present study, juvenile mice developed obese manifestation when fed with a high-fat diet continuously for 10 weeks, which stimulated the development process of childhood obesity. In PA1 and PA2 subgroups in each dietary treatment, the mice performed non-weight-bearing swimming training, which were applied to observe the different responses induced by exercise.
All mice completed the exercise programs without difficulty. Exercise hindered diet-induced obesity occurring in the development stage. In terms of physical outcomes, the HFD-S mice gained more body weight after 10 weeks of feeding than those in the CON-S group. Likewise, fat weight, body fat ratio, and epididymal and perirenal fat coefficients were significantly increased in the HFD-S group. Through the swimming training intervention, the fat mass of the exercising mice in the HFD-PA1 and HFD-PA2 groups was significantly less than that of non-exercising ones in the HFD-S group. Interestingly, the effects of exercise on body fat and lean body were not obvious in mice fed with a basal diet, and a similar phenomenon was also observed in a previous 10 week treadmill study in mice (25). Examining the mice in non-exercise groups (CON-S and HFD-S), the lean body mass of obese mice was smaller than that of the normal control; the consequence was probably due to fast growing of body fat induced by the HFD in young animals. Apart from this, the difference of lean body mass was low in each dietary group. These results indicated that exercise tended to favor regulation of body fat on diet-induced obesity.
Exercise-induced adaptive responses can be seen from cellular morphological changes. The size of the adipocyte volume is related to the intracellular lipid droplets. Adipocyte hypertrophy was observed in obese animals, which could be ameliorated by exercise, a similar effect also occurred in those mice on the basal diet. Bae JY et al. have substantiated that the catabolic rate of mice is higher than the anabolic rate after exercise, and lipid droplets are decomposed, thereby the volume of adipocytes is reduced (26). Exercise could also regulate skeletal muscle plasticity. The cross-sectional areas of muscle cells, especially in the 2 h exercise groups, were significantly larger than those in quiet groups either on the basal diet or HFD. Skeletal muscles are the material basis of body performance and physical activity, which can change its physical properties and composition in response to physiological demands. The influence of obesity on the intrinsic force-producing capacity of skeletal muscles is not completely resolved yet (27). Although contractile performance and constituent fibers of skeletal muscles were not measured in this study, the change in muscle fibers had been presented, which reflected high adaptable ability of skeletal muscles. Our results confirmed that for impeding the pandemic of childhood obesity, the duration of exercise in each session is crucial to mediate a certain beneficial effect on the skeletal muscle cell structure. In addition, interventions must be multifaceted, and we cannot emphasize the importance of diet, physical exercise, and developing healthy living habits in children too much at any time.
Obesity is a chronic metabolic disease which links to multiple factors and results from long-term imbalance between energy intake and consumption (3). Exercise can not only increase energy consumption and fat oxidation but also affect hormones that regulate appetite. As a result, energy intake is limited, and weight is controlled consequently (28). Although the hormones associated with regulating appetite were not included in measuring parameters in the present study, it was observed that food intake of exercising mice was less than the quiet ones, especially in the case of HFD feeding. The appetite of the mice under swimming training was influenced notably, which supported the opinion that higher habitual physical activity levels may better adjust body weight and body composition not only by increasing energy expenditure but also by reducing food intake (28, 29).
As is well known, long-term HFD feeding can lead to interference of glucolipid metabolism and increase the risk of various metabolic diseases. A large number of literature studies have been well documented that aerobic exercise can ameliorate obesity, modify the lipid profile, and improve insulin sensitivity (30–32). Our results showed that the serum levels of TCHO, TG, and LDL-C in the HFD-diet quiet group were significantly higher than those in the basal-diet quiet group, which indicated that the HFD caused lipid metabolism disorder in obese mice during the development stage. The influence of exercise on the lipid profile was more noticeable in the mice swimming longer, which happened both in obese bodies and non-obese bodies. Recently, researchers also observed significant reduction of LDL, HDL, and TG in rats which underwent continuous swimming training (33). Similar results have been obtained in patients with dyslipidemia, and serum LDL-C, intermediate-density lipoprotein, and very low-density lipoprotein levels were significantly reduced after 16 weeks of aerobic exercise (34). Combined with the results of adipose tissue morphological sections, we are convinced that a HFD may more easily cause body fat accumulation and hyperlipidemia, and non-weight-bearing swimming for 10 weeks can effectively improve dyslipidemia and facilitate weight control.
The high-fat diet and lack of exercise are considered as risk factors for insulin resistance (35), which is usually associated with impaired glucose metabolism (36). In this study, FPG, FINS, and HOMA-IR indexes were used to determine whether insulin resistance occurred in the HFD-fed mice, and the OGTT and ITT were used for further verification. The levels of FPG, FINS, HMOA-IR index, and fasting OGTT and ITT were significantly higher in the HFD-S group than in the CON-S group, which indicated that system insulin resistance had occurred in the non-exercise HFD-S group. Excitedly, swimming training altered the glucose tolerance test and improved insulin sensitivity in the 1 h and 2 h exercise HFD-S groups. Similar results were also obtained in several previous studies (37–39). Insulin resistance induced by the HFD is mainly related to oxidation of the glucose–fatty acid cycle (40, 41). Sandu O et al. demonstrated that fatty diets contain pro-oxidation and pro-inflammatory compounds that are associated with impaired insulin sensitivity (42). To the best of our knowledge, the mechanism of development of insulin resistance is unclear; it has been generally accepted that the HFD is responsible for insulin resistance, decreased insulin-stimulated glycolysis, and glycogen synthesis (40–43). The induction of insulin resistance in skeletal muscles is a key phenomenon, and impairments in insulin signaling in this tissue directly contribute to hyperglycemia, which has been confirmed by the following results of insulin signaling pathway experiment. Although the benefits of exercise for insulin resistance have not been clarified whether it is the exercise itself or the weight loss that has resulted from the exercise (5), our research presents a promising picture for the benefits of exercise in ameliorating metabolic disorders involved in HFD-induced obesity in the developing stage.
As the largest energy reservoir in the body, adipose tissues govern the physical function of energy metabolism and exert a key role in maintaining energy balance (44). There are three distinct types of adipose tissue in rodents and humans: BAT, WAT, and beige adipose tissue. Each adipose tissue or adipocyte type has distinct morphological features and physiological functions (45). In rodents, brown adipocytes could be induced by a variety of stimuli, including cold exposure, drug, and exercise (4, 46, 47). In response to these stimuli, WAT browns and energy expenditure is promoted. Therefore, converting WAT to BAT, and activating and expanding BAT are the most important targets for combating obesity and metabolic diseases.
Researchers have examined the role of exercise-induced adaptations to adipose tissue, and an important change in rodents is the increase in “beiging,” which occurs in the subcutaneous depot (23). It has been reported that exercise caused subcutaneous white adipocytes of HFD-induced obese animals to display beige adipocyte characteristics, which included abundant multilocular adipocytes and upregulation of the beige fat-specific gene UCP1, whereas visceral fat appeared to be resistant to stimulation inducing the browning switch (48, 49). Xu X et al. found that the expression of the beige fat-specific gene UCP1 in WAT was upregulated after 8 weeks of treadmill exercise in the mice, regardless of whether they were fed with a HFD or basal diet (50). Another experiment showed that UCP1 and PRDM16 were significantly upregulated in subcutaneous WAT of adult mice after 11 days of autonomous wheel running training with a basal diet. Several studies also reported brown fat-like development in swimming training mice, and the expression of browning markers in WAT depots, such as UCP1 and PGC-1α, was significantly increased (51–53). In the current study, the juvenile mice performed non-weight-bearing swimming exercise for 10 weeks. As previous studies reported, subcutaneous WAT is sensitive to exercise stimulation, and we observed browning-like cells emerged in H&E-stained sections of the CON-PA2 group. Although a cellular morphology change was seen only in the basal diet-fed mice, the biomarker of brown adipocytes, namely, UCP1, and adipose tissue brown-related proteins and genes were upregulated both in the HFD-PA2 and CON-PA2 groups. Our results indicated that adaption to exercise of HFD-induced obese WAT lagged behind normal WAT. In addition, the morphological structure and molecular alteration occurred in 2 h exercise groups, which suggested exercise duration was essential for effective physical response. The exercise intervention was delivered for 10 weeks in the current experiment. It does not rule out obvious physical adaption to exercise occurring in the 1 h exercise session in some cases, such as a prolonged exercise period and appropriate increase in exercise intension.
Circulating miRNA levels might be a promising biomarker for monitoring the impact of lifestyle or dietary interventions (8). Extracellular miRNAs are enclosed in vesicles. Exosomes are one of the main forms; they are highly sensitive to exercise stress, which enter the circulation or tissues faster than adrenaline and other hormones to maintain the homeostasis of the intracellular environment and participate in the regulation of various physiological functions, such as body metabolism (54). Since exercise can trigger alteration of the exosomal profile in the circulation, a possible mechanism for the systemic benefits of exercise has been propagated (55). It was reported that miR-27a was highly expressed in sera of obese individuals with prediabetes and T2DM (56). Another research found that serum levels of miR-27a were positively associated with obesity and insulin resistance in children and mice with obesity, and the researcher speculated that miR-27a might be another modulator of obesity-associated insulin resistance (14). However, exercise-induced changes in circulatory miR-27a have received little attention. In this study, we found a significantly high expression level of serum exosomal miR-27a in obese mice, and swimming exercise decreased the expression level in the HFD-fed mice. Biological information technology was used to predict the target genes of miR-27a, and the results showed that the 3′-untranslated region of PPAR-γ was the direct target of miR-27a. At the same time, real-time PCR and Western blot were used for verification. In agreement with Chen T et al.’s study (57), our results suggested that PPAR-γ could be negatively regulated by miR-27a in vivo.
For confirming the findings of morphological changes of subcutaneous WAT, we detected three main transcriptional regulators of classical BAT development. PPAR-γ is the obligatory transcription factor indispensable for the differentiation and survival of both white and brown adipocytes (58). During adipose formation, PPAR-γ can induce the expression of UCP1, a hallmark of brown fat-like phenotype (59). In both mouse and human WATs, it has been confirmed that treatment with PPAR-γ activator rosiglitazone could induce the expression of UCP1 (58, 60). PRDM16 was first discovered and reported as a zinc finger protein that is especially expressed in BAT, which is required and sufficient to promote brown adipogenesis in WAT (61, 62). PGC-1α is named after its role as a coactivator of PPAR-γ, needed for BAT thermogenesis but not differentiation (63). In this study, we found that the protein expression levels of PPAR-γ, PRDM16, and UCP1 in subcutaneous WAT of mice in the 2 h exercise group were significantly higher than those in the quiet group, regardless of the dietary treatment. These results suggest that 2 h swimming exercise can decrease the expression of circulating exosome miR-27a connected with HFD-induced obesity and promote the browning of WAT in growing mice.
Occupying approximately 40% of the total body mass, skeletal muscles account for more than 70% of the whole body glucose disposal (64). Obesity plays a key role in the onset and progression of insulin resistance, which is a reversible condition but if untreated, leads to type 2 diabetes alongside. Insulin binds to insulin receptors and initiates the intracellular cascade events. The IRS-1/Akt/GLUT-4 pathway is the most investigated mechanism involved in disease development. In insulin signaling, IRS-1 is the most important insulin receptor substrate protein isoform in muscles. The phosphorylation of IRS-1 on tyrosine residues is required for insulin-stimulated responses, but increased phosphorylation of specific serine residues can render IRS-1 inactive (65). Then, downstream factor AKT is phosphorylated and activated through phosphatidylinositol 3-kinase (PI3K). Phosphorylation of IRS-1 and AKT affects glucose uptake (66). GLUT-4, a member of the GLUT family, maintains glucose balance by moving to the plasma membrane and promoting the uptake and transport of glucose into the cells on the membrane when regulated by the IRS-1/PI3K/AKT signaling pathway (67). Muscle insulin resistance is due to disturbed phosphorylation and glucose transport (68). It has been confirmed that PPAR-γ is a transcriptional regulator of IRS-1 and GLUT-4 (69). Skeletal muscles that are insulin resistant do not respond to insulin, which causes impairment in the insulin signaling pathway and, as a result, a reduction in GLUT-4 levels (70). Therefore, we studied whether exercise activates the insulin signaling pathway, upregulates GLUT-4 expression, and eventually improves skeletal muscle insulin resistance. Compared with the CON-S group, we found that the expression of PPAR-γ, p-AKT, and GLUT-4 was significantly downregulated; p-IRS-1 (Ser307) was significantly upregulated, which indicated that the IRS-1/Akt/GLUT-4 signaling pathway was diminished in obese growing mice induced by the HFD. However, 2 h exercise modulated the expression of transcriptional regulators PPAR-γ and GLUT-4, and phosphorylation of key signaling molecules of the insulin effect. Our results showed that exercise plays an improved role in the phosphorylation of IRS1 and AKT and leads to the modulation of GLUT-4 expression that would increase insulin-dependent glucose uptake and storage in skeletal muscles.
Conclusion
In summary, juvenile mice developed obesity manifestation when fed with a HFD continuously, which is helpful to simulate the development process of childhood obesity. We demonstrated that the HFD more easily caused body fat accumulation. Non-weight-bearing swimming exercise for 10 weeks could effectively facilitate weight control and tend to favor the regulation of body fat on diet-induced obesity. Concerning the morphological observation of adipocytes and skeletal muscle cells, the changes of HFD-induced obese mice were consist with the normal control. Exercise modified glucolipid metabolism parameters differently in the obese/non-obese groups, and the changes in the 2 h exercise mice were not consistent, as well as 1 h exercise mice. The expression level of serum exosomal miR-27a was significantly high in obese mice, and swimming exercise decreased the expression level in the HFD-fed mice. Bioinformatics analysis and dual-luciferase reporter assay showed that miR-27a targeted PPAR-γ. Although UCP1 and adipose tissue brown-related proteins and genes were upregulated both in the HFD-PA2 and CON-PA2 mice, browning-like cells were seen only in H&E-stained sections of the CON-PA2 mice. Exercise activated the IRS-1/Akt/GLUT-4 signaling pathway in skeletal muscles of the HFD-PA2 group. Taken together, our findings implicated that exercise-induced beneficial effects are associated with the exercise duration, and the response of obese and non-obese bodies is different. Exosomal miR-27a might be a crucial node for the process of exercise-induced browning of WAT and improving skeletal muscle insulin sensitivity. However, future in vitro studies are required to validate the effects of miR-27a on adipocytes and skeletal muscle cells.
Data Availability Statement
The original contributions presented in the study are included in the article/supplementary material, further inquiries can be directed to the corresponding author.
Ethics Statement
The animal study was reviewed and approved by the Laboratory Animal Welfare and Ethics Committee of China Medical University.
Author Contributions
DW and XZ performed the majority of the experiments and wrote the manuscript. YL drafted the part of the manuscript. LJ, LZ, and HJ contributed to the study design and technological support. LZ and WW helped with the data analysis. YB designed and supervised the study and checked the final manuscript. All authors contributed to the manuscript and approved the submitted version.
Funding
This work was supported by the grants from the Educational Department of Liaoning Province of China (No. JC2019036) and National Natural Science Foundation of China (Grant No. 81300130).
Conflict of Interest
The authors declare that the research was conducted in the absence of any commercial or financial relationships that could be construed as a potential conflict of interest.
Publisher’s Note
All claims expressed in this article are solely those of the authors and do not necessarily represent those of their affiliated organizations, or those of the publisher, the editors and the reviewers. Any product that may be evaluated in this article, or claim that may be made by its manufacturer, is not guaranteed or endorsed by the publisher.
Acknowledgments
We were grateful to Hongjuan Li (Beijing Sport University) for her technical support in the animal exercise protocol.
References
1. Kumar S, Kelly AS. Review of childhood obesity: from epidemiology, etiology, and comorbidities to clinical assessment and treatment. Mayo Clin Proc. (2017) 92:251–65. doi: 10.1016/j.mayocp.2016.09.017
2. The Lancet Diabetes Endocrinology. Childhood obesity: a growing pandemic. Lancet Diab Endocrinol. (2022) 10:1. doi: 10.1016/S2213-8587(21)00314-4
3. Scudiero O, Pero R, Ranieri A, Terracciano D, Fimiani F, Cesaro A, et al. Childhood obesity: an overview of laboratory medicine, exercise and microbiome. Clin Chem Lab Med. (2020) 58:1385–406. doi: 10.1515/cclm-2019-0789
4. Aldiss P, Betts J, Sale C, Pope M, Budge H, Symonds ME. Exercise-induced ‘browning’ of adipose tissues. Metab Clin Exp. (2018) 81:63–70. doi: 10.1016/j.metabol.2017.11.009
5. Whillier S. Exercise and insulin resistance. Adv Exp Med Biol. (2020) 1228:137–50. doi: 10.1007/978-981-15-1792-1_9
6. Mu WJ, Zhu JY, Chen M, Guo L. Exercise-mediated browning of white adipose tissue: its significance, mechanism and effectiveness. Int J Mol Sci. (2021) 22:11512. doi: 10.3390/ijms222111512
8. Rome S. Use of miRNAs in biofluids as biomarkers in dietary and lifestyle intervention studies. Genes Nutr. (2015) 10:483. doi: 10.1007/s12263-015-0483-1
9. Di W, Amdanee N, Zhang W, Zhou Y. Long-term exercise-secreted extracellular vesicles promote browning of white adipocytes by suppressing miR-191a-5p. Life Sci. (2020) 263:118464. doi: 10.1016/j.lfs.2020.118464
10. Karbiener M, Scheideler M. MicroRNA functions in brite/brown fat - novel perspectives towards anti-obesity strategies. Comp Struct Biotechnol J. (2014) 11:101–5. doi: 10.1016/j.csbj.2014.09.005
11. Kim SY, Kim AY, Lee HW, Son YH, Lee GY, Lee JW, et al. miR-27a is a negative regulator of adipocyte differentiation via suppressing PPARgamma expression. Biochem Biophys Res Commun. (2010) 392:323–8. doi: 10.1016/j.bbrc.2010.01.012
12. Sun L, Trajkovski M. MiR-27 orchestrates the transcriptional regulation of brown adipogenesis. Metab Clin Exp. (2014) 63:272–82. doi: 10.1016/j.metabol.2013.10.004
13. Kim Y, Kim OK. Potential roles of adipocyte extracellular vesicle-derived miRNAs in obesity-mediated insulin resistance. Adv Nutr. (2021) 12:566–74. doi: 10.1093/advances/nmaa105
14. Yu Y, Du H, Wei S, Feng L, Li J, Yao F, et al. Adipocyte-derived exosomal MiR-27a induces insulin resistance in skeletal muscle through repression of PPARγ. Theranostics. (2018) 8:2171–88. doi: 10.7150/thno.22565
15. Deshmukh AS. Insulin-stimulated glucose uptake in healthy and insulin-resistant skeletal muscle. Hormone Mol Biol Clin Invest. (2016) 26:13–24. doi: 10.1515/hmbci-2015-0041
16. Petersen KF, Dufour S, Savage DB, Bilz S, Solomon G, Yonemitsu S, et al. The role of skeletal muscle insulin resistance in the pathogenesis of the metabolic syndrome. Proc Natl Acad Sci USA. (2007) 104:12587–94. doi: 10.1073/pnas.0705408104
17. Bei Y, Xu T, Lv D, Yu P, Xu J, Che L, et al. Exercise-induced circulating extracellular vesicles protect against cardiac ischemia-reperfusion injury. Basic Res Cardiol. (2017) 112:38. doi: 10.1007/s00395-017-0628-z
18. Martinez-Huenchullan SF, Maharjan BR, Williams PF, Tam CS, McLennan SV, Twigg SM. Differential metabolic effects of constant moderate versus high intensity interval training in high-fat fed mice: possible role of muscle adiponectin. Physiol Rep. (2018) 6:e13599. doi: 10.14814/phy2.13599
19. Broadfield LA, Duarte JAG, Schmieder R, Broekaert D, Veys K, Planque M, et al. Fat induces glucose metabolism in nontransformed liver cells and promotes liver tumorigenesis. Cancer Res. (2021) 81:1988–2001. doi: 10.1158/0008-5472.CAN-20-1954
20. Fernandes T, Baraúna VG, Negrão CE, Phillips MI, Oliveira EM. Aerobic exercise training promotes physiological cardiac remodeling involving a set of microRNAs. Am J Physiol Heart Circ Physiol. (2015) 309:H543–52. doi: 10.1152/ajpheart.00899.2014
21. Zhang J, Wu H, Ma S, Jing F, Yu C, Gao L, et al. Transcription regulators and hormones involved in the development of brown fat and white fat browning: transcriptional and hormonal control of brown/beige fat development. Physiol Res. (2018) 67:347–62. doi: 10.33549/physiolres.933650
22. Hyman SM. Integration of movement competency training to optimize behavioral interventions for child obesity: Comment on Wilfley et al. (2018). Am Psychol. (2019) 74:617–9. doi: 10.1037/amp0000459
23. Lehnig AC, Stanford KI. Exercise-induced adaptations to white and brown adipose tissue. J Exp Biol. (2018) 221(Pt Suppl. 1):jeb161570. doi: 10.1016/j.isci.2018.12.033
24. Touron J, Costes F, Coudeyre E, Perrault H, Richard R. Aerobic metabolic adaptations in endurance eccentric exercise and training: from whole body to mitochondria. Front Physiol. (2020) 11:596351. doi: 10.3389/fphys.2020.596351
25. Martinez-Huenchullan SF, Ban LA, Olaya-Agudo LF, Maharjan BR, Williams PF, Tam CS, et al. Constant-moderate and high-intensity interval training have differential benefits on insulin sensitive tissues in high-fat fed mice. Front Physiol. (2019) 10:459. doi: 10.3389/fphys.2019.00459
26. Bae JY, Woo J, Roh HT, Lee YH, Ko K, Kang S, et al. The effects of detraining and training on adipose tissue lipid droplet in obese mice after chronic high-fat diet. Lipids Health Dis. (2017) 16:13. doi: 10.1186/s12944-016-0398-x
27. Tallis J, James RS, Seebacher F. The effects of obesity on skeletal muscle contractile function. J Exp Biol. (2018) 221(Pt 13):jeb163840. doi: 10.1242/jeb.163840
28. Dorling J, Broom DR, Burns SF, Clayton DJ, Deighton K, James LJ, et al. Acute and chronic effects of exercise on appetite, energy intake, and appetite-related hormones: the modulating effect of adiposity, sex, and habitual physical activity. Nutrients. (2018) 10:1140. doi: 10.3390/nu10091140
29. Zhang N, Bi S. Effects of physical exercise on food intake and body weight: Role of dorsomedial hypothalamic signaling. Physiol Behav. (2018) 192:59–63. doi: 10.1016/j.physbeh.2018.03.018
30. Fiuza-Luces C, Garatachea N, Berger NA, Lucia A. Exercise is the real polypill. Physiology (Bethesda, Md). (2013) 28:330–58. doi: 10.1152/physiol.00019.2013
31. Celik O, Yildiz BO. Obesity and physical exercise. Minerva Endocrinol. (2021) 46:131–44. doi: 10.23736/S2724-6507.20.03361-1
32. Monteiro PA, Chen KY, Lira FS, Saraiva BT, Antunes BM, Campos EZ, et al. Concurrent and aerobic exercise training promote similar benefits in body composition and metabolic profiles in obese adolescents. Lipids Health Dis. (2015) 14:153. doi: 10.1186/s12944-015-0152-9
33. Heiat F, Heiat M, Shojaeifard M. Changes in mitochondrial biogenesis and fatty liver indicators in rat following continuous and high intensity interval training. J Sports Med Phys Fitness. (2021) 61:1416–22. doi: 10.23736/S0022-4707.20.11693-1
34. Yoshida H, Ishikawa T, Suto M, Kurosawa H, Hirowatari Y, Ito K, et al. Effects of supervised aerobic exercise training on serum adiponectin and parameters of lipid and glucose metabolism in subjects with moderate dyslipidemia. J Atheroscler Thromb. (2010) 17:1160–6. doi: 10.5551/jat.4358
35. Manu P, Tsang J, Napolitano BA, Lesser ML, Correll CU. Predictors of insulin resistance in the obese with metabolic syndrome. Eur J Int Med. (2010) 21:409–13. doi: 10.1016/j.ejim.2010.05.015
36. Ferrannini E, Balkau B, Coppack SW, Dekker JM, Mari A, Nolan J, et al. Insulin resistance, insulin response, and obesity as indicators of metabolic risk. J Clin Endocrinol Metab. (2007) 92:2885–92. doi: 10.1210/jc.2007-0334
37. Medeiros C, Frederico MJ, da Luz G, Pauli JR, Silva AS, Pinho RA, et al. Exercise training reduces insulin resistance and upregulates the mTOR/p70S6k pathway in cardiac muscle of diet-induced obesity rats. J Cell Physiol. (2011) 226:666–74. doi: 10.1002/jcp.22387
38. Maiorana A, O’Driscoll G, Goodman C, Taylor R, Green D. Combined aerobic and resistance exercise improves glycemic control and fitness in type 2 diabetes. Diab Res Clin Pract. (2002) 56:115–23. doi: 10.1016/s0168-8227(01)00368-0
39. Turcotte LP, Fisher JS. Skeletal muscle insulin resistance: roles of fatty acid metabolism and exercise. Phys Ther. (2008) 88:1279–96. doi: 10.2522/ptj.20080018
40. Kim CH, Youn JH, Park JY, Hong SK, Park KS, Park SW, et al. Effects of high-fat diet and exercise training on intracellular glucose metabolism in rats. Am J Physiol Endocrinol Metab. (2000) 278:E977–84. doi: 10.1152/ajpendo.2000.278.6.E977
41. Ravussin E, Smith SR. Increased fat intake, impaired fat oxidation, and failure of fat cell proliferation result in ectopic fat storage, insulin resistance, and type 2 diabetes mellitus. Ann N Y Acad Sci. (2002) 967:363–78. doi: 10.1111/j.1749-6632.2002.tb04292.x
42. Sandu O, Song K, Cai W, Zheng F, Uribarri J, Vlassara H. Insulin resistance and type 2 diabetes in high-fat-fed mice are linked to high glycotoxin intake. Diabetes. (2005) 54:2314–9. doi: 10.2337/diabetes.54.8.2314
43. Parillo M, Riccardi G. Diet composition and the risk of type 2 diabetes: epidemiological and clinical evidence. Br J Nutr. (2004) 92:7–19. doi: 10.1079/BJN20041117
44. Booth A, Magnuson A, Fouts J, Foster MT. Adipose tissue: an endocrine organ playing a role in metabolic regulation. Hormone Mol Biol Clin Invest. (2016) 26:25–42. doi: 10.1515/hmbci-2015-0073
45. Stanford KI, Goodyear LJ. Exercise regulation of adipose tissue. Adipocyte. (2016) 5:153–62. doi: 10.1080/21623945.2016.1191307
46. Xu Z, You W, Zhou Y, Chen W, Wang Y, Shan T. Cold-induced lipid dynamics and transcriptional programs in white adipose tissue. BMC Biol. (2019) 17:74. doi: 10.1186/s12915-019-0693-x
47. Kim OY, Chung JY, Song J. Effect of resveratrol on adipokines and myokines involved in fat browning: perspectives in healthy weight against obesity. Pharmacol Res. (2019) 148:104411. doi: 10.1016/j.phrs.2019.104411
48. Khalafi M, Mohebbi H, Symonds ME, Karimi P, Akbari A, Tabari E, et al. The impact of moderate-intensity continuous or high-intensity interval training on adipogenesis and browning of subcutaneous adipose tissue in obese male rats. Nutrients. (2020) 12:925. doi: 10.3390/nu12040925
49. Wang N, Liu Y, Ma Y, Wen D. High-intensity interval versus moderate-intensity continuous training: Superior metabolic benefits in diet-induced obesity mice. Life Sci. (2017) 191:122–31. doi: 10.1016/j.lfs.2017.08.023
50. Xu X, Ying Z, Cai M, Xu Z, Li Y, Jiang SY, et al. Exercise ameliorates high-fat diet-induced metabolic and vascular dysfunction, and increases adipocyte progenitor cell population in brown adipose tissue. Am J Physiol Regul Int Comp Physiol. (2011) 300:R1115–25. doi: 10.1152/ajpregu.00806.2010
51. Dewal RS, Stanford KI. Effects of exercise on brown and beige adipocytes. Biochim Biophys Mol Cell Biol Lipids. (2019) 1864:71–8. doi: 10.1016/j.bbalip.2018.04.013
52. Boström P, Wu J, Jedrychowski MP, Korde A, Ye L, Lo JC, et al. A PGC1-α-dependent myokine that drives brown-fat-like development of white fat and thermogenesis. Nature. (2012) 481:463–8. doi: 10.1038/nature10777
53. Sutherland LN, Bomhof MR, Capozzi LC, Basaraba SA, Wright DC. Exercise and adrenaline increase PGC-1{alpha} mRNA expression in rat adipose tissue. J Physiol. (2009) 587(Pt 7):1607–17. doi: 10.1113/jphysiol.2008.165464
54. Frühbeis C, Helmig S, Tug S, Simon P, Krämer-Albers EM. Physical exercise induces rapid release of small extracellular vesicles into the circulation. J Extracell Vesicles. (2015) 4:28239. doi: 10.3402/jev.v4.28239
55. Safdar A, Saleem A, Tarnopolsky MA. The potential of endurance exercise-derived exosomes to treat metabolic diseases. Nat Rev Endocrinol. (2016) 12:504–17. doi: 10.1038/nrendo.2016.76
56. Karolina DS, Tavintharan S, Armugam A, Sepramaniam S, Pek SL, Wong MT, et al. Circulating miRNA profiles in patients with metabolic syndrome. J Clin Endocrinol Metab. (2012) 97:E2271–6. doi: 10.1210/jc.2012-1996
57. Chen T, Zhang Y, Liu Y, Zhu D, Yu J, Li G, et al. MiR-27a promotes insulin resistance and mediates glucose metabolism by targeting PPAR-γ-mediated PI3K/AKT signaling. Aging. (2019) 11:7510–24. doi: 10.18632/aging.102263
58. Imai T, Takakuwa R, Marchand S, Dentz E, Bornert JM, Messaddeq N, et al. Peroxisome proliferator-activated receptor gamma is required in mature white and brown adipocytes for their survival in the mouse. Proc Natl Acad Sci USA. (2004) 101:4543–7. doi: 10.1073/pnas.0400356101
59. Kozak LP, Anunciado-Koza R. UCP1: its involvement and utility in obesity. Int J Obesity. (2005) 2008 (32 Suppl. 7):S32–8. doi: 10.1038/ijo.2008.236
60. Petrovic N, Walden TB, Shabalina IG, Timmons JA, Cannon B, Nedergaard J. Chronic peroxisome proliferator-activated receptor gamma (PPARgamma) activation of epididymally derived white adipocyte cultures reveals a population of thermogenically competent, UCP1-containing adipocytes molecularly distinct from classic brown adipocytes. J Biol Chem. (2010) 285:7153–64. doi: 10.1074/jbc.M109.053942
61. Seale P, Bjork B, Yang W, Kajimura S, Chin S, Kuang S, et al. PRDM16 controls a brown fat/skeletal muscle switch. Nature. (2008) 454:961–7. doi: 10.1038/nature07182
62. Seale P, Kajimura S, Yang W, Chin S, Rohas LM, Uldry M, et al. Transcriptional control of brown fat determination by PRDM16. Cell Metab. (2007) 6:38–54. doi: 10.1016/j.cmet.2007.06.001
63. Uldry M, Yang W, St-Pierre J, Lin J, Seale P, Spiegelman BM. Complementary action of the PGC-1 coactivators in mitochondrial biogenesis and brown fat differentiation. Cell Metab. (2006) 3:333–41. doi: 10.1016/j.cmet.2006.04.002
64. Ferrannini E, Bjorkman O, Reichard GA Jr., Pilo A, Olsson M, Wahren J, et al. The disposal of an oral glucose load in healthy subjects. A quantitative study. Diabetes. (1985) 34:580–8. doi: 10.2337/diab.34.6.580
65. Gual P, Le Marchand-Brustel Y, Tanti JF. Positive and negative regulation of insulin signaling through IRS-1 phosphorylation. Biochimie. (2005) 87:99–109. doi: 10.1016/j.biochi.2004.10.019
66. Zhu R, Zheng J, Chen L, Gu B, Huang S. Astragaloside IV facilitates glucose transport in C2C12 myotubes through the IRS1/AKT pathway and suppresses the palmitate-induced activation of the IKK/IκBα pathway. Int J Mol Med. (2016) 37:1697–705. doi: 10.3892/ijmm.2016.2555
67. Guo Q, Chen Z, Santhanam RK, Xu L, Gao X, Ma Q, et al. Hypoglycemic effects of polysaccharides from corn silk (Maydis stigma) and their beneficial roles via regulating the PI3K/Akt signaling pathway in L6 skeletal muscle myotubes. Int J Biol Macromol. (2019) 121:981–8. doi: 10.1016/j.ijbiomac.2018.10.100
68. Petersen MC, Shulman GI. Mechanisms of insulin action and insulin resistance. Physiol Rev. (2018) 98:2133–223. doi: 10.1152/physrev.00063.2017
69. Ahmadian M, Suh JM, Hah N, Liddle C, Atkins AR, Downes M, et al. PPARγ signaling and metabolism: the good, the bad and the future. Nat Med. (2013) 19:557–66. doi: 10.1038/nm.3159
Keywords: exercise, exosomes, miR-27a, browning effect of white adipose tissue, skeletal muscle, insulin sensitivity
Citation: Wang D, Zhang X, Li Y, Jia L, Zhai L, Wei W, Zhang L, Jiang H and Bai Y (2022) Exercise-Induced Browning of White Adipose Tissue and Improving Skeletal Muscle Insulin Sensitivity in Obese/Non-obese Growing Mice: Do Not Neglect Exosomal miR-27a. Front. Nutr. 9:940673. doi: 10.3389/fnut.2022.940673
Received: 10 May 2022; Accepted: 23 May 2022;
Published: 17 June 2022.
Edited by:
Amira M. G. Darwish, City of Scientific Research and Technological Applications, EgyptReviewed by:
Elsayed Hafez, City of Scientific Research and Technological Applications, EgyptFayed Megahed, City of Scientific Research and Technological Applications, Egypt
Copyright © 2022 Wang, Zhang, Li, Jia, Zhai, Wei, Zhang, Jiang and Bai. This is an open-access article distributed under the terms of the Creative Commons Attribution License (CC BY). The use, distribution or reproduction in other forums is permitted, provided the original author(s) and the copyright owner(s) are credited and that the original publication in this journal is cited, in accordance with accepted academic practice. No use, distribution or reproduction is permitted which does not comply with these terms.
*Correspondence: Yinglong Bai, eWxiYWlAY211LmVkdS5jbg==
†These authors have contributed equally to this work