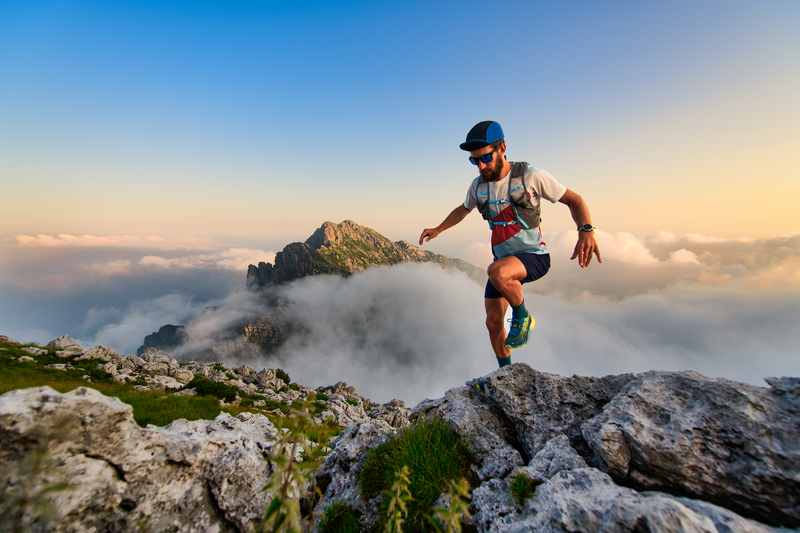
95% of researchers rate our articles as excellent or good
Learn more about the work of our research integrity team to safeguard the quality of each article we publish.
Find out more
REVIEW article
Front. Nutr. , 18 July 2022
Sec. Clinical Nutrition
Volume 9 - 2022 | https://doi.org/10.3389/fnut.2022.924036
This article is part of the Research Topic Breast Milk Composition and Infant Metabolism View all 14 articles
Bronchopulmonary dysplasia (BPD) is a severe chronic lung illness that affects neonates, particularly premature infants. It has far-reaching consequences for infant health and their families due to intractable short- and long-term repercussions. Premature infant survival and long-term quality of life are severely harmed by BPD, which is characterized by alveolarization arrest and hypoplasia of pulmonary microvascular cells. BPD can be caused by various factors, with oxidative stress (OS) being the most common. Premature infants frequently require breathing support, which results in a hyperoxic environment in the developing lung and obstructs lung growth. OS can damage the lungs of infants by inducing cell death, inhibiting alveolarization, inducing inflammation, and impairing pulmonary angiogenesis. Therefore, antioxidant therapy for BPD relieves OS and lung injury in preterm newborns. Many antioxidants have been found in human milk, including superoxide dismutase, glutathione peroxidase, glutathione, vitamins, melatonin, short-chain fatty acids, and phytochemicals. Human milk oligosaccharides, milk fat globule membrane, and lactoferrin, all unique to human milk, also have antioxidant properties. Hence, human milk may help prevent OS injury and improve BPD prognosis in premature infants. In this review, we explored the role of OS in the pathophysiology of BPD and related signaling pathways. Furthermore, we examined antioxidants in human milk and how they could play a role in BPD to understand whether human milk could prevent and treat BPD.
BPD is the most prevalent complication in preterm infants. In 1967, Northway et al. first defined BPD as a persistent lung injury caused by a high concentration of oxygen and high pressures during mechanical ventilation. “Classical BPD” or “old BPD” is diagnosed in “those newborns who require supplementary oxygen on post-natal day 28” and those who display radiographic abnormalities in the chest, such as emphysema, atelectasis, and vesical shadows (1). The survival rate of premature infants has improved dramatically in the decades since BPD was identified, owing to a substantial increase in the quality of nursing for premature infants, such as the development of non-invasive ventilation techniques and prenatal use of corticosteroids for increased alveolar surface area. However, the incidence of BPD remains relatively high. Moreover, the advancement of these tools has increased our understanding of BPD; the definition of “old BPD” is no longer appropriate for current diagnostic criteria.
In 2001, Jobe et al. updated the diagnostic criteria and definition of BPD (NIH), also known as “New BPD”: infants with oxygen dependence (inhaled oxygen concentration [FiO2] >21%) for >28 days should be diagnosed with BPD, and classified as mild, moderate, severe BPD based on aerobic conditions at 36 week post-menstrual age (PMA). The new BPD highlights the link between alveolar dysplasia and lung injury in preterm infants (2, 3). In 2018, the National Institute of Child Health and Human Development modified its rules, removing the need for oxygen dependence for >28 days (4). In 2019, Jensen et al. proposed that the degree of oxygen reliance should not be considered when diagnosing BPD; instead, the mode of assisted breathing should be considered (5). For clinicians, an accurate diagnosis of BPD would allow the development of individualized respiratory support measures and medication therapy, which are advantageous for the rehabilitation and prognosis of patients with BPD.
Between 2010 and 2019, the incidence of BPD among extremely premature newborns in China was >74%; the younger the PMA, the higher the incidence of BPD (6). However, the treatment of BPD is controversial. A worse prognosis usually accompanies a higher disease severity. Currently, the most common treatment options for BPD are delivery room intervention (7, 8), invasive mechanical ventilation (9, 10), steroids (11), diuretics (12), bronchodilators (13), and caffeine (14, 15). Furthermore, dietary support, infection management, and vasodilators are beneficial as therapy for BPD (16). The importance of OS in the etiology of BPD has been increasingly recognized, and the use of antioxidants in the treatment of BPD is becoming a popular research topic. Reducing the damage caused by oxidative free radicals to the lungs of preterm infants by maintaining the balance between oxidation and antioxidant systems is expected to provide a new method for preventing and treating BPD (17, 18).
Human milk is the most comprehensive and natural nourishment for infant growth. It contains all the nutrients and biologically active components that newborns require to meet their developmental demands and boost their immunity. Breast milk contains various antioxidants, such as glutathione (GSH), superoxide dismutase (SOD), glutathione peroxidase (GSH-Px), vitamins, phytochemicals, melatonin, probiotics, short-chain fatty acids (SCFAs), and unique human milk oligosaccharides(HMOs), milk fat globule membrane(MFGM), and lactoferrin, which provide a solid antioxidant capacity for newborns. A meta-analysis on breastfeeding and BPD found that the incidence of BPD decreased when premature newborns were exclusively breastfed compared to when they were formula fed (19). In a cohort study, the amount of human milk consumed by premature newborns was inversely associated with the incidence of BPD, with a daily intake of 7 ml/(kg·d) showing a BPD prevention effect (20). Aloka et al. found that providing sufficient nutritional care to premature children with low birth weight is significant for preventing BPD. For every 10% increase in breastfeeding in preterm children from birth to 36 weeks PMA, the risk of BPD is lowered by 9.5% (21). Breastfeeding also reduces the risk of newborn problems in the neonatal intensive care unit (NICU) (22). Yan et al. discovered that a daily intake of 50 ml/(kg·d) of human milk from the mother during the first 4 weeks after delivery can minimize the incidence of BPD in infants (23). According to these studies, human milk appears to serve a beneficial function in the prevention and treatment of BPD. Given the presence of different antioxidants in breast milk, we believe that this is the primary mechanism through which human milk contributes to decreased BPD. Therefore, this review examined the critical role of OS in BPD and the impact of antioxidants in human milk on the prevention and treatment of BPD. We have also discussed future considerations for clinical BPD treatment.
OS is a condition in which there is an imbalance between reactive oxygen species (ROS) production and antioxidant capability. Under normal conditions, electrons created by the aerobic metabolism of cells are readily accepted by oxygen, resulting in ROS production. Excessive levels of ROS can harm cell structure, proteins, lipids, and nucleic acids, leading to cell death (24). The factors that influence the results of cell injury include interaction between specific molecules, the body's internal environment, cell location, time, and concentration (25). Premature newborns are more likely to develop BPD, especially those who require mechanical ventilation (Figure 1). Owing to respiratory system immaturity and pulmonary hypoplasia, premature infants require long-term oxygen therapy, which can expose them to a greater risk of illness and inflammation than term infants. They are also more likely to accumulate ROS due to high concentration oxygen dependence. Additionally, the endogenous antioxidant enzyme systems are defective in premature infants. SOD, catalase (CAT), and GPX concentrations at birth are substantially lower in premature newborns than in full-term infants; therefore, they are unable to effectively eliminate excess ROS, resulting in ROS accumulation and OS (26).
OS can cause several lung injuries. Long-term hyperoxia in the lungs of premature newborns causes lung damage directly through ROS production (27). Alveolar epithelium type II cells (AEC II) are the most critical cells in the lung development stage and act as lung tissue stem cells. AEC II synthesizes and secretes pulmonary surfactant, regulates lung tissue growth, repairs wounds, and adjusts alveolar moisture to maintain homeostasis. AEC II can develop into alveolar epithelial type I cells to repair the alveolar wall and preserve alveolar function when the alveolar–capillary barrier is damaged. A high concentration of oxygen can induce the apoptosis of AEC II and inhibit their proliferation. Injury to airway epithelial cells causes pulmonary tracheal remodeling and vascular remodeling, both of which are important in BPD etiology (28, 29). Airway remodeling occurs throughout infant lung development via several pathogenic processes involving airway epithelial cells, inflammatory cells, smooth muscle cells, and extracellular matrix. The leading causes of airway remodeling are aberrant airway healing and airway epithelial damage caused by decreased AEC II levels (30).
In addition, endoplasmic reticulum stress (ES) in the lungs can be exacerbated by OS in hyperoxic environments (31). Hyperoxia exposure can harm mitochondrial activity in the lungs, resulting in ES and alveolar injury, activating the pulmonary unfolded protein response (UPR), and increasing UPR downstream effectors, in turn resulting in alveolar growth disorder (32).
Alveolarization (production of alveolar gas exchange units) can be impeded in newborns with BPD, although the mechanisms of alveolarization are unknown (33). Pulmonary alveoli are important gas exchange units, and alveolarization is a late goal in lung development. The terminal airspaces formed during early lung development are divided by secondary septation, resulting in an increasing number of smaller alveoli, thus improving gas exchangeability in the lungs. However, premature newborns with developed lungs are exposed to hyperoxia-obstructed gas exchange with continuous alternation of the bronchial, resulting in lung function disorder. In a previous study, neonatal mice treated with high oxygen (85% O2) showed delayed alveolar growth, loss of bronchoalveolar attachment, increased lung compliance, and increased medial arteriole wall thickness, which persisted into adulthood. Alveolar defects have also been linked to reduced functional gas exchange (34).
Supplemental oxygen levels influence the severity of alterations in airway anatomy and function in neonates. Mild (40% O2) and moderate (60% O2) oxygen exposure can cause alterations in airway function and hyperresponsiveness in animals, but the effects are minor. The degree of OS is directly associated with interrupted alveolarization due to severely high oxygen (80% O2) levels, which cause simplified alveolar, airway embolization, and elastin redistribution (35). Even a short period of hyperoxia lung injury (limited to cystic lung development) is sufficient to damage alveolar growth and reduce gas exchange function; irrespective of whether confined to the cystic period or chronic fetal hyperoxia, it will lead to reduced alveolar surface area volume and alveolar interval (36). Hyperoxia obstructs alveolarization, which is associated with mitochondrial malfunction. Both hyperoxia and direct suppression of mitochondrial oxidative phosphorylation can halt alveolar development. Long-term mechanical breathing inhibits the formation of pulmonary mitochondria, and this inhibition of oxidative phosphorylation persists throughout alveolar evolution, resulting in neonatal alveolar development delays (37, 38).
OS also impairs pulmonary angiogenesis. Pulmonary vascular abnormalities are another critical factor in BPD development; several growth factors play a role in pulmonary angiogenesis. The vascular endothelial growth factor (VEGF) can promote vascular endothelial cell proliferation and migration and reshaping of endothelial cells (39). VEGF also plays a crucial role in lung development by maintaining the typical structure and function of the alveoli during normal blood vessel development (40). OS caused by ROS accumulation has been confirmed to decrease VEGF expression in mouse models, and abnormal VEGF signal transduction can impair angiogenesis and reduce alveolarization, resulting in the arrest of pulmonary vascular development and leading to experimental BPD (41). Studies have shown that both VEGF expression and lung capillary density are significantly decreased in patients with BPD and animal models and that increased VEGF expression can inhibit alveolar destruction induced by high oxygen levels (42). The combination of mesenchymal stem cells (MSCs) and erythropoietin has been shown to enhance lung protection in newborn mice under high-oxygen conditions. Increased expression of VEGF was found to alleviate lung injury in newborn BPD mice by indirectly promoting angiogenesis (43). Wallace et al., using anti-sF1t-monoclonal antibody therapy found that sF1t, an endogenous antagonist of VEGF, could prevent structural abnormalities in lung development and pulmonary hypertension in infancy and effectively preserve lung structure and function (44). Thus, early endothelial cell injury damages pulmonary blood vessels and alveolar formation. In addition, downregulation of VEGF expression further disrupts lung epithelial and mesenchymal development. Premature infants, born with developed lungs and long-term exposure to high oxygen demand, develop ROS accumulation, resulting in OS and reduced VEGF expression. Under hyperoxia, reduced levels of VEGF cannot effectively induce pulmonary angiogenesis and repair, and pulmonary angiogenesis ceases during germination. Insufficient angiogenesis leads to pulmonary vascular dysplasia and prevents alveolarization, eventually leading to BPD (45).
Nuclear erythroid-E2-related factor 2 (Nrf2) is a major transcription factor involved in the antioxidant system that regulates OS. Nrf2 interacts with Keap1 in the cytoplasm and is destroyed by ubiquitination under normal conditions. The accumulation of ROS prevents Nrf2 from being ubiquitinated by modifying the cysteine in Keap1, allowing Nrf2 to be expressed stably in the nucleus and activating the antioxidant reaction elements (ARE), which include the expression of a series of antioxidant genes such as GSH, GCL, HMOX, and GSL (46). The oxidative equilibrium maintained by Nrf2 is especially crucial to the respiratory tract after long-term exposure to hyperoxia in the lungs of preterm newborns. Lack of Nrf2 can increase the sensitivity and severity of different respiratory conditions such as BPD, adult respiratory distress syndrome, chronic obstructive pulmonary disease, asthma, and lung cancer, whereas activation of Nrf2 protects against various respiratory diseases (47). The Nrf2-Keap1-ARE pathway is one of the most crucial protective mechanisms against BPD, probiotics, SCFAs, lactoferrin, arginine can activate this pathway and show their antioxidative effects. The pulmonary tissue structure of mice exposed to hyperoxia becomes disordered, the alveolar wall begins to weaken as the hyperoxia exposure period increases, and the concentration of antioxidative stress-related enzymes (CAT, SOD, and GSH-Px) decreases under hypoxia condition. The Nrf2 content is significantly higher in hyperoxic mice than in normal oxygen-treated mice. In contrast, the level of Keap1 is lower, indicating that the body can upregulate Nrf2 expression by inhibiting Keap1 expression in hyperoxic mice; subsequently, Nrf2 enters the nucleus to activate ARE and improve antioxidant capacity (48).
Targeting Keap1 knockdown to increase endogenous Nrf2 expression can be an approach for preventing low alveolarization in preterm infants. Keap1 knockdown has been shown to improve lung cell proliferation after birth in neonatal mouse models, accompanied by an increase in the expression level of the antioxidant gene (nuclear GSH). These findings indicate that Nrf2 plays an essential role in the development of neonatal lung tissue (49). The Nrf2 pathway has several effects on oxidation regulation, such as increasing heme oxygenase-1 (HO-1) expression to improve antioxidant and anti-inflammatory ability. Its protection against development of BPD is mainly dependent on the enzymatic products carbon monoxide, bilirubin, and iron, which prevent alveolar simplification, improve vascular remodeling, and avoid lipid peroxidation (50). In addition, Nrf2 can activate NAD (P)H:quinone oxidoreductase 1(NQO1) to mitigate lung damage by inhibiting cell apoptosis in hyperoxia (51).
Silent mating-type information regulation 2 homolog 1(SIRT1) is a deacetylase activated by nicotinamide adenosine dinucleotide (NAD+). It regulates gene expression by removing acetyl groups from proteins involved in processes such as apoptosis, inflammation, aging, and OS. Lack of SIRT1 can lead to a significant increase in ROS levels and inflammatory response, increasing NAD+ can activate SIRT1, such as resveratrol and some probiotics in breast milk. Small ubiquitin-like modifier (SUMO)-specific protease 1 (SENP1) regulates SIRT1 distribution in OS by participating in substrate de-SUMO modification (small ubiquitination related modification). SIRT1 undergoes SUMOylation, an essential post-translational modification. After SUMOylation, SIRT1 deacetylation is strengthened, and SIRT1 in the nucleus can suppress apoptosis by deacetylating p53. Through de-SUMO modification of SIRT1, SENP1 can reduce SIRT1 deacetylation. SIRT1 protein expression is considerably decreased in neonates with BPD, and the interaction between SUMO1 and SUMO2/3 is significantly weakened. These findings imply that a reduction in SIRT1 SUMOylation may play a role in the development of BPD (52–54).
Recent studies have shown that SIRT1 is linked to the pathogenesis of BPD (52). In peripheral blood mononuclear cells (PBMCs) of neonates with BPD, elevated ROS levels and upregulated SENP1 expression were found. Moreover, SIRT1 showed substantial spatial variations, with decreased expression in the nucleus and increased expression in the cytoplasm. SENP1 silencing can alleviate hyperoxic injury in the hyperoxic alveolar epithelial cell injury model, suggesting that SENP1 can inhibit SIRT1 deacetylation activity by regulating SIRT1 expression and distribution in the nucleus, promoting cell apoptosis, and playing an essential role in hyperoxia-induced lung injury (55). In newborns with BPD, nuclear SIRT1 expression in PBMCs collected by tracheal aspiration is lower and nuclear SIRT1 localization is substantially lower than those in normal neonates (56). The level of ROS and pace of SIRT1 translocation in premature newborns receiving assisted oxygen are strongly oxygen concentration dependence and are associated with a reduction in SIRT1 expression, suggesting that OS can change the activity and distribution of SIRT1. Resveratrol inhibits ROS formation under hyperoxia exposure by blocking the SIRT1 nuclear plasma shuttle and increasing SIRT1 expression (57). Furthermore, through the SIRT1 pathway, budesonide and porcine lung phospholipid injection can exert a protective effect on premature BPD (58).
The Wnt/β-catenin signaling cascade is critical for lung formation during early stages. Wnt signaling occurs at 7 weeks of pregnancy, peaks at 17 weeks, and subsequently declines at 21 weeks (59). According to Zhang et al., Wnt transcription factors are found in low amounts in the respiratory epithelium and mesenchymal lining of the lungs. It plays a vital role in regulating lung development. In neonatal rat lung injury caused by hyperoxia, abnormal activation of Wnt/β-catenin and decreased expression of peroxisome proliferator-activated receptor γ were observed. These changes were linked to reduction in alveolar septal thickness, radial alveolar count, and alveolar augmentation after hyperoxia exposure (60). Alapati et al. found that inhibiting β-catenin expression increased alveolar remodeling and reduced the incidence of pulmonary vascular remodeling and pulmonary hypertension in newborn rats, suggesting the involvement of Wnt/β-catenin pathway in hyperoxia-induced alveolar damage (61).
The expression time and regulation of Wnt signaling during lung development are minimal, and the activation of an aberrant Wnt gene can hinder normal lung development. The development of BPD is complicated by exposure of the lung to high oxygen levels during the cystic stage (62). Jennifer et al. discovered that at the cystic stage, increased oxygen exposure causes aberrant activation of mesenchymal Wnt5A. Furthermore, a three-dimensional organotypic coculture system has revealed that Wnt/β-catenin signal transduction occurs in AEC II and fibroblasts and that targeted suppression of Wnt5A can alleviate hyperoxia-induced alveolar constriction. There are several approaches to ameliorate hyperoxia-induced alveolar epithelial cell injury (YAP and ETS1) via the Wnt/β-catenin signaling pathway. However, given that the Wnt/β-catenin signaling pathway is essential for normal lung development, targeted therapy for this pathway is controversial. To ensure lung development and injury repair in premature infants, it is essential to accurately match the treatment objectives to restore the balance of the lung development signaling pathway (63, 64).
Thioredoxin (TXN) is a small-molecule protein that functions as a hydrogen carrier in cells. Thioredoxin reductase (TXNRD) is a dimer released with a NAPDH dependent FAD domain. The TXN system, which includes TXN, TXNRD, and NAPDH, regulates REDOX equilibrium, cell proliferation, and cell death. It is also involved in embryonic development and immunological metabolism (65). TXN and TXNRD are primarily expressed in pulmonary epithelial cells, alveolar macrophages, and bronchial chondrocytes in neonates, and they function as antioxidants (66). Recent studies have linked TXN system damage with hyperoxic injury and abnormal lung development, noting that the TXN system not only has a simple ROS detoxification function but also acts as a REDOX sensor when oxygen concentration changes, assisting newborns to complete the oxygen concentration conversion process from intrauterine relative hypoxia to hyperoxia exposure (67).
The TXN system efficiently prevents oxidative damage and contributes to REDOX-sensitive lung growth signals. TXN1 siRNA knockdown reduces cell viability after hyperoxia therapy, whereas TXN1 overexpression increases cell survival (68). To minimize lung injury in the BPD model, the antioxidant capacity of the TXN system is dependent on the activation of Nrf2 and an increase in lung epithelial HO-1 expression (69). According to Zhang et al., TXN1 overexpression reduced apoptosis and increased MSC proliferation in lung MSCs transplanted into recipients, SOD levels, and GPX levels (70). TXN1 is a new target for BPD treatment in neonatal lung disorders as it is resistant to hyperoxic lung injury. Increased expression levels of TXN1, TXN2, and TXNRD have been reported in hyperoxia. In GSH reductase-deficient mice, the TXN system showed an apparent antioxidant compensatory effect (71). Wall et al. found that using aurothioglucose (ATG) as a TXNRD1 inhibitor increased the total GSH content and GPX activity of ATG-treated mice under hyperoxia, suggesting that ATG could improve GSH-dependent antioxidant capacity (72). TXNRD inhibitors also have favorable effects on lung development. RNA sequencing in the lungs of mice exposed to >95% oxygen for the first 72 h of life revealed that ATG selectively controlled genes were primarily related to angiogenesis and vascular development and that ATG use increased pulmonary vascular density in mouse models (73).
OS stimulates nuclear factor κB (NF-κB), which regulates inflammatory factor synthesis and activation, nuclear protein translocation, and apoptosis (74). NF-κB is a significant regulator of the incidence, progression, and clearance of inflammation and plays a prominent role in lung inflammation. Although phosphorylation of IκBα (inhibitor of NF-κB α) and nuclear accumulation of NF-κB P65 have been linked to the severity of BPD in recent studies, the role of NF-κB in BPD is debated (75). In breast milk, melatonin, HMOs, and lactoferrin can regulate oxidative balance through NF-κB. OS simultaneously triggers the body's inflammatory response. When exposed to hyperoxia, monocytes, macrophages, lymphocytes, epithelial cells, endothelial cells, and the matrix produce many chemokines, resulting in an influx of inflammatory cells. These are the most critical factors in the mechanism of hyperoxia-induced lung injury. Late chronic inflammatory injury is an essential factor that leads to abnormal lung development (76). OS stimulates several pro-inflammatory factors and mediators, such as IL-1, IL-6, IL-8, IL-17, IL-24, MCP-1, and TNF-α, resulting in an inflammatory cascade (77–81). Inflammation levels in neonates with BPD continue to rise after birth, progressively diminishing after 2 weeks. This trend is particularly pronounced in males and infants with very low birth weight, implying that more targeted treatment interventions should be implemented early in BPD (82).
Previous research has shown that persistent hyperoxia-induced NF-κB activation helps survival and lung growth in neonates. NF-κB activation contributes to the activation of cell protection target genes such as VEGF receptor 2. Inhibition of NF-κB expression in the developing lung lowers vascular proliferation and alveolar–capillary density. It produces reduced alveolarization similar to BPD, indicating that NF-κB activation can be a therapeutic intervention to prevent BPD (83, 84). However, recent studies have suggested that inhibition of NF-κB can reduce hyperoxia-induced newborn lung injury. Tetralin (Tet) enhances antioxidant levels and lowers inflammatory factor levels in hyperoxia-induced lung function decline in rats. Jiao et al. have reported that Tet achieves this by suppressing NF-κB (85). Li et al. used a recombinant human elastase inhibitor, elafin, to inhibit the NF-κB pathway in hyperoxia-exposed mice. They found that elafin reduced apoptosis, inhibited inflammatory cytokines, improved nuclear accumulation of NF-κB P65, and improved alveolarization (86). According to Chen et al., caffeine can also reduce nuclear apoptosis and inflammatory lung injury in lung tissue, lower OS levels, enhance alveolar growth, and protect lung development from oxidative damage in hyperoxia (87). Excessive activation or inhibition of the NF-κB pathway is detrimental to the normal development of the lungs, and direct targeting of the NF-κB pathway may further lead to pulmonary dysplasia. New methods for the prevention and treatment of BPD include targeting NF-κB signaling and suppressing dangerous downstream signals of the NF-κB pathway.
Therefore, OS plays a significant role in the etiology of BPD. Antioxidant treatment can lower infant lung OS, using that antioxidant treatment is an essential study direction for BPD. However, as the free radicals produced by BPD are diverse, the clinical effect of a single antioxidant treatment for BPD does not achieve the desired effect. Therefore, applying various antioxidants in breast milk may positively affect the prevention and treatment of BPD.
Human milk is the safest and most natural nourishment for infants (88). It contains all the calories, proteins, and lipids that newborns require for growth and development and is also high in antioxidants (Table 1). Antioxidants are thought to exist in a range of active compounds. They can remove ROS or reactive nitrogen species directly, change the activity of enzyme boosting and antioxidant enzymes, or regulate REDOX signaling pathways to achieve their antioxidant actions. The overall antioxidant capacity of breastmilk is an important defense mechanism for preventing illnesses that affect newborns (Table 2). Breastfeeding has been shown to minimize the incidence of BPD in premature newborns in clinical practice.
Table 1. Antioxidants in human milk and differences between term (PMA > 37 weeks) and preterm (PMA < 37 weeks) newborn mothers' milk.
Probiotics are microbes that are beneficial to human health. Human milk contains a complex microbial community, providing probiotics and vital energy for neonates. The International Scientific Association for Probiotics and Prebiotics defined probiotic calibration in 2013 as “providing a health benefit to the host when administered in sufficient numbers of living microorganisms,” which includes microorganisms that have been shown to have a health benefit in controlled trials and new symbiotic strains from human samples (127). Lactobacillus and Bifidobacterium are the most prevalent probiotics found in human milk, which can dwell in the intestinal system of neonates along with other probiotics (128, 129). Chanettee et al. identified two Lactobacillus species, L. plantarum and L. pentosus, in human milk (130). Breast milk is the first source of intestinal bifidobacteria in newborns, and they dominate the intestinal flora of breastfed infants (131). The antioxidant capacity of probiotics can be observed in various ways. Probiotics can aid in the chelation of metal ions and removal of reactive free radicals. Their metal chelation action is strain specific. Moreover, probiotics can release antioxidant enzymes and active compounds, such as SOD, CAT, NADH oxidase, TXN, and GSH, which are abundant in Lactobacillus and Bifidobacterium, indicating that probiotics have a substantial antioxidant activity (132, 133). Furthermore, probiotics modulate oxidative equilibrium by modulating antioxidant signaling pathways, with antioxidant effects exerted via the Nrf2, SIRT1, MAPK, and PKC pathways, demonstrating strain specificity (134). Finally, by strengthening the integrity of the intestinal barrier, initiating the immune response, and avoiding inflammation and OS, probiotics and their metabolites can prevent additional microbial metabolites and endotoxins from entering the circulation (135).
Probiotics can lower the incidence of BPD in premature children with a PMA of <32 weeks, demonstrating the potential of probiotics in the treatment of BPD (136). Probiotics can enhance the recovery of the gut-lung axis, anti-inflammation, and anti-infection by modulating the balance of gut microbiota and their remarkable antioxidative powers. However, probiotics can also have adverse effects on the human body. Thus, the safety of probiotics in the human body should be carefully examined before using them to treat infant with BPD, and the species, dose, and frequency of administration should be carefully chosen (137, 138). Formula is an excellent substitute for human milk when new mothers cannot breastfeed owing to physical limitations. Formula adds a range of nutrients to approximate human milk constituents while meeting the nutritional needs of neonates. In addition to specific protein components, fatty acids, carbohydrates, probiotics, and prebiotics are added to formula to control the microbiota of infants (139).
HMOs are the third most abundant solids in human milk, after lactose and fat. HMOs have potent anti-inflammatory, anti-infection, and prebiotic properties. They can also modulate the intestinal epithelial cell response and promote normal neonatal development through interactions with the gut flora. HMOs also play significant roles in adult brain development and cognitive function. The HMO content in mature human milk is approximately 12–15 g/L, and it gradually declines from colostrum to mature milk (140). Studies have also demonstrated that mothers of highly malnourished newborns have lower levels of validated acidic HMOs and fucosylated neutral HMOs than those of normal newborns (141). HMOs in human milk can bind to lactose to form an indigestible trisaccharide or tetrasaccharide; sialic acid can be added to create sialyllactoses (3′-SL and 6′-SL) and fucose can be added to form fucosyllactoses (2′-FL and 3-FL) (142).
The most prevalent oligosaccharide in human milk is 2′-FL. It comprises 30% of HMO. Tu et al. demonstrated that 2′-FL could react with whey protein and remove 2, 2-diphenyl-1-hydrazide radicals, resulting in high antioxidant activity. Further, 2′-FL-fortified formula improves intestinal protection, is well accepted by newborns, and has absorption and excretion rates comparable to those of human milk (143). Lacto-N-neotetraose (LNnT) is a neutral HMO that is not fucosylated. Both 2′-FL and LNnT are new prebiotic additives in formula that make the composition of formula similar to that of human milk. They demonstrate clinical benefits in terms of intestinal probiotic protection, immunological modulation, and brain development and are a safe addition to infant formula (122, 144). Another HMO found in human milk and fermented formula is 3′-galactosyl lactose (3′-GL), which inhibits NF-κB inflammatory signaling and preserves the intestinal barrier. As 3′-GL is a by-product of fermented formula, its safety has been established over time (145).
Most HMOs can reach the intestine, operate as metabolic substrates for the intestinal flora, interact with the intestinal flora, and play a role in modifying the immune system and eliminating pathogens in newborns with low milk oligosaccharide absorption rates. HMOs also act as prebiotics, encouraging the development of newborn intestinal Bifidobacterium and enhancing SCFA synthesis (146). HMOs can also produce butyrate when they react with intestinal bacteria (147). Given the critical role of HMOs in the development and antioxidant capacity of the intestinal flora, more forward-looking, thorough clinical research is needed to evaluate the association between HMOs and BPD.
MFGM is a fat droplet that is essential for lipid transport in human milk. It comprises three layers—phospholipid (PL), sphingomyelin (SM), and various protein–membrane structures. PL and SM are derived from alveolar epithelial cells. PL comprises the main glycerol chain, whereas SM is composed of the main ceramide chain. MFGM promotes proper brain development by improving neonatal immunological function, controlling the intestinal flora, and boosting neonatal immune function (148, 149). MFGM can interact with intestinal probiotics, increase the adhesion of probiotic bacteria in the gut, and increase residence time in the gut (150). The addition of MFGM to formula can help minimize variations in the intestinal flora between breastfeeding and formula feeding (151). MFGM supplementation can assist in maintaining the integrity of intestinal epithelial cells and reducing inflammatory responses in mice with lipopolysaccharide-induced inflammation, implying that MFGM has anti-inflammatory properties (152). An increasing number of trials involving the addition of MFGM to formula have been conducted in recent years. Clinical studies have indicated that MFGM in formula is safe and well tolerated, with few side effects, similar to those observed in breastfeeding babies (120, 123). There are currently no recommended trials on MFGM supplementation in infants with BPD.
SCFAs, such as acetic acid, propionic acid, and butyric acid, are created by human gut flora metabolism and play a significant role in metabolism, the immune system, and anti-inflammation (153). The amount of SCFAs in human milk varies throughout the lactation period. Mature milk has the highest amount of SCFAs, which is four to seven times that in colostrum and transitional milk (105). SCFAs in human milk have been linked to newborn weight gain and obesity (154). SCFAs can enhance lung development and prevent lung disorders through the gut-lung axis. For example, butyrate, with a concentration of approximately 0.75 mM in human milk, protects the integrity of the intestinal barrier and significantly reduces mitochondrial damage in mouse models. OS is controlled by reducing hydrogen peroxide release and regulating the expression of related enzymes (155). Propionate plays a clear role in maintaining the pulmonary immune response; an increase in propionate in the intestinal flora has an anti-inflammatory effect (156). In a previous study, lung inflammation was improved, inflammatory factor expression was reduced, and intestinal microbiota was regulated in mice with BPD treated with acetate (157). In an Nrf2-dependent manner, sodium propionate can reduce lung inflammation and OS and facilitate alveolar simplification and aberrant angiogenesis generated by lipopolysaccharide in mice (158). These findings suggest that SCFAs are crucial for treating lung disorders. Pulmonary OS in newborns with BPD can encourage the production of inflammatory factors and trigger an inflammatory response, aggravating OS. SCFAs have been shown to reduce pulmonary inflammation, which is beneficial for treating BPD. Therefore, SCFAs are essential metabolites of the gut flora. Infants with BPD have a maladjusted gut flora, and human milk can deliver other SCFAs to neonates with BPD.
Lactoferrin is a transferrin found in human and other mammalian milks. Lactoferrin is the most abundant protein in colostrum and possesses antibacterial, antioxidant, antiviral, and anticancer activities (159). Lactoferrin protects the body from OS in two ways—[1] it reduces the Fenton reaction and ROS production by binding to iron and preventing lipid peroxidation (106) and [2] it activates the NF-κB/MAPK pathway to relieve cellular inflammation, maintain cell barrier integrity, reduce OS, activate Nrf2 expression, upregulate GSH activity, and reduce ROS and MDA production (160). Lactoferrin is well tolerated in very young newborns, is straightforward to administer, and serves as a reference for lactoferrin supplementation in clinical practice (161). Lactoferrin supplementation for very young newborns has been shown to minimize the risk of neonatal necrotizing enterocolitis and late sepsis (162, 163). However, some studies have refuted this finding, proposing that enteral supplementation of lactoferrin does not reduce the incidence of infection or other diseases in premature infants and does not reduce the incidence of BPD. The mechanism of lactoferrin in the prevention of BPD is unclear (124). In a study by Dobryk et al., enteral lactoferrin supplementation at 100 mg/day did not affect BPD morbidity and death in preterm infants at PMA ≤32 weeks. However, it may help achieve faster completion of enteral feeding and a shorter hospital stay in the most premature newborns (164).
Vitamin A is a fat-soluble vitamin with biological activity and is a principal exogenous antioxidant. The lung is a critical target organ for vitamin A. Vitamin A can promote pulmonary vascular development, remove overabundant oxidative free radicals, and inhibit lipid peroxidation of the cell membrane, thereby reducing the effects of hyperoxia on lung development at various stages of alveolar development (165). Many studies have examined the critical role of vitamin A in the etiology of BPD. However, opinions on the prevention and treatment of BPD with early vitamin A supplementation are divided. In a clinical trial, early oral vitamin A (5,000 IU vitamin A/kg/day) minimized BPD morbidity and mortality in extremely low-birth-weight infants 28 days after delivery (126). In a study of very-low-birth-weight infants, Chabra et al. found that intramuscular injections of vitamin A can lower the incidence of BPD (166). High-quality reviews have demonstrated that early vitamin A supplementation for preterm newborns has good efficacy and safety, especially for extremely low-birth-weight infants. Vitamin A treatment can also reduce oxygen dependence for infants at a PMA of 36 weeks. However, as this supplementation effect has only been linked to a lower risk of BPD and not to lower risk of early death, more clinical research on amount and delivery of vitamin A supplementation is needed (167–170).
Although Abhijeet et al. demonstrated that enteral supplementation of water-soluble vitamin A improved plasma retinol levels in premature infants, they were unable to confirm a preventive or therapeutic effect on BPD, which might have been due to newborns' limited intestinal absorption capacity (171–173). The authors claimed that vitamin A supplementation might have a baseline—only vitamin A intake of 1,500 IU/kg/day was shown to reduce the incidence of BPD, regardless of the route of administration (enteral and parenteral) (174). In a phase III randomized controlled trial of 807 infants in 14 university hospitals across the United States, Matthew et al. found that vitamin A treatment reduced BPD morbidity and mortality in low-risk infants more than those in high-risk infants (175). Therefore, vitamin A treatment should not be restricted to more than those in high-risk neonates. Craig et al. discovered that nebulized vitamin A is more effective than injectable vitamin A in reducing hyperoxia-induced lung injury and improving BPD treatment (176). Human milk is also rich in vitamin A; therefore, it can exert a therapeutic effect through intestinal pathways.
Vitamin E is a powerful antioxidant that eliminates free radicals from the human body. Human milk contains alpha- and gamma-tocopherol vitamin E. While infant vitamin E oral preparations are well tolerated throughout pregnancy, several investigations have found that vitamin E levels at birth are much lower in infants with BPD than in normal infants. Vitamin E insufficiency is inversely related to the period in which premature newborns require supplemental breathing, demonstrating an association between vitamin E deficiency and BPD severity (177). Most vitamin E clinical trials were halted in the 1990s, with several trials concluding that vitamin E had no evident clinical therapeutic effect on BPD. Due to the limitations of the research circumstances at the time and other considerations, such as other early diseases and understanding of BPD, the clinical application of vitamin E has not been extensively recognized (178–181).
However, compared to 30 years ago, qualitative changes in the understanding of BPD have occurred, namely, the revision from old BPD definition to the new BPD definition. With the improvement of premature infant nursing technology, birth gestational age has been raised from 30 weeks to around 26 weeks for children with BPD. At this time, it is in the tubular lung development or early capsule stage. The clinical characteristics of the new BPD were also altered from those of old BPD—“significant lung injury” was revised to “lung developmental arrest.” In the contemporary BPD context, it may be argued that research on vitamin E treatment for BPD has lost clinical importance. However, vitamin E supplementation has recently been shown to have a preventive effect on new BPD. BPD can be reduced by increasing the amount of vitamin E in the diet (182).
Phytochemicals are a broad range of biochemical molecules produced by plants, but not by the human body. Lactating women can obtain phytochemicals through their diet, resulting in the production of breast milk phytochemicals. Human milk phytochemicals contain polyphenols and carotenoids, which have been shown to have strong antioxidant properties (183).
Polyphenols are secondary metabolites with a polyphenolic structure found in plants. Flavonoids are an important subgroup of polyphenols, but there are no sources of polyphenols in the body. The amount of polyphenols in breast milk depends on the mother's food intake and absorption (184). Song et al. found epicatechin, epicatechin gallate, epicatechin gallate, naringin, kaempferol, hesperidin, and quercetin in the breast milk of full-term mothers (114).
Polyphenols, which are powerful antioxidants, have been shown to impact signal transmission and modulate intestinal enzyme activity through redox reactions. Polyphenol levels in vivo are inversely proportional to MDA, a lipid peroxidation product, implying that polyphenols can mitigate the effects of neonatal lipid peroxidation. Poniedziaek et al. discovered that nursing mothers who eat more vegetables had more polyphenols in their milk and higher antioxidant capacity (185). Currently, researchers are paying increasing attention to the antioxidant ability of polyphenols and flavonoids to avoid OS induced by ROS accumulation and diseases associated with OS. Polyphenol supplementation reduces chronic oxidative cell damage, DNA damage, inflammation, infection, and neurodegenerative disorders (186). Flavonoids have three main antioxidant effects— (1) metal chelation through different flavonoid structures under specific PH values, (2) reducing free radicals (superoxide, hydrogen peroxide, alkoxy) generated through hydrogen donation, where the newly generated free radicals react with other free radicals to form stable quinone structures, and (3) inhibiting oxidases (such as xanthine oxidase, microsomal oxygenase, and lipoxygenase) (187). Supplementing polyphenols in breast milk is a convenient and safe way to absorb them, but there is currently limited research on neonatal plasma polyphenol levels. And there is no evidence that polyphenols have positive effects for development of neonatal lungs, long-term clinical trials are indispensable to determine the benefits of polyphenols for newborns.
Carotenoids are phytochemicals with antioxidant properties that can reduce the risk of cancer and cardiovascular disease. They are essential for immunological modulation and anti-aging. Beta-carotene can also be converted to the most common form of vitamin A in the body. Carotenoids and vitamin A levels in children with BPD are lower than expected (188). Carotenoid concentrations in breast milk change significantly over time, with α-carotene, β-carotene, alpha-cryptoxanthin, β-cryptoxanthin, zeaxanthin, lutein, and lycopene decreasing during early lactation (1-4 weeks) and then during the following nine weeks. As carotenoids are produced in breast milk during lactation and are consumed continuously after lactation, they do not accumulate in the mother and require exogenous supplementation (114). Given the high antioxidant capacity and ability of carotenoids to increase vitamin A levels in the body, it is reasonable to conclude that carotenoid supplementation is beneficial in treating BPD. Premature newborns can be administered carotenoids in human milk to boost their antioxidant capacity. However, clinical evidence of the advantages of carotenoids in newborn development and their impact on BPD is currently lacking.
Melatonin is a hormone secreted by and retained in the pineal gland and subsequently processed in the liver. Melatonin secretion exhibits a distinct nocturnal circadian pattern. Melatonin is present in breast milk at higher concentrations at night and at lower concentrations during the day. Melatonin is a powerful endogenous antioxidant that can directly remove excess free radicals and promote the expression of other antioxidants such as SOD, CAT, and GPX (189). According to Li et al., melatonin can enhance the oxidative balance in mice with hyperoxia-induced lung injury by lowering MPO, nitrite/nitrate, and MDA levels; increasing GPX, CAT, and SOD activities; and reducing alveolar simplification and interstitial fibrosis. Given the similar lung development in mice and humans, it is plausible to assume that melatonin may protect against newborn hyperoxic lung injury (190). Selami et al. confirmed the therapeutic benefits of melatonin in rats with hyperoxia-induced lung damage. Melatonin treatment was found to increase the expression of lamellar protein and radiate alveolar count produced by AEC II, increase the levels of GPX and SOD, and decrease the levels of MDA, implying that melatonin can regulate the oxidative balance, protect pulmonary vascular endothelial cells, and promote alveolarization in hyperoxia-induced lung injury (191). For premature infants, melatonin treatment could reduce the mortality and hospital stay of BPD, which also need further studies to identify direct benefits of melatonin (125).
Trace elements are elements in the human body that contains <0.005–0.01% of body mass. Copper, selenium, iron, zinc, and other trace elements found in human milk form the foundation of the oxidation reaction of the body. Trace element supplementation can boost antioxidant function by catalyzing multiple redox processes by varying valence and activating endogenous antioxidants to eliminate ROS. Premature newborns acquire vital vitamins from human milk to fight excess reactive radicals. Trace elements are required by the body's antioxidant system; for example, selenium is necessary to maintain the activity of glutathione peroxidase, which is a powerful antioxidant that can remove lipid peroxidation. Selenium deficiency impairs the antioxidant function in the body. Supplementing premature newborns with selenium may improve their BPD prognosis, but it does not prevent BPD (192).
Free amino acids are amino acids that do not form peptides. Human milk contains a range of free amino acids, including arginine, a critical amino acid for premature newborns. Arginine is involved in the circulation of ornithine, which is crucial for immunological function and acid–base balance. The antioxidant properties of arginine are of interest. L-arginine has been shown to lower OS in the exercise state through NO production (193). The combined use of inhaled NO and vitamin A supplementation can minimize morbidity and mortality in premature infants who require early mechanical ventilation (194). In vivo, arginine is the raw material for GSH synthesis, and L-arginine supplementation can enhance GSH production and activate the Nrf2–ARE pathway, resulting in endogenous antioxidant responses (195).
Glutamine is a conditionally essential amino acid that can help build muscles, boost the immune system, and expand antioxidant capacity. Exogenous glutamine supplementation for a short period can increase serum CAT and SOD activities and has an antioxidant effect. Glutamine has been shown in some studies to protect mice from hyperoxia-induced lung injury. Arginine–glutamine dipeptide is a stable source of water-soluble glutamine and protects newborn mice from hyperoxic lung injury (196). But there are not direct researches to study the benefits of free amino acids in BPD.
Antioxidant enzymes are important substances for human body to against OS. Human milk contains antioxidant enzymes such as SOD, CAT, and GPX, which are crucial for preventing lung damage in neonates. SOD catalyzes the conversion of superoxide anion free radicals to hydrogen peroxide. In contrast, CAT and GPX are responsible for hydrogen peroxide breakdown and prevention of oxidative free radical accumulation in the body. Excess ROS cannot be removed in premature newborns due to abnormalities in the endogenous antioxidant enzyme system, leading to ROS accumulation and, ultimately, OS. Breastfeeding can improve the antioxidant capacity of premature infants. Colostrum has a higher antioxidant capacity than transitional milk or mature milk, and it can aid in the early transition from intrauterine hypoxia to extrauterine hyperoxia and improve the antioxidant system (189).
Human milk also contains GSH, a crucial non-enzymatic antioxidant. GSH participates in the circulation of vitamins C and E, boosting antioxidant capacity and engaging in several redox processes as a reducing agent. GSH levels in the alveolar lavage fluid of children with BPD have been shown to be considerably lower than those in children without BPD. A survey of GPX-deficient neonatal mice revealed that increased GSH-dependent redox reactions could lower oxidative lung damage and the prevalence of BPD (71).
BPD is a chronic respiratory condition with long-term detrimental implications for newborn health, such as reduced quality of life and poor clinical outcomes. Awareness of BPD has grown in recent years due to ongoing research on its etiology and pathogenesis. OS due to hyperoxia exposure is a significant risk factor for BPD. There are additional preventative and therapeutic targets for BPD. Antioxidant treatment has become an essential strategy for the adjuvant treatment of preterm newborns with BPD despite the lack of standardized clinical signs. OS has a range of effects on normal lung development. When premature infants are born, their lungs are still in the growth stage, and hyperoxia causes poor lung development, increases death of alveolar epithelial cells, and triggers pulmonary vascular remodeling. Although OS plays a role in the development of BPD, there are several limitations to clinical oxidation treatment—treatment is limited to a single type of antioxidant in clinical practice, antioxidant treatment is used only as a supplement to conventional treatment of BPD, and a poor understanding of antioxidant treatment level. However, studies on the degree of antioxidant therapy in preterm newborns using exogenous antioxidants are scarce. Determining how to target the OS site to administer exogenous antioxidant molecules is challenging, which directly impacts the stability and efficacy of antioxidant therapies. Therefore, clinical antioxidant therapy often does not have the desired effect. Antioxidant treatment is undeniably effective in combating oxidative imbalances. Inhibition of OS via the Nrf2, SIRT1, and TXN signaling pathways has shown promising results in animal models, demonstrating the potential of antioxidant therapy in preventing and controlling BPD. More clinical trials are needed to confirm the association between OS and BPD and further investigate the benefits of antioxidant therapy for BPD.
Human milk is the most natural and safe food for infants. The current review reveals that breastfeeding may help minimize the risk of developing BPD, although the role of human milk in preventing and treating BPD is unknown. Breastfeeding has many beneficial effects on BPD. Human milk delivers a high level of nutrients that aid in the normal development of premature newborns after birth, improve their nutritional status, and encourage the continuous development of their lungs. Furthermore, breastfeeding reduces postpartum inflammation, improves immunity, reduces newborn infections, and lowers the incidence of BPD. Human milk also contains several antioxidants that are thought to minimize OS in the BPD process. In this review, we examined several active compounds in human milk, considering them from different perspectives to collate how they may ameliorate BPD OS in lung development as follows: 1. direct secretion of antioxidant substances clears free radicals and prevents their accumulation, 2. metal chelation, 3. enhancement of the antioxidant signaling pathway, 4. interaction with the intestinal flora, 5. inhibition of lipid peroxidation, and 6. anti-inflammatory and anti-infection properties.
Human milk has a high antioxidant capacity, which supports the benefits of breastfeeding for preterm neonates. However, there are several limitations to this view. Firstly, we identify only the antioxidants that play an antioxidant role in BPD. It is unclear whether they act as effective antioxidants in breast milk and whether there is a synergistic effect between these substances. Secondly, most studies cited in this view associated with the antioxidant effect of BPD do not come from breast milk OS. The research about breast milk antioxidants and their effects on BPD are still limited based on ethical factors. thirdly, there are limited data on human milk from mothers of premature infants; therefore, differences in antioxidant capacity compared to that of human milk from mothers of full-term infants has not been clearly shown. Owing to ethical considerations, few randomized controlled trials to prevent premature BPD have been conducted. Hence, observational studies have been given precedence.
While the effects of breast milk on BPD have been addressed, there are much research in this territory that require future studies. In this view, we suggest researchers take these issues into account: 1. Should the combination of probiotics be added to the formula? 2. Does the antioxidants in breast milk have synergistic effects when breastfeeding? 3. What are the positive effects of MFGM on antioxidation? 4. What roles does the gut-lung axis play when SCFAs affect as antioxidative substances? 5. Do the Vitamin E treatments have effects accompanied by the evolution of BPD? 6. How does the addition of polyphenols and carotenoids to the formula impact the development of neonatal lungs? 7. Does melatonin have effects on BPD in preterm infants? These controversies require further clinical studies although the limitations are still hard. And we also noticed that stem cells in breast milk present a prominent potential for BPD treatment, it is worth studying their antioxidative effects on BPD.
Individual variables related to human milk, such as neonatal birth gestational age, whether the mother is breastfeeding, and whether exclusive breastfeeding is practiced, can have a significant impact on the composition of human milk. Human milk from mothers of premature newborns differs substantially from human milk from mothers of full-term newborns. Chrustek et al. found that compared to human milk from mothers of full-term newborns, that from mothers of premature infants may contain more antioxidants. Premature newborns with a higher total antioxidant status are protected from free radicals, preventing ROS accumulation and OS (197). Antioxidants in human milk have been modified to the PMA to provide improved protection for premature newborns. Some active compounds in breast milk can increase the antioxidant capacity of premature infants, which is conducive to the rapid establishment of oxidative balance in premature infants with insufficient antioxidant capacity.
Human milk contains many bioactive compounds that supply essential nutrients to newborns and act as antioxidants to protect premature babies from OS. Existing research suggests that breastfeeding can ameliorate the effects of BPD. However, more high-quality tests and studies are needed to provide evidence for the role of breastfeeding in the prevention and control of BPD. Breastfeeding is recommended for full-term and preterm infants, although their antioxidant capacities differ. High-dose breastfeeding has the potential to be an effective and inexpensive treatment option for BPD.
XY is responsible for the collection of data and writing of the original manuscript. SJ is responsible for the organization of the original manuscript. XD and ZL are responsible for editing. AC and RY are responsible for the concept development, revision, review of the manuscript, and funding acquisition. All authors contributed to the article and approved the submitted version.
This research was supported by National Natural Science Foundation of China (No. 82101812), Jiangsu Commission of Health and Family Planning (No. Z2020042), Wuxi Medical Innovation Team (No. CXTD2021013), and Wuxi Young and Middle-aged Medical Talents Project (Nos. BJ2020075 and BJ2020079).
The authors declare that the research was conducted in the absence of any commercial or financial relationships that could be construed as a potential conflict of interest.
All claims expressed in this article are solely those of the authors and do not necessarily represent those of their affiliated organizations, or those of the publisher, the editors and the reviewers. Any product that may be evaluated in this article, or claim that may be made by its manufacturer, is not guaranteed or endorsed by the publisher.
We would like to thank Editage (www.editage.cn) for English language editing.
BPD, Bronchopulmonary dysplasia; OS, Oxidative stress; NICHD, The National Institute of Child Health and Human Development; ES, Endoplasmic reticulum stress; UPR, Unfolded protein response; VEGF, Vascular endothelial growth factor; MSC, Mesenchymal stem cells; Nrf2, Nuclear erythroid-E2-related factor 2; ARE, Antioxidant reaction elements; HO-1, Heme oxygenase-1; SIRT1, Silent mating-type information regulation 2 homolog 1; NAD, Nicotinamide adenosine dinucleotide; SENP1, Small ubiquitin-like modifier (SUMO)-specific protease 1; PBMC, Peripheral blood mononuclear cells; PPARγ, Peroxy-proliferator-activated receptor γ; AT2, Primary alveolar type 2 cells; TXN, Thioredoxin; TXNRD, Thioredoxin reductase; ATG, Aurothioglucose; NF-κB, Nuclear factor κB; Tet, Tetralin; ISAPP, The International Scientific Association for Probiotics and Prebiotics; 2′-FL, 2′-fucosyllactose; LNnT, Lacto-N-neotetraose; 3′-GL, 3′-galactosyl lactose; HMO, Human milk oligosaccharides; DPPH, 2, 2-diphenyl-1-hydrazide; SCFA, Short chain fatty acids; SOD, Superoxide dismutase; GSH-Px, Glutathione peroxidase; NQO1, NAD (P)H:quinone oxidoreductase.
1. Northway WH, Rosan RC, Porter DY. Pulmonary disease following respirator therapy of hyaline-membrane disease. Bronchopulmonary dysplasia. N Engl J Med. (1967) 276:357–68. doi: 10.1056/NEJM196702162760701
2. Jobe AH, Bancalari E. Bronchopulmonary dysplasia. Am J Respir Crit Care Med. (2001) 163:1723–9. doi: 10.1164/ajrccm.163.7.2011060
3. Wang CH, Shen XX, Chen MY, Ma XL, Shi LP, Du LZ. Comparison of the Clinical Diagnosis and Outcome in Preterm Infants with Bronchopulmonary Dysplasia under Two Different Diagnostic Criteria. Zhonghua Er Ke Za Zhi. (2020) 58:381–6. doi: 10.3760/cma.j.cn112140-20200108-00017
4. Higgins RD, Jobe AH, Koso-Thomas M, Bancalari E, Viscardi RM, Hartert TV, et al. Bronchopulmonary dysplasia: executive summary of a workshop. J Pediatr. (2018) 197:300–8. doi: 10.1016/j.jpeds.2018.01.043
5. Jensen EA, Dysart K, Gantz MG, McDonald S, Bamat NA, Keszler M, et al. The diagnosis of bronchopulmonary dysplasia in very preterm infants. An evidence-based approach. Am J Respir Crit Care Med. (2019) 200:751–9. doi: 10.1164/rccm.201812-2348OC
6. Zhu Z, Yuan L, Wang J, Li Q, Yang C, Gao X, et al. Mortality and morbidity of infants born extremely preterm at tertiary medical centers in China from 2010 to 2019. JAMA Netw Open. (2021) 4:e219382. doi: 10.1001/jamanetworkopen.2021.9382
7. Askie LM, Darlow BA, Davis PG, Finer N, Stenson B, Vento M, et al. Effects of targeting lower versus higher arterial oxygen saturations on death or disability in preterm infants. Cochrane Database Syst Rev. (2017) 4:CD011190. doi: 10.1002/14651858.CD011190.pub2
8. Foglia EE, Jensen EA, Kirpalani H. Delivery room interventions to prevent bronchopulmonary dysplasia in extremely preterm infants. J Perinatol. (2017) 37:1171–9. doi: 10.1038/jp.2017.74
9. Cools F, Askie LM, Offringa M, Asselin JM, Calvert SA, Courtney SE, et al. Elective high-frequency oscillatory versus conventional ventilation in preterm infants: a systematic review and meta-analysis of individual patients' data. Lancet. (2010) 375:2082–91. doi: 10.1016/S0140-6736(10)60278-4
10. Keszler M. Volume-targeted ventilation: one size does not fit all. Evidence-Based Recommendations for Successful Use Arch. Dis Child Fetal Neonatal Ed. (2019) 104:F108–F12. doi: 10.1136/archdischild-2017-314734
11. Doyle LW, Cheong JL, Hay S, Manley BJ, Halliday HL. Early (<7 Days) Systemic postnatal corticosteroids for prevention of bronchopulmonary dysplasia in preterm infants. Cochrane Database Syst Rev. (2021) 10:CD001146. doi: 10.1002/14651858.CD001145.pub5
12. Greenberg RG, Gayam S, Savage D, Tong A, Gorham D, Sholomon A, et al. Furosemide exposure and prevention of bronchopulmonary dysplasia in premature infants. J Pediatr. (2019) 208:134–40 e2. doi: 10.1016/j.jpeds.2018.11.043
13. Duijts L, van Meel ER, Moschino L, Baraldi E, Barnhoorn M, Bramer WM, et al. European respiratory society guideline on long-term management of children with bronchopulmonary dysplasia. Eur Respir J. (2020) 55. doi: 10.1183/13993003.00788-2019
14. Dumpa V, Nielsen L, Wang H, Kumar VHS. Caffeine Is Associated with improved alveolarization and angiogenesis in male mice following hyperoxia induced lung injury. BMC Pulm Med. (2019) 19:138. doi: 10.1186/s12890-019-0903-x
15. Doyle LW, Ranganathan S, Cheong JLY. Neonatal caffeine treatment and respiratory function at 11 years in children under 1,251 G at birth. Am J Respir Crit Care Med. (2017) 196:1318–24. doi: 10.1164/rccm.201704-0767OC
16. Gilfillan M, Bhandari A, Bhandari V. Diagnosis and management of bronchopulmonary dysplasia. BMJ. (2021) 375:n1974. doi: 10.1136/bmj.n1974
17. Ofman G, Tipple TE. Antioxidants & bronchopulmonary dysplasia: beating the system or beating a dead horse? Free Radic Biol Med. (2019) 142:138–45. doi: 10.1016/j.freeradbiomed.2019.01.038
18. Wang J, Dong W. Oxidative stress and bronchopulmonary dysplasia. Gene. (2018) 678:177–83. doi: 10.1016/j.gene.2018.08.031
19. Villamor-Martinez E, Pierro M, Cavallaro G, Mosca F, Villamor E. Mother's Own Milk and bronchopulmonary dysplasia: a systematic review and meta-analysis. Front Pediatr. (2019) 7:224. doi: 10.3389/fped.2019.00224
20. Fonseca LT, Senna DC, Silveira RC, Procianoy RS. Association between breast milk and bronchopulmonary dysplasia: a single center observational study. Am J Perinatol. (2017) 34:264–9. doi: 10.1055/s-0036-1586503
21. Patel AL, Johnson TJ, Robin B, Bigger HR, Buchanan A, Christian E, et al. Influence of own mother's milk on bronchopulmonary dysplasia and costs. Arch Dis Child Fetal Neonatal Ed. (2017) 102:F256–F61. doi: 10.1136/archdischild-2016-310898
22. Johnson TJ, Patel AL, Schoeny ME, Meier PP. Cost savings of mother's own milk for very low birth weight infants in the neonatal intensive care unit. Pharmacoecon Open. (2022). doi: 10.1007/s41669-022-00324-8
23. Xu Y, Yu Z, Li Q, Zhou J, Yin X, Ma Y, et al. Dose-dependent effect of human milk on bronchopulmonary dysplasia in very low birth weight infants. BMC Pediatr. (2020) 20:522. doi: 10.1186/s12887-020-02394-1
24. Cadenas S. Ros and redox signaling in myocardial ischemia-reperfusion injury and cardioprotection. Free Radic Biol Med. (2018) 117:76–89. doi: 10.1016/j.freeradbiomed.2018.01.024
25. Auten RL, Davis JM. Oxygen toxicity and reactive oxygen species: the devil is in the details. Pediatr Res. (2009) 66:121–7. doi: 10.1203/PDR.0b013e3181a9eafb
26. Falsaperla R, Lombardo F, Filosco F, Romano C, Saporito MAN, Puglisi F, et al. Oxidative stress in preterm infants: overview of current evidence and future prospects. Pharmaceuticals (Basel). (2020) 13:145. doi: 10.3390/ph13070145
27. Jobe AH, Kallapur SG. Long term consequences of oxygen therapy in the neonatal period. Semin Fetal Neonatal Med. (2010) 15:230–5. doi: 10.1016/j.siny.2010.03.007
28. Hou A, Fu J, Yang H, Zhu Y, Pan Y, Xu S, et al. Hyperoxia stimulates the transdifferentiation of type II alveolar epithelial cells in newborn rats. Am J Physiol Lung Cell Mol Physiol. (2015) 308:L861–72. doi: 10.1152/ajplung.00099.2014
29. Zhang QY, Fu JH, Xue XD. Expression and function of aquaporin-1 in hyperoxia-exposed alveolar epithelial type II cells. Exp Ther Med. (2014) 8:493–8. doi: 10.3892/etm.2014.1739
30. Liu G, Philp AM, Corte T, Travis MA, Schilter H, Hansbro NG, et al. Therapeutic targets in lung tissue remodelling and fibrosis. Pharmacol Ther. (2021) 225:107839. doi: 10.1016/j.pharmthera.2021.107839
31. Oakes SA, Papa FR. The role of endoplasmic reticulum stress in human pathology. Annu Rev Pathol. (2015) 10:173–94. doi: 10.1146/annurev-pathol-012513-104649
32. Teng RJ, Jing X, Michalkiewicz T, Afolayan AJ, Wu TJ, Konduri GG. Attenuation of endoplasmic reticulum stress by caffeine ameliorates hyperoxia-induced lung injury. Am J Physiol Lung Cell Mol Physiol. (2017) 312:L586–L98. doi: 10.1152/ajplung.00405.2016
33. Lignelli E, Palumbo F, Myti D, Morty RE. Recent advances in our understanding of the mechanisms of lung alveolarization and bronchopulmonary dysplasia. Am J Physiol Lung Cell Mol Physiol. (2019) 317:L832–L87. doi: 10.1152/ajplung.00369.2019
34. Silva DM, Nardiello C, Pozarska A, Morty RE. Recent advances in the mechanisms of lung alveolarization and the pathogenesis of bronchopulmonary dysplasia. Am J Physiol Lung Cell Mol Physiol. (2015) 309:L1239–72. doi: 10.1152/ajplung.00268.2015
35. Dylag AM, Haak J, Yee M, O'Reilly MA. Pulmonary mechanics and structural lung development after neonatal hyperoxia in mice. Pediatr Res. (2020) 87:1201–10. doi: 10.1038/s41390-019-0723-y
36. Cox AM, Gao Y, Perl AT, Tepper RS, Ahlfeld SK. Cumulative effects of neonatal hyperoxia on murine alveolar structure and function. Pediatr Pulmonol. (2017) 52:616–24. doi: 10.1002/ppul.23654
37. Ratner V, Sosunov SA, Niatsetskaya ZV, Utkina-Sosunova IV, Ten VS. Mechanical ventilation causes pulmonary mitochondrial dysfunction and delayed alveolarization in neonatal mice. Am J Respir Cell Mol Biol. (2013) 49:943–50. doi: 10.1165/rcmb.2012-0172OC
38. Ratner V, Starkov A, Matsiukevich D, Polin RA, Ten VS. Mitochondrial dysfunction contributes to alveolar developmental arrest in hyperoxia-exposed mice. Am J Respir Cell Mol Biol. (2009) 40:511–8. doi: 10.1165/rcmb.2008-0341RC
39. Apte RS, Chen DS, Ferrara N. VEGF in signaling and disease: beyond discovery and development. Cell. (2019) 176:1248–64. doi: 10.1016/j.cell.2019.01.021
40. Muramatsu Y, Ito M, Oshima T, Kojima S, Ohno K. Hydrogen-rich water ameliorates bronchopulmonary dysplasia (BPD) in newborn rats. Pediatr Pulmonol. (2016) 51:928–35. doi: 10.1002/ppul.23386
41. Berger J, Bhandari V. Animal models of bronchopulmonary dysplasia. The term mouse models. Am J Physiol Lung Cell Mol Physiol. (2014) 307:L936–47. doi: 10.1152/ajplung.00159.2014
42. Mathew R. Signaling pathways involved in the development of bronchopulmonary dysplasia and pulmonary hypertension. Children (Basel). (2020) 7. doi: 10.3390/children7080100
43. Sun C, Zhang S, Wang J, Jiang W, Xin Q, Chen X, et al. EPO enhances the protective effects of MSCs in experimental hyperoxia-induced neonatal mice by promoting angiogenesis. Aging (Albany NY). (2019) 11:2477–87. doi: 10.18632/aging.101937
44. Wallace B, Peisl A, Seedorf G, Nowlin T, Kim C, Bosco J, et al. Anti-sFlt-1 therapy preserves lung alveolar and vascular growth in antenatal models of bronchopulmonary dysplasia. Am J Respir Crit Care Med. (2018) 197:776–87. doi: 10.1164/rccm.201707-1371OC
45. Zhang X, Lu A, Li Z, Sun J, Dai D, Qian L. Exosomes secreted by endothelial progenitor cells improve the bioactivity of pulmonary microvascular endothelial cells exposed to hyperoxia in vitro. Ann Transl Med. (2019) 7:254. doi: 10.21037/atm.2019.05.10
46. Mizumura K, Maruoka S, Shimizu T, Gon Y. Role of Nrf2 in the pathogenesis of respiratory diseases. Respir Investig. (2020) 58:28–35. doi: 10.1016/j.resinv.2019.10.003
47. Liu Q, Gao Y, Ci X. Role of Nrf2 and its activators in respiratory diseases. Oxid Med Cell Longev. (2019) 2019:7090534. doi: 10.1155/2019/7090534
48. Ma D, Gao W, Liu J, Kong D, Zhang Y, Qian M. Mechanism of oxidative stress and Keap-1/Nrf2 signaling pathway in bronchopulmonary dysplasia. Medicine (Baltimore). (2020) 99:e20433. doi: 10.1097/MD.0000000000020433
49. Tamatam CM, Reddy NM, Potteti HR, Ankireddy A, Noone PM, Yamamoto M, et al. Preconditioning the immature lung with enhanced Nrf2 activity protects against oxidant-induced hypoalveolarization in mice. Sci Rep. (2020) 10:19034. doi: 10.1038/s41598-020-75834-8
50. Amata E, Pittala V, Marrazzo A, Parenti C, Prezzavento O, Arena E, et al. Role of the Nrf2/HO-1 axis in bronchopulmonary dysplasia and hyperoxic lung injuries. Clin Sci (Lond). (2017) 131:1701–12. doi: 10.1042/CS20170157
51. Weng B, Zhang X, Chu X, Gong X, Cai C. Nrf2-Keap1-ARE-NQO1 signaling attenuates hyperoxiainduced lung cell injury by inhibiting apoptosis. Mol Med Rep. (2021) 23. doi: 10.3892/mmr.2021.11860
52. Tan F, Dong W, Lei X, Liu X, Li Q, Kang L, et al. Attenuated SUMOylation of sirtuin 1 in premature neonates with bronchopulmonary dysplasia. Mol Med Rep. (2018) 17:1283–8. doi: 10.3892/mmr.2017.8012
53. Singh V, Ubaid S. Role of silent information regulator 1 (SIRT1) in regulating oxidative stress and inflammation. Inflammation. (2020) 43:1589–98. doi: 10.1007/s10753-020-01242-9
54. Yang K, Dong W. SIRT1-related signaling pathways and their association with bronchopulmonary dysplasia. Front Med (Lausanne). (2021) 8:595634. doi: 10.3389/fmed.2021.595634
55. Dong W, Zhu X, Liu X, Zhao X, Lei X, Kang L, et al. Role of the SENP1-SIRT1 pathway in hyperoxia-induced alveolar epithelial cell injury. Free Radic Biol Med. (2021) 173:142–50. doi: 10.1016/j.freeradbiomed.2021.07.027
56. Mody K, Saslow JG, Kathiravan S, Eydelman R, Bhat V, Stahl GE, et al. Sirtuin1 in tracheal aspirate leukocytes: possible role in the development of bronchopulmonary dysplasia in premature infants. J Matern Fetal Neonatal Med. (2012) 25:1483–7. doi: 10.3109/14767058.2011.645925
57. Yang X, Dong WB, Lei XP Li QP, Zhang LY, Zhang LP. Resveratrol suppresses hyperoxia-induced nucleocytoplasmic shuttling of SIRT1 and ROS production in PBMC from preterm infants in vitro. J Matern Fetal Neonatal Med. (2018) 31:1142–50. doi: 10.1080/14767058.2017.1311310
58. Du FL, Dong WB, Zhang C, Li QP, Kang L, Lei XP, et al. Budesonide and poractant alfa prevent bronchopulmonary dysplasia via triggering SIRT1 signaling pathway. Eur Rev Med Pharmacol Sci. (2019) 23:11032–42. doi: 10.26355/eurrev_201912_19811
59. Zhang M, Shi J, Huang Y, Lai L. Expression of canonical WNT/β-CATENIN signaling components in the developing human lung. BMC Dev Biol. (2012) 12:21. doi: 10.1186/1471-213X-12-21
60. Dasgupta C, Sakurai R, Wang Y, Guo P, Ambalavanan N, Torday JS, et al. Hyperoxia-induced neonatal rat lung injury involves activation of TGF-β and Wnt signaling and is protected by rosiglitazone. Am J Physiol Lung Cell Mol Physiol. (2009) 296:L1031–41. doi: 10.1152/ajplung.90392.2008
61. Alapati D, Rong M, Chen S, Hehre D, Hummler SC, Wu S. Inhibition of β-catenin signaling improves alveolarization and reduces pulmonary hypertension in experimental bronchopulmonary dysplasia. Am J Respir Cell Mol Biol. (2014) 51:104–13. doi: 10.1165/rcmb.2013-0346OC
62. Sucre JMS, Vickers KC, Benjamin JT, Plosa EJ, Jetter CS, Cutrone A, et al. Hyperoxia injury in the developing lung is mediated by mesenchymal expression of Wnt5A. Am J Respir Crit Care Med. (2020) 201:1249–62. doi: 10.1164/rccm.201908-1513OC
63. Yang M, Gao XR, Meng YN, Shen F, Chen YP. ETS1 ameliorates hyperoxia-induced alveolar epithelial cell injury by regulating the TGM2-mediated Wnt/β-Catenin pathway. Lung. (2021) 199:681–90. doi: 10.1007/s00408-021-00489-9
64. Jia X, Wu B, Huang J, Fan L, Yang M, Xu W. YAP and Wnt3a independently promote AECIIs proliferation and differentiation by increasing nuclear β-catenin expression in experimental bronchopulmonary dysplasia. Int J Mol Med. (2021) 47:195–206. doi: 10.3892/ijmm.2020.4791
65. Muri J, Kopf M. Redox regulation of immunometabolism. Nat Rev Immunol. (2021) 21:363–81. doi: 10.1038/s41577-020-00478-8
66. Soini Y, Kahlos K, Napankangas U, Kaarteenaho-Wiik R, Saily M, Koistinen P, et al. Widespread expression of thioredoxin and thioredoxin reductase in non-small cell lung carcinoma. Clin Cancer Res. (2001) 7:1750–7.
67. Tipple TE. The thioredoxin system in neonatal lung disease. Antioxid Redox Signal. (2014) 21:1916–25. doi: 10.1089/ars.2013.5782
68. Floen MJ, Forred BJ, Bloom EJ, Vitiello PF. Thioredoxin-1 redox signaling regulates cell survival in response to hyperoxia. Free Radic Biol Med. (2014) 75:167–77. doi: 10.1016/j.freeradbiomed.2014.07.023
69. Dunigan K, Li Q, Li R, Locy ML, Wall S, Tipple TE. The thioredoxin reductase inhibitor auranofin induces heme oxygenase-1 in lung epithelial cells via Nrf2-dependent mechanisms. Am J Physiol Lung Cell Mol Physiol. (2018) 315:L545–L52. doi: 10.1152/ajplung.00214.2018
70. Zhang L, Wang J, Chen Y, Zeng L, Li Q, Liu Y, et al. Thioredoxin-1 protects bone marrow-derived mesenchymal stromal cells from hyperoxia-induced injury in vitro. Oxid Med Cell Longev. (2018) 2018:1023025. doi: 10.1155/2018/1023025
71. Robbins ME, Cho HY, Hansen JM, Luchsinger JR, Locy ML, Velten M, et al. Glutathione reductase deficiency alters lung development and hyperoxic responses in neonatal mice. Redox Biol. (2021) 38:101797. doi: 10.1016/j.redox.2020.101797
72. Wall SB, Wood R, Dunigan K, Li Q, Li R, Rogers LK, et al. Thioredoxin reductase-1 inhibition augments endogenous glutathione-dependent antioxidant responses in experimental bronchopulmonary dysplasia. Oxid Med Cell Longev. (2019) 2019:7945983. doi: 10.1155/2019/7945983
73. Dunigan-Russell K, Lin V, Silverberg M, Wall SB Li R, Gotham J, et al. Aurothioglucose enhances proangiogenic pathway activation in lungs from room air and hyperoxia-exposed newborn mice. Am J Physiol Lung Cell Mol Physiol. (2020) 318:L1165–L71. doi: 10.1152/ajplung.00086.2020
74. Zhang J, Wan J, Chen D, Yu B, He J. Low-molecular-weight chitosan attenuates lipopolysaccharide-induced inflammation in IPEC-J2 cells by inhibiting the nuclear factor-κB signalling pathway. Molecules. (2021) 26. doi: 10.3390/molecules26030569
75. Reicherzer T, Haffner S, Shahzad T, Gronbach J, Mysliwietz J, Hubener C, et al. Activation of the NF-κB pathway alters the phenotype of MSCs in the tracheal aspirates of preterm infants with severe BPD. Am J Physiol Lung Cell Mol Physiol. (2018) 315:L87–L101. doi: 10.1152/ajplung.00505.2017
76. Thomas W, Speer CP. Chorioamnionitis is essential in the evolution of bronchopulmonary dysplasia–the case in favour. Paediatr Respir Rev. (2014) 15:49–52. doi: 10.1016/j.prrv.2013.09.004
77. Kumar VH, Lakshminrusimha S, Kishkurno S, Paturi BS, Gugino SF, Nielsen L, et al. Neonatal hyperoxia increases airway reactivity and inflammation in adult mice. Pediatr Pulmonol. (2016) 51:1131–41. doi: 10.1002/ppul.23430
78. Rudloff I, Cho SX, Bui CB, McLean C, Veldman A, Berger PJ, et al. Refining anti-inflammatory therapy strategies for bronchopulmonary dysplasia. J Cell Mol Med. (2017) 21:1128–38. doi: 10.1111/jcmm.13044
79. Hsiao CC, Chang JC, Tsao LY, Yang RC, Chen HN, Lee CH, et al. Correlates of elevated interleukin-6 and 8-hydroxy-2′-deoxyguanosine levels in tracheal aspirates from very low birth weight infants who develop bronchopulmonary dysplasia. Pediatr Neonatol. (2017) 58:63–9. doi: 10.1016/j.pedneo.2016.01.004
80. Gao R, Li Z, Ai D, Ma J, Chen C, Liu X. Interleukin-24 as a pulmonary target cytokine in bronchopulmonary dysplasia. Cell Biochem Biophys. (2021) 79:311–20. doi: 10.1007/s12013-021-00968-z
81. Bhandari A, Carroll C, Bhandari V. BPD following preterm birth: a model for chronic lung disease and a substrate for ARDS in childhood. Front Pediatr. (2016) 4:60. doi: 10.3389/fped.2016.00060
82. Leroy S, Caumette E, Waddington C, Hebert A, Brant R, Lavoie PM. A time-based analysis of inflammation in infants at risk of bronchopulmonary dysplasia. J Pediatr. (2018) 192:60–5 e1. Epub 2017/11/03. doi: 10.1016/j.jpeds.2017.09.011
83. Iosef C, Alastalo TP, Hou Y, Chen C, Adams ES Lyu SC, et al. Inhibiting NF-κB in the developing lung disrupts angiogenesis and alveolarization. Am J Physiol Lung Cell Mol Physiol. (2012) 302:L1023–36. doi: 10.1152/ajplung.00230.2011
84. McKenna S, Michaelis KA, Agboke F, Liu T, Han K, Yang G, et al. Sustained hyperoxia-induced NF-κB activation improves survival and preserves lung development in neonatal mice. Am J Physiol Lung Cell Mol Physiol. (2014) 306:L1078–89. doi: 10.1152/ajplung.00001.2014
85. Jiao B, Tang Y, Liu S, Guo C. Tetrandrine attenuates hyperoxia-induced lung injury in newborn rats via NF-κB p65 and ERK1/2 pathway inhibition. Ann Transl Med. (2020) 8:1018. doi: 10.21037/atm-20-5573
86. Li K, Zhang F, Wei L, Han Z, Liu X, Pan Y, et al. Recombinant human elafin ameliorates chronic hyperoxia-induced lung injury by inhibiting nuclear factor-kappa b signaling in neonatal mice. J Interferon Cytokine Res. (2020) 40:320–30. doi: 10.1089/jir.2019.0241
87. Chen S, Wu Q, Zhong D, Li C, Du L. Caffeine prevents hyperoxia-induced lung injury in neonatal mice through NLRP3 inflammasome and NF-κB pathway. Respir Res. (2020) 21:140. doi: 10.1186/s12931-020-01403-2
88. Khan IT, Nadeem M, Imran M, Ullah R, Ajmal M, Jaspal MH. Antioxidant properties of milk and dairy products: a comprehensive review of the current knowledge. Lipids Health Dis. (2019) 18:41. doi: 10.1186/s12944-019-0969-8
89. Samuel TM, Binia A, de Castro CA, Thakkar SK, Billeaud C, Agosti M, et al. Impact of maternal characteristics on human milk oligosaccharide composition over the first 4 months of lactation in a cohort of healthy european mothers. Sci Rep. (2019) 9:11767. doi: 10.1038/s41598-019-48337-4
90. Huang X, Zhu B, Jiang T, Yang C, Qiao W, Hou J, et al. Improved simple sample pretreatment method for quantitation of major human milk oligosaccharides using ultrahigh pressure liquid chromatography with fluorescence detection. J Agric Food Chem. (2019) 67:12237–44. doi: 10.1021/acs.jafc.9b03445
91. Elwakiel M, Hageman JA, Wang W, Szeto IM, van Goudoever JB, Hettinga KA, et al. Human milk oligosaccharides in colostrum and mature milk of chinese mothers: lewis positive secretor subgroups. J Agric Food Chem. (2018) 66:7036–43. doi: 10.1021/acs.jafc.8b02021
92. Spevacek AR, Smilowitz JT, Chin EL, Underwood MA, German JB, Slupsky CM. Infant maturity at birth reveals minor differences in the maternal milk metabolome in the first month of lactation. J Nutr. (2015) 145:1698–708. doi: 10.3945/jn.115.210252
93. Austin S, De Castro CA, Sprenger N, Binia A, Affolter M, Garcia-Rodenas CL, et al. Human milk oligosaccharides in the milk of mothers delivering term versus preterm infants. Nutrients. (2019) 11:1282. doi: 10.3390/nu11061282
94. Borewicz K, Gu F, Saccenti E, Hechler C, Beijers R, de Weerth C, et al. The association between breastmilk oligosaccharides and faecal microbiota in healthy breastfed infants at two, six, and twelve weeks of age. Sci Rep. (2020) 10:4270. doi: 10.1038/s41598-020-61024-z
95. Thurl S, Munzert M, Boehm G, Matthews C, Stahl B. Systematic review of the concentrations of oligosaccharides in human milk. Nutr Rev. (2017) 75:920–33. doi: 10.1093/nutrit/nux044
96. Cabezuelo MT, Zaragoza R, Barber T, Vina JR. Role of vitamin a in mammary gland development and lactation. Nutrients. (2019) 12:80. doi: 10.3390/nu12010080
97. Jiang J, Xiao H, Wu K, Yu Z, Ren Y, Zhao Y, et al. Retinol and α-tocopherol in human milk and their relationship with dietary intake during lactation. Food Funct. (2016) 7:1985–91. doi: 10.1039/C5FO01293G
98. Souza G, Dolinsky M, Matos A, Chagas C, Ramalho A. Vitamin a concentration in human milk and its relationship with liver reserve formation and compliance with the recommended daily intake of vitamin a in pre-term and term infants in exclusive breastfeeding. Arch Gynecol Obstet. (2015) 291:319–25. doi: 10.1007/s00404-014-3404-4
99. Souza G, Saunders C, Dolinsky M, Queiroz J, Campos A, Ramalho A. Vitamin a concentration in mature human milk. J Pediatr (Rio J). (2012) 88:496–502. doi: 10.2223/JPED.2229
100. Martysiak-Zurowska D, Szlagatys-Sidorkiewicz A, Zagierski M. Concentrations of alpha- and gamma-tocopherols in human breast milk during the first months of lactation and in infant formulas. Matern Child Nutr. (2013) 9:473–82. doi: 10.1111/j.1740-8709.2012.00401.x
101. Zheng MC, Zhang GF, Zhou LS, Guo XG, Quan YF. Alpha-tocopherol concentrations in human milk from mothers of preterm and full-term infants in china. Biomed Environ Sci. (1993) 6:259–64.
102. Wu K, Zhu J, Zhou L, Shen L, Mao Y, Zhao Y, et al. Lactational changes of fatty acids and fat-soluble antioxidants in human milk from healthy chinese mothers. Br J Nutr. (2020) 123:841–8. doi: 10.1017/S0007114520000239
103. Lou Z, Wu K, Xu Y, Cai X, Tian F, Mao Y, et al. Contents and isomers distribution of vitamin e in human mature breast milk from six regions of china in 2018-2019. Wei Sheng Yan Jiu. (2021) 50:914–8.
104. Stinson LF, Gay MCL, Koleva PT, Eggesbo M, Johnson CC, Wegienka G, et al. Human milk from atopic mothers has lower levels of short chain fatty acids. Front Immunol. (2020) 11:1427. doi: 10.3389/fimmu.2020.01427
105. Dai X, Yuan T, Zhang X, Zhou Q, Bi H, Yu R, et al. Short-chain fatty acid (SCFA) and medium-chain fatty acid (MEFA) concentrations in human milk consumed by infants born at different gestational ages and the variations in concentration during lactation stages. Food Funct. (2020) 11:1869–80. doi: 10.1039/C9FO02595B
106. Hao L, Shan Q, Wei J, Ma F, Sun P. Lactoferrin: major physiological functions and applications. Curr Protein Pept Sci. (2019) 20:139–44. doi: 10.2174/1389203719666180514150921
107. Yang Z, Jiang R, Chen Q, Wang J, Duan Y, Pang X, et al. Concentration of lactoferrin in human milk and its variation during lactation in different chinese populations. Nutrients. (2018) 10(9). Epub 2018/09/08. doi: 10.3390/nu10091235
108. Turin CG, Zea-Vera A, Rueda MS, Mercado E, Carcamo CP, Zegarra J, et al. Lactoferrin concentration in breast milk of mothers of low-birth-weight newborns. J Perinatol. (2017) 37:507–12. doi: 10.1038/jp.2016.265
109. Albenzio M, Santillo A, Stolfi I, Manzoni P, Iliceto A, Rinaldi M, et al. Lactoferrin levels in human milk after preterm and term delivery. Am J Perinatol. (2016) 33:1085–9. doi: 10.1055/s-0036-1586105
110. Qin Y, Shi W, Zhuang J, Liu Y, Tang L, Bu J, et al. Variations in melatonin levels in preterm and term human breast milk during the first month after delivery. Sci Rep. (2019) 9:17984. doi: 10.1038/s41598-019-54530-2
111. Aparici-Gonzalo S, Carrasco-Garcia A, Gombert M, Carrasco-Luna J, Pin-Arboledas G, Codoner-Franch P. Melatonin content of human milk: the effect of mode of delivery. Breastfeed Med. (2020) 15:589–94. doi: 10.1089/bfm.2020.0157
112. Gila-Diaz A, Herranz Carrillo G, Canas S, Saenz de Pipaon M, Martinez-Orgado JA, et al. Influence of maternal age and gestational age on breast milk antioxidants during the first month of lactation. Nutrients. (2020) 12. doi: 10.3390/nu12092569
113. Katzer D, Pauli L, Mueller A, Reutter H, Reinsberg J, Fimmers R, et al. Melatonin concentrations and antioxidative capacity of human breast milk according to gestational age and the time of day. J Hum Lact. (2016) 32:NP105–NP10. doi: 10.1177/0890334415625217
114. Song BJ, Jouni ZE, Ferruzzi MG. Assessment of phytochemical content in human milk during different stages of lactation. Nutrition. (2013) 29:195–202. doi: 10.1016/j.nut.2012.07.015
115. Xavier AAO, Diaz-Salido E, Arenilla-Velez I, Aguayo-Maldonado J, Garrido-Fernandez J, Fontecha J, et al. Carotenoid content in human colostrum is associated to preterm/full-term birth condition. Nutrients. (2018) 10:1654. doi: 10.3390/nu10111654
116. L'Abbe MR, Friel JK. Superoxide dismutase and glutathione peroxidase content of human milk from mothers of premature and full-term infants during the first 3 months of lactation. J Pediatr Gastroenterol Nutr. (2000) 31:270–4. doi: 10.1097/00005176-200009000-00013
117. Mendelson RA, Anderson GH, Bryan MH. Zinc, copper and iron content of milk from mothers of preterm and full-term infants. Early Hum Dev. (1982) 6:145–51. doi: 10.1016/0378-3782(82)90101-3
118. Alves Peixoto RR, Bianchi Codo CR, Lacerda Sanches V, Guiraldelo TC, Ferreira da Silva F, Ribessi RL, et al. Trace Mineral Composition of Human Breast Milk from Brazilian Mothers. J Trace Elem Med Biol. (2019) 54:199–205. doi: 10.1016/j.jtemb.2019.05.002
119. Zhang Z, Adelman AS, Rai D, Boettcher J, Lonnerdal B. Amino acid profiles in term and preterm human milk through lactation: a systematic review. Nutrients. (2013) 5:4800–21. doi: 10.3390/nu5124800
120. Li X, Peng Y, Li Z, Christensen B, Heckmann AB, Stenlund H, et al. Feeding infants formula with probiotics or milk fat globule membrane: a double-blind, randomized controlled trial. Front Pediatr. (2019) 7:347. doi: 10.3389/fped.2019.00347
121. Qu Y, Guo S, Liu Y, Wang G, Wu H. Association between probiotics and bronchopulmonary dysplasia in preterm infants. Sci Rep. (2021) 11:17060. doi: 10.1038/s41598-021-96489-z
122. Puccio G, Alliet P, Cajozzo C, Janssens E, Corsello G, Sprenger N, et al. Effects of infant formula with human milk oligosaccharides on growth and morbidity: a randomized multicenter trial. J Pediatr Gastroenterol Nutr. (2017) 64:624–31. doi: 10.1097/MPG.0000000000001520
123. Jaramillo-Ospina AM, Toro-Campos R, Murguia-Peniche T, Wampler JL, Wu SS, Berseth CL, et al. Added bovine milk fat globule membrane in formula: growth, body composition, and safety through age 2: an RCT. Nutrition. (2022) 97:111599. doi: 10.1016/j.nut.2022.111599
124. Griffiths J, Jenkins P, Vargova M, Bowler U, Juszczak E, King A, et al. Enteral lactoferrin to prevent infection for very preterm infants: the ELFIN RCT. Health Technol Assess. (2018) 22:1–60. doi: 10.3310/hta22740
125. Gharehbaghi MM, Yeganedoust S, Shaseb E, Fekri M. Evaluation of melatonin efficacy in prevention of bronchopulmonary dysplasia in preterm newborn infants. Turk J Pediatr. (2022) 64:79–84. doi: 10.24953/turkjped.2021.1334
126. Meyer S, Gortner L, NeoVita ATI. Early postnatal additional high-dose oral vitamin a supplementation versus placebo for 28 days for preventing bronchopulmonary dysplasia or death in extremely low birth weight infants. Neonatology. (2014) 105:182–8. doi: 10.1159/000357212
127. Hill C, Guarner F, Reid G, Gibson GR, Merenstein DJ, Pot B, et al. Expert consensus document. The international scientific association for probiotics and prebiotics consensus statement on the scope and appropriate use of the term probiotic. Nat Rev Gastroenterol Hepatol. (2014) 11:506–14. doi: 10.1038/nrgastro.2014.66
128. Kozak K, Charbonneau D, Sanozky-Dawes R, Klaenhammer T. Characterization of bacterial isolates from the microbiota of mothers' breast milk and their infants. Gut Microbes. (2015) 6:341–51. doi: 10.1080/19490976.2015.1103425
129. Jamyuang C, Phoonlapdacha P, Chongviriyaphan N, Chanput W, Nitisinprasert S, Nakphaichit M. Characterization and probiotic properties of lactobacilli from human breast milk. 3 Biotech. (2019) 9:398. doi: 10.1007/s13205-019-1926-y
130. Damaceno QS, Souza JP, Nicoli JR, Paula RL, Assis GB, Figueiredo HC, et al. Evaluation of potential probiotics isolated from human milk and colostrum. Probiotics Antimicrob Proteins. (2017) 9:371–9. doi: 10.1007/s12602-017-9270-1
131. Peiroten A, Arques JL, Medina M, Rodriguez-Minguez E. Bifidobacterial strains shared by mother and child as source of probiotics. Benef Microbes. (2018) 9:231–8. doi: 10.3920/BM2017.0133
132. Wang Y, Wu Y, Wang Y, Xu H, Mei X, Yu D, et al. Antioxidant properties of probiotic bacteria. Nutrients. (2017) 9. doi: 10.3390/nu9050521
133. Do DU, DoGan I. Antioxidant activitiy of lactobacillus plantarum, lactobacillus sake and lactobacillus curvatus strains isolated from fermented turkish sucuk. An Acad Bras Cienc. (2020) 92:e20200105. doi: 10.1590/0001-3765202020200105
134. Averina OV, Poluektova EU, Marsova MV, Danilenko VN. Biomarkers and utility of the antioxidant potential of probiotic lactobacilli and bifidobacteria as representatives of the human gut microbiota. Biomedicines. (2021) 9. doi: 10.3390/biomedicines9101340
135. Feng T, Wang J. Oxidative stress tolerance and antioxidant capacity of lactic acid bacteria as probiotic: a systematic review. Gut Microbes. (2020) 12:1801944. doi: 10.1080/19490976.2020.1801944
136. Ghosh S, Whitley CS, Haribabu B, Jala VR. Regulation of intestinal barrier function by microbial metabolites. Cell Mol Gastroenterol Hepatol. (2021) 11:1463–82. doi: 10.1016/j.jcmgh.2021.02.007
137. Sotoudegan F, Daniali M, Hassani S, Nikfar S, Abdollahi M. Reappraisal of probiotics' safety in human. Food Chem Toxicol. (2019) 129:22–9. doi: 10.1016/j.fct.2019.04.032
138. Yang K, Dong W. Perspectives on probiotics and bronchopulmonary dysplasia. Front Pediatr. (2020) 8:570247. doi: 10.3389/fped.2020.570247
139. Ahern GJ, Hennessy AA, Ryan CA, Ross RP, Stanton C. Advances in infant formula science. Annu Rev Food Sci Technol. (2019) 10:75–102. doi: 10.1146/annurev-food-081318-104308
140. Tu Y, Xu Y, Ren F, Zhang H. Characteristics and antioxidant activity of maillard reaction products from α-lactalbumin and 2′-fucosyllactose. Food Chem. (2020) 316:126341. doi: 10.1016/j.foodchem.2020.126341
141. Charbonneau MR, O'Donnell D, Blanton LV, Totten SM, Davis JC, Barratt MJ, et al. Sialylated milk oligosaccharides promote microbiota-dependent growth in models of infant undernutrition. Cell. (2016) 164:859–71. doi: 10.1016/j.cell.2016.01.024
142. Salminen S, Stahl B, Vinderola G, Szajewska H. Infant formula supplemented with biotics: current knowledge and future perspectives. Nutrients. (2020) 12:1952. doi: 10.3390/nu12071952
143. Reverri EJ, Devitt AA, Kajzer JA, Baggs GE, Borschel MW. Review of the clinical experiences of feeding infants formula containing the human milk oligosaccharide 2′-fucosyllactose. Nutrients. (2018) 10:1346. doi: 10.3390/nu10101346
144. Vandenplas Y, Berger B, Carnielli VP, Ksiazyk J, Lagstrom H, Sanchez Luna M, et al. Human milk oligosaccharides: 2′-fucosyllactose (2′FL) and lacto-N-neotetraose (LNnT) in infant formula. Nutrients. (2018) 10:1161. doi: 10.3390/nu10091161
145. van de Heijning BJ, Berton A, Bouritius H, Goulet O. GI Symptoms in infants are a potential target for fermented infant milk formulae: a review. Nutrients. (2014) 6:3942–67. doi: 10.3390/nu6093942
146. Thomson P, Medina DA, Garrido D. Human milk oligosaccharides and infant gut bifidobacteria: molecular strategies for their utilization. Food Microbiol. (2018) 75:37–46. doi: 10.1016/j.fm.2017.09.001
147. Riviere A, Selak M, Lantin D, Leroy F, De Vuyst L. Bifidobacteria and butyrate-producing colon bacteria: importance and strategies for their stimulation in the human gut. Front Microbiol. (2016) 7:979. doi: 10.3389/fmicb.2016.00979
148. Brink LR, Lonnerdal B. Milk fat globule membrane: the role of its various components in infant health and development. J Nutr Biochem. (2020) 85:108465. doi: 10.1016/j.jnutbio.2020.108465
149. Kosmerl E, Rocha-Mendoza D, Ortega-Anaya J, Jimenez-Flores R, Garcia-Cano I. Improving human health with milk fat globule membrane, lactic acid bacteria, and bifidobacteria. Microorganisms. (2021) 9:341. doi: 10.3390/microorganisms9020341
150. Ortega-Anaya J, Marciniak A, Jimenez-Flores R. Milk fat globule membrane phospholipids modify adhesion of lactobacillus to mucus-producing caco-2/goblet cells by altering the cell envelope. Food Res Int. (2021) 146:110471. doi: 10.1016/j.foodres.2021.110471
151. Lee H, Slupsky CM, Heckmann AB, Christensen B, Peng Y, Li X, et al. Milk fat globule membrane as a modulator of infant metabolism and gut microbiota: a formula supplement narrowing the metabolic differences between breastfed and formula-fed infants. Mol Nutr Food Res. (2021) 65:e2000603. doi: 10.1002/mnfr.202000603
152. Huang S, Wu Z, Liu C, Han D, Feng C, Wang S, et al. Milk fat globule membrane supplementation promotes neonatal growth and alleviates inflammation in low-birth-weight mice treated with lipopolysaccharide. Biomed Res Int. (2019) 2019:4876078. doi: 10.1155/2019/4876078
153. van der Hee B, Wells JM. Microbial regulation of host physiology by short-chain fatty acids. Trends Microbiol. (2021) 29:700–12. doi: 10.1016/j.tim.2021.02.001
154. Prentice PM, Schoemaker MH, Vervoort J, Hettinga K, Lambers TT, van Tol EAF, et al. Human milk short-chain fatty acid composition is associated with adiposity outcomes in infants. J Nutr. (2019) 149:716–22. doi: 10.1093/jn/nxy320
155. Paparo L, Nocerino R, Ciaglia E, Di Scala C, De Caro C, Russo R, et al. Butyrate as a bioactive human milk protective component against food allergy. Allergy. (2021) 76:1398–415. doi: 10.1111/all.14625
156. Tian X, Hellman J, Horswill AR, Crosby HA, Francis KP, Prakash A. Elevated gut microbiome-derived propionate levels are associated with reduced sterile lung inflammation and bacterial immunity in mice. Front Microbiol. (2019) 10:159. doi: 10.3389/fmicb.2019.00518
157. Zhang Q, Ran X, He Y, Ai Q, Shi Y. Acetate downregulates the activation of NLRP3 inflammasomes and attenuates lung injury in neonatal mice with bronchopulmonary dysplasia. Front Pediatr. (2020) 8:595157. doi: 10.3389/fped.2020.595157
158. Chen D, Gao ZQ, Wang YY, Wan BB, Liu G, Chen JL, et al. Sodium propionate enhances Nrf2-mediated protective defense against oxidative stress and inflammation in lipopolysaccharide-induced neonatal mice. J Inflamm Res. (2021) 14:803–16. doi: 10.2147/JIR.S303105
159. Wang B, Timilsena YP, Blanch E, Adhikari B. Lactoferrin: structure, function, denaturation and digestion. Crit Rev Food Sci Nutr. (2019) 59:580–96. doi: 10.1080/10408398.2017.1381583
160. Hu P, Zhao F, Wang J, Zhu W. Lactoferrin attenuates lipopolysaccharide-stimulated inflammatory responses and barrier impairment through the modulation of NF-κB/MAPK/Nrf2 pathways in IPEC-J2 cells. Food Funct. (2020) 11:8516–26. doi: 10.1039/D0FO01570A
161. Barrington KJ, Assaad MA, Janvier A. The lacuna trial: a double-blind randomized controlled pilot trial of lactoferrin supplementation in the very preterm infant. J Perinatol. (2016) 36:666–9. doi: 10.1038/jp.2016.24
162. Manzoni P, Rinaldi M, Cattani S, Pugni L, Romeo MG, Messner H, et al. Bovine lactoferrin supplementation for prevention of late-onset sepsis in very low-birth-weight neonates: a randomized trial. JAMA. (2009) 302:1421–8. doi: 10.1001/jama.2009.1403
163. Manzoni P, Meyer M, Stolfi I, Rinaldi M, Cattani S, Pugni L, et al. Bovine lactoferrin supplementation for prevention of necrotizing enterocolitis in very-low-birth-weight neonates: a randomized clinical trial. Early Hum Dev. (2014) 90 Suppl 1:S60–5. doi: 10.1016/S0378-3782(14)70020-9
164. Dobryk D, Dobryk O, Dobryanskyy D. The effect of enteral lactoferrin supplementation in prevention of morbidity associated with immature digestive tract in premature infants: prospective cohort study. Georgian Med News. (2022) (323):94–101.
165. Schwartz E, Zelig R, Parker A, Johnson S. Vitamin a supplementation for the prevention of bronchopulmonary dysplasia in preterm infants: an update. Nutr Clin Pract. (2017) 32:346–53. doi: 10.1177/0884533616673613
166. Chabra S, Mayock DE, Zerzan J, Bittner R, Neufeld MD, Gleason CA. Vitamin a status after prophylactic intramuscular vitamin a supplementation in extremely low birth weight infants. Nutr Clin Pract. (2013) 28:381–6. doi: 10.1177/0884533613479132
167. Ding Y, Chen Z, Lu Y. Vitamin a supplementation prevents the bronchopulmonary dysplasia in premature infants: a systematic review and meta-analysis. Medicine (Baltimore). (2021) 100:e23101. doi: 10.1097/MD.0000000000023101
168. Huang L, Zhu D, Pang G. The effects of early vitamin a supplementation on the prevention and treatment of bronchopulmonary dysplasia in premature infants: a systematic review and meta-analysis. Transl Pediatr. (2021) 10:3218–29. doi: 10.21037/tp-21-496
169. Abiramalatha T, Ramaswamy VV, Bandyopadhyay T, Somanath SH, Shaik NB, Pullattayil AK, et al. Interventions to prevent bronchopulmonary dysplasia in preterm neonates: an umbrella review of systematic reviews and meta-analyses. JAMA Pediatr. (2022) 176:502–16. doi: 10.1001/jamapediatrics.2021.6619
170. Araki S, Kato S, Namba F, Ota E. Vitamin a to prevent bronchopulmonary dysplasia in extremely low birth weight infants: a systematic review and meta-analysis. PLoS ONE. (2018) 13:e0207730. doi: 10.1371/journal.pone.0207730
171. Rakshasbhuvankar AA, Pillow JJ, Patole SK, Nathan EA, Simmer K. Effect of enteral vitamin a on fecal calprotectin in extremely preterm infants: a nested prospective observational study. Neonatology. (2021) 118:720–6. doi: 10.1159/000518680
172. Rakshasbhuvankar AA, Simmer K, Patole SK, Stoecklin B, Nathan EA, Clarke MW, et al. Enteral vitamin a for reducing severity of bronchopulmonary dysplasia: a randomized trial. Pediatrics. (2021) 147:e2020009985. doi: 10.1542/peds.2020-009985
173. Rakshasbhuvankar A, Patole S, Simmer K, Pillow JJ. Enteral vitamin a for reducing severity of bronchopulmonary dysplasia in extremely preterm infants: a randomised controlled trial. BMC Pediatr. (2017) 17:204. doi: 10.1186/s12887-017-0958-x
174. Rakshasbhuvankar AA, Pillow JJ, Simmer KN, Patole SK. Vitamin a supplementation in very-preterm or very-low-birth-weight infants to prevent morbidity and mortality: a systematic review and meta-analysis of randomized trials. Am J Clin Nutr. (2021) nqab294. doi: 10.1093/ajcn/nqab294
175. Rysavy MA, Li L, Tyson JE, Jensen EA, Das A, Ambalavanan N, et al. Should vitamin a injections to prevent bronchopulmonary dysplasia or death be reserved for high-risk infants? reanalysis of the national institute of Child Health and Human Development Neonatal Research Network Randomized Trial. J Pediatr. (2021) 236:78–85 e5. doi: 10.1016/j.jpeds.2021.05.022
176. Gelfand CA, Sakurai R, Wang Y, Liu Y, Segal R, Rehan VK. Inhaled vitamin a is more effective than intramuscular dosing in mitigating hyperoxia-induced lung injury in a neonatal rat model of bronchopulmonary dysplasia. Am J Physiol Lung Cell Mol Physiol. (2020) 319:L576–L84. doi: 10.1152/ajplung.00266.2020
177. Ge H, Liu W, Li H, Zhang M, Zhang M, Liu C, et al. The association of vitamin d and vitamin e levels at birth with bronchopulmonary dysplasia in preterm infants. Pediatr Pulmonol. (2021) 56:2108–13. doi: 10.1002/ppul.25414
178. Puklin JE, Simon RM, Ehrenkranz RA. Influence on retrolental fibroplasia of intramuscular vitamin e administration during respiratory distress syndrome. Ophthalmology. (1982) 89:96–103. doi: 10.1016/S0161-6420(82)34830-7
179. Speer ME, Blifeld C, Rudolph AJ, Chadda P, Holbein ME, Hittner HM. Intraventricular hemorrhage and vitamin e in the very low-birth-weight infant: evidence for efficacy of early intramuscular vitamin e administration. Pediatrics. (1984) 74:1107–12. doi: 10.1542/peds.74.6.1107
180. Johnson L, Quinn GE, Abbasi S, Otis C, Goldstein D, Sacks L, et al. Effect of sustained pharmacologic vitamin E levels on incidence and severity of retinopathy of prematurity: a controlled clinical trial. J Pediatr. (1989) 114:827–38. doi: 10.1016/S0022-3476(89)80149-0
181. Watts JL, Milner R, Zipursky A, Paes B, Ling E, Gill G, et al. Failure of supplementation with vitamin e to prevent bronchopulmonary dysplasia in infants less than 1,500 G birth weight. Eur Respir J. (1991) 4:188–90.
182. Uberos J, Jimenez-Montilla S, Molina-Oya M, Nieto-Gomez P, Millan IC. Morbidity outcomes of very low birth weight neonates receiving parenteral nutrition with fish oil enriched lipid emulsion or lipid emulsion with soybean oil: an observational study. Am J Perinatol. (2021) 38:952–9. doi: 10.1055/s-0039-1701026
183. Rios J, Valero-Jara V, Thomas-Valdes S. Phytochemicals in breast milk and their benefits for infants. Crit Rev Food Sci Nutr. (2021):1–16. doi: 10.1080/10408398.2021.1906627
184. Tsopmo A. Phytochemicals in human milk and their potential antioxidative protection. Antioxidants (Basel). (2018) 7:32. doi: 10.3390/antiox7020032
185. Poniedzialek B, Rzymski P, Piet M, Gasecka M, Stroinska A, Niedzielski P, et al. Relation between polyphenols, malondialdehyde, antioxidant capacity, lactate dehydrogenase and toxic elements in human colostrum milk. Chemosphere. (2018) 191:548–54. doi: 10.1016/j.chemosphere.2017.10.098
186. Rudrapal M, Khairnar SJ, Khan J, Dukhyil AB, Ansari MA, Alomary MN, et al. Dietary polyphenols and their role in oxidative stress-induced human diseases: insights into protective effects, antioxidant potentials and mechanism(s) of action. Front Pharmacol. (2022) 13:806470. doi: 10.3389/fphar.2022.806470
187. Wojtunik-Kulesza K, Oniszczuk A, Oniszczuk T, Combrzynski M, Nowakowska D, Matwijczuk A. Influence of in vitro digestion on composition, bioaccessibility and antioxidant activity of food polyphenols-a non-systematic review. Nutrients. (2020) 12. doi: 10.3390/nu12051401
188. Vogelsang A, van Lingen RA, Slootstra J, Dikkeschei BD, Kollen BJ, Schaafsma A, et al. Antioxidant role of plasma carotenoids in bronchopulmonary dysplasia in preterm infants. Int J Vitam Nutr Res. (2009) 79:288–96. doi: 10.1024/0300-9831.79.56.288
189. Gila-Diaz A, Arribas SM, Algara A, Martin-Cabrejas MA, Lopez de Pablo AL, Saenz de Pipaon M, et al. A review of bioactive factors in human breastmilk: a focus on prematurity. Nutrients. (2019) 11(6). Epub 2019/06/13. doi: 10.3390/nu11061307
190. Pan L, Fu JH, Xue XD, Xu W, Zhou P, Wei B. Melatonin protects against oxidative damage in a neonatal rat model of bronchopulmonary dysplasia. World J Pediatr. (2009) 5:216–21. doi: 10.1007/s12519-009-0041-2
191. Suleymanoglu S, Cekmez F, Cetinkaya M, Tayman C, Canpolat FE, Kafa IM, et al. Protective effects of melatonin therapy in model for neonatal hyperoxic lung injury. Altern Ther Health Med. (2014) 20:24–9.
192. Staples S, Wall SB Li R, Tipple TE. Selenium-independent antioxidant and anti-inflammatory effects of thioredoxin reductase inhibition in alveolar macrophages. Life Sci. (2020) 259:118285. doi: 10.1016/j.lfs.2020.118285
193. Shan L, Wang B, Gao G, Cao W, Zhang Y. L-Arginine supplementation improves antioxidant defenses through l-arginine/nitric oxide pathways in exercised rats. J Appl Physiol (1985). (2013) 115:1146–55. doi: 10.1152/japplphysiol.00225.2013
194. Gadhia MM, Cutter GR, Abman SH, Kinsella JP. Effects of early inhaled nitric oxide therapy and vitamin a supplementation on the risk for bronchopulmonary dysplasia in premature newborns with respiratory failure. J Pediatr. (2014) 164:744–8. doi: 10.1016/j.jpeds.2013.11.040
195. Liang M, Wang Z, Li H, Cai L, Pan J, He H, et al. L-Arginine induces antioxidant response to prevent oxidative stress via stimulation of glutathione synthesis and activation of Nrf2 pathway. Food Chem Toxicol. (2018) 115:315–28. doi: 10.1016/j.fct.2018.03.029
196. Ma L, Li N, Liu X, Shaw L, Li Calzi S, Grant MB, et al. Arginyl-glutamine dipeptide or docosahexaenoic acid attenuate hyperoxia-induced lung injury in neonatal mice. Nutrition. (2012) 28:1186–91. doi: 10.1016/j.nut.2012.04.001
Keywords: bronchopulmonary dysplasia, premature infants, oxidative stress, human milk, antioxidants
Citation: Yang X, Jiang S, Deng X, Luo Z, Chen A and Yu R (2022) Effects of Antioxidants in Human Milk on Bronchopulmonary Dysplasia Prevention and Treatment: A Review. Front. Nutr. 9:924036. doi: 10.3389/fnut.2022.924036
Received: 20 April 2022; Accepted: 21 June 2022;
Published: 18 July 2022.
Edited by:
Li-Qiang Qin, Soochow University, ChinaReviewed by:
Reza Rastmanesh, American Physical Society, United StatesCopyright © 2022 Yang, Jiang, Deng, Luo, Chen and Yu. This is an open-access article distributed under the terms of the Creative Commons Attribution License (CC BY). The use, distribution or reproduction in other forums is permitted, provided the original author(s) and the copyright owner(s) are credited and that the original publication in this journal is cited, in accordance with accepted academic practice. No use, distribution or reproduction is permitted which does not comply with these terms.
*Correspondence: Ailing Chen, Y2hlbmFpbGluZ0Buam11LmVkdS5jbg==; Renqiang Yu, eXVyZW5xaWFuZzU1M0AxNjMuY29t
†These authors have contributed equally to this work
Disclaimer: All claims expressed in this article are solely those of the authors and do not necessarily represent those of their affiliated organizations, or those of the publisher, the editors and the reviewers. Any product that may be evaluated in this article or claim that may be made by its manufacturer is not guaranteed or endorsed by the publisher.
Research integrity at Frontiers
Learn more about the work of our research integrity team to safeguard the quality of each article we publish.