- 1Division of Medical Education, Weill Cornell Medicine-Qatar, Education City, Qatar Foundation, Doha, Qatar
- 2Division of Premedical Education, Weill Cornell Medicine-Qatar, Education City, Qatar Foundation, Doha, Qatar
- 3Department of Medicine—Nephrology and Hypertension, Tulane University, School of Medicine, New Orleans, LA, United States
- 4Distributed eLibrary, Weill Cornell Medicine-Qatar, Education City, Qatar Foundation, Doha, Qatar
Background: One in 10 adults suffer from type 2 diabetes (T2D). The role of the gut microbiome, its homeostasis, and dysbiosis has been investigated with success in the pathogenesis as well as treatment of T2D. There is an increasing volume of literature reporting interventions of pro-, pre-, and synbiotics on T2D patients.
Methods: Studies investigating the effect of pro-, pre-, and synbiotics on biomarkers of inflammation and oxidative stress in T2D populations were extracted from databases such as PubMed, Scopus, Web of Science, Embase, and Cochrane from inception to January 2022.
Results: From an initial screening of 5,984 hits, 47 clinical studies were included. Both statistically significant and non-significant results have been compiled, analyzed, and discussed. We have found various promising pro-, pre-, and synbiotic formulations. Of these, multistrain/multispecies probiotics are found to be more effective than monostrain interventions. Additionally, our findings show resistant dextrin to be the most promising prebiotic, followed closely by inulin and oligosaccharides. Finally, we report that synbiotics have shown excellent effect on markers of oxidative stress and antioxidant enzymes. We further discuss the role of metabolites in the resulting effects in biomarkers and ultimately pathogenesis of T2D, bring attention toward the ability of such nutraceuticals to have significant role in COVID-19 therapy, and finally discuss few ongoing clinical trials and prospects.
Conclusion: Current literature of pro-, pre- and synbiotic administration for T2D therapy is promising and shows many significant results with respect to most markers of inflammation and oxidative stress.
Introduction
Type 2 Diabetes (T2D) is considered an ever-growing burden on public welfare, impacting both high- and low-income nations worldwide. Obesogenic lifestyles, environmental changes, genetic predispositions, and aging have been identified as contributing factors to these increasing trends (1). According to the International Diabetes Federation, as of 2021, an estimated 573 million individuals between 20 and 79 years of age were affected by T2D, representing ∼10% of the world’s population; this figure is expected to cross 643 million by 2030 (2). T2D was responsible for more than 6.7 million deaths in the same year, making it one of the top 10 leading causes of death globally, costing almost 1 trillion USD in health expenditure. In the United States, approximately 21 million adults have been diagnosed with T2D, constituting 8.6% of the adult population (3). Generally, males have a slightly higher prevalence of T2D when compared to females, although this difference is insignificant (3). The onset of new diagnoses increases with increasing age, peaking at around the ages of 55–59 (3).
Type 2 Diabetes and Coronavirus Disease 2019
The high prevalence of T2D explains its significant role as commonly present comorbidity in patients of the severe acute respiratory syndrome coronavirus–2 (SARS-CoV-2) induced coronavirus disease (COVID-19) that has plagued the globe for two years and counting (4–8). Diabetics are not only increasingly susceptible to contracting the infection, but also have vastly higher mortality associated with the comorbidity, with rates ranging from 10.5% (China) to as high as 33.8% (NYC) and 35.5% (Italy) (9–11). This can be attributed to the compromised nature of the immune system in individuals with type 2 diabetes, where delayed and less effective immune reactions likely lead to longer recovery periods due to higher viral loads (12). The virus is also presumed to thrive in glucose-rich serum in conditions such as those in diabetic patients, since glycolysis induces viral proliferation due to the production of reactive oxygen species (ROS) in the mitochondria and the stimulation of hypoxia-inducible factor 1α (13). Diabetic patients who are infected with COVID-19 are more susceptible to uncontrolled inflammatory responses, thrombophilia, and morbidity (14, 15), although the most commonly presenting symptoms include diarrhea (up to 50%), nausea, vomiting and abdominal pain (16). Researchers elsewhere have recommended modulation of individual diet as a significant factor that should be considered during treatment of the disease given the strict relationship between diet and gut microbiota, and the latter to disease severity (12). It must be also noted, however, that a more pronounced and large-scale effect of the pandemic is the likely reduction of physical activity and healthy diet consumption with respect to not only the general population, but also diabetics, as a result of the lockdown that lags behind the shadow of the virus-mediated effects itself (17).
Microbiome Therapy in Type 2 Diabetes
The etiology of T2D is complex and is associated with both non-modifiable risk factors such as age, genetic predisposition, race, ethnicity, as well as modifiable factors such as diet, physical activity, apnea and the use of tobacco (18–20). Of these various factors, having poor dietary habits and following a sedentary lifestyle are the two major influences behind the rapidly rising incidence of the diabetes epidemic; corrective measures in one’s lifestyle, including sleep, and diet can help to reduce the risk of onset and prevent or delay the progression of T2D (21–24). Experimental and clinical trials have shown that changes in gut microbiome composition can contribute to T2D pathogenesis; in this regard, pre- and probiotics have closely investigated for the potential to influence the microbiota and thereby promote anti-diabetic activity in a therapeutic manner (25). Probiotics refer to bioactive agents, naturally found in many foods, that administered in adequate amounts, whereas prebiotics refer to substrates utilized by these bioactive agents within the host to grow (26, 27). When provided in combination, they are referred to as synbiotics, and all three are traditionally aimed at improving the quality and quantity of the gastrointestinal microbiome of the host, leading to health benefits. Additionally, a novel category of biologically active substances known as postbiotics, defined as “probiotic-derived products obtained from food-grade microorganisms that confer health benefits when administered in adequate amounts,” have also found to be promising in not only maintaining homeostasis of normal human health, but also for therapeutic purposes in diabetes mellitus (28). The concept of using natural dietary biomolecules, such as non-digestible fibers, flavonoids and other polyphenols, to serve as solutions to clinical challenges is not new, and their promise has been investigated extensively and verified across both literature and by legislative bodies of significance (29–34).
The Gut Microbiome and Its Dysbiosis
With an astounding composition of 1011–12 bacteria per gram in the large intestine, the gut microbiota is an active influence on the adiposity and fat storage capacity of the human body (25). In addition, the gut microbiota regulates the intestinal barrier along with the sensory, immune, neurological, and enteroendocrine systems (35–37). A dysbiosis in the microbiota alters the abundance of species and produces molecules like short chain fatty acids (SCFAs) and lipopolysaccharides (LPS) that affect these systems (26, 37–40). SCFAs, produced by the microbiota during the decomposition of indigestible polysaccharides, improve glucose tolerance by suppressing fat accumulation through binding a G-protein coupled receptor (GPRC) and ultimately increases insulin-sensitivity (38). It has been found that patients with T2D have lower levels of fecal SCFAs than control groups, which can be increased through a daily supplement of inulin-type fructans (41). Additionally, LPS has a high pro-inflammatory potential and drives endotoxemia low-grade inflammation which is suggested to be a potential cause for insulin resistance (38, 39). T2D patients are known to have an altered microbiota (42). Normally, species of the Bacteroidetes and the Firmicutes phyla are the dominant groups of bacteria in the human gut. However, it was found that microbiota from the phylum Firmicutes and class Clostridia are significantly decreased in people with T2D (43). Moreover, the ratios of Bacteroidetes to Firmicutes, and the ratios of Bacteroides-Prevotella group to Clostridium coccoides-Eubacterium rectale group correlated positively with plasma glucose concentration (43). Decreased proportions of butyrate-producing bacteria and increased proportions of the previously Lactobacillus genus species has also been reported (39). Some of the differences observed between the typical microbiota and a T2D-deceased one supports the low-grade inflammation theory suggesting that increased proteobacteria-derived LPS and flagella can induce inflammation (43). A recent review concludes that gut microbiota dysbiosis that promotes inflammation is a general feature of T2D, but a specific signature for diabetes vs. other diseases in terms of biomarkers is not found (39). The review also suggests that the low decrease in α-diversity in T2D patients may explain the low-grade inflammation (39). Gut microbiota modulation in disease and due to biotics involves a multimodal approach; there is competition between various species facilitated by factors such as luminal pH, limited sources, bacterial toxins and SCFAs, in addition to modulation of gut barrier function and promotion of mucus secretion. Moreover, probiotics have been shown to be involved in the differentiation of regulatory T-cells, cytokine modulation and are also important factors in the communication through gut-brain axis level (44).
Inflammation and Oxidative Stress in Type 2 Diabetes
Chronic activation of the innate immunity, often characterized by cytokine-induced acute inflammation (also called low-grade-inflammation) has been shown to be associated with T2D pathogenesis, in addition to other linked metabolic complications (45). Inflammation has also repeatedly been linked to diabetes pathophysiology through its mediated effects on endothelial and cardiovascular pathology. Characterized by recruitment of leukocytes and their cytokine release, as well as various endothelial and tissue-specific crosstalk, inflammation has shown to play an acute role in metabolic cardiomyopathy (46). Hence, it is not surprising that the elevated presence of pro-inflammatory C-reactive protein (CRP), tumor necrosis factor-α (TNF-α) and interleukin-6 (IL-6) and the reduction of anti-inflammatory cytokines such as IL-4 and IL-10 in the blood are predictive markers of T2D development and pathogenesis (45). Obesity and increased body weight, have been hypothesized to mediate the increased expression of inflammatory markers, leading to downregulation of the intracellular, downstream physiologic effects of insulin, and hence development of further insulin resistance (47). Increased oxidative stress arising from hyperglycemia also impairs glucose uptake in both muscle and fat cells, in addition to decreasing pancreatic β-cell mediated insulin secretion, further contributing to development of diabetes, and serving as an important marker for investigation of therapeutic intervention (48, 49). Whether as a cause or effect, dysbiosis of gut microbiome has been closely associated with T2DM presentation. Such an association could stem from the effect of microbiome dysbiosis on the levels of certain inflammatory markers such as CRP and TFN-α, further resulting in chronic low-grade inflammation following an atypical immune response (50, 51).
Pro-Inflammatory Markers in Type 2 Diabetes
Pro-inflammatory markers, such as acute-phase proteins, pro-inflammatory cytokines, and chemokines, are elevated in diabetic and obese patients, and are generally found to be at lower levels in individuals with a healthier lifestyle (52). Generally, there is a positive correlation between the levels of pro-inflammatory markers and insulin resistance (53). Several prospective studies have determined that these inflammatory markers may also be used to predict the onset of T2D (54).
C-reactive protein (CRP) is a plasma protein that serves as an early biomarker of inflammation, infection, or trauma (55). CRP is released through IL-6 induced hepatic synthesis and is the main mediator of the acute-phase response. Elevated levels of CRP has a significant association with the risk and onset of Type 2 diabetes, as well as insulin resistance syndrome (56). High concentrations of CRP in patients with T2D may be because of the stimulation of cells in the innate immune system due to increased amounts of nutrients (57). High-sensitivity CRP tests measure lower levels of the protein and is also positively correlated with the risk of type 2 diabetes (58).
Interleukin-6 (IL-6) secreted largely by Th cells, macrophages, fibroblasts, is a multifunctional cytokine involved in inflammatory responses as well as the regulation of cell growth and proliferation, activation, and differentiation of genes. IL-6 induces the production of several leukocytes and proteins such as CRP, and has been found to have parallel associations with T2D (56). The abnormal synthesis of IL-6 causes inflammation and instigates the formation of suppressor of cytokine signaling-3 (SOCS-3), which may serve as an inhibitor of insulin trans-signaling pathways (59). Thus, elevated amounts of this cytokine may serve as a predictor of T2D. Moreover, studies have also shown that IL-6 plays a role in anti-inflammatory processes and glucose metabolism (60).
Another pro-inflammatory cytokine that participates in the regulation of the body’s inflammatory response is Interleukin-1β (IL-1β). IL-β has been associated with several autoimmune diseases, such as Type 1 Diabetes, as well as metabolic syndromes, including cardiovascular diseases and T2D. These disorders are characterized by elevation in IL-1β levels, which lead to weakened secretion of insulin in the pancreas’s B cells (61). IL-1β also causes the breakdown of insulin receptor substrate (IRS) proteins by stimulating suppressors of cytokine signaling (SOCS) (62).
Interferon-gamma is also a pro-inflammatory cytokine that is involved in the preparation of macrophages for stimulation and start of an inflammatory response (63). IFN-γ is expressed by activated T cells and natural killer (NK) cells. In obese patients with T2D, an elevation in IFN-γ can be detected (64). This cytokine has a key role in the maintenance of inflammatory responses in adipose tissues in T2D (65).
Tumor necrosis factor alpha (TNF-α) is a homotrimer that acts as a proinflammatory cytokine and is synthesized by the activation of natural killer (NK) cells, T lymphocytes, and macrophages. It plays critical roles in infection control, bone remodeling, and insulin resistance (66). Obesity and T2D have been associated with increased levels of TNF-α as TNF-alpha instigates insulin resistance in adipose and peripheral tissues through the phosphorylation of serine (67). Furthermore, TNF-α induces low-grade chronic inflammation through the production of ROS and stimulation of several pathways mediated by transcription (68).
Interleukin 17 (IL-17) is a pro-inflammatory T helper (Th) 17 cytokine. IL-17 induces inflammation by binding to a family of IL-17 receptors, which then initiate signaling that activates nuclear factor-Kb (69). This leads to the production of proinflammatory cytokines by monocytes, fibroblasts, and epithelial and endothelial cells (70). IL-17 also moves and employs granulocytes. Insulin resistance and inflammation in diabetes mellitus are linked to the expansion of both Th17 and Th1 (71). Patients with poor blood sugar regulation have elevated serum levels of Th17 cytokines such as IL-17 when compared with individuals with healthy glucose regulation (72).
Interleukin-8 is another proinflammatory chemokine secreted by cells such as adipocytes, macrophages, and endothelial and epithelial cells (73). It is a multifunctional interleukin involved in local and systemic inflammation and macrophage infiltration, and has also been implicated in the pathogenesis of T2D. Increased circulating levels of IL-8 has been reported in patients with T2D (74).
Interleukin-12 (IL-12) is a proinflammatory cytokine that is produced by antigen presenting cells from the innate immune system when in the presence of pathogen-associated molecular patterns (PAMPs) and danger-associated molecular patterns (DAMPs) (75). IL-12 is important for the programming of T cells into Th1 cells, thereby inducing an immune response (76). IL-12, like the other inflammatory cytokines, has been implicated in the pathogenesis of type 2 diabetes and insulin resistance. Levels of IL-12 were found to be the highest in T2D patients (77).
Lipopolysaccharide-binding protein (LBP) is a plasma protein that facilitates the interaction of lipopolysaccharides with other receptors, including toll-like receptor-4 (TLR4) (59). TLR4 activation initiates an intracellular signaling pathway leading to the production of inflammatory cytokines and the activation of the innate immune system (78). Higher concentrations of plasma LPS have been detected in individuals affected by T2D (79).
Anti-inflammatory Markers in Type 2 Diabetes
Anti-inflammatory markers, such as IL-10, are generally detectable in lower quantities in diabetic and obese patients when compared to controls, and these decreased levels could play a key role in the development of insulin resistance as well as other chronic diseases (80).
Interleukin-10 (IL-10), an anti-inflammatory cytokine, is considered to have a protective function in T2D as it prevents inflammation of immune cells (81). IL-10 is best known for its inhibitory effect on macrophage stimulation (82). Elevated glucose levels in diabetic patients have been found to minimize IL10-mediated activation of STAT3, a signaling protein of IL-10 (81). Thus high serum glucose and HbA1c are associated with low capacity of IL10 production (83).
Oxidative Stress Markers in Type 2 Diabetes
Oxidative stress is caused by an imbalance of free radicals and antioxidant defenses, and is suggested to have a potential impact on the pathogenesis of diabetes and the development of its complications (84). Values of oxidative stress markers are measured to be mostly higher in diabetic vs. non-diabetic subjects (85).
Superoxide dismutase (SOD) is an oxidative stress-related parameters linked closely to Type 2 diabetes (86). SOD catalyzes the breakdown of reactive oxygen species (ROS) in all tissues and cells (87). Oxidative stress and synthesis of ROS have been associated with the development and complications of diseases such as diabetes mellitus (88). The downregulation of SOD may be associated with the pathogenesis of diabetes mellitus (89). In diabetic patients that have an imbalance of oxidants to antioxidants, there are higher levels of ROS, which causes the total activity of SOD to be significantly higher than the antioxidant’s activity in non-diabetic patients (90). However, the activity of SOD is also linked to the duration of the disease, with the level of antioxidant enzyme decreasing as the years of disease increase (91).
Another antioxidant enzyme is catalase (CAT), found in peroxisomes and the cytosol that mainly functions in the catalysis and disposal of hydrogen peroxide (H2O2) into O2 and H2O in erythrocytes (92). Catalase has significantly higher activities in patients T2D when compared with controls (93). Recent data suggests that the onset of diabetes in catalase-deficient patients happens more than 10 years earlier than subjects with normal levels of catalase (94).
Oxidative stress in diabetic patients leads to more severe diabetic complications. Lipid peroxidation results from the interaction of the lipid bilayer of a cell membrane with ROS and oxygen derived free radicals, producing malondialdehyde (MDA) (95, 96). Elevated levels of MDA lead in subjects with T2D to many physiological effects, such as influencing the structural integrity of the cell membrane, inactivating surface enzymes and receptors on the membrane, and causing errors in cell regulation (97).
Nitric oxide (NO) is a reactive nitrogen species (RNS) implicated in the pathogenesis of diabetes and complications. Nitric oxide is involved in impaired cellular function and increased expression of nitric oxide synthase (98). Since nitric oxide has a relatively short half-life, its metabolite nitrite, and nitrate are usually measured in blood and urine, and later used to calculate NO production. Diabetic patients have significantly higher basal levels of NO than non-diabetic individuals across multiple individual studies (99–101), as well as a meta-analysis (102), and were found to have hypertension and microvascular complications. Interestingly, one study based in India showed a lower mean plasma NO level in diabetics compared to control group (103).
Glutathione is the most abundant, low molecular weight non-protein antioxidant produced by cells (104). It exists in the thiol-reduced (GSH) and the disulfide-oxidized (GSSG) forms (105). GSH has a key function in preserving redox homeostasis, transport of amino acids, preventing damage of tissue, and serving as a coenzyme for several reactions (106). As an antioxidant, GSH plays a major role in GSH peroxidase (GPx)—catalyzed reduction of H2O2, which can in turn be reduced into GSH again by GSH reductase (GR). Because of its function and omnipresence, GSH can also be employed as a biomarker of oxidative stress. More specifically, it has been found that diabetic patients have reduced GSH/GSSG ratios, causing inflammation, hyperlipidemia, and antioxidant imbalance (107, 108).
Oxidative stress exhibits itself in elevated ROS synthesis and oxidation of circulating low-density lipoprotein molecules. Oxidized low-density lipoprotein (oxLDL) activates the immune system and promotes inflammation by inducing dendritic cell maturation and T cell activation (109). The levels of oxLDL were found to be higher in patients with T2D and obesity-related traits relative to controls (110). Other studies have shown that oxLDL levels can predict the onset of the metabolic syndrome (111).
Damage due to oxidative stress can be measured by the amounts of primary or secondary products of peroxidation. One of the secondary byproducts of this reaction are F2-isoprostanes (F2-IsoP) (112). F2-isoprostanes are a group of prostaglandins (PG). F2-like products and are involved in many diseases including T2D. Diabetic patients have higher levels of F2-IsoP than controls and can be used as a standard biomarker of oxidative stress in patients with T2D (113).
8-hydroxy-2’-deoxyguanosine (8-OHdG) is a modified guanine that is considered a sensitive indicator of oxidative DNA damage (114). A total of 8-OHdG can be used to measure the extent of oxidative stress in the human body as it relates to DNA oxidation ratio and repair of DNA (115, 116). The levels of urine 8-OHdG increases in diabetic patients, and the amount of 8-OHdG generally correlated with the severity of complications such as diabetic nephropathy (117).
Total antioxidant capacity (TAC) is a cumulative measure of small molecule antioxidants and proteins, or the amounts of small molecules alone (118). TAC is often employed to assess a biological sample’s total antioxidant status (TAS). Since the synergistic impact of antioxidants is known to serve greater defense against free radicals than any antioxidant by itself, evaluating overall capacity can provide more insight to the body’s collective mechanisms (119). A negative correlation was observed between HbA1c and plasma TAC and TAS among middle-aged diabetic patients in comparison to healthy controls of similar age (120, 121).
Various factors govern the potential development of T2D interventions using microorganisms to permeate gut microbiota in the host with the aim of targeting biomarkers of inflammation and oxidative stress. Therefore, there is a need in connecting and reporting of the complex data in the literature with aim of distilling T2D interventions using biotics as an effective treatment. Table 1 provides the reference values of each of the above listed biomarkers of inflammation and oxidative stress as seen in controls and those with T2D. 2.
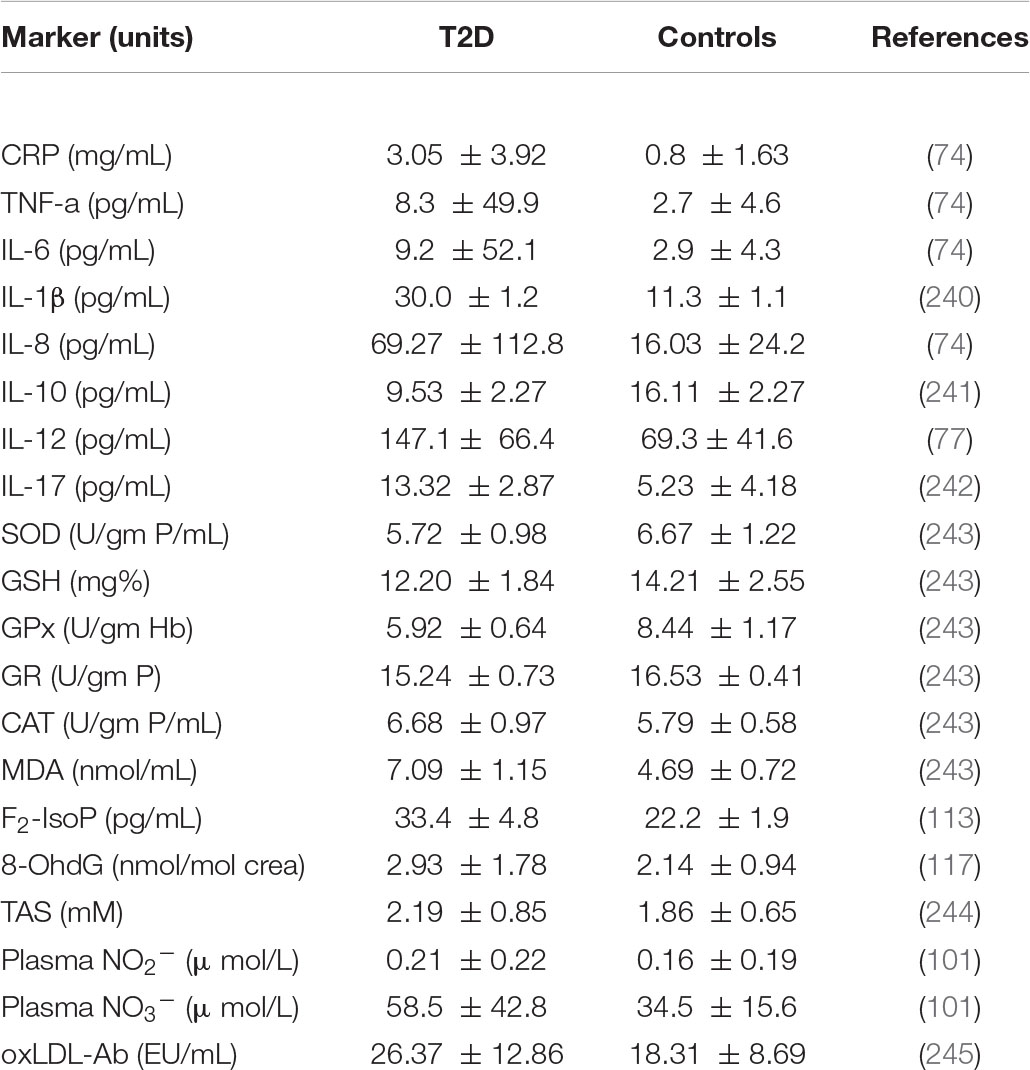
Table 1. Reference levels of markers of inflammation and oxidative stress in patients with type 2 diabetes and controls.
Methods
Literature Sources and Searches
We followed the Preferred Reporting Items for Systematic Reviews and Meta-Analyses (PRISMA) protocol (122). Searches were conducted in PubMed, Scopus, Web of Science, Embase, and Cochrane. We also searched for gray literature through ClinicalTrials.org and ProQuest Dissertations and Theses. The initial search took place in June 2020 and we reran a final search in January 2022 to gather any new records that might have been produced or published.
The search strategy in PubMed included the following elements:
(“Probiotics”[MeSH Terms] OR “probiotics”[Title/Abstract] OR “probiotic”[Title/Abstract] OR “Prebiotics”[MeSH Terms] OR “prebiotic”[Title/Abstract] OR “prebiotics”[Title/Abstract] OR “Synbiotics”[MeSH Terms] OR “synbiotics”[Title/Abstract] OR “synbiotic”[Title/Abstract] OR “symbiotic”[Title/Abstract] OR “symbiotics”[Title/Abstract] OR “gastrointestinal microbiome”[MeSH Terms] OR “gut microbiome”[Title/Abstract] OR “gut flora”[Title/Abstract]) AND (“diabetes mellitus, type 2”[MeSH Terms] OR “T2D”[Title/Abstract] OR “type 2 diabetes”[Title/Abstract]).
Inclusions
Studies had to be clinical trials and randomized studies relevant to the effect of diet using probiotics, prebiotics, and symbiotics on T2D to Prebiotics, Probiotics, and Synbiotics and T2D. Studies of adults of any age, sex, ethnicity, from any region worldwide, and published at any time were included. Further, only those studies reporting on markers of inflammation and/or oxidative stress were included among the final analysis. Reports available from inception of respective databases to accessed dates (final search: January 2022) were included. Covidence was utilized for importing and screening titles, abstracts and full-texts; extraction was performed using MS Excel.
Exclusions
The paper excluded studies on other type of diabetes, and any reviews, conferences, abstracts and proceedings, editorial and non-clinical papers, as well as animal studies. Studies in other language than English were excluded too. Studies not reporting on markers of inflammation and/or oxidative stress were excluded among the final analysis.
After removing duplicates, the authors independently scanned the title and abstract of all articles referring to the inclusion and exclusion criteria. The same process was also used for full-text screening and any conflicts were resolved by consensus. The included articles were processed for qualitative analysis and the relevant information were grouped by themes and expanded through the discussion.
Results
Review Process for Data Extraction
According to the PRISMA flowchart (see Figure 1), a total of 5,985 studies were imported from all databases, from which 3,250 duplicates were excluded, while 2,377 articles were found irrelevant through title and abstract screening and 20 others could not be retrieved. Therefore, a total of 338 articles were assessed for eligibility out of which only 47 were included in the final review. The reasons for excluding 311 full-text articles from the study can be summarized as follows: 68 studies did not have the adequate study protocol; 52 studies were non-clinical; 56 studies were not relevant; 35 did not report on the levels of inflammation or oxidative stress; 24 studies were systematic reviews and meta-analysis; 20 studies were not available in full-text; 19 studies had incorrect intervention; 17 studies were reviews, abstract, or proceedings; 17 studies were duplicates; and 3 studies were not in English language.
Effect of Probiotics on Inflammatory and Oxidative Stress Markers in Type 2 Diabetes
Pro-inflammatory Markers in Type 2 Diabetes
Effect on C-Reactive Protein and High-Sensitivity C-Reactive Protein
Sixteen studies have investigated and reported the effect of probiotics on either CRP or High-Sensitivity C-Reactive Protein (hs-CRP). While not all studies have reported significant (p ≤ 0.05) effects, most have shown that administration of probiotics leads to a general decreasing trend in these markers of inflammation (Table 2).
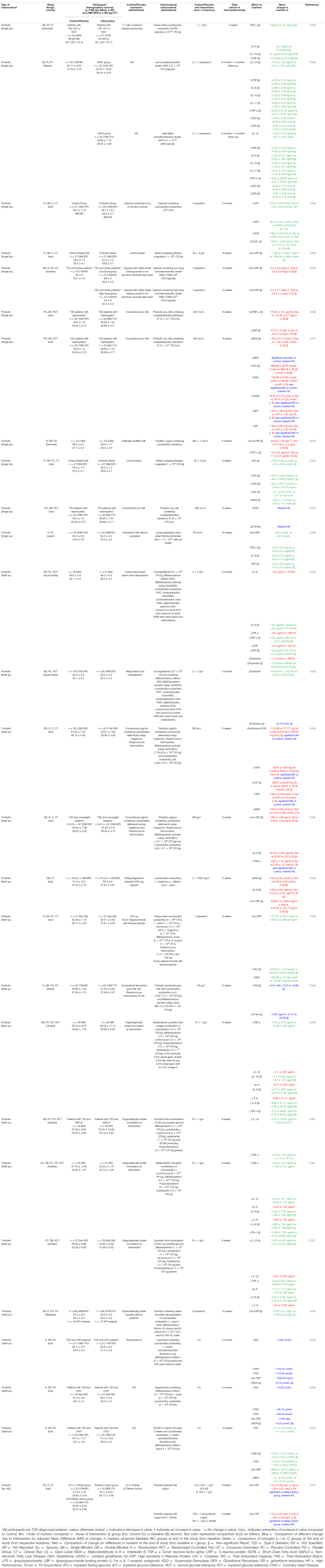
Table 2. Studies investigating the effect of probiotics on markers of inflammation and oxidative stress in T2D.
Sabico et al. (123) reported a significant decrease of 2.9 mg/L (-52.7%) in CRP following supplementation of a multi-species probiotic in a cohort of 31 T2D patients when compared to baseline values; however, these results were not found to be significant compared to control despite an increase of 0.4 mg/L (+ 13.3%) in CRP markers in this group. Another group of researchers from Iran reported a significant decrease of 0.777 ± 0.441 mg/L of hs-CRP following an 8-week intervention of probiotics compared to a control group (124). Similar significant results have been reported following 12-week multi-species probiotic supplementation in three cohorts of T2D patients with coronary heart disease (CHD), with adjusted mean differences of –1.04, –0.88, and –0.95 mg/L in hs-CRP compared to control (125–127). Three other studies investigating the effects of multi-species produced insignificant results, with two reporting slight increases and another reporting a decrease in hs-CRP compared to baseline values or that of control (128–130). Of interest, Bayat et al. (131) have shown that an 8-week probiotic of an unknown number of species administration with and without Cucurbita ficifolia resulted in significant reductions in serum hs-CRP compared to baseline, control, and another receiving only C. ficifolia.
The results of single-species probiotic supplementation on CRP and hs-CRP appear to be non-conclusive, with independent studies reporting largely non-significant (p > 0.05) mean increase and decrease in these markers in the intervention groups compared to control or baseline. Andreasen et al. (132) and Feizollahzadeh et al. (133) have reported mean decreases of 0.3 and 0.2 mg/L in CRP compared to baseline following supplementation with soymilk containing Lactiplantibacillus plantarum (previously Lactobacillus plantarum) and freeze-dried Lactobacillus acidophilus in T2D-associated nephropathic patients and a mixed group of diabetic or those with impaired or normal glucose tolerance, respectively. Hsieh et al. (134) have reported that consuming live and heat-killed Limosilactobacillus reuteri (previously Lactobacillus reuteri) resulted in an increase of 0.3 ± 1.9 and 0.5 ± 2.4 mg/L from baseline(s), respectively, in two cohorts of T2D patients without any specified/reported comorbidities; although, since these results were all non-significant, no solid conclusion can be made regarding the effect of the difference in probiotic species administered or the presence of diagnosed comorbidities. Similar conflicting and non-significant results have been shown for changes in hs-CRP following single-probiotic supplementation, while one study by Sato et al. (135) have shown that Shirota-fermented milk with Lacticaseibacillus casei (previously Lactobacillus casei) significantly increased detectable hs-CRP levels by 92.0 mg/dL compared to an increase of 32.5 mg/dL in the control group over a period of 16 weeks.
Effect on Tumor Necrosis Factor-α
Eleven studies have investigated and reported the effect of probiotics either single or multi-species, on TNF-α. While some studies have reported non-significant (p > 0.05) changes post probiotic supplementation, most trials have successfully concluded that administration of probiotics lead to significant overall decreases in levels of this pro-inflammatory marker (Table 2).
Sabico et al. (123) reported that a multi-species probiotic supplementation led to a 0.6 pg/mL or 66.7% decrease from baseline in a cohort of diabetic patients from Saudi Arabia. Similarly, another group reported a near 33% reduction in the marker from baseline, a change that was also found to be significant compared to control (129). Through a series of four studies, Kobyliak et al. (136–139) have reported significant changes of –5.81 ± 9.13, –7.95 ± 1.27, –5.02 ± 9.33, and –6.75 ± 7.73 pg/mL in TNF- α from baseline compared to control following multi-species probiotic supplementation.
On the other hand, there is no consensual trend among studies investigating the effect of single-species probiotics due to the lack of statistically significant observations. However, interestingly, L. reuteri supplementation produced a directionally different change depending on whether the bacteria was provided in heat-killed or live form (134). Other studies have reported little to no change in TNF-α following single-species probiotic administration (132, 133, 135).
Effect on Interleukin-1β
Information for the effect of single or multi species probiotics on IL-1β is available from five clinical studies, each reporting significant results of decrease in marker compared to either baseline or control in the intervention groups (Table 2).
Hsieh et al. (134) have demonstrated that supplementation with heat-killed L. reuteri over a course of 6 months lead to a significant decrease of 1.43 ± 2.70 pg/mL in IL-1β compared to the change in control group over the same period. Interestingly, supplementation of the live form of the same bacteria in a lower dose resulted in a 50% reduction in this change from baseline and was not significant compared to control in the same study.
The effect of multi-species probiotic supplementation has been more consistently reproduced across multiple studies by Kobyliak et al. (136–139). Based on multispecies probiotic “Symbiter Forte” supplementation across four cohorts of diabetics, the group has reported significant mean differences of –4.1 ± 8.36, –4.91 ± 8.23, –6.74 ± 15.59, and –5.44 ± 1.51 pg/mL in serum IL-1β from baseline, with the latter two results also reported to be statistically significant compared to effects of placebos with organoleptically similar formulation as the probiotic media.
Effect on Interleukin-6
Probiotic supplementation has been investigated among diabetics in a total of nine studies, including both single and multi-species probiotics; results were found to be inconclusive with reporting of both positive and negative, significant, and insignificant findings, across multiple studies (Table 2).
Across three single-species probiotic administering studies, organisms of the (previously) Lactobacillus genus have been associated with slight, statistically non-significant increases in IL-6. These range from average changes of + 0.1 and + 0.4 pg/mL levels following 1 and 4 months of intervention, respectively, to as high as + 0.95 and + 1.55 pg/mL following 6 months of supplementation and 3 months of follow-up (132, 134, 135).
On the other, hand, the six studies investigating the change in IL-6 following multi-species probiotics have all reported mean reductions in average IL-6 levels from baseline. Three of these presented significant changes from baseline; Sabico et al. (123) reported a difference of –3.9 pg/mL (-76.5%), while Kobyliak et al. (136, 138) reported changes of 3.45 ± 1.48 and –6.77 ± 9.62 pg/mL across two studies. Another study by Kobyliak et al. (137) revealed statistically significant changes of –4.11 ± 7.15 pg/mL from baseline compared to control. Other studies have also reported decreasing averages in IL-6 levels post supplementation, however, these changes were not found to be statistically significant (128, 129).
Effect on IFN-γ
Two studies by Kobliyak et al. (136, 137) have investigated and reported the effect of multi-species probiotics on IFN-γ; no studies have investigated the effects of single species probiotic supplementation on this pro-inflammatory marker (Table 2). These two “Symbiter Forte” regimens were associated with net decreases in IFN- γ, although statistically non-significant.
Effect on IL-8
Three multispecies probiotic administering probiotic combinations have been associated with significant efficacies following an 8-week intervention (Table 2). Kobliyak et al. (136–138) have reported reductions in IL-8 levels ranging from –3.80 ± 1.05 to –8.99 ± 21.11 pg/mL, although it must be noted that all three interventions were not statistically significant from the effect seen in control.
Effect on Interleukin 17
Only Hsieh et al. (134) have reported on the association of IL-17 levels with probiotic administration (Table 2). However, the authors report statistically insignificant effects; changes of + 0.66 ± 3.24 and + 0.47 ± 2.91 ng/mL following live and heat-killed probiotic use.
Effect on Endotoxin
Two studies by Sabico et al. (123, 140) have investigated the effects of multi-species probiotics on blood endotoxin levels in diabetic patients (Table 2). While one study reported that following probiotic supplementation, endotoxin levels significantly dropped by 3.2 IU/mL (-69.6%) from baseline, another reported a significant change of –2.40 IU/mL (-52.2%) compared to the change seen in control.
Effect on Lipopolysaccharide-Binding Protein
The one study reporting on the effect of probiotic on LBP concluded that there was no change in the mean levels of marker following a 16-week intervention with Lacticaseibacillus casei (135).
Anti-inflammatory Markers in Type 2 Diabetes
Effect on Interleukin-10
A study by Hsieh et al. (134) investigated the effect of a 6-month single species probiotic course on diabetic patients; although there was a generally positive trend in the change post-intervention compared to baseline and control (+ 1.48 ± 3.09 and + 2.05 ± 3.25 ng/mL in the live and heat-killed bacteria groups, respectively), these results were not statistically significant.
Effect on IL-1RA
Andreasen et al. (132) have reported an insignificant increase of + 2 pg/mL in IL-1RA from baseline following a 4-week regimen of single-species probiotic; however, this change was much more pronounced in control.
Markers of Oxidative Stress in Type 2 Diabetes
Effect on Malondialdehyde
Malondialdehyde (MDA) is one of the final products of polyunsaturated fatty acids peroxidation in the cells. An increase in free radicals causes overproduction of MDA. MDA has been extensively studied as a biomarker of inflammation in the context of metabolic disease. A total of seven studies investigated this inflammatory marker as a subject of change following supplementation with either single (2) or multispecies (5) probiotics (Table 2).
Miraghajani et al. (141) reported a beneficial 0.07 μmol/L change in mean MDA levels among a cohort of T2D patients with nephropathy after an 8-week course of single-species probiotics that was significantly different than the change observed among controls receiving conventional soy milk. On the other hand, another group reported an non-significant increase of 0.06 μmol/L following a course of another single-species probiotic in bread (142).
Among five multi-species probiotics investigated for their effects on MDA levels among diabetics, two have reported significant reductions in MDA levels, while three have presented statistically insignificant results. Ejtahed et al. (143) have reported a significant decrease of 0.26 μmol/L in the mean level of MDA from baseline following a 6-week course, while a more recent study by Raygan et al. (127) have presented a significant change of –0.1 ± 0.3 μmol/L from baseline compared to control following a twice-longer course. Mazloom et al. (128) and Raygan et al. (126) both reported net reductions in mean level of MDA markers; however, these were not found to be statistically significant.
Effect on Superoxide Dismutase
SOD levels post probiotic administration has been a subject of investigation under four studies included in this review (Table 2). All studies have reported positive trends, some have been reported as significant versus control. Mirmiranpour et al. (144) have reported slightly higher SOD levels post-intervention than control after the authors report that no significant change existed between the groups at baseline. Hariri et al. (145) have also presented the significant effects of another single-species probiotic on diabetic nephropathy patients following an 8-week course. In another study, the same authors reported changes of + 0.93 ± 0.95 and + 0.04 ± 1.31 U/mL after administration of live and heat-killed bacterium, however, these were not found to be statistically significant (134). Lastly, Ejtahed et al. (143) reported a significant increase of 137.87 U/g Hb in erythrocyte SOD from baseline following a 6-week course of a multi-species probiotic yogurt.
Effect on Catalase
A few studies have reported on the effect of nutraceuticals on CAT levels (Table 2). While one study reported a significant result, an overall conclusion cannot be made in this regard as two others have found no statistically significant changes nor similar trends. Mirmiranpour et al. (144) have reported that after starting from similar baseline values, the group receiving 3 months of a single-species probiotic capsule (2.44 ± 0.50 U/mL) had markedly increased CAT levels compared to control (1.95 ± 0.34 U/mL), a difference in mean of 0.49 U/mL that was statistically significant. While a slight positive change from baseline was also found by Bahmani et al. (142) this was not significantly different from the change observed in control; on the other hand, Ejtahed et al. (143) found an overall insignificant decrease in mean CAT.
Effect on GSH Peroxidase and GSH Reductase
Multiple studies have investigated the effect of probiotics on GPX activity levels post-intervention, however, the results have been conflicting (Table 2). Ejtahed et al. (143) reported that a 6-week regimen of multi-species probiotic was associated with a significant increase in mean GPX activity to hemoglobin ratio of 0.78 U/g Hb compared to both baseline and control. Similar significant increases (of + 0.43 U/g Hb in GPX and + 0.38 U/g Hb in GR) have been reported by Miraghajani et al. (141) following an 8-week administration of a single-species probiotic. More recently, Mirmiranpour et al. (144) have reported that after starting from similar levels at baseline, the difference between an intervention group receiving single-species probiotic and control was + 7.26 U/mL in GPX activity. Interestingly, another group of researchers have shown fractional and statistically insignificant changes in GPX activity after single-species probiotic activity; however, these changes were smaller in magnitude than that seen in control (134).
Effect on GSH and GSSG
The effect of probiotics on reduced (GSH) and oxidized (GSSG) glutathione levels have been studied across multiple studies with interstudy consenting trends (Table 2). All studies report a positive change in mean plasma GSH following intervention periods, although not all have been found to be statistically significant. Miraghajani et al. (141) reported a statistically significant increase from baseline in GSH (732.96 ± 61.95 vs. 600.66 ± 69.61 μmol/L) and a similar decrease in GSSG (19.00 ± 0.70 vs. 30.37 ± 0.20 μmol/L) following an 8-week supplementation period with a single-species probiotic soymilk; these changes were also statistically significant compared to changes observed in a control group. Although Bahmani et al. (142) also investigated another single-species probiotic in this regard, the results were not statistically significant given a larger increase in the control group.
Among multispecies probiotics investigation, Asemi et al. (124) reported a significant increase of + 240.63 ± 101.29 μmol/L from baseline compared to control the earliest. Raygan et al. (125–127) through a series of three, 3-months studies investigating different multi-species probiotics, a change of + 18.0 ± 112.7 μmol/L from baseline in one of the studies was reported, and baseline-adjusted intergroup mean difference of changes of + 45.15 and + 154.16 μmol/L was reported in the two other studies; all three reports were statistically significant.
Effect on Total Antioxidant Capacity and Total Antioxidant Status
The association of probiotic supplementation on the levels of TAC has been extensively studied (Table 2). While many of these reports a significant increase in mean levels of TAC, few others have presented a mean positive change that are not statistically significant.
Miraghajani et al. (141) and Bahmani et al. (142) have reported respective changes of + 39 and + 78.6 mmol/L in mean TAC levels among diabetics following an 8-week supplementation with different single-species probiotics; however, these were not statistically significant. Among the other studies reporting on the effect of multispecies probiotics, only Asemi et al. (124) failed to show a statistically significant change despite a large mean increase of + 379.97 ± 41.8 mmol/L from baseline levels. On the other hand, Raygan et al. (127) have shown that a mean increase of just 12.6 ± 41.6 mmol/L was significant due to the control group having a large decrease of –116.9 ± 324.2 mmol/L. However, through a series of two other multispecies probiotic-investigating studies among diabetics with congestive heart disease, Raygan et al. (125, 126) have reported larger significant baseline-adjusted intergroup mean differences of + 108.44 and + 119.30 mmol/L. Ejtahed et al. (143) and Tonucci et al. (146) have also presented significant decreases in TAS levels compared to baseline and control group, despite a less pronounced change.
Effect on Nitric Oxide
Few studies have investigated changes in NO levels as a measure of oxidative stress following probiotic use (Table 2). Bahmani et al. (142) reported a statistically non-significant rise (+ 18.5 ± 36.2 μmol/L) in mean NO levels following 8 weeks of single-species probiotic bread supplementation. On the other hand, Raygan et al. (125–127) successfully reported, through three different studies each using multispecies probiotics, net increases in mean NO levels in the order of + 7.86 μmol/L and + 4.28 μmol/L (both as intragroup baseline adjusted intergroup mean difference) and + 1.7 ± 4.0 μmol/L (change from baseline).
Effect on Oxidized Low-Density Lipoprotein, 8-Hydroxy-2’-Deoxyguanosine and F2-Isoprostanes
OxLDL, 8-OhdG and F2-IsoP remain some of the lesser used measures of oxidative stress utilized to investigate oxidative stress (Table 2). Mirmiranpour et al. (144) recently reported that compared to a control group (17.07 ± 1.01 mU/L) that did not have significantly different mean OxLDL levels at baseline, an intervention of single-species probiotics was associated with a lower mean OxLDL (16.85 ± 1.53 mU/L) at 3 months; however, this was not statistically significant. Similarly, Tonucci et al. (146) reported a decrease in F2-IsoP levels following a course of multispecies probiotics. Hariri et al. (145) described a significant intergroup mean decrease in 8-OhdG, however specific marker levels were not reported.
Pro-inflammatory Markers in Type 2 Diabetes
Effect on C-Reactive Protein and High-Sensitivity C-Reactive Protein
We found six studies investigating the effects of prebiotics on hs-CRP, with most studies reporting significant (p ≤ 0.05) decreases in this marker of inflammation following administration of inulin, resistant dextrin, or resistant starch in T2D patients (Table 3). Dehghan et al. have reported that high performance (HP) inulin supplementation in T2D patients resulted in a significant baseline adjusted mean hs-CRP difference of –3.8 ng/mL compared to a control group consuming maltodextrin, while another recent study has confirmed this significant effect of HP inulin using a cohort of diabetic and overweight patients consuming either HP inulin or HP inulin plus butyrate (147, 148). Moreover, a recent study by Farhangi et al. (149) reported that resistant dextrin supplementation was responsible for a significant baseline-adjusted mean difference of –8.02 ng/mL (-54.00%) compared to a similar control. Resistant dextrin has also been showed to be associated with a significant reduction of –2.40 ng/mL in hs-CRP from baseline in one study by Aliasgharzadeh et al. (47) Administration of oligofructose-enriched inulin and a formula containing 60% resistant starch type 2 were also shown to result in promising baseline-adjusted mean differences of –3.9 ng/mL (-31.70%) and –4.6 ng/mL (-11.9%) compared to their control(s), respectively, although these reductions were not found to be statistically significant (150, 151).
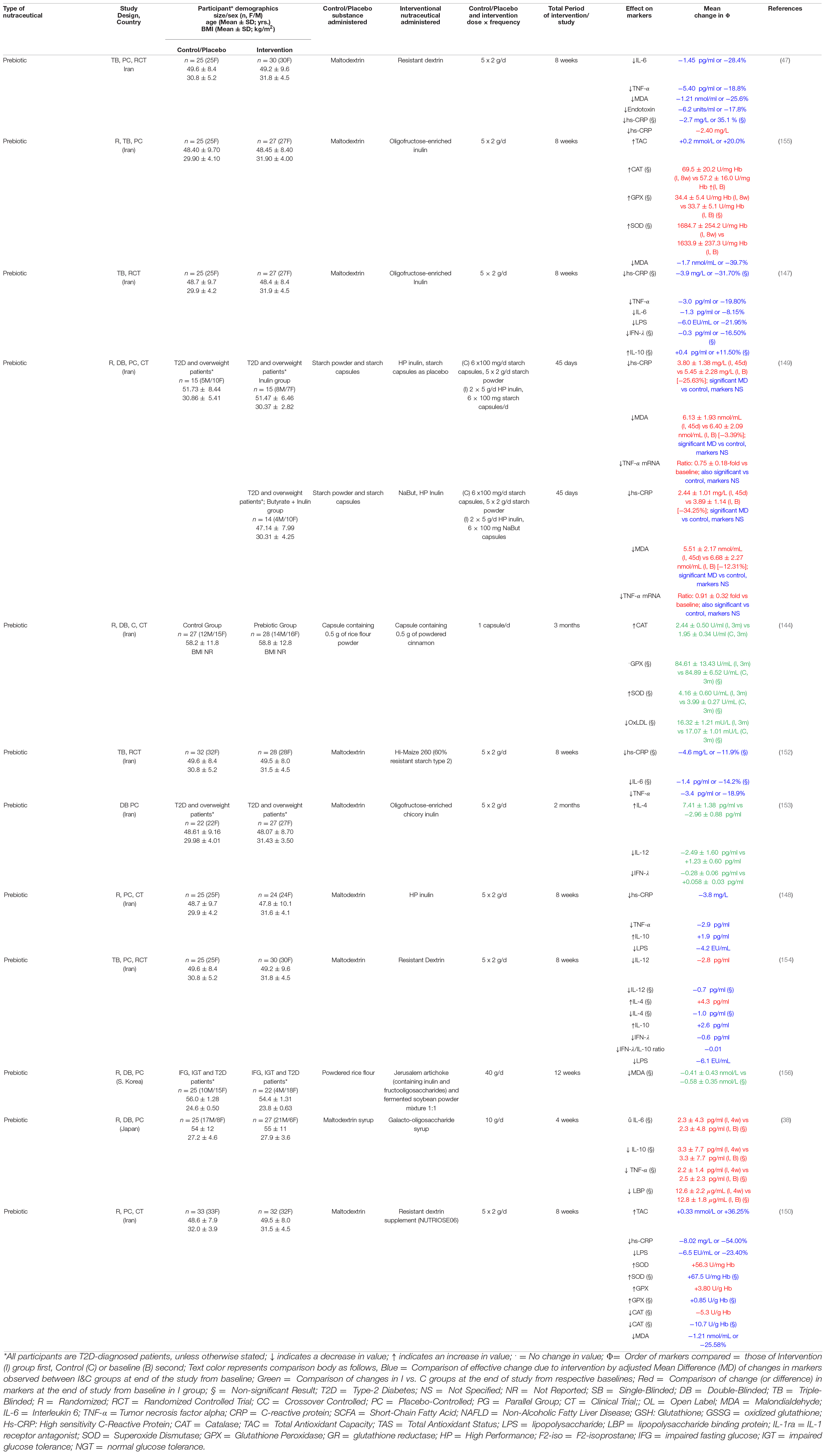
Table 3. Studies investigating the effect of prebiotics on markers of inflammation and oxidative stress in T2D.
Effect on Tumor Necrosis Factor-α
Five out of six studies investigating the effect of prebiotic supplementation on TNF-α have reported promising results, while another has reported a non-significant (p > 0.05) decrease in the levels of this inflammatory marker (Table 3) (38). Aliasgharzadeh et al. (47) have reported a significant baseline-adjusted mean difference of 5.40 pg/mL (-18.8%) between the prebiotic group receiving HP inulin and control. Two other studies have reported similar baseline-adjusted differences of 3.0 pg/mL (-19.80%) and 2.9 pg/mL between changes of prebiotic and control groups following supplementation with oligofructose-enriched and HP-inulin, respectively (147, 150). Similar results have been reported from the use of resistant starch by Gargari et al. while significant reductions of 25 and 9% in baseline have been reported following administration of inulin with starch or butyrate, respectively, by another group (148, 151).
Effect on Interleukin-6
Prebiotic supplementation on the levels of IL-6 has been investigated in four studies, two of which have reported significant decrease in levels compared to control after adjusting for baseline values, while two other studies investigating resistant starch and galacto-oligosaccharides have found statistically insignificant results (Table 3) (38, 151). Aliasgharzadeh et al. have reported an adjusted change of –1.45 pg/mL (-28.4%) in IL-6 compared to control following an 8-week course of resistant dextrin, while Dehghan et al. have reported a –1.3 pg/mL (-8.15%) in baseline-adjusted changes in IL-6 vs. control following an 8-week course of oligofructose-enriched Inulin (47, 150).
Effect on Interleukin-12
Two studies have reported the effect of prebiotics on the pro-inflammatory cytokine IL-12 with promising success (Table 3). Dehghan et al. reported that a 10 g/d regimen with prebiotics was associated with a significant reduction of 2.49 ± 1.60 pg/mL in IL-12 levels compared to the change of + 1.23 ± 0.60 pg/mL in those receiving placebo (152). Farhangi et al. also reported a significant change of –2.8 pg/mL from baseline in another group of diabetics receiving a different prebiotic; however, comparison with control after adjusting for baseline values did not yield significant results (153).
Effect on IFN-γ
Unlike the inconclusive results of the multispecies probiotic studies discussed before, of three studies investigating the effect of prebiotics on IFN-γ, two reported favorable results that were statistically significant. Only an early study by Dehghan et al. reported statistically insignificant mean difference of –0.3 pg/mL (16.50%) between intergroup effects (150). Later, Dehghan et al. and Farhangi et al. reported a change of –0.28 ± 0.06 pg/mL from baseline and a baseline-adjusted mean difference of –0.6 pg/mL between intervention and control, respectively (152, 153). The latter group also noted a significant reduction in the IFN- γ/IL-10 ratio of –0.01 (153).
Effect on Endotoxin or Lipopolysaccharide
A total of five studies have investigated the effects of prebiotics on blood endotoxin levels in diabetic patients, a cross-study trends point to prebiotics having a significant negative effect overall (Table 3). Aliasgharzadeh et al. have reported a significant baseline-adjusted mean difference of –6.2 units/mL following intervention with resistant dextrin (-17.8%) (47). Similar significant effects of resistant dextrin administration have been published by two other studies: –6.5 EU/mL (-23.40%), (149) –6.1 EU/mL (153). HP and oligofructose-enriched inulin were also shown to also be significantly negatively associated LPS levels: –4.2 and –6.0 EU/mL (-21.95%), respectively, (147, 150).
Effect on Lipopolysaccharide-Binding Protein
Gonai et al. are the only group that have reported the results of prebiotics on LBP; no mean statistically significant change was observed following a 4-week intervention with galacto-oligosaccharides (Table 3) (38).
Adaptive Immunity Markers in Type 2 Diabetes
Effect on IL-4
More research is required in context to the effect of pre- and synbiotics, on IL-4 post-intervention (Table 3). Dehghan et al. have reported that HP inulin significantly increased IL-4 levels by + 7.41 ± 1.38 pg/mL compared to control in a cohort of diabetic and overweight patients (152). However, more recent study by Farhangi et al. produced inconclusive results as the change due to intervention was positive compared to baseline, but negative compared to the baseline-adjusted change in control; both of these were statistically insignificant (153).
Anti-inflammatory Markers in Type 2 Diabetes
Effect on IL-10
Four studies have reported the effects of prebiotic supplementation in diabetic patients, with the majority following a trend of positive change compared to baseline and/or control (Table 3). Dehghan et al. reported a significant baseline-adjusted mean difference of + 1.9 pg/mL between changes in IL-10 post 8 weeks of intervention with HP inulin compared to control (147). Another study has also reported a similar significant mean difference of + 2.6 pg/mL using resistant dextrin (153). In addition, a study by Dehghan et al. also reported a positive change in IL-10 levels, however, this was not found to be statistically significant, similar to the results of Gonai et al. (38, 150).
Markers of Oxidative Stress in Type 2 Diabetes
Effect on Malondialdehyde
MDA levels have also been widely probed following prebiotic use; four studies have investigated and reported significant baseline-adjusted intergroup mean difference between intragroup changes, while another has reported changes from baseline (Table 3). Aliasgharzadeh et al. have reported net effects of intervention of –1.21 nmol/mL (-25.6%) and –1.7 nmol/mL (-39.7%) using two different prebiotics (47, 154). Farhangi et al. have recently confirmed the effects of resistant dextrin in another study, reporting an intervention effect of –1.21 nmol/mL (-25.58%) (149). Finally, Roshanravan et al. have interestingly also reported the effect of HP inulin with and without butyrate; both effects were significant with mean changes of 1.17 and 0.27 nmol/mL from baseline levels, respectively, after just 45 days (148). Finally, Ahn et al. reported a slight decrease in MDA levels from baseline using Jerusalem artichoke and soyabean mixture, but this was not found to be significant (155).
Effect on Superoxide Dismutase
Like probiotics, all three studies considered exploring the effect of prebiotics on SOD levels have reported positive changes (Table 3). Farhangi et al. have recently reported a significant increase of + 56.3 U/mg Hb from baseline in SOD levels after an 8-week course with resistant dextrin (149). Aliasgharzadeh et al. and Mirmiranpour et al. have also investigated the effects of other prebiotics on SOD levels; however, these were statistically insignificant (144, 154).
Effect on Catalase
Three trials administering prebiotics have reported on their effects on CAT levels, with little evidence to support any one hypothesis (Table 3). Mirmiranpour et al. have reported that a 3 months course of prebiotics was significantly associated with increased CAT activity levels (+ 2.44 ± 0.50 U/mL) compared to the control group (1.95 ± 0.34 U/mL) when baseline levels were not significantly different (144). Furthermore, a similar increase in mean CAT/Hb ratio (+ 12.3 U/g Hb) in the intervention group was reported by another group, this, however, was not statistically significant (154). Only Farhangi et al. reported a non-significant decrease in mean CAT following prebiotic use (149).
Effect on GPX
Three distinct clinical studies have reported the use of prebiotics to investigate changes in GPX levels among diabetic patients (Table 3). Of these, only Farhangi et al. have presented results that were significant; following an 8-week course of prebiotics, a difference of + 3.80 U/g Hb was observed from baseline in the intervention group (149). Aliasgharzadeh et al. and Mirmiranpour et al. have also reported on GPX levels post-prebiotic administration; however, almost no change was observed in both cases following supplementation using their respective prebiotics (144, 154).
Effect on Total Antioxidant Capacity
Two studies have investigated the effect of prebiotics on TAC levels, with each reporting a significant increase post-supplementation (Table 3). Aliasgharzadeh et al. reported a mean difference of + 0.2 mmol/L (+ 20.0%) associated with the prebiotic intervention (154), while Farhangi et al. more recently have reported a larger change of + 0.33 mmol/L (36.25%) (149).
Effect on OxLDL
Mirmiranpour et al. reported that compared to a control group without significantly different baseline OxLDL levels, an intervention of prebiotic was associated with a lower mean OxLDL (16.32 ± 1.21 vs. 17.07 ± 1.01 mU/L) at 3 months; however, this was not statistically significant (Table 3) (144).
Effect of Synbiotics on Inflammatory and Oxidative Stress Markers in Type 2 Diabetes
Pro-inflammatory Markers in Type 2 Diabetes
Effect on CRP and hs-CRP
A total of eight studies reported the association between synbiotic consumption in diabetics and either CRP or hs-CRP, with many studies reporting significant desired results (Table 4). Moreover, like the trend observed in probiotics, multispecies synbiotic supplementation outperform single-species probiotics in their ability to result in desired changes in CRP and hs-CRP levels.
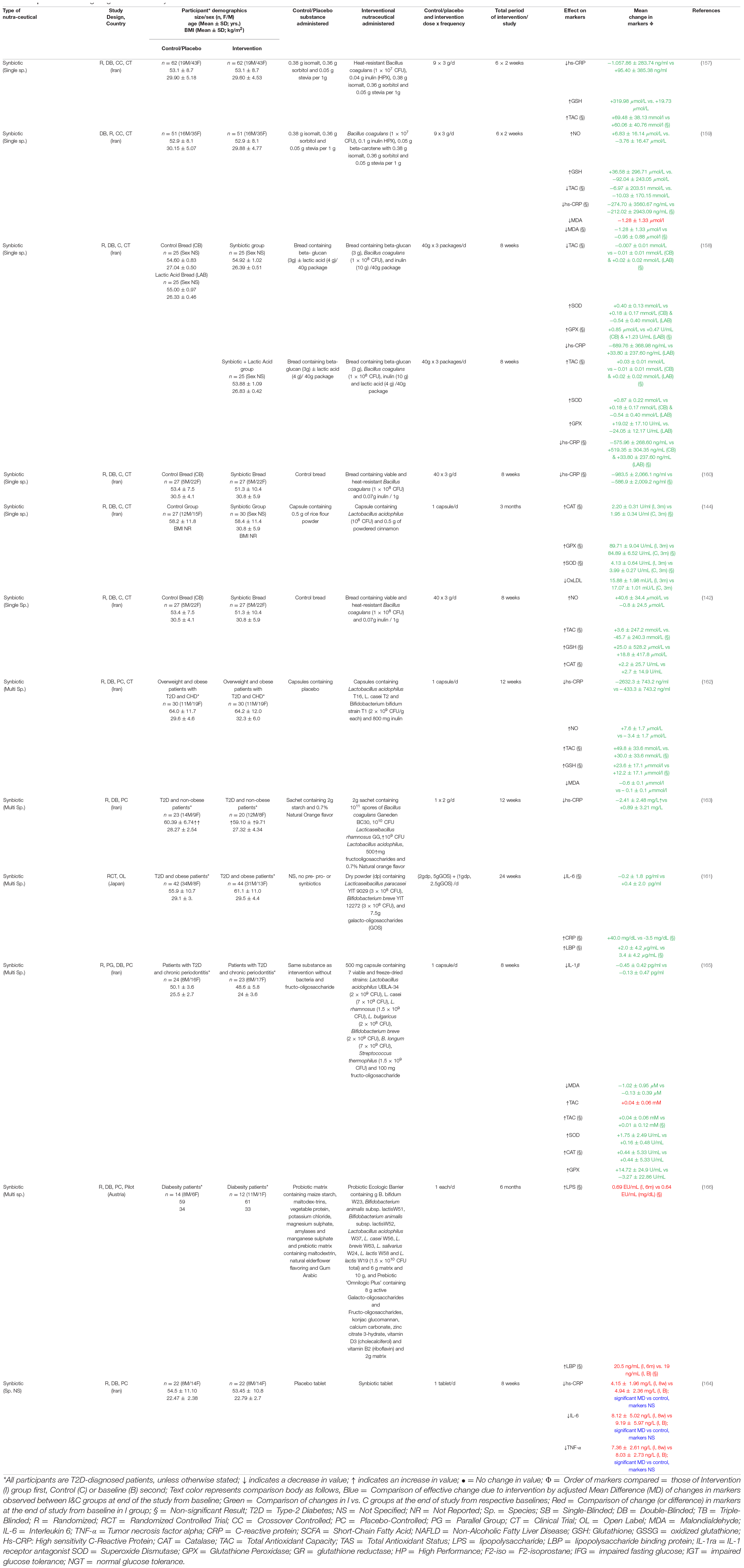
Table 4. Studies investigating the effects of synbiotics on markers of inflammation and oxidative stress.
Asemi et al. reported that a single species synbiotic mixture of Bacillus coagulans (previously Lactobacillus sporogenes) and inulin significantly correlated to hs-CRP level changes from baseline of –1.057 ± 0.283 mg/L compared to a slight mean increase of 0.0054 ± 0.385 mg/L in control, whereas another intervention group from a study using B. coagulans and inulin rich bread presented a significant change of –689.76 ± 368.98 μg/L compared to an increase of 33.80 ± 237.60 μg/L in a control group consuming lactic acid-enriched bread (156, 157). Similar studies investigating the effects of single species synbiotics using other formulations of B. coagulans and inulin have all showed a trend of decrease in the levels of either CRP or hs-CRP, although these results were not significant (157–159).
Among multi-species synbiotic nutraceuticals, only Kanazawa et al. recently reported a non-significant increase of 40.0 mg/dL in CRP from baseline following supplementation with Lacticaseibacillus paracasei, Bifidobacterium breve and galacto-oligosaccharides (GOS) in a cohort of diabetic and obese patients in Japan (160). However, two other studies based in Iran investigating cohorts with similar comorbidities reported significant inter-group reductions in the levels of hs-CRP compared to controls following supplementation consisting of Lactobacillus acidophilus, L. casei, Bifidobacterium bifidum and inulin, and B. Coagulans, Lacticaseibacillus rhamnosus, Lactobacillus acidophilus and fructooligosaccharides, respectively, (161, 162). A study by Kooshki et al. (163) reported significant decrease in hs-CRP from both baseline vs. control, although the composition of the tablet was not specified.
Effect on TNF-α
It is interesting to note that the effect of synbiotics on TNF-α is minimally reported (Table 4). Kooshki et al. (163) have found that multi-species synbiotic supplementation led to a mean decrease of ∼0.67 pg/mL following administration of a synbiotic tablet; however, the composition of this supplement was not reported.
Effect on IL-1β
Like TNF-α, the data on synbiotic supplementation on IL-1β is currently limited and requires further research (Table 4). Nonetheless, Bazyar et al. (164) have reported significant changes of –0.45 ± 0.42 pg/mL compared to the effect of control following an 8-week supplementation with a 7-species plus fructo-oligosaccharides synbiotic in a cohort of diabetics with chronic periodontitis from Iran.
Effect on IL-6
There is a limitation in the literature exploring the effects of synbiotic supplementation on IL-6, with only two studies reporting an overall average decrease in IL-6. However, only one of those reports was found to be statistically significant (Table 4). Kooshki et al. have reported a significant ∼1 ng/L change from baseline in IL-6 compared to control following an 8-week supplementation of synbiotic (composition unreported) (163). A further statistically insignificant change of –0.2 ± 1.8 pg/mL in IL-6 was reported by another group following a 24-week regimen of galacto-oligosaccharide and multispecies synbiotic (160).
Effect on Endotoxin (LPS)
Endotoxin (LPS) levels have not been widely investigated in the context of synbiotic administration (Table 4). Nevertheless, it is interesting to note that Horvath et al. have recently described a mean increase of 0.05 EU/mL from a baseline of 0.64 EU/mL in LPS levels following a 6-month intervention with a multi-species probiotic; however, this was not found to be significant with respect to intragroup baseline or the intergroup (control), perhaps owing to the small sample size in the pilot study (165).
Effect on Lipopolysaccharide-Binding Protein
Multispecies synbiotic intervention was associated with a larger change in mean LBP levels than either pro- or synbiotics, although both studies investigating their association failed to show statistically significant results (Table 4). Kanazawa et al. and Horvath et al. reported an increases in average marker levels from baseline (+ 2.0 ± 4.2 μg/mL and + 1.5 ng/mL, respectively) following a 6-month intervention (160, 165).
Markers of Oxidative Stress in Type 2 Diabetes
Effect on Malondialdehyde
MDA levels have been investigated as a marker of oxidative stress following synbiotic use in three studies; all three have reported some form of significant decrease in mean levels of MDA following supplementation (Table 4). Asemi et al. reported that a single-species probiotic was associated with a significant decrease of –1.28 ± 1.33 μmol/L from baseline; however, this was not significant compared to the change seen in control (158). However, two recent studies by Farrokhian et al. and Bazyar et al. have yielded significant changes of –0.6 ± 0.1 and –1.02 ± 0.95 μmmol/L following multi-species probiotic use (161, 164).
Effect on Superoxide Dismutase
The effect of synbiotics on SOD levels in diabetics were investigated by three studies, all of whom have reported an increasing effect following intervention (Table 4). Ghafouri et al. reported significant rise in SOD levels of + 0.40 ± 0.13 and + 0.87 ± 0.22 mmol/L following an 8-week supplementation with bread containing single-species probiotics without and with lactic acid, respectively (157). Mirmiranpour et al. presented a statistically insignificant increase of 0.14 U/mL in mean SOD levels between the two groups (144). While Bazyar et al. reported a large increase of + 1.75 ± 2.49 U/mL following a similar time frame of intervention using a multi-species probiotic in diabetic patients with chronic periodontitis (164).
Effect on Catalase
While three trials have reported the effect of various synbiotics on CAT activities, these were not found to be statistically significant, despite all showing upward slopes following intervention (Table 4). Mirmiranpour et al. reported that the mean CAT activities between the group receiving single-species synbiotic and the control differed by 0.25 U/mL at the end of trial, while another study reported a change of + 2.2 ± 25.7 U/mL from baseline in the intervention group (142, 144). Finally, although Bazyar et al. reported a slight increase in CAT activity from baseline, the change was found not to differ from control (164).
Effect on GPX
The results of synbiotic use on diabetics with respect to GPX has been investigated in three studies showing promising results (Table 4). Bazyar et al. has recently presented a significant promising change of + 14.72 ± 24.9 U/mL from baseline compared to control (164). On the other hand, Ghafouri et al. has reported another significant change of + 19.02 ± 17.10 U/mL in GPX following administration of a bread containing another synbiotic combination (157). The results of Mirmiranpour et al. show promise in their large mean increase from baseline compared to control, however, it was not statistically significant (144).
Effect on GSH
The effect of synbiotics on mean reduced glutathione (GSH) levels have also been investigated across literature; while all of five such studies report an increase in the mean GSH, only two have shown to be statistically significant (Table 4). Through two similar single-species synbiotics, Asemi et al. have shown increases of + 319.98 (vs. + 19.73 μmol/L in control) and + 36.58 ± 296.71 (vs. –92.04 ± 243.05 μmol/L in control) (156, 158). A more recent study by Raygan et al. demonstrated an increase in mean GSH (+ 18.0 ± 112.7 vs. –12.2 ± 122.5 μmol/L in control) following another single-species synbiotic supplement, these effects, however, were not statistically significant (127). In another study investigating multi-species synbiotic use among diabetics failed to show significant results given a similar rise in mean GSH in control (142). In addition, a recent study among severely comorbid diabetics had also failed to reach statistical significance in the findings (161).
Effect on Total Antioxidant Capacity
While multiple studies have investigated the effect of synbiotic use on TAC, the overall trends are not conclusive, with only a single study reporting a statistically significant association (Table 4). Bazyar et al. have reported a significant increase in TAC of + 0.04 ± 0.06 mM from baseline following a multispecies probiotic supplementation; however, this was not significant when compared to the change in control (164). On the other hand, Farrokhian et al. reported a larger absolute increase in mean TAC level (+ 49.8 ± 33.6 mmol/L), however, it was also not significant compared to a the change of + 30.0 ± 33.6 mmol/L in control (161).
Among single-species probiotics, Asemi et al. reported, across two studies, mean changes of + 69.48 ± 38.13 and –6.97 ± 203.51 mmol/L following different formulations; however, in both instances, the average change was positive when compared to control, although statistically insignificant (156, 158). A similar (yet statistically insignificant) trend is seen in the case of Bahmani et al. wherein a change of + 3.6 ± 247.2 mmol/L was found among the intervention group compared to –45.7 ± 240.3 mmol/L among the controls (142). Finally, Ghafouri et al. compared the effects of synbiotic bread with or without a metabolite, however, the effect of both on TAC levels were found to be both statistically and numerically insignificant (157).
Effect on Nitric Oxide
Very few studies have reported on the effect of NO following synbiotic supplementation among diabetics; however, of the available studies, all have shown significant associations following synbiotic supplementation (Table 4). Bahmani et al. reported an increase of + 40.6 ± 34.4 μmol/L in NO levels following an 8-week course of single-species synbiotic bread (142), while Asemi et al. reported an increase of + 6.83 ± 16.14 μmol/L following a 6 × 2 weeks crossover trial of another singles-species synbiotic (158). On the other hand, Farrokhian et al. showed a significant increase of + 7.6 ± 1.7 μmol/L after a 12 week intervention with a multi-species synbiotic (161).
Effect on Oxidized Low-Density Lipoprotein
Mirmiranpour et al. reported that compared to a baseline matched control group, an intervention of single-species synbiotic was significantly associated with a lower mean OxLDL (15.88 ± 1.98 vs. 17.07 ± 1.01 mU/L) at 3 months (Table 4) (144).
Discussion
This systematic review collectively pooled data from forty-seven randomized controlled trial (RCT) studies to investigate the effect of probiotic, prebiotic and synbiotic supplementation on various markers of inflammation and oxidative stress among patients with T2D, with or without other comorbidities. Our results point toward the successful capacity of gut-microbiome promoting therapeutics to have beneficial effects on multimodal inflammation and oxidative stress inducing factors in the pathogenesis of T2D. Here we discuss intervention-specific trends in our findings that may add to growing evidence of currently researched questions and/or incentivize novel discoveries.
The Promise of Probiotics
Described as “live microbial feed supplements which beneficially affect the host animal by improving its intestinal microbial balance,” (166) probiotics have been widely studied across literature given their capacity to have clinical therapeutic potential by antagonizing pathogenic or “harmful” bacteria and/or reversing pathogenic dysbiosis of the microbiome (166–170). Despite their role in metabolic diseases only being described from 2007 after a description of their potential role in obesogenesis in mice (171), a large array of mechanisms listing their potential against T2D and clinical trials testing their efficacies have surfaced.
A recent systematic review of association of different bacterial gut microbiome species in the T2D pathophysiology has reported that most species of Bifidobacterium are associated with a protective function in T2D, with lower levels of this probiotic compared to healthy controls being reported in T2D patients (172). Strain and species-specific associations of (previously) Lactobacillus have also been reported, although with more complexity in interpretation, given apparent opposite effects of different strains. Among studies investigating the effect of probiotics on inflammatory and oxidative stress biomarkers, multi-species probiotic supplementation has shown to be largely consistently more effective compared to single-species or monostrain probiotic administration. This can be observed in pro-inflammatory markers like hs-CRP (and CRP), TNF-α, IL-β, IL-6, IL-8, and LPS (endotoxin) across multiple trials (123, 136–140). Among these, (previously) Lactobacillus and Bifidobacterium strains such as L. casei, L. rhamnosus, L. gasseri, L. plantarum, B. infantis, B. longum, and B. breve, often in synergism, and to a lesser extent, Lactococcus, Propionibacterium, and Acetobacter strains, have been reported to be consistently promising. Bifidobacterium have been shown across multiple animal studies to have promising probiotic effects in multiple metabolic dysfunctions through a variety of mechanisms; these include restoring the lymphocyte-macrophage balance and gut microbiota structure, reducing B-cell infiltration and increasing Treg activity (173–175), and modulating gut microbiome resulting in higher acetate SCFA levels (176). Animal models have also cemented that Bifidobacterium and (previously) Lactobacillus strains including B. adolescentis N3, B. adolescentis 7-2, B. bifidum M2, L. rhamnosus 7-1, and L. rhamnosus YC, were independently correlated with reduced levels of inflammatory biomarkers such as TNFA, IL1B, and IL-6 (177). However, given that (previously) Lactobacillus single-species administration has been associated with insignificant differences across multiple clinical trials reported in this review (124, 129, 143, 178), we may conclude that their probiotic effects are best attained in humans when used in combination with other strains and species of the same or different genus. This superiority of multistrain and multispecies probiotic is not new and has been described across other diseases and trials of the gut, such as pouchitis and ulcerative colitis (179). This is likely due to the enhanced probability of at least one of the many strains/species administered to survive, adapt, and produce anti-pathogenic and dysbiosis-attenuating response upon administration and survival in diseased microbiome consisting of harmful bacteria, whereas a single species is more vulnerable to endogenous microflora. In addition, single-species probiotics are limited in their therapeutic potential given that they are limited to the species-specific ability of the probiotic to render beneficial changes, whereas a collection of multispecies bacteria may not only have multiple mechanisms of completing similar beneficial pathways but may also use different pathways to achieve similar end effects.
Additionally, probiotics like Lactobacillus acidophilus, L. reuteri, L. fermentum, L. bulgaricus, Bifidobacterium bifidum, Bifidobacterium animalis subsp. Lactis, and Streptococcus thermophilus have shown significant promise with respect to markers such as MDA (127), SOD, (143), GPX (144), GSH (124), TAC, and NO (125). Possible mechanisms reported include their effects on ascorbate autoxidation, metal-ions chelation, antioxidant enzymes system, and various antioxidant metabolites such as GSH (180), butyrate, and folate (181), and activity reduction and excretion of free radicals such as superoxide anion and hydrogen peroxide (182, 183). Their mode of delivery includes fermented milk, yogurt, bread, or simply as supplemental capsules. Hence a combination with sources that are shown to have probiotic-independent antioxidant abilities such as that of casein-derived peptides should be considered to maximize effectiveness (184). Using animal models, Hsieh et al. have shown that multi-strain probiotic composed of Lactobaccilus salivarius subsp. salicinius AP-32, L. johnsonii MH-68, L. reuteri GL-104, and Bifidobacterium animalis subsp. lactis CP-9 improved not only inflammatory markers, but also reduced MDA and increased SOD levels (185), potentially having β-cell protective function which would otherwise be disrupted due to oxidative stress (186).
The Promise of Prebiotics
Prebiotics refer to non-digestible and fermentable food ingredients serving as substrates that are selectively utilized by host gut-microbiota to provide health benefits through encouraging the growth of beneficial bacteria (187, 188). They are abundantly found in multiple fruits, vegetables, and cereals, including bananas, beans, garlic, onions, peas, and artichoke in the form of polysaccharides such as inulin, oligosaccharides, including both galactooligosaccharides and fructooligosaccharides, resistant starch, and even cinnamon (144, 167). By traveling undigested through the upper GI system, they are available for fermentation by the beneficial bacteria in the colon (189), leading to the production of beneficial metabolites such as SCFAs (acetate, butyrate, propionate) and lactic acid, which has significant effects on inflammation and intestinal membrane integrity (26), along with other mechanisms.
In our review, although we have highlighted the promise offered by a variety of prebiotic supplementations, resistant dextrin has been shown to be the most widely effective in both categories of markers (147, 149, 152, 153). Resistant dextrins are non-sweet short-chain glucose polymers with high resistance to digestive enzymes of the human gut, up to 75% of which is available for fermentation (172, 190). Ślizewska et al. have shown that resistant dextrin supplementation among mice was associated with lower levels of Clostridium strain (191), which is found in elevated levels among diabetics compared to normal gut (192). Resistant dextrin also led to higher levels of both the beneficial Bifidobacterium and prior Lactobacillus strains in the faces and cecum of rats without changing the overall bacterial count significantly (191). Similar results have been reported from clinical trials involving dextrin supplementation in humans (193). Valcheva et al. reported that IL-10 deficient mice fed with fiber dextrin diets over the course of 12 weeks secreted 47–88% less colonic IL-1β, TNF-α, IL-23, IL-12p70, IL-6, and CXCL1, with lower enterocyte injury scores and an increase in butyrate SCFA production (194). It has been priorly described (150) that through production of NF-κβ, butyrate modulates inflammation, controls macrophage and neutrophil activators and chemoattractant (195), and increases the expression of cytokine signaling 3 suppressor (196), all of which serve to promote anti-inflammatory Th2-lymphocyte differentiation rather than into Th1, ultimately increasing IL-10 levels among other mechanisms (197).
The prebiotic is also associated with beneficial changes in serum insulin, lipid, and gut microbial composition, with the promotion of the insulin signaling and the fatty acid β oxidation pathways in high-fat-high-fructose diet-fed rats and the enhancement of Parasutterella and Parabacteroid relative abundances and prevention of further harmful gut dysbiosis (198). Total SCFA concentrations and those of acetate, butyrate, and propionate individually were found to be dose-dependently higher among a group of rats fed increasing amounts of resistant dextrin compared to control (199). Finally, among two placebo-controlled RCTs investigating NUTRIOSE, a commercial resistant dextrin formula, increased levels of Bacteroides and SCFAs were observed (199). Resistant dextrin has also shown to significantly increase GSH/GSSG ratio (by 33%) in rat models (200). By promoting growth of acid-resistant bacteria (such as Roseburia) that produce butyrate, resistant dextrin has shown to reduce ROS levels and increase plasma antioxidant enzymes through an LPS-mediated process that includes multiple factors such as NF-κβ (201–203). In addition, inhibiting the growth of Clostridium perfringens has been shown to reduce ROS through PKC, MEK/ERK, and NFκB pathways (149). Other mechanisms through which prebiotics including resistant dextrin but also oligo-fructose-enriched inulin, may modulate oxidative stress markers include reducing advanced glycation end -products and by serving as scavengers of ROS (150). Our findings are consistent with prior reviews (188) in this field.
The Promise of Synbiotics
Synbiotics are combinations of probiotic and prebiotic that are administered together. The rationale behind acceptance of their co-administration has been multifaceted: it has been shown to improve probiotic survival through provision of metabolic substrates that facilitate gastrointestinal tract transition, increase viability (204) and possible synergistic effects that may be independent of but parallel to the effects of the probiotic itself (205). Such combinations have shown to have beneficial effects on insulin resistance and glucose metabolism (25). Moreover, adding a prebiotic such as inulin to probiotic milk, yogurt, ice cream, and cheese formulations was found to increase survival in storage, increase apparent viscosity (206). Green et al. have also argued that the rationale of specific matching of prebiotic and probiotic can also include the possibility that certain prebiotics promote the growth of some bacteria more than others, if at all, based on the findings of Scott et al. who report that chain length of fructans (in prebiotic) is an important factor determining fermentation-specificity between species (207). Perhaps the most significant effect of a synbiotic is conferred from the study by Nazzaro et al. where the authors show that Lactobacillus acidophilus growth using inulin was associated with 14.5 times more butyrate production than in the presence of pectin, both of which were still significantly higher than administration of the probiotic with just glucose, with undetectable levels of butyrate (208). In addition to the priorly discussed roles of SCFAs such as butyrate, it has also been shown that butyrate inhibits IFN-γ production and has an active role in regulating peroxisome proliferator activated receptor-g (209). Clinical trials have reported more effective beneficial effect of synbiotic administration than in the case of probiotics alone in the case of prediabetic individuals with focus on prediabetes (210).
In our study, we have shown that across multiple studies investigating probiotics, prebiotics and synbiotics, the latter group of supplements have shown to be more effective compared to the former two primarily when comparing the change in oxidative stress biomarkers and that of antioxidant enzymes, namely MDA (164), plasma (164) and erythrocyte SOD (143), GPX (157), GSH (156), NO (142), and OxLDL (144). A combination of multispecies probiotic of Lactobacillus acidophilus, L. casei, L. rhamnosus, L. bulgaricus, Bifidobacterium breve, B. longum, Streptococcus thermophilus, and the prebiotic fructo-oligosaccharide has shown exemplary promise among the greatest number of markers. Bacillus coagulans with inulin was the most effective single species synbiotic. Given the previously described rising importance to “mix-and-match” prebiotic with a probiotic that can derive maximal benefit from it, the work of Fuhren et al. with respect to synbiotic matchmaking using inulin, fructooligosaccharides and multiple strains of Lactiplantibacillus plantarum show an effective screening model that may enable researchers and industrial manufacturers to create the ideal synbiotic (211). One such study by Nagpal and Kaur revealed that L. casei, one of the seven probiotic species of the most promising synbiotic identified in this review, had higher viability in inulin compared to oligosaccharide media (212); it would be interesting to compare the other strains and species with various prebiotics, especially resistant dextrin, which was identified as the most promising in this review.
Intestinal Dysbiosis and Inflammation
Apart from the direct impact of poor nutrition on the immune response, changes in the intestinal microbiota due to obesogenic environmental factors can also stimulate inflammation (213). The altered composition of the gut microbiota due to the consumption of a high-fat, low-fiber diet has been directly correlated with a pronounced low-grade inflammatory state linked to T2D (214). This is because consumed fibers are broken down and fermented by the gut microbiota in the large intestine into short chain fatty acids (SCFAs), including butyrate, propionate, and acetate (215). These metabolites are recognized by GPR41 and GPR43, both of which are G protein-coupled receptor. SCFAs are considered a main source of nutrients and energy for colonocytes and microbes, are involved in the regulation of energy and pH levels, and stimulate the release of glucagon-like peptide-1 (GLP-1) (216). Lower fiber intake results in a decrease in metabolites such as SCFAs and eventually lead to cases of intestinal dysbiosis. Microbial metabolites also have significant implications on inflammatory responses. Butyrate controls the maturation of dendritic cells (DC) as well as preventing associations between adipocytes and macrophages (217). Propionate, on the other hand, decreases synthesis of adipokine in adipocytes (218). Acetate also participates in the maintenance of balance in the gut by suppressing the expression of inflammatory cytokines while upregulating the synthesis of anti-inflammatory cytokines (26). Thus, a decrease in these metabolites due to reduced fiber intake can stimulate pro-inflammatory responses in patients with T2D. Additionally, dysbiosis allows potentially pathogenic microbes to shift locations and replicate faster in the gut epithelium. Large numbers of translocated bacteria are recognized as invaders by the immune system (219), thereby evoking a chronic inflammatory response through the activation of toll-like receptors (TLRs) and upregulation of pro-inflammatory cytokines (220).
Since there is a proven alteration in the gut microbiota of T2D, researchers have investigated the merit of correcting this dysbiosis as a potential cure for T2D. It is unclear if measurable alteration in the gut microbiota directly correlates to or is needed for pro/prebiotics to exert their effect for treating T2D (165). The short study duration of some of the clinical studies can explain this; for example, Gonai et al. (38) have shown that while the prebiotic galacto-oligosaccharide (GOS) ameliorated the decrease of Bifidobacteriaceae in T2D, LPS- binding protein (LBP) and glucose tolerance did not improve during the trial period. LPB stimulates inflammatory cytokines through TLR4 and thus it provides a potential mechanism of how the gut microbiota dysbiosis causes T2D (38). However, in another small study examining the effect of GOS on gut permeability no significant improvement has been found (221). It is important to note that the first study had a bigger sample size and a larger administered dose of GOS than the second. Another prebiotic fiber that is extensively studied in the treatment of T2D is inulin-type fructans. Increased production of SCFAs improves T2D and a 50/50 mixture of inulin and oligofructose increases bifidobacteria and SCFAs in feces, while butyric acid and the microbial diversity are not affected (41).
Intestinal Permeability and Inflammation
The intestinal barrier is a semipermeable membrane that regulates the absorption of nutrients and electrolytes from the lumen into the blood stream, while preventing the entrance of infectious microorganisms as well as antigens, endotoxins, and proinflammatory substances (222). Intestinal permeability, therefore, is an intrinsic characteristic of the intestinal epithelium that allows for the exchange of luminal substances whilst maintaining an immunological barrier. However, intestinal hyperpermeability, commonly referred to as a “leaky gut,” has been linked to several disorders, including gastrointestinal, such as celiac disease and colon carcinoma, in addition to other extra-intestinal diseases, including diabetes (223). Even though defective intestinal barriers could be a result of disease aggravation, clinical studies hypothesize that it could also be a causal factor in the progression of disease and the initiation of autoimmune destruction (224). Altered intestinal barrier function leads to an unrestricted influx of antigens or toxins into the gut, consequently instigating an inflammatory response in the lumen and other proximal organs (225). Increased intestinal permeability has, thus, become a new target for disease prevention and therapy of type 1 and 2 diabetes (226). Considering the close relationship between intestinal permeability and gut dysbiosis, we can conclude that meticulous dietetic and probiotic approaches to recover healthy microbiota have the potential to make a breakthrough in the management of these diseases in the near future.
An epidemiological study has shown that the increased levels of 16S ribosomal DNA from gut bacteria in the blood is a risk factor for diabetes (227). Thus, it is hypothesized that bacterial translocation could play a role in T2D pathophysiology. It has been found that the administration of the probiotic Lacticaseibacillus casei strain Shirota could reduce gut bacterial translocation and can alter the gut microbiota in patients with T2D (135). Additionally, L. reuteri and L. gasseri both were found in higher levels in the probiotic fed subjects’ fecal samples. This may explain the decreased bacterial translocation as these bacteria improve the membrane integrity through mucus production expression of tight junctions and reduction of apoptosis (135). Further, it was also shown by Horvath et al. (165) that multispecies synbiotic strengthens the gut barrier function and thus reduces levels of c-peptide, LPS and bacterial DNA in the participants’ serum, indicating a decreased translocation of bacterial products to the blood.
Gut Microbiota, Nutraceuticals, COVID-19, and Diabetes
While age remains to be the most significant predictor of COVID-19 related morbidity and mortality, diabetes, along with other chronic conditions, was identified early as a significant comorbidity of the disease (228), with diabetes being present in over one-third of hospitalized individuals in one New York City cohort (11). Low-grade inflammation, characterized by chronically increased levels of inflammatory cytokines such as IL-6, TNF-α, and IL-1β, is present as a common feature of many metabolic diseases including T2D, as well as COVID-19 (229). Since diabetics with low-grade inflammation compared to healthy controls have shown notable reductions in serum inflammatory markers following insulin therapy (230) and COVID-19 associated mortality among diabetics was also significant reduced in a subgroup with controlled blood glucose (231), it may very well be the case that control of low-grade inflammation through modulation and correction of gut dysbiosis may serve as a significant therapeutic strategy against COVID-19 associated mortality and morbidity among those with pre-existing T2D. Perhaps unsurprisingly, COVID-19 hospitalized patients have been shown to have “significant alterations in fecal microbiomes, characterized by enrichment of opportunistic pathogens (such as Coprobacillus, Clostridium ramosum, and Clostridium hathewayi) and depletion of beneficial commensals (such as Faecalibacterium prausnitzii, an anti-inflammatory bacterium, and multiple Bacteroides species responsible for downregulation of ACE2 expression)” (232). It is then expected that probiotics may be able to assist host innate and adaptive immunity in COVID-19 struck patients as a form of adjuvant strategy (233). In addition to resolution of gut dysbiosis, probiotic have the potential to contribute to a healthy gut-lung axis by reducing translocation of pathogens through the intestinal mucosa, thereby reducing the potential of simultaneous infections, which may lead to poorer prognosis. The effects of bacteria from the previously Lactobacillus and Bifidobacterium genus have been elucidated in our systematic review; it is very encouraging to also note that these species have shown great promise in reducing the incidence of Ventilator associated pneumonia and upper-respiratory tract infections in venerable cohorts (234). In fact, probiotics have been shown to have a larger beneficial effect in inflammatory biomarker levels such as IL-6 and CRP as a measure of low-grade inflammation than even Angiotensin Receptors Blockers, omega-3, metformin, resveratrol, and vitamin D (235). These gut-microbiome modulating nutraceuticals have shown to serve also as immunomodulators leading to downregulation of the low-grade inflammation state (236, 237), leading to an overall reduction or attenuation of COVID-19 related symptoms such as “diarrhea, abdominal pain, vomiting, headache, cough, sore throat, fever, and viral infection complications such as acute respiratory distress syndrome (ARDS)” (238). By close monitoring of potential interactions with diabetes and antiviral drugs, especially conserving that many antibiotics given during COVID-19 infection may further lead to gut microbiome dysbiosis (233), probiotics, along with pre- and synbiotics, may serve as therapeutic agents with low adverse event incidence for treatment of diabetics with COVID-19 (239).
Conclusion
Systematic review of current literature showed that T2D patients have a different gut microbiome composition than healthy individuals which may result from dysbiosis due to the pathogenic state. On the other hand, it is also plausible that this altered gut microbiota has the potential to lead early onset and development of T2D. By altering the gut microbiota using pre-, pro-, and synbiotics, it is possible to modify factors causing inflammation and oxidative stress. In this review, we have reported on multiple promising and effective pre-, pro-, and synbiotics for their association to changes observed in markers of inflammation and oxidative stress. We have identified significant trends and observations between single and multistrain probiotics, identified the most promising rising synbiotic as resistant dextrin, and showed how synbiotics may be more effective compared to the other two types of nutraceuticals among oxidative stress markers. Furthermore, we have elucidated and reviewed the role of metabolites, signaling pathways, low-grade inflammation, gut permeability, and dysbiosis with respect their ultimate roles in the pathogenesis and possible therapy in T2D, as well as the potential of these nutraceuticals to attenuate COVID-19 infection related symptoms. Figure 2 provides a generalized summary of the role of microbiome-targeted nutraceuticals in T2D pathogenesis and potential correction of dysbiosis.
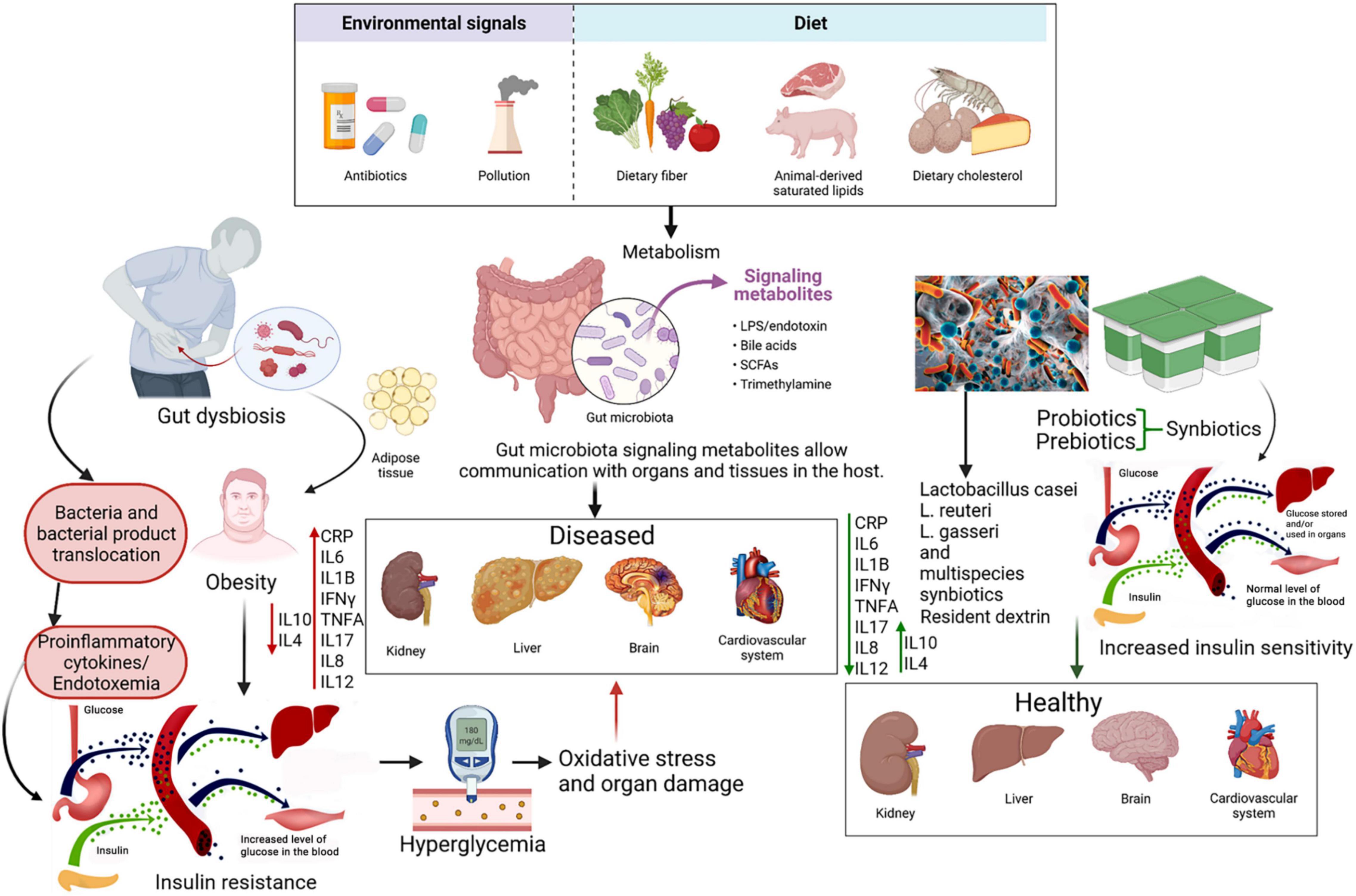
Figure 2. Summary diagram illustrating the effect of diet, pre and probiotics as a potential safe therapy in treating T2D.
Final Remarks, Prospects and Clinical Trials
While human clinical trials in literature have elucidated the great potential and promise of using pro-, pre-, and synbiotics as therapeutic agents for the treatment of T2D, further large-scale and multicenter trials and investigations are required given their controversial results in the past. What also needs to be assessed and delivered is the type of biological agents that are most likely to be accepted widely, wildtype or recombinant, single or multiple strain/species. Multi-center and longer-term trials that are coordinated in their research methodology and analysis of data need to be undertaken to compare the effects of variable factors such as genetic susceptibility to the disease and genetic acceptance to the biotic therapy suggested here. In the interim, clinicians and researchers alike should follow ongoing clinical trials such as NCT05110703 that investigates the effect of a daily prebiotic fiber meal replacement shake on the quality of life in T2D patients. In addition, trials such as NCT04089280 go a step beyond and aim to elucidate the effect of an 8-strain multispecies probiotic in their capacity to reduce metformin-induced GI adverse effects. Finally, NCT04769687 is perhaps the most promising trial that will attempt to investigate the effect of a twice-daily 8-strain multispecies, oligofructose and Raftiline HP synbiotic on a variety of parameters among T2D plus CKD patients, with the primary outcome of CRP levels, followed by inflammatory cytokines, circulating monocytes, microbial metabolome, membrane permeability, bacterial translation, quality of life and frailty.
Data Availability Statement
The original contributions presented in the study are included in the article/supplementary material, further inquiries can be directed to the corresponding author/s.
Author Contributions
AC designed the study, critically supervised the project, revised and reviewed the manuscript, and initially screened studies. RS performed the database search, references extraction, methodology section draft and flowchart, and reviewed and edited the manuscript. BA screened the study abstracts and full-texts. PP and RK further critically screened full-texts, extracted, and analyzed the data, updated the search, wrote majority of the manuscript, generated most of the tables, and further edited the manuscript. MA wrote parts of the manuscript and generated a table. MS and RU reviewed and edited the manuscript, and generated one figure. All authors have read and agreed to the published version of the manuscript.
Funding
This research was supported by Weill Cornell Medicine Qatar through a Student Research Mentorship Program SRMP-003-04: Identification and characterization of biological properties of probiotic content of Qatar’s dairy products.
Conflict of Interest
The authors declare that the research was conducted in the absence of any commercial or financial relationships that could be construed as a potential conflict of interest.
Publisher’s Note
All claims expressed in this article are solely those of the authors and do not necessarily represent those of their affiliated organizations, or those of the publisher, the editors and the reviewers. Any product that may be evaluated in this article, or claim that may be made by its manufacturer, is not guaranteed or endorsed by the publisher.
Acknowledgments
Biorender.com was used for creating some figures using appropriate licenses.
References
1. Chen L, Magliano DJ, Zimmet PZ. The worldwide epidemiology of type 2 diabetes mellitus – present and future perspectives. Nat Rev Endocrinol. (2012) 8:228–36. doi: 10.1038/nrendo.2011.183
2. Saeedi P, Petersohn I, Salpea P, Malanda B, Karuranga S, Unwin N, et al. Global and regional diabetes prevalence estimates for 2019 and projections for 2030 and 2045: results from the international diabetes federation diabetes atlas, 9th edition. Diabetes Res Clin Pract. (2019) 157:107843. doi: 10.1016/j.diabres.2019.107843
3. Menke A, Orchard TJ, Imperatore G, Bullard KMK, Mayer-Davis E, Cowie CC. The prevalence of type 1 diabetes in the United States. Epidemiology. (2013) 24:773–4. doi: 10.1097/EDE.0b013e31829ef01a
4. Maddaloni E, Buzzetti R. Covid-19 and diabetes mellitus: unveiling the interaction of two pandemics. Diabetes Metab Res Rev. (2020) 36:e33213321. doi: 10.1002/dmrr.3321
5. Bshesh K, Khan W, Vattoth AL, Janjua E, Nauman A, Almasri M, et al. Lymphadenopathy post-COVID-19 vaccination with increased FDG uptake may be falsely attributed to oncological disorders: a systematic review. J Med Virol. (2022) 94:1833–45. doi: 10.1002/jmv.27599
6. Shafiq A, Salameh MA, Laswi I, Mohammed I, Mhaimeed O, Mhaimeed N, et al. Neurological immune-related adverse events after COVID-19 vaccination: a systematic review. J Clin Pharmacol. (2022) 62:291–303. doi: 10.1002/jcph.2017
7. Al-Ali D, Elshafeey A, Mushannen M, Kawas H, Shafiq A, Mhaimeed N, et al. Cardiovascular and haematological events post COVID-19 vaccination: a systematic review. J Cell Mol Med. (2022) 26:636–53. doi: 10.1111/jcmm.17137
8. Mohammed I, Nauman A, Paul P, Ganesan S, Chen K-H, Jalil SMS, et al. The efficacy and effectiveness of the COVID-19 vaccines in reducing infection, severity, hospitalization, and mortality: a systematic review. Hum Vaccin Immunother. (2022) 18:2027160. doi: 10.1080/21645515.2022.2027160
9. Singh AK, Gupta R, Misra A. Comorbidities in COVID-19: outcomes in hypertensive cohort and controversies with renin angiotensin system blockers. Diabetes Metab Syndr Clin Res Rev. (2020) 14:283–7. doi: 10.1016/j.dsx.2020.03.016
10. Onder G, Rezza G, Brusaferro S. Case-fatality rate and characteristics of patients dying in relation to COVID-19 in Italy. JAMA. (2020) 323:1775–6. doi: 10.1001/jama.2020.4683
11. Richardson S, Hirsch JS, Narasimhan M, Crawford JM, McGinn T, Davidson KW, et al. Presenting characteristics, comorbidities, and outcomes among 5700 patients hospitalized with COVID-19 in the New York city area. JAMA. (2020) 323:2052–9. doi: 10.1001/jama.2020.6775
12. Vodnar D-C, Mitrea L, Teleky B-E, Szabo K, Cãlinoiu L-F, Nemeş S-A, et al. Coronavirus disease (COVID-19) caused by (SARS-CoV-2) infections: a real challenge for human gut microbiota. Front Cell Infect Microbiol. (2020) 10:575559. doi: 10.3389/fcimb.2020.575559
13. Lim S, Bae JH, Kwon HS, Nauck MA. COVID-19 and diabetes mellitus: from pathophysiology to clinical management. Nat Rev Endocrinol. (2021) 17:11–30. doi: 10.1038/s41574-020-00435-4
14. Corrao S, Pinelli K, Vacca M, Raspanti M, Argano C. Type 2 diabetes mellitus and COVID-19: a narrative review. Front Endocrinol (Lausanne). (2021) 12:609470. doi: 10.3389/fendo.2021.609470
15. Guo W, Li M, Dong Y, Zhou H, Zhang Z, Tian C, et al. Diabetes is a risk factor for the progression and prognosis of COVID-19. Diabetes Metab Res Rev. (2020) 36:e3319. doi: 10.1002/dmrr.3319
16. D’Amico F, Baumgart DC, Danese S, Peyrin-Biroulet L. Diarrhea during COVID-19 infection: pathogenesis, epidemiology, prevention, and management. Clin Gastroenterol Hepatol. (2020) 18:1663–72. doi: 10.1016/j.cgh.2020.04.001
17. Ruiz-Roso MB, Knott-Torcal C, Matilla-Escalante DC, Garcimartín A, Sampedro-Nuñez MA, Dávalos A, et al. COVID-19 lockdown and changes of the dietary pattern and physical activity habits in a cohort of patients with type 2 diabetes mellitus. Nutrients. (2020) 12:2327. doi: 10.3390/nu12082327
18. Upadhyay R, Robay A, Fakhro K, Khalil AA, Zirie M, Jayyousi A, et al. Role of SLMAP genetic variants in susceptibility of diabetes and diabetic retinopathy in Qatari population. J Transl Med. (2015) 13:61. doi: 10.1186/s12967-015-0411-6
19. Alyafei F, Soliman AT, Alkhalaf F, Sabt A, De Sanctis V, Elsayed N, et al. Prevalence of β-cell antibodies and associated autoimmune diseases in children and adolescents with type 1 diabetes (T1DM) versus type 2 diabetes (T2DM) in Qatar. Acta Biomed. (2018) 89:32–9. doi: 10.23750/abm.v89iS4.7359
20. Khandelwal D, Dutta D, Chittawar S, Kalra S. Sleep disorders in type 2 diabetes. Indian J Endocrinol Metab. (2017) 21:758–61.
21. American Diabetes Association. Evidence-based nutrition principles and recommendations for the treatment and prevention of diabetes and related complications. Diabetes Care. (2002) 25:202–12. doi: 10.2337/diacare.25.1.202
22. Paulweber B, Valensi P, Lindström J, Lalic NM, Greaves CJ, McKee M, et al. A European evidence-based guideline for the prevention of type 2 diabetes. Horm Metab Res. (2010) 42(Suppl. 1):S3–36. doi: 10.1055/s-0029-1240928
23. Zaccardi F, Webb DR, Yates T, Davies MJ. Pathophysiology of type 1 and type 2 diabetes mellitus: a 90-year perspective. Postgrad Med J. (2016) 92:63–9. doi: 10.1136/postgradmedj-2015-133281
24. Siegmann MJ, Athinarayanan SJ, Hallberg SJ, McKenzie AL, Bhanpuri NH, Campbell WW, et al. Improvement in patient-reported sleep in type 2 diabetes and prediabetes participants receiving a continuous care intervention with nutritional ketosis. Sleep Med. (2019) 55:92–9. doi: 10.1016/j.sleep.2018.12.014
25. Kim YA, Keogh JB, Clifton PM. Probiotics, prebiotics, synbiotics and insulin sensitivity. Nutr Res Rev. (2018) 31:35–51. doi: 10.1017/S095442241700018X
26. Al Theyab A, Almutairi T, Al-Suwaidi AM, Bendriss G, McVeigh C, Chaari A. Epigenetic effects of gut metabolites: exploring the path of dietary prevention of type 1 diabetes. Front Nutr. (2020) 7:563605. doi: 10.3389/fnut.2020.563605
27. Abdellatif B, McVeigh C, Bendriss G, Chaari A. The promising role of probiotics in managing the altered gut in autism spectrum disorders. Int J Mol Sci. (2020) 21:4159. doi: 10.3390/ijms21114159
28. Cabello-Olmo M, Araña M, Urtasun R, Encio IJ, Barajas M. Role of postbiotics in diabetes mellitus: current knowledge and future perspectives. Foods. (2021) 10:1590. doi: 10.3390/foods10071590
29. Chaari A, Bendriss G, Zakaria D, McVeigh C. Importance of dietary changes during the coronavirus pandemic: how to upgrade your immune response. Front public Heal. (2020) 8:476. doi: 10.3389/fpubh.2020.00476
30. Chaari A, Abdellatif B, Nabi F, Khan RH. Date palm (Phoenix dactylifera L.) fruit’s polyphenols as potential inhibitors for human amylin fibril formation and toxicity in type 2 diabetes. Int J Biol Macromol. (2020) 164:1794–808. doi: 10.1016/j.ijbiomac.2020.08.080
31. Kumar S, Paul P, Yadav P, Kaul R, Maitra SS, Jha SK, et al. A multi-targeted approach to identify potential flavonoids against three targets in the SARS-CoV-2 life cycle. Comput Biol Med. (2022) 142:105231. doi: 10.1016/j.compbiomed.2022.105231
32. Kaul R, Paul P, Kumar S, Büsselberg D, Dwivedi VD, Chaari A. Promising antiviral activities of natural flavonoids against SARS-CoV-2 targets: systematic review. Int J Mol Sci. (2021) 22:11069. doi: 10.3390/ijms222011069
33. Chaari A. Inhibition of human islet amyloid polypeptide aggregation and cellular toxicity by oleuropein and derivatives from olive oil. Int J Biol Macromol. (2020) 162:284–300. doi: 10.1016/j.ijbiomac.2020.06.170
34. EFSA Panel on Dietetic Products, Nutrition and Allergies (NDA). Guidance on the scientific requirements for health claims related to the immune system, the gastrointestinal tract and defence against pathogenic microorganisms. EFSA J. (2016) 14:4369.
35. Kootte RS, Vrieze A, Holleman F, Dallinga-Thie GM, Zoetendal EG, de Vos WM, et al. The therapeutic potential of manipulating gut microbiota in obesity and type 2 diabetes mellitus. Diabetes Obes Metab. (2012) 14:112–20. doi: 10.1111/j.1463-1326.2011.01483.x
36. Laswi I, Shafiq A, Al-Ali D, Burney Z, Pillai K, Salameh M, et al. A Comparative pilot study of bacterial and fungal dysbiosis in neurodevelopmental disorders and gastrointestinal disorders: commonalities, specificities and correlations with lifestyle. Microorganisms. (2021) 9:741. doi: 10.3390/microorganisms9040741
37. Bendriss G, Al-Ali D, Shafiq A, Laswi I, Mhaimeed N, Salameh M, et al. Targeting the gut microbiome: a brief report on the awareness, practice, and readiness to engage in clinical interventions in Qatar. Qatar Med J. (2020) 2020:47. doi: 10.5339/qmj.2020.47
38. Gonai M, Shigehisa A, Kigawa I, Kurasaki K, Chonan O, Matsuki T, et al. Galacto-oligosaccharides ameliorate dysbiotic Bifidobacteriaceae decline in Japanese patients with type 2 diabetes. Benef Microbes. (2017) 8:705–16. doi: 10.3920/BM2016.0230
39. Adeshirlarijaney A, Gewirtz AT. Considering gut microbiota in treatment of type 2 diabetes mellitus. Gut Microbes. (2020) 11:253–64. doi: 10.1080/19490976.2020.1717719
40. Al-Ali D, Ahmed A, Shafiq A, McVeigh C, Chaari A, Zakaria D, et al. Fecal microbiota transplants: a review of emerging clinical data on applications, efficacy, and risks (2015-2020). Qatar Med J. (2021) 2021:5. doi: 10.5339/qmj.2021.5
41. Birkeland E, Gharagozlian S, Birkeland KI, Valeur J, Måge I, Rud I, et al. Prebiotic effect of inulin-type fructans on faecal microbiota and short-chain fatty acids in type 2 diabetes: a randomised controlled trial. Eur J Nutr. (2020) 59:3325–38. doi: 10.1007/s00394-020-02282-5
42. Qin J, Li Y, Cai Z, Li S, Zhu J, Zhang F, et al. A metagenome-wide association study of gut microbiota in type 2 diabetes. Nature. (2012) 490:55–60. doi: 10.1038/nature11450
43. Larsen N, Vogensen FK, van den Berg FWJ, Nielsen DS, Andreasen AS, Pedersen BK, et al. Gut microbiota in human adults with type 2 diabetes differs from non-diabetic adults. PLoS One. (2010) 5:e9085. doi: 10.1371/journal.pone.0009085
44. Simon E, Cãlinoiu LF, Mitrea L, Vodnar DC. Probiotics, prebiotics, and synbiotics: implications and beneficial effects against irritable bowel syndrome. Nutrients. (2021) 13:2112. doi: 10.3390/nu13062112
45. Pickup JC. Inflammation and activated innate immunity in the pathogenesis of type 2 diabetes. Diabetes Care. (2004) 27:813–23. doi: 10.2337/diacare.27.3.813
46. Luc K, Schramm-Luc A, Guzik TJ, Mikolajczyk TP. Oxidative stress and inflammatory markers in prediabetes and diabetes. J Physiol Pharmacol. (2019) 70:809–24. doi: 10.26402/jpp.2019.6.01
47. Aliasgharzadeh A, Dehghan P, Gargari BP, Asghari-Jafarabadi M. Resistant dextrin, as a prebiotic, improves insulin resistance and inflammation in women with type 2 diabetes: a randomised controlled clinical trial. Br J Nutr. (2015) 113:321–30. doi: 10.1017/S0007114514003675
48. Maddux BA, See W, Lawrence JCJ, Goldfine AL, Goldfine ID, Evans JL. Protection against oxidative stress-induced insulin resistance in rat L6 muscle cells by mircomolar concentrations of alpha-lipoic acid. Diabetes. (2001) 50:404–10. doi: 10.2337/diabetes.50.2.404
49. Rudich A, Tirosh A, Potashnik R, Hemi R, Kanety H, Bashan N. Prolonged oxidative stress impairs insulin-induced GLUT4 translocation in 3T3-L1 adipocytes. Diabetes. (1998) 47:1562–9. doi: 10.2337/diabetes.47.10.1562
50. Rinninella E, Raoul P, Cintoni M, Franceschi F, Miggiano GA, Gasbarrini A, et al. What is the healthy gut microbiota composition↑ a changing ecosystem across age, environment, diet, and diseases. Microorganisms. (2019) 7:14. doi: 10.3390/microorganisms7010014
51. Frost F, Kacprowski T, Rühlemann M, Pietzner M, Bang C, Franke A, et al. Long-term instability of the intestinal microbiome is associated with metabolic liver disease, low microbiota diversity, diabetes mellitus and impaired exocrine pancreatic function. Gut. (2021) 70:522 LP–30. doi: 10.1136/gutjnl-2020-322753
52. Pickup JC, Mattock MB, Chusney GD, Butt D. NIDDM as a disease of the innate immune system: association of acute-phase reactants and interleukin-6 with metabolic syndrome X. Diabetologia. (1997) 40:1286–92. doi: 10.1007/s001250050822
53. Vozarova B, Stefan N, Lindsay RS, Saremi A, Pratley RE, Bogardus C, et al. High white blood cell count is associated with a worsening of insulin sensitivity and predicts the development of type 2 diabetes. Diabetes. (2002) 51:1889–95. doi: 10.2337/diabetes.51.2.455
54. Festa A, Agostino RD, Tracy RP, Haffner SM. Elevated levels of acute-phase proteins and plasminogen activator inhibitor-1 predict the development of type 2 diabetes. Diabetes. (2002) 51:1131–7. doi: 10.2337/diabetes.51.4.1131
55. Du Clos TW, Mold C. C-reactive protein: an activator of innate immunity and a modulator of adaptive immunity. Immunol Res. (2004) 30:261–77. doi: 10.1385/IR:30:3:261
56. Pradhan AD, Manson JE, Rifai N, Buring JE, Ridker PM. C-reactive protein, interleukin 6, and risk of developing type 2 diabetes mellitus. J Am Med Assoc. (2001) 286:327–34. doi: 10.1001/jama.286.3.327
57. Donath MY, Shoelson SE. Type 2 diabetes as an inflammatory disease. Nat Rev Immunol. (2011) 11:98–107.
58. Eun SK, Hyeong JK, Chul WA, Cheol WP, Bong SC, Sung KL, et al. Relationship of serum high sensitivity C-reactive protein to metabolic syndrome and microvascular complications in type 2 diabetes. Diabetes Res Clin Pract. (2005) 69:151–9. doi: 10.1016/j.diabres.2004.11.014
59. Akbari M, Hassan-Zadeh V. IL-6 signalling pathways and the development of type 2 diabetes. Inflammopharmacology. (2018) 26:685–98. doi: 10.1007/s10787-018-0458-0
60. Pedersen BK. IL-6 signalling in exercise and disease. Biochem Soc Trans. (2007) 35(Pt 5):1295–7. doi: 10.1042/BST0351295
61. Maedler K, Dharmadhikari G, Schumann DM, Størling J. Expert opinion on biological therapy interleukin-1 beta targeted therapy for type 2 diabetes interleukin-1 beta targeted therapy for type 2 diabetes. Expert Opin Biol Ther. (2009) 9:1177–88. doi: 10.1517/14712590903136688
62. Weksler-Zangen S, Raz I, Lenzen S, Jörns A, Ehrenfeld S, Amir G, et al. Impaired glucose-stimulated insulin secretion is coupled with exocrine pancreatic lesions in the cohen diabetic rat. Diabetes. (2008) 57:279–87. doi: 10.2337/db07-0520
63. Reinehr T, Roth CL. Inflammation markers in type 2 diabetes and the metabolic syndrome in the pediatric population. Curr Diabetes Rep. (2018) 18:131. doi: 10.1007/s11892-018-1110-5
64. Pacifico L, Di Renzo L, Anania C, Osborn JF, Ippoliti F, Schiavo E, et al. Increased T-helper interferon-γ-secreting cells in obese children. Eur J Endocrinol. (2006) 154:691–7. doi: 10.1530/eje.1.02138
65. Zhang H, Potter BJ, Cao JM, Zhang C. Interferon-gamma induced adipose tissue inflammation is linked to endothelial dysfunction in type 2 diabetic mice. Basic Res Cardiol. (2011) 106:1135–45. doi: 10.1007/s00395-011-0212-x
66. Atzeni F, Sarzi-Puttini P. Tumor necrosis factor. 2nd ed. In: Maloy S, Hughes K, editors. Brenner’s Encyclopedia of Genetics. New York, NY: Elsevier (2013). p. 229–31.
67. Akash MSH, Rehman K, Liaqat A. Tumor necrosis factor-alpha: role in development of insulin resistance and pathogenesis of type 2 diabetes mellitus. J Cell Biochem. (2018) 119:105–10. doi: 10.1002/jcb.26174
68. Alzamil H. Elevated serum TNF- α is related to obesity in type 2 diabetes mellitus and is associated with glycemic control and insulin resistance. J Obes. (2020) 2020:5076858. doi: 10.1155/2020/5076858
69. Gaffen SL. Recent advances in the IL-17 cytokine family. Curr Opin Immunol. (2011) 23:613–9. doi: 10.1016/j.coi.2011.07.006
70. Gaffen SL. Structure and signalling in the IL-17 receptor family. Nat Publ Gr. (2009) 9:556–67. doi: 10.1038/nri2586
71. Jagannathan-Bogdan M, McDonnell ME, Shin H, Rehman Q, Hasturk H, Apovian CM, et al. Elevated proinflammatory cytokine production by a skewed T cell compartment requires monocytes and promotes inflammation in type 2 diabetes. J Immunol. (2011) 186:1162–72. doi: 10.4049/jimmunol.1002615
72. Sumarac-Dumanovic M, Jeremic D, Pantovic A, Janjetovic K, Stamenkovic-Pejkovic D, Cvijovic G, et al. Therapeutic improvement of glucoregulation in newly diagnosed type 2 diabetes patients is associated with a reduction of IL-17 levels. Immunobiology. (2013) 218:1113–8. doi: 10.1016/j.imbio.2013.03.002
74. Cimini FA, Barchetta I, Porzia A, Mainiero F, Costantino C, Bertoccini L, et al. Circulating IL-8 levels are increased in patients with type 2 diabetes and associated with worse inflammatory and cardiometabolic profile. Acta Diabetol. (2017) 54:961–7. doi: 10.1007/s00592-017-1039-1
75. Ryba-Stanisławowska M, Rybarczyk-Kapturska K, Myśliwiec M, Myśliwska J. Elevated levels of serum IL-12 and IL-18 are associated with lower frequencies of CD4+CD25highFOXP3+ regulatory T cells in young patients with type 1 diabetes. Inflammation. (2014) 37:1513–20. doi: 10.1007/s10753-014-9878-1
76. Trembleau S, Penna G, Gregori S, Giarratana N, Adorini L. IL-12 administration accelerates autoimmune diabetes in both wild-type and IFN-γ-deficient nonobese diabetic mice, revealing pathogenic and protective effects of IL-12-induced IFN-γ. J Immunol. (2003) 170:5491–501. doi: 10.4049/jimmunol.170.11.5491
77. Wegner M, Winiarska H, Bobkiewicz-Kozłowska T, Dworacka M. IL-12 serum levels in patients with type 2 diabetes treated with sulphonylureas. Cytokine. (2008) 42:312–6. doi: 10.1016/j.cyto.2008.02.014
78. Shoelson SE, Lee J, Goldfine AB. Inflammation and insulin resistance. J Clin Invest. (2006) 116:1793–801.
79. Cox AJ, Zhang P, Bowden DW, Devereaux B, Davoren PM, Cripps AW, et al. Increased intestinal permeability as a risk factor for type 2 diabetes. Diabetes Metab. (2017) 43:163–6. doi: 10.1016/j.diabet.2016.09.004
80. Kir S, Ekiz K, Alacam H, Turkel R, Koroglu E, Altintop BL. The association between pro and anti-inflammatory markers with the components of metabolic syndrome. Acta Endocrinol (Copenh). (2019) 15:430–5. doi: 10.4183/aeb.2019.430
81. Barry JC, Shakibakho S, Durrer C, Simtchouk S, Jawanda KK, Cheung ST, et al. Hyporesponsiveness to the anti-inflammatory action of interleukin-10 in type 2 diabetes OPEN. Sci Rep. (2016) 6:21244. doi: 10.1038/srep21244
82. Moore KW, Malefyt RDW, Robert L, Garra AO. Interleukin –10 and the interleukin –10 receptor. Mol Cell Biol. (2001) 1:683–765. doi: 10.1146/annurev.immunol.19.1.683
83. Van Exel E, Gussekloo J, De Craen AJM, Frölich M, Van Der Wiel AB, Westendorp RGJ. Low production capacity of interleukin-10 associates with the metabolic syndrome and type 2 diabetes: the Leiden 85-plus study. Diabetes. (2002) 51:1088–92. doi: 10.2337/diabetes.51.4.1088
84. Jakus V. The role of free radicals, oxidative stress and antioxidant systems in diabetic vascular disease. Bratisl Lek List. (2000) 101:541–51.
85. Aouacheri O, Saka S, Krim M, Messaadia A, Maidi I. The investigation of the oxidative stress-related parameters in type2 diabetes mellitus. Can J Diabetes. (2015) 39:44–9. doi: 10.1016/j.jep.2019.112038
86. Dworzañski J, Strycharz-Dudziak M, Kliszczewska E, Kiełczykowska M, Dworzañska A, Drop B, et al. Glutathione peroxidase (GPx) and superoxide dismutase (SOD) activity in patients with diabetes mellitus type 2 infected with Epstein-Barr virus. PLoS One. (2020) 15:e0230374. doi: 10.1371/journal.pone.0230374
87. Maxwell SRJ. Prospects for the use of antioxidant therapies. Drugs. (1995) 49:345–61. doi: 10.2165/00003495-199549030-00003
88. Southerland JH, Taylor GW, Moss K, Beck JD, Offenbacher S. Commonality in chronic inflammatory diseases: periodontitis, diabetes, and coronary artery disease. Periodontology 2000. (2006) 40:130–43. doi: 10.1111/j.1600-0757.2005.00138.x
89. Fujita H, Fujishima H, Chida S, Takahashi K, Qi Z, Kanetsuna Y, et al. Reduction of renal superoxide dismutase in progressive diabetic nephropathy. J Am Soc Nephrol. (2009) 20:1303–13. doi: 10.1681/ASN.2008080844
90. Bandeira SDM, Da G, Guedes S, José L, Da Fonseca S, Pires AS, et al. Clinical study characterization of blood oxidative stress in type 2 diabetes mellitus patients: increase in lipid peroxidation and SOD activity. Oxid Med Cell Longev. (2012) 2012:819310. doi: 10.1155/2012/819310
91. Ngoye Briggs O, Brown H, Elechi-amadi K, Ezeiruaku F, Nduka N. Superoxide dismutase and glutathione peroxidase levels in patients with long standing type 2 diabetes in Port Harcourt, Rivers State, Nigeria. Int J Sci Res. (2016) 5:1282–8. doi: 10.21275/v4i5.nov162149
92. Gaetani GF, Ferraris AM, Rolfo M, Mangerini R, Arena S, Kirkman HN. Predominant role of catalase in the disposal of hydrogen peroxide within human erythrocytes. Blood. (1996) 87:1595–9. doi: 10.1182/blood.v87.4.1595.bloodjournal8741595
93. Ahmed FN, Naqvi FN, Shafiq F. Lipid peroxidation and serum antioxidant enzymes in patients with type 2 diabetes mellitus. Ann N Y Acad Sci. (2006) 1084:481–9. doi: 10.1196/annals.1372.022
94. Góth L. Catalase deficiency and type 2 diabetes. Diabetes Care. (2008) 31:e93. doi: 10.2337/dc08-1607
95. Baynes JW. Role of oxidative stress in development of complications in diabetes. Diabetes. (1991) 40:405–12. doi: 10.2337/diabetes.40.4.405
96. Majidi Z, Hosseinkhani S, Amiri-Dashatan N, Emamgholipour S, Tutunchi S, Hashemi J, et al. Effect of rosiglitazone on circulating malondialdehyde (MDA) level in diabetes based on a systematic review and meta-analysis of eight clinical trials. J Investig Med Off Publ Am Fed Clin Res. (2021) 69:697–703. doi: 10.1136/jim-2020-001588
97. Mahreen R, Mohsin M, Nasreen Z, Siraj M, Ishaq M. Significantly increased levels of serum malonaldehyde in type 2 diabetics with myocardial infarction. Int J Diabetes Dev Ctries. (2010) 30:49. doi: 10.4103/0973-3930.60006
98. Pitocco D, Zaccardi F, Di Stasio E, Romitelli F, Santini SA, Zuppi C, et al. Oxidative stress, nitric oxide, and diabetes. Rev Diabet Stud. (2010) 7:15–25. doi: 10.1900/RDS.2010.7.15
99. Apakkan Aksun S, Özmen B, Özmen D, Parildar Z, Şenol B, Habif S, et al. Serum and urinary nitric oxide in type 2 diabetes with or without microalbuminuria: relation to glomerular hyperfiltration. J Diabetes Complications. (2003) 17:343–8. doi: 10.1016/s1056-8727(02)00196-4
100. Adela R, Nethi SK, Bagul PK, Barui AK, Mattapally S, Kuncha M, et al. Hyperglycaemia enhances nitric oxide production in diabetes: a study from South Indian patients. PLoS One. (2015) 10:e0125270. doi: 10.1371/journal.pone.0125270
101. Maejima K, Nakano S, Himeno M, Tsuda SI, Makiishi H, Ito T, et al. Increased basal levels of plasma nitric oxide in type 2 diabetic subjects: relationship to microvascular complications. J Diabetes Complications. (2001) 15:135–43. doi: 10.1016/s1056-8727(01)00144-1
102. Assmann TS, Brondani LA, Bouças AP, Rheinheimer J, de Souza BM, Canani LH, et al. Nitric oxide levels in patients with diabetes mellitus: a systematic review and meta-analysis. Nitric Oxide. (2016) 61:1–9. doi: 10.1016/j.niox.2016.09.009
103. Pal R, Dahal S, Ghosh A, Sherpa M, Bhutia Y. Serum nitric oxide status in patients with type 2 diabetes mellitus in Sikkim. Int J Appl Basic Med Res. (2011) 1:31. doi: 10.4103/2229-516X.81977
105. Kaplowitz N, Aw TY, Ookhtens M. The regulation of hepatic glutathione. Annu Rev Pharmacol Toxicol. (1985) 25:715–44.
106. Tsai CJ, Hsieh CJ, Tung SC, Kuo MC, Shen FC. Acute blood glucose fluctuations can decrease blood glutathione and adiponectin levels in patients with type 2 diabetes. Diabetes Res Clin Pract. (2012) 98:257–63. doi: 10.1016/j.diabres.2012.09.013
107. Das J, Vasan V, Sil PC. Taurine exerts hypoglycemic effect in alloxan-induced diabetic rats, improves insulin-mediated glucose transport signaling pathway in heart and ameliorates cardiac oxidative stress and apoptosis. Toxicol Appl Pharmacol. (2012) 258:296–308. doi: 10.1016/j.taap.2011.11.009
108. Calabrese V, Cornelius C, Leso V, Trovato-Salinaro A, Ventimiglia B, Cavallaro M, et al. Oxidative stress, glutathione status, sirtuin and cellular stress response in type 2 diabetes. Biochim Biophys Acta Mol Basis Dis. (2012) 1822:729–36. doi: 10.1016/j.bbadis.2011.12.003
109. Frostegård J, Zhang Y, Sun J, Yan K, Liu A. Oxidized low-density lipoprotein (OxLDL)-treated dendritic cells promote activation of T cells in human atherosclerotic plaque and blood, which is repressed by statins: microRNA let-7c is integral to the effect. J Am Heart Assoc. (2016) 5:1–14. doi: 10.1161/JAHA.116.003976
110. Njajou OT, Kanaya AM, Holvoet P, Connelly S, Strotmeyer ES, Harris TB, et al. Association between oxidized LDL, obesity and type 2 diabetes in a population-based cohort, the health, aging and body composition study. Diabetes Metab Res Rev. (2009) 25:733–9. doi: 10.1002/dmrr.1011
111. Rösen P, Nawroth PP, King G, Möller W, Tritschler HJ, Packer L. The role of oxidative stress in the onset and progression of diabetes and its complications: a summary of a congress series sponsored by UNESCO-MCBN, the American diabetes association and the German diabetes society. Diabetes Metab Res Rev. (2001) 17:189–212. doi: 10.1002/dmrr.196
112. Morrow JD, Harris TM, Jackson Roberts L. Noncyclooxygenase oxidative formation of a series of novel prostaglandins: analytical ramifications for measurement of eicosanoids. Anal Biochem. (1990) 184:1–10. doi: 10.1016/0003-2697(90)90002-q
113. Kaviarasan S, Muniandy S, Qvist R, Ismail IS. F2-isoprostanes as novel biomarkers for type 2 diabetes: a review. J Clin Biochem Nutr. (2009) 45:1–8. doi: 10.3164/jcbn.08-266
114. Dizdaroglu M. Facts about the artifacts in the measurement of oxidative DNA base damage by gas chromatography-mass spectrometry. Free Rad Res. (2009) 29:551–63. doi: 10.1080/10715769800300591
115. Maritim AC, Sanders RA, Watkins JB. Diabetes, oxidative stress, and antioxidants: a review. J Biochem Mol Toxicol. (2003) 17:24–38.
116. Shigenaga MK, Ames BN. Assays for 8-hydroxy-2’-deoxyguanosine: a biomarker of in vivo oxidative DNA damage. Free Radic Biol Med. (1991) 10:211–6. doi: 10.1016/0891-5849(91)90078-h
117. Serdar M, Sertoglu E, Uyanik M, Tapan S, Akin K, Bilgi C, et al. Comparison of 8-hydroxy-2’-deoxyguanosine (8-OHdG) levels using mass spectrometer and urine albumin creatinine ratio as a predictor of development of diabetic nephropathy. Free Radic Res. (2012) 46:1291–5. doi: 10.3109/10715762.2012.710902
118. Young IS. Measurement of total antioxidant capacity. J Clin Pathol. (2001) 54:339. doi: 10.1136/jcp.54.5.339
119. Valkonen M, Kuusi T. Spectrophotometric assay for total peroxyl radical-trapping antioxidant potential in human serum. J Lipid Res. (1997) 38:823–33. doi: 10.1016/s0022-2275(20)37249-7
120. Çapaş M, Kaner G, Soylu M, Ýnanç N, Başmısırlı E. The relationship between plasma total antioxidant capacity and dietary antioxidant status in adults with type 2 diabetes. Prog Nutr. (2018) 20:67–75. doi: 10.3945/jn.114.205674
121. Jamuna Rani A, Mythili SV. Study on total antioxidant status in relation to oxidative stress in type 2 diabetes mellitus. J Clin Diagnostic Res. (2014) 8:108–10. doi: 10.7860/JCDR/2014/7603.4121
122. Page MJ, Moher D, Bossuyt PM, Boutron I, Hoffmann TC, Mulrow CD, et al. PRISMA 2020 explanation and elaboration: updated guidance and exemplars for reporting systematic reviews. BMJ. (2021) 372:160. doi: 10.1136/bmj.n160
123. Sabico S, Al-Mashharawi A, Al-Daghri NM, Wani K, Amer OE, Hussain DS, et al. Effects of a 6-month multi-strain probiotics supplementation in endotoxemic, inflammatory and cardiometabolic status of T2DM patients: a randomized, double-blind, placebo-controlled trial. Clin Nutr. (2019) 38:1561–9. doi: 10.1016/j.clnu.2018.08.009
124. Asemi Z, Zare Z, Shakeri H, Sabihi SS, Esmaillzadeh A. Effect of multispecies probiotic supplements on metabolic profiles, hs-CRP, and oxidative stress in patients with type 2 diabetes. Ann Nutr Metab. (2013) 63:1–9. doi: 10.1159/000349922
125. Raygan F, Ostadmohammadi V, Asemi Z. The effects of probiotic and selenium co-supplementation on mental health parameters and metabolic profiles in type 2 diabetic patients with coronary heart disease: a randomized, double-blind, placebo-controlled trial. Clin Nutr. (2019) 38:1594–8. doi: 10.1016/j.clnu.2018.07.017
126. Raygan F, Rezavandi Z, Bahmani F, Ostadmohammadi V, Mansournia MA, Tajabadi-Ebrahimi M, et al. The effects of probiotic supplementation on metabolic status in type 2 diabetic patients with coronary heart disease IRCT2017082733941N5 IRCT. Diabetol Metab Syndr. (2018) 10:1–7. doi: 10.1186/s13098-018-0353-2
127. Raygan F, Ostadmohammadi V, Bahmani F, Asemi Z. The effects of vitamin D and probiotic co-supplementation on mental health parameters and metabolic status in type 2 diabetic patients with coronary heart disease: a randomized, double-blind, placebo-controlled trial. Prog Neuro Psychopharmacol Biol Psychiatry. (2018) 84:50–5. doi: 10.1016/j.pnpbp.2018.02.007
128. Mazloom Z, Yousefinejad A, Dabbaghmanesh MH. Effect of probiotics on lipid profile, glycemic control, insulin action, oxidative stress, and inflammatory markers in patients with type 2 diabetes: a clinical trial. Iran J Med Sci. (2013) 38:38–43.
129. Mohamadshahi M, Veissi M, Haidari F, Shahbazian H, Kaydani GA, Mohammadi F. Effects of probiotic yogurt consumption on inflammatory biomarkers in patients with type 2 diabetes. Bioimpacts. (2014) 4:83–8. doi: 10.5681/bi.2014.007
130. Firouzi S, Majid HA, Ismail A, Kamaruddin NA, Barakatun-Nisak MY. Effect of multi-strain probiotics (multi-strain microbial cell preparation) on glycemic control and other diabetes-related outcomes in people with type 2 diabetes: a randomized controlled trial. Eur J Nutr. (2017) 56:1535–50. doi: 10.1007/s00394-016-1199-8
131. Bayat A, Azizi-Soleiman F, Heidari-Beni M, Feizi A, Iraj B, Ghiasvand R, et al. Effect of cucurbita ficifolia and probiotic yogurt consumption on blood glucose, lipid profile, and inflammatory marker in type 2 diabetes. Int J Prev Med. (2016) 2016:30. doi: 10.4103/2008-7802.175455
132. Andreasen AS, Larsen N, Pedersen-Skovsgaard T, Berg RMG, Mller K, Svendsen KD, et al. Effects of Lactobacillus acidophilus NCFM on insulin sensitivity and the systemic inflammatory response in human subjects. Br J Nutr. (2010) 104:1831–8. doi: 10.1017/S0007114510002874
133. Feizollahzadeh S, Ghiasvand R, Rezaei A, Khanahmad H, Sadeghi A, Hariri M. Effect of probiotic soy milk on serum levels of adiponectin, inflammatory mediators, lipid profile, and fasting blood glucose among patients with type II diabetes mellitus. Probiotics Antimicrob Proteins. (2017) 9:41–7. doi: 10.1007/s12602-016-9233-y
134. Hsieh MC, Tsai WH, Jheng YP, Su SL, Wang SY, Lin CC, et al. The beneficial effects of Limosilactobacillus reuteri ADR-1 or ADR-3 consumption on type 2 diabetes mellitus: a randomized, double-blinded, placebo-controlled trial. Sci Rep. (2018) 8:16791.
135. Sato J, Kanazawa A, Azuma K, Ikeda F, Goto H, Komiya K, et al. Probiotic reduces bacterial translocation in type 2 diabetes mellitus: a randomised controlled study. Sci Rep. (2017) 7:1–10. doi: 10.1038/s41598-017-12535-9
136. Kobyliak N, Falalyeyeva T, Mykhalchyshyn G, Kyriienko D, Komissarenko I. Effect of alive probiotic on insulin resistance in type 2 diabetes patients: randomized clinical trial. Diabetes Metab Syndr Clin Res Rev. (2018) 12:617–24. doi: 10.1016/j.dsx.2018.04.015
137. Kobyliak N, Abenavoli L, Falalyeyeva T, Kovalchuk O, Kyriienko D, Komisarenko I. Metabolic benefits of probiotic combination with absorbent smectite in type 2 diabetes patients a randomised controlled trial. Rev Recent Clin Trials. (2021) 16:109–19. doi: 10.2174/1574887115666200709141131
138. Kobyliak N, Falalyeyeva T, Mykhalchyshyn G, Molochek N, Savchuk O, Kyriienko D, et al. Probiotic and omega-3 polyunsaturated fatty acids supplementation reduces insulin resistance, improves glycemia and obesity parameters in individuals with type 2 diabetes: a randomised controlled trial. Obes Med. (2020) 19:100248. doi: 10.1016/j.obmed.2020.100248
139. Kobyliak N, Abenavoli L, Mykhalchyshyn G, Falalyeyeva T, Tsyryuk O, Kononenko L, et al. Probiotics and smectite absorbent gel formulation reduce liver stiffness, transaminase and cytokine levels in NAFLD associated with type 2 diabetes: a randomized clinical study. Clin Diabetol. (2019) 8:205–14. doi: 10.5603/dk.2019.0016
140. Sabico S, Al-Mashharawi A, Al-Daghri NM, Yakout S, Alnaami AM, Alokail MS, et al. Effects of a multi-strain probiotic supplement for 12 weeks in circulating endotoxin levels and cardiometabolic profiles of medication naïve T2DM patients: a randomized clinical trial. J Transl Med. (2017) 15:1–9. doi: 10.1186/s12967-017-1354-x
141. Miraghajani M, Zaghian N, Mirlohi M, Feizi A, Ghiasvand R. The impact of probiotic soy milk consumption on oxidative stress among type 2 diabetic kidney disease patients: a randomized controlled clinical trial. J Ren Nutr. (2017) 27:317–24. doi: 10.1053/j.jrn.2017.04.004
142. Bahmani F, Tajadadi-Ebrahimi M, Kolahdooz F, Mazouchi M, Hadaegh H, Jamal AS, et al. The consumption of synbiotic bread containing Lactobacillus sporogenes and inulin affects nitric oxide and malondialdehyde in patients with type 2 diabetes mellitus: randomized, double-blind, placebo-controlled trial. J Am Coll Nutr. (2016) 35:506–13. doi: 10.1080/07315724.2015.1032443
143. Ejtahed HS, Mohtadi-Nia J, Homayouni-Rad A, Niafar M, Asghari-Jafarabadi M, Mofid V. Probiotic yogurt improves antioxidant status in type 2 diabetic patients. Nutrition. (2012) 28:539–43. doi: 10.1016/j.nut.2011.08.013
144. Mirmiranpour H, Huseini HF, Derakhshanian H, Khodaii Z, Tavakoli-Far B. Effects of probiotic, cinnamon, and synbiotic supplementation on glycemic control and antioxidant status in people with type 2 diabetes; a randomized, double-blind, placebo-controlled study. J Diabetes Metab Disord. (2020) 19:53–60. doi: 10.1007/s40200-019-00474-3
145. Hariri M, Salehi R, Feizi A, Mirlohi M, Ghiasvand R, Habibi N. A randomized, double-blind, placebo-controlled, clinical trial on probiotic soy milk and soy milk: effects on epigenetics and oxidative stress in patients with type II diabetes. Genes Nutr. (2015) 10:1–8. doi: 10.1007/s12263-015-0503-1
146. Tonucci LB, Olbrich dos Santos KM, Licursi de Oliveira L, Rocha Ribeiro SM, Duarte Martino HS. Clinical application of probiotics in type 2 diabetes mellitus: a randomized, double-blind, placebo-controlled study. Clin Nutr. (2017) 36:85–92. doi: 10.1016/j.clnu.2015.11.011
147. Dehghan P, Gargari BP, Jafar-Abadi MA, Aliasgharzadeh A. Inulin controls inflammation and metabolic endotoxemia in women with type 2 diabetes mellitus: a randomized-controlled clinical trial. Int J Food Sci Nutr. (2014) 65:117–23. doi: 10.3109/09637486.2013.836738
148. Roshanravan N, Mahdavi R, Alizadeh E, Ghavami A, Saadat YR, Alamdari NM, et al. The effects of sodium butyrate and inulin supplementation on angiotensin signaling pathway via promotion of Akkermansia muciniphila abundance in type 2 diabetes; a randomized, double-blind, placebo-controlled trial. J Cardiovasc Thorac Res. (2017) 9:183. doi: 10.15171/jcvtr.2017.32
149. Farhangi MA, Dehghan P, Namazi N. Prebiotic supplementation modulates advanced glycation end-products (AGEs), soluble receptor for AGEs (sRAGE), and cardiometabolic risk factors through improving metabolic endotoxemia: a randomized-controlled clinical trial. Eur J Nutr. (2020) 59:3009–21. doi: 10.1007/s00394-019-02140-z
150. Dehghan P, Pourghassem Gargari B, Asghari Jafar-abadi M. Oligofructose-enriched inulin improves some inflammatory markers and metabolic endotoxemia in women with type 2 diabetes mellitus: a randomized controlled clinical trial. Nutrition. (2014) 30:418–23. doi: 10.1016/j.nut.2013.09.005
151. Gargari BP, Namazi N, Khalili M, Sarmadi B, Jafarabadi MA, Dehghan P. Is there any place for resistant starch, as alimentary prebiotic, for patients with type 2 diabetes↑ Complement Ther Med. (2015) 23:810–5. doi: 10.1016/j.ctim.2015.09.005
152. Dehghan P, Farhangi MA, Tavakoli F, Aliasgarzadeh A, Akbari AM. Impact of prebiotic supplementation on T-cell subsets and their related cytokines, anthropometric features and blood pressure in patients with type 2 diabetes mellitus: a randomized placebo-controlled trial. Complement Ther Med. (2016) 24:96–102. doi: 10.1016/j.ctim.2015.12.010
153. Farhangi MA, Javid AZ, Sarmadi B, Karimi P, Dehghan P. A randomized controlled trial on the efficacy of resistant dextrin, as functional food, in women with type 2 diabetes: targeting the hypothalamic–pituitary–adrenal axis and immune system. Clin Nutr. (2018) 37:1216–23. doi: 10.1016/j.clnu.2017.06.005
154. Aliasgharzadeh A, Khalili M, Mirtaheri E, Gargari BP, Tavakoli F, Farhangi MA, et al. A combination of prebiotic inulin and oligofructose improve some of cardiovascular disease risk factors in women with type 2 diabetes: a randomized controlled clinical trial. Adv Pharm Bull. (2015) 5:507. doi: 10.15171/apb.2015.069
155. Ahn HY, Kim M, Seo CR, Yoo HJ, Lee SH, Lee JH. The effects of Jerusalem artichoke and fermented soybean powder mixture supplementation on blood glucose and oxidative stress in subjects with prediabetes or newly diagnosed type 2 diabetes. Nutr Diabetes. (2018) 8:1–13. doi: 10.1038/s41387-018-0052-y
156. Asemi Z, Khorrami-Rad A, Alizadeh SA, Shakeri H, Esmaillzadeh A. Effects of synbiotic food consumption on metabolic status of diabetic patients: a double-blind randomized cross-over controlled clinical trial. Clin Nutr. (2014) 33:198–203. doi: 10.1016/j.clnu.2013.05.015
157. Ghafouri A, Zarrati M, Shidfar F, Heydari I, Shokouhi Shoormasti R, Eslami O. Effect of synbiotic bread containing lactic acid on glycemic indicators, biomarkers of antioxidant status and inflammation in patients with type 2 diabetes: a randomized controlled trial. Diabetol Metab Syndr. (2019) 11:1–9. doi: 10.1186/s13098-019-0496-9
158. Asemi Z, Alizadeh SA, Ahmad K, Goli M, Esmaillzadeh A. Effects of beta-carotene fortified synbiotic food on metabolic control of patients with type 2 diabetes mellitus: a double-blind randomized cross-over controlled clinical trial. Clin Nutr. (2016) 35:819–25. doi: 10.1016/j.clnu.2015.07.009
159. Tajadadi-Ebrahimi M, Bahmani F, Shakeri H, Hadaegh H, Hijijafari M, Abedi F, et al. Effects of daily consumption of synbiotic bread on insulin metabolism and serum high-sensitivity c-reactive protein among diabetic patients: a double-blind, randomized, controlled clinical trial. Ann Nutr Metab. (2014) 65:34–41. doi: 10.1159/000365153
160. Kanazawa A, Aida M, Yoshida Y, Kaga H, Katahira T, Suzuki L, et al. Effects of synbiotic supplementation on chronic inflammation and the gut microbiota in obese patients with type 2 diabetes mellitus: a randomized controlled study. Nutrients. (2021) 13:558. doi: 10.3390/nu13020558
161. Farrokhian A, Raygan F, Soltani A, Tajabadi-Ebrahimi M, Sharifi Esfahani M, Karami AA, et al. The effects of synbiotic supplementation on carotid intima-media thickness, biomarkers of inflammation, and oxidative stress in people with overweight, diabetes, and coronary heart disease: a randomized, double-blind, placebo-controlled trial. Probiotics Antimicrob Proteins. (2019) 11:133–42. doi: 10.1007/s12602-017-9343-1
162. Velayati A, Kareem I, Sedaghat M, Sohrab G, Nikpayam O, Hedayati M, et al. Does symbiotic supplementation which contains Bacillus coagulans Lacticaseibacillus rhamnosus, Lactobacillus acidophilus and fructooligosaccharide has favourite effects in patients with type-2 diabetes↑ A randomised, double-blind, placebo-controlled trial. Arch Physiol Biochem. (2021). doi: 10.1080/13813455.2021.1928225 [Epub ahead of print].
163. Akram Kooshki A, Tofighiyan T, Rakhshani MH. Effects of synbiotics on inflammatory markers in patients with type 2 diabetes mellitus. Glob J Health Sci. (2015) 7:1. doi: 10.5539/gjhs.v7n7p1
164. Bazyar H, Maghsoumi-Norouzabad L, Yarahmadi M, Gholinezhad H, Moradi L, Salehi P, et al. The impacts of synbiotic supplementation on periodontal indices and biomarkers of oxidative stress in type 2 diabetes mellitus patients with chronic periodontitis under non-surgical periodontal therapy. A double-blind, placebo-controlled trial. Diabetes Metab Syndr Obes Targets Ther. (2020) 13:19–29. doi: 10.2147/DMSO.S230060
165. Horvath A, Leber B, Feldbacher N, Tripolt N, Rainer F, Blesl A, et al. Effects of a multispecies synbiotic on glucose metabolism, lipid marker, gut microbiome composition, gut permeability, and quality of life in diabesity: a randomized, double-blind, placebo-controlled pilot study. Eur J Nutr. (2020) 59:2969–83. doi: 10.1007/s00394-019-02135-w
166. Fuller R. Probiotics in man and animals. J Appl Bacteriol. (1989) 66:365–78. doi: 10.1111/j.1365-2672.1989.tb05105.x
167. Nakamura YK, Omaye ST. Metabolic diseases and pro- and prebiotics: mechanistic insights. Nutr Metab. (2012) 9:1–9. doi: 10.1186/1743-7075-9-60
168. Björkstén B. Evidence of probiotics in prevention of allergy and asthma. Curr Drug Targets Inflamm Allergy. (2005) 4:599–604. doi: 10.2174/156801005774322135
169. Rautava S, Kalliomäki M, Isolauri E. Probiotics during pregnancy and breast-feeding might confer immunomodulatory protection against atopic disease in the infant. J Allergy Clin Immunol. (2002) 109:119–21. doi: 10.1067/mai.2002.120273
170. Parracho H, McCartney AL, Gibson GR. Probiotics and prebiotics in infant nutrition. Proc Nutr Soc. (2007) 66:405–11.
171. Bäckhed F, Manchester JK, Semenkovich CF, Gordon JI. Mechanisms underlying the resistance to diet-induced obesity in germ-free mice. Proc Natl Acad Sci USA. (2007) 104:979–84. doi: 10.1073/pnas.0605374104
172. Gurung M, Li Z, You H, Rodrigues R, Jump DB, Morgun A, et al. Role of gut microbiota in type 2 diabetes pathophysiology. EBioMedicine. (2020) 51:102590. doi: 10.1016/j.ebiom.2019.11.051
173. Moya-Pérez A, Neef A, Sanz Y. Bifidobacterium pseudocatenulatum CECT 7765 reduces obesity-associated inflammation by restoring the lymphocyte-macrophage balance and gut microbiota structure in high-fat diet-fed mice. PLoS One. (2015) 10:e0126976. doi: 10.1371/journal.pone.0126976
174. Kikuchi K, Ben Othman M, Sakamoto K. Sterilized bifidobacteria suppressed fat accumulation and blood glucose level. Biochem Biophys Res Commun. (2018) 501:1041–7. doi: 10.1016/j.bbrc.2018.05.105
175. Le TKC, Hosaka T, Nguyen TT, Kassu A, Dang TO, Tran HB, et al. Bifidobacterium species lower serum glucose, increase expressions of insulin signaling proteins, and improve adipokine profile in diabetic mice. Biomed Res. (2015) 36:63–70. doi: 10.2220/biomedres.36.63
176. Aoki R, Kamikado K, Suda W, Takii H, Mikami Y, Suganuma N, et al. A proliferative probiotic Bifidobacterium strain in the gut ameliorates progression of metabolic disorders via microbiota modulation and acetate elevation. Sci Rep. (2017) 7:43522. doi: 10.1038/srep43522
177. Wang G, Si Q, Yang S, Jiao T, Zhu H, Tian P, et al. Lactic acid bacteria reduce diabetes symptoms in mice by alleviating gut microbiota dysbiosis and inflammation in different manners. Food Funct. (2020) 11:5898–914. doi: 10.1039/c9fo02761k
178. Tajabadi-Ebrahimi M, Sharifi N, Farrokhian A, Raygan F, Karamali F, Razzaghi R, et al. A randomized controlled clinical trial investigating the effect of synbiotic administration on markers of insulin metabolism and lipid profiles in overweight type 2 diabetic patients with coronary heart disease. Exp Clin Endocrinol Diabetes. (2017) 125:21–7. doi: 10.1055/s-0042-105441
179. Timmerman HM, Koning CJM, Mulder L, Rombouts FM, Beynen AC. Monostrain, multistrain and multispecies probiotics–a comparison of functionality and efficacy. Int J Food Microbiol. (2004) 96:219–33. doi: 10.1016/j.ijfoodmicro.2004.05.012
180. Bordalo Tonucci L, Dos Santos KMO, De Luces Fortes Ferreira CL, Ribeiro SMR, De Oliveira LL, Martino HSD. Gut microbiota and probiotics: focus on diabetes mellitus. Crit Rev Food Sci Nutr. (2017) 57:2296–309. doi: 10.1080/10408398.2014.934438
181. Wang Y, Wu Y, Wang Y, Xu H, Mei X, Yu D, et al. Antioxidant properties of probiotic bacteria. Nutr. (2017) 9:521. doi: 10.3390/nu9050521
182. Wang X, Bao W, Liu J, Ouyang Y-Y, Wang D, Rong S, et al. Inflammatory markers and risk of type 2 diabetes: a systematic review and meta-analysis. Diabetes Care. (2013) 36:166–75. doi: 10.2337/dc12-0702
183. Lin MY, Chang FJ. Antioxidative effect of intestinal bacteria Bifidobacterium longum ATCC 15708 and Lactobacillus acidophilus ATCC 4356. Dig Dis Sci. (2000) 45:1617–22. doi: 10.1023/a:1005577330695
184. Sabeena Farvin KH, Baron CP, Nielsen NS, Otte J, Jacobsen C. Antioxidant activity of yoghurt peptides: part 2 – characterisation of peptide fractions. Food Chem. (2010) 123:1090–7. doi: 10.1016/j.foodchem.2010.05.029
185. Hsieh P-S, Ho H-H, Tsao SP, Hsieh S-H, Lin W-Y, Chen J-F, et al. Multi-strain probiotic supplement attenuates streptozotocin-induced type-2 diabetes by reducing inflammation and β-cell death in rats. PLoS One. (2021) 16:e0251646. doi: 10.1371/journal.pone.0251646
186. Kajimoto Y, Kaneto H. Role of oxidative stress in pancreatic beta-cell dysfunction. Ann N Y Acad Sci. (2004) 1011:168–76.
187. Wang X, Yang J, Qiu X, Wen Q, Liu M, Zhou D, et al. Probiotics, pre-biotics and synbiotics in the treatment of pre-diabetes: a systematic review of randomized controlled trials. Front Public Health. (2021) 9:645035. doi: 10.3389/fpubh.2021.645035
188. Colantonio AG, Werner SL, Brown M. The effects of prebiotics and substances with prebiotic properties on metabolic and inflammatory biomarkers in individuals with type 2 diabetes mellitus: a systematic review. J Acad Nutr Diet. (2020) 120:587–607.e2. doi: 10.1016/j.jand.2018.12.013
189. Gibson GR, Probert HM, Van Loo J, Rastall RA, Roberfroid MB. Dietary modulation of the human colonic microbiota: updating the concept of prebiotics. Nutr Res Rev. (2004) 17:259–75. doi: 10.1079/NRR200479
190. Włodarczyk M, Śliżewska K. Efficiency of resistant starch and dextrins as prebiotics: a review of the existing evidence and clinical trials. Nutrients. (2021) 13:3808. doi: 10.3390/nu13113808
191. Ślizewska K, Libudzisz Z, Barczyñska R, Kapuśniak J, Zduñczyk Z, Juśkiewicz J. Dietary resistant dextrins positively modulate fecal and cecal microbiota composition in young rats. Acta Biochim Pol. (2015) 62:677–81. doi: 10.18388/abp.2015_1101
192. Zhang Z, Tian T, Chen Z, Liu L, Luo T, Dai J. Characteristics of the gut microbiome in patients with prediabetes and type 2 diabetes. PeerJ. (2021) 9:e10952–10952. doi: 10.7717/peerj.10952
193. Berard M, Deremaux L, Lefranc-Millot C. Prebiotic Effects of NUTRIOSE§: A Review. Bangkok: International Congress of Nutrition (2009).
194. Valcheva R, Hotte N, Gillevet P, Sikaroodi M, Thiessen A, Madsen KL. Soluble dextrin fibers alter the intestinal microbiota and reduce proinflammatory cytokine secretion in male IL-10-deficient mice. J Nutr. (2015) 145:2060–6. doi: 10.3945/jn.114.207738
195. Fusunyan RD, Quinn JJ, Fujimoto M, MacDermott RP, Sanderson IR. Butyrate switches the pattern of chemokine secretion by intestinal epithelial cells through histone acetylation. Mol Med. (1999) 5:631–40. doi: 10.1007/bf03402075
196. Schwab M, Reynders V, Loitsch S, Steinhilber D, Stein J, Schröder O. Involvement of different nuclear hormone receptors in butyrate-mediated inhibition of inducible NFκB signalling. Mol Immunol. (2007) 44:3625–32. doi: 10.1016/j.molimm.2007.04.010
197. Hobden MR, Guérin-Deremaux L, Rowland I, Gibson GR, Kennedy OB. Potential anti-obesogenic properties of non-digestible carbohydrates: specific focus on resistant dextrin. In: Proceedings of the Nutrition Society. Cambridge: Cambridge University Press (2015). p. 258–67. doi: 10.1017/S0029665115000087
198. Hu F, Niu Y, Xu X, Hu Q, Su Q, Zhang H. Resistant dextrin improves high-fat-high-fructose diet induced insulin resistance. Nutr Metab (Lond). (2020) 17:36. doi: 10.1186/s12986-020-00450-2
199. Guerin-Deremaux L, Ringard F, Desailly F, Wils D. Effects of a soluble dietary fibre NUTRIOSE§on colonic fermentation and excretion rates in rats. Nutr Res Pract. (2010) 4:470–6. doi: 10.4162/nrp.2010.4.6.470
200. Kozmus CEP, Moura E, Serrão MP, Real H, Guimarães JT, Guedes-de-Pinho P, et al. Influence of dietary supplementation with dextrin or oligofructose on the hepatic redox balance in rats. Mol Nutr Food Res. (2011) 55:1735–9. doi: 10.1002/mnfr.201100287
201. Kellow NJ, Coughlan MT, Savige GS, Reid CM. Effect of dietary prebiotic supplementation on advanced glycation, insulin resistance and inflammatory biomarkers in adults with pre-diabetes: a study protocol for a double-blind placebo-controlled randomised crossover clinical trial. BMC Endocr Disord. (2014) 14:55. doi: 10.1186/1472-6823-14-55
202. Shimazu T, Hirschey MD, Newman J, He W, Shirakawa K, Le Moan N, et al. Suppression of oxidative stress by β-hydroxybutyrate, an endogenous histone deacetylase inhibitor. Science. (2013) 339:211–4. doi: 10.1126/science.1227166
203. Rezaie A, Parker RD, Abdollahi M. Oxidative stress and pathogenesis of inflammatory bowel disease: an epiphenomenon or the cause↑ Dig Dis Sci. (2007) 52:2015–21. doi: 10.1007/s10620-006-9622-2
204. Flach J, van der Waal MB, van den Nieuwboer M, Claassen E, Larsen OFA. The underexposed role of food matrices in probiotic products: reviewing the relationship between carrier matrices and product parameters. Crit Rev Food Sci Nutr. (2018) 58:2570–84. doi: 10.1080/10408398.2017.1334624
205. Wang X, Yang J, Qiu X, Wen Q, Liu M, Zhou D, et al. Probiotics, pre-biotics and synbiotics in the treatment of pre-diabetes: a systematic review of randomized controlled trials. Front Public Health. (2021) 9:265. doi: 10.3389/fpubh.2021.645035
206. Green M, Arora K, Prakash S. Microbial medicine: prebiotic and probiotic functional foods to target obesity and metabolic syndrome. Int J Mol Sci. (2020) 21:2890. doi: 10.3390/ijms21082890
207. Scott KP, Martin JC, Duncan SH, Flint HJ. Prebiotic stimulation of human colonic butyrate-producing bacteria and bifidobacteria, in vitro. FEMS Microbiol Ecol. (2014) 87:30–40. doi: 10.1111/1574-6941.12186
208. Nazzaro F, Fratianni F, Nicolaus B, Poli A, Orlando P. The prebiotic source influences the growth, biochemical features and survival under simulated gastrointestinal conditions of the probiotic Lactobacillus acidophilus. Anaerobe. (2012) 18:280–5. doi: 10.1016/j.anaerobe.2012.03.002
209. Parvez S, Malik KA, Ah Kang S, Kim H-Y. Probiotics and their fermented food products are beneficial for health. J Appl Microbiol. (2006) 100:1171–85. doi: 10.1111/j.1365-2672.2006.02963.x
210. Kassaian N, Feizi A, Aminorroaya A, Amini M. Probiotic and synbiotic supplementation could improve metabolic syndrome in prediabetic adults: a randomized controlled trial. Diabetes Metab Syndr. (2019) 13:2991–6. doi: 10.1016/j.dsx.2018.07.016
211. Fuhren J, Rösch C, Ten Napel M, Schols HA, Kleerebezem M. Synbiotic matchmaking in Lactiplantibacillus plantarum: substrate screening and gene-trait matching to characterize strain-specific carbohydrate utilization. Appl Environ Microbiol. (2020) 86:e01081–20. doi: 10.1128/AEM.01081-20
212. Nagpal R, Kaur A. Synbiotic effect of various prebiotics on in vitro activities of probiotic lactobacilli. Ecol Food Nutr. (2011) 50:63–8. doi: 10.1080/03670244.2011.539161
213. Hampe CS, Roth CL. Probiotic strains and mechanistic insights for the treatment of type 2 diabetes. Endocrine. (2020) 58:207–27. doi: 10.1007/s12020-017-1433-z
214. Wu X, Ma C, Han L, Nawaz M, Gao F, Zhang X, et al. Molecular characterisation of the faecal microbiota in patients with type II diabetes. Curr Microbiol. (2010) 61:69–78. doi: 10.1007/s00284-010-9582-9
215. Nicholson JK, Holmes E, Kinross J, Burcelin R, Gibson G, Jia W, et al. Host-Gut Microbiota Metabolic Interactions. Science. (2012) 336:1262–7. doi: 10.1126/science.1223813
216. Gibson GR, Macfarlane S, Macfarlane GT. Metabolic interactions involving and methanogenic bacteria in the human large intestine sulphate-reducing. FEMS Microbiol Ecol. (1993) 12:117–25. doi: 10.1111/j.1574-6941.1993.tb00023.x
217. Millard AL, Mertes PM, Ittelet D, Villard F, Jeannesson P, Bernard J. Butyrate affects differentiation, maturation and function of human monocyte-derived dendritic cells and macrophages. Clin Exp Immunol. (2002) 130:245–55. doi: 10.1046/j.0009-9104.2002.01977.x
218. Al-Lahham SH, Roelofsen H, Priebe M, Weening D, Dijkstra M, Hoek A, et al. Regulation of adipokine production in human adipose tissue by propionic acid. Eur J Clin Invest. (2010) 40:401–7. doi: 10.1111/j.1365-2362.2010.02278.x
219. Weiss GA, Hennet T. Mechanisms and consequences of intestinal dysbiosis. Cell Mol Life Sci. (2017) 74:2959–77. doi: 10.1007/s00018-017-2509-x
220. Karczewski J, Poniedziałek B, Adamski Z, Rzymski P. The effects of the microbiota on the host immune system. Autoimmunity. (2014) 47:494–504. doi: 10.3109/08916934.2014.938322
221. Pedersen C, Gallagher E, Horton F, Ellis RJ, Ijaz UZ, Wu H, et al. Host-microbiome interactions in human type 2 diabetes following prebiotic fibre (galacto-oligosaccharide) intake. Br J Nutr. (2016) 116:1869–77. doi: 10.1017/S0007114516004086
222. Toejing P, Khampithum N, Sirilun S, Chaiyasut C, Lailerd N. Influence of Lactobacillus paracasei HII01 supplementation on glycemia and inflammatory biomarkers in type 2 diabetes: a randomized clinical trial. Foods. (2021) 10:1455. doi: 10.3390/foods10071455
223. Vancamelbeke M, Vermeire S. The intestinal barrier: a fundamental role in health and disease. Exp Rev Gastroenterol Hepatol. (2017) 11:821–34. doi: 10.1080/17474124.2017.1343143
224. Arrieta MC, Bistritz L, Meddings JB. Recent advances in clinical practice alterations in intestinal permeability. Gut. (2006) 55:1512–20. doi: 10.1136/gut.2005.085373
225. Meddings J. The significance of the gut barrier in disease. Gut. (2008) 57:438–40. doi: 10.1136/gut.2007.143172
226. De Kort S, Keszthelyi D, Masclee AAM. Leaky gut and diabetes mellitus: what is the link↑ Obes Rev. (2011) 12:449–58. doi: 10.1111/j.1467-789X.2010.00845.x
227. Suppli MP, Bagger JI, Lelouvier B, Broha A, Demant M, Kønig MJ, et al. Hepatic microbiome in healthy lean and obese humans. JHEP Rep Innov Hepatol. (2021) 3:100299. doi: 10.1016/j.jhepr.2021.100299
228. Rastad H, Ejtahed H-S, Mahdavi-Ghorabi A, Arzaghi M, Safari A, Shahrestanaki E, et al. Factors associated with the poor outcomes in diabetic patients with COVID-19. J Diabetes Metab Disord. (2020) 19:1293–302. doi: 10.1007/s40200-020-00646-6
229. Ciornei RT. Prevention of severe coronavirus disease 2019 outcomes by reducing low-grade inflammation in high-risk categories. Front Immunol. (2020) 11:1762. doi: 10.3389/fimmu.2020.01762
230. Goyal R, Faizy AF, Siddiqui SS, Singhai M. Evaluation of TNF-α and IL-6 levels in obese and non-obese diabetics: pre- and postinsulin effects. N Am J Med Sci. (2012) 4:180–4. doi: 10.4103/1947-2714.94944
231. Zhu L, She ZG, Cheng X, Qin JJ, Zhang XJ, Cai J, et al. Association of blood glucose control and outcomes in patients with COVID-19 and pre-existing type 2 diabetes. Cell Metab. (2020) 31:1068–77.e3. doi: 10.1016/j.cmet.2020.04.021
232. Zuo T, Zhang F, Lui GCY, Yeoh YK, Li AYL, Zhan H, et al. Alterations in gut microbiota of patients with COVID-19 during time of hospitalization. Gastroenterology. (2020) 159:944–55.e8. doi: 10.1053/j.gastro.2020.05.048
233. Santacroce L, Inchingolo F, Topi S, Del Prete R, Di Cosola M, Charitos IA, et al. Potential beneficial role of probiotics on the outcome of COVID-19 patients: an evolving perspective. Diabetes Metab Syndr. (2021) 15:295–301. doi: 10.1016/j.dsx.2020.12.040
234. Song H, Hu W, Zhou X, Tao J, Zhang S, Su X, et al. Clinical benefits from administering probiotics to mechanical ventilated patients in intensive care unit: a PRISMA-guided meta-analysis. Front Nutr. (2022) 8:798827. doi: 10.3389/fnut.2021.798827
235. Custodero C, Mankowski RT, Lee SA, Chen Z, Wu S, Manini TM, et al. Evidence-based nutritional and pharmacological interventions targeting chronic low-grade inflammation in middle-age and older adults: a systematic review and meta-analysis. Ageing Res Rev. (2018) 46:42–59. doi: 10.1016/j.arr.2018.05.004
236. Plaza-Díaz J, Ruiz-Ojeda FJ, Vilchez-Padial LM, Gil A. Evidence of the anti-inflammatory effects of probiotics and synbiotics in intestinal chronic diseases. Nutrients. (2017) 9:555. doi: 10.3390/nu9060555
237. Azad MAK, Sarker M, Wan D. Immunomodulatory effects of probiotics on cytokine profiles. Biomed Res Int. (2018) 23:8063647. doi: 10.1155/2018/8063647
238. Batista KS, de Albuquerque JG, de Vasconcelos MHA, Bezerra MLR, da Silva Barbalho MB, Pinheiro RO, et al. Probiotics and prebiotics: potential prevention and therapeutic target for nutritional management of COVID-19↑ Nutr Res Rev. (2021) 2021:1–18. doi: 10.1017/S0954422421000317
239. Chattopadhyay I, Shankar EM. SARS-CoV-2-indigenous microbiota nexus: does gut microbiota contribute to inflammation and disease severity in COVID-19↑ Front Cell Infect Microbiol. (2021) 11:590874. doi: 10.3389/fcimb.2021.590874
240. Hye Joung K, Min Kim J, Choung S, Hee Lee J, Jin Kim H, Jeong Ku B. Association between IL-1beta and cardiovascular disease risk in patients with newly diagnosed, drug-naïve type 2 diabetes mellitus: a cross-sectional study. Ann Transl Med. (2020) 8:225. doi: 10.21037/atm.2020.01.17
241. Yaghini N, Mahmoodi M, Asadikaram GR, Hassanshahi GH, Khoramdelazad H, Kazemi Arababadi M. Serum levels of interleukin 10 (IL-10) in patients with type 2 diabetes. Iran Red Cresc Med J. (2011) 13: 751–2.
242. Parhi A, Das S, Mahapatra S, Pradhan N, Behera M, Patnaik B, et al. The level and role of interleukin-17 in patients of type 2 diabetes mellitus with and without complications. J Diabetes Mellit. (2019) 09:176–85. doi: 10.4236/jdm.2019.94017
243. Kumawat M, Sharma TK, Singh I, Singh N, Ghalaut VS, Vardey SK, et al. Antioxidant enzymes and lipid peroxidation in type 2 diabetes mellitus patients with and without nephropathy. N Am J Med Sci. (2013) 5:213–9. doi: 10.4103/1947-2714.109193
244. Kharroubi AT, Darwish HM, Akkawi MA, Ashareef AA, Almasri ZA, Bader KA, et al. Total antioxidant status in type 2 diabetic patients in palestine. J Diabetes Res. (2015) 2015:461271. doi: 10.1155/2015/461271
245. Kuppan K, Mohanlal J, Mohammad AM, Babu KA, Sen P, Das Undurti N, et al. Elevated serum OxLDL is associated with progression of type 2 diabetes mellitus to diabetic retinopathy. Exp Eye Res. (2019) 186:107668. doi: 10.1016/j.exer.2019.05.008
246. Mobini R, Tremaroli V, Ståhlman M, Karlsson F, Levin M, Ljungberg M, et al. Metabolic effects of Limosilactobacillus reuteri DSM 17938 in people with type 2 diabetes: a randomized controlled trial. Diabetes Obes Metab. (2017) 19:579–89. doi: 10.1111/dom.12861
247. Hove KD, Brøns C, Færch K, Lund SS, Rossing P, Vaag A. Effects of 12 weeks of treatment with fermented milk on blood pressure, glucose metabolism and markers of cardiovascular risk in patients with type 2 diabetes: a randomised double-blind placebo-controlled study. Eur J Endocrinol. (2015) 172:11–20. doi: 10.1530/EJE-14-0554
Keywords: gut microbiome, gastrointestinal microbiota, clinical trial, inflammatory markers, dysbiosis, resistant dextrin, Lactobacillus, Bifidobacterium
Citation: Paul P, Kaul R, Abdellatif B, Arabi M, Upadhyay R, Saliba R, Sebah M and Chaari A (2022) The Promising Role of Microbiome Therapy on Biomarkers of Inflammation and Oxidative Stress in Type 2 Diabetes: A Systematic and Narrative Review. Front. Nutr. 9:906243. doi: 10.3389/fnut.2022.906243
Received: 28 March 2022; Accepted: 08 April 2022;
Published: 25 May 2022.
Edited by:
Carlos Gómez-Gallego, University of Eastern Finland, FinlandReviewed by:
Parameth Thiennimitr, Chiang Mai University, ThailandLaura Mitrea, University of Agricultural Sciences and Veterinary Medicine of Cluj-Napoca, Romania
Copyright © 2022 Paul, Kaul, Abdellatif, Arabi, Upadhyay, Saliba, Sebah and Chaari. This is an open-access article distributed under the terms of the Creative Commons Attribution License (CC BY). The use, distribution or reproduction in other forums is permitted, provided the original author(s) and the copyright owner(s) are credited and that the original publication in this journal is cited, in accordance with accepted academic practice. No use, distribution or reproduction is permitted which does not comply with these terms.
*Correspondence: Ali Chaari, YWxjMjAyM0BxYXRhci1tZWQuY29ybmVsbC5lZHU=