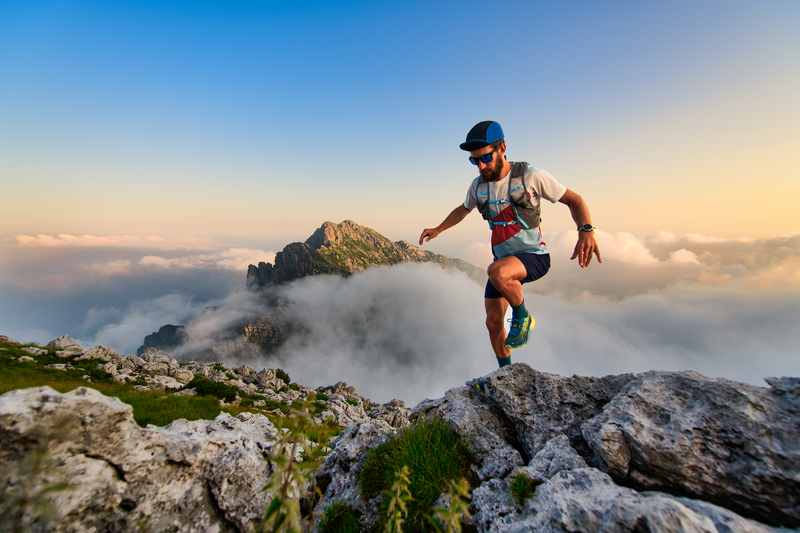
95% of researchers rate our articles as excellent or good
Learn more about the work of our research integrity team to safeguard the quality of each article we publish.
Find out more
ORIGINAL RESEARCH article
Front. Nutr. , 04 July 2022
Sec. Nutrition and Microbes
Volume 9 - 2022 | https://doi.org/10.3389/fnut.2022.921137
This article is part of the Research Topic Next-Generation Prebiotics and Probiotics: Current Status and Future Development View all 7 articles
In this study, the effects of zymosan (HG, hydrolyzed glucan) on the structure and metabolism of fecal microbiota in Chinese healthy people was investigated by an in vitro simulated intestinal microecology fermentation model. We found that HG significantly regulated fecal microbiota composition, including the increase of Bifidobacterium, Faecalibacterium, Prevotella and the decrease of Escherichia-Shigella. Moreover, HG significantly increased the total production of short chain fatty acids (SCFAs) and gases, in which the production of Acetic acid, Propionic acid, CO2, and H2 significantly increased while the production of Isovaleric acid and NH3 significantly decreased. Additionally, the supplement of HG showed certain differences in the regulation of microbiota from four groups. HG significantly increased the relative abundance of Bifidobacterium and significantly decreased the relative abundance of Escherichia-Shigella excluding the older men group. Meanwhile, and the relative abundance of Lactobacillus was significantly increased in young populations. And the relative abundance of Bacteroides was significantly decreased only in the young women. Furthermore, HG significantly increased H2 concentration only in older men. These findings suggest that HG, as a new generation of prebiotics, could regulate the structure of fecal microbiota and its metabolites in a better direction, but when HG participates in precision nutrition formula, it may be necessary to consider the differences in the utilization of different populations.
Recently, with deepening understanding of intestinal microbiota, the relationship between intestinal microbiota and human health has attracted attention. The human intestinal microbiota is composed of nearly one trillion microorganisms, whose diversity and composition are often closely correlated with human health (1). Different dietary habits, age and gender have been shown to lead to different compositions of the intestinal microbiota (2). Some yeasts, such as Saccharomyces boulardii and Saccharomyces baumannii play an important role in human intestines and human health (3, 4). For instance, yeast glucans from yeast cell walls were associated with many prebiotic roles, including anti-tumor, constipation relieving, cholesterol lowering, and gastrointestinal regulation. As an immune enhancer, zymosan is widely used as a feed additive in the field of aquaculture and animal husbandry (5, 6). Besides, it has also been used as a thickener and emulsifier in industries (7).
Zymosan significantly reduces the number of pathogenic bacteria, such as Salmonella and Escherichia coli (8), promoted the proliferation of beneficial bacteria, and increased the contents of total short-chain fatty acids (SCFAs) in pig intestines (9), thereby exerting beneficial effects on the body. Moreover, zymosan effectively improved the intestinal permeability and structural integrity of tight junctions in mice with ulcerative colitis (10). The importance of zymosan on animal intestinal microbiota may contribute to human intestinal health. However, the direct effects of zymosan on human intestinal microbiota have not been conclusively determined.
We evaluated the effects of zymosan on human intestinal microbiota and their metabolites via an in vitro batch fermentation model. Our findings provide a theoretical basis for the use of zymosan as a functional food.
Zymosan (HG, hydrolyzed glucan), a glucan with good water solubility, was obtained by moderate enzymatic hydrolysis of yeast β-glucan. It was provided by Angel Yeast Co. Ltd. It was obtained as: Saccharomyces cerevisiae was cultured, utolyzed, enzymatically hydrolyzed and centrifuged to obtain yeast cell walls and contents. The cell wall was diluted to a concentration of 10% after a certain amount of sodium hydroxide was added, heat treated for 4–6 h, centrifuged, re-dispersed for washing, and spray-dried. Zymosan was obtained by collecting them, and the content with molecular weight in the range of 200–300 kDa is about 95% with good water solubility. Tryptone, yeast extract, L-cysteine, NaCl, KH2PO4, K2HPO4, heme, MgSO4, CaCl2, and crotonic acid were purchased from Sigma Company, USA.
Forty-one volunteers were recruited from local healthy people in Hangzhou (who had no intestinal diseases and had not been administered with antibiotics, prebiotics, and probiotics, et al. in the last 4 weeks). This study was approved by the Ethical Committee of the Hangzhou Center for Disease Control and Prevention (No. 202047). Those aged 20–30 formed the young group, while those aged 40–60 formed the older group. There were 10 people in the young women group (YW), older men group (OM), and older women group (OW). There were 11 people in the young men group (YM). The baseline characteristics of the volunteers included in the study are shown in Table 1. They were provided with sterile stool sampling boxes and asked to quickly pick up no <3 g intermediate stool with less food residues and less oxygen exposure during defecation, mark the name, age and sampling date of the donor. The collected samples were stored at 4°C and assayed within 4 h.
We separately weighed 0.2 g of fresh fecal samples from fecal sampling boxes into three 1.5 mL sterile centrifuge tubes and stored them in a −80°C freezer as raw fecal samples. Then, 0.8 g of the fecal samples were weighed into 10 mL sterile centrifuge tubes, 8 mL of sterile PBS buffer solution was added, and the interfaces sealed using a tape. Finally, fecal and buffer solutions were mixed in the shaker, and the supernatant collected by filtration to obtain a 10% fecal suspension inoculum.
In this study, in vitro fecal fermentation was performed according to the method of Zhao et al. (11). The blank control (Ctrl) medium contained: 10 g/L tryptone, 2.5 g/L yeast extract, 1 g/L L-cysteine, 2 mL/L heme solution, 0.9 g/L NaCl, 0.09 g/L CaCl2·6H2O, 0.45 g/L KH2PO4, 0.45 g/L K2HPO4, and 0.09 g/L MgSO4·7H2O. Immediately after dissolving and boiling, nitrogen was filled to keep the liquid surface of the medium free of oxygen. Then, 4.5 mL culture medium was dispensed into vials using a peristaltic pump. Finally, the vial was sealed with a cap and sterilized by high pressure steaming. HG medium is to add HG to Ctrl medium at a ratio of 0.8 g/100 mL.
In the anaerobic bench, 500 μL of the fresh fecal suspension inoculum was inoculated into 4.5 mL HG medium and Ctrl medium, respectively, using sterile disposable syringes. Then, they were incubated at 37°C for 24 h and stored in a refrigerator at 4°C for use in fermentation. The same group of experiments was conducted three times in parallel.
Microbial community genomic DNA was extracted from fecal and fermentation samples using FastDNA® Spin Kit for Soil (MP Biomedicals, U.S.), according to the manufacturer's instructions. On a thermocycler PCR system (GeneAmp 9700, ABI, San Diego, CA, USA), the V3–V4 hypervariable regions of bacterial 16S rRNA gene were amplified with primers 341F (5′-CCTAYGGGRBGCASCAG-3′) and 806R (5′-GGACTACHVGGGTWTCTAAT-3′). The PCR conditions were: pre-denaturation for 3 min, 27 cycles at 95°C for 30 s, annealing at 55°C for 30 s, elongation at 72°C for 45 s, and 10 min extension at 72°C. Purified amplicons were pooled in equimolar and paired-end sequenced on a NovaSeq PE250 platform (Illumina, San Diego, USA) according to standard protocols by Majorbio Bio-Pharm Technology Co. Ltd. (Shanghai, China).
Amplicon sequence variants (ASVs) were obtained by denoising the optimized sequences after quality control splicing using the DADA2 (12) plugin in the Qiime2 (version 2020.2) (13) process. Taxonomic assignment of ASVs was performed using the Naive bayes consensus taxonomy classifier implemented in Qiime2 and the SILVA 16S rRNA database (v138). All consensus sequence data of raw fecal samples and fermentation samples were submitted to the National Center for Biotechnology Information Short Read Archive under accession no. PRJNA774123.
A 2.5 g of metaphosphoric acid was dissolved in 100 mL deionized water to prepare 100 mL metaphosphoric acid solution that was supplemented with 0.6464 g crotonic acid to prepare a crotonic acid/metaphosphoric acid solution. The fermentation broth (500 μL) and crotonic acid/metaphosphoric acid solution (100 μL) were evenly mixed and stored in a −40°C refrigerator for acidification for 24 h. After acidification, samples were centrifuged for 3 min to separate the supernatants from the precipitate at a speed of 13,000 r/min at 4°C, and filtered via a 0.22 μm hydrophilic micron membrane. Then, 150 μL of the filtered solution was aspirated into a sample vial.
The gas chromatograph was prepared, loaded with the sample after which the aging procedure was performed. Column temperature heating conditions were: column temperature: 80°C for 1 min, 10°C/min, increased to 190°Cand maintained for 0.50 min; then increased to 240°C at a rate of 40°C/min and maintained for 5 min; FID detector: 240°C; gasification chamber: 240°C; carrier gas: nitrogen flow rate 20 mL/min, hydrogen flow rate 40 mL/min, air flow rate 400 mL/min. The obtained data were recorded.
After fermentation, the vial was restored to room temperature after which the gas was automatically analyzed using a gas analyzer to determine gas composition and concentration (14).
The microflora data were analyzed on the online platform of Majorbio Cloud Platform (www.majorbio.com). The alpha diversity was determined by the Wilcoxon rank-sum test between the two groups. And the beta diversity (PCoA and NMDS graphs) was based on the ASV table and bray-curtis distance algorithm to analyze the structural changes of the microbial community at the genus level. Statistical comparisons of taxa abundances at the phylum and genus levels for each group were performed and displayed as Veen and Bar plots. The correlation Heatmap used the correlation coefficient Spearman to evaluate the correlation of each bacterial genera in the fermentation group with SCFAs and gases. KEGG functional prediction analysis was performed by PICRUSt2. GraphPad Prism 8.0.1 was used for data analysis and mapping of gases, SCFAs, four bacterial genera and metabolic functions. SPSS 23.0 was used for data and statistical significance analysis. The Shapiro-Wilk normality test was used to determine whether the data fit a normal distribution. For normally distributed data, paired t-test was performed between two groups, and one-way ANOVA followed by Tukey's multiple comparison test was performed between multiple groups (four populations). The paired Wilcoxon rank test was performed between two groups and the Kruskal-Wallis test was performed between multiple groups when data did not conform to a normal distribution. All results are presented as mean ± SEM (41 independent experiments × 3 parallel experiments). Significant differences were defined as p ≤ 0.05.
The 16S rRNA sequencing technology was used to analyze variations of fecal microbiota in different populations before and after fermentation. After fermentation, the alpha-diversity of the Ctrl group and the HG group was shown in Figure 1A. The Shannon index of the HG group was significantly decreased (P = 0.032), indicating that the community diversity of the HG group was significantly lower than that of the Ctrl group. In addition, PCoA analysis and NMDS analysis showed that there was a significant difference in the genus-level bacterial community structure between the Ctrl group and the HG group after fermentation (P = 0.001, Figures 1B,C). As shown in Figure 1D, at the genus level, Bifidobacterium had the highest abundance in original fecal samples, followed by Blautia, Collinsella, and Escherichia-Shigella. The abundance of these bacteria also varied in the original fecal samples of the four populations. However, the 15 bacterial genera with the highest relative abundance did not show statistical differences among the four populations (Supplementary Figure 2). After 24 h of fermentation, compared to the Ctrl group, the relative abundances of Bifidobacterium (P = 0.002), Streptococcus (P < 0.001), Faecalibacterium (P = 0.028) and Prevotella (P = 0.006) were significantly increased in the HG group. While the relative abundance of Escherichia-Shigella (P = 0.001), Phascolarctobacterium (P = 0.004) and Dorea (P < 0.001) in the HG group were significantly lower than in the Ctrl group (Figure 1E).
Figure 1. Composition of original fecal microbiota and the microbiota after fermentation. (A) Alpha-diversity analysis and (B) Beta-diversity PCoA analysis and (C) NMDS analysis of Ctrl group and HG group; (D) Microbiota composition of original fecal samples and fermentation groups; (E) Abundance comparison at genus level between Ctrl group and HG group. Data are means ± SEM (41 independent experiments × 3 replication experiments). Statistical significance thresholds were: *0.01 < p ≤ 0.05; **0.001 < p ≤ 0.01; ***p ≤ 0.001.
We further analyzed the effect of gender and age on the fermentation results (Figure 2). The results showed that the trend of relative abundance of mainly bacteria at genus level was independent of gender and age. In the HG group, the relative abundance of Escherichia-Shigella decreased and the relative abundance of Bifidobacterium increased in all populations. The three bacterial genera with the highest abundance after fermentation are Escherichia-Shigella, Bacteroides, Bifidobacterium, and Lactobacillus is a probiotic that is often studied. We performed statistical tests on the relative abundance of these four bacteria in the two media. The results showed that the relative abundance of Bifidobacterium was significantly increased in the young men (P = 0.004), the young women (P = 0.002) and the older women population (P = 0.009). While the relative abundance of Escherichia-Shigella was significantly decreased in the young men (P = 0.003), the young women (P = 0.005) and the older women population (P = 0.009). Additionally, in the HG group, the relative abundance of Lactobacillus was significantly increased in young men (P = 0.025) and young women population (P = 0.040). And the relative abundance of Bacteroides was significantly decreased only in the young women population (P = 0.04). For the older men population, the effects of HG on these four bacteria did not show statistical significance. Therefore, fecal microbiota in different populations varied differentially in response to HG and were susceptible to gender and age.
Figure 2. Bacterial composition of four populations in two media after fermentation. (A) Genus-level microbiota composition for all groups; the relative abundance ratios of the four bacteria in (B) YM, (C) OM, (D) YW, and (E) OW after fermentation. Data are means ± SEM (41 independent experiments × 3 replication experiments). Statistical significance thresholds were: *0.01 < p ≤ 0.05; **0.001 < p ≤ 0.01.
Fecal microbiota of different populations generated a lot of SCFAs after 24 h fermentation. As shown in Figure 3, the levels of six SCFAs in fermentation broth, including acetic acid (Ace), propionic acid (Pro), isobutyric acid (Isob), butyric acid (But), isovaleric acid (Isov), and pentanoic acid (Pen), were determined. Compared with the Ctrl group, the levels of total SCFAs were significantly increased in the HG group (Figure 3A). The levels of Ace were higher than those of the other SCFAs, followed by Pro and But (Figure 3B). Levels of total SCFAs (P = 0.006), Ace (P = 0.023), and Pro (P = 0.003) in the HG medium were significantly increased. While the levels of Isov (P = 0.034) was significantly decreased.
Figure 3. Effects of HG on the levels of SCFAs produced by fecal microbiota in different populations in the in vitro fermentation model. The levels of total acids (A) and six SCFAs (B) produced by the fecal microbiota from all populations, and the levels of acetic acid (C), propionic acid (D), isobutyric acid (E), butyric acid (F), isovaleric acid (G), and pentanoic acid (H) produced by the fecal microbiota in the four populations. Data are means ± SEM (41 independent experiments × 3 replication experiments). Statistical significance thresholds were: *0.01 < p ≤ 0.05; **0.001 < p ≤ 0.01.
Collectively, these observations indicated that the varying trends of SCFAs in different populations are not identical after HG intervention based on gender and age. Compared with the Ctrl group, HG relatively increased the levels of Ace and Pro in different populations (Figures 3C,D), and relatively reduced the levels of Isob and Isov in some women (Figures 3E,G). The levels of But were relatively higher in young women and old women in the HG group (Figure 3F). In addition, HG only relatively increased the Pen levels in some young women and men (Figure 3H).
The composition and content of gas produced during fecal microbiota fermentation are shown in Figure 4. After 24 h of fermentation by fecal microbiota, the HG medium of the experimental group and Ctrl medium of the control group had large amounts of CO2, H2 and small amounts of H2S, CH4, and NH3. And the concentrations of gas in the HG group was significantly higher than that in the Ctrl group (P < 0.001, Figure 4A). CO2, as the most abundant gas produced by fecal microbiota, was significantly higher in the HG group, relative to the Ctrl group (P < 0.001). Additionally, the concentrations of H2 in the HG group were significantly increased (P < 0.001, Figure 4B), while the concentrations of NH3 in the HG group were significantly lower than those of the Ctrl group (P = 0.002). Differences in concentrations of CH4 and H2S were insignificant between the Ctrl and HG group (Figure 4C).
Figure 4. Effects of HG on the concentration of gas produced by fecal microbiota in different populations in the in vitro fermentation model. The concentration of total gases (A) and five gases (B,C) produced by the fecal microbiota from all populations, and the concentration of CO2 (D), H2 (E), CH4 (F), H2S (G), and NH3 (H) produced by fecal microbiota in the four populations. Data are means ± SEM (41 independent experiments × 3 replication experiments). Statistical significance thresholds were: *0.01 < p ≤ 0.05; **0.001 < p ≤ 0.01; ***p ≤ 0.001.
There were no statistically significant differences among the four populations for the five gases in the Ctrl group or HG group. And the concentrations of CH4, H2S, and NH3 did not exhibit differences in each population group (Figures 4F–H). However, compared with the Ctrl group, the supplement of HG significantly increased the concentration of CO2 in different populations. Of note, fecal microbiota in the older women group produced relatively higher concentrations of CO2 than the other groups. In the HG group, the concentrations of CO2 in young men (P = 0.028), older men (P < 0.001), young women (P = 0.010), and older women (P < 0.001) was significantly higher than those in the Ctrl group (Figure 4D). The concentrations of H2 for older men in the HG group were also significantly increased (P = 0.040, Figure 4E). In addition, the concentrations of NH3 were relatively high in the women group relative to the men group (Figure 4H).
Previous experimental results showed that HG could significantly affect the microbiota structure and the production of SCFAs and gases. Therefore, we used heatmaps and Spearman correlation coefficients to analyze correlations between gases, SCFAs and fecal microbiota (Figure 5). Among the top 15 fecal microbiota in genus-level abundance, all gases and SCFAs were jointly correlated various bacteria, except for methane, which was only significantly negatively correlated with Streptococcus. The concentrations of H2S were found to be correlated with the most bacteria. With regards to individual bacteria, there was no bacterial genus that had marked effects on the production of all gases or SCFAs, however, Faecalibacterium had the most extensive influence and could significantly affect the production of NH3, CO2, H2S, Ace, Pro, But, Pen, and Isov. Escherichia-Shigella, which accounted for the highest relative abundance, had a significant negative correlation with the concentrations of CO2, H2S, Pro, But, Pen, and Isov. As two probiotics, Bifidobacterium was only significantly negatively correlated with NH3, while Lactobacillus was only significantly negatively correlated with H2.
Figure 5. Correlation between fecal microbiota and gas (A) and short-chain fatty acid (B) in fermentation samples. Data are means ± SEM (41 independent experiments × 3 replication experiments). Statistical significance thresholds were: *0.01 < p ≤ 0.05; **0.001 < p ≤ 0.01; ***p ≤ 0.001.
On the basis of 16S rRNA sequencing data, we used PICRUSt2 to predict the metabolic functional of the fecal microbiota, and the results are shown in Figure 6. At level 2, nucleotide metabolism (p = 0.003) was significantly improved in the HG group compared with the Ctrl group. While energy metabolism (P < 0.001) and metabolism of cofactors and vitamins (p = 0.024) were significantly lower in the HG group. There were no significant differences in other metabolic functions. In order to ensure the accuracy, we only analyzed the metabolic functions of 15 bacterial groups whose relative abundance accounted for more than 1% at the level 3. Twelve of these metabolic functions were significantly different between the HG and Ctrl groups (Figure 6B). Biosynthesis of secondary metabolites (P < 0.001), biosynthesis of amino acids (P = 0.002), starch and sucrose metabolism (P < 0.001), amino sugar and nucleotide sugar metabolism (P < 0.001), glycolysis/gluconeogenesis (P < 0.001), cysteine and methionine metabolism (p = 0.006), glycine, serine, and threonine metabolism (P < 0.001), pyrimidine metabolism (P < 0.001), and purine metabolism (p = 0.020) were all significantly elevated in the HG group. However, the relative abundances of genes related to microbial metabolism in diverse environments (P < 0.001), pyruvate metabolism (p = 0.001), and oxidative phosphorylation (p = 0.017) were significantly reduced in the HG group.
Figure 6. Variation in bacteria function profiles during anaerobic fermentation analyzed by PICRUSt2: (A) metabolic pathways abundance on KEGG categories (level 2); (B) metabolic pathways abundance on KEGG categories (level 3). Data are means ± SEM (41 independent experiments × 3 replication experiments). Statistical significance thresholds were: *0.01 < p ≤ 0.05; **0.001 < p ≤ 0.01; ***p ≤ 0.001.
There are nearly one trillion microorganisms in the human intestines, and the diversity and composition of these microbiota are often closely correlated with human health (1). In vitro simulated fermentation models of the intestinal have been often used to analyze the effects of functional substances on the intestinal microbiota (15–18). We analyzed the effects of HG on fecal microbiota and metabolite compositions in different populations of varying age and genders using an in vitro fermentation model.
There were variations in microbiota structure before and after fermentation. Before fermentation, Bifidobacterium accounted for the highest abundance of fecal microbiota, which is also the main intestinal commensal bacteria colonizing human infants since birth (19). The second genus with the highest relative abundance was Blautia, an anaerobic bacterium with probiotic characteristics (20). However, in the Ctrl group after fermentation, Escherichia-Shigella and Bacteroides had the highest abundances. The abundance of these two bacteria in the intestines of colorectal cancer patients exhibited an increasing trend (21, 22). And Escherichia-Shigella are harmful to the intestinal mucosa (23). After completion of fermentation, compared to the Ctrl group, HG significantly decreased the abundance of Escherichia-Shigella and relatively decreased the abundance of Bacteroides. Besides, the relative abundance of Bifidobacterium, an important probiotic for maintaining intestinal homeostasis (24), was significantly increased. Some species of Streptococcus such as S. thermophiles and S. salivarius are used as probiotics (25). Faecalibacterium can serve as a marker for healthy intestinal and can be associated with anti-inflammatory properties (26, 27). And the relative abundances of Streptococcus and Faecalibacterium were also significantly increased in the HG group. In addition, as important producers of acetate and propionate, the relative abundance of Prevotella (28) significantly increased. These results indicate that HG enriched and increased the abundance of beneficial bacteria such as Bifidobacterium and Prevotella in the intestines, reduced the abundance of harmful bacteria such as Escherichia-Shigella and Bacteroides, and finally improved the imbalance of fecal microbiota.
The abundance of fecal microbiota from different groups exhibited certain differences, which were established to be significant after fermentation (Supplementary Figure 1), indicating that the regulation ability of HG on fecal microbiota is affected by gender and age. For instance, it may be concluded from Figure 2 that the promotion effect of HG on Bifidobacterium and the inhibition effect of HG on Escherichia-Shigella were not significant in older men. Moreover, the promoting effect of HG on Lactobacillus was only significant in the young population.
During fermentation, changes in fecal microbiota structure of different populations are associated with changes in bacterial metabolites. The degradation products of carbohydrates by intestinal microbiota are mainly SCFAs, including Ace, Pro, Isob, But, Isov and Pen (29, 30). In this study, compared with the Ctrl group, the levels of total SCFAs were significantly increased in the HG group (Figure 3A). SCFAs are mediators of metabolic interactions between intestinal microbiota and hosts (31), they can regulate the activation immune cell functions by activating G protein-coupled receptors and inhibiting histone deacetylase (32, 33), and also promote intestinal homeostasis and oral tolerance (34, 35). Among them, the HG medium significantly increased the levels of Ace and Pro (Figure 3B). Pro has been shown to alleviate multiple sclerosis through immune regulation mechanisms (36), or reduce hypertension and cardiovascular injury (37). And Ace and Pro are important energy and signaling molecules (38). As other important metabolites for maintaining human health, the levels of Isob, But and Pen were no statistical difference between two groups, and were not significantly different between different populations (Figure 3). The reason may be due to the large individual differences, and more samples may be required to show significant differences.
The levels of each SCFAs were significantly correlated with various microbiota (Figure 5). Among the top 15 microbial groups by relative abundance, Ace, Pro, and But were significantly positively correlated with bacteria such as Faecalibacterium (39, 40), Blautia (41, 42), and Megasphaera (43, 44), however, they were significantly negatively correlated with Escherichia-Shigella. After fermentation, the relative abundance of Faecalibacterium, Blautia and Megasphaera in the HG medium was increased while that of Escherichia-Shigella was decreased. Therefore, it could be concluded that the levels of Ace, Pro, and But in HG medium were increased.
Carbohydrates that are available to intestinal microbiota can influence microbiota composition and the levels of gas and SCFAs produced (45). In addition, there are also significant differences in the abundance of intestinal microbiota among individuals in different regions, genders, and ages (46). In this study, we found gender- and age-associated variations in fecal microbiota. Fecal microbiota structures of the four populations showed significant differences after fermentation. During fermentation, fecal microbiota from women groups produced relatively more But, Pen, Isob, and Isov. The older groups produced relatively more But, while the young groups produced relatively more Pen. Apart from CH4, which was less affected by age and gender, the concentrations of the other four gases exhibited age- and gender-associated variations. For instance, the concentrations of CO2 produced by fecal microbiota of the older women population were relatively higher than those of the other groups. In contrast, the young population produced relatively more H2S, compared to the older population. While the women population produced relatively more H2S and NH3, relative to the men population. Therefore, people of different genders and ages had variations in fecal microbiota structures, which were further exacerbated by fermentation. These variations led to differences in microbiota products (such as SCFAs and gases) between the four populations.
In addition to SCFAs, gas production is an important index for maintaining intestinal microbiota balance (47). The composition and volume of intestinal gas are often adjusted by regulating the carbohydrates, proteins and fats in diets, which may achieve the purpose of preventing and treating the related gastrointestinal diseases (48). In this study, after 24 h of fermentation, fecal microbiota produced large amounts of CO2, H2 and small amounts of H2S, CH4, and NH3. Compared to the Ctrl group, the concentrations of CO2 and H2 in the HG group were significantly increased. As the final product of carbohydrate fermentation, H2 is a core component of metabolic homeostasis in the human gastrointestinal tract (49). H2S and NH3 are by-products of colonic bacterial fermentation of amino acids, such as methionine, cystine, cysteine, and taurine, which are detrimental to intestinal health (50). Both H2S and NH3 are linked to gastrointestinal diseases, such as ulcerative colitis, Crohn's disease and irritable bowel syndrome (51). The concentrations of NH3 were significantly reduced during the fermentation of the HG substrate, and the concentration of H2S was relatively reduced, indicating that HG is beneficial for intestinal health.
Intestinal microbiota composition may also affect the metabolic capacity of intestinal bacteria (52). CO2 is a key molecule in many biological processes (53). We found that CO2 was significantly negatively correlated with conditionally pathogenic Escherichia-Shigella, and significantly positively correlated with Faecalibacterium. And Faecalibacterium are potential indicators of gastrointestinal health (54). Therefore, the concentrations of CO2 in the HG group imply beneficial effects of the probiotics on human health. Similarly, there were significant correlations between in vivo H2 production and specific taxa of microbes, including Bacteroides and Parasutterella. Biologically, H2 has beneficial effects on the gastrointestinal tract (55). We found that H2 was significantly positively correlated with Streptococcus and negatively correlated with Lactobacillus. After HG intervention, the abundance of Streptococcus was highly increased, relative to that of Lactobacillus. Therefore, the concentration of H2 in the HG medium was increased. In addition, NH3 and H2S were significantly negatively correlated with Bifidobacterium, which were highly abundant. This may be the reason for the decreased concentration of NH3 and H2S in the HG medium.
At present, the utility of PICRUSt2 in predicting microbiota function has been validated (56). The results of KEGG showed that, among the top 15 metabolism-related functions in abundance, the addition of HG significantly increased the relative abundance of glycolysis/gluconeogenesis, biosynthesis of amino acids and secondary metabolites, amino acid (such as glycine, serine, threonine, cysteine, methionine) and nucleotide metabolism. At the same time, HG significantly suppressed pyruvate metabolism and oxidative phosphorylation, which eventually led to significant reduction in energy metabolism in the HG group. These results indicated that addition of HG change the metabolism pathway of fecal microbiota. Additionally, HG could significantly promote or inhibit certain metabolic functions in some populations. For example, the relative abundance of Biosynthesis of secondary metabolites increases significantly only in women populations. However, these metabolic functions did not differ significantly between the four populations (Supplementary Figures 3, 4).
In general, during fermentation, HG regulates the composition of fecal microbiota by inhibiting the growth of Escherichia-Shigella and Bacteroides while promoting the abundance of Bifidobacterium, Lactobacillus, and other beneficial bacteria, so as to regulate the metabolic products of fecal microbiota, including SCFAs and gases. Moreover, different groups may be regulated by HG due to differences in fecal microbiota and present different characteristics. However, the metabolic mechanism of HG needs to be further studied.
Our study identified that the fermentation of HG changed the fecal microbial composition by increasing the abundance of beneficial bacteria such as Bifidobacterium, Faecalibacterium, Prevotella and reducing the abundance of harmful bacteria such as Escherichia-Shigella. As important metabolites of the fecal microbiota, the total production of SCFAs and gases was significantly increased in the HG group, among which the production of Ace, Pro, CO2, and H2 was significantly increased, while the production of Isov and NH3 was significantly decreased. Additionally, the supplement of HG showed certain differences in the regulation of microbiota from four populations. HG significantly increased the relative abundance of Bifidobacterium and significantly decreased the relative abundance of Escherichia-Shigella in the populations other than older men. The relative abundance of Lactobacillus was significantly increased in young populations. And the relative abundance of Bacteroides was significantly decreased only in the young women. Moreover, HG significantly increased H2 concentration in older men group. Taken together, HG could regulate the structure of fecal microbiota and its metabolites in a better direction, but different populations may be regulated by HG due to differences in fecal microbiota and present different characteristics. This should be taken into account when HG participates in precision nutrition formulations as functional foods.
The datasets presented in this study can be found in online repositories. The names of the repository/repositories and accession number(s) can be found at: https://www.ncbi.nlm.nih.gov/, PRJNA774123.
The studies involving human participants were reviewed and approved by the Ethics Committee of the Hangzhou Center for Disease Control and Prevention (No. 202047). The patients/participants provided their written informed consent to participate in this study.
XP: funding acquisition, data curation, formal analysis, and writing—original draft. ZY: data curation, formal analysis, and methodology. XY: data curation and formal analysis. ZD: sample collection. WL: methodology, supervision, project administration, and review. All authors contributed to the article and approved the submitted version.
This work was supported by Hangzhou Agricultural and Society Development Project (Grant No. 202004A20).
The authors declare that the research was conducted in the absence of any commercial or financial relationships that could be construed as a potential conflict of interest.
All claims expressed in this article are solely those of the authors and do not necessarily represent those of their affiliated organizations, or those of the publisher, the editors and the reviewers. Any product that may be evaluated in this article, or claim that may be made by its manufacturer, is not guaranteed or endorsed by the publisher.
The Supplementary Material for this article can be found online at: https://www.frontiersin.org/articles/10.3389/fnut.2022.921137/full#supplementary-material
1. Altamura F, Maurice CF, Castagner B. Drugging the gut microbiota: toward rational modulation of bacterial composition in the gut. Curr Opin Chem Biol. (2020) 56:10–5. doi: 10.1016/j.cbpa.2019.09.005
2. De La Cuesta-Zuluag J, Kelley ST, Chen Y, Escobar JS, Mueller NT, Ley RE. Age- and sex-dependent patterns of gut microbial diversity in human adults. Msystems. (2019) 4:e00261-19. doi: 10.1128/mSystems.00261-19
3. Offei B, Vandecruys P, De Graeve S, Foulquie-Morenot MR, Thevelein JM. Unique genetic basis of the distinct antibiotic potency of high acetic acid production in the probiotic yeast Saccharomyces cerevisiae Var. Boulardii. Genome Res. (2019) 29:1478–94. doi: 10.1101/gr.243147.118
4. Saber A, Alipour B, Faghfoori Z, Khosroushahi AY. Cellular and molecular effects of yeast probiotics on cancer. Crit Rev Microbiol. (2017) 43:96–115. doi: 10.1080/1040841X.2016.1179622
5. Gong P, Zhang L. Biological function of zymosan and its application in animal breeding. Feed Res. (2021) 44:128–31. doi: 10.13557/j.cnki.issn1002-2813.2021.16.029
6. Pan YF. Research and application of zymosan in animal nutrition. Anim Husbandry Vet Sci Technol Information. (2020) 7:12–3. doi: 10.D3969/J.ISSND1671-6027.D2020.D07.007
7. Zhao J, Ren JQ, Lu CX, Diao XY, Zhang TJ. Research progress of yeast cell wall polysaccharides. Chinese Food Additives. (2009) 1:82–5. doi: 10.3969/j.issn.1006-2513.2009.01.012
8. He Q, Wang Z, You J, Chen L, Xiong H. Effects of yeast wall polysaccharide on intestinal volatile fatty acids and microbial flora of weaned piglets. J Animal Nutr. (2017) 29:177–83. doi: 10.3969/j.issn.1006-267x.2017.01.020
9. Sweeney T, Collins CB, Reilly P, Pierce KM, Ryan M, O"Doherty JV. Effect of purified B-glucans derived from Laminaria digitata, Laminaria hyperborea and Saccharomyces cerevisiae on piglet performance, selected bacterial populations, volatile fatty acids and pro-inflammatory cytokines in the gastrointestinal tract of pigs. Br J Nutr. (2012) 108:1226–34. doi: 10.1017/S0007114511006751
10. Han F, Fan H, Yao M, Yang S, Han J. Oral administration of yeast beta-glucan ameliorates inflammation and intestinal barrier in dextran sodium sulfate-induced acute colitis. J Functional Foods. (2017) 35:115–26. doi: 10.1016/j.jff.2017.05.036
11. Zhao Z, Liu W, Pi X. In vitro effects of stachyose on the human gut microbiota. Starch. (2021) 73:7–8. doi: 10.1002/star.202100029
12. Callahan BJ, Mcmurdie PJ, Rosen MJ, Han AW, Johnson AJ, Holmes SP. DADA2: high-resolution sample inference from Illumina amplicon data. Nat Methods. (2016) 13:581–3. doi: 10.1038/nmeth.3869
13. Bolyen E, Rideout JR, Dillon MR, Bokulich NA, Abnet CC, Al-Ghalith GA, et al. Reproducible, interactive, scalable and extensible microbiome data science using QIIME 2. Nat Biotechnol. (2019) 37:852–7. doi: 10.1038/s41587-019-0209-9
14. Ye XW, Zheng WX, Cen YX, Ni JH, Xu LY, Wu KF, et al. The methanol extract of Polygonatum odoratum ameliorates colitis by improving Intestinal Scfas and gas production to regulate microbiota dysbiosis in mice. Front Nutr. (2022) 9:899421. doi: 10.3389/fnut.2022.899421
15. Mao YH, Song AX, Li LQ, Siu KC, Yao ZP, Wu JY. Effects of exopolysaccharide fractions with different molecular weights and compositions on fecal microflora during in vitro fermentation. Int J Biol Macromol. (2019) 144:76–84. doi: 10.1016/j.ijbiomac.2019.12.072
16. Wang M, Wichienchot S, He X, Fu X, Huang Q, Zhang B. In vitro colonic fermentation of dietary fibers: fermentation rate, short-chain fatty acid production and changes in microbiota. Trends Food Sci Technol. (2019) 88:7. doi: 10.1016/j.tifs.2019.03.005
17. Gu I, Lam WS, Marasini D, Savary BJ, Lee J, Carbonero F, et al. In vitro fecal fermentation patterns of arabinoxylan from rice bran on fecal microbiota from normal-weight and overweight/obese subjects. Nutrients. (2021) 13:2052. doi: 10.3390/nu13062052
18. Pham VT, Mohajeri MH. The application of in vitro human intestinal models on the screening and development of pre- and probiotics. Beneficial Microbes. (2018) 9:725–42. doi: 10.3920/BM2017.0164
19. Milani C, Mangifesta M, Mancabelli L, Lugli GA, James K, Duranti S, et al. Unveiling bifidobacterial biogeography across the mammalian branch of the tree of life. ISME J. (2017) 11:2834–47. doi: 10.1038/ismej.2017.138
20. Liu X, Mao B, Gu J, Wu J, Chen W. Blautia—a new functional genus with potential probiotic properties? Gut Microbes. (2021) 13:1–21. doi: 10.1080/19490976.2021.1875796
21. Andrews MC, Duong CPM, Gopalakrishnan V, Iebba V, Chen W-S, Derosa L, et al. Gut microbiota signatures are associated with toxicity to combined Ctla-4 and Pd-1 blockade. Nat Med. (2021) 27:1432. doi: 10.1038/s41591-021-011406-6
22. Frost F, Kacprowski T, Ruehlemann M, Pietzner M, Bang C, Franke A, et al. Long-term instability of the intestinal microbiome is associated with metabolic liver disease, low microbiota diversity, diabetes mellitus and impaired exocrine pancreatic function. Gut. (2021) 70:522–30. doi: 10.1136/gutjnl-2020-322753
23. Flemer B, Warren RD, Barrett MP, Cisek K, Das A, Jeffery IB, et al. The oral microbiota in colorectal cancer is distinctive and predictive. Gut. (2018) 67:1454–63. doi: 10.1136/gutjnl-2017-314814
24. Guo M, Wang H, Xu S, Zhuang Y, An J, Su C, et al. Alteration in gut microbiota is associated with dysregulation of cytokines and glucocorticoid therapy in systemic lupus erythematosus. Gut Microbes. (2020) 11:1758–73. doi: 10.1080/19490976.2020.1768644
25. Wei X, Tsai T, Knapp J, Bottoms K, Deng F, Story R, et al. ZnO modulates swine gut microbiota and improves growth performance of nursery pigs when combined with peptide cocktail. Microorganisms. (2020) 8:146. doi: 10.3390/microorganisms8020146
26. Rebeca M, Sylvie M, Leandro B, Bridonneau C, Robert V, Hudault S, et al. Functional characterization of novel Faecalibacterium prausnitzii strains isolated from healthy volunteers: a step forward in the use of F. prausnitzii as a next-generation probiotic. Front Microbiol. (2017) 8:1226. doi: 10.3389/fmicb.2017.01226
27. Tomova A, Bukovsky I, Rembert E, Yonas W, Alwarith J, Barnard ND, et al. The effects of vegetarian and vegan diets on gut microbiota. Frontiers in Nutrition. (2019) 6:47. doi: 10.3389/fnut.2019.00047
28. Emerson EL, Weimer PJ. Fermentation of model hemicelluloses by prevotella strains and Butyrivibrio fibrisolvens in pure culture and in ruminal enrichment cultures. Appl Microbiol Biotechnol. (2017) 101:4269–78. doi: 10.1007/s00253-017-8150-7
29. Morrison DJ, Preston T. Formation of short chain fatty acids by the gut microbiota and their impact on human metabolism. Gut Microbes. (2016) 7:189–200. doi: 10.1080/19490976.2015.1134082
30. Rodriguez-Carrio J, Salazar N, Margolles A, Gonzalez S, Gueimonde M, de los Reyes-Gavilan CG, et al. Free fatty acids profiles are related to gut microbiota signatures and short-chain fatty acids. Front Immunol. (2017) 8, 823. doi: 10.3389/fimmu.2017.00823
31. Serino M. Scfas - the thin microbial metabolic line between good and bad. Nat Rev Endocrinol. (2019) 15:318–9. doi: 10.1038/s41574-019-0205-7
32. Park J, Kim M, Kang SG, Jannasch AH, Cooper B, Patterson J, et al. Short-chain fatty acids induce both effector and regulatory T cells by suppression of histone deacetylases and regulation of the Mtor-S6k pathway. Mucosal Immunol. (2015) 8:80–93. doi: 10.1038/mi.2014.44
33. Vieira AT, Galvao I, Macia LM, Sernaglia EM, Vinolo MAR, Garcia CC, et al. Dietary fiber and the short-chain fatty acid acetate promote resolution of neutrophilic inflammation in a model of gout in mice. J Leukocyte Biol. (2017) 101:275. doi: 10.1189/jlb.3A1015-453RRR
34. Correa RO, Fachi JL, Vieira A, Sato FT, Vinolo MAR. Regulation of immune cell function by short-chain fatty acids. Clin Transl Immunol. (2016) 5:e73. doi: 10.1038/cti.2016.17
35. Smith PM, Howitt MR, Panikov N, Michaud M, Gallini CA, Bohlooly YM, et al. The microbial metabolites, short-chain fatty acids, regulate colonic T-Reg cell homeostasis. Science. (2013) 341:569–73. doi: 10.1126/science.1241165
36. Flossmann V, Cross A, Issa F. Propionic acid shapes the multiple sclerosis disease course by an immunomodulatory mechanism. Transplantation. (2020) 104:1112. doi: 10.1097/TP.0000000000003273
37. Bartolomaeus H, Balogh A, Yakoub M, Homann S, Marko L, Hoeges S, et al. Short-chain fatty acid propionate protects from hypertensive cardiovascular damage. Circulation. (2019) 139:1407–21. doi: 10.1161/CIRCULATIONAHA.118.036652
38. Koh A, De Vadder F, Kovatcheva-Datchary P, Backhed F. From dietary fiber to host physiology: short-chain fatty acids as key bacterial metabolites. Cell. (2016) 165:1332–45. doi: 10.1016/j.cell.2016.05.041
39. Louis P, Flint HJ. Diversity, metabolism and microbial ecology of butyrate-producing bacteria from the human large intestine. FEMS Microbiol Lett. (2009) 294:1–8. doi: 10.1111/j.1574-6968.2009.01514.x
40. Rivière A, Selak M, Lantin D, Leroy F, de Vuyst L. Bifidobacteria and butyrate-producing colon bacteria: importance and strategies for their stimulation in the human gut. Front Microbiol. (2016) 7:979. doi: 10.3389/fmicb.2016.00979
41. Li F, Hitch T, Chen Y, Creevey CJ, Guan LL. Comparative metagenomic and metatranscriptomic analyses reveal the breed effect on the rumen microbiome and its associations with feed efficiency in beef cattle. Microbiome. (2019) 7:6. doi: 10.1186/s40168-019-0618-5
42. Kim HJ, Kim D, Kim KW, Lee SH, Jang A. Comparative analysis of the gut microbiota of mice fed a diet supplemented with raw and cooked beef loin powder. Sci Rep. (2021) 11:1. doi: 10.1038/s41598-021-90461-7
43. Shabat S, Sasson G, Doron-Faigenboim A, Durman T, Yaacoby S, Berg Miller ME, et al. Specific microbiome-dependent mechanisms underlie the energy harvest efficiency of ruminants. ISME J. (2016) 10:2958–72. doi: 10.1038/ismej.2016.62
44. Duncan SH, Louis P, Flint HJ. Lactate-utilizing bacteria, isolated from human feces, that produce butyrate as a major fermentation product. Appl Environ Microbiol. (2004) 70:5810–7. doi: 10.1128/AEM.70.10.5810-5817.2004
45. Rastall RA, Gibson GR, Gill HS, Fransisco G, Klaenhammer TR, Bruno P, et al. Modulation of the microbial ecology of the human colon by probiotics, prebiotics and synbiotics to enhance human health: an overview of enabling science and potential applications. FEMS Microbiol Ecol. (2005) 52:145–52. doi: 10.1016/j.femsec.2005.01.003
46. Falony G, Joossens M, Vieira-Silva S, Wang J, Darzi Y, Faust K, et al. Population-level analysis of gut microbiome variation. Science. (2016) 352:560. doi: 10.1126/science.aad3503
47. Kalantar-Zadeh K, Berean KJ, Burgell RE, Muir JG, Gibson PR. Intestinal gases: influence on gut disorders and the role of dietary manipulations. Nat Rev Gastroenterol Hepatol. (2019) 16:733–47. doi: 10.1038/s41575-019-0193-z
48. Lena B, Stine S, Therese L, Lena C, Perjohan L, Hans T, et al. Diet low in fodmaps reduces symptoms of irritable bowel syndrome as well as traditional dietary advice: a randomized controlled trial. Gastroenterology. (2015) 149:1399–407.e2. doi: 10.1053/j.gastro.2015.07.054
49. Wolf PG, Biswas A, Morales SE, Greening C, Gaskins HR. H-2 metabolism is widespread and diverse among human colonic microbes. Gut Microbes. (2016) 7:235–45. doi: 10.1080/19490976.2016.1182288
50. Windey K, Preter VD, Verbeke K. Relevance of protein fermentation to gut health. Mol Nutr Food Res. (2012) 56:184–96. doi: 10.1002/mnfr.201100542
51. Singh SB, Lin HC. Hydrogen sulfide in physiology and diseases of the digestive tract. Microorganisms. (2015) 3:866–89. doi: 10.3390/microorganisms3040866
52. De Preter V, Machiels K, Joossens M, Arijs I, Matthys C, Vermeire S, et al. Faecal metabolite profiling identifies medium-chain fatty acids as discriminating compounds in IBD. Gut. (2015) 64:447–58. doi: 10.1136/gutjnl-2013-306423
53. Sharabi K, Charar C, Friedman N, Mizrahi I, Zaslaver A, Sznajder JI, et al. The response to high CO2 levels requires the neuropeptide secretion component HID-1 to promote pumping inhibition. PLoS Genet. (2014) 10:e1004529. doi: 10.1371/journal.pgen.1004529
54. Li H, Li HW, Xie PF, Li ZH, Yin YL, Blachier F, et al. Dietary supplementation with fermented Mao-Tai Lees beneficially affects gut microbiota structure and function in pigs. Amb Express. (2019) 9:1–14. doi: 10.1186/s13568-019-0747-z
55. Fernández-Calleja JF, Konstanti P, Swarts H, Bouwman L, Garcia-Campayo V, Billecke N, et al. Non-invasive continuous real-time in vivo analysis of microbial hydrogen production shows adaptation to fermentable carbohydrates in mice. Sci Rep. (2018) 8:1–6. doi: 10.1038/s41598-018-33619-0
Keywords: zymosan, intestinal microbiota, in vitro fermentation, short-chain fatty acids, gas
Citation: Pi X, Yu Z, Yang X, Du Z and Liu W (2022) Effects of Zymosan on Short-Chain Fatty Acid and Gas Production in in vitro Fermentation Models of the Human Intestinal Microbiota. Front. Nutr. 9:921137. doi: 10.3389/fnut.2022.921137
Received: 15 April 2022; Accepted: 06 June 2022;
Published: 04 July 2022.
Edited by:
Qingsen Shang, Ocean University of China, ChinaReviewed by:
Xinzhong Hu, Shaanxi Normal University, ChinaCopyright © 2022 Pi, Yu, Yang, Du and Liu. This is an open-access article distributed under the terms of the Creative Commons Attribution License (CC BY). The use, distribution or reproduction in other forums is permitted, provided the original author(s) and the copyright owner(s) are credited and that the original publication in this journal is cited, in accordance with accepted academic practice. No use, distribution or reproduction is permitted which does not comply with these terms.
*Correspondence: Wei Liu, YmlvbHdlaUBzaW5hLmNvbQ==
Disclaimer: All claims expressed in this article are solely those of the authors and do not necessarily represent those of their affiliated organizations, or those of the publisher, the editors and the reviewers. Any product that may be evaluated in this article or claim that may be made by its manufacturer is not guaranteed or endorsed by the publisher.
Research integrity at Frontiers
Learn more about the work of our research integrity team to safeguard the quality of each article we publish.