- 1Food and Nutritional Sciences, University of Reading, Reading, United Kingdom
- 2MRC Human Immunology Unit, MRC Weatherall Institute of Molecular Medicine, University of Oxford, Oxford, United Kingdom
- 3School of Biological Sciences, University of Reading, Reading, United Kingdom
Iron deficiency is the most prevalent human micronutrient deficiency, disrupting the physiological development of millions of infants and children. Oral iron supplementation is used to address iron-deficiency anemia and reduce associated stunting but can promote infection risk since restriction of iron availability serves as an innate immune mechanism against invading pathogens. Raised iron availability is associated with an increase in enteric pathogens, especially Enterobacteriaceae species, accompanied by reductions in beneficial bacteria such as Bifidobacteria and lactobacilli and may skew the pattern of gut microbiota development. Since the gut microbiota is the primary driver of immune development, deviations from normal patterns of bacterial succession in early life can have long-term implications for immune functionality. There is a paucity of knowledge regarding how both iron deficiency and luminal iron availability affect gut microbiota development, or the subsequent impact on immunity, which are likely to be contributors to the increased risk of infection. Piglets are naturally iron deficient. This is largely due to their low iron endowments at birth (primarily due to large litter sizes), and their rapid growth combined with the low iron levels in sow milk. Thus, piglets consistently become iron deficient within days of birth which rapidly progresses to anemia in the absence of iron supplementation. Moreover, like humans, pigs are omnivorous and share many characteristics of human gut physiology, microbiota and immunity. In addition, their precocial nature permits early maternal separation, individual housing, and tight control of nutritional intake. Here, we highlight the advantages of piglets as valuable and highly relevant models for human infants in promoting understanding of how early iron status impacts physiological development. We also indicate how piglets offer potential to unravel the complexities of microbiota-immune responses during iron deficiency and in response to iron supplementation, and the link between these and increased risk of infectious disease.
Introduction
Inadequate nutrition is recognized as a major factor in anomalous development during early life and has serious implications for long-term health (1–3). Iron is an essential nutrient since it acts as a key prosthetic component (e.g., for haem and iron-sulfur clusters) associated with proteins that have vital roles in a wide range of key biochemical processes such as oxygen transport, the respiratory chain and the Krebs cycle. Iron deficiency (ID), where body iron stores are becoming depleted occurs when iron requirements exceed intake. As these store become exhausted, iron deficiency anemia (IDA) develops and affects around 1.2 billion people worldwide (4, 5). IDA is defined as serum hemoglobin (Hb) levels of < 105 and < 100 g/L in 4 and 9 month old infants, respectively (6). Those at particular risk of ID are the under-fives and pregnant women from low- and middle-income countries (7). In full-term infants born to iron-sufficient mothers, transplacental delivery of iron results in high neonatal reserves, around 75 mg/kg, primarily as hemoglobin and in the form of ferritin and hemosiderin (8, 9). Following birth, excess iron, derived from hemoglobin, is immediately transferred to storage compartments and consequently, under normal conditions, iron supplied through the diet is not required during the first 4–6 months of life (10). This may partly explain why human breastmilk possesses such a low iron content (∼0.35 mg/L; bioavailability of 45–100%) (11). However, after ∼6 months, infant iron stores derived from the mother become depleted whilst the developmental demand for iron increases due to higher erythropoietic and brain activity, along with increased tissue accretion as a result of high growth rate (12, 13). Demand for iron soon exceeds that available from breast milk prompting the need for iron from complementary foods and/or supplementation (14).
Dietary iron requirements during pregnancy are significantly increased. Based on a pre-pregnancy weight of 55 kg, it is estimated that an additional 1 g of iron is required during pregnancy, equating to around 3.6 mg/day on average (15). This is due to increasingly higher demands from the fetus and placenta, and rapid expansion of the maternal vascular volume during the latter half of gestation especially (16). However, in both poor and affluent societies a large proportion of women enter pregnancy with ID or IDA (17) since around 50% of women of childbearing age in low and middle income countries are anemic, although there is significant regional variation (18). In both ID and IDA, the iron endowment that new-borns receive from their mothers is often reduced such that iron reserves are depleted well before 4–6 months (when weaning generally commences) leading to the rapid onset of IDA. Infants born to anemic or iron-deficient mothers, and those with low birth weight, begin life with reduced iron stores and are at higher risk of developing ID before 4–6 months (19, 20). Preterm infants are also at increased risk of developing ID as maternal iron transfer to the fetus mostly occurs during the final trimester (21).
Rapid erythropoiesis, inadequate dietary iron consumption and limited iron bioavailability (linked to reduced absorption following enteric infection and/or dietary inhibitors) all contribute to increased risk for ID throughout infancy and childhood. At this time, iron requirements are high due to rapid growth (22). School-age children primarily consuming unfortified cereal-based diets are at greater risk of ID owing to low dietary iron intake (23). In addition, non-haem iron, the form derived from plant sources, has lower bioavailability and is more sensitive to enhancers (e.g., ascorbic acid) and inhibitors (e.g., phytate) of iron absorption compared to haem iron, the form derived from animal sources.
The iron demands of fully functioning adult immune systems are high and consequences of ID include the inhibition of neutrophil function, reduced microbicidal ability of macrophages, reductions in T-cell numbers and thymic atrophy (24–27). However, the mechanisms underlying such effects are poorly characterized and even less is understood regarding the impact of ID on the rapidly developing immunological architecture and immune-associated cell population expansion during infancy. These are important aspects of childhood IDA since the functionality of the adult immune system is highly dependent on appropriate development in early life. This indicates that childhood IDA could have longer-term detrimental consequences on immune function, even if iron-sufficiency is subsequently achieved in adulthood. It is now recognized that even in the absence of IDA, dietary ID has adverse functional consequences and is detrimental to many areas of growth during infancy, as previously reviewed (28).
The WHO report on “Daily iron supplementation” endorses iron supplementation as a public-health intervention for infants and children aged 6–23 months across the globe, where the prevalence of anemia in this age group is high (>40%). Recommendations are for daily doses of 10–12.5 mg elemental iron for 3 consecutive months per year for infants and children aged 6–23 months, which increases to 30 mg for preschool-aged children (29). However, a recent placebo-controlled study of iron supplementation for 12 weeks in ∼8 month old Bangladeshi infants concluded that although rates of anemia were reduced, there was no effect on developmental functional outcomes, including cognitive ability (30). An important caveat to iron supplementation recommendations is in areas with endemic malaria where iron supplementation is only recommended to those infants with access to malaria-prevention strategies (30). This follows comprehensive meta-analyses which concluded that there is a possible link between iron supplementation and increased risk of mortality or hospitalization from malaria (31).
Iron deficiency and infection risk—A trade-off
Complex relationships exist between anemia and infection. ID may result in increased infection morbidity, presumably due (in part at least) to inadequate iron supply to the immune system (32). However, ID can also protect against infection (33) in both humans and animal models. Since iron is crucial for bacterial growth, in particular for pathogens such as Escherichia coli (34, 35), it follows that insufficient iron availability, achieved by nutritional immunity mechanisms in the host, is an effective strategy to limit pathogenic growth and thus reduce infection risk (36). Consistent with this, various in vitro studies have reported reduced growth of potential enteropathogens, including Campylobacter jejuni and E. coli, within iron-deprived environments (37, 38). For example, limiting iron availability resulted in reductions in E. coli abundance to 0.8% compared to 10.7% in an iron-sufficient controls. Non-nutritional factors, such as infection and inflammation, also influence iron metabolism and can cause “anemia of inflammation,” previously termed anemia of chronic disease. This response is instigated by the host to limit systemic iron availability and thus combat ongoing infection (39), and is predominantly mediated through the upregulation of hepcidin, as we have previously reviewed (25). This peptide hormone acts as a major regulator of iron homeostasis. Hepcidin is secreted primarily by hepatocytes in response to various factors including body iron stores and plasma iron levels. It controls iron absorption, recycling, and release from stores by binding to the cellular iron exporter, ferroportin, causing it to internalize, leading to iron retention within cells. This consequently limits iron availability to extra-cellular pathogens (40, 41). Hepcidin expression is also responsive to elevated inflammatory cytokines including IL-6 and IL-22 (33, 42). Therefore, anemia of inflammation is characterized by adequate or high iron stores but low serum iron (bound to the serum iron chaperone, transferrin) (42). In contrast to ID, anemia of inflammation cannot be prevented or resolved by iron supplementation and may even be intensified by increased dietary iron (40).
To mitigate the increased risk of enteric infection driven by oral iron supplementation, perhaps an alternative route of iron administration could be considered. Intravenous (IV) iron sucrose can be administered to children with ID and is especially useful for those who have failed to respond to oral iron supplementation, for example those who suffer from iron malabsorption due to short bowel syndrome or anemia of inflammation. A further advantage of this IV route is that it bypasses the hepcidin-ferroportin pathway that controls iron absorption in the gut. Such infusions can mitigate ID following 1–5 doses of between 25 and 500 mg for up to 6 months following treatment and appears to be safe, effective and well tolerated (43, 44). However, infrequent, high doses are linked with an increased risk of transferrin oversaturation, although to a lesser extent than earlier non-sucrose-based formulations (45). Although promising at this stage, most of the trials exploring IV iron administration have focused on adolescence and adult populations and it is questionable as to whether it is feasible for young infants in rural settings in Low and Middle-income countries where ID is prevalent.
Role of iron in the development of the gut microbiota
Bacterial numbers and microbiota diversity increase in relation to distance from the stomach with the largest, most diverse population residing in the colon due to relatively more favorable conditions (46). Due to the influence of several factors, including pH and the chemical form of iron present, predicting the bioavailability of iron to the microbiota for each of the various sections of the intestinal tract remains problematic. However, it is estimated that on average ∼85% of dietary iron remains unabsorbed and colonic iron concentrations are in the region of 25 mM, of which approximately 0.4 mM is in the form of readily absorbable Fe2+ (47).
The majority of gut bacteria have essential requirements for iron and thus require its availability in the gut. However, the Lactobacillaceae are considered to be iron-independent members of the gut microbiota and preferentially utilize manganese instead (48). It is likely that iron availability influences microbial succession and the developmental stability of the longer-term microbiota. Increased iron availability has also been associated with a raised risk of the presence, or virulence potential, of enteric pathogens including Salmonella typhimurium, E. coli and Enterobacteriaceae, along with reductions in more beneficial bacteria such as bifidobacteria (49–51). Gut fermentation models inoculated with fecal matter from a child and propagated under iron limited conditions (1.56 ± 0.1 mg Fe L–1) showed a relative reduction in Roseburia spp., Eubacterium rectale, Clostridium cluster IV members and Bacteroides spp. along with relative increases in Lactobacillus spp. and Enterobacteriaceae compared to iron sufficient controls (52). Similarly a recent pilot study assessed the composition of the microbiota in IDA infants and young children concluding that ID is associated with distinct microbial signatures with increased abundance of Enterobacteriacea and Veillonellaceae, and decreased Coriobacteriaceae relative to healthy non-ID controls (53). Consistent with this, several large-scale human trials using various doses for different durations (2–18 months) have, in general, reported that oral iron supplementation is associated with higher risk (1–23%) of developing diarrhea in infants (54–56). However, the outcomes of such studies have been inconsistent (57, 58), which suggests the link is complex and that perhaps other factors are at play. One theory is that the effects of iron supplementation on the risks of enteric infections are highly dependent on the composition and metabolic activity of the underlying microbiota (59), which in turn is influenced by iron availability during the earlier developmental stages. These studies largely reported compositional changes to the microbiota during ID and IDA (Figure 1). However, an important consideration is how sustained these effects may be once sufficient iron levels have been attained. A recent early-life piglet study showed that ID (rapidly progressing to IDA during the trial) reported 27 bacterial genera differences in feces following 32 days of iron restriction (from 2 day of age) compared to iron-sufficient controls. It went on to demonstrate that when both groups received oral iron supplementation (standard 180–300 mg Fe/kg of diet) in weaner mix for a further 30 days, no differences in either bacterial populations or bacterial products of fermentation (volatile fatty acids) were detected. Although normal microbial communities appeared to have been be restored following this period of ID and IDA, bacterial colonization and succession in the gut is the primary driver of immune development which occurs in a programmed and sequential manner and is largely complete by 49 days following birth (60), well before the completion of the trial. Therefore different patterns of microbial colonization driven by iron limiting conditions in the gut is highly likely to have had considerable impact on immune function in later life and could effect susceptibility to infection. In addition, this study reported findings from limited pigs (∼n = 10/treatment group). Being outbred there are considerable inter-litter and inter-individual variations and in the absence of litter-matching to accommodate this, treatment differences in gut microbiotas at 61 days resulting from earlier ID/IDA would have to have been larger than physiological differences between piglets to have been observed. In addition, the trial was completed using 2 replicate groups and we have previously demonstrated that minor environmental variations during the first day of life exerted sustained influences on both the microbiota and metabolic phenotype which were of a higher magnitude then differences linked to divergent nutrition (61). Taken together, this suggests that important questions of longer-term impacts of early-life ID and IDA on the gut microbiota, and wider development, remain unanswered.
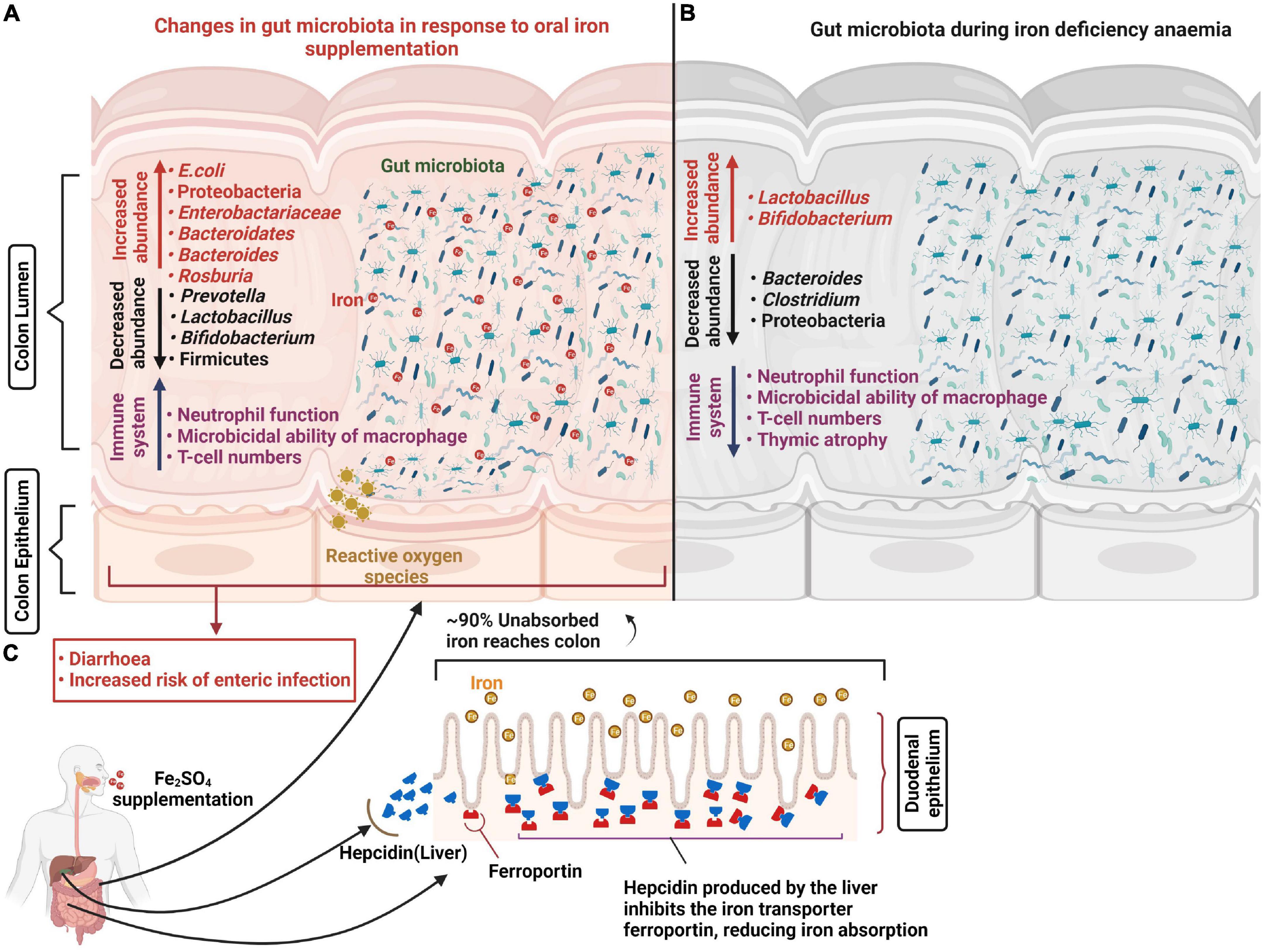
Figure 1. Changes in the gut microbiota in response to oral iron supplementation and anemia. Iron supplementation prevents iron-deficiency anemia and aids the generation of appropriate immune responses to invading pathogens. However, approximately 90% of unabsorbed iron reaches the colon where most bacteria have substantial iron requirements for growth. This excess iron results in decreased abundance of beneficial bacteria including lactobacilli and bifidobacteria and increased abundance of potential pathogens including E. coli which increase the risk of diarrhea and enteric infection. In addition, oral iron supplementation may result in the generation of reactive oxygen species (ROS) causing oxidative stress and epithelial damage (A). Abundance of potential pathogens including Bacteroides and Clostridium decrease in response to iron deficiency anemia and promote beneficial bacteria population growth (B). Hepatic cells release hepcidin in response to oral iron supplementation which internalizes the ferroportin (iron transport protein), reducing iron absorption in the duodenum (C).
Given the importance of the early microbiota for immune development (62) and susceptibility to infectious disease (63, 64), and the prevalence of global infant ID and IDA, it is perhaps surprising that early bacterial colonization and succession in the infant gut under such conditions have not been explored in more detail. Understanding the underlying mechanism, and the links with the early iron-deficient microbiota and developing immune systems could enable the development of improved approaches for reducing the burden of ID and IDA whilst avoiding the unwelcome side-effects of oral-iron supplements including epigastric comfort, nausea, vomiting (65) and the increased risk of infection. So far, studies have tended to focus directly on the response of the gut microbiota and immune development following iron supplementation in ID and IDA infants, rather than underlying mechanisms.
Iron and gut-associated immune system development
Immune systems are immature at birth and along with considerable thymic activity (66), the gut is a principal location for immune development in infants. This is because exposure to resident microbial populations (and to a lesser extent non-microbially derived ingested antigens) is the primary driver of both adaptive and innate immune-associated cell proliferation, education, and expansion. The gut microbiota is both maternally- (67) and environmentally acquired (68–70) and develops rapidly postnatally and, as highlighted above, the vast majority of these microbes have fundamental requirements for iron. The composition of the microbiota plays a key role in reducing enteric infection rates by competing for nutrients and inhibiting the colonization and proliferation of pathogenic organisms. In addition, the pattern of microbial succession in the gut is a key factor in the development of the microbiota (69–72) and variations from normal patterns of sequential colonization can alter the developmental trajectory of both the microbiota and immune system, as demonstrated by exposure to antibiotics during early life (73). Thus, luminal iron availability is likely to have major impacts on pathogen proliferation in the gut directly, through modifying the composition of resident gut microbiota population (which could promote or inhibit pathogen colonization and expansion), and also through changes in the development of immune function.
The B-cell population is one of the key players in microbiota homeostasis as it leads to abundant production of secretory immunoglobulin A (IgA), the dominant Ig in the gut, antibodies which recognize commensal bacteria (74, 75). Gut commensals, in addition to shaping B cells present in the gut, may also provide the control points to step down autoreactivity from the systemic B cell population (76). The association between gut microbiota and intestinal IgA is mutualistic, in that diverse and selective IgA production contributes to the development and stability of the gut microbiota which in turn promotes the development of regulatory T-cells supporting homeostatic IgA responses. In addition, gut bacteria influence T-cell proliferation and drive differential development of T-helper (Th) (77), T-regulatory (Treg) (78) and memory T-cell populations (79, 80). Secretary IgA is manufactured in both T-cell dependent and independent manners, therefore changes to T-cell populations can influence IgA production. Given the complex interactions between immunity and the microbiota, it follows that anemia- and oral iron-induced changes to intestinal bacterial succession in early-life have the potential to impact immune development, although mechanisms remain unclear.
Iron deficiency during later childhood (< 15 years) has been shown to significantly reduce CD4+ T-cell numbers and decrease CD4:CD8 T-cell ratios without impacting IgA, IgM or IgG levels (81). A further study in children found no reduction in CD4:CD8 T-cell ratios, IgA, or IgM levels in response to IDA, but did report significantly lower IgG levels along with reductions in systemic IL-6, and both macrophage and neutrophil phagocytic function (82). These data arise from small scale, limited and contradictory studies in children, rather than infants, and highlight the paucity of knowledge regarding the impact of ID and IDA during the crucial, early stages of immune development. However, a study of immune responses to vaccination in Tanzanian, Mozambican and Dutch children under 5 years of age reported correlations between anemia and lower frequencies of recent thymic emigrant T-cells, isotype-switched memory B-cells and plasmablasts, and showed that modulating iron bioavailability in vitro could recapitulate B-cell defects (83). However, this was in the absence of in vivo trials where the gut microbiota is likely to have been modified by luminal iron availability and therefore immune development is also likely to be affected. In summary, despite its clear importance (especially when considering the global prevalence of ID, IDA and oral iron supplementation), the relationship between anemia, oral iron supplementation, the microbiota and immune development remains relatively uncharacterized.
Chemical kinetics and absorption of iron
Dietary iron mostly occurs in the oxidized, ferric (Fe3+) form which is poorly absorbed. For iron absorption to occur, it must either undergo conversion to the ferrous (Fe2+) ionic state, or be present as haem or in nanoparticulate form (e.g., ferritin cores) (84, 85). A combination of stomach acidity and reducing agents, such as ascorbic acid and ferric reductase enzymes (including duodenal cytochrome B; DcytB) results in reduction of Fe3+ to Fe2+. Consequently, dietary non-haem iron is mainly absorbed across the duodenum and proximal jejunum in the highly soluble ferrous form via the divalent metal cation transporter 1 (DMT1, also known as NRAMP2) (86, 87). To combat poor absorption and gastrointestinal issues associated with oral iron supplementation, an innovative nano iron supplement is currently being trialed in Gambian children (age 6–35 months). Here, the iron supplement, hydroxide adipate tartrate (HAT) remains in a nano particulate form in the gut which may permit adsorption with fewer symptoms (88).
Partial absorption of dietary iron contained in food and supplements is a persistent and considerable obstacle in the treatment of ID and IDA. Typically, oral iron salt absorption varies between 2 and 13% under normal conditions but can be improved, to some extent, to between 5 and 28% if administered following fasting (89). However, a recent randomized, single-blind, crossover study in ID women demonstrated that coadministration of 15 g of galacto-oligosaccharides (GOS) or fructo-oligosaccharides (FOS) and a single 100 mg Fe tablet, labeled with 4 mg 57Fe or 58Fe, resulted in 45 and 51% increases in absorption, respectively compared to counterparts who received carbohydrate-based placebos (90). Similarly, a 4 week study in iron-sufficient adult Sprague-Dawley rats demonstrated an 8% increase in iron adsorption from standard feed when fortified (5/100 g feed) with GOS (91). The disparities in efficacy between these human and rodent trials could be linked with iron status, or species differences. Some probiotic strains have also been demonstrated to improve iron uptake, as recently reviewed by Rusu et al. (92). For example, meta-analysis of 15 studies found significant improvements in iron uptake in response to administration of Lactobacillus plantarum 299v (Lp299v), but not for other probiotic lactobacilli strains (93). The mechanism appears to be largely via probiotic-induced reduction of luminal Fe3+ to Fe2+ and thus promotion of iron uptake by enterocytes (92).
Since both GOS and FOS are established prebiotics known to selectively stimulate growth and activity of beneficial microbial strains residing in the gut (94, 95), there is potential for both to reduce the impact of increased luminal iron availability on microbial population skewing to a less beneficial phenotype. This could be especially relevant for the Lactobacillaceae which are abundant in healthy infant guts (96). This is because, as previously mentioned, they do not have requirements for iron and so are disadvantaged under iron-rich conditions. However, further research is required to determine whether such prebiotics increase iron absorption in infants and young children, and what effect GOS/FOS-iron combinations may have on the composition and metabolic activity of the gut microbiota during the critical stage of early-life development of other physiological systems. In addition to enhancing iron status, Lp299v (along with several other lactobacilli) has been shown to reduce the incidence and/or severity of a range of enteric infections, and to prevent E. coli attachment to enterocytes and the associated tight cell junction disruption in Caco-2 monolayers (97–100). Given that oral iron availability is also associated with increased enteric infection, such probiotics may also be beneficial in limiting some of the side-effects associated with oral iron supplementation.
Dietary iron negatively impacts host-pathogen competition
The universal iron supplementation policy in areas with high prevenance of ID and IDA results in the delivery of additional oral iron to significant numbers of children who are not iron deficient. This is also the case in more affluent societies where infant formula milk is consistently fortified with iron. This “additional” oral iron may ultimately result in higher quantities of luminal iron being made available to the developing gut microbiota (25). Importantly, an ex vivo study reported considerably raised growth of bacteria (including E. coli, Salmonella and Staphylococcus epidermis) in the serum of subjects receiving dietary iron supplementation (2 mg of iron/kg body weight). A strong correlation was observed between transferrin saturation and bacterial growth rates (101). This suggests that even modest levels of oral iron supplementation may contribute to bacteremia and may have implications for iron administered intravenously. Furthermore, a randomized controlled study in iron deficient and/or anemic Kenyan infants demonstrated that iron supplementation caused a deleterious shift in the gut microbial profile which included an increase in pathogenic bacterial population levels and decreases in beneficial lactobacillus and bifidobacterial numbers (59, 102). Consistent with this, a study in Swedish infants (non-anemic) demonstrated that the intake of infant formula high in iron (6.6 mg/day) was linked with a relatively lower population of bifidobacteria than counterparts consuming low iron formula (1.2 mg/day). Similarly, a reduction in the abundance of lactobacilli was observed in response to the administration of oral iron drops (6.6 mg/day) along with an increased prevalence of bacterial infection (103). Even modest levels of iron supplementation, equivalent to that received by infants in fortified formula milk (150 mg Fe/day) and substantially below levels considered toxic, was associated with significant changes in the gut microbiota in young rats. This excess iron was also associated with increased 3-hydroxybutyrate and decreased amino acids, urea and myo-inositol. These parameters were linked with adverse cognitive development quantified by memory and learning scoring using the passive avoidance test (104). Taking the above together, the results suggest that although oral iron supplementation is adequate in preventing IDA, it also causes a detrimental shift in bacterial populations which is likely to have long-term effects on microbiota and immune system development, and possibly cognitive function. Thus, there appears to be a health trade-off whereby treatment with oral iron supplements can have deleterious as well as beneficial consequences.
The piglet model of iron deficiency
A suitable animal model would be invaluable in generating mechanistic understanding of the multilateral interactions which occur between the gut microbiota, immune system and iron status. Such a model may also be instrumental in unraveling the reasons why oral iron supplementation may increase the risk of infection and thus aid the development of novel ID treatment strategies to limit such side effects. Rodent models have generated important biomedical information in this field and have several advantages over other animal species including transgenicity, rapid generation time, and accessibility of targeted reagents (105, 106). However, there are important disadvantages to using rodent models for ID which require iron-deficient diets over long periods of time, which is problematic for modeling early-life ID and IDA (107). Furthermore, mice have not adapted to adsorb haem which could limit the translational potential of some studies (107). A summary of human, pig and mouse characteristics relevant to assessing the effect of iron deficiency anemia and iron supplementation is presented in Figure 2.
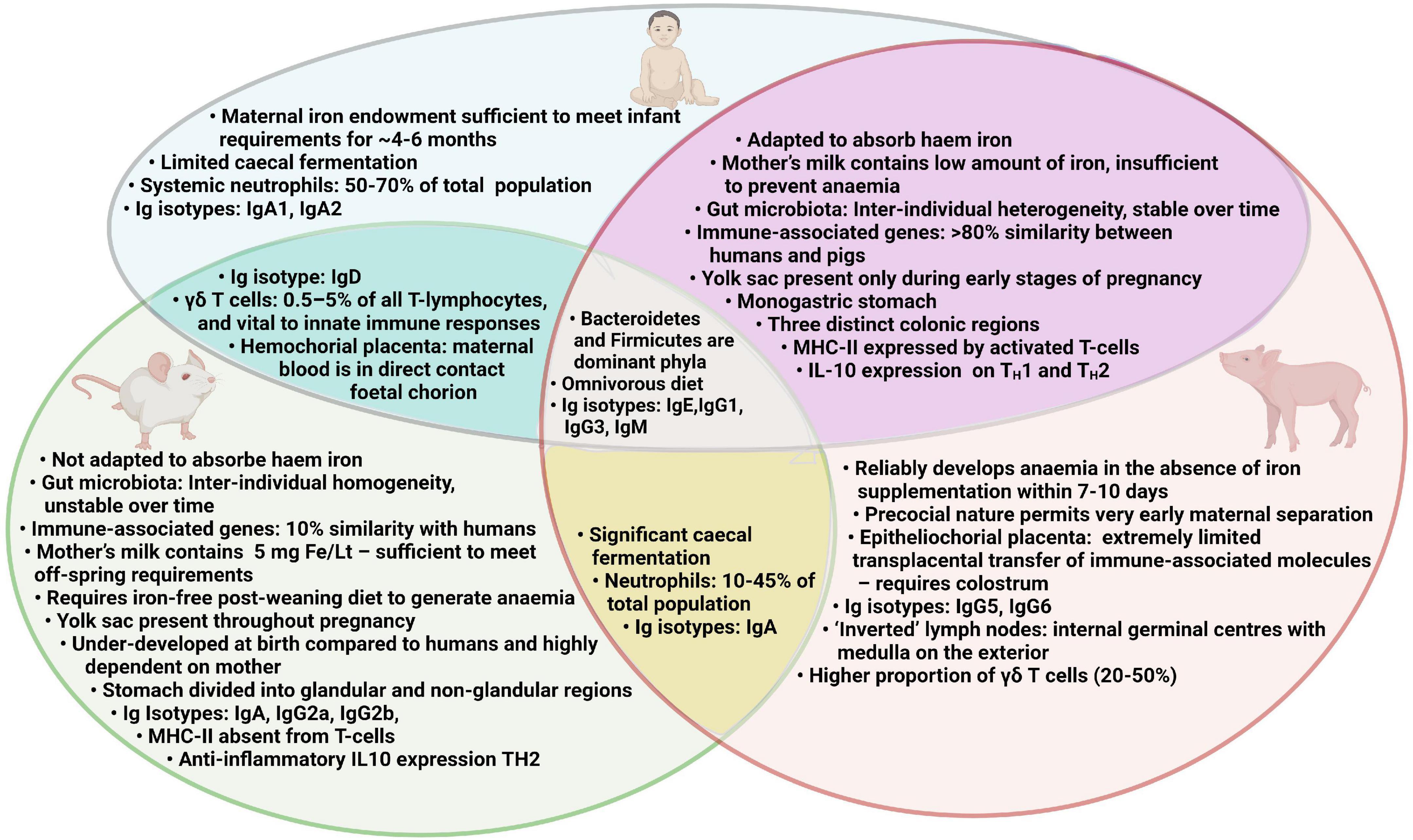
Figure 2. Comparison of human, pig and mouse characteristics relevant to assessing the effect of iron deficiency anemia and iron supplementation.
In contrast, in the absence of iron supplementation neonatal piglets start to become iron deficient within the first week of life. Since early-life environmental and dietary factors can have sustained impact on physiological development (73, 108), it is highly desirable that maternal influence is limited from a very young age and environmental factors are tightly controlled which is far less challenging in precocial species such as the pig; rodents are born relatively underdeveloped and are therefore highly reliant on their mothers during infancy. Consequently, the litter is often the unit which doesn’t conform to the 3Rs (replacement, reduction, and refinement) required by UK legislation, to reduce the number of animals required to generate power. In addition an outbred, rather than inbred, model better reflects the human population, We propose that the piglet model fulfills these criteria, and we provide evidence below to support this.
It is well established that omnivorous pigs are a valuable and tractable model for humans (109) as they share several key features including gastrointestinal immunology, physiology, microbiology, pathologies and dietary requirements (110–114). Full genome studies show that there are fewer difference between pigs and humans, than rodents and humans (115, 116). These factors suggest that pigs are valuable intermediates between highly reductionist, mechanistic studies in rodents, and epidemiological studies and clinical trials in humans. Pup-in-a-cup trials, where rats can be individually accommodated from 5 days of age, have been useful for assessing the impact of early nutrition on physiological development. However, precocial piglets are especially valuable models for early life since their self-sufficiency permits very early separation from their mothers and individual housing within a few hours of birth, thus limiting the maternal influence at this critical period of developmental plasticity. Comparative assessment of pig, mouse and human genomes demonstrated that structural and functional analyses of murine genes involved in immunity an inflammation shared only 10% similarity with humans for measured parameters, whereas in pigs this figure is > 80% (117, 118), Key similarities include the intra-epithelial lymphocytes, and a majority of cytotoxic suppressor T-cells and fewer TH cells (119). In pigs and humans, gut microbiotas are considerably more stable over the passage of time than in rodent models. Additionally, the intra-individual variability is reduced in mice compared to that of humans and pigs (120). Generally, the microbiota of pigs and humans also share similar diversities and dominant phyla, including Firmicutes and Bacteroides (121, 122). For these reasons, there is increased potential for determining the mechanisms underlying early microbiota-host interactions in human infants using piglet rather than rodent pup models.
As food animals, there is wide public acceptance of piglet use in research, which can be problematic for other non-rodent species such as primates, dogs and horses. Piglets are particularly prone to ID and will consistently and rapidly develop anemia if iron supplements are not provided (123, 124). Indeed, iron deficiency has been an established issue in the pig industry since the early twentieth century (125) when oral administration of iron salts, as a preventive measure, was first proposed. However, today an early intra-muscular injection of 200 mg of iron is standard husbandry practice throughout the pig industry to prevent the early onset of ID/IDA (126). Piglets are born with very low iron reserves (35–50 mg) which are only sufficient for 3–4 days since daily iron requirements range from 7 to 16 mg (127). Serum iron at day 4 after birth reduces by 5 fold in non-supplemented piglets and is barely detectable after 6 days (128). The situation is exacerbated by the rapid increase in litter size over recent years from 12 to 16 piglets (129) thus placing further iron demands on sows. Therefore, the sow-piglet dyad provides a highly useful potential model in the exploration of how manipulation of maternal feed practices and other interventions may improve iron status in offspring.
Poor efficiency of iron transfer through the placenta is an important contributing factor for the relatively low maternal iron endowment received prior to birth in both humans (130) and pigs (131). In the study by Colomer et al. (132), 156 infants were closely monitored during their first postnatal year. The risk of developing anemia was increased by 6.57-fold in infants born to mothers with anemia (<12 ng/ml) at the time of delivery. The “perfect parasite” is a phrase often used due to the misconception that the fetus is capable of procuring enough iron irrespective of the mother’s iron status. Although iron is transferred to fetal piglets during gestation, iron supplementation in sows during pregnancy leads to only limited improvements in iron status in offspring and is insufficient to combat the development of IDA in piglets (133). Similarly in anemic humans, while iron supplementation during pregnancy improves maternal iron status and may improve pregnancy outcome, including birth weight and reductions in pre-term births, brief periods of iron supplementation are unlikely to counter anemia in off-spring. Increasing the iron endowment received by infants probably requires improved maternal iron status before the pregnancy begins (134).
A further factor contributing to the development of ID in piglets is the relatively low iron content in sow milk (0.2–0.4 mg per L) (135). From this piglets can absorb ∼60–90% resulting in around 1 mg of iron per day which is insufficient to prevent ID in suckling piglets (133). This is similar to humans where breast milk contains around 0.4 mg/Lt (136). However, there is a remarkable capacity for transfer of serum iron to milk in rodents resulting in concentrations of ∼5 mg/L, sufficient to sustain off-spring iron status before weaning (137). Moreover, piglets have the highest growth rates of livestock animals typically increasing their plasma volume by 30% as well as doubling their weight in the first week of life (138) followed by a 10-fold increase from birth weight over the following 5 weeks (139). Most of the functionally active iron (60%) resides in the form of hemoglobin and the majority of the remainder is required for adequate enzymic function and the generation of myoglobin (127). Liver iron stores and sow milk together cannot meet such high iron requirement of piglets.
Although there are numerous physiological similarities between humans and pigs it is important to consider the differences in placentation and other anatomical features. In humans, placentation is haemochorial; maternal blood is in direct contact with the fetal chorion and thus transfer of passive immunity (and other maternal factors) occurs during gestation (140). However, pigs have an epitheliochorial placenta resulting in extremely limited transplacental transfer of immunoglobulins, leukocytes and various T-lymphocyte subsets during gestation (141, 142). For this reason, the neonatal piglet depends almost entirely on passive transfer from colostrum and, to a degree, subsequent milk. Another immunological difference between humans and pigs is lymph node structure. In comparison to humans, pig lymph nodes are inverted with internally placed germinal centers, a medulla located on the exterior and the afferent lymph diffusing to the periphery from the center, although the functional consequences remain unclear (143). Moreover, jejunal Peyer’s patches (JPP) and ileal Peyer’s patches (IPP) occur as multiple isolated follicles in humans, however, in pigs, IPP are continuous structures, but JPP present as isolated follicles. This may suggest functional differences but this is also unclear (144).
In conclusion, here we provide evidence promoting the piglet model as a valuable tool for the provision of novel insight into the mechanisms underlying host-microbe interactions during iron deficiency and in response to oral iron supplementation, especially during early-life. Given the global prevelance of iron deficiency-driven oral iron supplementation, there is an urgent need to identify alternatives to the current strategy (or refine those already used) since this has been correlated with increased risk of infection in already vulnerable infants and children. Exploration of this dilema using traditional model species suffers from challenging constraints that can be overcome within the piglet model.
Author contributions
MA, ML, and ZH conceived the idea. MA and ML performed literature review, composed the manuscript, and designed the figures. ZH, HD, and SA edited and provided critical feedback and expertise on iron biology. ML was responsible for the overall direction of the manuscript. All authors contributed to the article and approved the submitted version.
Conflict of interest
The authors declare that the research was conducted in the absence of any commercial or financial relationships that could be construed as a potential conflict of interest.
Publisher’s note
All claims expressed in this article are solely those of the authors and do not necessarily represent those of their affiliated organizations, or those of the publisher, the editors and the reviewers. Any product that may be evaluated in this article, or claim that may be made by its manufacturer, is not guaranteed or endorsed by the publisher.
References
1. Symonds ME, Mendez MA, Meltzer HM, Koletzko B, Godfrey K, Forsyth S, et al. Early life nutritional programming of obesity: Mother-child cohort studies. Ann Nutr Metab. (2013) 62:137–45. doi: 10.1159/000345598
2. Vaiserman AM. Early-life nutritional programming of type 2 diabetes: Experimental and quasi-experimental evidence. Nutrients. (2017) 9:236. doi: 10.3390/nu9030236
3. Carolan-Olah M, Duarte-Gardea M, Lechuga J. A critical review: Early life nutrition and prenatal programming for adult disease. J Clin Nurs. (2015) 24:3716–29. doi: 10.1111/jocn.12951
5. Li M, Hu Y, Mao D, Wang R, Chen J, Li W, et al. Prevalence of Anemia among Chinese rural residents. Nutrients. (2017) 9:192. doi: 10.3390/nu9030192
6. Domellöf M, Dewey KG, Lönnerdal B, Cohen RJ, Hernell O. The diagnostic criteria for iron deficiency in infants should be reevaluated. J Nutr. (2002) 132:3680–6. doi: 10.1093/jn/132.12.3680
7. World Health Organization. The global prevalence of anaemia in 2011. Geneva: World Health Organization (2015).
8. Dallman PR, Siimes MA, Stekel A. Iron deficiency in infancy and childhood. Am J Clin Nutr. (1980) 33:86–118. doi: 10.1093/ajcn/33.1.86
9. Alleyne M, Horne MK, Miller JL. Individualized treatment for iron-deficiency anemia in adults. Am J Med. (2008) 121:943–8. doi: 10.1016/j.amjmed.2008.07.012
10. Ziegler EE, Nelson SE, Jeter JM. Iron stores of breastfed infants during the first year of life. Nutrients. (2014) 6:2023–34. doi: 10.3390/nu6052023
11. Qasem WA, Friel JK. An overview of iron in term breast-fed infants. Clin Med Insights Pediatr. (2015) 9:79–84. doi: 10.4137/CMPed.S26572
12. Armitage AE, Agbla SC, Betts M, Sise EA, Jallow MW, Sambou E, et al. Rapid growth is a dominant predictor of hepcidin suppression and declining ferritin in Gambian infants. Haematologica. (2019) 104:1542–53. doi: 10.3324/haematol.2018.210146
13. Andrew EA, Schadrac CA, Modupeh B, Ebrima AS, Momodou WJ, Ellen S, et al. Rapid growth is a dominant predictor of hepcidin suppression and declining ferritin in Gambian infants. Haematologica. (2019) 104:1542–53.
14. Gilley SP, Krebs NF. Infant nutrition. 11 ed. In: Marriott BP, Birt DF, Stallings VA, Yates AA editors. Present knowledge in nutrition. (Cambridge, MA: Academic Press) (2020). p. 3–22. doi: 10.1016/B978-0-12-818460-8.00001-0
15. Fisher AL, Nemeth E. Iron homeostasis during pregnancy. Am J Clin Nutr. (2017) 106:1567S–74S. doi: 10.3945/ajcn.117.155812
16. Bothwell TH. Iron requirements in pregnancy and strategies to meet them. Am J Clin Nutr. (2000) 72:257S–64S. doi: 10.1093/ajcn/72.1.257S
17. McMahon LP. Iron deficiency in pregnancy. Obstet Med. (2010) 3:17–24. doi: 10.1258/om.2010.100004
18. Kinyoki D, Osgood-Zimmerman AE, Bhattacharjee NV, Schaeffer LE, Lazzar-Atwood A, Lu D, et al. Anemia prevalence in women of reproductive age in low- and middle-income countries between 2000 and 2018. Nat Med. (2021) 27:1761–82.
19. Lönnerdal B, Georgieff MK, Hernell O. Developmental physiology of iron absorption, homeostasis, and metabolism in the healthy term infant. J Pediatr. (2015) 167:S8–14. doi: 10.1016/j.jpeds.2015.07.014
20. World Health Organization. Nutritional anaemias: Tools for effective prevention and control. Geneva: World Health Organization (2017).
21. Moreno-Fernandez J, Ochoa JJ, Latunde-Dada GO, Diaz-Castro J. Iron deficiency and iron homeostasis in low birth weight preterm infants: A systematic review. Nutrients. (2019) 11:1090. doi: 10.3390/nu11051090
22. World Health Organization. Guideline: Daily iron supplementation in infants and children. Geneva: World Health Organization (2016).
23. World Health Organization. Vitamin and mineral requirements in human nutrition. Geneva: World Health Organization (2004).
24. Kumar V, Choudhry VP. Iron deficiency and infection. Indian J Pediatr. (2010) 77:789–93. doi: 10.1007/s12098-010-0120-3
25. Drakesmith H, Prentice AM. Hepcidin and the iron-infection axis. Science. (2012) 338:768–72. doi: 10.1126/science.1224577
26. Cherayil BJ. Iron and immunity: Immunological consequences of iron deficiency and overload. Arch Immunol Ther Exp. (2010) 58:407–15. doi: 10.1007/s00005-010-0095-9
27. Nairz M, Haschka D, Demetz E, Weiss G. Iron at the interface of immunity and infection. Front Pharmacol. (2014) 5:152. doi: 10.3389/fphar.2014.00152
28. Soliman AT, De Sanctis V, Kalra S. Anemia and growth. Indian J Endocrinol Metab. (2014) 18(Suppl. 1):S1–5. doi: 10.4103/2230-8210.145038
29. World Health Organization. Daily iron supplementation in infants and children Guideline. Geneva: World Health Organization (2016).
30. Pasricha S-R, Hasan MI, Braat S, Larson LM, Tipu SMM-U, Hossain SJ, et al. Benefits and risks of iron interventions in infants in rural Bangladesh. N Engl J Med. (2021) 385:982–95. doi: 10.1056/NEJMoa2034187
31. Neuberger A, Okebe J, Yahav D, Paul M. Oral iron supplements for children in malaria-endemic areas. Cochrane Database Syst Rev. (2016) 2:Cd006589. doi: 10.1002/14651858.CD006589.pub4
32. Keusch GT, Farthing MJ. Nutrition and infection. Annu Rev Nutr. (1986) 6:131–54. doi: 10.1146/annurev.nu.06.070186.001023
33. Spottiswoode N, Duffy PE, Drakesmith H. Iron, anemia and hepcidin in malaria. Front Pharmacol. (2014) 5:125. doi: 10.3389/fphar.2014.00125
34. Yu X, Gan Z, Wang Z, Tang X, Guo B, Du H. Increased iron availability aggravates the infection of Escherichia coli O157: H7 in mice. Biol Trace Elem Res. (2019) 190:457–65. doi: 10.1007/s12011-018-1579-4
35. Nandal A, Huggins CCO, Woodhall MR, McHugh J, Rodríguez-Quiñones F, Quail MA, et al. Induction of the ferritin gene (ftnA) of Escherichia coli by Fe2+–Fur is mediated by reversal of H-NS silencing and is RyhB independent. Mol Microbiol. (2010) 75:637–57. doi: 10.1111/j.1365-2958.2009.06977.x
36. Cassat JE, Skaar EP. Iron in infection and immunity. Cell Host Microbe. (2013) 13:509–19. doi: 10.1016/j.chom.2013.04.010
37. Parmanand BA, Kellingray L, Le Gall G, Basit AW, Fairweather-Tait S, Narbad A. A decrease in iron availability to human gut microbiome reduces the growth of potentially pathogenic gut bacteria; an in vitro colonic fermentation study. J Nutr Biochem. (2019) 67:20–7. doi: 10.1016/j.jnutbio.2019.01.010
38. Naikare H, Palyada K, Panciera R, Marlow D, Stintzi A. Major role for FeoB in &ıt;em>Campylobacter jejuni&ıt;/em> ferrous iron acquisition, gut colonization, and intracellular survival. Infect Immunity. (2006) 74:5433. doi: 10.1128/IAI.00052-06
39. Hadley C, DeCaro JA. Does moderate iron deficiency protect against childhood illness? A test of the optimal iron hypothesis in Tanzania. Am J Phys Anthropol. (2015) 157:675–9. doi: 10.1002/ajpa.22756
40. Camaschella C. New insights into iron deficiency and iron deficiency anemia. Blood Rev. (2017) 31:225–33. doi: 10.1016/j.blre.2017.02.004
41. Piovanetti Y. Breastfeeding beyond 12 months: An historical perspective. Pediatr Clin North Am. (2001) 48:199–206. doi: 10.1016/s0031-3955(05)70294-7
42. Nemeth E, Valore EV, Territo M, Schiller G, Lichtenstein A, Ganz T. Hepcidin, a putative mediator of anemia of inflammation, is a type II acute-phase protein. Blood. (2003) 101:2461–3. doi: 10.1182/blood-2002-10-3235
43. Crary SE, Hall K, Buchanan GR. Intravenous iron sucrose for children with iron deficiency failing to respond to oral iron therapy. Pediatr Blood Cancer. (2011) 56:615–9. doi: 10.1002/pbc.22930
44. Nestor JA, Afridi FA, Suarez R, Karem JB, Hardiman M, Hunter K, et al. intravenous iron infusions in pediatric patients: A single institution assessment of efficacy and adverse effects of IV iron infusions. Blood. (2019) 134:5872. doi: 10.1182/blood-2019-123800
45. Mantadakis E. Advances in pediatric intravenous iron therapy. Pediatr Blood Cancer. (2016) 63:11–6. doi: 10.1002/pbc.25752
46. Thursby E, Juge N. Introduction to the human gut microbiota. Biochem J. (2017) 474:1823–36. doi: 10.1042/BCJ20160510
47. Lund EK, Wharf SG, Fairweather-Tait SJ, Johnson IT. Increases in the concentrations of available iron in response to dietary iron supplementation are associated with changes in crypt cell proliferation in rat large intestine. J Nutr. (1998) 128:175–9. doi: 10.1093/jn/128.2.175
48. Wooldridge KG, Williams PH. Iron uptake mechanisms of pathogenic bacteria. Fems Microbiol Rev. (1993) 12:325–48. doi: 10.1111/j.1574-6976.1993.tb00026.x
49. Kortman GAM, Boleij A, Swinkels DW, Tjalsma H. Iron availability increases the pathogenic potential of Salmonella typhimurium and other enteric pathogens at the intestinal epithelial interface. PLoS One. (2012) 7:e29968. doi: 10.1371/journal.pone.0029968
50. Mevissen-Verhage EA, Marcelis JH, Harmsen-Van Amerongen WC, de Vos NM, Verhoef J. Effect of iron on neonatal gut flora during the first three months of life. Eur J Clin Microbiol. (1985) 4:273–8. doi: 10.1007/BF02013651
51. Zimmermann MB, Chassard C, Rohner F, N’Goran EK, Nindjin C, Dostal A, et al. The effects of iron fortification on the gut microbiota in African children: A randomized controlled trial in Cote d’Ivoire. Am J Clin Nutr. (2010) 92:1406–15. doi: 10.3945/ajcn.110.004564
52. Dostal A, Fehlbaum S, Chassard C, Zimmermann MB, Lacroix C. Low iron availability in continuous in vitro colonic fermentations induces strong dysbiosis of the child gut microbial consortium and a decrease in main metabolites. FEMS Microbiol Ecol. (2013) 83:161–75. doi: 10.1111/j.1574-6941.2012.01461.x
53. Muleviciene A, D’Amico F, Turroni S, Candela M, Jankauskiene A. Iron deficiency anemia-related gut microbiota dysbiosis in infants and young children: A pilot study. Acta Microbiol Immunol Hung. (2018) 65:551–64. doi: 10.1556/030.65.2018.045
54. Gera T, Sachdev HPS. Effect of iron supplementation on incidence of infectious illness in children: Systematic review. BMJ. (2002) 325:1142. doi: 10.1136/bmj.325.7373.1142
55. Richard SA, Zavaleta N, Caulfield LE, Black RE, Witzig RS, Shankar AH. Zinc and iron supplementation and malaria, diarrhea, and respiratory infections in children in the Peruvian Amazon. Am J Trop Med Hyg. (2006) 75:126–32. doi: 10.4269/ajtmh.2006.75.1.0750126
56. Chang S, El Arifeen S, Bari S, Wahed MA, Rahman KM, Rahman MT, et al. Supplementing iron and zinc: Double blind, randomized evaluation of separate or combined delivery. Eur J Clin Nutr. (2010) 64:153–60. doi: 10.1038/ejcn.2009.127
57. Moretti D, Zimmermann MB, Muthayya S, Thankachan P, Lee TC, Kurpad AV, et al. Extruded rice fortified with micronized ground ferric pyrophosphate reduces iron deficiency in Indian schoolchildren: A double-blind randomized controlled trial. Am J Clin Nutr. (2006) 84:822–9. doi: 10.1093/ajcn/84.4.822
58. Manger MS, McKenzie JE, Winichagoon P, Gray A, Chavasit V, Pongcharoen T, et al. A micronutrient-fortified seasoning powder reduces morbidity and improves short-term cognitive function, but has no effect on anthropometric measures in primary school children in northeast Thailand: A randomized controlled trial. Am J Clin Nutr. (2008) 87:1715–22. doi: 10.1093/ajcn/87.6.1715
59. Jaeggi T, Kortman GAM, Moretti D, Chassard C, Holding P, Dostal A, et al. Iron fortification adversely affects the gut microbiome, increases pathogen abundance and induces intestinal inflammation in Kenyan infants. Gut. (2015) 64:731. doi: 10.1136/gutjnl-2014-307720
60. Stokes CR, Bailey M, Haverson K, Harris C, Jones P, Inman C, et al. Postnatal development of intestinal immune system in piglets: Implications for the process of weaning. Anim Res. (2004) 53:325–34. doi: 10.1051/animres:2004020
61. Merrifield CA, Lewis MC, Berger B, Cloarec O, Heinzmann SS, Charton F, et al. Neonatal environment exerts a sustained influence on the development of the intestinal microbiota and metabolic phenotype. ISME J. (2015) 10:145–57. doi: 10.1038/ismej.2015.90
62. de Steenhuijsen Piters WAA, Binkowska J, Bogaert D. Early life microbiota and respiratory tract infections. Cell Host Microbe. (2020) 28:223–32. doi: 10.1016/j.chom.2020.07.004
63. Stevens EJ, Bates KA, King KC. Host microbiota can facilitate pathogen infection. PLoS Pathogens. (2021) 17:e1009514. doi: 10.1371/journal.ppat.1009514
64. Khosravi A, Yáñez A, Price Jeremy G, Chow A, Merad M, Goodridge Helen S, et al. Gut microbiota promote hematopoiesis to control bacterial infection. Cell Host Microbes. (2014) 15:374–81. doi: 10.1016/j.chom.2014.02.006
65. Cancelo-Hidalgo MJ, Castelo-Branco C, Palacios S, Haya-Palazuelos J, Ciria-Recasens M, Manasanch J, et al. Tolerability of different oral iron supplements: A systematic review. Curr Med Res Opin. (2013) 29:291–303. doi: 10.1185/03007995.2012.761599
66. Owen DL, Sjaastad LE, Farrar MA. Regulatory T cell development in the thymus. J Immunol. (2019) 203:2031. doi: 10.4049/jimmunol.1900662
67. Asnicar F, Manara S, Zolfo M, Truong DT, Scholz M, Armanini F, et al. Studying vertical microbiome transmission from mothers to infants by strain-level metagenomic profiling. mSystems. (2017) 2:e164–116. doi: 10.1128/mSystems.00164-16
68. Merrifield CA, Lewis MC, Berger B, Cloarec O, Heinzmann SS, Charton F, et al. Neonatal environment exerts a sustained influence on the development of the intestinal microbiota and metabolic phenotype. ISME J. (2016) 10:145–57.
69. Mulder IE, Schmidt B, Lewis M, Delday M, Stokes CR, Bailey M, et al. Restricting microbial exposure in early life negates the immune benefits associated with gut colonization in environments of high microbial diversity. PLoS One. (2011) 6:e28279. doi: 10.1371/journal.pone.0028279
70. Schmidt B, Mulder IE, Musk CC, Aminov RI, Lewis M, Stokes CR, et al. Establishment of normal gut microbiota is compromised under excessive hygiene conditions. PLoS One. (2011) 6:e28284. doi: 10.1371/journal.pone.0028284
71. Zhuang L, Chen H, Zhang S, Zhuang J, Li Q, Feng Z. Intestinal microbiota in early life and its implications on childhood health. Genomics Proteomics Bioinformatics. (2019) 17:13–25. doi: 10.1016/j.gpb.2018.10.002
72. Mulder IE, Schmidt B, Stokes CR, Lewis M, Bailey M, Aminov RI, et al. Environmentally-acquired bacteria influence microbial diversity and natural innate immune responses at gut surfaces. BMC Biol. (2009) 7:79. doi: 10.1186/1741-7007-7-79
73. Christoforidou Z, Burt R, Mulder I, Gill BP, Pluske J, Kelly D, et al. Development of immune cells in the intestinal mucosa can be affected by intensive and extensive farm environments, and antibiotic use. Front Immunol. (2018) 9:1061. doi: 10.3389/fimmu.2018.0106
74. Peterson DA, McNulty NP, Guruge JL, Gordon JI. IgA response to symbiotic bacteria as a mediator of gut homeostasis. Cell Host Microbe. (2007) 2:328–39. doi: 10.1016/j.chom.2007.09.013
75. Sterlin D, Fadlallah J, Adams O, Fieschi C, Parizot C, Dorgham K, et al. Human IgA binds a diverse array of commensal bacteria. J Exp Med. (2020) 217:e20181635. doi: 10.1084/jem.20181635
76. Vossenkämper A, Blair PA, Safinia N, Fraser LD, Das L, Sanders TJ, et al. A role for gut-associated lymphoid tissue in shaping the human B cell repertoire. J Exp Med. (2013) 210:1665–74. doi: 10.1084/jem.20122465
77. Yang Y, Torchinsky MB, Gobert M, Xiong H, Xu M, Linehan JL, et al. Focused specificity of intestinal T H 17 cells towards commensal bacterial antigens. Nature. (2014) 510:152–6. doi: 10.1038/nature13279
78. Ivanov II, Atarashi K, Manel N, Brodie EL, Shima T, Karaoz U, et al. Induction of intestinal Th17 cells by segmented filamentous bacteria. Cell. (2009) 139:485–98. doi: 10.1016/j.cell.2009.09.033
79. Su LF, Kidd BA, Han A, Kotzin JJ, Davis MM. Virus-specific CD4+ memory-phenotype T cells are abundant in unexposed adults. Immunity. (2013) 38:373–83. doi: 10.1016/j.immuni.2012.10.021
80. Campion SL, Brodie TM, Fischer W, Korber BT, Rossetti A, Goonetilleke N, et al. Proteome-wide analysis of HIV-specific naive and memory CD4+ T cells in unexposed blood donors. J Exp Med. (2014) 211:1273–80. doi: 10.1084/jem.20130555
81. Das I, Saha K, Mukhopadhyay D, Roy S, Raychaudhuri G, Chatterjee M, et al. Impact of iron deficiency anemia on cell-mediated and humoral immunity in children: A case control study. J Nat Sci Biol Med. (2014) 5:158–63. doi: 10.4103/0976-9668.127317
82. Hassan TH, Badr MA, Karam NA, Zkaria M, El Saadany HF, Abdel Rahman DM, et al. Impact of iron deficiency anemia on the function of the immune system in children. Medicine. (2016) 95:e5395–e. doi: 10.1097/MD.0000000000005395
83. Hill DL, Carr EJ, Rutishauser T, Moncunill G, Campo JJ, Innocentin S, et al. Immune system development varies according to age, location, and anemia in African children. Sci Transl Med. (2020) 12:eaaw9522. doi: 10.1126/scitranslmed.aaw9522
84. Dan W, Qinghua W, Hengjia N, Gang L, Zheng R, Yulong Y. Treatments for iron deficiency (ID): Prospective organic iron fortification. Curr Pharmaceutical Des. (2019) 25:325–32. doi: 10.2174/1381612825666190319111437
85. Powell JJ, Bruggraber SF, Faria N, Poots LK, Hondow N, Pennycook TJ, et al. A nano-disperse ferritin-core mimetic that efficiently corrects anemia without luminal iron redox activity. Nanomedicine. (2014) 10:1529–38. doi: 10.1016/j.nano.2013.12.011
86. Ovesen L, Bendtsen F, Tage-Jensen U, Pedersen N, Gram B, Rune S. Intraluminal pH in the stomach, duodenum, and proximal jejunum in normal subjects and patients with exocrine pancreatic insufficiency. Gastroenterology. (1986) 90:958–62. doi: 10.1016/0016-5085(86)90873-5
87. Jacobs A, Miles P. Intraluminal transport of iron from stomach to small-intestinal mucosa. Br Med J. (1969) 4:778–81. doi: 10.1136/bmj.4.5686.778
88. Pereira D, Mohammed N, Ofordile O, Camara F, Baldeh B, Mendy T, et al. A novel nano-iron supplement to safely combat iron deficiency and anaemia in young children: The IHAT-GUT double-blind, randomised, placebo-controlled trial protocol. Gates Open Res. (2018) 2:48. doi: 10.12688/gatesopenres.12866.2
89. Cook JD. Diagnosis and management of iron-deficiency anaemia. Best Pract Res Clin Haematol. (2005) 18:319–32. doi: 10.1016/j.beha.2004.08.022
90. Giorgetti A, Husmann FMD, Zeder C, Herter-Aeberli I, Zimmermann MB. Prebiotic galacto-oligosaccharides and fructo-oligosaccharides, but not acacia gum, increase iron absorption from a single high-dose ferrous fumarate supplement in iron-depleted women. J Nutr. (2022) 152:1015–21. doi: 10.1093/jn/nxac003
91. Maawia K, Iqbal S, Qamar TR, Rafiq P, ullah A, Ahmad M-u-D. Production of impure prebiotic galacto-oligosaccharides and their effect on calcium, magnesium, iron and zinc absorption in Sprague-Dawley rats. PharmaNutrition. (2016) 4:154–60. doi: 10.1016/j.phanu.2016.10.003
92. Rusu IG, Suharoschi R, Vodnar DC, Pop CR, Socaci SA, Vulturar R, et al. Iron supplementation influence on the gut microbiota and probiotic intake effect in iron deficiency—a literature-based review. Nutrients. (2020) 12:1993. doi: 10.3390/nu12071993
93. Vonderheid SC, Tussing-Humphreys L, Park C, Pauls H, OjiNjideka Hemphill N, LaBomascus B, et al. A systematic review and meta-analysis on the effects of probiotic species on iron absorption and iron status. Nutrients. (2019) 11:2938. doi: 10.3390/nu11122938
94. Walton GE, van den Heuvel EG, Kosters MH, Rastall RA, Tuohy KM, Gibson GR. A randomised crossover study investigating the effects of galacto-oligosaccharides on the faecal microbiota in men and women over 50 years of age. Br J Nutr. (2012) 107:1466–75. doi: 10.1017/S0007114511004697
95. Wang S, Xiao Y, Tian F, Zhao J, Zhang H, Zhai Q, et al. Rational use of prebiotics for gut microbiota alterations: Specific bacterial phylotypes and related mechanisms. J Funct Foods. (2020) 66:103838. doi: 10.1016/j.jff.2020.103838
96. Tandon D, Haque MM, Gote M, Jain M, Bhaduri A, Dubey AK, et al. A prospective randomized, double-blind, placebo-controlled, dose-response relationship study to investigate efficacy of fructo-oligosaccharides (FOS) on human gut microflora. Sci Rep. (2019) 9:5473. doi: 10.1038/s41598-019-41837-3
97. Liévin-Le Moal V, Servin AL. Anti-infective activities of lactobacillus strains in the human intestinal microbiota: From probiotics to gastrointestinal anti-infectious biotherapeutic agents. Clin Microbiol Rev. (2014) 27:167–99. doi: 10.1128/CMR.00080-13
98. Kaźmierczak-Siedlecka K, Daca A, Folwarski M, Witkowski JM, Bryl E, Makarewicz W. The role of Lactobacillus plantarum 299v in supporting treatment of selected diseases. Central Eur J Immunol. (2020) 45:488–93. doi: 10.5114/ceji.2020.101515
99. Potoènjak M, Pušiæ P, Frece J, Abram M, Jankoviæ T, Gobin I. Three New Lactobacillus plantarum strains in the probiotic toolbox against gut pathogen Salmonella enterica Serotype Typhimurium. Food Technol Biotechnol. (2017) 55:48–54. doi: 10.17113/ftb.55.01.17.4693
100. Qin H, Zhang Z, Hang X, Jiang Y. L. plantarum prevents Enteroinvasive Escherichia coli-induced tight junction proteins changes in intestinal epithelial cells. BMC Microbiol. (2009) 9:63. doi: 10.1186/1471-2180-9-63
101. Cross JH, Bradbury RS, Fulford AJ, Jallow AT, Wegmüller R, Prentice AM, et al. Oral iron acutely elevates bacterial growth in human serum. Sci Rep. (2015) 5:16670. doi: 10.1038/srep16670
102. Paganini D, Uyoga MA, Kortman GAM, Cercamondi CI, Moretti D, Barth-Jaeggi T, et al. Prebiotic galacto-oligosaccharides mitigate the adverse effects of iron fortification on the gut microbiome: A randomised controlled study in Kenyan infants. Gut. (2017) 66:1956. doi: 10.1136/gutjnl-2017-314418
103. Simonyté Sjödin K, Domellöf M, Lagerqvist C, Hernell O, Lönnerdal B, Szymlek-Gay EA, et al. Administration of ferrous sulfate drops has significant effects on the gut microbiota of iron-sufficient infants: A randomised controlled study. Gut. (2019) 68:2095–7. doi: 10.1136/gutjnl-2018-316988
104. Alexeev EE, He X, Slupsky CM, Lönnerdal B. Effects of iron supplementation on growth, gut microbiota, metabolomics and cognitive development of rat pups. PLoS One. (2017) 12:e0179713. doi: 10.1371/journal.pone.0179713
105. Lubs HA, Stevenson RE, Schwartz CE. Fragile X and X-linked intellectual disability: Four decades of discovery. Am J Hum Genet. (2012) 90:579–90. doi: 10.1016/j.ajhg.2012.02.018
106. Bryda EC. The mighty mouse: The impact of rodents on advances in biomedical research. Mo Med. (2013) 110:207–11.
107. Fillebeen C, Gkouvatsos K, Fragoso G, Calvé A, Garcia-Santos D, Buffler M, et al. Mice are poor heme absorbers and do not require intestinal Hmox1 for dietary heme iron assimilation. Haematologica. (2015) 100:e334–7. doi: 10.3324/haematol.2015.126870
108. Merrifield CA, Lewis MC, Claus SP, Pearce JTM, Cloarec O, Duncker S, et al. Weaning diet induces sustained metabolic phenotype shift in the pig and influences host response to Bifidobacterium lactis NCC2818. Gut. (2013) 62:842–51. doi: 10.1136/gutjnl-2011-301656
109. Groenen MAM, Archibald AL, Uenishi H, Tuggle CK, Takeuchi Y, Rothschild MF, et al. Analyses of pig genomes provide insight into porcine demography and evolution. Nature. (2012) 491:393–8.
110. Meurens F, Summerfield A, Nauwynck H, Saif L, Gerdts V. The pig: A model for human infectious diseases. Trends Microbiol. (2012) 20:50–7. doi: 10.1016/j.tim.2011.11.002
111. Sangild PT, Thymann T, Schmidt M, Stoll B, Burrin DG, Buddington RK. Invited review: The preterm pig as a model in pediatric gastroenterology. J Anim Sci. (2013) 91:4713–29. doi: 10.2527/jas.2013-6359
112. Bassols A, Costa C, Eckersall PD, Osada J, Sabria J, Tibau J. The pig as an animal model for human pathologies: A proteomics perspective. Proteomics Clin Appl. (2014) 8:715–31. doi: 10.1002/prca.201300099
113. Pacha J. Development of intestinal transport function in mammals. Physiol Rev. (2000) 80:1633–67. doi: 10.1152/physrev.2000.80.4.1633
114. Pabst R. The pig as a model for immunology research. Cell Tissue Res. (2020) 380:287–304. doi: 10.1007/s00441-020-03206-9
115. Wernersson R, Schierup MH, Jorgensen FG, Gorodkin J, Panitz F, Staerfeldt HH, et al. Pigs in sequence space: A 0.66X coverage pig genome survey based on shotgun sequencing. BMC Genomics. (2005) 6:70. doi: 10.1186/1471-2164-6-70
116. Jorgensen FG, Hobolth A, Hornshoj H, Bendixen C, Fredholm M, Schierup MH. Comparative analysis of protein coding sequences from human, mouse and the domesticated pig. BMC Biol. (2005) 3:2. doi: 10.1186/1741-7007-3-2
118. Zhang Q, Widmer G, Tzipori S. A pig model of the human gastrointestinal tract. Gut Microbes. (2013) 4:193–200.
119. Ekiz C, Agaoglu L, Karakas Z, Gurel N, Yalcin I. The effect of iron deficiency anemia on the function of the immune system. Hematol J. (2005) 5:579–83. doi: 10.1038/sj.thj.6200574
120. Thompson CL, Hofer MJ, Campbell IL, Holmes AJ. Community dynamics in the mouse gut microbiota: A possible role for IRF9-regulated genes in community homeostasis. PLoS One. (2010) 5:e10335. doi: 10.1371/journal.pone.0010335
121. Rinninella E, Raoul P, Cintoni M, Franceschi F, Miggiano GAD, Gasbarrini A, et al. What is the healthy gut microbiota composition? A Changing ecosystem across age, environment, diet, and diseases. Microorganisms. (2019) 7:14. doi: 10.3390/microorganisms7010014
122. Hatcher HC, Singh RN, Torti FM, Torti SV. Synthetic and natural iron chelators: Therapeutic potential and clinical use. Future Med Chem. (2009) 1:1643–70. doi: 10.4155/fmc.09.121
123. Antonides A, van Laarhoven S, van der Staay FJ, Nordquist RE. Non-anemic iron deficiency from birth to weaning does not impair growth or memory in piglets. Front Behav Neurosci. (2016) 10:112. doi: 10.3389/fnbeh.2016.00112
124. Schubert R, Frank F, Nagelmann N, Liebsch L, Schuldenzucker V, Schramke S, et al. Neuroimaging of a minipig model of huntington’s disease: Feasibility of volumetric, diffusion-weighted and spectroscopic assessments. J Neurosci Methods. (2016) 265:46–55. doi: 10.1016/j.jneumeth.2015.11.017
125. McGowan JP, Crichton A. Iron deficiency in pigs. Biochem J. (1924) 18:265. doi: 10.1042/bj0180265
126. Bhattarai S, Nielsen JP. Association between hematological status at weaning and weight gain post-weaning in piglets. Livest Sci. (2015) 182:64–8. doi: 10.1016/j.livsci.2015.10.017
127. Jolliff J, Mahan D. Effect of injected and dietary iron in young pigs on blood hematology and postnatal pig growth performance. J Anim Sci. (2011) 89:4068–80. doi: 10.2527/jas.2010-3736
128. Lipinski P, Starzyñski RR, Canonne-Hergaux F, Tudek B, Oliñski R, Kowalczyk P, et al. Benefits and risks of iron supplementation in anemic neonatal pigs. Am J Pathol. (2010) 177:1233–43. doi: 10.2353/ajpath.2010.091020
129. Ward SA, Kirkwood RN, Plush KJ. Are larger litters a concern for piglet survival or an effectively manageable trait? Animals. (2020) 10:309. doi: 10.3390/ani10020309
130. Radlowski EC, Johnson RW. Perinatal iron deficiency and neurocognitive development. Front Hum Neurosci. (2013) 7:585. doi: 10.3389/fnhum.2013.00585
131. Szudzik M, Starzyñski RR, Joñczy A, Mazgaj R, Lenartowicz M, Lipiñski P. Iron supplementation in suckling piglets: An ostensibly easy therapy of neonatal iron deficiency anemia. Pharmaceuticals. (2018) 11:128. doi: 10.3390/ph11040128
132. Colomer J, Colomer C, Gutierrez D, Jubert A, Nolasco A, Donat J, et al. Anaemia during pregnancy as a risk factor for infant iron deficiency: Report from the Valencia Infant Anaemia Cohort (VIAC) study. Paediatr Perinat Epidemiol. (1990) 4:196–204. doi: 10.1111/j.1365-3016.1990.tb00638.x
133. Svoboda M, Drabek J. Iron deficiency in suckling piglets: Etiology, clinical aspects and diagnosis. Folia Vet. (2005) 49:104–11.
134. Scholl TO. Maternal iron status: Relation to fetal growth, length of gestation, and iron endowment of the neonate. Nutr Rev. (2011) 69(Suppl. 1):S23–9. doi: 10.1111/j.1753-4887.2011.00429.x
135. Li Y, Yang W, Dong D, Jiang S, Yang Z, Wang Y. Effect of different sources and levels of iron in the diet of sows on iron status in neonatal pigs. Anim Nutr. (2018) 4:197–202. doi: 10.1016/j.aninu.2018.01.002
136. Cai C, Harding SV, Friel JK. Breast milk iron concentrations may be lower than previously reported: Implications for exclusively breastfed infants. Matern Pediatr Nutr J. (2015) 2:2. doi: 10.4172/2472-1182.1000104
137. Zhang P, Sawicki V, Lewis A, Hanson L, Monks J, Neville MC. The effect of serum iron concentration on iron secretion into mouse milk. J Physiol. (2000) 522:479–91. doi: 10.1111/j.1469-7793.2000.t01-2-00479.x
138. Svoboda M, Píšt’ková K. Oral iron administration in suckling piglets–a review. Acta Vet Brno. (2018) 87:77–83. doi: 10.2754/avb201887010077
139. Kim JC, Wilcock P, Bedford MR. Iron status of piglets and impact of phytase superdosing on iron physiology: A review. Anim Feed Sci Technol. (2018) 235:8–14. doi: 10.1016/j.anifeedsci.2017.11.001
140. Fuchs R, Ellinger I. Endocytic and transcytotic processes in villous syncytiotrophoblast: Role in nutrient transport to the human fetus. Traffic. (2004) 5:725–38. doi: 10.1111/j.1600-0854.2004.00221.x
141. Pomorska-Mol M, Markowska-Daniel I, Bednarek D. Flow cytometric analysis of leukocytes in porcine mammary secretions. Bull Vet Inst Pulawy. (2010) 54:188–92.
142. Song G, Bailey DW, Dunlap KA, Burghardt RC, Spencer TE, Bazer FW, et al. Cathepsin B, cathepsin L, and cystatin C in the porcine uterus and placenta: Potential roles in endometrial/placental remodeling and in fluid-phase transport of proteins secreted by uterine epithelia across placental areolae. Biol Reprod. (2010) 82:854–64. doi: 10.1095/biolreprod.109.080929
143. Bordet E, Frétaud M, Crisci E, Bouguyon E, Rault S, Pezant J, et al. Macrophage-B Cell interactions in the inverted porcine lymph node and their response to porcine reproductive and respiratory syndrome virus. Front Immunol. (2019) 10:953. doi: 10.3389/fimmu.2019.00953
Keywords: iron deficiency, enteric infection, iron supplementation, neonatal gut health, anaemia, immunity, gut microbiota, dysbiosis
Citation: Abbas M, Hayirli Z, Drakesmith H, Andrews SC and Lewis MC (2022) Effects of iron deficiency and iron supplementation at the host-microbiota interface: Could a piglet model unravel complexities of the underlying mechanisms? Front. Nutr. 9:927754. doi: 10.3389/fnut.2022.927754
Received: 24 April 2022; Accepted: 05 August 2022;
Published: 04 October 2022.
Edited by:
Annelies Geirnaert, ETH Zürich, SwitzerlandReviewed by:
Katrin Giller, ETH Zürich, SwitzerlandPascale Vonaesch, Université de Lausanne, Switzerland
Copyright © 2022 Abbas, Hayirli, Drakesmith, Andrews and Lewis. This is an open-access article distributed under the terms of the Creative Commons Attribution License (CC BY). The use, distribution or reproduction in other forums is permitted, provided the original author(s) and the copyright owner(s) are credited and that the original publication in this journal is cited, in accordance with accepted academic practice. No use, distribution or reproduction is permitted which does not comply with these terms.
*Correspondence: Marie C. Lewis, bWFyaWUubGV3aXNAcmVhZGluZy5hYy51aw==