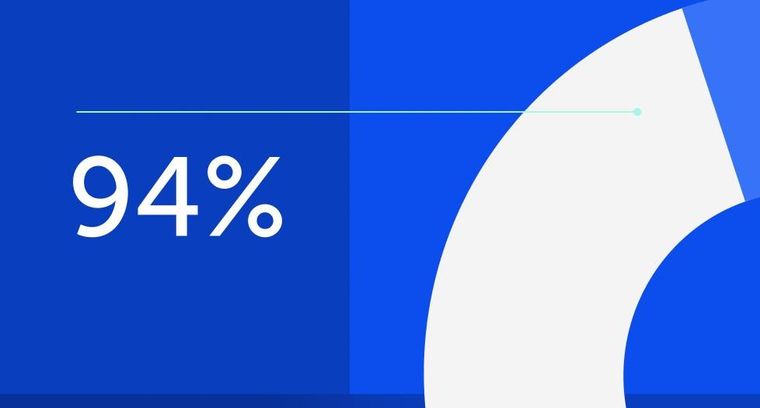
94% of researchers rate our articles as excellent or good
Learn more about the work of our research integrity team to safeguard the quality of each article we publish.
Find out more
ORIGINAL RESEARCH article
Front. Nutr., 22 August 2022
Sec. Nutrition and Microbes
Volume 9 - 2022 | https://doi.org/10.3389/fnut.2022.902159
This article is part of the Research TopicNovel Developments for Promoting Health Through Microbiota ModulationView all 16 articles
Establishing the relationship between gut microbiota and host health has become a main target of research in the last decade. Human gut microbiota-associated animal models represent one alternative to human research, allowing for intervention studies to investigate causality. Recent cohort and in vitro studies proposed an altered gut microbiota and lactate metabolism with excessive H2 production as the main causes of infant colic. To evaluate H2 production by infant gut microbiota and to test modulation of gut colonizer lactose- and lactate-utilizer non-H2-producer, Cutibacterium avidum P279, we established and validated a gnotobiotic model using young germ-free rats inoculated with fecal slurries from infants younger than 3 months. Here, we show that infant microbiota-associated (IMA) rats inoculated with fresh feces from healthy (n = 2) and colic infants (n = 2) and fed infant formula acquired and maintained similar quantitative and qualitative fecal microbiota composition compared to the individual donor’s profile. We observed that IMA rats excreted high levels of H2, which were linked to a high abundance of lactate-utilizer H2-producer Veillonella. Supplementation of C. avidum P279 to colic IMA rats reduced H2 levels compared to animals receiving a placebo. Taken together, we report high H2 production by infant gut microbiota, which might be a contributing factor for infant colic, and suggest the potential of C. avidum P279 in reducing the abdominal H2 production, bloating, and pain associated with excessive crying in colic infants.
Human cohort studies often reveal associations between the gut microbiota and host health, but valid in vitro and in vivo models are needed to provide mechanistic understanding (1). Animal models allow experimental procedures otherwise limited in humans due to ethical and safety concerns or due to limited accessibility to biological samples, while providing a controlled environment and standardized genetic background. Inoculation of germ-free animals with fecal microbiota from human donors has been used successfully to study the role of gut microbiota in various pathophysiological conditions. For example, Crouzet et al. (2) showed that the transfer of fecal microbiota from irritable bowel syndrome patients in germ-free rats was associated with increased abdominal sensitivity, compared to animals inoculated with fecal microbiota from healthy human subjects. Increased adiposity was also observed in germ-free mice that received fecal microbiota from obese individuals compared to mice inoculated with the microbiota of lean individuals (3). In a more recent study, germ-free mice inoculated with feces from patients suffering from inflammatory bowel disease showed a dysregulated immune response compared to mice receiving feces from healthy individuals (4). However, validated gnotobiotic models to study young infant gut microbiota are still lacking.
Infants are born with low numbers of microbes in their gut, and first colonizers are mainly transferred from the mother’s skin, mouth, vagina, and breast microbiota (5–7). The gut microbiota of breast- and formula-fed newborns is characterized by a high abundance of lactate-producing bacteria (LPB), mainly Bifidobacterium, Streptococcus, Enterococcus, and Enterobacteria (8–10). Lactate is an important intermediate metabolite that, if not further metabolized by lactate-utilizing bacteria (LUB), might accumulate leading to acidosis, neurotoxicity, and cardiac arrhythmia (11–13). The LUB community in infants is mainly composed of propionate-producing Propionibacterium/Cutibacterium and Veillonella spp., together with some species of the genus Bacteroides and with a lower abundance of butyrate-producing bacteria like Anaerostipes spp., Anaerobutyricum hallii, and Eubacterium limosum, and sulfate-reducing bacteria (SRB) like Desulfovibrio (8, 14–17). Hydrogen (H2), which is formed by Anaerostipes spp., A. hallii, and Veillonella spp., can accumulate and serve as a substrate for H2S formation by SRB (16, 15). Imbalances in lactate, H2, and H2S metabolism may induce flatulence and bloating and associated pain in colic infants (defined as infants younger than 5 months crying more than 3 h per day, for at least 3 days in a week) (16, 18–21). Several studies have identified higher breath H2 excretion in colic infants compared to healthy controls, and positively correlated breath H2 with crying time (22–24). Pham et al. (16) reported higher ratios of H2-producing to H2-utilizing LUB in crying infants compared to healthy controls at 3 months of age, and higher colonization by H2-producer A. hallii in colic infants compared to healthy controls at 2 weeks of age.
Because lactate is an important substrate for H2 production by LUB and accumulation of lactate and H2 might be contributing factors for infant colic (IC), supplementation of non-H2-producing LUB has been suggested as a potential strategy for IC alleviation. Pham et al. (16) demonstrated that infant isolates of non-H2-producing LUB E. limosum reduced H2 production by LUB Veillonella ratii when grown in co-culture in lactate-containing media. Alternatively, non-H2-producing LUB Cutibacterium avidum strains of infant origin have shown to persist, reduce lactate, and compete with Veillonella when supplemented in a recently developed and validated continuous colonic fermentation model (PolyFermS platform), inoculated with immobilized fecal microbiota and mimicking proximal colon conditions of 2-month-old formula-fed infants (17, 25). Considering the previous observations, we hypothesize that Cutibacterium avidum P279, selected for its colonization ability and effects observed in in vitro infant colonic fermentations, can decrease H2 excretion in a rat model of colic infants and has therefore potential for the treatment of IC. In order to test this hypothesis, we (1) developed and validated a rat model based on the inoculation of young germ-free animals with fecal slurries from healthy and colicky young infants (< 3 months) and (2) investigated the impact on gut microbiota composition and activity of supplementation of C. avidum P279 to colic infant microbiota-associated (IMA) rats.
Fecal samples from two healthy infants (H1: 59 days old and H2: 92 days old) and two infants suffering from colic (C1: 71 days old and C2: 68 days old) who satisfied Rome IV criteria (20) were used for inoculation of germ-free Fischer 344 rats (Figure 1). Inclusion criteria were as follows: good health, a full-term delivery (gestation time 37–42 weeks), normal birth weight (female: 2.7–5.0 kg; male: 2.9– 5.2 kg), and an exclusively milk-based diet. Infants with a history of antibiotic treatment were excluded. Healthy infants (H1 and H2) did not present gastrointestinal (GIT) disorders, while colic donors (C1 and C2) complied with the following criteria: i) caregiver reported infant crying or fussing, for more than 3 per day during 3 h or more days in the last week to the nurse; ii) total 24-h crying confirmed to be 3 or more h measured prospectively by caregivers; and iii) no evidence of failure to thrive (20). Infants were born by vaginal delivery and living in Zürich (Switzerland). Healthy donors were breastfed, while colic donors received infant formula complementary to breast-feeding. Parents of donors were asked to provide a fresh fecal sample under highly controlled conditions (presented below) to a study nurse, and the data and material were subsequently anonymized. Anonymized biological material is not under the scope of the Swiss Federal Human Research Act (Art. 2 para. 2 let. b and c), which excluded this study from requiring ethical approval. Fecal samples (2 - 4 g) were collected by the parents by scraping from diapers with a sterile spatula into 50-mL sterile conical-shaped polypropylene tubes and immediately placed into closed airtight jars (Mitsubishi AnaeroPack, Thermo Fisher Diagnostics AG, Pratteln, Switzerland) containing one AnaeroGen sachet (Oxoid, Thermo Fisher Diagnostic AG). Samples were kept under cold conditions until they were picked up from the family house and transported into a Styrofoam box with frozen gel packs to INRAE - Theix (France). Fecal slurries from healthy and colic donors were inoculated in germ-free rats after 24–36 h of fecal deposition.
Figure 1. Diagram of the experimental design. Fecal samples from two healthy (H1 and H2) and two colic infants (C1 and C2) were used for inoculation of germ-free Fischer 344 rats (n = 39). The microbiota was allowed to establish for 10–14 days before baseline (T0) measurements. Rats inoculated with donors C1 and C2 were divided into treatment group (gavaged daily with 109 colony forming units of Cutibacterium avidum P279 in 350 μL of sterile skim milk) and control group (received 350 μL of sterile skim milk). End-point measurements (T1) were obtained on days 26–28. Rats were sacrificed at 7–8 weeks of age. Rats were fed a gamma-irradiated infant formula to mimic an infant diet and supplemented with 1–3 pellets per day of standard diet for growing animals. All rats were given free access to sterile water.
Cutibacterium avidum P279 was isolated from feces of a 13 week-old infant and selected for its colonization ability observed in in vitro infant colonic fermentations, and was obtained from the strain collection of the Laboratory of Food Biotechnology (ETH-Zürich) and used for supplementation of colic IMA rats (26). C. avidum P279 was grown in yeast extract sodium lactate medium (YEL) consisting of 1% (w/v) trypticase soy broth without dextrose (Becton Dickinson AG, Allschwil, Switzerland), 1% (w/v) yeast extract (Merck, Darmstadt, Germany), 117 mM sodium DL-lactate (60% v/v syrup, Central Drug House, New Delhi, India), 0.025% (w/v) KH2PO4 (VWR International AG, Dietikon, Switzerland), and 0.0005% (w/v) MnSO4 (Sigma-Aldrich, Buchs, Switzerland). Glycerol stocks stored at -80°C were reactivated on agar plates and incubated in airtight jars (Mitsubishi AnaeroPack, Thermo Fisher Diagnostics) containing one AnaeroGen sachet (Oxoid, Thermo Fisher Diagnostics AG) at 37°C for 5 days. Single colonies were inoculated into liquid YEL and incubated for 48 h at 37°C. After incubation, cultures were centrifuged for 10 min at 7,000 g, and pellets were resuspended in the same volume of sterile reconstituted skim milk (10% w/v). Aliquots of 350 μL (containing approximately 109 CFU) were transferred into cryovials (Huber Co, Reinach, Switzerland). For supplementation of the control group, aliquots of 350 μL of sterile reconstituted skim milk (10% w/v) were prepared in cryovials (Huber Co). Cryovials were snap-frozen in liquid nitrogen and stored at -80°C for a maximum of 5 weeks until supplementation.
Germ-free Fischer 344 rats bred at INRAE-Theix facilities were kept in sterile isolators with positive pressure over the entire trial period as described before (2). All experimental protocols were approved by the Local Institutional Animal Care and Use Committee (Approval reference N° 2019101512192520).
Rats were weaned at 3–4 weeks of age and inoculated with 0.2 mL of infant fecal slurries from single donors by a single intragastric gavage [100-fold dilution of fecal sample in anaerobic mineral solution (27) that was composed of 7.5% v/v mineral solution I (0.3% w/v K2HPO4), 7.5% v/v mineral solution II [0.3% w/v K2HPO4; 0.6% w/v NaCl; 0.6% w/v (NH4)2SO4; 0.06% w/v MgSO4; 0.06% w/v CaCl2], 0.01% w/v resazurin, 0.4% w/v NaHCO3, 0.05% w/v cysteine hydrochloride, and distilled water; all chemicals were obtained from Sigma-Aldrich]. IMA rats were placed in cages housing two animals. They were fed with gamma-irradiated infant formula (Aptamil Pre, Milupa GmbH, Germany) reconstituted in sterile water (13% w/v; approximately 80 mL per day during the first 2 weeks and afterwards 100 mL per day until the end of experimentation) mimicking infant diet. The commercial formula contained 8% (w/v) galacto-oligosaccharides (GOS) and fructo-oligosaccharides (FOS) (ratio of 9:1). Two to three pellets of the standard diet for growing rats (A03/R03, SAFE, France) per day were also supplied to each cage (after 2 weeks from weaning for healthy IMA rats and during entire intervention period for colic IMA rats) in order to prevent bloating due to the lack of indigestible fibers and high content of lactose of the infant formula. All rats were given free access to sterile water.
Ten germ-free rats were inoculated with fecal slurries from healthy donors H1 (n = 5) and H2 (n = 5), and were allocated to two different isolators (Figure 1). Seventeen rats inoculated with fecal slurries from colic donor C1 were divided into two isolators housing a control group (n = 8) and a treatment group (n = 9). Fecal slurries from colic donor C2 were used to inoculate 12 rats housed in two isolators (control group n = 6 and treatment group n = 6).
After inoculation, the microbiota was established for a period of 10–14 days before further measurements (Figure 1).
Fecal samples from rats were collected directly from the anus by manual perineal stimulation and were immediately used for microbial enumeration (see below) or stored at –80°C until further processing. Feces collection and fecal microbial enumeration were done 10–14 days from inoculation (baseline measurements) and at the end of experimentation (Figure 1). After baseline measurements, rats inoculated with fecal microbiota from donors C1 and C2 were gavaged daily for 12–15 days with 109 CFU of C. avidum P279 in 350 μL of sterile skim milk (treatment groups) or with the same amount of sterile milk alone (control groups). Rats were sacrificed at 7-8 weeks of age, and the cecum contents were harvested for analyzing fermentation metabolites.
Freshly voided feces from donors and rats were serially 10-fold diluted (wet w/v) in an anaerobic mineral solution. Total anaerobes, and non-SRB-LUB and SRB-LUB communities were enumerated by most probable number estimation (MPN) in a three-replicate design (28). Total anaerobes in all donors and rat feces were enumerated in an O2-free CO2 complex medium containing clarified rumen fluid (29). Oxygen-free CO2 basal medium with L-lactate (35 mM) as the sole energy source and Postgate E medium were used for the enumeration of non-SRB-LUB and SRB-LUB communities, respectively (30, 31). After incubation for 5 days at 37°C, lactate concentration in supernatant of culture tubes of each inoculated dilution (10–5 to 10–10) was determined by high-performance liquid chromatography with refractive index detection (HPLC-RI) (described below). Cultures with a final lactate concentration below 25 mM (lactate consumption of at least 10 mM) were considered positive for non-SRB-LUB bacteria (32). Cultures on Postgate E medium were inoculated with 0.3 mL of fecal dilutions (10–2 to 10–5) and were considered positive for MPN determination when a black precipitate of FeS was visually observed.
Genomic DNA was extracted from infant and rat stool samples (200 mg) and from MPN-positive cultures (2 mL) in Postgate E and basal medium with L-lactate, using the Fast DNA SPIN kit for soil (MP Biomedicals, Illkirch, France) according to the manufacturer’s instructions. The extracted DNA was then used to amplify regions of the 16S rDNA gene by qPCR using specific primers (Supplementary Table 1). Reactions were performed using LightCycler 480 Real-Time PCR System (Roche Diagnostics, Rotkreuz, Switzerland) and the SensiFAST SYBR No-ROX 2X mix (5 μL), and 500 nM primers (Biolab Scientifics Instruments SA, Chatel-St-Denis, Switzerland) in a total reaction volume of 10 μL. Thermal cycling started with an initial denaturation step at 95°C for 3 min, followed by 40 cycles of a two-step PCR consisting of a hold at 95°C for 5 s and at 60°C for 60 s. The cycle threshold (Ct) values were obtained using automatic baseline and threshold settings provided by the LightCycler 480 Software, Version 1.5. Samples were analyzed in duplicate. To generate standards, PCR amplicons were cloned into the pGEM-T Easy Vector and heterologously expressed in E. coli according to instructions of the supplier (Promega AG, Dübendorf, Switzerland). Standard curves were prepared from 10-fold dilutions of linearized plasmids harboring the target gene of interest. Bacterial groups abundant in the infant feces were amplified using primers listed in Supplementary Table 1. Melting curve analysis was conducted to confirm amplification specificity. To estimate cell counts for Cutibacterium, gene copies were corrected for multiple copies (n = 3) of 16S rRNA genes (33).
The bacterial profiles in fecal samples obtained from donors and rats and in pellets from cultures in Postgate E and basal medium with lactate were determined using tag-encoded 16S rRNA gene Miseq-based (Illumina, San Diego, CA, United States) high-throughput sequencing, as presented before (17). Briefly, DNA concentration was standardized to 20 ng μL–1. The V3 region of the 16S rRNA gene was amplified using primers including adapters for the Nextera Index Kit, NXt_388_F, and NXt_518_R (Supplementary Table 1). One MiSeq flow cell and the V2 2 × 250 bp paired-end Nextera chemistry were used, and were supplemented with 20% of PhiX. Library preparation and sequencing were performed at the Genomic Diversity Center (ETH Zurich, Zurich, Switzerland). The raw datasets containing pair-ended reads with corresponding quality scores were merged and trimmed using settings as previously described (34). The minimum length of merged reads was 200 bp. Following analysis steps were done using Quantitative Insight Into Microbial Ecology (QIIME) open source software package (1.8.0 and 1.9.0) (35). Purging the datasets from chimeric reads and constructing de novo operational taxonomic units (OTUs) were conducted using the UPARSE pipeline (36) and the Greengenes database as a reference (37). Taxonomic assignment of OTUs at the genus level could not differentiate between Propionibacterium and Cutibacterium because the new taxonomy (a division of former Propionibacterium spp. in gen. nov. Cutibacterium including cutaneous species and Propionibacterium spp. including only dairy species) has not been updated in the reference database (38). Alpha-diversity was analyzed using observed species (species richness in individual samples) and Shannon index (species richness and evenness estimator, which increase when the number of species and evenness increase). The beta-diversity (compositional diversity between two samples) was analyzed as previously described using iterative subsampling (39). Permutational multivariate analysis of variance using distance matrices (PERMANOVA) based on 999 Monte Carlo simulations was used to analyze the differences in unweighted and weighted UNIFRAC distances between infant and IMA rat fecal samples from different donors. Linear discriminant analysis effect size (LEfSe) was applied to identify genera that differed significantly between IMA rats inoculated with fecal samples from the same donor, from treatment and control groups at baseline (T0) and after the intervention period (T1). LEfSe analysis was done with default parameters and a logarithmic linear discriminant analysis score threshold of two (40).
The concentration of acetate, propionate, butyrate, isobutyrate, isovalerate, formate, succinate, and lactate were determined in cecum content and in the supernatant of LUB enumeration media after centrifugation for 10 min at 13,000 g. The supernatant (500 μL) was filtered through a 0.45-μm membrane (Millipore AG, Zug, Switzerland) into glass HPLC vials (Infochroma, Hitachi LaChrome, Merck, Dietikon, Switzerland) and sealed with crimp caps. An HPLC (Hitachi LaChrome) equipped with a Security Guard Cartridges Carbo-H column (4 × 3 mm; Phenomenex Inc., Torrance, CA, United States), a Rezex ROA-Organic Acid H + column (8%, 300 × 7.8 mm; Phenomenex), and a refractive index detector (HPLC-RI) was used. The column was eluted with 10 mM H2SO4 (Fluka, Buchs, Switzerland) as a mobile phase at a flow rate of 0.4 mL min–1 at 25°C. All tested metabolites were quantified using external standards using EZChrom Elite software (version 3.3.2 SP2, Agilent Technologies, CA, United States).
The amount of H2 excreted by each rat was measured by housing a single animal in a respiratory chamber for 24 h (41). Measurements were done on days 27 and 28 for rats inoculated with feces from healthy donors (H1 and H2) and before (baseline measurements T0, 10–14 days from inoculation) and after treatment (end-point measurements T1, after 12-15 days of intervention) for rats inoculated with feces from colic donors (C1 and C2). H2 concentration in the chamber atmosphere was determined using a gas phase chromatograph (Microanalyzer DP, Quintron Instruments, Milwaukee, WI, United States).
Statistical analyses were done using SigmaPlot (Systat Software, San Jose, CA, United States) with statistical significance set at a p-value of less than 0.05. The Student’s t-test with two-tailed distribution was used, after testing for normal distribution using the Shapiro–Wilk test, to compare changes in the abundance of microbial taxa detected by qPCR (log10-transformed), cecum metabolites (mmol L–1) and H2 excretion (ppm) values between baseline and after intervention for the same IMA rat group and between control and treatment IMA rat groups after the intervention period. The Mann–Whitney test was used when data were not normally distributed.
Sequence information is available in the NCBI database, BioProject ID PRJNA590392.
The bacterial composition in feces of infant donors was analyzed using qPCR and 16S rRNA gene amplicon sequencing. Species richness in donors ranged from 10 to 46 observed species (Table 1). Bifidobacterium [Log 9.6 gene copies g feces–1, 70.5% relative abundance (rel. ab.)] and Bacteroides (Log 10.2 gene copies g feces–1, 13.4% rel. ab.) were the most abundant genera in donor H1, while Bifidobacterium dominated in donor H2 (Log 10.1 gene copies g feces–1, 96.4% rel. ab.) and C1 (Log 8.9 gene copies g feces–1, 80.3% rel. ab.) (Figure 2 and Table 1). Donor C2 fecal microbiota was dominated by Firmicutes (Log 9.1 gene copies g feces–1, 70.7% rel. ab.), mainly represented by Clostridium cluster I (Log 9.0 gene copies g feces–1). Enterobacteriaceae were detected in high abundance in donors H1, C1, and C2 (Log 8.0-8.8 gene copies g feces–1 and 11.6 - 29.1% rel. ab.) but at low concentrations in donor H2 (Log 3.8 gene copies g feces –1, < 0.0001% rel. ab.). Veillonella was identified in all donors at sub-dominant levels (Log 4.6-7.5 gene copies g feces –1, 0.01 - 0.3% rel. ab.) (Figure 2 and Table 1). The donor fecal samples used to inoculate IMA rats are representative of the highly variable taxonomic composition of the infant gut microbiota.
Table 1. Main bacterial communities in feces from infant donors and IMA rats at baseline and fermentative metabolites in cecal supernatant from IMA rats after euthanasia.
Figure 2. Bacterial community composition was determined by 16S rRNA gene amplicon sequencing in feces from infant donors and respective infant microbiota-associated (IMA) rats. For IMA rats, each column represents the average relative abundance. The relative abundance of taxa with an average relative abundance of >0.1% are depicted with the taxa nomenclature; lower abundant taxa (< 0.1%) are shown as Others.
Viable cell numbers in donor slurries used for inoculating germ-free rats were estimated by an MPN-based cultivation approach. Slurries from healthy donors had higher viable cell numbers at inoculation (H1: Log 11.5 cells g feces–1 and H2: Log 10.2 cells g feces–1) than colic donors (C1: Log 9.5 cells g feces–1 and C2: Log 9.6 cells g feces–1). After implantation, viable cell numbers of healthy and colic IMA rats were very similar, averaging Log 10.6 ± 0.5 cells g feces–1, while some differences were observed among donor feces using MPN (Table 1).
Quantification of bacterial groups in IMA rats by qPCR revealed higher levels of Firmicutes (Log 9.5–9.8 gene copies g feces–1) in rats compared to donors (Table 1). The levels of other bacterial taxa were donor-dependent and generally matched the composition of the donor microbiota. Bifidobacterium colonized H1, H2, and C1 rats at high abundance (Log 10.0–10.4 gene copies g feces–1) and C2 rats at a much lower level (Log 5.9 ± 0.4 gene copies g feces–1) similar to donor C2 (Log 6.5 gene copies g feces–1). Engraftment of Bacteroides in H1 rats (Log 10.7 ± 0.1 gene copies g feces–1) and H2 rats (Log 5.1 ± 0.3 gene copies g feces–1) also corresponded with donor fecal concentrations (H1: Log 10.2 gene copies g feces–1 and H2: Log 5.2 gene copies g feces–1). Bacteroides were enriched approximately 100-fold in C1 (Log 10.4 ± 0.1 gene copies g feces–1) and C2 rats (Log 9.4 ± 0.4 gene copies g feces–1) compared to respective donors (C1: Log 8.2 gene copies g feces–1 and C2: Log 7.1 gene copies g feces–1). Enterobacteriaceae were well maintained in H1, C1, and C2 rats, but reached a much higher number in H2 rats (Log 9.0 ± 0.2 gene copies g feces–1) than in donor H2 which had a very low number of Enterobacteriaceae (Log 3.8 gene copies g feces–1) compared to the other donors. Veillonella was much higher in H1 (Log 9.8 ± 0.2 gene copies g feces–1), C1 (Log 9.7 ± 0.2 gene copies g feces–1), and C2 (Log 8.6 ± 0.5 gene copies g feces–1) rats compared to the respective donors (H1: Log 6.1 gene copies g feces–1, C1: Log 4.6 gene copies g feces–1, and C2: Log 7.5 gene copies g feces–1) but not in H2 rats (Log 3.8 ± 0.4 gene copies g feces–1) compared to donor H2 (Log 5 gene copies g feces–1) (Table 1).
The number of observed species and Shannon indices were higher in rats compared to donors (Table 1). Except for C1 rats, fecal microbiota communities from IMA rats and respective donors were qualitatively similar, as indicated by the clustering of donor samples together with recipient rats when considering unweighted and weighted UniFrac distances in a principal component analysis (PCoA) plot (Supplementary Figure 1). When comparing groups of donors and respective recipient rats, cluster separation by donors was significant under unweighted and weighted UniFrac distances (PERMANOVA, p = 0.001) (Supplementary Figure 1).
Main shifts in the bacterial populations identified by gene amplicon sequencing were aligned with qPCR observations (Figure 2). Bacteroidaceae increased from 0.02% in donor C1 to 32.2% ± 14.8% in C1 rats and from 0.07% in donor C2 to 10.5% ± 8.4% in C2 rats. Colonization by Enterobacteriaceae was higher in feces of H2 rats (10.7% ± 3.5%) compared to donor H2 (< 0.01%) (Figure 2). Higher abundance of Veillonellaceae was also detected for H1 (15.4% ± 5.0%), C1 (12.9% ± 5.9%), and C2 rats (2.4% ± 1.6%) compared to respective donor levels (H1: 0.3%, C1: 0.1%, and C2: 0.2%) (Figure 2). In contrast, shifts in the relative abundance of Bifidobacteriaceae detected by sequencing were not aligned with qPCR observations for donors H1 and C1 and the corresponding IMA rats. In contrast to qPCR, the members of Bifidobacteriaceae were at a lower relative abundance in H1 rats (48.0% ± 11.4%) than in donor H1 (70.5%) and C1 rats (32.7% ± 9.1%) compared to donor C1 rats (80.3%) (Figure 2).
Overall, dominant microbes and microbiota profiles of infant donors were transferred and established with similar composition in H1, H2, and C2 rats as shown by qPCR and sequencing data. Microbiota engraftment in C1 rats measured with qPCR was consistent with donor microbiota composition, but differences among C1 rats were observed with sequencing, with an overall increased abundance of Bacteroidaceae compared to donor C1 feces.
Different metabolic profiles were detected in cecal water from rats inoculated with different donors by HPLC-RI. Acetate (73.2% ± 2.4%) and propionate (15.8% ± 6.1%) were main metabolites identified in H1 rats, while acetate (67.3% ± 13.8%) and lactate (24.0% ± 14.4%) were the main metabolites detected in H2 rats. In colic rats, acetate (72.5% ± 4.2%) and propionate (21.2% ± 5.0%) were the main SCFAs identified in the cecal supernatant of C1 rats, while acetate (43.2% ± 5.4%), butyrate (33.7% ± 6.8%), and propionate (23.1% ± 5.7%) were the main SCFAs in C2 rats (Table 1).
After 2 weeks of inoculation, H2 accumulation was measured in respiratory chambers after housing single IMA rats for a 24-h period. H1 rats excreted higher H2 levels (105.2 ± 91.3 ppm) compared to H2 rats (28.6 ± 26.4 ppm; p 0.09) (Figure 3). However, significantly higher amounts of H2 were measured in colic than in healthy IMA rats (C1 rats control group: 200 ± 97.3 ppm and C2 rats control group: 3,248.0 ± 1,686.9 ppm; p 0.001) (Figure 3). Rats inoculated with feces from donor C2 excreted significantly higher levels of H2 than the C1 rats (p 0.002).
Figure 3. Hydrogen excretion by IMA rats. Hydrogen concentration (ppm) accumulated in the respiratory chambers after 24 h of housing single IMA rats. Measurements were done at the end of experimentation for rats inoculated with feces from healthy donors (H1 and H2) and at baseline (T0) and after intervention for rats inoculated with feces from colic donors (C1 and C2). Treatment groups (P279) were gavaged daily for 12–15 days with 109 CFU of Cutibacterium avidum P279 in 350 μL of sterile skim milk, and control groups received daily 350 μL of sterile skim milk. Values are mean (H1 n = 5; H2 n = 5; C1 T0 n = 9; C1 P279 n = 9; C1 control n = 8; C2 T0 n = 11; C2 P279 n = 4; and C2 control n = 5). Central horizontal solid lines show the median and dashed lines the mean, upper and lower box borders show the 90th and 10th centiles, respectively, and upper and lower whiskers show the 95th and 5th centiles, respectively.
The LUB community was enumerated in colic IMA rat feces at baseline using an MPN-based cultivation approach (Table 1). Non-SRB LUB colonized C1 (Log 10.1 ± 0.3 cells g feces–1) and C2 rats (Log 10.4 ± 0.3 cells g feces–1) at comparable and high levels. 16S rRNA gene amplicon sequencing of pellets from MPN cultures (in basal medium with L-lactate) detected mainly Veillonella (82.5 ± 5.6% rel. ab.) followed by Enterobacteriaceae (8.9 ± 3.9%) and Bacteroides (2.0 ± 1.8%). Acetate and propionate were the main products of lactate metabolism, which was in agreement with the bacterial populations recovered.
The SRB LUB (Log 4.4 cells g feces–1) cultured in Postgate E medium from feces of C2 donor were mainly identified as Desulfovibrio (80.1 ± 0.0% rel. ab.). SRB LUB were below detection limit in C2 rat feces at all time points and were not detected in feces from donor C1 and corresponding C1 rats.
The effect of daily supplementation of 109 CFU C. avidum P279 in sterile milk, a non-H2-producing LUB, after 12–15 days of intervention on gut microbiota composition and metabolism was tested in colic IMA rats and compared to control groups receiving only sterile milk. The colonization of C. avidum P279 in IMA rats from treatment groups was tested by qPCR and 16S rRNA gene amplicon sequencing. Donor-dependent effects were observed on microbiota composition and metabolism.
Cutibacterium avidum P279 colonized C1 rats with fecal abundance of Log 7.5 ± 0.2 cells g feces –1 (Table 2). LEfSe analysis based on 16S rRNA gene amplicon sequencing of the fecal microbiota of C1 rats also identified an increase in Propionibacteriaceae in the supplemented rats after the intervention period compared to baseline (Supplementary Figure 2D). When treated C1 rats were compared to the control group, LEfSe analysis identified increased Propionibacteriaceae in the supplemented rats, while Veillonellaceae abundance was higher in the control rats (Supplementary Figure 2B). In both groups, the Enterobacteriaceae population decreased after the intervention period, which corresponded with a significant reduction of Log 1.0 gene copies g feces–1 (Table 2 and Supplementary Figures 2C,D). Interestingly, formate and H2 excretion were significantly lower in the treatment group compared to control animals (0.4 ± 0.6 mM vs. 1.7 ± 1.2 mM for formate, respectively, p 0.02; 106.3 ± 53.8 ppm vs. 200.0 ± 97.3 ppm for H2 production, respectively, p 0.01) (Figure 3 and Table 2).
Table 2. Main bacterial communities in feces from colic IMA rats at baseline and after intervention.
Cutibacterium avidum P279 colonized C2 rats with fecal abundance of Log 5.4 ± 0.3 cells g feces–1, which was about log 2 lower than in C1 rats (Table 2). After treatment, the abundance of Firmicutes and Clostridium cluster I was significantly reduced by Log 0.5 ± 0.2 and Log 0.5 ± 0.2 gene copies g feces–1 (p < 0.01), respectively (Table 2). In agreement, LEfSe analysis identified a decrease in Clostridiaceae, and an increase of Propionibacteriaceae in treated animals. In C2 rats, Veillonellaceae increased after C. avidum P279 supplementation (Supplementary Figure 3D). The abundance of Propionibacteriaceae and Veillonellaceae was higher in the C2 treated than in the control rats, while the abundance of Bifidobacteriaceae and Enterobacteriaceae was lower (Supplementary Figure 3B).
Acetate and propionate levels, but not butyrate, were significantly higher in the C2 treatment group (11.9 ± 2.9 mM, 7.2 ± 3.3 mM, and 5.6 ± 2.2, respectively) compared to the control animals (acetate 6.4 ± 2.5 mM, p < 0.01; propionate 3.3 ± 0.8 mM, p < 0.05; and butyrate 5.1 ± 2.7 mM p > 0.05) after intervention. We measured a high although not significant reduction of the mean H2 production in the treated C2 rats with C. avidum P279 compared to the rats from the control group, which is likely due to the large spread observed in the control group (2,152.5 ± 228.96 ppm vs. 3,248.0 ± 1,686.86 ppm, respectively; p 0.2). Surprisingly, much lower variability in H2 excretion among the treated animals (1,815–2,325 ppm) was observed compared to the control rats (1,085–5,620 ppm) (Figure 3).
Human microbiota-associated animal models are valuable tools to study the effect of the resident gut microbiota on host pathobiology, and to perform invasive procedures that are not possible in humans due to ethical constraints. In this study, we focused on the establishment of a rat model for studying gut microbiota in infants younger than 3 months and tested the modulation potential of C. avidum P279 supplementation on H2 excretion in colic IMA rats. In general, microbiota diversity and taxonomic composition in IMA rats and donors were similar, while inter-individual differences in the microbiota composition could be reproduced.
We inoculated 3- to 4- week-old germ-free rats with fresh fecal microbiota collected from healthy and colic donors ensuring high viability at inoculation by storing fresh samples immediately after deposition under anaerobic conditions and at cooling temperatures. We chose to use feces from single donors and not pooled samples for inoculation, as there is a high inter-individual variability of the gut microbiota during the first year of life (8, 14, 28). The gut microbiota of the four donors participating in this study was representative of infant profiles dominated by Bifidobacteriaceae and Clostridiaceae as reported previously (14, 28, 42). The donors gut microbiota profiles were overall highly conserved in IMA rats. The fecal microbiota profiles of C1 rats identified by 16S rRNA gene amplicon sequencing were discordant with qPCR findings for Bifidobacterium and Bacteroides. The bias can be partly explained by the occurrence of species with different numbers of 16S rRNA gene copies within the targeted taxa, and differences in the efficiency of primers to amplify different members within a taxonomic group (43). The metabolic profiles identified in the ceca of rats corresponded with the metabolic capacity of the most abundant fecal colonizers identified. Preserving donor’s individuality in models used to investigate gut microbiota modulation strategies is of high relevance, as the effect of such interventions might be dependent on the individual microbiome characteristics as it has been previously shown in in vitro fermentation models (25). This is the first report of IMA rat model. The model will be helpful for studying the gut microbiome of young infants with a personalized approach.
The individual microbiota profiles of donors, H1, H2, and C2, were preserved in IMA rats, while C1 rats showed decreased Bifidobacteriaceae and enriched Bacteroides. Bifidobacteriaceae and Bacteroides are common infant colonizers that are able to degrade HMOs (10, 44–46). Providing IMA rats with infant formula that lacked HMOs and contained FOS and GOS could be a contributing factor to the quantitative differences observed in the establishment of these taxa in C1 recipient rats. Furthermore, important differences in the morphology and physiology of the rat gastrointestinal system compared to infants, as well as individual and variable digestive and environmental conditions in infants, can also explain differences between infant donors and IMA rat fecal microbiota. A lower relative abundance of Bifidobacterium and higher colonization of Bacteroides were recently reported for IMA mice and piglets inoculated with fecal samples from a 5-month-old infant (47). The differential establishment of these two core infant taxa might be also explained by differences in the host physiology that could determine bacterial colonization ability.
Working with rats instead of mice has a clear advantage in our goal to model microbiota in young animals (3–4 weeks old). The small size of mice at that same age would not have allowed to handle and gavage the animals and obtain reliable measurements of fecal samples and respiratory activities. Moreover, rats develop slower than mice, which was an advantage for our study which involved testing a 2-week supplementation in a model of infant colic. Rats reach puberty at day 50 after birth (48), while mice reach it at 28 days (49).
We initially fed IMA rats inoculated with the microbiota of healthy donors with only infant formula, but severe bloating was observed for some animals after approximately 2 weeks, possibly due to a surplus of rapidly metabolized di- and oligosaccharides (lactose, FOS, and GOS) and a lack of fibers in the infant formula. The appearance of bloating was coincidental with previous reports of decreased lactase expression and activity in rats from day 21 of life (50). Supplementation with a standard chow diet (two to three pellets per cage per day) from the second week of experimentation improved this condition, probably due to a bulking effect of the dietary fibers included in the pellets as reported before, although some animals still showed little cecal content and bloating at euthanasia (51).
The high availability of lactose, FOS, and GOS might have supported LPB metabolism resulting in high lactate concentrations, which would explain the higher abundance of Veillonella, an H2-producing LUB identified in H1, C1, and C2 rats compared to the donor levels. Strikingly, Veillonella remained low in rats inoculated with feces from H2 donor, suggesting limited enrichment when originally present at low abundance.
Acetate and propionate or lactate are the main fermentation metabolites identified in feces of infants younger than 6 months of age (28, 52, 53). In agreement, acetate was the main microbial metabolite identified in the ceca of all rats, while propionate was also produced in the ceca of H1 and C1 rats, which could be linked to a high abundance of propionate-producing Bacteroides and Veillonella (54). Lactate was the other metabolite identified with acetate in the ceca of H2 rats in alignment with the dominant colonizer LPB Bifidobacterium, while butyrate detection in C2 rats might be due to the high presence of butyrate producer C. neonatale (64% rel. ab.) belonging to Clostridium cluster I, as identified in C2 donors and rats by 16S rRNA gene amplicon sequencing.
The H2 production varied greatly according to the IMA rat group. H2 excretion of H2 rats was the lowest in agreement with the lowest abundance of lactate-utilizer H2-producer Veillonella. The extremely high H2 production in C2 rats might be due to C. neonatale which is able to ferment lactose to acetate, lactate, and butyrate while also producing H2. In vitro gas production by C. neonatale infant isolates during milk fermentation has been reported (55).
Surprisingly, compared to previous experiments in germ-free rats inoculated with fecal microbiota from healthy adults and irritable bowel syndrome patients (12.3 ± 7.3 ppm and 33.6 ± 3.6 ppm excreted H2, respectively) (2), H2 released by IMA rats was up to 100-fold higher. High breath H2 excretion values were reported for ca. 30% of non-colic and ca. 60% of colic infants due to physiologic lactose malabsorption followed by gut microbial metabolism (22–24, 56). The higher H2 excretion detected for colic IMA rats compared to healthy IMA rats could result from a functional dysbiosis in relation to high production and accumulation of H2, as has been proposed for colic infants previously (16, 22–24). More trials should be done with different donors, both healthy and colicky, to corroborate these findings.
Colic infants are diagnosed based on their crying time (more than 3 h per day, during at least 3 days in a week). Studies consider an intervention to be successful if crying time is reduced by at least 50% compared to the baseline values (20, 57, 58). The number of trials reporting the supplementation of probiotics in IC has steadily increased in recent years, but until now there is no clear evidence of beneficial effects of any strains/products on crying time reduction (20, 59, 60).
In this study, we evaluated infant gut colonizer C. avidum P279 as a candidate probiotic for inhibition of H2 production for IC treatment, based on its capability to metabolize lactose and lactate to propionate and acetate without producing H2, and on the hypothesis that imbalances in lactate and H2 metabolism may be the cause of bloating and associated pain in colic infants (16). We observed a reduction and lower variability in H2 excreted by colic IMA rats supplemented with C. avidum compared to control animals receiving the sterile milk carrier.
In an already propionigenic gut microbiota (C1), the SCFA profile in rat feces indicated a slightly lower formation of formate, but a significant reduction in excreted H2 and Veillonellaceae abundance in colic C1 rats compared to the control animals. We have recently reported that the addition of C. avidum P279 to an in vitro colonic fermentation also led to a lower abundance of Veillonella and formate production (17).
On the other hand, in C2 IMA rats, C. avidum P279 supplementation shifted the SCFA profile from a butyrogenic to higher propionate concentrations with an observed high decrease in H2 excretion, although not significant due to the high variability observed in the control rats. Interestingly, lower variability in H2 excretion was also observed in treated compared to non-treated C2 rats. Supplementation with C. avidum P279 in C2 IMA rats decreased colonization of dominant Clostridium in treated rats after the intervention compared to baseline values. These observations might have been consequence of a possible competition for lactose between C. avidum P279 and H2-producing Cl. neonatale (26, 55). Cl. neonatale has only recently been isolated and described as main colonizer in preterm infants (9, 55). C. avidum P279 also increased the abundance of taxa involved in lactate metabolism in C2 IMA rats, mainly LPB Lactobacillaceae and LUB Veillonellaceae. We previously reported the modulation in the lactate trophic chain by a C. avidum strain of infant origin, in an in vitro PolyFermS model mimicking infant proximal colon conditions and inoculated with fecal microbiota from a 2-month-old infant, with enhanced lactate consumption and increased LUB Eubacterium limosum (25).
Overall, our results indicate that C. avidum P279 supplementation can reduce H2 excretion and donor-dependently modulate the gut microbiota composition and metabolic activity in colic IMA rats, with no adverse effects observed on the health status of IMA rats.
We showed for the first time that fecal microbiota composition and functional profiles of single infants younger than 3 months old could be transferred upon transplantation to young germ-free rats fed infant formula and supplemented with a conventional chow diet. Our results indicate that this gnotobiotic rat model is suitable for investigating the role of gut microbiota in the nutrition and health of young infants. By transferring fecal microbiota of milk-fed infants to germ-free rats, we first report an increased level of H2 production by infant microbiota in vivo, compared to adult microbiota, and a very high H2 excretion in colic IMA rats. Furthermore, a pronounced reduction in H2 excretion was shown after supplementing C. avidum P279, lactose- and lactate-utilizing non-H2-producing strain, supporting the potential of the strain to reduce abdominal H2 production and IC-associated symptoms. Further experiments using this rat model with additional infant donors could help to identify the range of effects that the strain could have on different microbiota profiles. In order to confirm the potential efficacy of C. avidum P279 in colic alleviation, crying time reduction has to be evaluated in human clinical trials previous safety assessment of the strain.
The datasets presented in this study can be found in online repositories. The names of the repository/repositories and accession number(s) can be found in the article/Supplementary material.
The animal study was reviewed and approved by Local Institutional Animal Care and Use Committee.
VRM, CD, CC, AB-D, and CL designed the experiments. VRM and CD performed the gnotobiotic experiments and data analysis. VRM, CS, AB-D, and CL supported with result interpretation. VRM wrote the manuscript which was subsequently edited and revised by all authors. All authors contributed to the article and approved the submitted version.
This work was supported by own group resources (ETH-Zurich, University Children’s Hospital Zurich, and INRAE UMR 454 MEDIS Unit). No additional external funding was received for this study. Open access funding provided by ETH Zürich.
We would like to thank Vanessa Rueger and Rebekka Koller at the University Children’s Hospital, Zurich, Switzerland, for their valuable effort in volunteer recruitment and sampling. We thank Eve Delmas, Laureen Crouzet, and Christophe de Martin for skilled technical assistance in gnotobiotic animal experiments. We also would like to thank Alfonso Die and Markus Reichlin for their technical assistance in the HPLC analysis. We thank the Genetic Diversity Centre (GDC) at ETH Zurich for their support with 16S rRNA gene amplicon sequencing data generation.
The authors declare that the research was conducted in the absence of any commercial or financial relationships that could be construed as a potential conflict of interest.
All claims expressed in this article are solely those of the authors and do not necessarily represent those of their affiliated organizations, or those of the publisher, the editors and the reviewers. Any product that may be evaluated in this article, or claim that may be made by its manufacturer, is not guaranteed or endorsed by the publisher.
The Supplementary Material for this article can be found online at: https://www.frontiersin.org/articles/10.3389/fnut.2022.902159/full#supplementary-material
1. Schmidt TSB, Raes J, Bork P. The human gut microbiome: from association to modulation. Cell. (2018) 172:1198–215. doi: 10.1016/j.cell.2018.02.044
2. Crouzet L, Gaultier E, Del’Homme C, Cartier C, Delmas E, Dapoigny M, et al. The hypersensitivity to colonic distension of IBS patients can be transferred to rats through their fecal microbiota. Neurogastroenterol Motil. (2013) 25:e272–82. doi: 10.1111/nmo.12103
3. Ridaura VK, Faith JJ, Rey FE, Cheng J, Duncan AE, Kau AL, et al. Gut microbiota from twins discordant for obesity modulate metabolism in mice. Science. (2013) 6:1241214. doi: 10.1126/science.1241214
4. Britton GJ, Contijoch EJ, Mogno I, Vennaro OH, Sean R, Ng R, et al. Microbiotas from humans with inflammatory bowel disease alter the balance of gut Th17 and RORɤ+ regulatory T cells and exacerbate colitis in mice. Immunity. (2019) 50:212–24.e4. doi: 10.1016/j.immuni.2018.12.015
5. Asnicar F, Manara S, Zolfo M, Truong DT, Scholz M, Armanini F, et al. Studying vertical microbiome transmission from mothers to infants by strain-level metagenomic profiling. mSystems. (2017) 2:e00164–16. doi: 10.1128/mSystems.00164-16
6. Ferretti P, Pasolli E, Tett A, Asnicar F, Gorfer V, Fedi S, et al. Mother-to-infant microbial transmission from different body sites shapes the developing infant gut microbiome. Cell Host Microbe. (2018) 24:133–45.e5. doi: 10.1016/j.chom.2018.06.005
7. Jost T, Lacroix C, Braegger CP, Rochat F, Chassard C. Vertical mother-neonate transfer of maternal gut bacteria via breastfeeding. Environ Microbiol. (2014) 16:2891–904. doi: 10.1111/1462-2920.12238
8. Bäckhed F, Roswall J, Peng Y, Feng Q, Jia H, Kovatcheva-Datchary P, et al. Dynamics and stabilization of the human gut microbiome during the first year of life. Cell Host Microbe. (2015) 17:690–703. doi: 10.1016/j.chom.2015.04.004
9. Laursen MF, Sakanaka M, von Burg N, Andersen D, Mörbe U, Rivollier A, et al. Bifidobacterium species associated with breastfeeding produce aromatic lactic acids in the infant gut. Nat Microbiol. (2021) 6:1367–82. doi: 10.1038/s41564-021-00970-4
10. Stewart CJ, Ajami NJ, O’Brien JL, Hutchinson DS, Smith DP, Wong MC, et al. Temporal development of the gut microbiome in early childhood from the TEDDY study. Nature. (2018) 562:583–8. doi: 10.1038/s41586-018-0617-x
11. Ewaschuk JB, Naylor JM, Zello GA. D-Lactate in human and ruminant metabolism. J Nutr. (2005) 135:1619–25. doi: 10.1093/jn/135.7.1619
12. Wallis A, Ball M, McKechnie S, Butt H, Lewis DP, Bruck D. Examining clinical similarities between myalgic encephalomyelitis/chronic fatigue syndrome and d-lactic acidosis: a systematic review. J Transl Med. (2017) 15:129. doi: 10.1186/s12967-017-1229-1
13. Wang SP, Rubio LA, Duncan SH, Donachie GE, Holtrop G, Lo G, et al. Pivotal roles for pH, lactate, and lactate-utilizing bacteria in the stability of a human colonic microbial ecosystem. mSystems. (2020) 5:e00645–20. doi: 10.1128/msystems.00645-20
14. Casaburi G, Duar RM, Brown H, Mitchell RD, Kazi S, Chew S, et al. Metagenomic insights of the infant microbiome community structure and function across multiple sites in the United States. Sci Rep. (2021) 11:1472. doi: 10.1038/s41598-020-80583-9
15. Marquet P, Duncan SH, Chassard C, Bernalier-Donadille A, Flint HJ. Lactate has the potential to promote hydrogen sulphide formation in the human colon. FEMS Microbiol Lett. (2009) 299:128–34. doi: 10.1111/j.1574-6968.2009.01750.x
16. Pham VT, Lacroix C, Braegger CP, Chassard C. Lactate-utilizing community is associated with gut microbiota dysbiosis in colicky infants. Sci Rep. (2017) 7:11176. doi: 10.1038/s41598-017-11509-1
17. Rocha Martin VN, Schwab C, Krych L, Voney E, Geirnaert A, Braegger C, et al. Colonization of Cutibacterium avidum during infant gut microbiota establishment. FEMS Microbiol Ecol. (2019) 95:fiy215. doi: 10.1093/femsec/fiy215
18. Eutamène H, Garcia-Rodenas CL, Yvon S, d’Aldebert E, Foata F, Berger B, et al. Luminal contents from the gut of colicky infants induce visceral hypersensitivity in mice. Neurogastroenterol Motil. (2016) 29:e12994. doi: 10.1111/nmo.12994
19. Savino F, Cordisco L, Tarasco V, Calabrese R, Palumeri E, Matteuzzi D. Molecular identification of coliform bacteria from colicky breastfed infants. Acta Paediatr. (2009) 98:1582–8. doi: 10.1111/j.1651-2227.2009.01419.x
20. Benninga MA, Nurko S, Faure C, Hyman PE, St James Roberts I, Schechter NL. Childhood functional gastrointestinal disorders: neonate/toddler. Gastroenterology. (2016) 150:1443–55.e2. doi: 10.1053/j.gastro.2016.02.016
21. Parga JJ, Lewin S, Lewis J, Montoya-williams D, Alwan A, Shaul B, et al. Defining and distinguishing infant behavioral states using acoustic cry analysis: is colic painful? Pediatr Res. (2020) 87:576–80. doi: 10.1038/s41390-019-0592-4
22. Miller JJ, McVeagh P, Fleet GH, Petocz P, Brand JC. Breath hydrogen excretion in infants with colic. Arch Dis Child. (1989) 64:725–9. doi: 10.1136/adc.64.5.725
23. Moore DJ, Robb TA, Davidson GP. Breath hydrogen response to milk containing lactose in colicky and noncolicky infants. J Pediatr. (1988) 113:979–84. doi: 10.1016/s0022-3476(88)80567-5
24. Rhoads JM, Fatheree NY, Norori J, Liu Y, Lucke JF, Tyson JE, et al. Altered fecal microflora and increased fecal calprotectin in infants with colic. J Pediatr. (2009) 155:823–8.e1. doi: 10.1016/j.jpeds.2009.05.012
25. Pham VT, Chassard C, Rifa E, Braegger CP, Geirnaert A, Rocha Martin VN, et al. Lactate metabolism is strongly modulated by fecal inoculum, pH, and retention time in PolyFermS continuous colonic fermentation models mimicking young infant proximal colon. mSystems. (2019) 4:e00264–18. doi: 10.1128/mSystems.00264-18
26. Rocha Martin VN, Lacroix C, Killer J, Bunesova V, Voney E, Braegger C, et al. Cutibacterium avidum is phylogenetically diverse with a subpopulation being adapted to the infant gut. Syst Appl Microbiol. (2019) 4:506–16. doi: 10.1016/j.syapm.2019.05.001
27. Bryant MP, Burkey LA. Cultural methods and some characteristics of some of the more numerous groups of bacteria in the bovine rumen. J Dairy Sci. (1953) 36:205–17. doi: 10.3168/jds.s0022-0302(53)91482-9
28. Pham VT, Lacroix C, Braegger CP, Chassard C. Early colonization of functional groups of microbes in the infant gut. Environ Microbiol. (2016) 18:2246–58. doi: 10.1111/1462-2920.13316
29. Leedle JA, Hespell RB. Differential carbohydrate media and anaerobic replica plating techniques in delineating carbohydrate-utilizing subgroups in rumen bacterial populations. Appl Environ Microbiol. (1980) 39:709–19. doi: 10.1128/aem.39.4.709-719.1980
30. Gibson GR, Macfarlane GT. Chemostat enrichment of sulphate-reducing bacteria from the large gut. Lett Appl Microbiol. (1988) 7:127–33. doi: 10.1111/j.1472-765X.1988.tb01308.x
31. Robert C, Bernalier-Donadille A. The cellulolytic microflora of the human colon: evidence of microcrystalline cellulose-degrading bacteria in methane-excreting subjects. FEMS Microbiol Ecol. (2003) 46:81–9. doi: 10.1016/S0168-6496(03)00207-1
32. Chassard C, Dapoigny M, Scott KP, Crouzet L, Del’Homme C, Marquet P, et al. Functional dysbiosis within the gut microbiota of patients with constipated-irritable bowel syndrome. Aliment Pharmacol Ther. (2012) 35:828–38. doi: 10.1111/j.1365-2036.2012.05007.x
33. Stoddard SF, Smith BJ, Hein R, Roller BRK, Schmidt M. rrnDB: improved tools for interpreting rRNA gene abundance in bacteria and archaea and a new foundation for future development. Nucleic Acids Res. (2015) 43:D593–8. doi: 10.1093/nar/gku1201
34. Zachariassen LF, Krych L, Engkilde K, Nielsen DS, Kot W, Hansen CHF, et al. Sensitivity to oxazolone induced dermatitis is transferable with gut microbiota in mice. Sci Rep. (2017) 7:44385. doi: 10.1038/srep44385
35. Caporaso GJ, Bhute SS, Pande P, Shetty SA, Shelar R, Mane S, et al. QIIME allows analysis of high-throughput community sequencing data. Nat Methods. (2010) 7:335–6. doi: 10.1038/nmeth.f.303
36. Edgar RC. UPARSE: highly accurate OTU sequences from microbial amplicon reads. Nat Methods. (2013) 10:996–8. doi: 10.1038/nmeth.2604
37. Werner JJ, Koren O, Hugenholtz P, Desantis TZ, Walters WA, Caporaso JG, et al. Impact of training sets on classification of high-throughput bacterial 16s rRNA gene surveys. ISME J. (2012) 6:94–103. doi: 10.1038/ismej.2011.82
38. Scholz CFP, Kilian M. The natural history of cutaneous propionibacteria and reclassification of selected species within the genus Propionibacterium to the proposed novel genera Acidipropionibacterium gen. nov., Cutibacterium gen. nov. and Pseudopropionibacterium gen. nov. Int J Syst Evol Microbiol. (2016) 66:4422–32. doi: 10.1099/ijsem.0.001367
39. Krych L, Hansen CHF, Hansen AK, van den Berg FWJ, Nielsen DS. Quantitatively different, yet qualitatively alike: a meta-analysis of the mouse core gut microbiome with a view towards the human gut microbiome. PLoS One. (2013) 8:e62578. doi: 10.1371/journal.pone.0062578
40. Segata N, Izard J, Waldron L, Gevers D, Miropolsky L, Garrett WS, et al. Metagenomic biomarker discovery and explanation. Genome Biol. (2011) 12:R60. doi: 10.1186/gb-2011-12-6-r60
41. LeBlanc JG, Ledue-Clier F, Bensaada M, De Giori GS, Guerekobaya T, Sesma F, et al. Ability of Lactobacillus fermentum to overcome host α-galactosidase deficiency, as evidenced by reduction of hydrogen excretion in rats consuming soya α-galacto-oligosaccharides. BMC Microbiol. (2008) 8:22. doi: 10.1186/1471-2180-8-22
42. Yassour M, Vatanen T, Siljander H, Hämäläinen A, Härkönen T, Ryhänen SJ, et al. Natural history of the infant gut microbiome and impact of antibiotic treatment on bacterial strain diversity and stability. Sci Transl Med. (2016) 8:343ra81. doi: 10.1126/scitranslmed.aad0917
43. Dreier M, Meola M, Berthoud H, Shani N, Wechsler D, Junier P. High-throughput qPCR and 16S rRNA gene amplicon sequencing as complementary methods for the investigation of the cheese microbiota. BMC Microbiol. (2022) 22:48. doi: 10.1186/s12866-022-02451-y
44. Jost T, Lacroix C, Braegger CP, Chassard C. New insights in gut microbiota establishment in healthy breast fed neonates. PLoS One. (2012) 7:e44595. doi: 10.1371/journal.pone.0044595
45. Marcobal A, Sonnenburg JL. Human milk oligosaccharide consumption by intestinal microbiota. Clin Microbiol Infect. (2012) 18:12–5. doi: 10.1111/j.1469-0691.2012.03863.x
46. Marcobal A, Barboza M, Sonnenburg ED, Pudlo N, Martens EC, Desai P, et al. Bacteroides in the infant gut consume milk oligosaccharides via mucus-utilization pathways. Cell Host Microbe. (2011) 10:507–14. doi: 10.1016/j.chom.2011.10.007
47. Aluthge ND, Tom WA, Bartenslager AC, Burkey TE, Miller PS, Heath KD, et al. Differential longitudinal establishment of human fecal bacterial communities in germ-free porcine and murine models. Commun Biol. (2020) 3:760. doi: 10.1038/s42003-020-01477-0
48. Agoston DV. How to translate time? The temporal aspect of human and rodent biology. Front Neurol. (2017) 8:92. doi: 10.3389/fneur.2017.00092
49. Wang S, Lai X, Deng Y, Song Y. Correlation between mouse age and human age in anti-tumor research: significance and method establishment. Life Sci. (2020) 242:117242. doi: 10.1016/j.lfs.2019.117242
50. Motohashi Y, Fukushima A, Kondo T, Sakuma K. Lactase decline in weaning rats is regulated at the transcriptional level and not caused by termination of milk ingestion. J Nutr. (1997) 127:1737–43. doi: 10.1093/jn/127.9.1737
51. Riva A, Kuzyk O, Pfann C, Herbold C, Warth B, Siuzdak G, et al. A fiber-deprived diet disturbs the fine-scale spatial and metabolic architecture of the murine colon microbiome and the mucus barrier. Nat Commun. (2019) 10:4366. doi: 10.1038/s41467-019-12413-0
52. Midtvedt AC, Midtvedt T. Production of short chain fatty acids by the intestinal microflora during the first 2 years of human life. J Pediatr Gastroenterol Nut. (1992) 15:395–403. doi: 10.1097/00005176-199211000-00005
53. Bridgman SL, Azad MB, Field CJ, Haqq AM, Becker AB, Mandhane PJ, et al. Fecal Short-Chain Fatty Acid variations by breastfeeding status in infants at 4 months: differences in relative versus absolute concentrations. Front Nutr. (2017) 4:11. doi: 10.3389/fnut.2017.00011
54. Flint HJ, Duncan SH, Scott KP, Louis P. Links between diet, gut microbiota composition and gut metabolism. Proc Nutr Soc. (2015) 74:13–22. doi: 10.1017/S0029665114001463
55. Bernard K, Burdz T, Wiebe D, Alfa M, Bernier AM. Clostridium neonatale sp. nov. linked to necrotizing enterocolitis in neonates and a clarification of species assignable to the genus Clostridium (Prazmowski 1880) emend. Lawson and Rainey 2016. Int J Syst Evoly Microbiol. (2018) 68:2416–23. doi: 10.1099/ijsem.0.002827
56. Douwes AC, Oosterkamp RF, Fernandes J, Los T, Jongbloed AA. Sugar malabsorption in healthy neonates estimated by breath hydrogen. Arch Dis Child. (1980) 55:512–5. doi: 10.1136/adc.55.7.512
57. Sung V, Cabana MD, D’Amico F, Deshpande G, Dupont C, Indrio F, et al. Lactobacillus reuteri DSM 17938 for managing infant colic: protocol for an individual participant data meta-analysis. BMJ Open. (2014) 4:e006475. doi: 10.1136/bmjopen-2014-006475
58. Wolke D, Bilgin A, Samara M. Systematic review and meta-analysis: fussing and crying durations and prevalence of colic in infants. J Pediatr. (2017) 185:55–61.e4. doi: 10.1016/j.jpeds.2017.02.020
59. Ong TG, Gordon M, Banks S, Thomas MR, Akobeng AK. Probiotics to prevent infantile colic. Cochrane Database Syst Rev. (2019) 3:CD012473. doi: 10.1002/14651858.CD012473.pub2
60. Turco R, Russo M, Bruzzese D, Staiano A. Efficacy of a partially hydrolysed formula, with reduced lactose content and with Lactobacillus reuteri DSM 17938 in infant colic: a double blind, randomised clinical trial. Clin Nutr. (2021) 40:412–9. doi: 10.1016/j.clnu.2020.05.048
61. Bartosch S, Fite A, Macfarlane GT, McMurdo ME. Characterization of bacterial communities in feces from healthy elderly volunteers and hospitalized elderly patients by using real-time PCR and effects of antibiotic treatment on the fecal microbiota. Appl Environ Microbiol. (2004) 70:3575–81. doi: 10.1128/AEM.70.6.3575-3581.2004
62. Fierer N, Jackson J. Assessment of soil microbial community structure by use of taxon-specific quantitative PCR assays. Appl Environ Microbiol. (2005) 71:4117–20. doi: 10.1128/AEM.71.7.4117-4120.2005
63. Guo X, Xia X, Tang R, Zhou J, Zhao H, Wang K. Development of a real-time PCR method for Firmicutes and Bacteroidetes in faeces and its application to quantify intestinal population of obese and lean pigs. Lett Appl Microbiol. (2008) 47:367–73. doi: 10.1111/j.1472-765X.2008.02408.x
64. Price RR, Viscount HB, Stanley MC, Leung KP. Targeted profiling of oral bacteria in human saliva and in vitro biofilms with quantitative real-time PCR. Biofouling. (2007) 23:203–13. doi: 10.1080/08927010701251169
65. Ramirez-Farias C, Slezak K, Füller Z, Duncan A, Holtrop G, Louis P. Effect of inulin on the human gut microbiota: stimulation of Bifidobacterium adolescentis and Faecalibacterium prausnitzii. Br J Nutr. (2009) 101:541–50. doi: 10.1017/S0007114508019880
Keywords: infant gut microbiota, infant colic, human microbiota-associated rats, gnotobiotic, Cutibacterium (Propionibacterium) avidum, hydrogen
Citation: Rocha Martin VN, Del’Homme C, Chassard C, Schwab C, Braegger C, Bernalier-Donadille A and Lacroix C (2022) A proof of concept infant-microbiota associated rat model for studying the role of gut microbiota and alleviation potential of Cutibacterium avidum in infant colic. Front. Nutr. 9:902159. doi: 10.3389/fnut.2022.902159
Received: 22 March 2022; Accepted: 28 July 2022;
Published: 22 August 2022.
Edited by:
Carlos Gómez-Gallego, University of Eastern Finland, FinlandReviewed by:
Franck Carbonero, Washington State University Health Sciences Spokane, United StatesCopyright © 2022 Rocha Martin, Del’Homme, Chassard, Schwab, Braegger, Bernalier-Donadille and Lacroix. This is an open-access article distributed under the terms of the Creative Commons Attribution License (CC BY). The use, distribution or reproduction in other forums is permitted, provided the original author(s) and the copyright owner(s) are credited and that the original publication in this journal is cited, in accordance with accepted academic practice. No use, distribution or reproduction is permitted which does not comply with these terms.
*Correspondence: Christophe Lacroix, Y2hyaXN0b3BoZS5sYWNyb2l4QGhlc3QuZXRoei5jaA==
Disclaimer: All claims expressed in this article are solely those of the authors and do not necessarily represent those of their affiliated organizations, or those of the publisher, the editors and the reviewers. Any product that may be evaluated in this article or claim that may be made by its manufacturer is not guaranteed or endorsed by the publisher.
Research integrity at Frontiers
Learn more about the work of our research integrity team to safeguard the quality of each article we publish.