- 1Neurofarba—Pharmacology and Toxicology Section, Department of Neuroscience, Psychology, Drug Research and Child Health, University of Florence, Florence, Italy
- 2Department of Pharmacy, School of Medical and Pharmaceutical Sciences, University of Genoa, Genoa, Italy
- 3Centre of Excellence for Biomedical Research, University of Genoa, Genoa, Italy
- 4Innovation and Medical Science Division, Aboca SpA Società Agricola, Sansepolcro, Italy
- 5Anatomy and Histology Section, Department of Experimental and Clinical Medicine, University of Florence, Florence, Italy
- 6Psychiatry Section, Department of Neurofarba, University of Florence, Florence, Italy
- 7Department of Psychiatry and Behavioral Sciences, Albert Einstein College of Medicine, New York, NY, United States
- 8Institute of Neuroscience, Florence, Italy
Experimental evidence suggests that neuroinflammation is a key pathological event of many diseases affecting the nervous system. It has been well recognized that these devastating illnesses (e.g., Alzheimer’s, Parkinson’s, depression, and chronic pain) are multifactorial, involving many pathogenic mechanisms, reason why pharmacological treatments are unsatisfactory. The purpose of this study was to evaluate the efficacy of a vegetal mixture capable of offering a multiple approach required to manage the multifactoriality of neuroinflammation. A mixture composed of Zingiber officinale (150 mg kg−1), Echinacea purpurea (20 mg kg−1), and Centella asiatica (200 mg kg−1) was tested in a mouse model of systemic neuroinflammation induced by lipopolysaccharide (LPS, 1 mg kg−1). Repeated treatment with the vegetal mixture was able to completely counteract thermal and mechanical allodynia as reported by the Cold plate and von Frey tests, respectively, and to reduce the motor impairments as demonstrated by the Rota rod test. Moreover, the mixture was capable of neutralizing the memory loss in the Passive avoidance test and reducing depressive-like behavior in the Porsolt test, while no efficacy was shown in decreasing anhedonia as demonstrated by the Sucrose preference test. Finally, LPS stimulation caused a significant increase in the activation of glial cells, of the central complement proteins and of inflammatory cytokines in selected regions of the central nervous system (CNS), which were rebalanced in animals treated with the vegetal mixture. In conclusion, the vegetal mixture tested thwarted the plethora of symptoms evoked by LPS, thus being a potential candidate for future investigations in the context of neuroinflammation.
Introduction
Neuroinflammation is a pivotal factor in the pathogenesis of many neurodegenerative diseases (1) and psychiatric illnesses like depression (2), Parkinson’s disease (PD) (3), Alzheimer’s disease (AD), (4), Huntington’s disease (5), and multiple sclerosis (6). Moreover, it has been implicated in sickness behavior (7), reduced cognition (8), and memory (9), as well as in age-related increased sensitization of the immune system to intrinsic and extrinsic stimuli (10, 11). Lastly, it has been demonstrated that the coronavirus 2019 (COVID-19) infection has triggered and reinforced obsessive and compulsive behavior leading to an aggravation of anxiety and depressive symptoms in some patients (12, 13). This could be partially explained due to an increase in the levels of proinflammatory chemokines and cytokines that are responsible for the pathophysiology of comorbid neuropsychiatric manifestations (14, 15). Also, neuropathic pain, caused by different etiology, is characterized by excessive inflammation in both the peripheral and central nervous systems (CNS), which may contribute to the initiation and maintenance of persistent pain (16). Overall, neuroinflammation can be considered as a normal response that origins in order to maintain homeostasis in the nervous system. This response involves the synthesis and release of molecules endowed with inflammatory properties; however, a neuroinflammatory process that is too strong or protracted becomes maladaptive, leading to cellular dysfunction which is seen during stress, chronic pain, and chronic neurodegeneration (17).
In this scenario, effective therapies are lacking, and a relatively dry CNS drug pipeline poses a formidable challenge to pharmacologists who have to develop drugs that can counteract the multitude of brain diseases and symptoms, such as cognitive impairment (from mild to more severe), motor impairment, memory loss, mood alterations, and pain. Indeed, the use of anti-inflammatory drugs like non-steroidal anti-inflammatory drugs (NSAIDs) can ameliorate some symptoms and reduce the extent of neurodegeneration (18); however, the primary mechanism of neuroinflammation is not well-understood and new therapeutic approaches are mandatory to provide significant relief for these patients.
Traditional medicine has been based on the use of medicinal plants for millennia, and mankind has been educated from experience and observation to use plants correctly, strengthening an extensive culture of their use. Indeed, phytotherapeutic preparations can offer a multiple approach to the multifactorial nature of neuroinflammation relying on the complex mixture of one or more plants (19).
Zingiber officinale, Centella asiatica, and Echinacea purpurea are plants rich in bioactive compounds with several pharmacological effects. Zingiber officinale Roscoe (ginger), belonging to the Zingiberaceae family, has been commonly consumed as a spice and used as herbal medicine for a long time (20). From a pharmacological point of view, ginger demonstrated to share with NSAIDs the properties of inhibiting prostaglandin synthesis and to be dual inhibitors of cyclooxygenase-2 (COX-2) and lipoxygenase (LOX) enzymes; therefore, it can effectively reduce the synthesis of both prostaglandins (PGs) and leukotrienes (LTs) (21). Additionally, it can also reduce the synthesis of proinflammatory cytokines, such as TNF-α, IL-1, and IL-8 (22, 23). These preclinical findings were supported by a study in which ginger reduced TNF-α and hs-C-reactive protein (CRP) levels in patients with diabetes (24). Moreover, ginger showed protective properties against rheumatic diseases, via rebalancing musculoskeletal disorders and joint inflammation-inducing T-helper-2 and anti-inflammatory cytokine production (e.g., IL-4 and IL-10) (25); decreasing IL-2, IL-6, and IL-1β cytokine levels; and inhibiting the release of substance P as pain and inflammation mediator (26). The beneficial effects of ginger have been mainly attributed to the presence of gingerols and shogaols (phenolic compounds) (27).
Echinacea purpurea (L.) Moench is an endemic plant in the US Great Plains and in the Canadian prairies of North America. Even though Echinacea originates in North America, the plant’s beneficial effects were discovered by Europeans who used it for its immunomodulatory properties. The bioactive constituents are mainly phenols, which are reported to exert neuroprotective effects by reducing apoptotic markers (28), polar caffeic acid derivatives (cicoric acid and echinacoside), and lipophilic alkamides (29, 30). Alkamides, in early investigations, have been demonstrated to evoke stimulatory effects on phagocytosis, and these constituents have been found in relevant concentrations in the human blood after the oral intake of Echinacea extract. Alkamides share similarities with anandamide, the endogenous ligand of cannabinoid (CB) receptors, and consequently bind to CB receptors, which are supposed to be the theoretical mechanism of action of Echinacea alkamides as immunomodulatory agents (31). In addition, Centella asiatica was successfully used for a long time in traditional Chinese medicine as a nerve tonic (32). The bioactive micronutrients are mainly asiatic acid, asiaticoside, madecassoside, and madecassic acid and are reported to be effective in retarding brain aging and assisting in the renewal of the neural tissue; therefore, it is effective in improving memory, increasing attention, and concentration (33). Centella asiatica has also shown an anti-hyperalgesic and protective profile in a rat model of osteoarthritis when the extract of this plant was intra-articularly injected (34). Despite the beneficial effects of these plants, as previously reported, no preclinical data are available about the use of a mixture of these three plants against the plethora of neuroinflammation symptoms.
The aim of this study was to highlight the efficacy of a repeated treatment with a vegetal mixture composed of Zingiber officinale, Echinacea purpurea, and Centella asiatica in a mouse model of neuroinflammation obtained by the sub-chronic injection of LPS. In particular, we focused our attention on a plethora of neuroinflammatory symptoms, such as memory impairment, depression, anhedonia, pain, and motor alterations. Moreover, ex vivo analyses were performed to determine the effect of the treatment on astrocytosis and microgliosis in the spinal cord and prefrontal cortex (PFC) and on the selected proteins of the complement system in the PFC.
Materials and methods
Animals
Eight- to ten-week-old male CD-1 mice (Envigo, Varese, Italy) weighing approximately 20–25 g were used for all the experiments described in the present study. Animals were housed in CeSAL (Centro Stabulazione Animali da Laboratorio, University of Florence) and used at least 1 week after their arrival. Eight mice were housed per cage (size 26 × 41 cm) kept at 23 ± 1°C with a 12-h light/dark cycle, the light at 7 a.m., and were fed a standard laboratory diet and tap water ad libitum.
All animal manipulations were carried out according to the Directive 2010/63/EU of the European Parliament and the European Union Council (22 September 2010) on the protection of animals used for scientific purposes. The ethical policy of the University of Florence complies with the Guide for the Care and Use of Laboratory Animals of the US National Institutes of Health (NIH Publication No. 85–23, revised 1996; University of Florence assurance number: A5278-01). Formal approval to conduct the experiments described was obtained from the Animal Subjects Review Board of the University of Florence. Experiments involving animals have been conducted according to the ARRIVE guidelines (35). All efforts were made to minimize animal suffering and reduce the number of animals used.
Lipopolysaccharide-induced neuroinflammation
Lipopolysaccharide (LPS, 1 mg kg–1; Sigma-Aldrich, St. Louis, MO, United States) was dissolved in sterile saline solution and intraperitoneally injected on four alternate days (days 1, 3, 5, and 8). Behavioral measurements were started from day 9. Control animals were treated with a vehicle.
Plant materials
Vegetal extracts were supplied by ABOCA (Sansepolcro, Italy). Zingiber officinale Roscoe Rhizoma was subjected to extraction by using 30% ethanol (ethanol: water, 30:70 v/v) for 8 h at 50°C and filtered to remove solid exhausted material. The resulting clarified extract was concentrated by ethanol evaporation under vacuum, until reaching the concentration factor of 10:1 (v:v, initial alcoholic extract volume compared to the volume after the evaporation step), and then freeze-dried for 72 h. The resulting extract was stored until use at 4°C, away from light and humidity. The flowering tops of Echinacea Purpurea were subjected to extraction by using 45% ethanol (ethanol: water, 45:55 v/v) for 8 h at 50°C and filtered to remove solid exhausted material. The resulting clarified extract was concentrated by ethanol evaporation under vacuum, until reaching the concentration factor of 8:1 (v:v, initial volume of alcoholic extract compared to the volume after evaporation step), and then freeze-dried for 72 h. The resulting extract was stored until use at 20°C, away from light and humidity. Centella asiatica extract was prepared following the protocol described by Micheli et al. (34). The characterization of all extracts was also performed by ABOCA. Tables 1, 2 present the characterization of Echinacea and Zingiber, respectively. Centella asiatica was characterized and described previously (34). Zingiber characterization was performed according to the methods reported in USP-NF.
Echinacea purpurea was characterized by means of a reverse-phase HPLC-UV method, which involved the sample extraction with 37% ethanol: water: hydrochloric acid (44.5:54.5:1.0, v/v and) in an ultrasonic bath at 35°C. The corresponding solution was filtered and analyzed by means of an HPLC-DAD at 330 nm. The mobile phase was ultrapure water acidified with 0.2% phosphoric acid (solvent A) and acetonitrile (solvent). The program involved a linear gradient elution with solvent A from 90% to 80% and solvent B from 10% to 20% for 40 min. At 40.5 min solvent A was 10% and solvent B from 90% and remained in these conditions for 5 min. Caftaric acid, chlorogenic acid, echinacoside, and chicoric acid were eluted at 9.6, 10.7, 22.4, and 39.5 min, respectively. Quantitative data were obtained using the external regression curve method.
Treatment
The plant extracts of Zingiber officinale (150 mg kg–1), Echinacea purpurea (20 mg kg–1), and Centella asiatica (200 mg kg–1) were suspended in 1% carboxymethylcellulose sodium salt (CMC; Sigma-Aldrich, St. Louis, MO, United States) and orally administered daily starting from day 1 till the end of the experiment (day 12). Control animals received an equal volume of vehicle. Behavioral measurements were conducted from days 9 to 12 when animals were sacrificed for the ex vivo analyses.
von Frey test
The animals were placed in 20 × 20 cm Plexiglas boxes equipped with a metallic mesh floor, 20 cm above the bench. A habituation of 15 min was allowed before the test. An electronic von Frey hair unit (Ugo Basile, Varese, Italy) was used, and the withdrawal threshold was evaluated by applying force ranging from 0 to 5 g with a 0.2 g accuracy. Punctuate stimulus was applied to the mid-plantar area of each anterior paw from below the meshy floor through a plastic tip, and the withdrawal threshold was automatically displayed on the screen.
The paw sensitivity threshold was defined as the minimum pressure required to elicit a robust and immediate withdrawal reflex of the paw. Voluntary movements associated with locomotion were not taken as a withdrawal response. Stimuli were applied on each anterior paw with an interval of 5 s. The measurement was repeated five times, and the final value was obtained by averaging the five measures (36, 37).
Cold plate test
Thermal allodynia was assessed using the Cold plate test. With minimal animal–handler interaction, mice were taken from home cages and placed onto the surface of the cold plate (Ugo Basile, Varese, Italy) maintained at a constant temperature of 4°C ± 1°C. Ambulation was restricted by a cylindrical Plexiglas chamber (diameter, 10 cm; height, 15 cm) with an open top. A timer controlled by a foot peddle was used to monitor timing response latency from the moment the mouse was placed onto the cold plate. Pain-related behavior (licking of the hind paw) was observed, and the time (seconds) of the first sign was recorded. The cutoff time of the latency of paw lifting or licking was set at 30 s (38, 39).
Passive avoidance test
We followed the protocol of the step-through method described previously (40). The apparatus consisted of a two-compartment acrylic box with a lighted compartment connected to a darkened one by a guillotine door. As soon as the mouse entered the dark compartment, it received a punishing electrical shock (0.5 mA, 1 s). The latency times for entering the dark compartment were measured during the training test and after 24 h of the retention test. The data recorded during the retention session are reported in the “Results” section. The maximum entry latency allowed in the training and retention sessions was 60 and 180 s, respectively.
Porsolt test
The forced swimming test used was similar to the method described previously (41). Briefly, mice were placed individually in glass cylinders (height: 25 cm, diameter: 10 cm) containing 12 cm of water maintained at 22–23°C and left there for 6 min. A mouse was considered to be immobile when it floated in the water, in an upright position, and made only small movements to keep its head above water. The duration of mobility was recorded during the last 4 min of the 6-min test. An increase in the duration of immobility is indicative of a depressant-like effect (42).
Sucrose preference test
Sucrose preference was examined as a test to assess anhedonia. Mice were placed in cages equipped with two bottles: a bottle containing 2% sucrose solution and a bottle containing water for 24 h after the beginning of the experiment. The preference index was calculated according to the following formula: preference index = volume of consumed sucrose solution/(volume of consumed sucrose solution + volume of consumed water).
Rota rod test
The apparatus consisted of a base platform and a rotating rod with a diameter of 3 cm and a non-slippery surface. The rod was placed at a height of 15 cm from the base. The rod, 30 cm in length, was divided into five equal sections by six disks. Thus, up to five mice were tested simultaneously on the apparatus, with the rotating speed of the rod at 16 revolutions per minute. The integrity of motor coordination was assessed on the basis of the number of falls from the rod in 10 min (43).
Tissue collection
At the end of the behavioral experiments, animals were killed by decapitation. The lumbar spinal cord was collected, and one part was fixed by immersion in 4% neutral buffered formalin and one part was frozen using liquid nitrogen. The prefrontal cortex (PFC) was also collected and frozen using liquid nitrogen.
Enzyme-linked immunosorbent assay
The prefrontal cortex and spinal cord samples were homogenized (100 mg of tissue/mL of cold PBS). The samples were centrifuged at 12,000 × g for 15 min at 4°C. The supernatant was collected for protein quantification using a bicinchoninic acid (BCA) protein assay reagent kit (Merck, Milan, Italy). The levels of IL-1β (Thermo Fisher Scientific, Milan, Italy) and TNF-α (Thermo Fisher Scientific, Milan, Italy) in the PFC and spinal cord were measured using enzyme-linked immunosorbent assay (ELISA) kits according to the manufacturer’s instructions. The content of cytokines is expressed as pg of cytokines/mg of protein.
Western blot analysis of prefrontal cortex lysates
Mouse PFC samples were homogenized in modified RIPA buffer (10 mM Tris, pH 7.4, 150 mM NaCl, 1 mM EDTA, 0.1% SDS, 1% Triton X-100, 1 mM sodium orthovanadate, and protease inhibitors), sonicated, and centrifuged at 20,000 × g for 10 min at 4°C. The supernatant was kept for the immunoblot analysis and quantified for protein content with a BCA assay. Samples were boiled for 5 min at 95°C, separated by SDS-10% PAGE (30 μg/lane), and then blotted onto the PVDF membrane. Membranes were blocked for 1 h at room temperature with Tris-buffered saline Tween (t-TBS: 20 mM Tris, pH 7.4, 150 mM NaCl, and 0.05% Tween 20) containing 5% (w/v) non-fat dried milk, and then probed with the following primary antibodies overnight at 4°C: rabbit anti-CD11b (1:1,000, ab200999, Abcam, Cambridge, United Kingdom), mouse anti-GFAP (1:10,000, G3893, Sigma, St. Louis, MO, United States), mouse anti-C1q (1:25, Abcam, ab71940), rabbit anti-C3 (1:1,000, Abcam, ab200999), and mouse anti-β-actin (1:5,000, Sigma). After extensive washes in t-TBS, membranes were incubated for 1 h at room temperature with the appropriate horseradish peroxidase-linked secondary antibodies (1:20,000, A9044 and A9169, Sigma). Immunoblots were visualized with an enhanced chemiluminescence (ECL) Western blotting detection system. Images were acquired using the Alliance LD6 images capture system (Uvitec, Cambridge, United Kingdom) and analyzed using UVI-1D software (Uvitec, Cambridge, United Kingdom).
Immunohistochemistry of the spinal cord
On day 12, after behavioral measurements, mice were sacrificed, and the lumbar spinal cord segments were removed, post-fixed in 4% paraformaldehyde, and then cryoprotected in 30% sucrose solution at 4°C. Slide-mounted cryostat sections (5 μm) were processed for indirect immunofluorescence histochemistry.
Formalin-fixed cryostat sections (5 μm) were incubated for 30 min in a ready-to-go blocking solution (Bio-Optica, Milan, Italy) at room temperature to block unspecific binding. The primary antibodies, incubated overnight at 4°C, were directed against Iba1 (rabbit, 1:200; Wako Chemicals, Richmond, United States) for microglial staining or against the glial fibrillary acidic protein (GFAP; rabbit, 1:200; Dako, United States) for astrocyte staining. After rinsing in PBST, sections were incubated in donkey anti-rabbit IgG secondary antibody labeled with Alexa Fluor 488 (1:500, Invitrogen, Milan, Italy) at room temperature for 1 h. Nuclei were stained with 4’,6-diamidin-2-fenilindolo (DAPI, 1:2,000; Invitrogen, Milan, Italy).
Negative control sections (no exposure to the primary antisera) were processed concurrently with the other sections for all the immunohistochemical studies. We obtained a single optical density value for the dorsal horns by averaging the two sides in each mouse, and these values were compared to the homologous average values from the vehicle-treated animals.
Images were acquired by using a motorized Leica DM6000B microscope equipped with a DFC350FX camera (Leica, Mannheim, Germany). Microglia and astrocyte morphology was assessed by inspecting at least three fields (×40, 0.75NA objective) in the dorsal horn.
Quantitative analysis of GFAP and Iba1-positive cells was performed by viewing at least three independent fields through a ×40 0.5NA objective. Immunofluorescent staining was measured as mean fluorescence intensity using ImageJ software (ImageJ, National Institute of Health, United States)1 by an automatic thresholding algorithm. Furthermore, GFAP or Iba1-positive cells were quantified by thresholding automatic count. The results, given as mean fluorescent intensity (arbitrary units) by the thresholded fluorescent signal, revealed a common trend between GFAP/Iba1 expression and astrocyte/microglial cell number (data not shown). Five spinal cord sections were analyzed for each animal.
Statistical analysis
Researchers not informed about the specific treatment of each animal group carried out the tests. Results were expressed as mean ± SEM, and the analysis of variance was performed by ANOVA test and then by applying post hoc Bonferroni’s multiple comparison test. The p-values less than 0.05 were considered significant. Data were analyzed using the “Origin 9.1” software.
Results
Neuroinflammation was established by the sub-chronic injection of 1 mg kg–1 LPS (for a total of four administrations performed on days 1, 3, 5, and 8). The vegetal mixture was composed of dry extracts obtained from Zingiber officinale (150 mg kg–1), Echinacea purpurea (20 mg kg–1), and Centella asiatica (200 mg kg–1) and was daily per os administered from day 1 till the end of the behavioral experiments. From day 9 onward, several symptoms of neuroinflammation were analyzed. As reported in Figure 1, LPS induced a significant decrease in the animal’s pain threshold measured by thermal and mechanical non-noxious stimuli (Cold plate and von Frey tests, respectively). Indeed, on day 9, the licking latency significantly decreased from a value of about 17 s in the control group to about 9 s in the animals treated with LPS when the mice were exposed to a cold surface at 4°C (Cold plate test; Figure 1A). The daily administration of the vegetal mixture was able to counteract the thermal allodynia caused by LPS. One point to be noted is that the results were obtained 24 h after the last treatment with the mixture and were not evoked by the daily acute administration. Similarly, the paw stimulation with a non-noxious mechanical stimulus allowed us to observe a decrease in the withdrawal response in LPS-treated mice that was recovered in the group treated with LPS + vegetal mixture (Figure 1B). The LPS treatment also induced motor incoordination which was evaluated by the Rota rod test. The results showed a massive increase in the number of falls from the rod during 10 min in comparison to the vehicle + vehicle-treated animals. The combination of Zingiber officinale, Echinacea purpurea, and Centella asiatica improved the mouse performance determining a significant reduction in the number of falls, which was evident by the scores similar to those measured in the control animals (Figure 2). With regard to the impairments affecting memory and mood, we analyzed the depression-like behavior by the Porsolt test, anhedonia by the sucrose preference test, and memory by the passive avoidance test. In the Porsolt test, repeated administration of LPS caused a reduction in the mobility time after the mice were placed in a cylinder filled with water from where they could not escape. The treatment with the vegetal mixture significantly increased this parameter, reaching a value of about 150 s in comparison to 90 s observed in the LPS-treated animals (Figure 3). Moreover, the extracts were shown to reduce the amnesic effects of LPS in the passive avoidance test (Figure 4). The repeated administration of the vegetal mixture significantly antagonized the cognitive impairments induced by the lipopolysaccharide. In the retention test, the time spent in the light box was 73 ± 11 s for vehicle + vehicle group, 31 ± 4 s for LPS + vehicle group, and 103 ± 6 s for LPS + vegetal mixture-treated animals (Figure 4). Anhedonia, the incapacity to experience pleasure, is a hallmark symptom of several nervous system diseases. Anhedonia was induced by LPS injections and was evaluated as a significant reduction in sucrose intake in comparison to control animals; however, the treatment with the vegetal mixture was unable to restore this parameter (Figure 5). At the end of the behavioral measurements, the dorsal horn of the lumbar spinal cord was analyzed. Four LPS injections induced a significant increase in the number of GFAP and Iba1-positive cells (Figures 6, 7, respectively). Also, the fluorescence intensity of astrocytes and microglia was upregulated by LPS in comparison to the control group. The vegetal mixture prevented the activation of glial cells, as shown in Figures 6, 7. LPS treatment also caused a significant increase in the density of CD11b, a specific marker of microglia and macrophages, and GFAP in the PFC of the inflamed animals, which suggests that microgliosis and astrocytosis also occur in this region (Figure 8). The vegetal mixture recovered the CD11b signal to the level detected in the vehicle-treated animals (Figures 8 A,B), but did not affect the GFAP density, which was unmodified with respect to LPS-treated mice (Figures 8C,D). In recent years, the involvement of the complement system in the progression of neurodegenerative and neuroinflammatory disorders emerged in the interest of researchers. Because of the huge increase in the CD11b, which selectively binds the C3 component of the complement system, we determined whether LPS could also impact the expression of the C1q and the C3 complement proteins, i.e., those complement components that are mainly involved in the classic and the alternative pathways of the activation of the immune complex complement cascade and that are associated to the mechanisms of synaptic pruning and remodeling of central synapses (44, 45). Both C1q and C3 proteins were over-expressed in the PFC of the LPS-treated mice. The vegetal mixture significantly reduced the C3 overproduction, leaving unaltered the density of the C1q protein (Figure 9). Finally, the mixture was also able to significantly reduce the overproduction of TNF-α and IL-1β cytokines in the PFC and spinal cord, which were found to be increased in the LPS-treated animals (Figure 10).
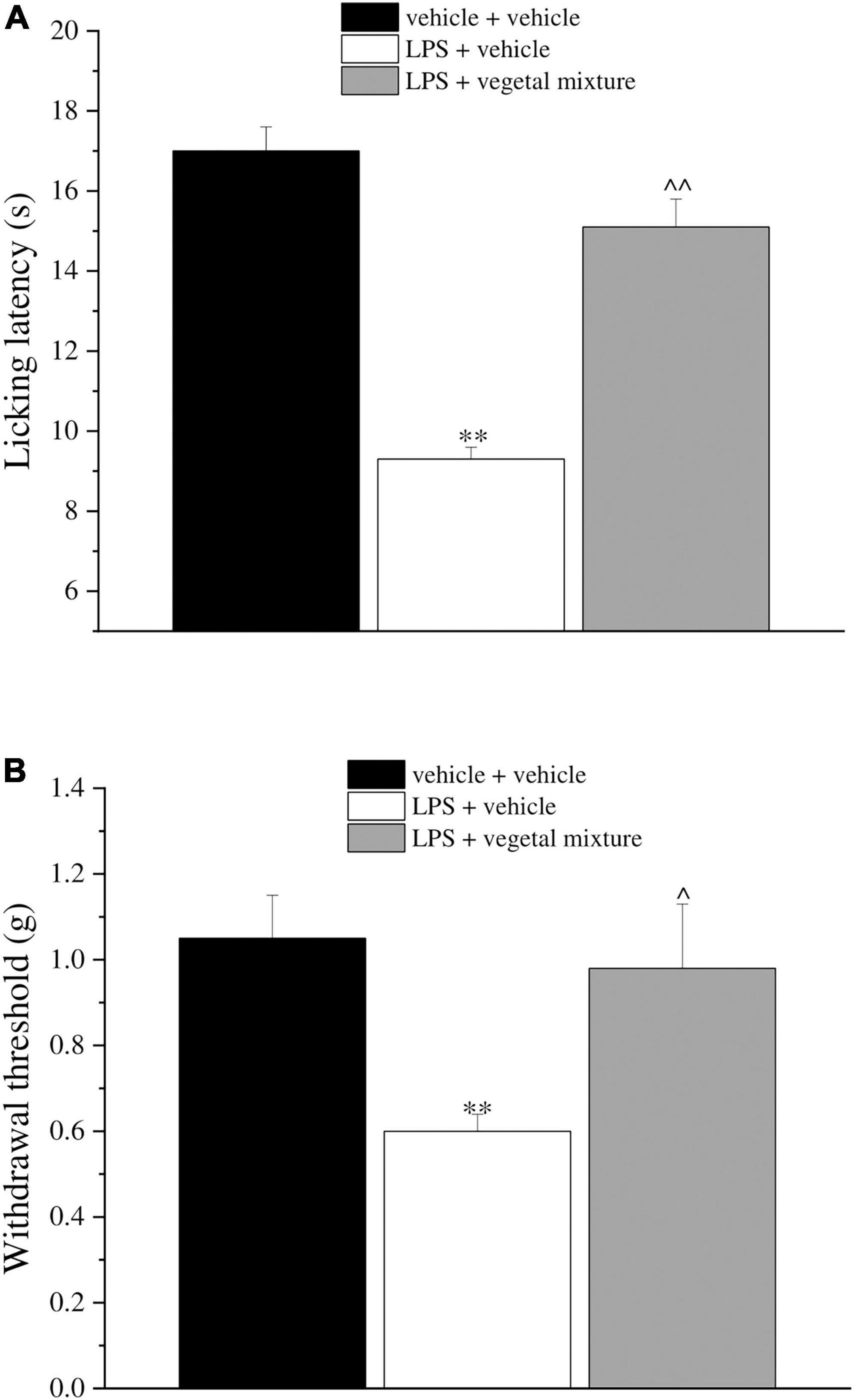
Figure 1. Effect of a vegetal mixture on LPS-induced thermal and mechanical allodynia. LPS (1 mg kg– 1) was intraperitoneally injected on days 1, 3, 5, and 8. The vegetal mixture was composed of Zingiber officinale (150 mg kg– 1), Echinacea purpurea (20 mg kg– 1), and Centella asiatica (200 mg kg– 1), suspended in 1% carboxymethylcellulose sodium salt (CMC) and orally administered daily starting from day 1 till the end of the experiment. Control animals were treated with vehicles. On day 9 and 24 h after the last administration of the vegetal mixture, (A) thermal and (B) mechanical allodynia was assessed by the Cold plate and von Frey tests, respectively. Data are expressed as the mean ± SEM of values from eight mice analyzed in two different experimental sets. **p < 0.01 vs. vehicle + vehicle; ∧p < 0.05 and ∧∧p < 0.01 vs. LPS + vehicle.
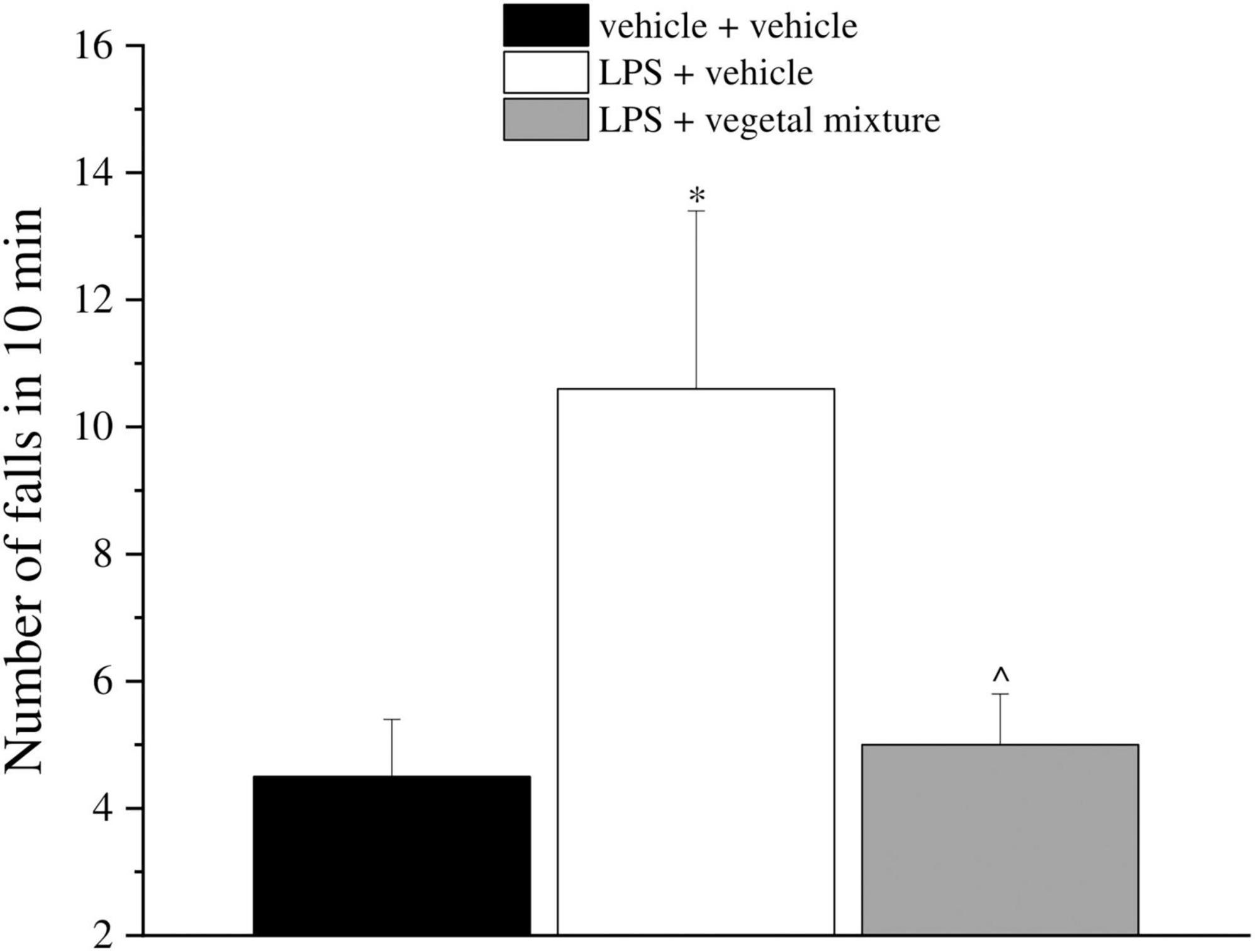
Figure 2. Effect of a vegetal mixture on LPS-induced motor alterations. LPS (1 mg kg– 1) was intraperitoneally injected on days 1, 3, 5, and 8. The vegetal mixture was composed of Zingiber officinale (150 mg kg– 1), Echinacea purpurea (20 mg kg– 1), and Centella asiatica (200 mg kg– 1), suspended in 1% carboxymethylcellulose sodium salt (CMC) and orally administered daily starting from day 1 till the end of the experiment. Control animals were treated with vehicles. Starting from day 9 and 24 h after the last administration of the vegetal mixture, the motor coordination was assessed by the Rota rod test. Data are expressed as the mean ± SEM of values from eight mice analyzed in two different experimental sets. *p < 0.05 vs. vehicle + vehicle; ∧p < 0.05 vs. LPS + vehicle.
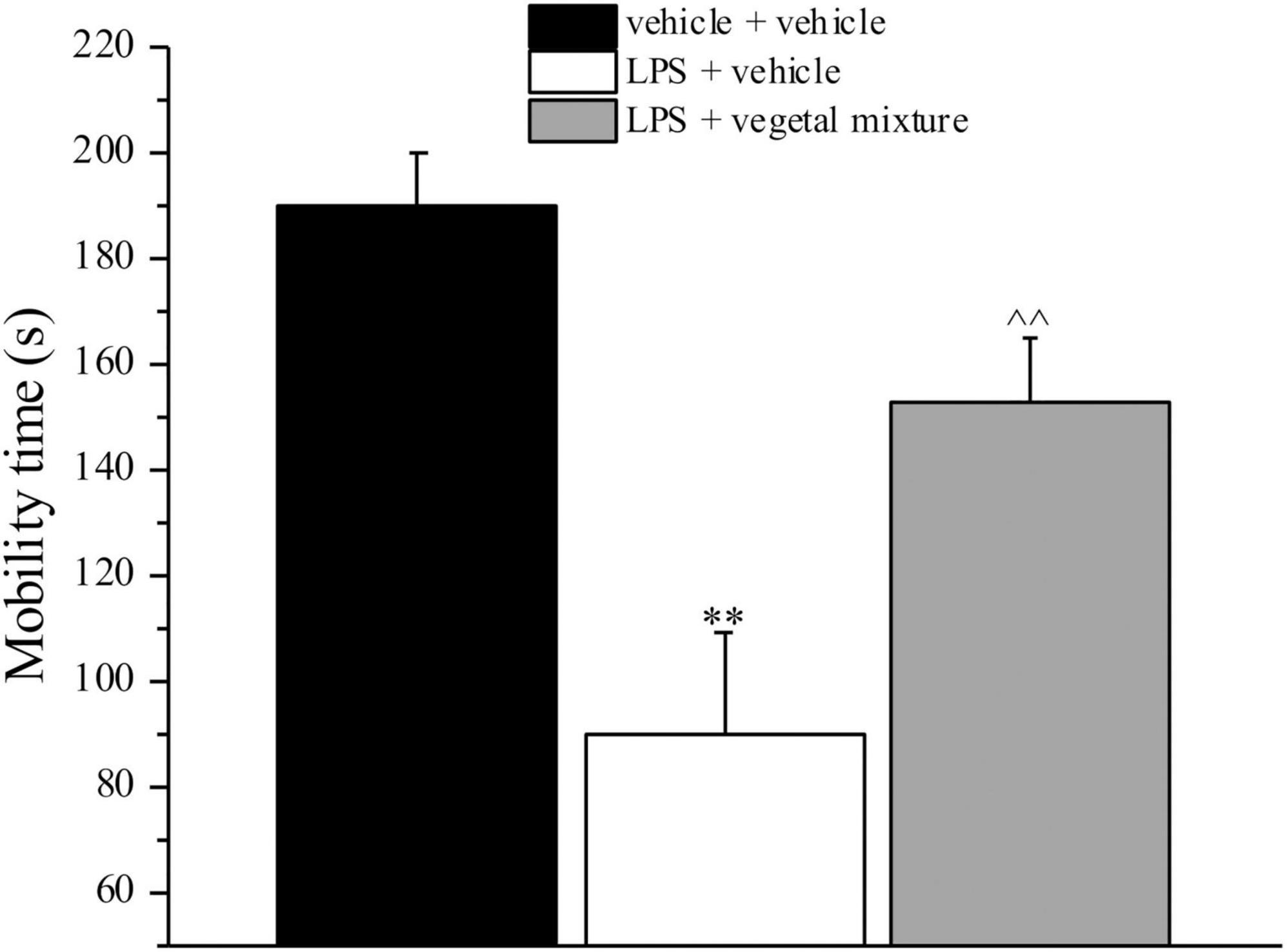
Figure 3. Effect of a vegetal mixture on LPS-induced depression-like behavior. LPS (1 mg kg– 1) was intraperitoneally injected on days 1, 3, 5, and 8. The vegetal mixture was composed of Zingiber officinale (150 mg kg– 1), Echinacea purpurea (20 mg kg– 1), and Centella asiatica (200 mg kg– 1), suspended in 1% carboxymethylcellulose sodium salt (CMC) and orally administered daily starting from day 1 till the end of the experiment. Control animals were treated with vehicles. Starting from day 9 and 24 h after the last administration of the vegetal mixture, the depression-like behavior was assessed by the Porsolt test. Data are expressed as the mean ± SEM of values from eight mice analyzed in two different experimental sets. **p < 0.01 vs. vehicle + vehicle; ∧∧p < 0.01 vs. LPS + vehicle.
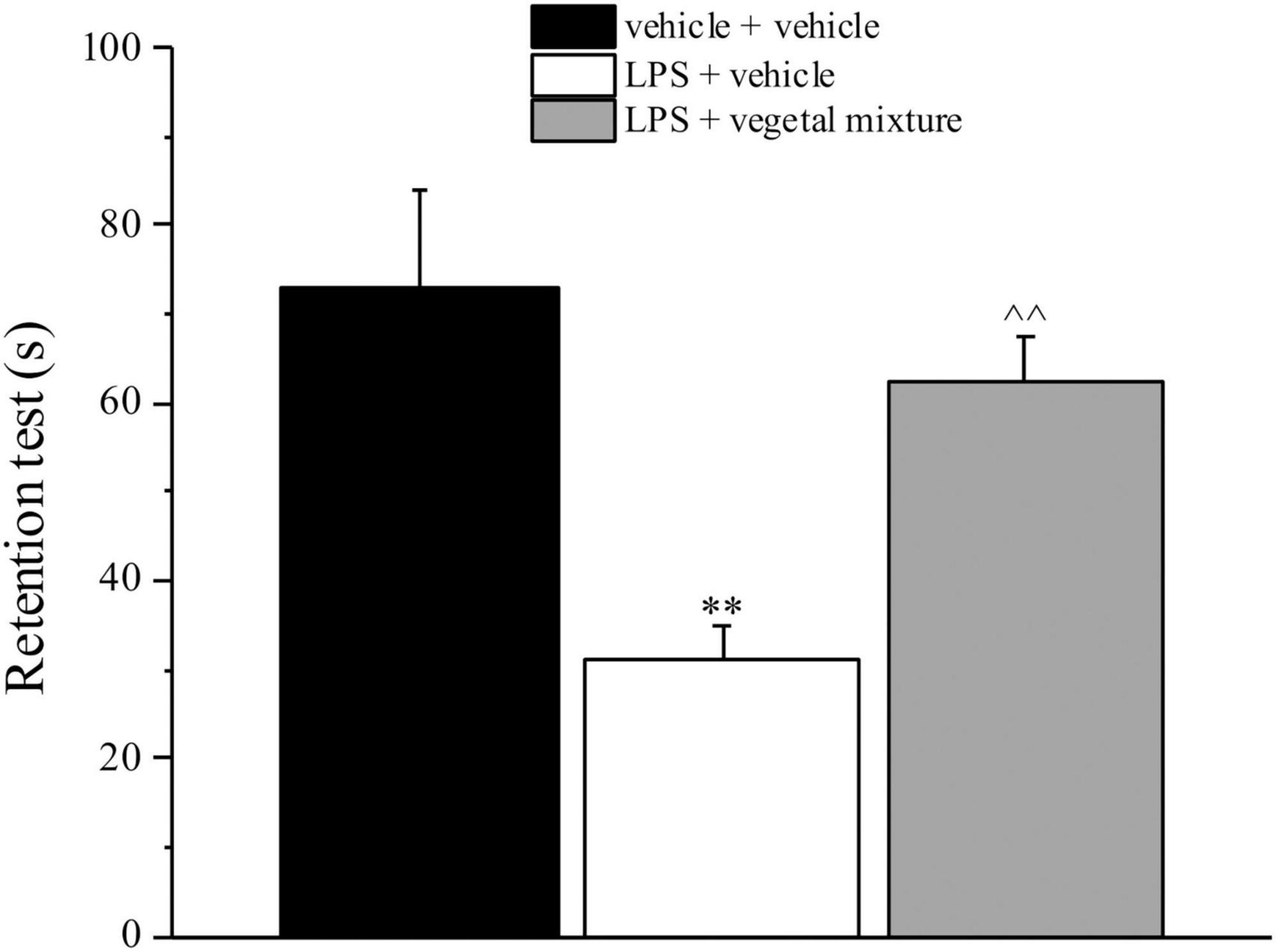
Figure 4. Effect of a vegetal mixture on LPS-induced memory loss. LPS (1 mg kg– 1) was intraperitoneally injected on days 1, 3, 5, and 8. The vegetal mixture was composed of Zingiber officinale (150 mg kg– 1), Echinacea purpurea (20 mg kg– 1), and Centella asiatica (200 mg kg– 1), suspended in 1% carboxymethylcellulose sodium salt (CMC) and orally administered daily starting from day 1 till the end of the experiment. Control animals were treated with vehicles. Starting from day 9 and 24 h after the last administration of the vegetal mixture, the memory was assessed by the Passive avoidance test. Data are expressed as the mean ± SEM of values from eight mice analyzed in two different experimental sets. **p < 0.01 vs. vehicle + vehicle; ∧∧p < 0.01 vs. LPS + vehicle.
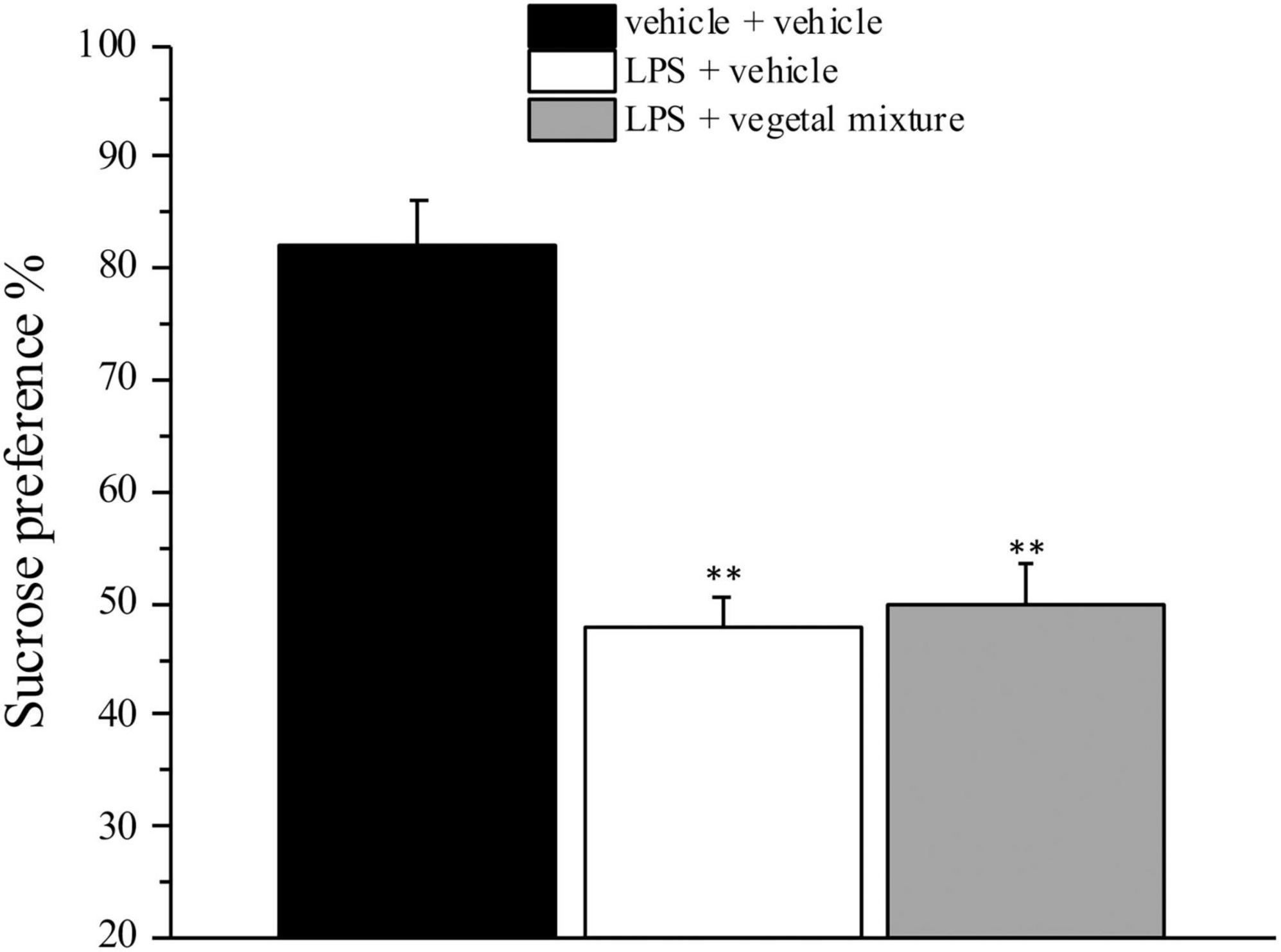
Figure 5. Effect of a vegetal mixture on LPS-induced anhedonia. LPS (1 mg kg– 1) was intraperitoneally injected on days 1, 3, 5, and 8. The vegetal mixture was composed of Zingiber officinale (150 mg kg– 1), Echinacea purpurea (20 mg kg– 1), and Centella asiatica (200 mg kg– 1), suspended in 1% carboxymethylcellulose sodium salt (CMC) and orally administered daily starting from day 1 till the end of the experiment. Control animals were treated with vehicles. Starting from day 9 and 24 h after the last administration of the vegetal mixture, anhedonia was assessed by the Sucrose preference test. Data are expressed as the mean ± SEM of values from eight mice analyzed in two different experimental sets. **p < 0.01 vs. vehicle + vehicle.
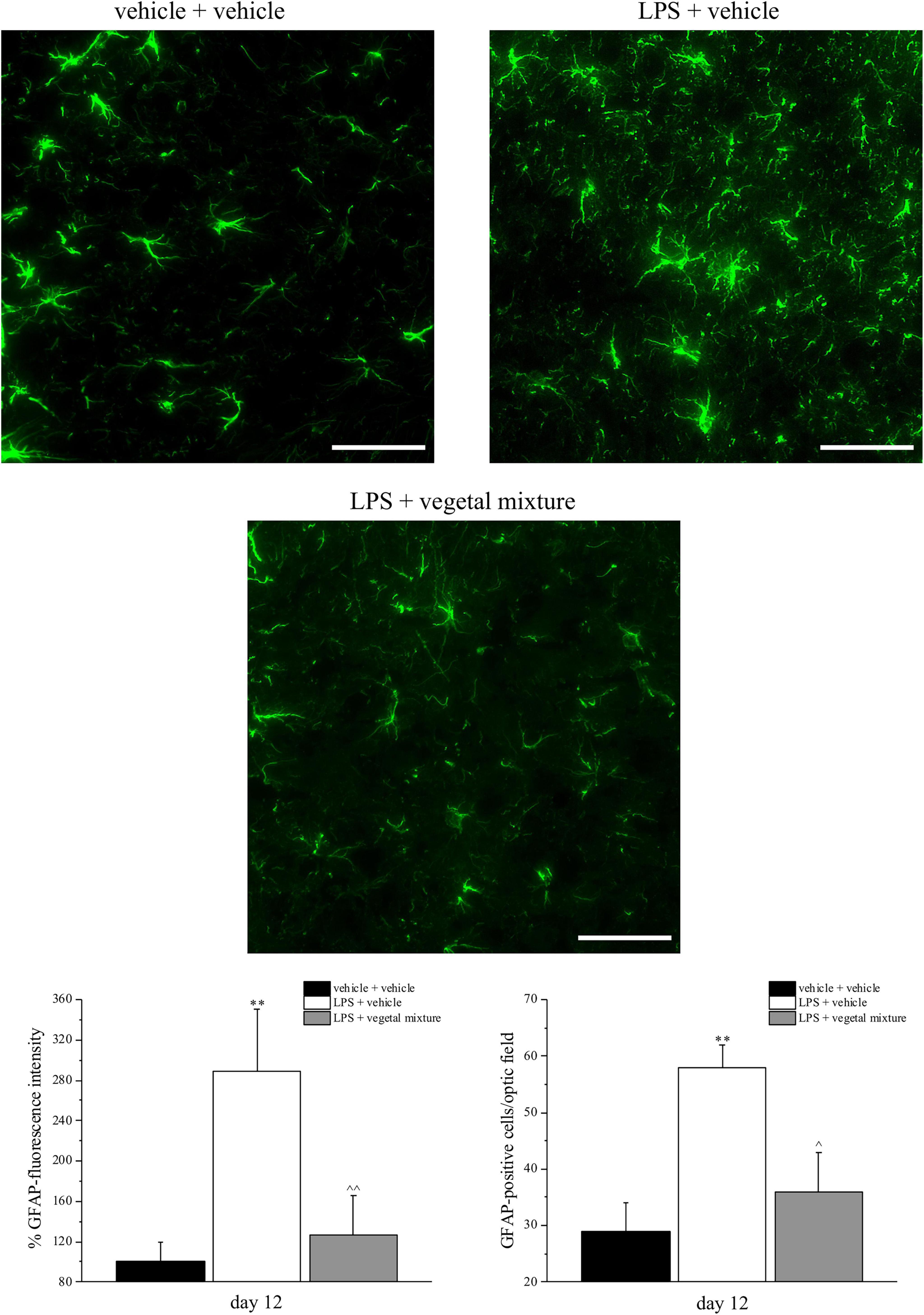
Figure 6. Effect of a vegetal mixture on LPS-induced astrocyte activation. LPS (1 mg kg– 1) was intraperitoneally injected on days 1, 3, 5, and 8. The vegetal mixture was composed of Zingiber officinale (150 mg kg– 1), Echinacea purpurea (20 mg kg– 1), and Centella asiatica (200 mg kg– 1), suspended in 1% carboxymethylcellulose sodium salt (CMC) and orally administered daily starting from day 1 till the end of the experiment. Control animals were treated with vehicles. At the end of the behavioral experiments and 24 h after the last administration of the vegetal mixture, animals were sacrificed and the lumbar spinal cord was collected. The number of GFAP-positive cells was measured in the dorsal horn of the L4–L5 spinal cord. Transverse sections of the spinal cord were imaged with a × 40 objective lens (scale bar = 50 μm). Histograms show the quantitative analysis of GFAP fluorescence intensity and the number of GFAP-positive cells/optic fields. Data are expressed as the mean ± SEM of values from eight mice analyzed in two different experimental sets. **p < 0.01 vs. vehicle + vehicle; ∧p < 0.05 and ∧∧p < 0.01 vs. LPS + vehicle.
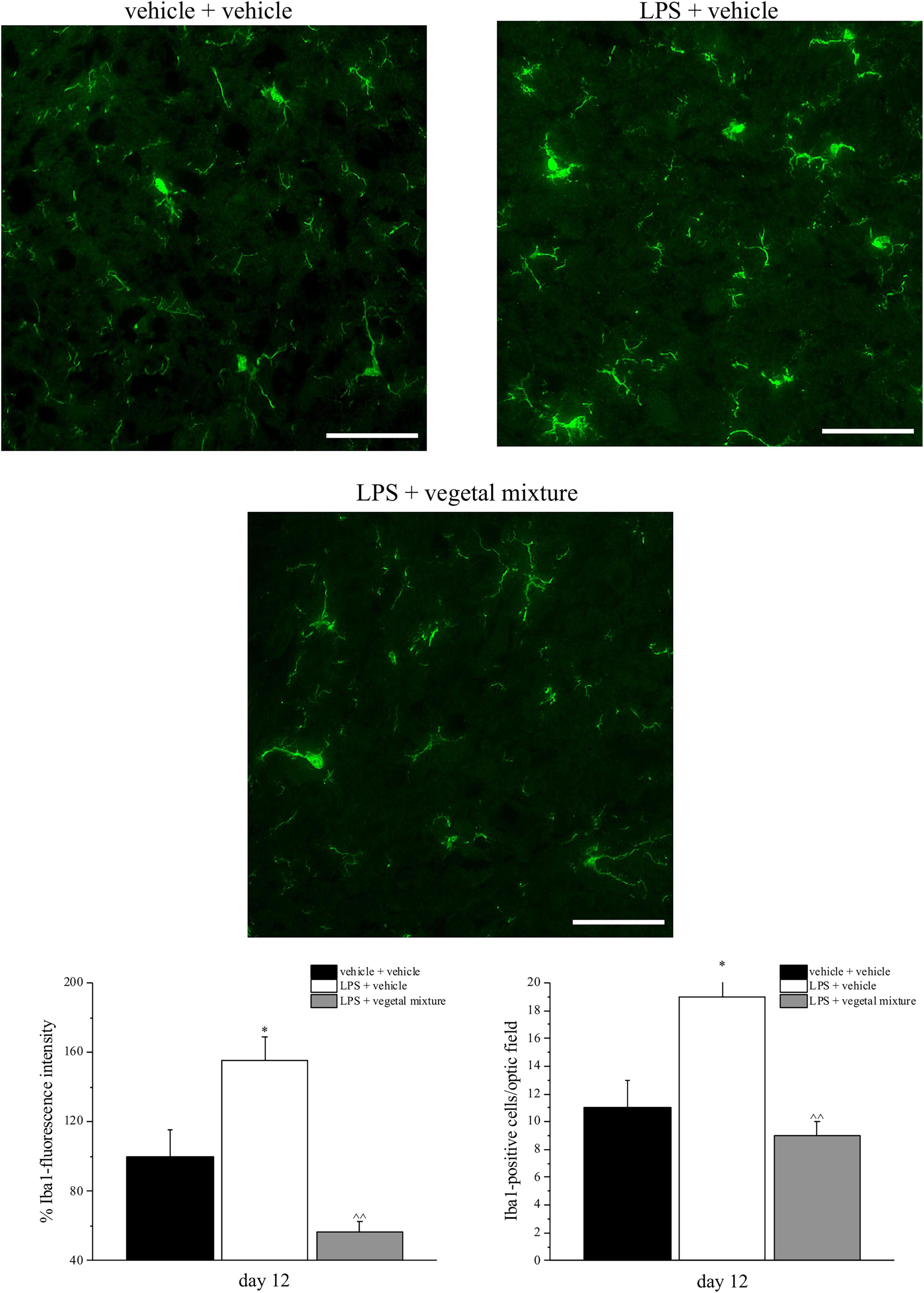
Figure 7. Effect of a vegetal mixture on LPS-induced microglial activation. LPS (1 mg kg– 1) was intraperitoneally injected on days 1, 3, 5, and 8. The vegetal mixture was composed of Zingiber officinale (150 mg kg– 1), Echinacea purpurea (20 mg kg– 1), and Centella asiatica (200 mg kg– 1), suspended in 1% carboxymethylcellulose sodium salt (CMC) and orally administered daily starting from day 1 till the end of the experiment. Control animals were treated with vehicles. At the end of the behavioral experiments and 24 h after the last administration of the vegetal mixture, animals were sacrificed and the lumbar spinal cord was collected. The number of Iba1-positive cells was measured in the dorsal horn of the L4–L5 spinal cord. Transverse sections of the spinal cord were imaged with a × 40 objective lens (scale bar = 50 μm). Histograms show the quantitative analysis of Iba1 fluorescence intensity and the number of Iba1-positive cells/optic fields. Data are expressed as the mean ± SEM of values from eight mice analyzed in two different experimental sets. *p < 0.05 vs. vehicle + vehicle; ∧∧p < 0.01 vs. LPS + vehicle.
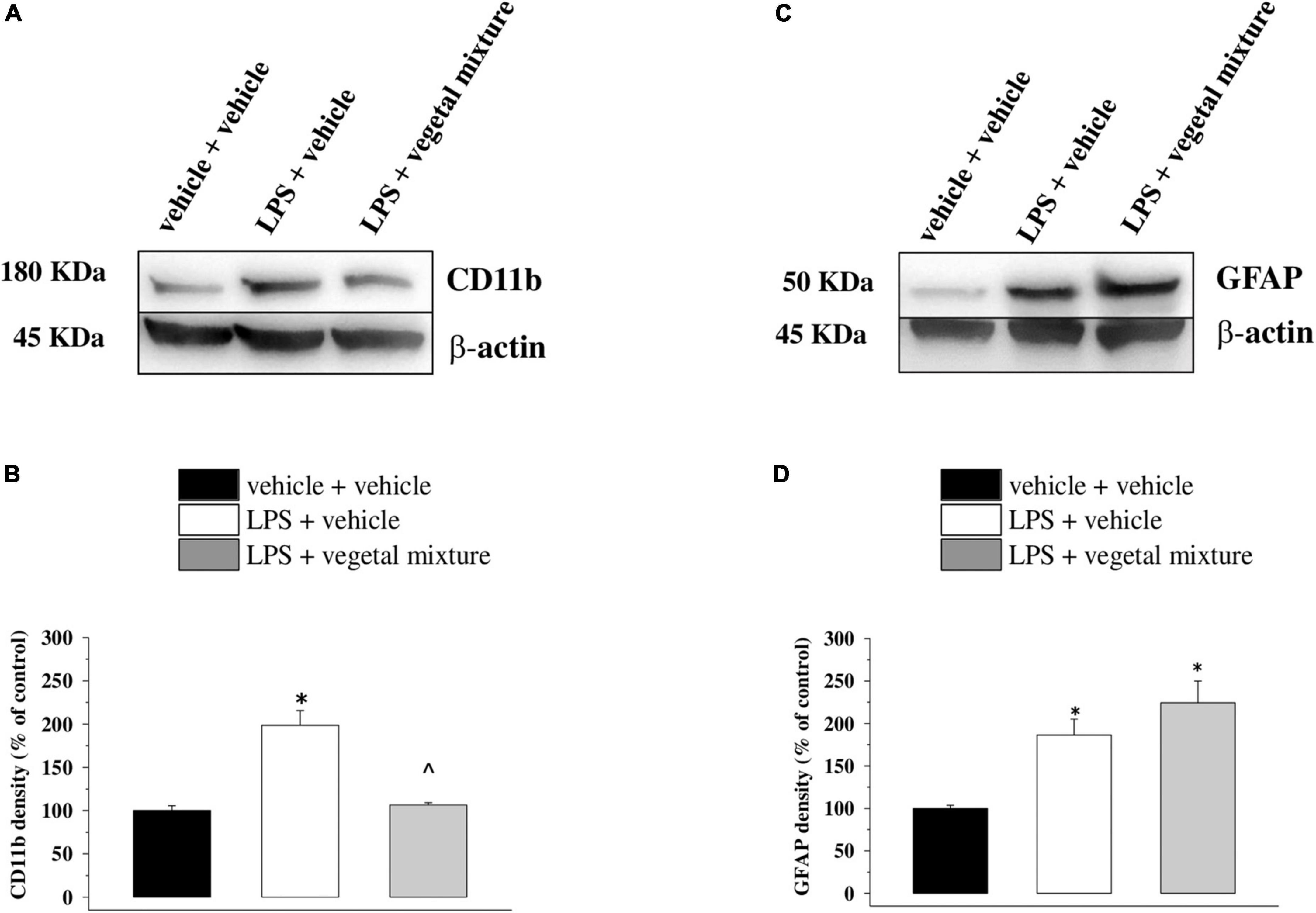
Figure 8. Effect of a vegetal mixture on LPS-induced microglia and astrocyte activation in the prefrontal cortex. LPS treatment and sacrifice of mice were carried out as described in the legend in Figure 7. The prefrontal cortex (PFC) was collected and analyzed for the density of the CD11b protein, a marker of microglia, and of GFAP, a marker of astrocytes. (A) Representative Western blot of the immunostainings for CD11b and β-actin, in vehicle-treated, LPS-treated, and LPS + vegetal mixture-treated mouse PFC lysates. The protein β-actin was used as an internal control. The blot is representative of the analysis of four animals for each experimental group. (B) Quantification of the change of CD11b density in the PFC in vehicle-treated, LPS + vehicle, and LPS + vegetal mixture-treated mice. Results are calculated as CD11b/β-actin ratio and are expressed as a percentage of the respective ratio in vehicle-treated mice. (C) Representative Western blot of the immunostainings for GFAP and β-actin, in vehicle-treated, LPS-treated, and LPS + vegetal mixture-treated mouse PFC lysates. The protein β-actin was used as an internal control. The blot is representative of the analysis of four animals for each experimental group. (D) Quantification of the change of GFAP density in the PFC of mice as described above, respectively. Data are expressed as mean ± SEM of eight mice analyzed in two different experimental sets. *p < 0.05 vs. vehicle-treated mice; ∧p < 0.05 vs. LPS-treated mice.
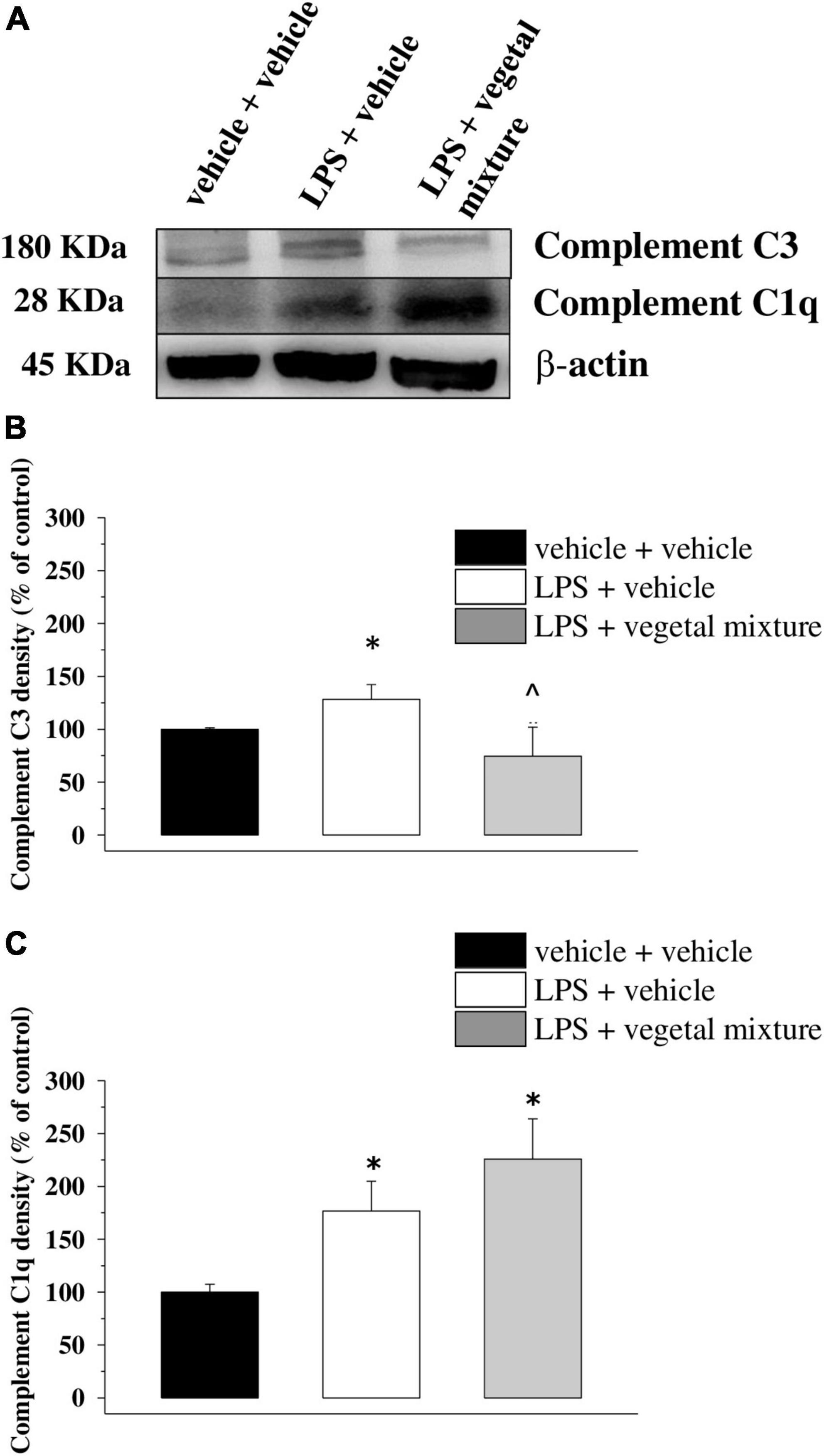
Figure 9. Effect of a vegetal mixture on LPS-induced overproduction of complement components in the prefrontal cortex. The prefrontal cortex (PFC) lysates were analyzed for the density of the C1q and the C3 proteins. (A) Representative Western blot of the immunostainings for C3, C1q, and β-actin, in the PFC lysates of vehicle-treated, LPS-treated, and LPS + vegetal mixture-treated mice. The protein β-actin was used as an internal control. The blot is representative of the analysis of four animals for each experimental group. (B) Quantification of the change of C3 density in the PFC of vehicle-treated, LPS + vehicle, and LPS + vegetal mixture-treated mice. Results are calculated as C3 ÷β-actin ratio and are expressed as a percentage of the respective ratio in vehicle-treated mice. (C) Quantification of the change of C1q density in the PFC of mice as above. Data are expressed as mean ± SEM of eight mice analyzed in two different experimental sets. *p < 0.05 vs. vehicle-treated mice; ∧p < 0.05 vs. LPS-treated mice.
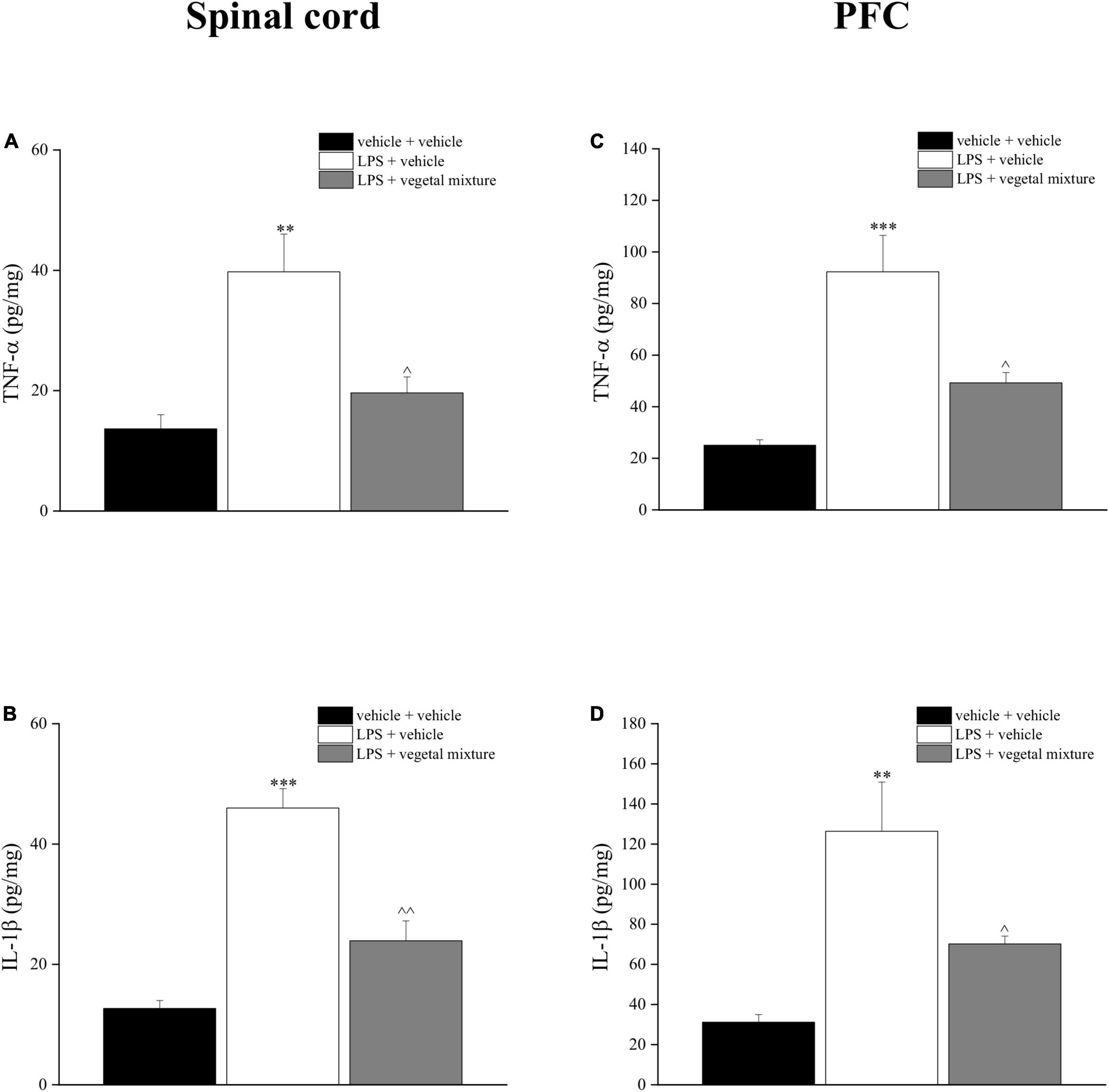
Figure 10. Effect of a vegetal mixture on LPS-induced overproduction of TNF-α and IL-1β levels in the prefrontal cortex and spinal cord. LPS (1 mg kg– 1) was intraperitoneally injected on days 1, 3, 5, and 8. The vegetal mixture was composed of Zingiber officinale (150 mg kg– 1), Echinacea purpurea (20 mg kg– 1), and Centella asiatica (200 mg kg– 1), suspended in 1% carboxymethylcellulose sodium salt (CMC) and orally administered daily starting from day 1 till the end of the experiment. Control animals were treated with vehicles. At the end of the behavioral experiments and 24 h after the last administration of the vegetal mixture, animals were sacrificed and the prefrontal cortex and the lumbar spinal cord were collected. (A) TNF-α and (B) IL-1β concentrations in the spinal cord of vehicle-treated, LPS-treated, and LPS + vegetal mixture-treated mice. (C) TNF-α and (D) IL-1β concentrations in the PFC of vehicle-treated, LPS-treated, and LPS + vegetal mixture-treated mice. Data are expressed as mean ± SEM of eight mice analyzed in two different experimental sets. **p < 0.01 and ***p < 0.001 vs. vehicle-treated mice; ∧p < 0.05 and ∧∧p < 0.01 vs. LPS-treated mice.
Discussion
Neuroinflammation has been implicated as a pathological contributor in several diseases affecting the nervous system, and alleviation of neuroinflammation is believed to reduce disease severity and improve patient outcomes in the majority of cases. Diseases involving the nervous system and different neurodegenerative syndromes, excluding those simply associated with aging, are currently one of the great problems in global health, and there is no doubt that neuroinflammation is involved to a lesser or greater extent in most brain pathologies, whether chronic, neurodegenerative, or acute brain damage, for a multitude of different causes. To note, recent studies have highlighted that SARS-CoV-2 infection also induces neuroinflammation with severe long-term consequences (46) due to the evoking of cytokine storm (47).
In this work, we highlighted the efficacy of a vegetal mixture composed of Echinacea Purpurea, Centella asiatica, and Zingiber officinale in a mouse model of neuroinflammation evoked by the sub-chronic injection of LPS. In particular, the mixture was able to counteract pain, depression, and motor and memory impairments, all pathologies which are associated with neuroinflammation. Moreover, ex vivo analysis demonstrated the efficacy of the treatment in reducing glial cell activation in the spinal cord and in stabilizing the central component proteins that were increased by LPS.
It has been described that systemic infection is associated with an increased frequency of behavioral and cognitive complications (48, 49). The stimulation of the peripheral immune system in mice evoked massive neuroinflammation, which results in prolonged sickness (10), impaired working memory (50), and depressive-like behavior (51).
In this context, it is crucial to design new therapies that can simultaneously act on various aspects, such as improving the recovery from symptoms, controlling the inflammatory and antioxidant processes, and modulating the immune response to attenuate the progression of the disease.
Over the years, the phytotherapeutic approach has been shown to be a valid strategy against human diseases and disorders. The plants were used for medicinal purposes since ancient times because the transcripts describe their effectiveness against various disorders. Several epidemiological studies reported a close correlation between the intake of a diet rich in antioxidant phytochemicals and a lower incidence of age-related neurological disorders for which inflammation is the core pathophysiological mechanism (52). In this study, the neuroinflammatory processes were evoked by the sub-chronic injection of LPS, a toll-like receptor 4 (TLR-4) ligand (53). TLR-4 is primarily expressed in microglia in the CNS (54), and its activation determined the production of proinflammatory cytokines, prostaglandins, and nitric oxide (NO) (55, 56), the key mediators of the neuroinflammatory processes. After four injections of LPS, mice reported mechanical and thermal allodynia as measured by the von Frey and Cold plate tests, confirming that inflammation causes an alteration in the mouse pain threshold and induces pathological hypersensitivity, which is the first step from physiological nociception to persistent pain (57). Concomitantly, animals developed behavioral symptoms predictive of mood and cognitive alterations that were largely prevented by the vegetal treatment. All the pain-relieving effects evoked by the treatment can be attributed to the complexity of the bioactive compounds present in the vegetal mixture. An important phenolic component emerged from the phytochemical characterization, in particular, Zingiber officinale extract contained gingerols (3.107%) and 6-shogaol (0.647%), which have been found to be effective in alleviating inflammation, especially in inflammatory bowel diseases (58, 59), and in reducing pain evoked by formalin and acetic acid injection in mice (60). In this context, LPS caused an overproduction of proinflammatory cytokines, such as TNF-α and IL-1β in the prefrontal cortex and spinal cord regions, which was counteracted by the vegetal mixture. A study by Ha and colleagues demonstrated the neuroprotective role of 6-shogaol in a BV-2 and primary microglial cell cultures stimulated by LPS and in an in vivo model of neuroinflammation. In particular, these effects seem to be related to both anti-inflammatory and antioxidant properties exerted by 6-shogaol on activated microglia, the resident immune cells of the nervous system (61). Centella asiatica is characterized by the presence of 2.6% phenols, mainly constituted by flavonoids and phenylpropanoid derivatives (34). This extract has already been demonstrated to possess anti-hyperalgesic properties in a rat model of osteoarthritis and reduce the NO imbalance in a cell line of macrophages stimulated by LPS, thus highlighting the antioxidant capacity of these compounds (34). In addition, Centella is enriched in polyphenols, including rosmarinic acid which possesses anti-inflammatory activities (62, 63) because of its ability to positively control the complement cascade pathways (64). Moreover, extensive literature reported the neuroprotective role of Centella asiatica. Indeed, its oral supplementation resulted in a strong memory enhancement effect in the preclinical studies (65), and the positive modulation of cognitive functions has also been confirmed in elderly volunteers (66). In an aged Alzheimer’s disease mouse model, water extract of Centella asiatica improved cognitive functions via increasing the Nrf2 gene expression in the hippocampus and by reducing Aβ plaque-associated SOD1 in the hippocampus and cortex (67).
Echinacea purpurea extract is predominantly (6%) constituted by phenolic compounds (0.037% chlorogenic acid, 3.825% cicoric acid, and 2.083% caftaric acid), which exhibit immunomodulatory and antioxidant properties (28). It is important to mention that Echinacea preparations, particularly obtained from E. purpurea, have been proposed to be used as a preventive treatment or therapeutic adjuvant against COVID-19 and as an immune-boosting agent in people at risk or with comorbidities, owing to its plausible effects on immunity, infection, and inflammation (68). Reports regarding the enhancement of immune function suggest that this effect is related to increased secretion of several cytokines by monocytes, including TNF-α, IL-1, IL-6, and IL-10 (69). Even if each extract produces effective results on its own, the mixture contains dozens of compounds with different therapeutic effects that act by different mechanisms, thus making possible a manifold approach to the complexity of the disease evoked by LPS.
The alteration in the mouse pain threshold was not the only symptom caused by LPS, but it also caused mood disorders like depression and anhedonia, and memory and motor impairments. All these alterations were counteracted by the treatment with plant extract except for anhedonia. A number of studies indicate how neuroinflammation is linked to the neurotransmitter acetylcholine which helps organisms to filter the enormous amounts of information received from the environment.
Particularly, in neuroinflammation, the α7 nicotinic acetylcholine receptor (α7 nAChR) is mostly involved, since it regulates cognitive functions and inflammatory reactions.
In the sensory cortex, it acts by fine-tuning the activity of neurons to enhance attention, which subsequently helps with learning and memory (70–72). Patients with neurodegenerative diseases exhibit a lower expression of acetylcholinesterase neurons, choline acetyltransferase, and acetylcholine synthesis, release, and re-uptake. Moreover, it is well-known how α7 nicotinic acetylcholine receptor (nAChRs) plays an important role in the management of neuropathic pain (73–75). It has been demonstrated that systematic injections of LPS reduced the density of α7 nAChRs in the brain that physiologically promote the internalization of processed Aβ forms (76, 77). Subsequently, the decrease in the density of α7 nAChR on the plasma membrane impairs Aβ metabolism and promotes the accumulation of extracellular Aβ (78, 79); this might be the mechanism by which LPS leads to neuroinflammation and cognitive impairments. It has been reported by the literature that zingiber extract restores acetylcholine levels and exhibits protective ability and cognitive enhancing properties during ethanol withdrawal (80), as well as Centella asiatica extract inhibits acetylcholinesterase, inflammation, and oxidative stress activities (81). These findings support the data obtained in this study regarding the efficacy of the vegetal mixture on all symptoms analyzed.
The important cell population in the CNS is represented by glial cells, predominantly microglia and astrocytes (82). Among their functions are the regulation of PH and ionic balance for the maintaining of homeostasis, uptake and degradation of neurotransmitters, and modulation of neuroinflammation in physiological and pathological conditions (83, 84). Several studies pointed out the pivotal role of glial cells in the generation and preservation of chronic pain (85, 86). Also, neuropathic (87) and pharmacological strategies to suppress the activity of microglial cells and astrocytes are being explored as therapies demonstrating a valid option in the management of chronic and neuropathic pain conditions (87). Although LPS may induce the activation of satellite glial cells in the dorsal root ganglia (88), the importance of glial cell activation in the spinal cord has recently been suggested (89, 90). In LPS-treated mice, we recorded an increase in the number of GFAP-positive cells and upregulation of GFAP expression, as well as an increase in the number of Iba1-positive cells and upregulation of Iba1 expression. The maladaptive plasticity of the glial cells in the spinal cord was attenuated by the treatment with the vegetal mixture, thus highlighting the importance of the inhibition of these cell populations in the control of neuroinflammation. Astrocytosis and microgliosis also emerged in the PFC and could be predictive of amnesic symptoms observed in LPS-treated mice. Activated microglia and astrocytes are also central sources of the complement proteins, particularly C1q and C3 proteins, which in physiological conditions regulate neuronal development by suppressing weak synapses, but if aberrantly expressed, exacerbate synaptic derangements and central inflammation, contributing to the cognitive and mood decline associated with aging or to neurodegenerative disorders, i.e., Alzheimer’s disease (91, 92).
In this context, a significant reduction of the C3 density in the PFC of the LPS-treated mice receiving the mixture could be predictive of anti-inflammatory activity and the amelioration of the cognitive performances observed in in vivo studies. The positive impact of the vegetal mixture is of even more interest considering the concomitant persistence of a high level of C1q, which if over-expressed in the absence of other complement components modulates the gene expression critical for neuronal survival (93).
Conclusion
In conclusion, the present work demonstrated the efficacy of daily treatment with a vegetal mixture composed of Zingiber officinale, Centella asiatica, and Echinacea purpurea extracts in the control of neuroinflammation induced by LPS in mice. In particular, mood disorder, pain, and motor and cognition impairments were counteracted. Moreover, the vegetal mixture induced a reduction in the activation of glial cells in the spinal cord and PFC. The results obtained in this study represent an important milestone for the use of phytotherapies in the control of neuroinflammation.
Data availability statement
The raw data supporting the conclusions of this article will be made available by the authors, without undue reservation.
Ethics statement
Formal approval to conduct the experiments described was obtained from the Animal Subjects Review Board of the University of Florence.
Author contributions
LM, CG, and LD: conceptualization. CP, JL, EL, and AT: methodology. CC and VF: software. LM and EL: validation. GO: formal analysis. MB and DC: resources. LM and AP: data curation. LuM: original draft preparation. LuM, CG, LD, SP, and AP: reviewing and editing. CG, AP, and LD: supervision. CG and LD: project administration. CG: funding acquisition. All authors have read and agreed to the published version of the manuscript and have contributed substantially to the work reported.
Funding
This research was funded by the Italian Ministry of Instruction, University and Research (MIUR) and by the University of Florence.
Conflict of interest
LuM, CP, MB, and JL were employed by the company Aboca SpA Società Agricola.
The remaining authors declare that the research was conducted in the absence of any commercial or financial relationships that could be construed as a potential conflict of interest.
Publisher’s note
All claims expressed in this article are solely those of the authors and do not necessarily represent those of their affiliated organizations, or those of the publisher, the editors and the reviewers. Any product that may be evaluated in this article, or claim that may be made by its manufacturer, is not guaranteed or endorsed by the publisher.
Footnotes
References
1. Frank-Cannon TC, Alto LT, McAlpine FE, Tansey MG. Does neuroinflammation fan the flame in neurodegenerative diseases? Mol Neurodegener. (2009) 4:47. doi: 10.1186/1750-1326-4-47
2. Walker AK, Kavelaars A, Heijnen CJ, Dantzer R. Neuroinflammation and comorbidity of pain and depression. Pharmacol Rev. (2014) 66:80–101. doi: 10.1124/pr.113.008144
3. Hirsch EC, Vyas S, Hunot S. Neuroinflammation in Parkinson’s disease. Parkinsonism Relat Disord. (2012) 18:S210—-S212. doi: 10.1016/S1353-8020(11)70065-7
4. Pimplikar SW. Neuroinflammation in Alzheimer’s disease: From pathogenesis to a therapeutic target. J Clin Immunol. (2014) 34:64–9. doi: 10.1007/s10875-014-0032-5
5. Möller T. Neuroinflammation in Huntington’s disease. J Neural Transm. (2010) 117:1001–8. doi: 10.1007/s00702-010-0430-7
6. Frohman EM, Racke MK, Raine CS. Multiple sclerosis – The plaque and its pathogenesis. N Engl J Med. (2006) 354:942–55. doi: 10.1056/NEJMra052130
7. Biesmans S, Meert TF, Bouwknecht JA, Acton PD, Davoodi N, De Haes P, et al. Systemic immune activation leads to neuroinflammation and sickness behavior in mice. Mediators Inflamm. (2013) 2013:1–14. doi: 10.1155/2013/271359
8. Ownby RL. Neuroinflammation and cognitive aging. Curr Psychiatry Rep. (2010) 12:39–45. doi: 10.1007/s11920-009-0082-1
9. Hein AM, O’Banion MK. Neuroinflammation and memory: The role of prostaglandins. Mol Neurobiol. (2009) 40:15–32. doi: 10.1007/s12035-009-8066-z
10. Godbout JP, Chen J, Abraham J, Richwine AF, Berg BM, Kelley KW, et al. Exaggerated neuroinflammation and sickness behavior in aged mice after activation of the peripheral innate immune system. FASEB J. (2005) 19:1329–31. doi: 10.1096/fj.05-3776fje
11. Sparkman NL, Johnson RW. Neuroinflammation associated with aging sensitizes the brain to the effects of infection or stress. Neuroimmunomodulation. (2008) 15:323–30. doi: 10.1159/000156474
12. Rivera RM, Carballea D. Coronavirus: A trigger for OCD and illness anxiety disorder? Psychol Trauma. (2020) 12:S66. doi: 10.1037/tra0000725
13. Nezgovorova V, Ferretti CJ, Pallanti S, Hollander E. Modulating neuroinflammation in COVID-19 patients with obsessive-compulsive disorder. J Psychiatr Res. (2021) 149:367–73. doi: 10.1016/j.jpsychires.2021.11.025
14. Azkur AK, Akdis M, Azkur D, Sokolowska M, van de Veen W, Brüggen M-C, et al. Immune response to SARS-CoV-2 and mechanisms of immunopathological changes in COVID-19. Allergy. (2020) 75:1564–81. doi: 10.1111/all.14364
15. Nile SH, Nile A, Qiu J, Li L, Jia X, Kai G. COVID-19: Pathogenesis, cytokine storm and therapeutic potential of interferons. Cytokine Growth Factor Rev. (2020) 53:66–70. doi: 10.1016/j.cytogfr.2020.05.002
16. Ellis A, Bennett DLH. Neuroinflammation and the generation of neuropathic pain. Br J Anaesth. (2013) 111:26–37. doi: 10.1093/bja/aet128
17. Xanthos DN, Sandkühler J. Neurogenic neuroinflammation: Inflammatory CNS reactions in response to neuronal activity. Nat Rev Neurosci. (2014) 15:43–53. doi: 10.1038/nrn3617
18. Chen W-W, Zhang X, Huang W-J. Role of neuroinflammation in neurodegenerative diseases (review). Mol Med Rep. (2016) 13:3391–6. doi: 10.3892/mmr.2016.4948
19. Colalto C. What phytotherapy needs: Evidence-based guidelines for better clinical practice. Phyther Res. (2018) 32:413–25. doi: 10.1002/ptr.5977
20. Han YA, Song CW, Koh WS, Yon GH, Kim YS, Ryu SY, et al. Anti-inflammatory effects of the Zingiber officinale roscoe constituent 12-dehydrogingerdione in lipopolysaccharide-stimulated raw 264.7 cells: Anti-inflammatory effect of 12-dehydrogingerdione. Phyther Res. (2013) 27:1200–5. doi: 10.1002/ptr.4847
21. Grzanna R, Lindmark L, Frondoza CG. Ginger–an herbal medicinal product with broad anti-inflammatory actions. J Med Food. (2005) 8:125–32. doi: 10.1089/jmf.2005.8.125
22. Tjendraputra E, Tran VH, Liu-Brennan D, Roufogalis BD, Duke CC. Effect of ginger constituents and synthetic analogues on cyclooxygenase-2 enzyme in intact cells. Bioorg Chem. (2001) 29:156–63. doi: 10.1006/bioo.2001.1208
23. Verma SK, Singh M, Jain P, Bordia A. Protective effect of ginger, Zingiber officinale Rosc on experimental atherosclerosis in rabbits. Indian J Exp Biol. (2004) 42:736–8.
24. Mahluji S, Ostadrahimi A, Mobasseri M, Ebrahimzade Attari V, Payahoo L. Anti-inflammatory effects of Zingiber officinale in type 2 diabetic patients. Adv Pharm Bull. (2013) 3:273–6. doi: 10.5681/apb.2013.044
25. Srivastava KC, Mustafa T. Ginger (Zingiber officinale) and rheumatic disorders. Med Hypotheses. (1989) 29:25–8. doi: 10.1016/0306-9877(89)90162-x
26. Stratz T, Müller W. The use of 5-HT3 receptor antagonists in various rheumatic diseases–a clue to the mechanism of action of these agents in fibromyalgia? Scand J Rheumatol Suppl. (2000) 113:66–71. doi: 10.1080/030097400446689
27. Mao QQ, Xu XY, Cao SY, Gan RY, Corke H, Beta T, et al. Bioactive compounds and bioactivities of ginger (Zingiber officinale Roscoe). Foods. (2019) 8:185. doi: 10.3390/foods8060185
28. Panickar KS, Anderson RA. Effect of polyphenols on oxidative stress and mitochondrial dysfunction in neuronal death and brain edema in cerebral ischemia. Int J Mol Sci. (2011) 12:8181–207. doi: 10.3390/ijms12118181
29. Sloley BD, Urichuk LJ, Tywin C, Coutts RT, Pang PK, Shan JJ. Comparison of chemical components and antioxidants capacity of different Echinacea species. J Pharm Pharmacol. (2001) 53:849–57. doi: 10.1211/0022357011776009
30. Hu C, Kitts DD. Studies on the antioxidant activity of Echinacea root extract. J Agric Food Chem. (2000) 48:1466–72.
31. Woelkart K, Bauer R. The role of alkamides as an active principle of Echinacea. Planta Med. (2007) 73:615–23. doi: 10.1055/s-2007-981531
32. Belcaro G, Maquart F-X, Scoccianti M, Dugall M, Hosoi M, Cesarone MR, et al. TECA (Titrated extract of Centella Asiatica): New microcirculatory, biomolecular, and vascular application in preventive and clinical medicine. A status paper. Panminerva Med. (2011) 53:105–18.
33. Singh RH, Narsimhamurthy K, Singh G. Neuronutrient impact of ayurvedic rasayana therapy in brain aging. Biogerontology. (2008) 9:369–74. doi: 10.1007/s10522-008-9185-z
34. Micheli L, Di Cesare Mannelli L, Mattoli L, Tamimi S, Flamini E, Garetto S, et al. Intra-articular route for the system of molecules 14G1862 from Centella asiatica: Pain relieving and protective effects in a rat model of osteoarthritis. Nutrients. (2020) 12:1618. doi: 10.3390/nu12061618
35. McGrath JC, Lilley E. Implementing guidelines on reporting research using animals (ARRIVE etc.): New requirements for publication in BJP. Br J Pharmacol. (2015) 172:3189–93. doi: 10.1111/bph.12955
36. Sakurai M, Egashira N, Kawashiri T, Yano T, Ikesue H, Oishi R. Oxaliplatin-induced neuropathy in the rat: Involvement of oxalate in cold hyperalgesia but not mechanical allodynia. Pain. (2009) 147:165–74. doi: 10.1016/j.pain.2009.09.003
37. Micheli L, Durante M, Lucarini E, Sgambellone S, Lucarini L, Di Cesare Mannelli L, et al. The histamine H4 receptor participates in the anti-neuropathic effect of the adenosine A3 receptor agonist IB-MECA: Role of CD4+ T cells. Biomolecules. (2021) 11:1447. doi: 10.3390/biom11101447
38. Baptista-de-Souza D, Di Cesare Mannelli L, Zanardelli M, Micheli L, Nunes-de-Souza RL, Canto-de-Souza A, et al. Serotonergic modulation in neuropathy induced by oxaliplatin: Effect on the 5HT2C receptor. Eur J Pharmacol. (2014) 735:141–9. doi: 10.1016/j.ejphar.2014.04.028
39. Micheli L, Di Cesare Mannelli L, Del Bello F, Giannella M, Piergentili A, Quaglia W, et al. The use of the selective imidazoline I1 receptor agonist carbophenyline as a strategy for neurpathic pain relief: Preclinical evaluation in a mouse model of oxaliplatin-induced neurotoxicity. Neurotherapeutics. (2020) 17:1005–15. doi: 10.1007/s13311-020-00873-y
40. Jarvik ME, Kopp R. An improved one-trial passive avoidance learning situation. Psychol Rep. (1967) 21:221–4. doi: 10.2466/pr0.1967.21.1.221
41. Porsolt RD, Le Pichon M, Jalfre M. Depression: A new animal model sensitive to antidepressant treatments. Nature. (1977) 266:730–2. doi: 10.1038/266730a0
42. Micheli L, Spitoni S, Di Cesare Mannelli L, Bilia AR, Ghelardini C, Pallanti S. Bacopa monnieri as augmentation therapy in the treatment of anhedonia, preclinical and clinical evaluation. Phytother Res. (2020) 34:2331–40. doi: 10.1002/ptr.6684
43. Di Cesare Mannelli L, Maresca M, Micheli L, Farina C, Scherz MW, Ghelardini C. A rat model of FOLFOX-induced neuropathy: Effects of oral dimiracetam in comparison with duloxetine and pregabalin. Cancer Chemother Pharmacol. (2017) 80:1091–103. doi: 10.1007/s00280-017-3449-8
44. Tenner AJ, Stevens B, Woodruff TM. New tricks for an ancient system: Physiological and pathological roles of complement in the CNS. Mol Immunol. (2018) 102:3–13. doi: 10.1016/j.molimm.2018.06.264
45. Kanmogne M, Klein RS. Neuroprotective versus neuroinflammatory roles of complement: From development to disease. Trends Neurosci. (2021) 44:97–109. doi: 10.1016/j.tins.2020.10.003
46. Almutairi MM, Sivandzade F, Albekairi TH, Alqahtani F, Cucullo L. Neuroinflammation and its impact on the pathogenesis of COVID-19. Front Med. (2021) 8:745789. doi: 10.3389/fmed.2021.745789
47. Zhou Y, Fu B, Zheng X, Wang D, Zhao C, Qi Y, et al. Aberrant pathogenic GM-CSF+ T cells and inflammatory CD14+CD16+ monocytes in severe pulmonary syndrome patients of a new coronavirus. bioRxiv. (2020). [Preprint]. doi: 10.1101/2020.02.12.945576
48. Evans DL, Charney DS, Lewis L, Golden RN, Gorman JM, Krishnan KRR, et al. Mood disorders in the medically Ill: Scientific review and recommendations. Biol Psychiatry. (2005) 58:175–89. doi: 10.1016/j.biopsych.2005.05.001
49. Penninx BWJH, Kritchevsky SB, Yaffe K, Newman AB, Simonsick EM, Rubin S, et al. Inflammatory markers and depressed mood in older persons: Results from the health, aging and body composition study. Biol Psychiatry. (2003) 54:566–72. doi: 10.1016/S0006-3223(02)01811-5
50. Chen J, Buchanan JB, Sparkman NL, Godbout JP, Freund GG, Johnson RW. Neuroinflammation and disruption in working memory in aged mice after acute stimulation of the peripheral innate immune system. Brain Behav Immun. (2008) 22:301–11. doi: 10.1016/j.bbi.2007.08.014
51. Godbout JP, Moreau M, Lestage J, Chen J, Sparkman NL, Connor JO, et al. Aging exacerbates depressive-like behavior in mice in response to activation of the peripheral innate immune system. Neuropsychopharmacology. (2008) 33:2341–51. doi: 10.1038/sj.npp.1301649
52. Lau FC, Shukitt-Hale B, Joseph JA. Nutritional intervention in brain aging: Reducing the effects of inflammation and oxidative stress. Subcell Biochem. (2007) 42:299–318.
53. Beutler B. Tlr4: Central component of the sole mammalian LPS sensor. Curr Opin Immunol. (2000) 12:20–6. doi: 10.1016/S0952-7915(99)00046-1
54. Lehnardt S, Lachance C, Patrizi S, Lefebvre S, Follett PL, Jensen FE, et al. The toll-like receptor TLR4 is necessary for lipopolysaccharide-induced oligodendrocyte injury in the CNS. J Neurosci. (2002) 22:2478–86. doi: 10.1523/JNEUROSCI.22-07-02478.2002
55. McGeer PL, McGeer EG, Yasojima K. Alzheimer disease and neuroinflammation. In: Jellinger K, Schmidt R, Windisch M editors. Advances in dementia research. (Vienna: Springer Vienna) (2000). p. 53–7. doi: 10.1007/978-3-7091-6781-6_8
56. Mrak RE, Griffin WST. Glia and their cytokines in progression of neurodegeneration. Neurobiol Aging. (2005) 26:349–54. doi: 10.1016/j.neurobiolaging.2004.05.010
57. Xu Q, Yaksh TL. A brief comparison of the pathophysiology of inflammatory versus neuropathic pain. Curr Opin Anaesthesiol. (2011) 24:400–7. doi: 10.1097/ACO.0b013e32834871df
58. Zhang G, Nitteranon V, Chan LY, Parkin KL. Glutathione conjugation attenuates biological activities of 6-dehydroshogaol from ginger. Food Chem. (2013) 140:1–8. doi: 10.1016/j.foodchem.2013.02.073
59. Yan H, Zhang E, Feng C, Zhao X. Role of A3 adenosine receptor in diabetic neuropathy: Adenosine and neuropathy. J Neurosci Res. (2016) 94:936–46. doi: 10.1002/jnr.23774
60. Young H-Y, Luo Y-L, Cheng H-Y, Hsieh W-C, Liao J-C, Peng W-H. Analgesic and anti-inflammatory activities of [6]-gingerol. J Ethnopharmacol. (2005) 96:207–10. doi: 10.1016/j.jep.2004.09.009
61. Ha SK, Moon E, Ju MS, Kim DH, Ryu JH, Oh MS, et al. 6-Shogaol, a ginger product, modulates neuroinflammation: A new approach to neuroprotection. Neuropharmacology. (2012) 63:211–23. doi: 10.1016/j.neuropharm.2012.03.016
62. Rampart M, Beetens JR, Bult H, Herman AG, Parnham MJ, Winkelmann J. Complement-dependent stimulation of prostacyclin biosynthesis: Inhibition by rosmarinic acid. Biochem Pharmacol. (1986) 35:1397–400. doi: 10.1016/0006-2952(86)90289-3
63. Nuytinck JKS, Goris RJA, Kalter ES, Schillings PHM. Inhibition of experimentally induced microvascular injury by rosmarinic acid. Agents Actions. (1986) 17:373–4. doi: 10.1007/BF01982651
64. Sahu A, Rawal N, Pangburn MK. Inhibition of complement by covalent attachment of rosmarinic acid to activated C3b. Biochem Pharmacol. (1999) 57:1439–46. doi: 10.1016/S0006-2952(99)00044-1
65. Subathra M, Shila S, Devi MA, Panneerselvam C. Emerging role of Centella asiatica in improving age-related neurological antioxidant status. Exp Gerontol. (2005) 40:707–15. doi: 10.1016/j.exger.2005.06.001
66. Tiwari S, Singh S, Patwardhan K, Gehlot S, Gambhir I. Effect of Centella asiatica on mild cognitive impairment (MCI) and other common age-related clinical problems. Dig J Nanomater Biostructures. (2008) 3:215–20.
67. Matthews DG, Caruso M, Murchison CF, Zhu JY, Wright KM, Harris CJ, et al. Centella Asiatica improves memory and promotes antioxidative signaling in 5XFAD mice. Antioxidants. (2019) 8:630. doi: 10.3390/antiox8120630
68. Nagoor Meeran MF, Javed H, Sharma C, Goyal SN, Kumar S, Jha NK, et al. Can Echinacea be a potential candidate to target immunity, inflammation, and infection – The trinity of coronavirus disease 2019. Heliyon. (2021) 7:e05990. doi: 10.1016/j.heliyon.2021.e05990
69. Burger RA, Torres AR, Warren RP, Caldwell VD, Hughes BG. Echinacea-induced cytokine production by human macrophages. Int J Immunopharmacol. (1997) 19:371–9. doi: 10.1016/s0192-0561(97)00061-1
70. Sarter M, Lustig C. Cholinergic double duty: Cue detection and attentional control. Curr Opin Psychol. (2019) 29:102–7. doi: 10.1016/j.copsyc.2018.12.026
71. Lee SH, Dan Y. Neuromodulation of brain states. Neuron. (2012) 76:209–22. doi: 10.1016/j.neuron.2012.09.012
72. Picciotto MR, Higley MJ, Mineur YS. Acetylcholine as a neuromodulator: Cholinergic signaling shapes nervous system function and behavior. Neuron. (2012) 76:116–29. doi: 10.1016/j.neuron.2012.08.036
73. Di Cesare Mannelli L, Micheli L, Maresca M, Cravotto G, Bellumori M, Innocenti M, et al. Anti-neuropathic effects of Rosmarinus officinalis L. terpenoid fraction: Relevance of nicotinic receptors. Sci Rep. (2016) 6:34832. doi: 10.1038/srep34832
74. Di Cesare Mannelli L, Ghelardini C, Calvani M, Nicolai R, Mosconi L, Toscano A, et al. Neuroprotective effects of acetyl-L-carnitine on neuropathic pain and apoptosis: A role for the nicotinic receptor. J Neurosci Res. (2009) 87:200–7. doi: 10.1002/jnr.21815
75. Di Cesare Mannelli L, Pacini A, Matera C, Zanardelli M, Mello T, De Amici M, et al. Involvement of α7 nAChR subtype in rat oxaliplatin-induced neuropathy: Effects of selective activation. Neuropharmacology. (2014) 79:37–48. doi: 10.1016/j.neuropharm.2013.10.034
76. Wang H-Y, Lee DHS, D’Andrea MR, Peterson PA, Shank RP, Reitz AB. β-amyloid1–42 binds to α7 nicotinic acetylcholine receptor with high affinity. J Biol Chem. (2000) 275:5626–32. doi: 10.1074/jbc.275.8.5626
77. Parri RH, Dineley TK. Nicotinic acetylcholine receptor interaction with beta-amyloid: Molecular, cellular, and physiological consequences. Curr Alzheimer Res. (2010) 7:27–39. doi: 10.2174/156720510790274464
78. Gouras GK, Almeida CG, Takahashi RH. Intraneuronal Aβ accumulation and origin of plaques in Alzheimer’s disease. Neurobiol Aging. (2005) 26:1235–44. doi: 10.1016/j.neurobiolaging.2005.05.022
79. Dziewczapolski G, Glogowski CM, Masliah E, Heinemann SF. Deletion of the 7 nicotinic acetylcholine receptor gene improves cognitive deficits and synaptic pathology in a mouse model of Alzheimer’s disease. J Neurosci. (2009) 29:8805–15. doi: 10.1523/JNEUROSCI.6159-08.2009
80. Maralla S, Reddy KS. Effect of ginger on acetylcholine activity in brain and it’s possible role in learning and memory during ethanol withdrawal. Asian J Pharm Clin Res. (2014) 7:192–7.
81. Hafiz ZZ, Amin M, Afif M, Johari James RM, Teh LK, Salleh MZ, et al. Inhibitory effects of raw-extract Centella asiatica (RECA) on acetylcholinesterase, inflammations, and oxidative stress activities via in vitro and in vivo. Molecules. (2020) 25:892. doi: 10.3390/molecules25040892
82. Jha MK, Suk K. Glia-based biomarkers and their functional role in the CNS. Expert Rev Proteomics. (2013) 10:43–63. doi: 10.1586/epr.12.70
83. Gwak YS, Kang J, Unabia GC, Hulsebosch CE. Spatial and temporal activation of spinal glial cells: Role of gliopathy in central neuropathic pain following spinal cord injury in rats. Exp Neurol. (2012) 234:362–72. doi: 10.1016/j.expneurol.2011.10.010
84. Parpura V, Heneka MT, Montana V, Oliet SHR, Schousboe A, Haydon PG, et al. Glial cells in (patho)physiology: Glial cells in (patho)physiology. J Neurochem. (2012) 121:4–27. doi: 10.1111/j.1471-4159.2012.07664.x
85. Barragán-Iglesias P, Pineda-Farias JB, Cervantes-Durán C, Bravo-Hernández M, Rocha-González HI, Murbartián J, et al. Role of spinal P2Y6 and P2Y11 receptors in neuropathic pain in rats: Possible involvement of glial cells. Mol Pain. (2014) 10:29. doi: 10.1186/1744-8069-10-29
86. Watkins LR, Maier SF. Beyond neurons: Evidence that immune and glial cells contribute to pathological pain states. Physiol Rev. (2002) 82:981–1011. doi: 10.1152/physrev.00011.2002
87. Di Cesare Mannelli L, Pacini A, Micheli L, Tani A, Zanardelli M, Ghelardini C. Glial role in oxaliplatin-induced neuropathic pain. Exp Neurol. (2014) 261:22–33. doi: 10.1016/j.expneurol.2014.06.016
88. Blum E, Procacci P, Conte V, Hanani M. Systemic inflammation alters satellite glial cell function and structure. A possible contribution to pain. Neuroscience. (2014) 274:209–17. doi: 10.1016/j.neuroscience.2014.05.029
89. Cao FL, Xu M, Wang Y, Gong KR, Zhang JT. Tanshinone IIA attenuates neuropathic pain via inhibiting glial activation and immune response. Pharmacol Biochem Behav. (2015) 128:1–7. doi: 10.1016/j.pbb.2014.11.004
90. Silva GD, Lopes PSS, Fonoff ET, Pagano RL. The spinal anti-inflammatory mechanism of motor cortex stimulation: Cause of success and refractoriness in neuropathic pain? J Neuroinflamm. (2015) 12:10. doi: 10.1186/s12974-014-0216-1
91. Kettenmann H, Kirchhoff F, Verkhratsky A. Microglia: New roles for the synaptic stripper. Neuron. (2013) 77:10–8. doi: 10.1016/j.neuron.2012.12.023
92. Spejo AB, Oliveira ALR. Synaptic rearrangement following axonal injury: Old and new players. Neuropharmacology. (2015) 96:113–23. doi: 10.1016/j.neuropharm.2014.11.002
Keywords: LPS, neuroinflammation, vegetal mixture, glial cells, pain, mood disorders, CNS complement proteins
Citation: Micheli L, Toti A, Lucarini E, Ferrara V, Ciampi C, Olivero G, Pittaluga A, Mattoli L, Pelucchini C, Burico M, Lucci J, Carrino D, Pacini A, Pallanti S, Di Cesare Mannelli L and Ghelardini C (2022) Efficacy of a vegetal mixture composed of Zingiber officinale, Echinacea purpurea, and Centella asiatica in a mouse model of neuroinflammation: In vivo and ex vivo analysis. Front. Nutr. 9:887378. doi: 10.3389/fnut.2022.887378
Received: 11 March 2022; Accepted: 25 July 2022;
Published: 30 August 2022.
Edited by:
Arpita Roy, Sharda University, IndiaReviewed by:
Marcella Reale, University of Studies G. d’Annunzio Chieti and Pescara, ItalyMd. Jamal Hossain, State University of Bangladesh, Bangladesh
Copyright © 2022 Micheli, Toti, Lucarini, Ferrara, Ciampi, Olivero, Pittaluga, Mattoli, Pelucchini, Burico, Lucci, Carrino, Pacini, Pallanti, Di Cesare Mannelli and Ghelardini. This is an open-access article distributed under the terms of the Creative Commons Attribution License (CC BY). The use, distribution or reproduction in other forums is permitted, provided the original author(s) and the copyright owner(s) are credited and that the original publication in this journal is cited, in accordance with accepted academic practice. No use, distribution or reproduction is permitted which does not comply with these terms.
*Correspondence: Laura Micheli, laura.micheli@unifi.it
†These authors have contributed equally to this work and share first authorship
‡These authors have contributed equally to this work and share last authorship