- Centro de Investigación en Alimentación y Desarrollo, A.C. Coordinación de Tecnología de Alimentos de Origen Animal, Carretera Gustavo Enrique Astiazarán Rosas, Hermosillo, Mexico
Introduction
Humans and animals coexist with microbes and have a vital relationship with many. Most of these microbes are vastly contained in the gastrointestinal tract, defined as the gut microbiota, a critical “symbiont” for the development and survival of humans and animals due to its multiple functionalities favoring the host (1).
The gut microbiota is unique in each individual in terms of microbial structure and function; however, stress, social interactions, diet, nutrient, pharmacologic factors, and many other stimuli, including biological agents like antibiotics and xenobiotics, play critical roles in the modulation of gut microbial composition (2, 3). In this last regard, environmental xenobiotics are chemicals to which an organism is exposed that are extrinsic to its normal metabolism (4); some of these are substances of known toxicity, substances known to be inert, and substances whose toxicity or inertness remains to be established. Toxic xenobiotics include natural (e.g., mycotoxins) as well as synthetic compounds such as pesticides, drugs, additives, heavy metals, food additives. From the mechanistic view, they may come into contact with the gastrointestinal system causing physiological and/or anatomical abnormalities by interfering in the host biological processes. These also can affect the structure and functions of the host's gut microbiota, disrupting the synergistic relationship between the host and its microbiota with subsequent physiological, metabolic, and immune implications (3, 5).
Over the last decade, extensive evidence has shown that probiotics, defined as “live microorganisms that, when administered in adequate amounts, confer a health benefit on the host” (6), are capable of surviving at the gastrointestinal tract after oral intake and reduce the bioaccessibility of xenobiotics by acting as binding agents (7). However, studying the effect of probiotics on shaping the gut microbiota to modulate/modify the dietary xenobiotic lifetime and bioavailability has not been as extensive. Hence, in this document, we support the hypothesis that probiotics can shape the functions of the gut microbiota to withstand and metabolize toxic agents.
Xenobiotics and the Gut Microbiota
Xenobiotics can gain access into living organisms by multiple routes; however, most xenobiotics enter the human body through the gastrointestinal tract. Evidence has determined several scenarios after incorporating a xenobiotic (8–10); here, we list at least five of them: 1 the gut microbiota can directly metabolize the xenobiotic, 2 the gut microbiota can metabolize the xenobiotic after conjugation with the liver (via enteropathic cycling) or, if not metabolized, 3 the xenobiotic may induce dysbiosis, 4 interfere with the enzymatic activity of the gut microbiota, 5 inactivate the xenobiotic by direct interaction (binding) on the cell surface and others (Figure 1).
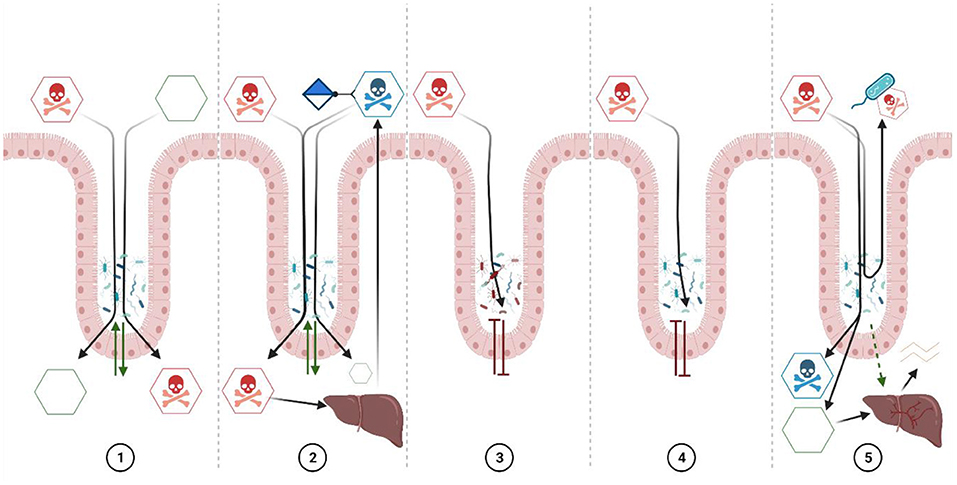
Figure 1. Xenobiotic interaction with the intestinal microbiota. 1. Xenobiotics not affected by oral cavity, stomach, and small intestine digestion, or poorly adsorbed, are displaced to the distal small intestine and cecum by peristalsis, and the microbiota residing in the large intestine may directly metabolize those partitioned through the intestinal wall from the blood. 2. Due to the non-polar nature of most xenobiotics, they are easily absorbed by the intestinal tract and subsequently transported through the bloodstream to the liver where some xenobiotics are oxidized, forming conjugates (with sulfates, glucuronic acid or glutathione) that can be excreted by the bile and metabolized by the microbiota which, in turn, transforms these conjugates into non-polar molecular conjugates of low molecular weight with low toxicity that are adsorbed again; however, the microbiota can also deconjugate these compounds and “release” the xenobiotic again. 3. Some xenobiotics can affect the gut microbiota, either by affecting specific taxonomic groups or favoring others. The function of the intestinal microbiota is affected and, with it, the symbiotic relationship with the host. 4. Although some xenobiotics do not affect the abundance of gut microbiota members, they can affect the function of the microbiota. Some changes may occur in the activity of endogenous metabolites or the general metabolic capacity of the microbiota, also affecting the symbiotic relationship with the host. 5. The intestinal microbiota could induce an enzymatic response at the liver tissue level, increasing the host's detoxification capacity. Also, the microbiota can produce active and inactive variants of the xenobiotic, which can be metabolized by liver tissue. Finally, some microbiota bacteria inactivate the xenobiotic by direct interaction (binding) on the cell surface, decreasing the adsorption of the xenobiotic. However, there could be multiple additional mechanisms that have yet to be elucidated. Based on Claus et al. (8) with modifications and insertions. Created with BioRender.com.
The gut microbiota harbors a diversity of about >1,000 microbial species providing an extensive set of metabolic functions (11). For instance, gut microbes can metabolize dozens of drugs via acetylation, deacetylation, deconjugation, deglycosylation, dehydroxylation, demethylation, denitration, reduction, N-oxide bounds cleavage, proteolysis, etcetera (12). Furthermore, it has been reported that some gut microorganisms can directly alter the chemical structures of xenobiotics; however, the microbial strains and enzymes (and their associated genes) involved in the metabolism of xenobiotics are still poorly understood (13). In this regard, enzyme families such as β-glucuronidases, β-lyases, azoreductases, nitroreductases, and sulfatases have been detected in the gut microbiota (14–16). For instance, gut microbes can reduce a considerable diversity of functional groups such as α,β-unsaturated carboxylic acid derivatives, alkenes, nitro, N-oxide, azo, and sulfoxide groups, decreasing the polarity of compounds with the consequent charge alteration, hybridization, and electrophilicity, their toxicities and lifetimes in the body (13).
Overall, the activity of the gut microbiota reduces the final absorption of xenobiotics by hosts and their bioavailability (17, 18); however, if xenobiotics breach the intestinal epithelium, the gut microbiota can trigger the host's immune response. In this regard, members of the gut microbiota can synthesize and release metabolites capable of modulating the host immune response, altering hepatic gene expression for dealing with xenobiotics, competing for enzymes and drug transporters, and acting as intermediates (18, 19). Withal, as stated, xenobiotics may not always be metabolized, causing dysbiosis by inhibiting, promoting, or eliminating gut microbiota members; therefore, such microbiota members must be protected, and probiotics could play a critical protective role.
Several factors can influence gut microbiome xenobiotic metabolism, including host genome, age, geography, diet, gender, hormonal status, circadian rhythms, and others (9). However, interventions aiming to modulate the gastrointestinal tract's microbial ecology and favoring groups with these functions may provide new insights regarding microbially mediated xenobiotics-transformations for human health benefits.
Use of Probiotics as Regulators of Microbial Interaction Networks in the Gastrointestinal Microbiota: A Hypothetical Approach
Advances in sequencing technologies have revealed the association between dysbiosis (the loss of beneficial gut species, absence of microbial diversity and/or pathogen domination of the gut) and a wide range of human diseases. For instance, studies have evidenced that the imbalance of intestinal microbiota and changes in microbiota composition is closely related to immune-mediated neuropathies, such as Guillain-barre syndrome (GBS), which is characterized by elevated levels of Th1 and Th17 proinflammatory cytokines, reduction of anti-inflammatory Th2 and Th3 cytokines, along with quantitative and qualitative defects of regulatory T cells (Treg), suggesting the crucial roles of these important mediators for the onset and progression of GBS (20). Considering that the microbiota modulates host immune responses and affects the production of cytokines, it has been hypothesized that clinical symptoms of GBS may be improved by regulating the imbalance of intestinal microbiota. In this regard, studies have shown that probiotics (e.g., Lactobacillus helveticus R0052, Bifidobacterium infantis) may improve GBS immune balance by reducing the most common bacteria associated with GBS, namely Campylobacter jejuni, Escherichia, and Coprococcus (21). Besides, probiotic Bifidobacterium infantis administration improves the imbalance of Th cell subsets (Th1, Th2, Th17) and promotes Treg cells' levels, thereby ameliorating GBS symptoms (22, 23).
In the same context, gut dysbiosis is closely associated with colorectal cancer (CRC) development. At the genus and species level, Bacteroides fragilis, Enterococcus faecalis, Streptococcus bovis, Escherichia coli, Fusobacterium spp., Ruminococcus spp., and Peptostreptococcus spp., are suspected to be involved in colorectal carcinogenesis (24). Despite the exact mechanisms involving these bacteria in colorectal pathogenesis are still unclear, in vitro and in vivo studies have evidenced the key role of enterotoxigenic strains, whose respective toxin produced (e.g., colibactin, polyamines) affect pathways leading to increased cell proliferation, the release of proinflammatory effectors, and DNA damage (25). Moreover, considering that bacterial adherence is often a prerequisite step to tumor promotion, virulence factors, such as the afa, eae, and Fap2 adhesins, may induce CRC by selectively binding to E-cadherin and activating the β-catenin signaling pathway, thus inducing oncogenic and inflammatory responses. In this same context, aggregations of microbial communities encased in a polymeric matrix (biofilms) enhanced colonic epithelial permeability, which facilitates bacterial antigen translocation and promotes pro-carcinogenic tissue inflammation. Besides, ROS-producing bacteria can activate pro-carcinogenic factors and CRC-promoting pathways (26).
On the other hand, coincidence in reducing the abundance of butyrate-producing bacteria and in the carbohydrate-degradation genes responsible for SCFA production has been reported in CRC patients. Furthermore, fat-mediated alterations of the gut microbiota link bile acid metabolism to CRC since changes in the gut microbiota may promote an altered overall bile acid pool, which activates or restricts intestinal and hepatic cross-signaling of the bile acid receptor, farnesoid X receptor (FXR), which plays a crucial role in regulating intestinal cell proliferation and carcinogenesis (26, 27).
Based on the aforementioned, gut microbiota dysbiosis, bacterial virulence factors, and microbial-derived metabolism play vital roles in colorectal carcinogenesis; hence, it has been suggested that probiotics may be used to shape the gut microbiota to reduce the risk of colorectal cancer pathogenesis. In this sense, the beneficial effects of probiotics in CRC have been demonstrated in vitro, in vivo, and in preclinical trials, particularly for species of Lactobacillus, Enterococcus, and Bifidobacterium. However, the beneficial impact of probiotic supplementation depends on the strain, dosage, duration of the intervention, host physiology, and other food supplements. Proposed mechanisms of action include modulation or enhancement of immune function, the release of specific bioactive metabolites (e.g., antimicrobial peptides, organic acids, enzymes), improvement of barrier function, epithelial structure and metabolism, and even the microRNAs expression profile in the context of the microbiota composition (28, 29).
Despite the above research progresses, using probiotics for shaping and favoring a specific microbial community that tolerates and can metabolize toxic agents is a rare approach in the area, mainly because understanding the interaction networks between microorganisms to manipulate them is a complex challenge. However, some clues and partial evidence could provide a moderate insight; for instance, the survival of some bacteria even under adverse conditions may be favored by the presence of others. For example, Narisawa et al. (30) demonstrated that resistant bacteria could mediate the coexistence of excluding groups of bacteria like antibiotic-sensitive and antibiotic-producing bacteria. Even though this evidence was obtained from biofilms, the principle could apply to the gut microbiota, which is also a kind of biofilm. In this sense, we arise the question: could some probiotics favor the tolerance of essential but sensitive members of the gut microbiota, even under the presence of toxic agents? Considering that some probiotic mechanisms include components secretions, metabolic activity, and cell to cell interactions that could directly or indirectly favor sensitive members, the theoretical answer is affirmative.
Interaction network models could provide deeper insights to search for bacteria or groups that favor gut microbiota members' persistence with specific functions. In practice, the modulation of commensal microbiota by the consumption of transiting probiotics has been associated with antimicrobial compounds produced by probiotics or indirectly by modulating the immune system and gut barrier function (31); however, these are single links in a vast interaction network. For example, Kato et al. (32) monitored the network relationship of four different bacteria strains capable of degrading cellulose (strains S, F, 3, and 5). Briefly, results revealed that the survival of strain S depended on the effects of strain 5, but strain 5 was susceptible to strain 3 that showed bactericidal activity against this strain. However, strain F quenched the growth of strain 3 and favored the persistence of strain 5. These direct and indirect network relationships, including suppressive effects, resulted in balance, maintaining the colony's coexistence and functioning. Probiotics can be used in this way to favor networks that assist bacteria with specific capabilities, in this case, metabolize toxic agents. For instance, finding co-occurrence and core networks involving microbial specimens capable of metabolizing xenobiotics may provide evidence for designing single-strain or multispecies consortia formulations favoring such networks. Herein, the design of synthetic microbial consortia for gut microbiota modulation is an approach to consider (33).
Whether prebiotics can support the persistence of specific bacterial groups, sometimes these may not be as effective under adverse conditions involving toxic agents harming important microbes. However, the presence of bacteria mediating the persistence of others through the modification of interaction networks may be used; in other words, it is essential to gain insight into the principles driving bacteria-bacteria interactions because bacterial community networks determine central microbiota functions. In addition, the chemical communications between microbes through quorum sensing must be incorporated in this approach because predicting and manipulating these processes could favor the probability of success. However, knowing in deep these networks is a monumental challenge that must be solved by using metadata and robust computer systems that allow predicting the behavior of microbial communities in the presence of a specific probiotic. Also, using Oligo-Mouse-Microbiota 12 (OMM12) synthetic bacterial community as model research to understand microbiota interactions could provide relevant information (34). In this regard, OMM12 is a known community of 12 mouse intestinal bacteria used for microbiome research in gnotobiotic mice (35).
Finally, understanding the impact of intestinal microbiota on toxic agents' metabolism and how probiotics can be used from the different approaches to favor these functions could be a step forward in probiotics science.
Author Contributions
AH-M and MM-P: article conceptualization, writing, and review. AG-C: writing and review. All authors contributed to the article and approved the submitted version.
Conflict of Interest
The authors declare that the research was conducted in the absence of any commercial or financial relationships that could be construed as a potential conflict of interest.
Publisher's Note
All claims expressed in this article are solely those of the authors and do not necessarily represent those of their affiliated organizations, or those of the publisher, the editors and the reviewers. Any product that may be evaluated in this article, or claim that may be made by its manufacturer, is not guaranteed or endorsed by the publisher.
Acknowledgments
We gratefully acknowledge the work of our institution, numerous students, technical staff, and colleagues who have contributed to the body of knowledge related to the subject of this document.
References
1. McKnite AM, Perez-Munoz ME, Lu L, Williams EG, Brewer S, Andreux PA, et al. Murine gut microbiota is defined by host genetics and modulates variation of metabolic traits. PLoS One. (2012) 7:e39191. doi: 10.1371/journal.pone.0039191
2. Kumbhare SV, Patangia DV, Patil RH, Shouche YS, Patil NP. Factors influencing the gut microbiome in children: from infancy to childhood. J Biosci. (2019) 44:1–19. doi: 10.1007/s12038-019-9860-z
3. Chi L, Tu P, Ru H, Lu K. Studies of xenobiotic-induced gut microbiota dysbiosis: from correlation to mechanisms. Gut Microbes. (2021) 13:1921912. doi: 10.1080/19490976.2021.1921912
4. Croom E. Metabolism of xenobiotics of human environments. Prog Mol Biol Transl Sci. (2012) 112:31–88. doi: 10.1016/B978-0-12-415813-9.00003-9
5. Wang G, Pan R, Liang X, Wu X, Wu Y, Zhang H, et al. Perfluorooctanoic acid-induced liver injury is potentially associated with gut microbiota dysbiosis. Chemosphere. (2021) 266:129004. doi: 10.1016/j.chemosphere.2020.129004
6. Hill C, Guarner F, Reid G, Gibson GR, Merenstein DJ, Pot B, et al. The International Scientific Association for Probiotics and Prebiotics consensus statement on the scope and appropriate use of the term probiotic. Nat Rev Gastroenterol Hepatol. (2014) 11:506–14. doi: 10.1038/nrgastro.2014.66
8. Claus SP, Guillou H, Ellero-Simatos S. The gut microbiota: a major player in the toxicity of environmental pollutants? NPJ Biofilms Microbiomes. (2016) 2:1–11. doi: 10.1038/npjbiofilms.2016.3
9. Clarke G, Sandhu KV, Griffin BT, Dinan TG, Cryan JF, Hyland NP. Gut reactions: breaking down xenobiotic–microbiome interactions. Pharmacol Rev. (2019) 71:198–224. doi: 10.1124/pr.118.015768
10. Guerrero-Encinas I, González-González JN, Santiago-López L, Muhlia-Almazán A, Garcia HS, Mazorra-Manzano MA, et al. Protective effect of Lacticaseibacillus casei CRL 431 postbiotics on mitochondrial function and oxidative status in rats with aflatoxin b1–induced oxidative stress. Probiotics Antimicrob Proteins. (2021) 13:1033–43. doi: 10.1007/s12602-021-09747-x
11. Lagier J-C, Dubourg G, Million M, Cadoret F, Bilen M, Fenollar F, et al. Culturing the human microbiota and culturomics. Nat Rev Microbiol. (2018) 16:540–50. doi: 10.1038/s41579-018-0041-0
12. Xie Y, Hu F, Xiang D, Lu H, Li W, Zhao A, et al. The metabolic effect of gut microbiota on drugs. Drug Metab Rev. (2020) 52:139–56. doi: 10.1080/03602532.2020.1718691
13. Koppel N, Maini Rekdal V, Balskus EP. Chemical transformation of xenobiotics by the human gut microbiota. Science. (2017) 356:eaag2770. doi: 10.1126/science.aag2770
14. Asano Y, Hiramoto T, Nishino R, Aiba Y, Kimura T, Yoshihara K, et al. Critical role of gut microbiota in the production of biologically active, free catecholamines in the gut lumen of mice. Am J Physiol Gastrointest Liver Physiol. (2012) 303:G1288–95. doi: 10.1152/ajpgi.00341.2012
15. Zhang J, Zhang J, Wang R, Jia Z. Effects of gut microbiota on drug metabolism and guidance for rational drug use under hypoxic conditions at high altitudes. Curr Drug Metab. (2019) 20:155–65. doi: 10.2174/1389200219666181019145159
16. Misal SA, Gawai KR. Azoreductase: a key player of xenobiotic metabolism. Bioresour Bioprocess. (2018) 5:1–9. doi: 10.1186/s40643-018-0206-8
17. Collins SL, Patterson AD. The gut microbiome: an orchestrator of xenobiotic metabolism. Acta Pharm Sin B. (2020) 10:19–32. doi: 10.1016/j.apsb.2019.12.001
18. Nakov R, Velikova T. Chemical metabolism of xenobiotics by gut microbiota. Curr Drug Metab. (2020) 21:260–9. doi: 10.2174/1389200221666200303113830
19. Feng W, Liu J, Ao H, Yue S, Peng C. Targeting gut microbiota for precision medicine: focusing on the efficacy and toxicity of drugs. Theranostics. (2020) 10:11278. doi: 10.7150/thno.47289
20. Sun T, Chen X, Shi S, Liu Q, Cheng Y. Peripheral blood and cerebrospinal fluid cytokine levels in Guillain Barre syndrome: a systematic review and meta-analysis. Front Neurosci. (2019) 13:717. doi: 10.3389/fnins.2019.00717
21. Saxena A. Probiotics as a potential alternative for relieving peripheral neuropathies: a case for Guillain-Barre syndrome. Front Microbiol. (2016) 6:1497. doi: 10.3389/fmicb.2015.01497
22. Shi P, Qu H, Nian D, Chen Y, Liu X, Li Q, et al. Treatment of Guillain-Barré syndrome with Bifidobacterium infantis through regulation of T helper cells subsets. Int Immunopharmacol. (2018) 61:290–6. doi: 10.1016/j.intimp.2018.06.015
23. Shi P, Nian D, Qu H, Sun J, Li Q, Li Q, et al. Pathogenic mechanism of intestinal microbiota involved in Guillain-Barre syndrome and with Bifidobacterium intervention. Arch Med Sci. (2022). doi: 10.5114/aoms/128103
24. Pino A, De Angelis M, Chieppa M, Caggia C, Randazzo C. Gut microbiota, probiotics and colorectal cancer: a tight relation. World Cancer Res J. (2020) 7:1456. Available online at: https://www.wcrj.net/wp-content/uploads/sites/5/2020/01/e1456-Gut-microbiota-probiotics-and-colorectal-cancer-a-tight-relation.pdf
25. Louis P, Hold GL, Flint HJ. The gut microbiota, bacterial metabolites and colorectal cancer. Nat Rev Microbiol. (2014) 12:661–72. doi: 10.1038/nrmicro3344
26. Cheng Y, Ling Z, Li L. The intestinal microbiota and colorectal cancer. Front Immunol. (2020) 11:615056. doi: 10.3389/fimmu.2020.615056
27. Torres-Maravilla E, Boucard A-S, Mohseni AH. Taghinezhad-S S, Cortes-Perez NG, Bermúdez-Humarán LG. Role of gut microbiota and probiotics in colorectal cancer: onset and progression. Microorganisms. (2021) 9:1021. doi: 10.3390/microorganisms9051021
28. Wierzbicka A, Mańkowska-Wierzbicka D, Mardas M, Stelmach-Mardas M. Role of probiotics in modulating human gut microbiota populations and activities in patients with colorectal cancer—A systematic review of clinical trials. Nutrients. (2021) 13:1160. doi: 10.3390/nu13041160
29. Sivamaruthi BS, Kesika P, Chaiyasut C. The role of probiotics in colorectal cancer management. Evid Based Comp Altern Med. (2020) 2020:3535982. doi: 10.1155/2020/3535982
30. Narisawa N, Haruta S, Arai H, Ishii M, Igarashi Y. Coexistence of antibiotic-producing and antibiotic-sensitive bacteria in biofilms is mediated by resistant bacteria. Appl Environ Microbiol. (2008) 74:3887–94. doi: 10.1128/AEM.02497-07
31. Bajaj BK, Claes IJ, Lebeer S. Functional mechanisms of probiotics. J Microbiol Biotechnol Food Sci. (2021) 2021:321–7. doi: 10.15414/jmbfs.2015.4.4.321-327
32. Kato S, Haruta S, Cui ZJ, Ishii M, Igarashi Y. Network relationships of bacteria in a stable mixed culture. Microb Ecol. (2008) 56:403–11. doi: 10.1007/s00248-007-9357-4
33. Vázquez-Castellanos JF, Biclot A, Vrancken G, Huys GR, Raes J. Design of synthetic microbial consortia for gut microbiota modulation. Curr Opin Pharmacol. (2019) 49:52–9. doi: 10.1016/j.coph.2019.07.005
34. Weiss AS, Burrichter AG, Durai Raj AC, von Strempel A, Meng C, Kleigrewe K, et al. In vitro interaction network of a synthetic gut bacterial community. ISME J. (2022) 16:1095–109. doi: 10.1038/s41396-021-01153-z
Keywords: probiotics, gut microbiota, xenobiotic absorption, bioremediation, dysbiosis, toxic agent
Citation: Hernández-Mendoza A, González-Córdova AF and Martínez-Porchas M (2022) Influence of Probiotics on the Animal Gut Microbiota and Their Impact on the Bioavailability of Toxic Agents: An Opinion Paper. Front. Nutr. 9:870162. doi: 10.3389/fnut.2022.870162
Received: 06 February 2022; Accepted: 04 April 2022;
Published: 18 April 2022.
Edited by:
Xiangkai Li, Lanzhou University, ChinaReviewed by:
Iman Zarei, University of Eastern Finland, FinlandCopyright © 2022 Hernández-Mendoza, González-Córdova and Martínez-Porchas. This is an open-access article distributed under the terms of the Creative Commons Attribution License (CC BY). The use, distribution or reproduction in other forums is permitted, provided the original author(s) and the copyright owner(s) are credited and that the original publication in this journal is cited, in accordance with accepted academic practice. No use, distribution or reproduction is permitted which does not comply with these terms.
*Correspondence: Marcel Martínez-Porchas, bWFyY2VsQGNpYWQubXg=
†These authors have contributed equally to this work and share first authorship