- 1Department of Anesthesiology, National-Local Joint Engineering Research Centre of Translational Medicine of Anesthesiology, West China Hospital of Sichuan University, Chengdu, China
- 2Laboratory of Mitochondria and Metabolism, West China Hospital of Sichuan University, Chengdu, China
- 3Department of Radiology, Huaxi MR Research Center (HMRRC), Functional and Molecular Imaging Key Laboratory of Sichuan Province, West China Hospital of Sichuan University, Chengdu, China
Obesity is a significant health concern as a result of poor-quality diet, for example, high-fat diet (HFD). Although multiple biological and molecular changes have been identified to contribute to HFD-induced pain susceptibility, the mechanisms are not fully understood. Here, we show that mice under 8 weeks of HFD were sensitive to mechanical and thermal stimuli, which was coupled with an accumulation of branched-chain amino acids (BCAAs) in lumbar dorsal root ganglia (DRG) due to local BCAA catabolism deficiency. This HFD-induced hyperalgesic phenotype could be exacerbated by supply of excessive BCAAs or mitigated by promotion of BCAA catabolism via BT2 treatment. In addition, our results suggested that HFD-related pain hypersensitivity was associated with a pro-inflammatory status in DRG, which could be regulated by BCAA abundance. Therefore, our study demonstrates that defective BCAA catabolism in DRG facilitates HFD-induced pain hypersensitivity by triggering inflammation. These findings not only reveal metabolic underpinnings for the pathogenesis of HFD-related hyperalgesia but also offer potential targets for developing diet-based therapy of chronic pain.
Introduction
High-fat diet (HFD), consisting of at least 35% of calories from fats, is correlated with increased risks of several chronic diseases, such as obesity, diabetes and cardiovascular diseases (1). Also, prolonged HFD intake is implicated in aberrant nociception and manifestations of allodynia (2). Although multiple biological and molecular changes have been identified to contribute to HFD-induced pain susceptibility, the mechanisms are not fully understood (3).
Branched-chain amino acids (BCAAs), including leucine, isoleucine, and valine, are essential amino acids for mammals and comprised of ∼ 20% of the amino acids incorporated into body protein (4). The rate of protein turnover, BCAA intake, and catabolism are key mechanisms in governing BCAA homeostasis in the body (5). In the BCAA catabolic pathway, BCAAs are first converted into branched-chain alpha-ketoacids (BCKAs) by branched-chain amino-transferase (BCAT) in a reversible reaction, followed by irreversible decarboxylation by branched-chain alpha-ketoacid dehydrogenase (BCKDH) complex, and eventually metabolized to acetyl-CoA or succinyl-CoA for oxidation in the tricarboxylic acid (TCA) cycle (4).
Epidemiological evidence shows that elevation of circulating BCAAs and related metabolites are strongly associated with insulin resistance and diabetes (6, 7). An accumulation of BCAAs in dorsal root ganglia (DRG) have also been observed in diabetic neuropathy, of which one third of patients will develop neuropathic pain (8), supporting a link between defective BCAA catabolism and chronic pain. Indeed, defective BCAA catabolism has been shown to correlate with inflammation in rodents and obese patients, and inflammation is believed to contribute to HFD-induced chronic pain (9, 10). Nonetheless, whether BCAA homeostasis regulates HFD-related pain behaviors is still elusive.
In the present study, we sought to elucidate the relationship between BCAA catabolism and pain susceptibility in HFD-fed mice. Our results reveal that excessive BCAAs in DRG is a culprit in HFD-induced pain susceptibility, and the findings may shed light on the diet-based treatment of chronic pain by targeting BCAA catabolism.
Materials and Methods
Mice
All animal experiments were approved by the Animal Ethics Committee of West China Hospital, Sichuan University, China. The animals received humane care in accordance with the Guides for the Care and Use of Laboratory Animals published by NIH. Male C57BL/6J mice were purchased from Dossy Experimental Animals Co. Ltd, Chengdu, China. Mice were maintained in a vivarium with a 12-hour light/dark cycle at 22oC and water was available ad libitum. The 4-week-old mice were randomly fed with high-fat diet (HFD), composed of 60% kcal per kg fat (D12492, Research Diets Inc, New Brunswick, NJ, United States), or chow diet (CD), composed of 11.85% kcal per kg fat (SWC9101, Xietong LTD, CN) for 8 or 16 weeks. The body weight and random blood glucose of mice were recorded weekly. For the measurement of blood glucose, approximately 5 μl of blood was collected via tail and analyzed with Blood Glucose Meter (Roche, Switzerland). At the end of the experimental protocol, mice were sacrificed to collect plasma and bilateral lumbar 4–5 (L4-5) DRG. Samples were quickly frozen in liquid nitrogen and stored until analysis.
Glucose and Insulin Tolerance Tests
Glucose tolerance test (GTT) and insulin tolerance test (ITT) were performed after 8 weeks on HFD and CD, as described previously (11). Prior to ITT and GTT, mice were fasted for 4 or 6 h, respectively. After the measurement of fasting blood glucose, insulin (0.75 U/kg body weight) or D-glucose (2 g/kg body weight) were administrated through intraperitoneal injection. Blood glucose levels were determined at 15, 30, 60, 90, and 120 min by a Blood Glucose Meter (Roche, Switzerland).
Branched-Chain Amino Acid or BT2 Administration
After 4 weeks on HFD, mice were randomly divided into three groups to receive water (Vehicle; Veh), 2% BCAA (weight ratio, isoleucine: leucine: valine = 0.8:1.5:1, Sigma Aldrich, St. Louis, MO, United States) or BT2. Compound BT2 (3,6-dichlorobenzo[b]thiophene-2-carboxylic acid, Santa Cruz Biotechnology, Santa Cruz, CA, United States), an inhibitor of branched-chain alpha-ketoacid dehydrogenase kinase (BCKDK), is diluted with solution (5% DMSO, 10% cremophor EL, and 85% 0.1 M sodium bicarbonate, pH 9.0) and administered by oral gavage at 40 mg/kg body weight per day.
Behavioral Analyses
For evaluating mechanical allodynia, paw withdrawal thresholds in response to mechanical stimuli were measured by calibrated von Frey filaments (Stoelting Company, Wood Dale, IL, United States). Briefly, mice were placed in an individual Plexiglas chamber on an elevated mesh grid floor. After adaption for 1 h, von Frey filaments were used to stimulate the middle of the plantar surface of left and right hind paws. In the paradigm of the up-down method, test was initiated with a 0.16 g in the middle of the series force (0.008, 0.02, 0.04, 0.07, 0.16, 0.4, 0.6, 1.0, and 1.4 g). If a negative response occurred, the next stronger stimulus was applied; if a positive response appeared, the next weaker stimulus was chosen. The test was terminated when positive response (e.g., raising, retracting, rapidly swinging or licking feet reaction) was stimulated 3 times with an interval of 5 min by certain increasing irritation. We took the average value of filaments as mechanical threshold.
In the Hargreaves test, paw withdrawal latencies to thermal stimulation were examined with a radiant heat source (Model 37370; Ugo-Basile, Italy). In quiet room, mice were placed in a transparent organic chamber on a glass plate. After mouse adapting to circumstances, the plantar surface of each hind paw was stimulated by light source (IR = 35 mW/cm2). The light source was automatically turned off when hind paws moved and withdrawal latencies were recorded. Each plantar was repeated 3 times at 10 min intervals. To avoid damage to the hind paw, a cut-off time of 20 s was set up. Experimenters were blinded to the group allocation.
Measurement of Branched-Chain Amino Acid Content
Plasma or DRG tissue samples were mixed into equal volume of acetonitrile. Then the supernatant was obtained by high-speed centrifugation and analyzed the BCAA levels by liquid chromatography-tandem mass spectrometry (1260-6460, Agilent, United States).
RNA Isolation and Quantitative Real-Time PCR
Total RNA was isolated from frozen DRG tissues using the RNeasy Kit (Invitrogen, Thermo Fisher Scientific, Grand Island, NY, United States), and cDNA was synthesized using iScript cDNA Synthesis Kit (1708890, BIO-RAD, Hercules, CA, United States). qPCR was performed using the iTaq Universal SYBR Green Supermix (1725124, BIO-RAD, CA) with template cDNA and primers for each gene in a BIO-RAD CFX96 real-time PCR system. The relative mRNA expression levels were calculated by the ΔΔCt method and the results were normalized to β-actin levels. The primer sequences are listed as below.
Il-6: F-CCAAGAGGTGAGTGCTTCCC, R-CTGTTGTTCA GACTCTCTCCCT;
Il-1β: F-GCAACTGTTCCTGAACTCAACT, R-ATCTT TTGGGGTCCGTCAACT;
Ccl3: F-TTCTCTGTACCATGACACTCTGC, R-CGTGG AATCTTCCGGCTGTAG;
Cxcl1: F-CTGGGATTCACCTCAAGAACATC, R-CAGGG TCAAGGCAAGCCTC;
β-actin: F- GCAGGAGTACGATGAGTCCG, R-ACGCAGC TCAGTAACAGTCC.
Western Blot
Frozen DRG tissues were lysed with RIPA buffer with protease inhibitor cocktail (Roche), PMSF and Na2VO3. Protein sample (5–20 μg) was separated by SDS–PAGE and transferred to a PVDF membrane. Membrane was blocked in 5% non-fat milk and incubated with primary antibodies at 4oC overnight with mild agitation, including anti-PP2Cm (DF4348, Affinity, San Francisco, CA, United States), anti-BCKDHA-E1α (#90198, Cell Signaling Technology, Danvers, MA, United States), anti-phosphor-BCKDHA-E1α (Ser293) (#40368, Cell Signaling Technology, Danvers, MA, United States), and anti-GAPDH (10494-1-AP, Proteintech, Rosamond, IL, United States) antibodies. After incubation with secondary antibodies (SA000001-2, Proteintech, Rosamond, IL, United States) at room temperature for 1 h, the signal intensities were visualized by an ECL Western blot detection kit (#P10100, ECM, Pleasant Prairie, WI, United States) and analyzed with Image J software (NIH).
Branched-Chain Alpha-Ketoacid Dehydrogenase Activity Assay
The BCKDH complex activity in the DRG was analyzed as described previously (12). The BCKDH enzymatic activity was defined as the rate of formation of 1 μmol of NADH/min at 30°C. Both actual and total activities were measured; the former corresponded to in vivo dephosphorylated enzyme levels and the latter was obtained by treating the tissue extract with lambda protein phosphatase to fully dephosphorylate enzymes. The BCKDH activity is calculated as the percentage of actual activity relative to total activity.
Hematoxylin and Eosin Staining
L4-5 DRG were fixed in 4% polyformaldehyde followed by dehydration in an ascending ethanol series. Tissues were embedded in paraffin and cut into 5 μm sections for Hematoxylin and Eosin (H&E) staining (ab245880, Abcam, Cambridge, United Kingdom). Morphological analysis was performed on three sections per DRG using OlyVIA digital software.
Statistical Analysis
Statistical analysis was performed with GraphPad Prism 8 software. All data were presented as the mean ± SEM. Grayness of protein strips were analyzed by Image J software. Fold change of protein expression were analyzed with two-tailed unpaired Student’s t-tests. Body weight, blood glucose changes, and BCAA content were analyzed with two-way repeated measures analysis of variance (ANOVA). Behavioral time course data were analyzed with two-way ANOVA to determine overall differences between diet groups, with Bonferroni posttests to determine significance of differences between groups at each individual time point. The area of DRG cells, cell gaps, and the number of inflammatory cells in L4-5 DRG were counted and measured by the Image-Pro plus 6.0 pathological analysis software. The expression of inflammatory mediator was analyzed with one-way ANOVA with Dunnett’s posttest within each group. Differences were considered significant when P < 0.05.
Results
High-Fat Diet Induces Mechanical and Heat Hyperalgesia in Mice
To determine whether HFD affects nociceptive responses, we fed 4-week-old male mice with either standard CD or HFD for 8 weeks. As expected, mice under 8 weeks of HFD showed a greater body weight, higher blood glucose levels after overnight fasting, and impaired glucose tolerance as compared to the CD group (Figures 1A–D). By utilizing von Frey test and Hargreaves test, we found that the mice exhibited reduced nociceptive thresholds to both mechanical and heat stimuli after 8 weeks on HFD (Figures 1E,F). These results suggest that HFD lowers nociceptive thresholds and induces mechanical and thermal hyperalgesia.
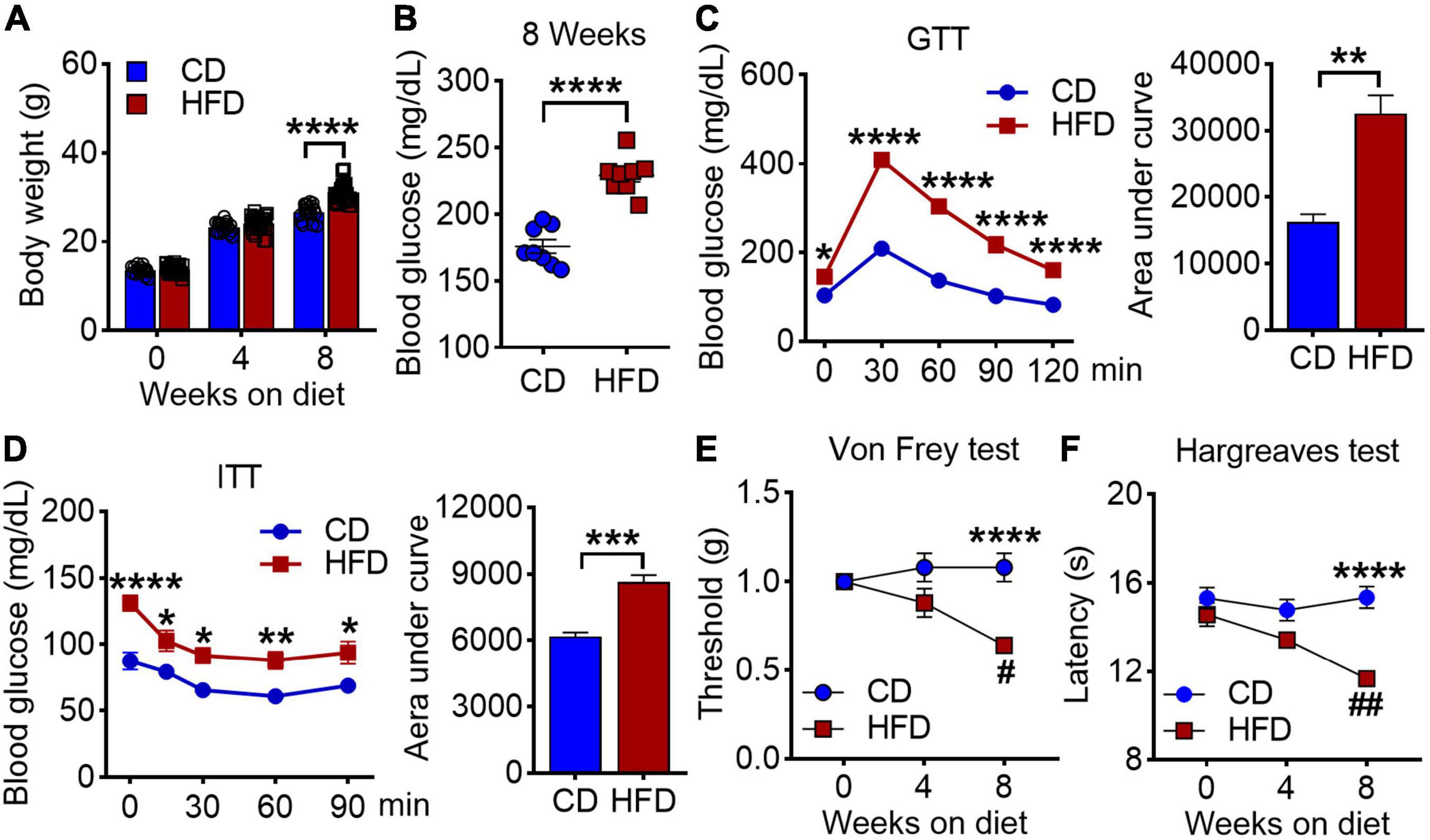
Figure 1. Effects of high-fat diet on body weight, blood glucose and pain behaviors in mice. (A) Body weights were measured at 0, 4 and 8 weeks on either chow diet (CD) or high fat diet (HFD) (n = 20–40). (B) Blood glucose levels were determined on 8 weeks of CD and HFD feeding (n = 4–8). (C) Glucose tolerance test (GTT) of mice at 8 weeks CD or HFD diet after 6 h fasting, and the area under curve (n = 20). (D) Insulin tolerance tests (ITT) of mice at 8 weeks CD or HFD diet after 4 h fasting, and the area under curve (n = 7). (E,F) Mechanical withdrawal thresholds (E) and withdrawal latencies to heat stimulus (F) after 4 and 8 weeks on CD or HFD (n = 5–15). Data are present as mean ± SEM. *P < 0.05, **P < 0.01, ***P < 0.005, and ****P < 0.001 for CD vs. HFD mice. #P < 0.05, ##P < 0.01 for HFD mice at 4 weeks on diet vs. HFD mice at 8 weeks on diet.
High-Fat Diet-Induced Hyperalgesia Is Coupled With an Accumulation of Branched-Chain Amino Acids in Dorsal Root Ganglia
A strong association between disrupted BCAA homeostasis and diabetes has been repeatedly observed in human and rodent models (13). In line with it, we found an accumulation of plasma BCAAs in the mice on 8 weeks of HFD (Figure 2A). Moreover, an ∼2-fold increase in BCAA level was also observed in L4-L5 DRG, the hub that senses and transmits periphery stimuli to central nervous system (Figure 2B). Intriguingly, the DRG BCAA levels were linearly correlated with the graded decrease of the mechanical and thermal thresholds (Figures 2C,D and Table 1). BCKDH complex, the rate-limiting enzyme of the BCAA catabolic pathway, is activated by dephosphorylation via a mitochondrial localized 2C-type serine-threonine protein phosphatase (PP2Cm). Further study demonstrated that this increase of BCAAs in DRG was accompanied by a downregulation of protein abundance of PP2Cm, a hyperphosphorylation of BCKDH-E1α at Ser293 and a reduced BCKDH activity, suggesting that 8 weeks of HFD induces a defective BCAA catabolism in DRG (Figures 2E,F). Of note, with no pain behavioral changes, the mice on 4 weeks of HFD did not present an aberrance of BCAA levels. Together, these findings suggest that HFD-induced hyperalgesia is couple with a defective BCAA catabolism in DRG.
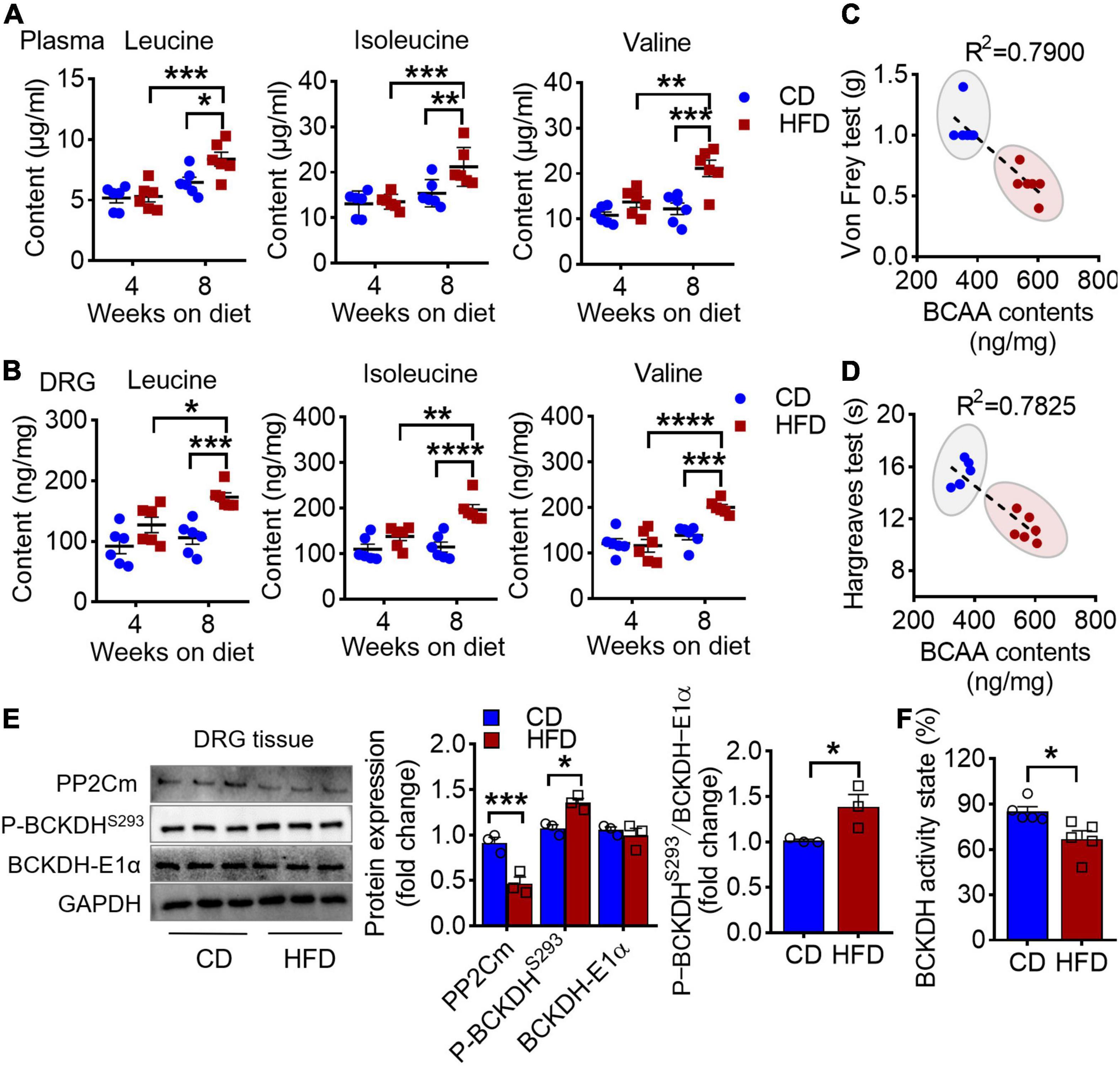
Figure 2. HFD-induced hyperalgesia is coupled with an accumulation of BCAAs in DRG. (A) BCAA (leucine, isoleucine, and valine) levels in plasma after 4 and 8 weeks feeding in CD (n = 6) and HFD mice (n = 6). (B) BCAA levels in L4-L5 DRG under 4 and 8 weeks of CD (n = 6) and HFD diet (n = 6). (C,D) The linear regression analysis between mechanical (C) or thermal (D) withdrawal thresholds and BCAA levels (leucine + isoleucine + valine) in L4-L5 DRG under 8 weeks of diet. (E) Western blots analysis of BCAAs catabolic enzymes, PP2Cm, P-BCKDHS293 and BCKDH-E1α, in L4-L5 DRG after 8 weeks on CD (n = 3) or HFD (n = 3). (F) The BCKDH activity state in DRG after 8 weeks on CD (n = 3) or HFD (n = 3). Activity state means percentage of the BCKDH complex in the active dephosphorylated form. Data are shown as mean ± SEM. *P < 0.05, **P < 0.01, ***P < 0.005 and ****P < 0.001.
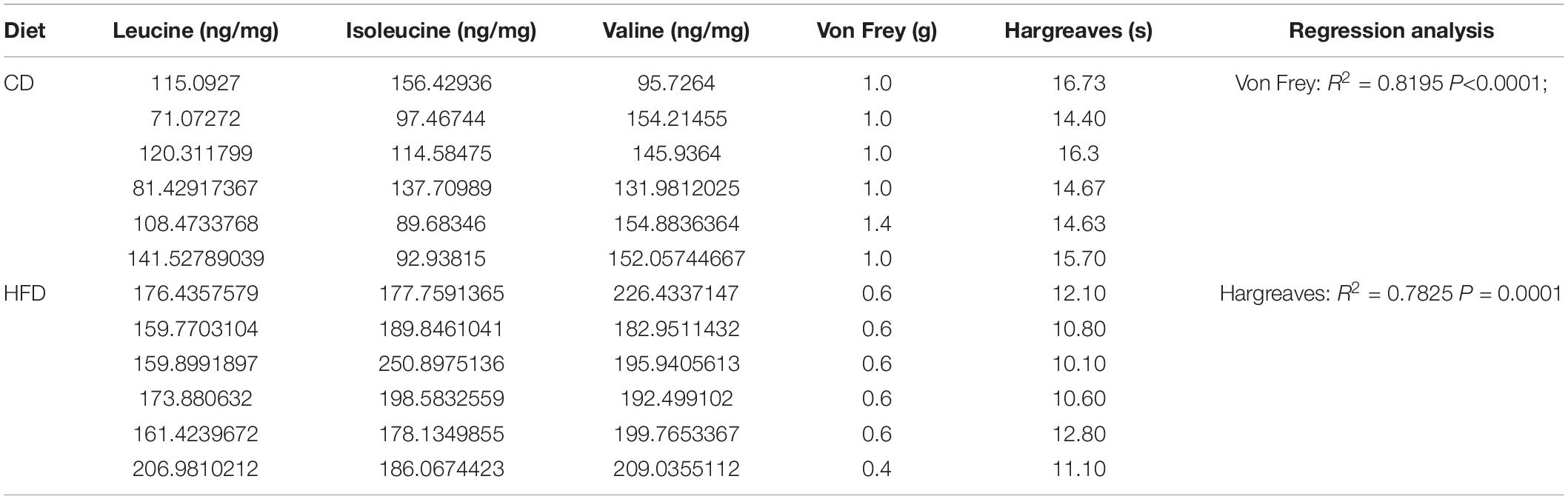
Table 1. The data of mechanical and thermal withdrawal thresholds and BCAA levels in DRG of every mouse, and the linear regression analysis.
Branched-Chain Amino Acids Supplementation Exacerbates High-Fat Diet-Induced Hyperalgesia
The above observation raised the question of whether BCAAs play a causal role in HFD-induced hyperalgesia. To study it, we supplemented BCAAs in the drinking water of the HFD-fed mice at 4 weeks, the time point having no hyperalgesic phenotype yet, and performed pain behavioral tests once a week during the following 4 weeks (Figure 3A). After 4 weeks of BCAAs and HFD co-feeding, the abundance of all the three BCAAs in the DRG was significantly increased as compared to the CD group and HFD-fed only group (Figure 3B). Von Frey test showed that simultaneous supply of BCAAs progressively and further reduced HFD-induced downregulation of mechanical withdrawal thresholds; hypersensitivity to mechanical stimuli was observed in the HFD-fed mice (5 weeks) with 1 week of BCAAs supplementation, and the response was comparable to that of the mice under 8 weeks of HFD only (Figure 3C). A similar effect of BCAAs in reducing thermal paw withdrawal latencies was also observed in the Hargreaves test (Figure 3D). Therefore, these results suggest that supply of BCAAs increases their abundance in DRG and exacerbates HFD-induced hyperalgesia.
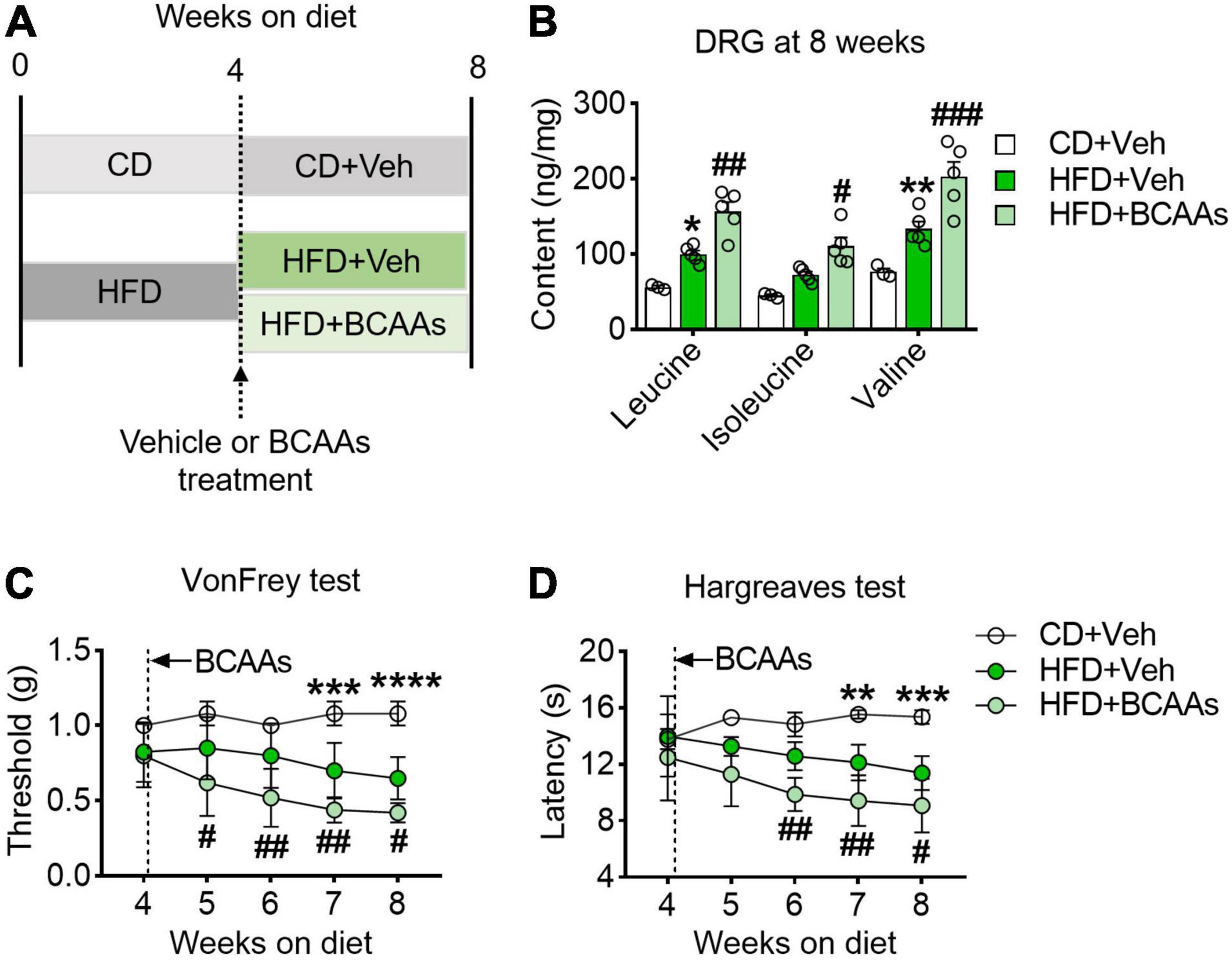
Figure 3. BCAAs supplementation aggravates pain behaviors in HFD-fed mice. (A) Schematic of 8-week HFD with BCAA supplementation paradigm. To demonstrate whether BCAAs play a causal role in HFD-induced hyperalgesia, mice were changed to a 2.2% BCAAs drinking water at 4 weeks. (B) BCAA levels in L4-L5 DRG after 8 weeks diet with or without BCAAs supplementation in CD + Veh (n = 3), HFD + Veh (n = 5) and HFD + BCAAs (n = 5). (C,D) Mechanical withdrawal thresholds (C) and withdrawal latencies to heat stimulus (D) at 8 weeks diet in CD + Veh (n = 5), HFD + Veh (n = 8–9) and HFD + BCAAs (n = 9–10). Data are shown as mean ± SEM. *P < 0.05, **P < 0.01, ***P < 0.005 and ****P < 0.001 for HFD + Veh vs. CD + Veh; #P < 0.05, ##P < 0.01, ###P < 0.005, for HFD + Veh vs. HFD + BCAAs.
Promoting Branched-Chain Amino Acid Catabolism Prevents and Rescues High-Fat Diet-Induced Hyperalgesia
To further determine whether accumulation of BCAAs caused by defective catabolism was responsible for the pain phenotype, we sought to enhance BCAA catabolism in the HFD-fed mice via daily oral gavage of BT2, an activator of BCKDH, for 8 weeks (40 mg/kg/day) (Figure 4A). After treatment, the suppressed BCKDH activity in DRG caused by HFD was increased by BT2, and the excessive BCAAs were also largely eliminated (Figures 4B,C). The mechanical withdrawal thresholds and thermal paw withdrawal latencies of the HFD-fed mice were also upregulated throughout the course of treatment (Figures 4D,E). Notably, along with the decreased BCAA contents in DRG, both nociceptive responses of the HFD mice were normalized to the CD levels at the end of the 8-week treatment (Figures 4B–E). We next asked if promoting BCAA catabolism delayed the development of hyperalgesia under HFD, and thus supplemented BT2 to the 4-week-HFD mice (Figure 4F). We found that, along with increased BCKDH activity, the accumulation of BCAAs in DRG was mitigated by BT2, and the progressive decrease in nociceptive thresholds to mechanical and heat stimulations was also prevented (Figures 4G–J). Taken together, these data suggest that enhancing BCAA catabolism prevents the emerge of pain phenotypes and rescues established hyperalgesia under HFD.
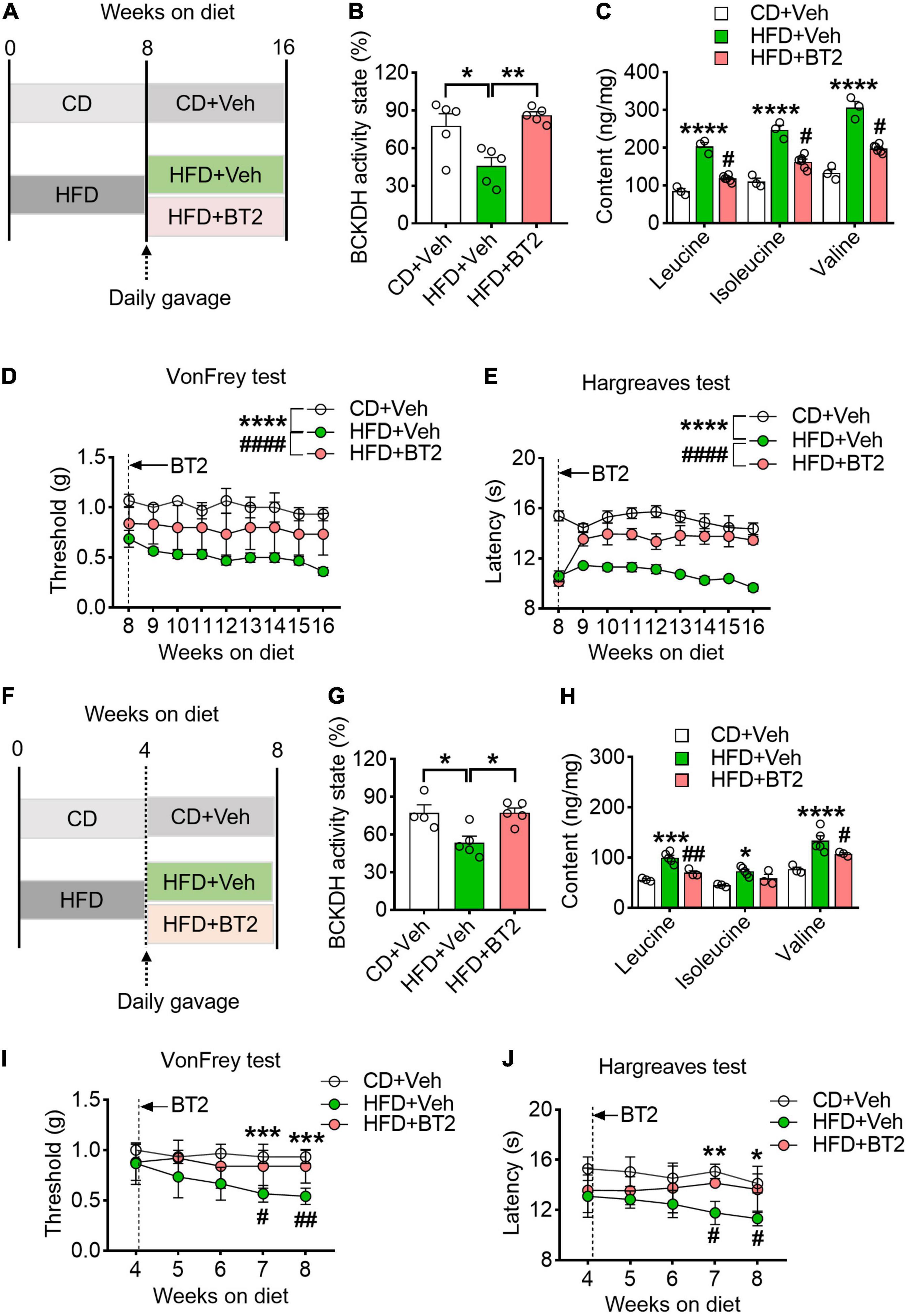
Figure 4. Promoting BCAA catabolism prevents and rescues HFD-induced hyperalgesia. (A) Schematic of the BT2 diet-reversal paradigm, showing mice after 8 weeks HFD either continuing the HFD or receiving BT2 for 8 weeks. (B,C) The BCKDH activity state and BCAA levels in L4-L5 DRG at 16 weeks on HFD with or without BT2 supplementation in CD + Veh (n = 3), HFD + Veh (n = 3), and HFD + BT2 (n = 6). (D,E) Mechanical withdrawal thresholds (D) and withdrawal latencies to heat stimulus (E) from 8 to 16 weeks diet in CD + Veh (n = 5–6), HFD + Veh (n = 4–6) and HFD + BT2 (n = 5–6). (F) Schematic of the BT2 diet-reversal paradigm, to demonstrate whether promoting BCAA catabolism delay the development of hyperalgesia under HFD, the 4-week-HFD mice were supplemented with BT2. (G,H) The BCKDH activity state and BCAA abundance in L4-L5 DRG at 8 weeks on HFD with or without BT2 supplementation in CD + Veh (n = 3), HFD + Veh (n = 5), and HFD + BT2 (n = 3). (I,J) Mechanical withdrawal thresholds (I) and withdrawal latencies to heat stimulus (J) from 4 to 8 weeks in CD + Veh (n = 6), HFD + Veh (n = 6), and HFD + BT2 (n = 5–6). Activity state means percentage of the BCKDH complex in the active dephosphorylated form. Data are present as mean ± SEM. *P < 0.05, **P < 0.01, ***P < 0.005 and ****P < 0.001 for HFD + Veh vs. CD + Veh; #P < 0.05, ##P < 0.01 and ####P < 0.001 for HFD + Veh vs. HFD + BT2.
High-Fat Diet-Induced Accumulation of Branched-Chain Amino Acids Evokes a Pro-inflammatory Response in Dorsal Root Ganglia
We hypothesized that BCAA-modulated pain behavior in HFD mice is associated with an altered inflammatory status in DRG. To test it, we isolated the mouse DRG at the end of experiment and measured the pro-inflammatory cytokines/chemokines. In line with previous reports, 8 weeks on HFD boosted the mRNA expression of pro-inflammatory cytokines/chemokines in DRG, including Il-1β, Il-6, Ccl3, and Cxcl1 (Figure 5A). This local inflammation in DRG was persisted or aggravated at 16 weeks (Figure 5A). Strikingly, promoting BCAA catabolism via BT2 treatment was able to abolish the pro-inflammatory effect of HFD on DRG at both time points (Figure 5A). By contrast, supplementation of BCAAs in the HFD-fed mice further upregulated the mRNA levels of these cytokines/chemokines, suggesting that excessive BCAAs in DRG may synergize with HFD to evoke local inflammation (Figure 5B). In addition, the H&E staining images showed a more severe inflammatory infiltration in DRG after 16 weeks of HFD feeding, and the cross-sectional area of ganglion cells and the gap between cells were also increased. All these pathological changes could be mitigated by 8 weeks of BT2 treatment (Figure 5C). Taken together, these results suggest that excessive BCAAs lead to a pro-inflammatory status in DRG, which might contribute to the HFD-induced hyperalgesic phenotype.
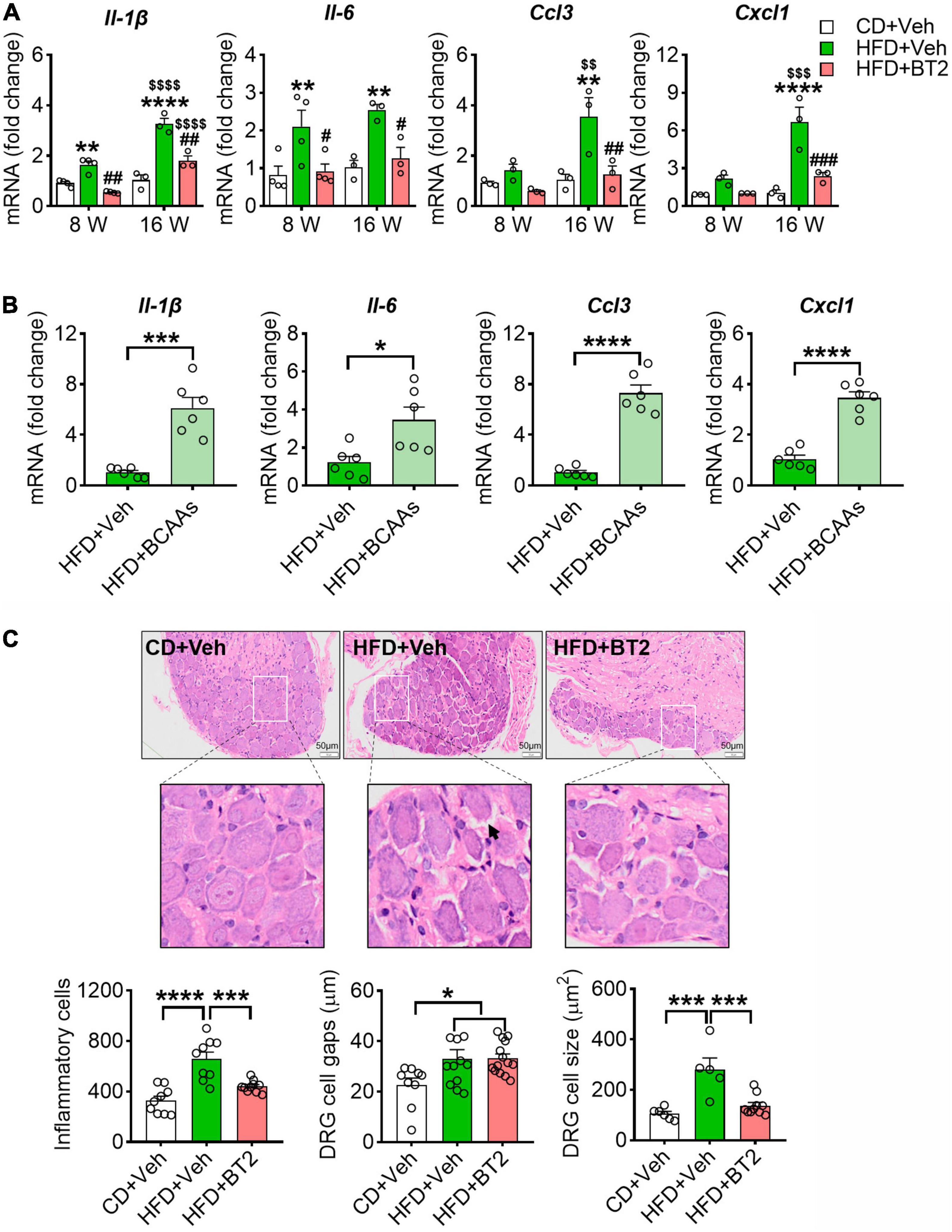
Figure 5. HFD-induced accumulation of BCAAs evokes a pro-Inflammatory response in DRG. (A) The relative mRNA expression of Il-1β, Il-6, Ccl3 and Cxcl1 (to β-actin) at 8 and 16 weeks in L4-L5 DRG of CD + Veh (n = 3-4), HFD + Veh (n = 3-4), and HFD + BT2 (n = 3-4) mice. Data are present as mean ± SEM. (B) The relative mRNA expression of Il-1β, Il-6, Ccl3 and Cxcl1 (to β-actin) on 8 weeks diet in L4-L5 DRG of HFD + Veh (n = 6) and HFD + BCAAs (n = 6) mice. (C) Representative images of HE-stained L4-L5 DRG section under 16 weeks of diet in CD + Veh, HFD + Veh, and HFD + BT2 mice. 200X magnification, bar indicating 50 μm. The cell gaps, area of ganglion cells, and number of inflammatory cells in L4-L5 DRG on 16 weeks diet of CD + Veh (n = 6–9), HFD + Veh (n = 5–11), and HFD + BT2 (n = 10–14). Data are present as mean ± SEM. *P < 0.05, **P < 0.01, ***P < 0.005, and ****P < 0.001 for HFD + Veh vs. CD + Veh or indicated comparisons; #P < 0.05, ##P < 0.01 and ###P < 0.005 for HFD + Veh vs. HFD + BT2. $$P < 0.01, $$$P < 0.005 and $$$$P < 0.001 for HFD + Veh at 8 weeks vs. HFD + Veh at 16 weeks, HFD + BT2 at 8 weeks vs. HFD + BT2 at 16 weeks.
Discussion
In the present study, we demonstrated that HFD-induced mechanical and thermal hyperalgesia is couple with a defective BCAA catabolism in DRG. This hyperalgesic phenotype could be exacerbated by supply of extra BCAAs or mitigated by promotion of BCAAs catabolism via BT2 treatment. In addition, our results suggest that BCAAs-regulated inflammatory status in DRG is responsible for the HFD-related pain behaviors. These findings, therefore, not only reveal metabolic underpinnings for the pathogenesis of HFD-related hyperalgesia but also offer potential targets for developing diet-based therapy of chronic pain.
How obesity regulates pain manifestation is still under debate (14). The findings in human studies comparing nociception in obese and lean individuals are seemingly paradoxical; some studies reporting lower thresholds (15, 16), while others reporting obesity-associated hypoalgesia (17). In HFD-fed rodent models, studies find either decreased response thresholds or no alterations under innocuous mechanical stimuli (18–20), and for the noxious thermal stimuli, studies present longer latencies, unaltered responses, or hyperalgesia in comparison to lean controls (21–23). In most studies using subacute and chronic pain models, including subcutaneous inflammation, arthritis and perineural inflammation, decreased thresholds and/or prolonged pain manifestations were reported in obesity models (24–26). In the present study, we found that HFD-fed obese mice displayed significant mechanical allodynia and thermal hyperalgesia, supporting the notion that obesity is associated with abnormal pain manifestation. In our opinion, in addition to genetic factors of different mouse strains, the time course of the pathology might account for some of the discrepancies observed across studies, because that a prolonged inflammation could lead to structural/functional alterations in the peripheral nerves and potentially blunt the perception of pain (2).
Accumulating evidence shows that circulating BCAA levels are elevated in obese humans and rodents, emerging as a predictor for the future risk of diabetes (27). The elevated BCAA levels in obese and/or diabetic individuals could be attributed to defective BCAA catabolism, as corroborate by the findings that BCAA catabolic enzymes are decreased in the obese ob/ob mice and fa/fa rat as well as obese humans (28), and that increasing BCAA catabolism by BT2 attenuates insulin resistance in ob/ob mice (13). The exact mechanisms responsible for the obesity-related BCAA dysregulation remain poorly understood, but may be attributed to the following two reasons: 1) the impaired central nerves system insulin signaling under obesity (28); 2) HFD-induced hyperglycemia which inhibits CREB-stimulated KLF15 transcription resulting in downregulation of enzymes in the BCAA catabolism pathway (29). In turn, the increased circulating BCAA levels experienced by obese individuals may lead to hyperactivation of the mTOR pathway that worsens systemic insulin resistance and KLF15 transcription, thus forming a vicious cycle.
Our study identified BCAA catabolism in DRG as a key regulatory point through which HFD modulates pain sensitivity. Unlike most of the amino acids, which are degraded in the liver, BCAAs are oxidized extensively in extra-hepatic tissue, although the significance of such a metabolic pattern has not been completely understood (5). The rate-limiting step in BCAA catabolism is BCKA decarboxylation in the mitochondria catalyzed by the BCKDH complex. PP2Cm is responsible for the dephosphorylation of the E1a subunit (Ser293) of the BCKDH, and hence activation of the complex (30). Here, we report that 8 weeks of HFD feeding impairs BCAA catabolism in mouse DRG, as evidenced by decreased protein expression of PPC2m and increased phosphorylation of BCKDH, and the resulted higher level of BCAAs in DRG is closely correlated with the sensitivity of mice to mechanical and thermal stimuli. Moreover, the effects of HFD on pain behaviors can be overridden by promotion of BCAA catabolism and exacerbated by supply of extra BCAAs, our results thereby indicate an emerging link between BCAA homeostasis in DRG and pain sensitivity. Similar downregulation of BCAA catabolism has also been reported in subcutaneous adipose of the HFD-fed mice (31). However, the current study did not address the mechanism by which HFD impairs BCAA catabolism. It could be due to HFD-induced elevation of blood glucose, for that high glucose has been shown to inhibit CREB stimulated KLF15 transcription resulting in downregulation of enzymes in the BCAA catabolism pathway (29). Further study is required to unveil the underlying regulatory machinery.
In line with a prevailing notion that obesity is a low-grade inflammatory condition (32), we found increased expression of pro-inflammatory cytokines/chemokines in mouse DRGs after 8 weeks of HFD feeding. It has been reported that Il-1β and Ccl3 act as key neuroinflammatory regulators that directly increase the excitability of primary sensory neurons, prolong neuroinflammation and promote activation of the downstream cellular oxidative stress, facilitating neuropathic pain behavior (33–36). Cxcl1 can promote central sensitization which may also lead to chronic pain (37). Intriguingly, our results show that BCAA homeostasis plays a vital role in modulation of these changes. Along with reduced pain manifestation, promoting of BCAA catabolism by BT2 suppressed the pro-inflammatory status in DRG, while supplementation of BCAAs on top of HFD further enhanced the expressions of these cytokines/chemokines. Recent studies have suggested that increased BCAA abundance could activate mTORC1, upregulate the NF-κB signaling pathway, and boost the release of pro-inflammatory cytokines (38). Excessive BCAAs could also induce ROS production, and subsequently activate the Nod-like receptor pyrin domain containing 3 (NLRP3) inflammasome to produce IL-1β and IL-18 (39, 40). However, our study does not exclude the possibility that other forms of BCAAs-related damage also contributed to the pathogenesis, such as oxidative stress, calcium overload, and lipid peroxidation toxicity (41, 42). Nevertheless, our data propose a previously unappreciated effect of BCAA homeostasis on DRG inflammation and obese/HFD related pain hypersensitivity.
Conclusion
The present study demonstrates that defective BCAA catabolism in DRG facilitates HFD-induced pain hypersensitivity by triggering inflammation. The present study unveils a critical role of BCAA catabolism in HFD-induced hyperalgesic phenotype, which may serve as a basis for the pathogenesis, as well as a target for therapy.
Data Availability Statement
The raw data supporting the conclusions of this article will be made available by the authors, without undue reservation.
Ethics Statement
The animal study was reviewed and approved by Animal Ethics Committee of West China Hospital, Sichuan University, China.
Author Contributions
TL and PL conceived the project idea, design the protocol, and wrote the manuscript. NL and KL performed the experiments. NL, KL, HX, and KT performed the data analysis. All authors contributed to the article and approved the submitted version.
Funding
This study was supported in part by grants from the National Natural Science Foundation of China (81801117, 81970715, and 81971806), the National Key Research and Development Program of China (2020YFC2005601), the Key Research Program of National Clinical Research Center for Geriatrics (Z20191004), the Key Research and Development Program of Sichuan Province (22ZDYF2138 and 22ZDYF2649), the Innovation Spark Project of Sichuan University (2018SCUH0065), and the 1.3.5 Project for Disciplines of Excellence, West China Hospital, Sichuan University (ZYGD18014).
Conflict of Interest
The authors declare that the research was conducted in the absence of any commercial or financial relationships that could be construed as a potential conflict of interest.
Publisher’s Note
All claims expressed in this article are solely those of the authors and do not necessarily represent those of their affiliated organizations, or those of the publisher, the editors and the reviewers. Any product that may be evaluated in this article, or claim that may be made by its manufacturer, is not guaranteed or endorsed by the publisher.
Acknowledgments
We thank Siqi Bai and Chengdu Lilai Biotechnology Co., Ltd., for technical assistance in H&E staining.
References
1. Piche ME, Tchernof A, Despres JP. Obesity Phenotypes, diabetes, and cardiovascular diseases. Circ Res. (2020) 126:1477–500. doi: 10.1161/CIRCRESAHA.120.316101
2. Marques Miranda C, de Lima Campos M, Leite-Almeida H. Diet, body weight and pain susceptibility – a systematic review of preclinical studies. Neurobiol Pain. (2021) 10:100066. doi: 10.1016/j.ynpai.2021.100066
3. Brandao AF, Bonet IJM, Pagliusi M Jr., Zanetti GG, Pho N, Tambeli CH, et al. Physical activity induces nucleus accumbens genes expression changes preventing chronic pain susceptibility promoted by high-fat diet and sedentary behavior in mice. Front Neurosci. (2019) 13:1453. doi: 10.3389/fnins.2019.01453
5. Neinast MD, Jang C, Hui S, Murashige DS, Chu Q, Morscher RJ, et al. Quantitative analysis of the whole-body metabolic fate of branched-chain amino acids. Cell Metab. (2019) 29:417–29.e4. doi: 10.1016/j.cmet.2018.10.013
6. Newgard CB. Interplay between lipids and branched-chain amino acids in development of insulin resistance. Cell Metab. (2012) 15:606–14. doi: 10.1016/j.cmet.2012.01.024
7. Newgard CB, An J, Bain JR, Muehlbauer MJ, Stevens RD, Lien LF, et al. A branched-chain amino acid-related metabolic signature that differentiates obese and lean humans and contributes to insulin resistance. Cell Metab. (2009) 9:311–26. doi: 10.1016/j.cmet.2009.02.002
8. Rojas DR, Kuner R, Agarwal N. Metabolomic signature of type 1 diabetes-induced sensory loss and nerve damage in diabetic neuropathy. J Mol Med (Berl). (2019) 97:845–54. doi: 10.1007/s00109-019-01781-1
9. Cosentino RG, Churilla JR, Josephson S, Molle-Rios Z, Hossain MJ, Prado WL, et al. Branched-chain amino acids and relationship with inflammation in youth with obesity: a randomized controlled intervention study. J Clin Endocrinol Metab. (2021) 106:3129–39. doi: 10.1210/clinem/dgab538
10. Kelly B, Pearce EL. Amino assets: how amino acids support immunity. Cell Metab. (2020) 32:154–75. doi: 10.1016/j.cmet.2020.06.010
11. Ou W, Liang Y, Qin Y, Wu W, Xie M, Zhang Y, et al. Hypoxic acclimation improves cardiac redox homeostasis and protects heart against ischemia-reperfusion injury through upregulation of O-glcnacylation. Redox Biol. (2021) 43:101994. doi: 10.1016/j.redox.2021.101994
12. Obayashi M, Shimomura Y, Nakai N, Jeoung NH, Nagasaki M, Murakami T, et al. Estrogen controls branched-chain amino acid catabolism in female rats. J Nutr. (2004) 134:2628–33. doi: 10.1093/jn/134.10.2628
13. Zhou M, Shao J, Wu CY, Shu L, Dong W, Liu Y, et al. Targeting BCAA catabolism to treat obesity-associated insulin resistance. Diabetes. (2019) 68:1730–46. doi: 10.2337/db18-0927
14. Okifuji A, Hare BD. The association between chronic pain and obesity. J Pain Res. (2015) 8:399–408. doi: 10.2147/JPR.S55598
15. Zhang Y, Zhang S, Gao Y, Tan A, Yang X, Zhang H, et al. Factors associated with the pressure pain threshold in healthy Chinese men. Pain Med. (2013) 14:1291–300. doi: 10.1111/pme.12158
16. Okifuji A, Donaldson GW, Barck L, Fine PG. Relationship between fibromyalgia and obesity in pain, function, mood, and sleep. J Pain. (2010) 11:1329–37. doi: 10.1016/j.jpain.2010.03.006
17. Torensma B, Oudejans L, van Velzen M, Swank D, Niesters M, Dahan A. Pain sensitivity and pain scoring in patients with morbid obesity. Surg Obes Relat Dis. (2017) 13:788–95. doi: 10.1016/j.soard.2017.01.015
18. Bonomo RR, Cook TM, Gavini CK, White CR, Jones JR, Bovo E, et al. Fecal transplantation and butyrate improve neuropathic pain, modify immune cell profile, and gene expression in the pns of obese Mice. Proc Natl Acad Sci USA. (2020) 117:26482–93. doi: 10.1073/pnas.2006065117
19. Cooper MA, O’Meara B, Jack MM, Elliot D, Lamb B, Khan ZW, et al. Intrinsic activity of C57bl/6 substrains associates with high-fat diet-induced mechanical sensitivity in mice. J Pain. (2018) 19:1285–95. doi: 10.1016/j.jpain.2018.05.005
20. Gavini CK, Bookout AL, Bonomo R, Gautron L, Lee S, Mansuy-Aubert V. Liver X receptors protect dorsal root ganglia from obesity-induced endoplasmic reticulum stress and mechanical allodynia. Cell Rep. (2018) 25:271–7.e4. doi: 10.1016/j.celrep.2018.09.046
21. Davidson EP, Coppey LJ, Kardon RH, Yorek MA. Differences and similarities in development of corneal nerve damage and peripheral neuropathy and in diet-induced obesity and type 2 diabetic rats. Invest Ophthalmol Vis Sci. (2014) 55:1222–30. doi: 10.1167/iovs.13-13794
22. Guilford BL, Ryals JM, Wright DE. Phenotypic changes in diabetic neuropathy induced by a high-fat diet in diabetic C57bl/6 mice. Exp Diabetes Res. (2011) 2011:848307. doi: 10.1155/2011/848307
23. Xu L, Tang D, Guan M, Xie C, Xue Y. Effect of high-fat diet on peripheral neuropathy in C57bl/6 mice. Int J Endocrinol. (2014) 2014:305205. doi: 10.1155/2014/305205
24. Loredo-Perez AA, Montalvo-Blanco CE, Hernandez-Gonzalez LI, Anaya-Reyes M, Fernandez Del Valle-Laisequilla C, Reyes-Garcia JG, et al. High-fat diet exacerbates pain-like behaviors and periarticular bone loss in mice with CFA-induced knee arthritis. Obesity (Silver Spring). (2016) 24:1106–15. doi: 10.1002/oby.21485
25. Song Z, Xie W, Chen S, Strong JA, Print MS, Wang JI, et al. High-fat diet increases pain behaviors in rats with or without Obesity. Sci Rep. (2017) 7:10350. doi: 10.1038/s41598-017-10458-z
26. Song Z, Xie W, Strong JA, Berta T, Ulrich-Lai YM, Guo Q, et al. High-fat diet exacerbates postoperative pain and inflammation in a sex-dependent manner. Pain. (2018) 159:1731–41. doi: 10.1097/j.pain.0000000000001259
27. Neinast M, Murashige D, Arany Z. Branched chain amino acids. Annu Rev Physiol. (2019) 81:139–64. doi: 10.1146/annurev-physiol-020518-114455
28. Shin AC, Fasshauer M, Filatova N, Grundell LA, Zielinski E, Zhou JY, et al. Brain insulin lowers circulating BCAA levels by inducing hepatic BCAA catabolism. Cell Metab. (2014) 20:898–909. doi: 10.1016/j.cmet.2014.09.003
29. Shao D, Villet O, Zhang Z, Choi SW, Yan J, Ritterhoff J, et al. Glucose promotes cell growth by suppressing branched-chain amino acid degradation. Nat Commun. (2018) 9:2935. doi: 10.1038/s41467-018-05362-7
30. Li T, Zhang Z, Kolwicz SC Jr., Abell L, Roe ND, Kim M, et al. Defective branched-chain amino acid catabolism disrupts glucose metabolism and sensitizes the heart to ischemia-reperfusion injury. Cell Metab. (2017) 25:374–85. doi: 10.1016/j.cmet.2016.11.005
31. Estrada-Alcalde I, Tenorio-Guzman MR, Tovar AR, Salinas-Rubio D, Torre-Villalvazo I, Torres N, et al. metabolic fate of branched-chain amino acids during adipogenesis, in adipocytes from obese mice and C2c12 myotubes. J Cell Biochem. (2017) 118:808–18. doi: 10.1002/jcb.25755
32. Pereira SS, Alvarez-Leite JI. Low-grade inflammation, obesity, and diabetes. Curr Obes Rep. (2014) 3:422–31. doi: 10.1007/s13679-014-0124-9
33. Sommer C, Kress M. Recent findings on how proinflammatory cytokines cause pain: peripheral mechanisms in inflammatory and neuropathic hyperalgesia. Neurosci Lett. (2004) 361:184–7. doi: 10.1016/j.neulet.2003.12.007
34. Scholz J, Woolf CJ. The neuropathic pain triad: neurons, immune cells and glia. Nat Neurosci. (2007) 10:1361–8. doi: 10.1038/nn1992
35. Lee S, Zhang J. Heterogeneity of macrophages in injured trigeminal nerves: cytokine/chemokine expressing vs. phagocytic macrophages. Brain Behav Immun. (2012) 26:891–903. doi: 10.1016/j.bbi.2012.03.004
36. Saika F, Kiguchi N, Kobayashi Y, Fukazawa Y, Kishioka S. Cc-chemokine ligand 4/macrophage inflammatory protein-1beta participates in the induction of neuropathic pain after peripheral nerve injury. Eur J Pain. (2012) 16:1271–80. doi: 10.1002/j.1532-2149.2012.00146.x
37. Zychowska M, Rojewska E, Pilat D, Mika J. The role of some chemokines from the CXC subfamily in a mouse model of diabetic neuropathy. J Diabetes Res. (2015) 2015:750182. doi: 10.1155/2015/750182
38. Ye Z, Wang S, Zhang C, Zhao Y. Coordinated modulation of energy metabolism and inflammation by branched-chain amino acids and fatty acids. Front Endocrinol (Lausanne). (2020) 11:617. doi: 10.3389/fendo.2020.00617
39. Zhenyukh O, Civantos E, Ruiz-Ortega M, Sanchez MS, Vazquez C, Peiro C, et al. High concentration of branched-chain amino acids promotes oxidative stress, inflammation and migration of human peripheral blood mononuclear cells via mtorc1 activation. Free Radic Biol Med. (2017) 104:165–77. doi: 10.1016/j.freeradbiomed.2017.01.009
40. Deng Y, Xie M, Li Q, Xu X, Ou W, Zhang Y, et al. Targeting mitochondria-inflammation circuit by beta-hydroxybutyrate mitigates HFPEF. Circ Res. (2021) 128:232–45. doi: 10.1161/CIRCRESAHA.120.317933
41. Zhenyukh O, Gonzalez-Amor M, Rodrigues-Diez RR, Esteban V, Ruiz-Ortega M, Salaices M, et al. Branched-chain amino acids promote endothelial dysfunction through increased reactive oxygen species generation and inflammation. J Cell Mol Med. (2018) 22:4948–62. doi: 10.1111/jcmm.13759
Keywords: obesity, high-fat diet, pain behaviors, branched-chain amino acids, inflammation
Citation: Lian N, Luo K, Xie H, Kang Y, Tang K, Lu P and Li T (2022) Obesity by High-Fat Diet Increases Pain Sensitivity by Reprogramming Branched-Chain Amino Acid Catabolism in Dorsal Root Ganglia. Front. Nutr. 9:902635. doi: 10.3389/fnut.2022.902635
Received: 23 March 2022; Accepted: 26 April 2022;
Published: 12 May 2022.
Edited by:
Fenghua Sun, The Education University of Hong Kong, Hong Kong SAR, ChinaReviewed by:
Zhihua Yi, Nanchang University, ChinaKerry Loomes, The University of Auckland, New Zealand
Copyright © 2022 Lian, Luo, Xie, Kang, Tang, Lu and Li. This is an open-access article distributed under the terms of the Creative Commons Attribution License (CC BY). The use, distribution or reproduction in other forums is permitted, provided the original author(s) and the copyright owner(s) are credited and that the original publication in this journal is cited, in accordance with accepted academic practice. No use, distribution or reproduction is permitted which does not comply with these terms.
*Correspondence: Tao Li, c2N1dGFvbGkxOTgxQHNjdS5lZHUuY24=; Peilin Lu, cGVpbGlubHl1QHFxLmNvbQ==
†These authors have contributed equally to this work and share first authorship