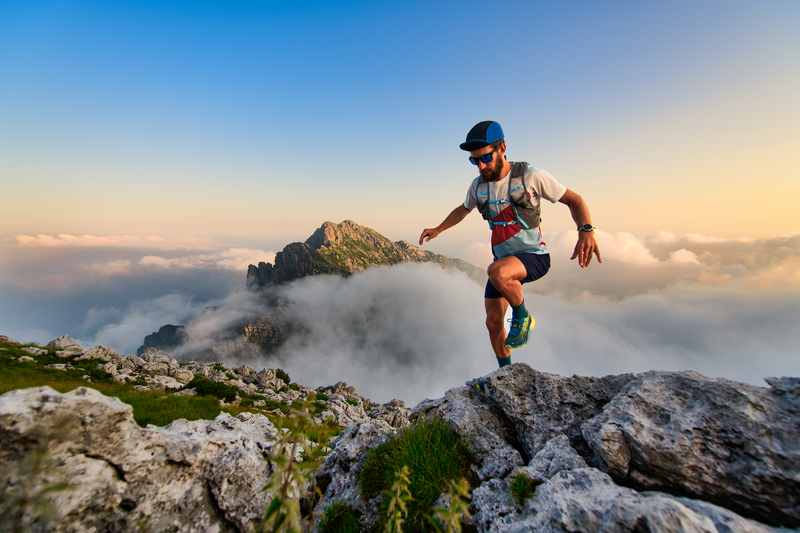
95% of researchers rate our articles as excellent or good
Learn more about the work of our research integrity team to safeguard the quality of each article we publish.
Find out more
MINI REVIEW article
Front. Nutr. , 27 May 2022
Sec. Food Chemistry
Volume 9 - 2022 | https://doi.org/10.3389/fnut.2022.925846
This article is part of the Research Topic Physical-Chemical Interactions and Composition-Structure-Property Modifications During Processing: Food Quality, Nutrition, and Health View all 15 articles
Over the latest decade, lipidomics has been extensively developed to give robust strength to the qualitative and quantitative information of lipid molecules derived from physiological animal tissues and edible muscle foods. The main lipidomics analytical platforms include mass spectrometry (MS) and nuclear magnetic resonance (NMR), where MS-based approaches [e.g., “shotgun lipidomics,” ultra-performance liquid chromatography-tandem mass spectrometry (UPLC-MS/MS), matrix-assisted laser desorption and ionization time-of-flight mass spectrometry (MALDI-TOF-MS)] have been widely used due to their good sensitivity, high availability, and accuracy in identification/quantification of basal lipid profiles in complex biological point of view. However, each method has limitations for lipid-species [e.g., fatty acids, triglycerides (TGs), and phospholipids (PLs)] analysis, and necessitating the extension of effective chemometric-resolved modeling and novel bioinformatic strategies toward molecular insights into alterations in the metabolic pathway. This review summarized the latest research advances regarding the application of advanced lipidomics in muscle origin and meat processing. We concisely highlighted and presented how the biosynthesis and decomposition of muscle-derived lipid molecules can be tailored by intrinsic characteristics during meat production (i.e., muscle type, breed, feeding, and freshness). Meanwhile, the consequences of some crucial hurdle techniques from both thermal/non-thermal perspectives were also discussed, as well as the role of salting/fermentation behaviors in postmortem lipid biotransformation. Finally, we proposed the inter-relationship between potential/putative lipid biomarkers in representative physiological muscles and processed meats, their metabolism accessibility, general nutritional uptake, and potency on human health.
The global meat industry is a continuously growing sector with an ever-increasing demand. The Organization for Economic Co-operation and Development (OECD) and the Food and Agriculture Organization of the United Nations (FAO) have recently stated that worldwide meat production is projected to expand by approximately 44 million tons by 2030, despite the detrimental impacts of the coronavirus disease 2019 (COVID-19) pandemic and other possible restrictions (1). Meat is considered a unique animal-derived food providing high biological value proteins with all essential amino acids and various micronutrients (2). Alongside proteins, lipids are abundant constituents in meat and meat products that play critical roles in providing desirable mouth-feel perception, characteristic flavor, favorable texture, juiciness, and enhanced cooking yield (3). According to the complexity of structure and biosynthesis, lipids are divided into eight categories, depending on their differences in the level of unsaturation, the type of the covalent bond, the fatty acyl chain length, double bond location, the head groups, Z/E geometric isomerism, and the branched functional groups (4, 5). Specifically, these muscle-derived lipid species mainly include non-esterified/free fatty acids (FFAs), glycerolipids (GLs), glycerophospholipids (GPs), sphingolipids (SLs), sterol lipids, prenol lipids, saccharolipids, and polyketides (6). Among them, triglycerides (TGs) or triacylglycerols (TAGs) and phospholipids (PLs), two common and most abundant categories of lipids, are highly associated with health and nutritional functions in the body (7, 8). TGs are mainly composed of FAs, such as capric acid (Ca) and lauric acid (La), but their contents can vary greatly among different breeds and muscle tissues (9). Membrane phospholipid composition may play a critical role in subsequent lipid oxidation development in raw and cooked meats (10, 11), while SLs and glycolipids contribute more functions to human health, such as increasing anti-inflammatory and anticarcinogenic activities and alleviating the risk of cardiovascular diseases and cholesterol absorption (7, 12). Traditional studies utilize gas chromatography (GC) with known standards or mass spectrometry (MS) to detect and identify total fatty acids in the samples, where saponification and derivatization protocols are usually required to generate the volatile fatty acid analytes and may result in the loss of information about the original esterified structure (neutral or polar lipids) (9, 13). Thus, the modern meat industry deserves the exploration of lipid composition from different biological sources through global profiling for the qualitative and quantitative characterization of individual lipid species (11).
During the latest decade, scientific expertise and technologies are constantly being developed with commercial or industrial aspects to advance the traceability and authentication of meat products and to address the safety concerns of the public, and for economic and quality reasons as well (14). Although meat from different species can be easily detected using deoxyribonucleic acid (DNA)-based techniques, the mixing of meat from different biological sources (e.g., geographical origins) is more difficult to detect. In this regard, MS- and nuclear magnetic resonance (NMR)-based lipidomics, such as both untargeted and targeted approaches, have been suggested as a promising strategy for this detection (15–17). The high-throughput untargeted analysis has the advantage of detecting lipid metabolites as comprehensively as possible by emphasizing the changes in quantity in biological importance (14, 15). Additionally, the targeted approach focuses on identifying and acquiring a number of specific fractions of known lipid species, for instance, FFAs, PLs, and cholesterol, in the presence of external chemical standards or available databases (18, 19). To achieve more persuasive results, the sample/lipid-extract preparation, chromatographic separation or direct-infusion MS (DI-MS, “shotgun lipidomics”), method validation, multivariate data processing, and bioinformatics evaluation have been well considered in the entire analytical platform (4, 15).
Exploratory analysis, classification/discriminant analysis, and regression analysis/prediction models have been proven to be useful in lipidomics data analysis. Apart from these chemometric tools or machine learning methods, however, bioinformatic validation should be crucial for determining the potential biomarkers of lipid species present in a biological system and providing molecular insights into alterations in energetic/metabolic pathways of lipid biosynthesis (4). Hence, in this short review, we examined the mainstream of recently published investigation available with discussions regarding the applications of MS-/NMR-based lipidomics in muscle foods, such as meat origin and adulteration identification, meat safety assessment, and dietary lipid nutrition during representative processing conditions and/or in vitro treatment.
Meat is originally skeletal muscles of livestock and thereby suffers from some factors in the livestock production system, mainly such as animal genetic/breed background, feeding types, geographical location, and environmental stress, particularly associated with spoilage developed by microbiological activity (20). As highlighted in literature evidence (Table 1), different efforts have been undertaken to achieve lipid-label validation depending on diagnostic and reliable features that can reflect the origins/authenticity of suspected muscles and their freshness status through the lipidomics strategy. The consequent lipid molecules that are inherently from muscle tissues or result from the metabolism of indigenous microorganisms vary among different species, such as beef (14, 18, 21–24), pork (24–27), sheep/goat (28), poultry (16), and marine products (29–32). To investigate the effect of genetic background, MALDI-TOF-MS and Phosphorus-31 NMR (31P NMR)-based lipidomics were successfully applied to capture the differences in fatty acid biosynthesis among German Simmental bulls fed with different diets. Consequently, TGs, phosphatidylethanolamines (PEs), phosphatidylcholine (PCs), phosphatidylinositol (PIs), cardiolipins (CLs), and cholesterol were identified as potential biomarkers (22). Furthermore, untargeted and targeted MS-based lipidomics based on the use of ultra-performance liquid chromatography (UPLC) coupled with high-resolution MS (HRMS) has shown good discriminative power between different species/breeds and feeding conditions toward extended global lipid information with appropriate multivariate data analysis (Table 1). A panel of lipids, particularly GLs [diacylglycerols (DAGs) and TAGs], lysophosphatidylcholines (LPCs), lysophosphatidylethanolamines (LPEs), and n-6 polyunsaturated fatty acids (PUFAs) have been screened out as specific markers for differentiation of animal diets (18, 21, 25, 28, 29). More intermuscular differences traceable properties in lipidomics can be evaluated as a function of geographical origin and for adulteration (Table 1). For instance, GLs [TGs and diglycerides (DGs)] and GPs were screened as main lipid biomarkers by principal component analysis (PCA) and partial least squares-discriminant analysis (PLS-DA) modeling through UPLC-Q-TOF-MS/MS approach for China’s domestic pork (from Tibetan, Jilin, and Sanmenxia black pigs), suggesting the difference in production systems, feeds and genetic backgrounds (26).
Table 1. Representative applications of MS-/NMR-based lipidomics in meat origin/adulteration identification, nutritional/microbial quality, and biological function.
It is also noticeable that exposure to microbes may significantly influence the lipid compositions during meat production where other indicators are usually involved, e.g., isotopic ratios and feeding (23). Particularly for marine products, such as fish, fatty acids, and GPs, metabolism is identified as the major pathway through microbial contamination during cutting, storage, and distribution processes after slaughter. A study was conducted to evaluate the spoilage of farmed Atlantic salmon (Salmo salar L.) during storage at 4°C for up to 15 days by adopting the UPLC-Q-Exactive-MS with high sensitivity (30). According to the results, the increase of LPC (C17:0) and LPC (C18:0) could result from the hydrolysis of PC (C18:4/C16:1) as a major freshness index. Assisted by “shotgun lipidomics,” PCs, PEs, PIs, phosphatidylserines (PSs), and sphingomyelins (SMs) were profiled as the lipid biomarkers of interest for the naturally spoiled muscle from Ctenopharyngodon idellus during room-temperature storage. UPLC-HRMS-based lipidomics has also shown good strength in identifying PCs, ceramides (CERs), and SLs metabolism as differentiated by pork and beef ground meat from different grades or due to death from diseases/abnormalities (24, 27). However, NMR-based lipidomics can screen more polar lipid metabolites and thus provides characteristic information on the metabolic profile of adulterated muscle. The presence of o-phosphocholine and a reduced level of Myo-inositol in turkey breast muscle injected with protein hydrolysates were observed through Proton NMR (1H NMR) untargeted lipidomics, suggesting the possible role of Myo-inositol deficiency in enhanced lipolysis (16, 33).
Up to date, various meat processing strategies, such as castration (34), thermal/non-thermal techniques (8, 17, 19, 35–38), freezing/thawing intervention (39), in vitro oxidation (40, 41), postmortem aging/storage (11, 42), modified atmosphere packaging (MAP) (42), and brining/drying-curing/preservatives treatments (43–47) have been implicated to improve the microbiological safety, color, flavor, and texture for the development of favorable meat products (Table 2). Accordingly, DI-MS and/or a combination of GC and liquid chromatography (GC/LC) and MS techniques have been utilized in the application of thermal processing to identify dozens of different lipids as potential biomarkers. Consequently, the lipolysis of TGs and PLs was noted as a strong flavor-binding precursors through different thermal degradation (boiling, steaming, and roasting) and showed specific losses of representative lipids (19, 35). It is worthwhile to note that fresh fish fillets, particularly Pleuronectes platessa upon high-pressure processing (HPP) were characterized by a distinctly high level of lipid-derived polar metabolites, serine-phosphoethanolamine species (Ser-PETA) (36). Specifically, studies on the discrimination of untreated/irradiated raw/ground meat from commercially produced goat, chicken, turkey, and pork through global lipid profile using UPLC-Q-Exactive-Orbitrap-MS/MS, targeted GC-resolved fatty acids composition, and chemometric tools (PCA and PLS-DA) have been recently reported (8, 37, 38). These authors observed γ-ray irradiation dose-dependent increase in docosahexaenoic acid (DHA)-enriched PC (C18:4/C22:6) + H in goat meat, while X-ray irradiation tended to result in n-3 PUFA-enriched lipids in chicken and turkey meat, accompanied with a noticeable accumulation of PLs and oxidized short- and long-chain FFAs. Comprehensive lipidomics based on GC-technique and phosphorus-31/carbon-13 NMR (31P/13C NMR) spectroscopy was devoted for hoki co-product (i.e., roe) treated with a pulsed electric field (PEF), where abundant PLs and a high level of lyso-diphosphatidylglycerols (LDPGs), LPEs, lysophosphatidylserines (LPSs), and LPCs were finally characterized, and PEF transformed more sn-2 phospholipid eicosapentaenoic acid (EPA) and DHA into sn-1,3 positions with potentially compromised bioavailability in terms of re-esterified structures (17). Additionally, when frozen Atlantic salmon and bullet tuna were thawed, an untargeted MS-based lipidomics approach detected abundant water-soluble phospholipid metabolites (i.e., L-α-glyceryl-phosphoryl-choline and N-methyl-ethanolamine phosphate) (39). However, hydroxyl radical (⋅OH) attacks can significantly alter the lipidomics profiles of shrimp and yak muscle to a large extent. PEs enriched in PUFAs were highly vulnerable to in vitro oxidation but both GPs metabolism and fatty acid biosynthesis were enriched during the subsequent deterioration and spoilage process of oxidized yak hindquarter meat (40, 41). On the other hand, PLs in porcine meat can undergo enzymatic hydrolysis during postmortem aging. For example, by employing targeted UPLC-TQ-MS/MS (with PLs as internal standards) to determine the PLs lipolysis tendency, researchers found that postmortem porcine loin (M. Longissimus) up to 21 days at 4°C was rich in PIs, PSs, and PAs, particularly C38:4 and C36:2 lipid species. Phospholipase A2 (PLA2) can be activated by postmortem calcium influx from the sarcoplasmic reticulum (SR) with a surge of LPCs in aging muscles (11). Apart from the targeted MS approach, untargeted “shotgun lipidomics” [electrospray ionization (ESI)-QTrap-MS/MS] became useful in identifying the lipid biomarkers derived from water-boiled dry-cured Pekin duck as a function of salting and ripening times (43, 44). In those studies, the findings pointed out that low-salt (< 6%) dry-cured duck significantly promoted the degradation of individual PLs (e.g., PCs, PGs, PEs, PSs, and PIs) probably resulting from a robust release of phospholipase, though some LPCs were damaged possibly due to oxidation and thermal degradation provided by boiling. In other cases, by combining the GC-system and untargeted hydrophilic interaction liquid chromatography (HILIC) coupled to QTrap-MS, saturated fatty acids (SFAs) (C16:0), monounsaturated fatty acids (MUFAs) (C18:1), and PUFAs (C18:2) were observed to be important lipid-derived flavor precursors in low-salted salmon and PCs content was kept at a high level even at 30% NaCl replacement rather than PSs (45). Following untargeted UPLC-QTrap-MS/MS lipidomics, salting/preservatives treatment of goat meat led to decrements in TGs concentration (47) while dry-curing of mutton ham (M. biceps femoris) significantly contributed to GLs, DGs, and specifically C20:3 and C18:4 FFAs released, showing characteristic metabolisms of GPs and SLs (46).
Table 2. The implications of MS-/NMR-based lipidomics in processed meat, quality control, and metabolism monitoring.
Lipids are inherently abundant in muscle tissues, which play critical roles in a series of cellular processes and physiological/biological activities (e.g., cell membrane architecture, cell signaling and energy-storing) (48). Breeds and feeding conditions, which usually have a large impact on animal physiology, determine the skeletal muscle growth and maturation, the final meat yield, and nutritional/flavor quality (21, 28, 29). NMR-/MS-based approaches have been attempted to obtain lipid-metabolite signatures and their relationship to physiological alteration in tissues as well as the potency in meat production. Differences in muscle energy metabolism, lipolysis in adipocytes, fat digestion/absorption, and cholesterol metabolism, β-oxidation of fatty acids, in particular, would offer the dynamics of redox status, oxidative stability, and consumer acceptability as influenced by the nutrients and ingredients in the animal feeds (20). For instance, concentrate-fed sheep are generally more obese compared with pasture-grazing sheep. High-fat diets may thereby promote the levels of isoleucine, lipids, glutamate, and 3-methylhistidine and lead to decreased citrate and glycerophosphorylcholines (GPCs) in animals (28). Consequently, as putatively revealed from lipidomics analysis, DAGs tend to accumulate in meat from concentrate-fed sheep/goats due to fat deposition, while some saturated TGs (e.g., C40:0, C42:0, and C44:0) in meats can be favored by pasture-grazing feeding strategy, showing a positive biological function of energy storage (28). PLs are the primary structural constituents of biological membranes and serve as critical nutrients owing to their physiological and nutritional properties. Following lipidomics, some species of fatty acid components and PLs have been useful markers in muscle tissues for differentiating breeds/dietary supplementation (21, 28, 29), detecting adulteration (27), and monitoring microbial accessibility (30–32). For instance, n-3 long-chain PUFA, such as EPA (C20:5n-3) and DHA (C22:6n-3) are essential fatty acids (EFA) for marine fish and crustaceans. An appropriate ratio of DHA/EPA feeding diet can improve the lipogenesis, integrity of membrane PLs, and DHA biosynthesis/deposition, meanwhile, inhibiting the mitochondrial β-oxidation of fatty acid and supporting growth performance in the hepatopancreas of S. paramamosain (29).
Lipids in the meat matrix are usually involved in thousands of metabolites that may be affected by species, nutrients, microbial diversity, production, and storage. These muscle-derived lipid metabolites are not only the phenotypic consequences of physiological muscle metabolism but also the major molecular basis for characterizing organoleptic components following different processing conditions (20, 49–51). For dry-cured meats, lipolysis usually involves a set of endogenous adipose tissue TGs lipases [e.g., neutral and basic ones including hormone-sensitive lipases (HSL) and lipoprotein lipases (LPL)] and some phospholipases (classified as A1, A2, C, and D) responsible for PLs degradation followed by auto-oxidation, which contributes to the formation of aromatic volatile compounds (10, 52, 53). However, we should note that the identification efficacy of bioactive lipid species through classical MS-based lipidomics approaches would be significantly affected by the applied lipid extraction protocols [e.g., liquid–liquid extraction (LLE), some alternative methods, such as methyl tert-butyl ether (MTBE)] mainly due to the characteristic amphipathic properties of lipids to achieve a differential partition (54, 55). In particular, the good recovery, ionization efficiency, and identification of global phospholipid species by LC-MS are still difficult to achieve, arising from their complexity in molecular structures (i.e., the length of the fatty acid chains and the difference in fatty acyl substitution at the glycerol backbone), and hydrophilicity across the entire chromatographic separation (28, 45, 56). Regarding the MS instruments showing high sensitivity, multi-sourced trace impurities that could result from biological matrices (e.g., remaining proteins in muscle tissues), solvents used for lipid extraction, preparation devices, such as siloxenes and phthalates, and even sample containers, such as plasticizers could be detected when they are carried to the lipid extract and thus these impurities may influence the reproducibility of the lipidomics profile (54). So, the extension of GC-system and NMR-based lipidomics and their combination with LC-resolved MS would exert unique superiority to enrich the entire lipid metabolism pathway (e.g., biosynthesis, oxidative decomposition, and enzymatic lipolysis) by detecting FAs, TGs, and sterols as well as some specific short and polar secondary lipid-metabolites (8, 16, 17, 19, 22). Indeed, during non-thermal processing, such as HPP, some important water-soluble lipid-metabolites (e.g., Ser-PETA) might be active in fatty acid transformation and participate in GPs metabolism (36). Overall, the lipolysis of TGs and PLs in meats are closely related to some relevant factors, mainly including (i) the feeding processes of animals (28, 29), (ii) circumstances in slaughtering (30, 31), (iii) postmortem aging (11, 42), (iv) thermal/non-thermal processing (8, 13, 17, 19, 35, 37, 57), and (v) brining/dry-ripening processes (45–47, 58). As a result, the physical/redox status of muscle/adipose tissues can be changed with the difference in bioavailability and bioactivity of endogenous and microbial lipases/phospholipases (10, 13, 52, 53, 59–63), consequently determining the fat deposition, lipolysis, and lipidomics profile (9, 20). In most cases, PLs are the main substrates for lipolysis in dry-cured meat products (10). However, some protein chaperones (heat shock protein 90, Hsp90) are reported to stabilize cell membranes and preserve membrane integrity in muscle tissues, and particularly act as an inherent antioxidant by providing additional protection against ROS-induced PLs oxidation (64). For seafood, such as in fish fillets, the total lipid content generally decreases during cold storage as TGs and PLs are either hydrolyzed by lipolytic enzymes, such as lipase and PLA2 and/or susceptible to oxidative damage from the myoglobin-mediated mechanism of action (39, 65, 66), though the activity of mitochondrial enzymes may be different during subsequent thawing. Indeed, the water-enriched external medium could induce hydrostatic pressure in cells and impair plasma membrane integrity, provoking an enrichment of intracellular enzymes in the final exudate (39). These knowledge of the adipose tissue TGs and PLs hydrolysis and oxidation during meat processing suggests a complicated overall lipid degradation mechanism and cellular protection under oxidative stress. A more comprehensive understanding based on multi-omics techniques is still required for improving both the quality and nutritional value of specific end products.
During the transition from “farm-to-fork” to the modern meat industry, the lipidomics disciplines successfully encompass a comprehensive and high-throughput understanding of meat composition, nutritional value, and safety with a combination of biochemical and mechanical mechanisms. Overall, the techniques for lipidomics have been steadily progressing, particularly regarding the omics-data-mining and multivariate statistical analyses, whereby new efforts are contributed toward new algorithms of developed prediction models for identified lipid biomarkers. Untargeted MS-/NMR-based lipidomics gives molecular insight into meat origin/adulteration and microbial safety with more tentative lipid markers being screened out on a global scale, though additional targeted analytes (e.g., the lipolysis fate of PLs resulting from foodborne microbe bred in muscle) still require further validation in their adulteration detection. In addition, the exhaustive analysis of lipids and their alterations during meat production favors the selective design of processing methods for specific muscle matrices (e.g., irradiation and PEF). Many putative lipid biomarkers following computational approaches and possible metabolism pathways enriched by bioinformatics provide valuable suggestions on food safety and health concerns regarding their potential during the treatment with preservatives, fermentation, aging, and storage. However, challenges remain due to the complexity of meat lipidome, the nature of key intermediate lipid-metabolites, and their evaluation concerning the quality and nutritional value of the final product.
CL and BO-K performed literature review, analyzed and interpreted the data, and drafted the manuscript. CL, BO-K, and GJ reviewed the first draft and revised the manuscript accordingly. All authors contributed to the article and approved the final submitted version.
This research was supported by the National Natural Science Foundation of China (No. 31871824).
The authors declare that the research was conducted in the absence of any commercial or financial relationships that could be construed as a potential conflict of interest.
All claims expressed in this article are solely those of the authors and do not necessarily represent those of their affiliated organizations, or those of the publisher, the editors and the reviewers. Any product that may be evaluated in this article, or claim that may be made by its manufacturer, is not guaranteed or endorsed by the publisher.
1. OECD/Food and Agriculture Organization of the United Nations. “Meat”, in OECD-FAO Agricultural Outlook 2021-2030. Paris: OECD Publishing (2021). doi: 10.1787/cf68bf79-en
2. De Smet S, Vossen E. Meat: the balance between nutrition and health. A review. Meat Sci. (2016) 120:145–56. doi: 10.1016/j.meatsci.2016.04.008
3. Chen Y, Jia X, Sun F, Jiang S, Liu H, Liu Q, et al. Using a stable pre-emulsified canola oil system that includes porcine plasma protein hydrolysates and oxidized tannic acid to partially replace pork fat in frankfurters. Meat Sci. (2020) 160:107968. doi: 10.1016/j.meatsci.2019.107968
4. Han X, Ye H. Overview of lipidomic analysis of triglyceride molecular species in biological lipid extracts. J Agric Food Chem. (2021) 69:8895–909. doi: 10.1021/acs.jafc.0c07175
5. Jia W, Di C, Zhang R, Shi L. Application of liquid chromatography mass spectrometry-based lipidomics to dairy products research: an emerging modulator of gut microbiota and human metabolic disease risk. Food Res Int. (2022) 157:111206. doi: 10.1016/j.foodres.2022.111206
6. Fahy E, Cotter D, Sud M, Subramaniam S. Lipid classification, structures and tools. Biochim Biophys Acta Mol Cell Biol Lipids. (2011) 1811:637–47. doi: 10.1016/j.bbalip.2011.06.009
7. Liu Z, Rochfort S, Cocks B. Milk lipidomics: what we know and what we don’t. Prog Lipid Res. (2018) 71:70–85. doi: 10.1016/j.plipres.2018.06.002
8. Chiesa LM, Di Cesare F, Mosconi G, Pavlovic R, Campaniello M, Tomaiuolo M, et al. Lipidomics profile of irradiated ground meat to support food safety. Food Chem. (2022) 375:131700. doi: 10.1016/j.foodchem.2021.131700
9. Wood JD, Enser M, Fisher AV, Nute GR, Sheard PR, Richardson RI, et al. Fat deposition, fatty acid composition and meat quality: a review. Meat Sci. (2008) 78:343–58. doi: 10.1016/j.meatsci.2007.07.019
10. Wang D, Zhang M, Bian H, Xu W, Xu X, Zhu Y, et al. Changes of phospholipase A2 and C activities during dry-cured duck processing and their relationship with intramuscular phospholipid degradation. Food Chem. (2014) 145:997–1001. doi: 10.1016/j.foodchem.2013.09.007
11. Chao MD, Donaldson EA, Wu W, Welter AA, O’Quinn TG, Hsu WW, et al. Characterizing membrane phospholipid hydrolysis of pork loins throughout three aging periods. Meat Sci. (2020) 163:108065. doi: 10.1016/j.meatsci.2020.108065
12. Merrill AH Jr, Schmelz EM, Wang E, Schroeder JJ, Dillehay DL, Riley RT. Role of dietary sphingolipids and inhibitors of sphingolipid metabolism in cancer and other diseases. J Nutr. (1995) 125:1677S–82S. doi: 10.1093/jn/125.suppl_6.1677S
13. Li C, He L, Jin G, Ma S, Wu W, Gai L. Effect of different irradiation dose treatment on the lipid oxidation, instrumental color and volatiles of fresh pork and their changes during storage. Meat Sci. (2017) 128:68–76. doi: 10.1016/j.meatsci.2017.02.009
14. Man KY, Chan CO, Tang HH, Dong NP, Capozzi F, Wong KH, et al. Mass spectrometry-based untargeted metabolomics approach for differentiation of beef of different geographic origins. Food Chem. (2021) 338:127847. doi: 10.1016/j.foodchem.2020.127847
15. Wu B, Wei F, Xu S, Xie Y, Lv X, Chen H, et al. Mass spectrometry-based lipidomics as a powerful platform in foodomics research. Trends Food Sci Technol. (2021) 107:358–76. doi: 10.1016/j.tifs.2020.10.045
16. Wagner L, Peukert M, Kranz B, Gerhardt N, Andr S, Busch U, et al. Comparison of targeted (HPLC) and nontargeted (GC-MS and NMR) approaches for the detection of undeclared addition of protein hydrolysates in Turkey breast muscle. Foods. (2020) 9:1084. doi: 10.3390/foods9081084
17. Ahmmed MK, Carne A, Tian HS, Bekhit AEDA. The effect of pulsed electric fields on the extracted total lipid yield and the lipidomic profile of hoki roe. Food Chem. (2022) 384:132476. doi: 10.1016/j.foodchem.2022.132476
18. Artegoitia VM, Foote AP, Lewis RM, Freetly HC. Metabolomics profile and targeted lipidomics in multiple tissues associated with feed efficiency in beef steers. ACS Omega. (2019) 4:3973–82. doi: 10.1021/acsomega.8b02494
19. Liu H, Hui T, Zheng X, Li S, Wei X, Li P, et al. Characterization of key lipids for binding and generating aroma compounds in roasted mutton by UPLC-ESI-MS/MS and Orbitrap Exploris GC. Food Chem. (2022) 374:131723. doi: 10.1016/j.foodchem.2021.131723
20. Muroya S, Ueda S, Komatsu T, Miyakawa T, Ertbjerg P. MEATabolomics: muscle and meat metabolomics in domestic animals. Metabolites. (2020) 10:188. doi: 10.3390/metabo10050188
21. Gu X, Sun W, Yi K, Yang L, Chi F, Luo Z, et al. Comparison of muscle lipidomes between cattle-yak, yak, and cattle using UPLC–MS/MS. J Food Compos Anal. (2021) 103:104113. doi: 10.1016/j.jfca.2021.104113
22. Dannenberger D, Süss R, Teuber K, Fuchs B, Nuernberg K, Schiller J. The intact muscle lipid composition of bulls: an investigation by MALDI-TOF MS and 31P NMR. Chem Phys Lipids. (2010) 163:157–64. doi: 10.1016/j.chemphyslip.2009.10.011
23. Wang K, Xu L, Wang X, Chen A, Xu Z. Discrimination of beef from different origins based on lipidomics: a comparison study of DART-QTOF and LC-ESI-QTOF. LWT Food Sci Technol. (2021) 149:1–10. doi: 10.1016/j.lwt.2021.111838
24. Trivedi DK, Hollywood KA, Rattray NJW, Ward H, Trivedi DK, Greenwood J, et al. Meat, the metabolites: an integrated metabolite profiling and lipidomics approach for the detection of the adulteration of beef with pork. Analyst. (2016) 141:2155–64. doi: 10.1039/c6an00108d
25. Zhang Z, Liao Q, Sun Y, Pan T, Liu S, Miao W, et al. Lipidomic and transcriptomic analysis of the longissimus muscle of Luchuan and Duroc pigs. Front Nutr. (2021) 8:667622. doi: 10.3389/fnut.2021.667622
26. Mi S, Shang K, Li X, Zhang CH, Liu JQ, Huang DQ. Characterization and discrimination of selected China’s domestic pork using an LC-MS-based lipidomics approach. Food Control. (2019) 100:305–14. doi: 10.1016/j.foodcont.2019.02.001
27. Cao M, Han Q, Zhang J, Zhang R, Wang J, Gu W, et al. An untargeted and pseudotargeted metabolomic combination approach to identify differential markers to distinguish live from dead pork meat by liquid chromatography–mass spectrometry. J Chromatogr A. (2020) 1610:460553. doi: 10.1016/j.chroma.2019.460553
28. Wang J, Xu Z, Zhang H, Wang Y, Liu X, Wang Q, et al. Meat differentiation between pasture-fed and concentrate-fed sheep/goats by liquid chromatography quadrupole time-of-flight mass spectrometry combined with metabolomic and lipidomic profiling. Meat Sci. (2021) 173:108374. doi: 10.1016/j.meatsci.2020.108374
29. Wang X, Jin M, Cheng X, Hu X, Zhao M, Yuan Y, et al. Hepatopancreas transcriptomic and lipidomic analyses reveal the molecular responses of mud crab (Scylla paramamosain) to dietary ratio of docosahexaenoic acid to eicosapentaenoic acid. Aquaculture. (2022) 551:737903. doi: 10.1016/j.aquaculture.2022.737903
30. Chen J, Kong Q, Sun Z, Liu J. Freshness analysis based on lipidomics for farmed Atlantic salmon (Salmo salar L.) stored at different times. Food Chem. (2022) 373:131564. doi: 10.1016/j.foodchem.2021.131564
31. Chen Y, Ning Q, Wang S, Chen Y, Mo X, Liang P, et al. Effects of cold treatments on lipidomics profiles of large yellow croaker (Larimichthys crocea) fillets by UPLC-Q-Exactive Orbitrap MS analysis. J Food Compos Anal. (2022) 109:104481. doi: 10.1016/j.jfca.2022.104481
32. Wang Y, Zhang H. Tracking phospholipid profiling of muscle from Ctennopharyngodon idellus during storage by shotgun lipidomics. J Agric Food Chem. (2011) 59:11635–42. doi: 10.1021/jf2030852
33. Hayashi E, Maeda T, Hasegawa R, Tomita T. The effect of myo-inositol deficiency on lipid metabolism in rats: III. The mechanism of an enhancement in lipolysis due to myo-inositol deficiency in rats. Biochim Biophys Acta Lipids Lipid Metab. (1978) 531:197–205. doi: 10.1016/0005-2760(78)90143-1
34. Li J, Tang C, Zhao Q, Yang Y, Li F, Qin Y, et al. Integrated lipidomics and targeted metabolomics analyses reveal changes in flavor precursors in psoas major muscle of castrated lambs. Food Chem. (2020) 333:127451. doi: 10.1016/j.foodchem.2020.127451
35. Jia W, Li R, Wu X, Liu S, Shi L. UHPLC-Q-Orbitrap HRMS-based quantitative lipidomics reveals the chemical changes of phospholipids during thermal processing methods of Tan sheep meat. Food Chem. (2021) 360:130153. doi: 10.1016/j.foodchem.2021.130153
36. Castrica M, Pavlovic R, Balzaretti CM, Curone G, Brecchia G, Copelotti E, et al. Effect of high-pressure processing on physico-chemical, microbiological and sensory traits in fresh fish fillets (Salmo salar and Pleuronectes platessa). Foods. (2021) 10:1775. doi: 10.3390/foods10081775
37. Jia W, Shi Q, Shi L. Effect of irradiation treatment on the lipid composition and nutritional quality of goat meat. Food Chem. (2021) 351:129295. doi: 10.1016/j.foodchem.2021.129295
38. Panseri S, Arioli F, Pavlovic R, Di Cesare F, Nobile M, Mosconi G, et al. Impact of irradiation on metabolomics profile of ground meat and its implications toward food safety. LWT. (2022) 161:113305. doi: 10.1016/j.lwt.2022.113305
39. Chiesa LM, Pavlovic R, Nobile M, Di Cesare F, Malandra R, Pessina D, et al. Discrimination between fresh and frozen-thawed fish involved in food safety and fraud protection. Foods. (2020) 9:1–15. doi: 10.3390/foods9121896
40. Tu CH, Qi XE, Shui SS, Lin HM, Benjakul S, Zhang B. Investigation of the changes in lipid profiles induced by hydroxyl radicals in whiteleg shrimp (Litopenaeus vannamei) muscle using LC/MS-based lipidomics analysis. Food Chem. (2022) 369:130925. doi: 10.1016/j.foodchem.2021.130925
41. Huang Q, Dong K, Wang Q, Huang X, Wang G, An F, et al. Changes in volatile flavor of yak meat during oxidation based on multi-omics. Food Chem. (2022) 371:131103. doi: 10.1016/j.foodchem.2021.131103
42. Dyer JM, Deb-Choudhury S, Cornellison CD, Krsinic G, Dobbie P, Rosenvold K, et al. Spatial and temporal mass spectrometric profiling and imaging of lipid degradation in bovine M. longissimus dorsi lumborum. J Food Compos Anal. (2014) 33:203–9. doi: 10.1016/j.jfca.2013.12.001
43. Li C, Li X, Huang Q, Zhuo Y, Xu B, Wang Z. Changes in the phospholipid molecular species in water-boiled salted duck during processing based on shotgun lipidomics. Food Res Int. (2020) 132:109064. doi: 10.1016/j.foodres.2020.109064
44. Li C, Li X, Huang Q, Zhou Y, Xu B, Wang Z. Influence of salt content used for dry-curing on lipidomic profiles during the processing of water-boiled salted duck. J Agric Food Chem. (2020) 68:4017–26. doi: 10.1021/acs.jafc.0c01513
45. Wang J, Wang H, Lu W, Zhang M, Xue J, Yu X, et al. Low-salted salmon: effects of salt reduction on physicochemical, lipidomic, and sensory characteristics. LWT. (2021) 152:112311. doi: 10.1016/j.lwt.2021.112311
46. Guo X, Shi D, Liu C, Huang Y, Wang Q, Wang J, et al. UPLC-MS-MS-based lipidomics for the evaluation of changes in lipids during dry-cured mutton ham processing. Food Chem. (2022) 377:131977. doi: 10.1016/j.foodchem.2021.131977
47. Jia W, Wu X, Zhang R, Shi L. UHPLC-Q-Orbitrap-based lipidomics reveals molecular mechanism of lipid changes during preservatives treatment of Hengshan goat meat sausages. Food Chem. (2022) 369:130948. doi: 10.1016/j.foodchem.2021.130948
48. Corino C, Mourot J, Magni S, Pastorelli G, Rosi F. Influence of dietary conjugated linoleic acid on growth, meat quality, lipogenesis, plasma leptin and physiological variables of lipid metabolism in rabbits. J Anim Sci. (2002) 80:1020–8. doi: 10.2527/2002.8041020x
49. Xu L, Zheng Y, Zhou C, Pan D, Geng F, Cao J, et al. Kinetic response of conformational variation of duck liver globular protein to ultrasonic stimulation and its impact on the binding behavior of n-alkenals. LWT. (2021) 150:111890. doi: 10.1016/j.lwt.2021.111890
50. Pateiro M, Domínguez R, Munekata PES, Barba FJ, Lorenzo JM. Lipids and fatty acids. In: FJ Barba, JMA Saraiva, G Cravotto, JM Lorenzo editors. Innovative Thermal and Non-Thermal Processing, Bioaccessibility and Bioavailability of Nutrients and Bioactive Compounds. Cambridge: Woodhead Publishing (2019). p. 107–37.
51. Xia Q, Zheng Y, Liu Z, Cao J, Chen X, Liu L, et al. Nonthermally driven volatilome evolution of food matrices: the case of high pressure processing. Trends Food Sci Technol. (2020) 106:365–81. doi: 10.1016/j.tifs.2020.10.026
52. Toldrá F. Proteolysis and lipolysis in flavour development of dry-cured meat products. Meat Sci. (1998) 49:S101–10. doi: 10.1016/s0309-1740(98)90041-9
53. Xiao S, Zhang W, Yang Y, Ma C, Ahn DU, Li X, et al. Changes of hormone-sensitive lipase (HSL), adipose tissue triglyceride lipase (ATGL) and free fatty acids in subcutaneous adipose tissues throughout the ripening process of dry-cured ham. Food Chem. (2010) 121:191–5. doi: 10.1016/j.foodchem.2009.12.029
54. Aldana J, Romero-Otero A, Cala MP. Exploring the lipidome: current lipid extraction techniques for mass spectrometry analysis. Metabolites. (2020) 10:231. doi: 10.3390/metabo10060231
55. Zhou J, Wang M, Saraiva JA, Martins AP, Pinto CA, Prieto MA, et al. Extraction of lipids from microalgae using classical and innovative approaches. Food Chem. (2022) 384:132236. doi: 10.1016/j.foodchem.2022.132236
56. Shen Q, Dai Z, Huang YW, Cheung HY. Lipidomic profiling of dried seahorses by hydrophilic interaction chromatography coupled to mass spectrometry. Food Chem. (2016) 205:89–96. doi: 10.1016/j.foodchem.2016.02.151
57. Jadhav HB, Annapure US, Deshmukh RR. Non-thermal technologies for food processing. Front Nutr. (2021) 8:657090. doi: 10.3389/fnut.2021.657090
58. Chiesa L, Panseri S, Bonacci S, Procopio A, Zecconi A, Arioli F, et al. Authentication of Italian PDO lard using NIR spectroscopy, volatile profile and fatty acid composition combined with chemometrics. Food Chem. (2016) 212:296–304. doi: 10.1016/j.foodchem.2016.05.180
59. Petrón MJ, Muriel E, Timón ML, Martín L, Antequera T. Fatty acids and triacylglycerols profiles from different types of Iberian dry-cured hams. Meat Sci. (2004) 68:71–7. doi: 10.1016/j.meatsci.2004.01.012
60. Zhou GH, Zhao GM. Biochemical changes during processing of traditional Jinhua ham. Meat Sci. (2007) 77:114–20. doi: 10.1016/j.meatsci.2007.03.028
61. Siciliano C, Belsito E, De Marco R, Di Gioia ML, Leggio A, Liguori A. Quantitative determination of fatty acid chain composition in pork meat products by high resolution 1H NMR spectroscopy. Food Chem. (2013) 136:546–54. doi: 10.1016/j.foodchem.2012.08.058
62. Ying W, Ya-Ting J, Jin-Xuan C, Yin-Ji C, Yang-Ying S, Xiao-Qun Z, et al. Study on lipolysis-oxidation and volatile flavour compounds of dry-cured goose with different curing salt content during production. Food Chem. (2016) 190:33–40. doi: 10.1016/j.foodchem.2015.05.048
63. Pan J, Zhao S, He L, Zhang M, Li C, Huang S, et al. Promotion effect of salt on intramuscular neutral lipid hydrolysis during dry-salting process of porcine (biceps femoris) muscles by inducing phosphorylation of ATGL, HSL and their regulatory proteins of perilipin1, ABHD5 and G0S2. Food Chem. (2022) 373:131597. doi: 10.1016/j.foodchem.2021.131597
64. Zhang M, Wang D, Geng Z, Li P, Sun Z, Xu W. Effect of heat shock protein 90 against ROS-induced phospholipid oxidation. Food Chem. (2018) 240:642–7. doi: 10.1016/j.foodchem.2017.08.005
65. Wu H, Richards MP, Undeland I. Lipid oxidation and antioxidant delivery systems in muscle food. Compr Rev Food Sci Food Saf. (2022) 21:1275–99. doi: 10.1111/1541-4337.12890
Keywords: meat lipidomics, mass spectrometry, nuclear magnetic resonance, lipid biomarkers, lipolysis, biosynthesis, meat processing, nutritional value
Citation: Li C, Ozturk-Kerimoglu B, He L, Zhang M, Pan J, Liu Y, Zhang Y, Huang S, Wu Y and Jin G (2022) Advanced Lipidomics in the Modern Meat Industry: Quality Traceability, Processing Requirement, and Health Concerns. Front. Nutr. 9:925846. doi: 10.3389/fnut.2022.925846
Received: 22 April 2022; Accepted: 02 May 2022;
Published: 27 May 2022.
Edited by:
Qiang Xia, Ningbo University, ChinaReviewed by:
Haizhou Wu, Chalmers University of Technology, SwedenCopyright © 2022 Li, Ozturk-Kerimoglu, He, Zhang, Pan, Liu, Zhang, Huang, Wu and Jin. This is an open-access article distributed under the terms of the Creative Commons Attribution License (CC BY). The use, distribution or reproduction in other forums is permitted, provided the original author(s) and the copyright owner(s) are credited and that the original publication in this journal is cited, in accordance with accepted academic practice. No use, distribution or reproduction is permitted which does not comply with these terms.
*Correspondence: Guofeng Jin, amdmQG1haWwuaHphdS5lZHUuY24=
†Present address: Chengliang Li, Instituto de Agroquímica y Tecnología de Alimentos (IATA-CSIC), Paterna, Spain
Disclaimer: All claims expressed in this article are solely those of the authors and do not necessarily represent those of their affiliated organizations, or those of the publisher, the editors and the reviewers. Any product that may be evaluated in this article or claim that may be made by its manufacturer is not guaranteed or endorsed by the publisher.
Research integrity at Frontiers
Learn more about the work of our research integrity team to safeguard the quality of each article we publish.