- Department of Biomedical Sciences, University of Cagliari, Cagliari, Italy
Palmitic acid (PA) is ubiquitously present in dietary fat guaranteeing an average intake of about 20 g/d. The relative high requirement and relative content in the human body, which accounts for 20–30% of total fatty acids (FAs), is justified by its relevant nutritional role. In particular physiological conditions, such as in the fetal stage or in the developing brain, the respectively inefficient placental and brain blood–barrier transfer of PA strongly induces its endogenous biosynthesis from glucose via de novo lipogenesis (DNL) to secure a tight homeostatic control of PA tissue concentration required to exert its multiple physiological activities. However, pathophysiological conditions (insulin resistance) are characterized by a sustained DNL in the liver and aimed at preventing the excess accumulation of glucose, which result in increased tissue content of PA and disrupted homeostatic control of its tissue concentration. This leads to an overaccumulation of tissue PA, which results in dyslipidemia, increased ectopic fat accumulation, and inflammatory tone via toll-like receptor 4. Any change in dietary saturated FAs (SFAs) usually reflects a complementary change in polyunsaturated FA (PUFA) intake. Since PUFA particularly n-3 highly PUFA, suppress lipogenic gene expression, their reduction in intake rather than excess of dietary SFA may promote endogenous PA production via DNL. Thereby, the increase in tissue PA and its deleterious consequences from dysregulated DNL can be mistakenly attributed to dietary intake of PA.
Introduction
Palmitic acid (PA) is one of the most abundant saturated fatty acids (SFAs) in nature, which is present in animal and human tissues, plants, algae, fungus, yeast, and bacteria. Its distribution varies both within species and among species, and its content can be influenced by several environmental factors as the variation of soil pH, nutrient–ion interaction, age, water, and climate (1, 2).
The average dietary intake of PA is around 20–30 g/d representing about 8–10%en (3–5) and can be found in different vegetable and animal fat sources (Table 1) (6), with levels of 20–30% in animal lipids and 10–45% in vegetable oils. Methods that are used to prepare the food also impact on PA amount; for example, in processed and preserved meats, the content is higher than fresh meat with values up to 7.6/100 g of edible portion in salami and in lard 21/100 g of edible portion. It should be pointed out that due to high within-food variability of PA content, it is very difficult to assess its precise dietary intake. In addition, PA absorption and metabolic fate are strongly influenced by several factors, such as food matrix and pathological or physiological conditions.
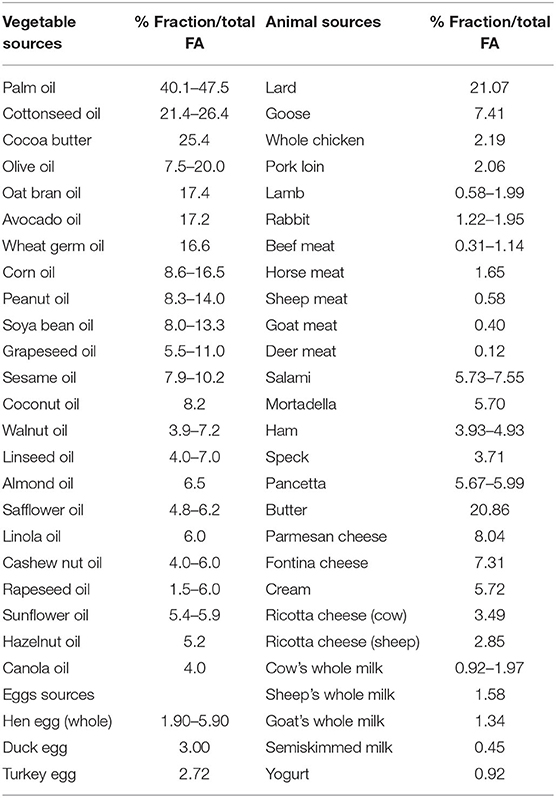
Table 1. Palmitic acid content of oils and fats from vegetable and animal sources (expressed as percentage mass fraction of total FAs) (6).
Importance of the Matrix and PA Distribution on Metabolism
In evaluating the effects of food on health, the overall macronutrient composition and structure need to be considered, i.e. the “food matrix” (7), meaning that food chemical compounds behave differently in isolated form in comparison to part of food structures (8), as well as the resistance of a food to the mastication and the viscosity of aliments, which may affect the bioavailability and digestibility of dietary lipids (9). Dietary fats comprise cholesterol and fatty acids (FAs), which can be free or components of complex lipids, as triacylglycerols (TAGs), and phospholipids (PLs), organized in structures able to modulate FA final metabolic fate. FA esterification on different positions of the TAG glycerol backbone (central sn-2 position, external sn-1 and sn-3 positions) or on a PL may also impact on their digestibility and metabolism (10–16). PA in foods is mainly present esterified in PL and TAG.
Dietary PL represents 1–10% of total daily fat intake (17). PL is mainly catabolized by pancreatic phospholipase A2 (PLA2) that produces free FA (FFA) and lysophosphatidylcholine (lysoPL), which once absorbed by the intestinal epithelium are reacylated or hydrolyzed to form PL or glycerol-3-phosphorylcholine, respectively. FFAs are instead used for TAG synthesis that are subsequently incorporated into chylomicrons (17).
Over 90% of dietary FAs are esterified to TAG preferentially hydrolyzed by digestive lipases (18) on sn-1,3 positions followed by pancreatic lipase to give 2-monoacylglycerol (2-MAG) and FFA (15), which cross the apical membranes of the enterocytes and are reassembled into TAG for secretion to plasma in chylomicrons. SFA released from positions sn-1 and sn-3 may form insoluble soaps with ions as calcium that are not absorbed, a singularity lost if SFA is in the TAG sn-2 position (19), as confirmed by animal and human infant studies which demonstrate that sn-2 esterified FA is efficiently absorbed as 2-MAG (20, 21). The peculiar sn-2 position of PA in human milk results from the activity of the glycerol-3-phosphate (G-3-P) acyltransferase, present in the mammary gland, which acylates an unsaturated FA at the sn-1 position of G-3-P and subsequently a PA at the sn-2 position (22, 23). Human milk, which contains 20–25% of PA with respect to the total FA whose 70% is in sn-2 of TAG, limits PA malabsorption providing the infant with high PA (19, 23–25). Conversely, 45 and 58% of cow and rodent milk fat (25 and 15% of PA on the total FA, respectively) are esterified at the TAG sn-2 position (23, 26). FA composition of the early diet influences intestinal membrane FA, which affects nutrient transport, permeability, and inflammatory pathways that persist into later life (27, 28). Notably, PA also plays an important role in the developing fetus with the term infant reaching 13–15% of body fat of which 45–50% is PA, mostly derived from endogenous synthesis in the fetus (29).
PA peculiar tissue distribution results in its better incorporation in several tissues, for example, adipose tissues, with a lower deposition of fat in the visceral depots and higher in the subcutaneous fat (30). Interestingly, several studies demonstrated the protective effect of breastfeeding against obesity in childhood (31, 32) and adulthood (33–36). Also, donkey milk contains high concentrations of PA in sn-2 of TAG and is recognized as the best potential substitute for human milk due to its remarkable nutritional value, good palatability, and reduced allergenicity (37, 38). A recent study in rats showed that oral supplementation with human or donkey milk ameliorated metabolism and reduced inflammation potentially mediated by an improved redox status, mitochondrial uncoupling, and dynamics (39). In addition, it has been demonstrated that PA in sn-2 by modifying endocannabinoids and congeners biosynthesis in different tissues may potentially concur in the physiological regulation of energy metabolism, brain function, and body fat distribution (40). In contrast to milk, in animal tissues that include human adipose tissue and also beef tallow and in soybean oil and cocoa butter, PA is mainly at sn-1,3 position, whereas the sn-2 is occupied by an unsaturated FA (41–45). Lard, having high amounts of PA at TAG sn-2, represents an exception (23), and in animal studies, PA from lard was better absorbed with respect to PA from cocoa butter and palm oil (46, 47). This PA peculiar position led food industries to often use interesterification to produce functional infant formula containing TAG with a high amount of sn-2 PA (48, 49). Amounts of PA in the sn-2 position in breast-fed infants (81%) or in infants fed formula prepared with synthesized TAG (39%), plasma chylomicron TAG containing PA in sn-2 position were higher with respect to those fed with standard infant formula with 6% of PA in sn-2 position (50), and it was shown in infants that PA loss in stools was 8-folds less using infant formula with lard TAG with respect to randomized lard (51). Also, an increase in the proportion of sn-2 PA by interesterification of TAG in coconut oil and palm olein improved PA absorption and metabolism in rats (52, 53). Therefore, the matrix/esterified position plays a crucial role in determining the metabolic fate of dietary PA.
Assessment of Dietary PA Intake in Humans
Most of the studies aimed at evaluating dietary FA intake rely on food frequency questionnaires (FFQs), and food diaries where even repeated measurements do not necessarily provide valid measures of individual intake. Extreme intakes may reflect under- and overreporting rather than true low or high intakes, and subjects most prone to reporting bias may be repeatedly misclassified in quantiles of the distribution (54). In addition, assessing the precise nutrient intake is quite difficult because of the errors made in recalling or the identification of the amounts of foods eaten, especially in processed foods (55).
Measurement of circulating PA is not also a reliable marker of its dietary intake; in fact, the dietary consumption of PA has low impact on plasma levels compared with its endogenous biosynthesis; data from a controlled human feeding trial showed that variations in SFA intake from 11 to 30%en did not change circulating SFA, including PA (56). Accordingly, cohort studies did not show a solid correlation between the PA dietary intake (evaluated by FFQ) and its plasma levels (r = −0.02 to 0.09) (57–60).
Factors other than dietary intake have been suggested to influence FA composition in tissues, first FA metabolism efficiency, genetic variations, and even intrauterine and perinatal program. In fact, considering the relationship between the tissue FA composition and dietary fat, among plasma lipid fractions, only TAGs appear to reflect dietary polyunsaturated FA (PUFA) and SFA, but not monounsaturated FA (MUFA) (61) within the first hours after intake (62). Whereas, FA in serum cholesteryl esters (CEs) and in PL is related to average intake of dietary FA composition during the previous 3–6 weeks, FA of erythrocyte membrane PL and adipose tissue TAG reflect the dietary fat intake of previous months or years, respectively (62).
Noteworthy, it has been demonstrated, by isotope labeling studies in men, that low-fat high-carbohydrate diet stimulates de novo lipogenesis (DNL) with the accumulation of VLDL-TAG PA that led to linoleic acid (LA) reduction probably due to dilution effect, whereas with high-fat (40% fat, 45% carbohydrate) DNL is neglectable (63). This suggests that circulating PA levels are largely driven by endogenous synthesis through DNL rather than direct dietary intake. Therefore, the relative strict regulation of PA tissue concentration, with variable amount of the endogenously produced, leads to a high unreliability of the use of PA plasma levels as a tool to determine its dietary intake.
The potential increase of tissue PA by dietary intake is prevented by the contribution of its conversion to palmitoleic (POA), by the insertion of one double-bond through stearoyl-CoA desaturase-1 (SCD1) (62), which reduces PA availability in tissues, but also via elongation to stearic acid (SA) and further desaturation via SCD1 to form oleic acid (OA). A possible protective capacity of OA to drive PA to be deposited in the neutral form of TAG (64, 65) and POA to improve insulin sensibility has been described (66).
Fate or Metabolism of PA From DNL
When the energetic sources are in excess, the non-fat surplus, mainly carbohydrates, is converted to FA by DNL, a pathway that begins with the conversion of acetyl-CoA into malonyl-CoA by acetyl-CoA carboxylase (ACC). During fed and insulin-stimulated conditions, ACC increases malonyl-CoA levels whereas AMP-activated protein kinase (AMPK) stops the synthesis, probably by inhibiting sterol regulatory element-binding protein (SREBP) (67).
Further evidence indicates that adipose tissue DNL supports metabolic homoeostasis of distant organs, as in liver and muscle, by producing cytokine-like lipids, lipokines, with antidiabetogenic and antiinflammatory activities, such as POA and branched FA esters of hydroxy FA (FAHFA) (66, 68).
In normal conditions, adipose tissue is the major site for DNL, which significantly contributes to body lipid reserves, energy storage, and to the maintenance of serum TAG homeostasis that derived instead from dietary sources (69–75). Furthermore, adipose tissue DNL is considered as an energy-inefficient source of lipids because it yields fewer lipids per calorie consumed, thus being a promising strategy for the treatment of lipotoxicity-related diseases. In fact, adipose tissue DNL is positively correlated with postprandial energy expenditure (76) subsequently to carbohydrate overfeeding, but not fat overfeeding which failed to significantly increase any component of energy expenditure (77, 78).
On the other hand, under specific conditions in the liver, such as insulin resistance, the impaired glycogen biosynthesis and consequent accumulation of glucose induce DNL that may contribute up to 26% to ectopically intrahepatocellular lipids in the pathogenesis of nonalcoholic fatty liver disease (NAFLD). In fact, hepatic DNL is positively correlated with insulin resistance and fatty liver, whereas the correlation with adipose tissue DNL is the opposite (73, 79–81).
In addition, a high-carbohydrate diet, particularly rich in simple sugars as fructose (82–84), activates a lipogenic response and increases the synthesis and secretion of VLDL in liver (85) contributing to hypertriglyceridemia (74). DNL contributes to 10–35% of the total VLDL-TAG pool, probably increasing the size (~130 nm), but not the number of VLDL secreted (86), and is in general higher in insulin-resistant states, and in overweight subjects compared to lean individuals (87–91).
Regulation of DNL occurs through the regulation of transcriptional factors as SREBP-1c and carbohydrate-responsive element-binding protein (ChREBP), activated by increased insulin signaling and increased glucose concentrations, respectively, and both induced by feeding (85, 92–95).
In liver, PUFA downregulates DNL via decreased expression of SREBP-1c (96), and leptin reduced adipogenesis through the inhibition of SREBP-1c expression (97). In addition, insulin and SREBP-1c stimulate peroxisome proliferator-activated receptor-γ (PPAR γ) expression (98, 99), which regulates glucose and lipid metabolism thus having adipogenic and lipogenic effects (100) and promotes FA storage in mature adipocytes by the stimulation of lipoprotein lipase (LPL), CD36, and glucose transporter GLUT-4 (101–103).
Therefore, DNL has a dual function, to supply PA in deficiency conditions, such as in the fetus (29) and developing brain (104) to overcome the difficulties of PA to pass, respectively, the placenta and the brain–blood barrier and to prevent the excess accumulation of glucose in the liver. In the latter case, significant increase of tissue PA is detected eluding the homeostatic control of tissue PA concentration and increased endogenous PA production may enhance inflammatory susceptibility through toll-like receptor (TLR4) activation (105) and insulin resistance by ceramide accumulation (106).
Considerations Over High SFA Diets vs. PUFA-Deficient Diets on Metabolism
Dietary guidelines recommend limiting SFA intake to <10% of calories per day. Correlation between dietary SFA intake and cardiovascular disease (CVD) is quite controversial (107). The Cochrane analysis showed an association between reducing SFA intake and a reduction in cardiovascular events and replacing the energy from SFA with PUFA appear to be useful strategies, whereas effects of replacement with MUFA are unclear (108). SFA increases LDL plasma particle concentration but also their size, which is less associated with CVD (109) because more rapidly cleared than small-dense LDL particles from the circulation due to reduced receptor-mediated uptake (110). SFA increases blood total, LDL, and HDL cholesterol concentrations and decreases fasting TAG concentrations not changing the total–HDL cholesterol (TC/HDL) ratio. The capacity of increasing circulating HDL levels decreases with increasing chain length of SFA and for some studies, but not all, myristic acid and PA, and also carbohydrate intake, negatively affect TC/HDL ratio (111).
In vitro cell culture studies showed that PA in the free form in the medium elicits, insulin resistance (112), inflammation via TLR4 (105) and prometastatic activities (113), which implies that increased dietary PA may result in higher PA availability to cell tissues in the free form, while as already mentioned, higher intake of SFA results in a decrease of circulating PA in the free form and increase of its monounsaturated metabolites POA and OA. Therefore, in vitro models, while may elucidate a limited molecular mechanism, are by far not mimicking pathophysiological conditions. The extremely high concentrations typically used in vitro are not achievable in vivo, thus the results obtained do not prove any relevant pathophysiological information.
Any change in dietary SFA reflects a complementary change in MUFA and/or PUFA intake. As mentioned above, PUFA (70), particularly n-3 highly PUFA such as EPA and DHA, suppresses lipogenic gene expression by reducing the nuclear abundance and DNA-binding affinity of transcription factors responsible for imparting insulin and carbohydrate control to lipogenic and glycolytic genes (114); thereby, most of the detrimental effects should be ascribed to the lower PUFA intake rather than high dietary SFA.
From, a meta-analysis of randomized controlled trials emerged that replacing 5% energy from carbohydrate with SFA had no significant effect on fasting glucose but lowered insulin, and replacing SFA with PUFA lowered glucose, HbA1c and HOMA. This suggests that consuming more unsaturated FA in place of either carbohydrates or SFA will help to improve blood glucose control while exchanging dietary carbohydrate with SFA does not appreciably influence markers of blood glucose control, and therefore an approach based only on reducing carbohydrates or SFA intake, without considering the source of energy replacement would not be optimal (115).
Data are often contradictory and may be difficult to interpret into dietary advise: some studies suggested that n-6 PUFA would increase CVD risk (116, 117), and therefore the Institute of Medicine recommends a relatively modest range of 5%–10% energy consumption from PUFA, limiting its plausibility as a meaningful replacement for SFA (118). Increasing dietary PUFA may not be desirable as dietary levels of LA are already higher than recommended (119), particularly the n-6/n-3 PUFA ratio (119). The physiological role played by the SREBP-1c, which is inhibited by n-3 FA (114) and in general by PUFA (18), for glycogen biosynthesis and overall glucose homeostasis (120), stresses the point that balance between different dietary FA is strongly recommended and any unbalance may lead or increase the chance to set into motion a disrupted metabolism. In fact, while replacing SFA with LA has an established cholesterol lowering effect, it has not been shown that this lowering reduces mortality (107).
In addition, recently, it has been shown that lower dietary PUFA/MUFA and n-3/n-6, and not SFA, were associated with disturbances in metabolic syndrome-related indices in postmenopausal women, and that polymorphisms of FA desaturase FADS1 (rs174546) and FADS2 (rs3834458) were associated with unfavorable FA profile in red blood cells (121). It has also been demonstrated that the polymorphism rs1761667 of multifunctional CD36 scavenger receptor that facilitates FA uptake and oxidation, leads to a distinct metabolic pattern in normal weight and in obese subjects (122). Thus, changes of tissue FA profile and associated metabolic changes may also be determined by different genetic polymorphisms, which should be considered in developing personalized therapeutic strategies for ameliorating dyslipidemia and other metabolic disorders.
From several studies exploring the molecular mechanism of dietary FA interactions emerged that the reduction of PUFA intake, especially n-3 PUFA, rather than the excess of dietary SFA, may favor insulin resistance (123), promoting endogenous PA production via DNL. Thus, the increase in tissue PA from dysregulated DNL and its deleterious consequences can be mistakenly attributed to dietary intake of PA (Figure 1).
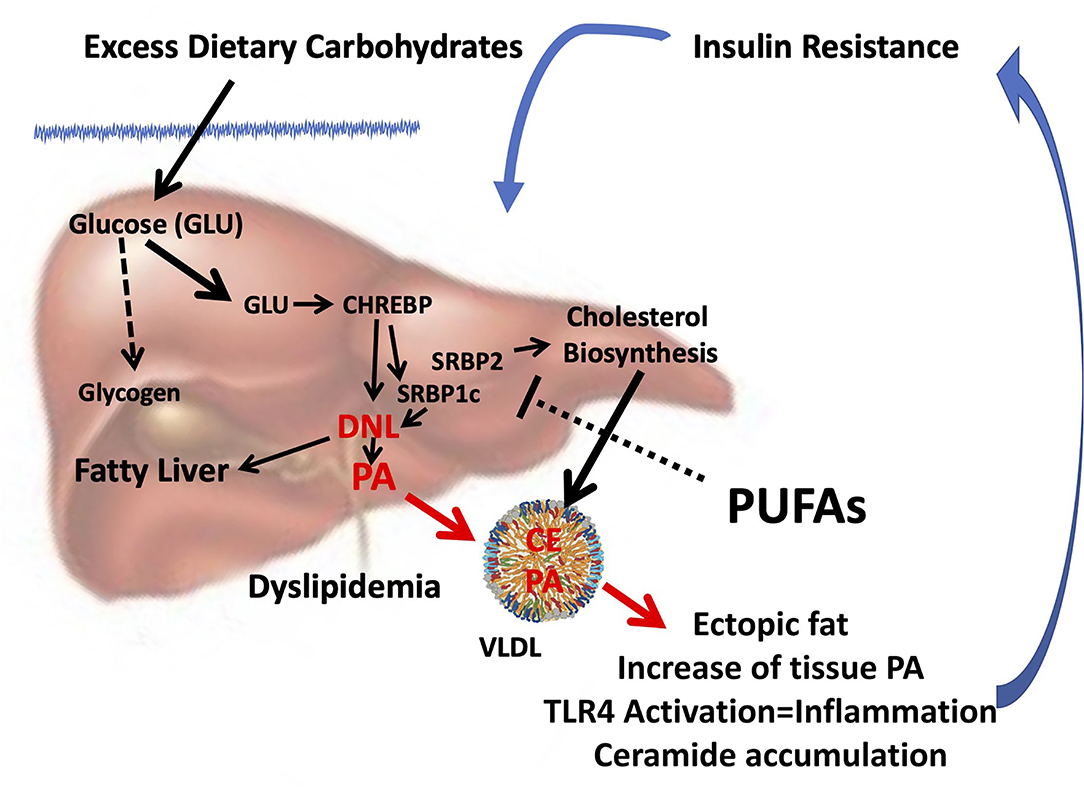
Figure 1. Combined consequences of liver insulin resistance and reduced PUFA intake. Insulin resistance in the liver is characterized by hyperinsulinemia and a reduced ability to store glycogen. In the presence of excess glucose, CHREBP is activated which, in turn, together with hyperinsulinemia, induces SREBP1c, and synergistically induces DNL (124) and thereby the biosynthesis of endogenous PA. Reduced PUFA intake can further promote PA and cholesterol biosynthesis since PUFAs inhibit both SREBP1c (123) and SREBP2 (125). Enhanced DNL can cause fatty liver and formation and release of VLDL enriched with PA and cholesterol esters. As a result, the accumulation of ectopic fat occurs in different tissues, and the increase in tissue PA can sustain insulin resistance by inducing inflammation through the activation of TLR4 (105) and accumulation of ceramides (106), setting in motion a vicious circle. Because reduced PUFA intake is often associated with an unbalanced increase in dietary SFA/PUFA, the rise in tissue PA can be mistakenly attributed to its dietary intake. CHREBP, carbohydrate-responsive element-binding protein; SREBP, sterol regulatory element-binding protein; DNL, de novo lipogenesis; PA, palmitic acid; PUFA, polyunsaturated fatty acid; TLR4, toll-like receptor 4; SFA, saturated fatty acid.
Interestingly, it has been proposed that the claimed adverse effect on cholesterol exerted by high dietary SFA/PUFA ratio may represent a physiological mechanism aimed at fulfilling the needs of tissues for cholesterol (126) and the yield of larger LDL makes this increase not to be related to CVD (7).
Many of the purported harmful effects of dietary PA are based on experimental animal studies, mainly on mice on a high-fat diet, which consists of 45–60%en whereas the optimal fat content in the rodents diets ranges from 9 to 16%en (127). Therefore, high-fat diets contain from 3- to 6-folds of the fat content required, with usually an extremely high percentage of PA and low in PUFA and n-3/n-6 PUFA ratio, which makes difficult to pinpoint the effects of a high-fat content, high concentration of PA, or high n-6/n-3 PUFA ratio. These diets were created to induce obesity as quickly as possible (128) and not to assess the nutritional impact of dietary FA. Therefore, whereas they might be suitable as a model of obesity, they cannot be taken into consideration for translational nutritional studies on the effects of dietary FA (128).
Conclusions
Several pieces of evidence suggest that the nutritional impact of dietary FA is strictly related to the balance among them and with other macronutrients. Most of the studies claiming negative effect of PA rely on in vitro cell culture studies, incubating cells with extremely high concentrations and as a single FA without considering that dietary PA does not modify its tissue concentration, or with animal models of obesity with an extremely high-fat content not achievable by humans (128) and not specifically designed for studying dietary FA and thereby without any translatability to human conditions. More preclinical and clinical studies are needed to better discern the metabolic fate and interaction between dietary and de novo PA particularly in relation to PUFA intake, macronutrient balance, and pathophysiological states.
To blame a single nutrient, such as PA, widely present in our diet from several sources and with several well recognized fundamental physiological properties (129), as detrimental, suggesting that is sufficient to reduce its dietary intake for improving our health and prevent pathological states from CVD to cancer, is rather simplistic but it has a great praise probably because of the human nature to choose less time and energy consuming solutions for complex issues (130).
Thus, guidelines or recommendations to the general population to avoid or increase the intake of single nutrients, without considering the complexity of nutrient-nutrient interactions and the individual-specific nutritional response in relation to age, genetic, environmental, physiological and pathophysiological conditions, do not follow the amount of growing scientific data that suggest we should not be focusing on single nutrients but on increasing diet variability within a personalized nutritional approach.
Author Contributions
EM, CM, GC, and SB: conception and design of the review, organized the literature search, wrote the first draft of the manuscript, and contributed to wrote sections of the manuscript. All authors contributed to manuscript revision, read, and approved the submitted version.
Funding
This research was funded by Grants to GC and SB from the University of Cagliari: Fondo Integrativo per la Ricerca (FIR 2020).
Conflict of Interest
The authors declare that the research was conducted in the absence of any commercial or financial relationships that could be construed as a potential conflict of interest.
Publisher's Note
All claims expressed in this article are solely those of the authors and do not necessarily represent those of their affiliated organizations, or those of the publisher, the editors and the reviewers. Any product that may be evaluated in this article, or claim that may be made by its manufacturer, is not guaranteed or endorsed by the publisher.
References
1. Clegg AJ. Composition and related nutritional and organoleptic aspects of palm oil. J Am Oil Chem Soc. (1973) 50:321–4. doi: 10.1007/BF02641365
2. Griffiths RG, Dancer J, O'Neill E, Harwood JL. Lipid composition of Botrytis cinerea and inhibition of its radiolabelling by the fungicide iprodione. New Phytol. (2003) 160:199–207. doi: 10.1046/j.1469-8137.2003.00848.x
3. Sette S, Le Donne C, Piccinelli R, Arcella D, Turrini A, Leclercq C, et al. The third Italian National Food Consumption Survey, INRAN-SCAI 2005-06–part 1: nutrient intakes in Italy. Nutr Metab Cardiovasc Dis. (2011) 21:922–32. doi: 10.1016/j.numecd.2010.03.001
5. Innis SM, Nelson CM. Dietary triacyglycerols rich in sn-2 palmitate alter post-prandial lipoprotein and unesterified fatty acids in term infants. Prostagl Leukot Essent Fatty Acids. (2013) 89:145–51. doi: 10.1016/j.plefa.2013.03.003
6. Peiretti PG. Palmitic Acid: Effect of Diet Supplementation and Occurrence in Animal Origin Food. Porto LF, editor. New York, NY: Nova Science Publishers Inc. (2014).
7. Agostoni C, Boccia S, Banni S, Mannucci PM, Astrup A. Sustainable and personalized nutrition: from earth health to public health. Eur J Intern Med. (2021) 86:12–6. doi: 10.1016/j.ejim.2021.02.012
8. Aguilera JM. The food matrix: implications in processing, nutrition and health. Crit Rev Food Sci Nutr. (2019) 59:3612–29. doi: 10.1080/10408398.2018.1502743
9. Pasquier B, Armand M, Castelain C, Guillon F, Borel P, Lafont H, et al. Emulsification and lipolysis of triacylglycerols are altered by viscous soluble dietary fibres in acidic gastric medium in vitro. Biochem J. (1996) 314:269–75. doi: 10.1042/bj3140269
10. Astrup A, Bertram HC, Bonjour JP, de Groot LC, de Oliveira Otto MC, Feeney EL, et al. WHO draft guidelines on dietary saturated and trans fatty acids: time for a new approach? BMJ. (2019) 366:l4137. doi: 10.1136/bmj.l4137
11. Berry SE. Triacylglycerol structure and interesterification of palmitic and stearic acid-rich fats: an overview and implications for cardiovascular disease. Nutr Res Rev. (2009) 22:3–17. doi: 10.1017/S0954422409369267
12. Berry SE, Sanders TA. Influence of triacylglycerol structure of stearic acid-rich fats on postprandial lipaemia. Proc Nutr Soc. (2005) 64:205–12. doi: 10.1079/PNS2005422
13. Bracco U. Effect of triglyceride structure on fat absorption. Am J Clin Nutr. (1994) 60(Suppl. 6):1002S−9S. doi: 10.1093/ajcn/60.6.1002S
14. Hunter JE. Studies on effects of dietary fatty acids as related to their position on triglycerides. Lipids. (2001) 36:655–68. doi: 10.1007/s11745-001-0770-0
15. Michalski MC, Genot C, Gayet C, Lopez C, Fine F, Joffre F, et al. Multiscale structures of lipids in foods as parameters affecting fatty acid bioavailability and lipid metabolism. Prog Lipid Res. (2013) 52:354–73. doi: 10.1016/j.plipres.2013.04.004
16. Murru E, Banni S, Carta G. Nutritional properties of dietary omega-3-enriched phospholipids. Biomed Res Int. (2013) 2013:965417. doi: 10.1155/2013/965417
17. Cohn JS, Wat E, Kamili A, Tandy S. Dietary phospholipids, hepatic lipid metabolism and cardiovascular disease. Curr Opin Lipidol. (2008) 19:257–62. doi: 10.1097/MOL.0b013e3282ffaf96
18. Livesey G. The absorption of stearic acid from triacylglycerols: an inquiry and analysis. Nutr Res Rev. (2000) 13:185–214. doi: 10.1079/095442200108729061
19. Gesteiro E, Guijarro L, Sanchez-Muniz FJ, Vidal-Carou MDC, Troncoso A, Venanci L, et al. Palm Oil on the Edge. Nutrients. (2019) 11:2008. doi: 10.3390/nu11092008
20. Innis SM, Dyer R, Nelson CM. Evidence that palmitic acid is absorbed as sn-2 monoacylglycerol from human milk by breast-fed infants. Lipids. (1994) 29:541–5. doi: 10.1007/BF02536625
21. Yang LY, Kuksis A. Apparent convergence (at 2-monoacylglycerol level) of phosphatidic acid and 2-monoacylglycerol pathways of synthesis of chylomicron triacylglycerols. J Lipid Res. (1991) 32:1173–86. doi: 10.1016/S0022-2275(20)41980-7
22. Gimeno RE, Cao J. Thematic review series: glycerolipids. Mammalian glycerol-3-phosphate acyltransferases: new genes for an old activity. J Lipid Res. (2008) 49:2079–88. doi: 10.1194/jlr.R800013-JLR200
23. Innis SM. Dietary triacylglycerol structure and its role in infant nutrition. Adv Nutr. (2011) 2:275–83. doi: 10.3945/an.111.000448
24. Martin JC, Bougnoux P, Antoine JM, Lanson M, Couet C. Triacylglycerol structure of human colostrum and mature milk. Lipids. (1993) 28:637–43. doi: 10.1007/BF02536059
25. Straarup EM, Lauritzen L, Faerk J, Hoy Deceased CE, Michaelsen KF. The stereospecific triacylglycerol structures and Fatty Acid profiles of human milk and infant formulas. J Pediatr Gastroenterol Nutr. (2006) 42:293–9. doi: 10.1097/01.mpg.0000214155.51036.4f
26. Breckenridge WC, Kuksis A. Molecular weight distributions of milk fat triglycerides from seven species. J Lipid Res. (1967) 8:473–8. doi: 10.1016/S0022-2275(20)38904-5
27. Drozdowski LA, Clandinin T, Thomson AB. Ontogeny, growth and development of the small intestine: understanding pediatric gastroenterology. World J Gastroenterol. (2010) 16:787–99. doi: 10.3748/wjg.v16.i7.787
28. Innis SM Dai C, Wu X, Buchan AM, Jacobson K. Perinatal lipid nutrition alters early intestinal development and programs the response to experimental colitis in young adult rats. Am J Physiol Gastrointest Liver Physiol. (2010) 299:G1376–85. doi: 10.1152/ajpgi.00258.2010
29. Innis SM. Palmitic acid in early human development. Crit Rev Food Sci Nutr. (2016) 56:1952–9. doi: 10.1080/10408398.2015.1018045
30. Petrus P, Edholm D, Rosqvist F, Dahlman I, Sundbom M, Arner P, et al. Depot-specific differences in fatty acid composition and distinct associations with lipogenic gene expression in abdominal adipose tissue of obese women. Int J Obes. (2017) 41:1295–8. doi: 10.1038/ijo.2017.106
31. Arenz S, Ruckerl R, Koletzko B, von Kries R. Breast-feeding and childhood obesity–a systematic review. Int J Obes Relat Metab Disord. (2004) 28:1247–56. doi: 10.1038/sj.ijo.0802758
32. Gillman MW, Rifas-Shiman SL, Camargo CA Jr, Berkey CS, Frazier AL, Rockett HR, et al. Risk of overweight among adolescents who were breastfed as infants. JAMA. (2001) 285:2461–7. doi: 10.1001/jama.285.19.2461
33. Harder T, Bergmann R, Kallischnigg G, Plagemann A. Duration of breastfeeding and risk of overweight: a meta-analysis. Am J Epidemiol. (2005) 162:397–403. doi: 10.1093/aje/kwi222
34. Owen CG, Martin RM, Whincup PH, Davey-Smith G, Gillman MW, Cook DG. The effect of breastfeeding on mean body mass index throughout life: a quantitative review of published and unpublished observational evidence. Am J Clin Nutr. (2005) 82:1298–307. doi: 10.1093/ajcn/82.6.1298
35. Owen CG, Martin RM, Whincup PH, Smith GD, Cook DG. Effect of infant feeding on the risk of obesity across the life course: a quantitative review of published evidence. Pediatrics. (2005) 115:1367–77. doi: 10.1542/peds.2004-1176
36. Quigley MA. Re: Duration of breastfeeding and risk of overweight: a meta-analysis. Am J Epidemiol. (2006) 163:870–2; author reply 2–3. doi: 10.1093/aje/kwj134
37. Fiocchi A, Brozek J, Schunemann H, Bahna SL, von Berg A, Beyer K, et al. World Allergy Organization (WAO) Diagnosis and Rationale for Action against Cow's Milk Allergy (DRACMA) guidelines. World Allergy Organ J. (2010) 3:57–161. doi: 10.1097/WOX.0b013e3181defeb9
38. Tafaro A, Magrone T, Jirillo F, Martemucci G, D'Alessandro AG, Amati L, et al. Immunological properties of donkey's milk: its potential use in the prevention of atherosclerosis. Curr Pharm Des. (2007) 13:3711–7. doi: 10.2174/138161207783018590
39. Trinchese G, Cavaliere G, De Filippo C, Aceto S, Prisco M, Chun JT, et al. Human milk and donkey milk, compared to cow milk, reduce inflammatory mediators and modulate glucose and lipid metabolism, acting on mitochondrial function and oleylethanolamide levels in rat skeletal muscle. Front Physiol. (2018) 9:32. doi: 10.3389/fphys.2018.00032
40. Carta G, Murru E, Lisai S, Sirigu A, Piras A, Collu M, et al. Dietary triacylglycerols with palmitic acid in the sn-2 position modulate levels of N-acylethanolamides in rat tissues. PLoS ONE. (2015) 10:e0120424. doi: 10.1371/journal.pone.0120424
41. Bottino NR, Vandenburg GA, Reiser R. Resistance of certain long-chain polyunsaturated fatty acids of marine oils to pancreatic lipase hydrolysis. Lipids. (1967) 2:489–93. doi: 10.1007/BF02533177
42. Christensen MS, Hoy CE, Redgrave TG. Lymphatic absorption of n - 3 polyunsaturated fatty acids from marine oils with different intramolecular fatty acid distributions. Biochim Biophys Acta. (1994) 1215:198–204. doi: 10.1016/0005-2760(94)90111-2
43. Lawson LD, Hughes BG. Human absorption of fish oil fatty acids as triacylglycerols, free acids, or ethyl esters. Biochem Biophys Res Commun. (1988) 152:328–35. doi: 10.1016/S0006-291X(88)80718-6
44. Porsgaard T, Xu X, Gottsche J, Mu H. Differences in the intramolecular structure of structured oils do not affect pancreatic lipase activity in vitro or the absorption by rats of (n-3) fatty acids. J Nutr. (2005) 135:1705–11. doi: 10.1093/jn/135.7.1705
45. Yang LY, Kuksis A, Myher JJ. Lipolysis of menhaden oil triacylglycerols and the corresponding fatty acid alkyl esters by pancreatic lipase in vitro: a reexamination. J Lipid Res. (1990) 31:137–47. doi: 10.1016/S0022-2275(20)42768-3
46. Aoe S, Yamamura J, Matsuyama H, Hase M, Shiota M, Miura S. The positional distribution of dioleoyl-palmitoyl glycerol influences lymph chylomicron transport, composition and size in rats. J Nutr. (1997) 127:1269–73. doi: 10.1093/jn/127.7.1269
47. Porsgaard T, Hoy CE. Lymphatic transport in rats of several dietary fats differing in fatty acid profile and triacylglycerol structure. J Nutr. (2000) 130:1619–24. doi: 10.1093/jn/130.6.1619
48. Lucas A, Quinlan P, Abrams S, Ryan S, Meah S, Lucas PJ. Randomised controlled trial of a synthetic triglyceride milk formula for preterm infants. Arch Dis Child Fetal Neonatal Ed. (1997) 77:F178–84. doi: 10.1136/fn.77.3.F178
49. de Fouw NJ, Kivits GA, Quinlan PT, van Nielen WG. Absorption of isomeric, palmitic acid-containing triacylglycerols resembling human milk fat in the adult rat. Lipids. (1994) 29:765–70. doi: 10.1007/BF02536698
50. Nelson CM, Innis SM. Plasma lipoprotein fatty acids are altered by the positional distribution of fatty acids in infant formula triacylglycerols and human milk. Am J Clin Nutr. (1999) 70:62–9. doi: 10.1093/ajcn/70.1.62
51. Filer LJ Jr, Mattson FH, Fomon SJ Triglyceride configuration and fat absorption by the human infant. J Nutr. (1969) 99:293–8. doi: 10.1093/jn/99.3.293
52. Renaud SC, Ruf JC, Petithory D. The positional distribution of fatty acids in palm oil and lard influences their biologic effects in rats. J Nutr. (1995) 125:229–37.
53. Lien EL, Yuhas RJ, Boyle FG, Tomarelli RM. Corandomization of fats improves absorption in rats. J Nutr. (1993) 123:1859–67. doi: 10.1093/jn/123.11.1859
54. Black AE, Cole TJ. Biased over- or under-reporting is characteristic of individuals whether over time or by different assessment methods. J Am Diet Assoc. (2001) 101:70–80. doi: 10.1016/S0002-8223(01)00018-9
55. Ozanne SE, Martensz ND, Petry CJ, Loizou CL, Hales CN. Maternal low protein diet in rats programmes fatty acid desaturase activities in the offspring. Diabetologia. (1998) 41:1337–42. doi: 10.1007/s001250051074
56. Lee Y, Lai HTM, de Oliveira Otto MC, Lemaitre RN, McKnight B, King IB, et al. Serial biomarkers of de novo lipogenesis fatty acids and incident heart failure in older adults: the cardiovascular health study. J Am Heart Assoc. (2020) 9:e014119. doi: 10.1161/JAHA.119.014119
57. Patel PS, Sharp SJ, Jansen E, Luben RN, Khaw KT, Wareham NJ, et al. Fatty acids measured in plasma and erythrocyte-membrane phospholipids and derived by food-frequency questionnaire and the risk of new-onset type 2 diabetes: a pilot study in the European Prospective Investigation into Cancer and Nutrition (EPIC)-Norfolk cohort. Am J Clin Nutr. (2010) 92:1214–22. doi: 10.3945/ajcn.2010.29182
58. Kroger J, Zietemann V, Enzenbach C, Weikert C, Jansen EH, Doring F, et al. Erythrocyte membrane phospholipid fatty acids, desaturase activity, and dietary fatty acids in relation to risk of type 2 diabetes in the European Prospective Investigation into Cancer and Nutrition (EPIC)-Potsdam Study. Am J Clin Nutr. (2011) 93:127–42. doi: 10.3945/ajcn.110.005447
59. Hodge AM, Simpson JA, Gibson RA, Sinclair AJ, Makrides M, O'Dea K, et al. Plasma phospholipid fatty acid composition as a biomarker of habitual dietary fat intake in an ethnically diverse cohort. Nutr Metab Cardiovasc Dis. (2007) 17:415–26. doi: 10.1016/j.numecd.2006.04.005
60. Warensjo Lemming E, Nalsen C, Becker W, Ridefelt P, Mattisson I, Lindroos AK. Relative validation of the dietary intake of fatty acids among adults in the Swedish National Dietary Survey using plasma phospholipid fatty acid composition. J Nutr Sci. (2015) 4:e25. doi: 10.1017/jns.2015.1
61. Nikkari T, Luukkainen P, Pietinen P, Puska P. Fatty acid composition of serum lipid fractions in relation to gender and quality of dietary fat. Ann Med. (1995) 27:491–8. doi: 10.3109/07853899709002458
62. Vessby B, Gustafsson IB, Tengblad S, Boberg M, Andersson A. Desaturation and elongation of Fatty acids and insulin action. Ann N Y Acad Sci. (2002) 967:183–95. doi: 10.1111/j.1749-6632.2002.tb04275.x
63. Hudgins LC, Hellerstein M, Seidman C, Neese R, Diakun J, Hirsch J. Human fatty acid synthesis is stimulated by a eucaloric low fat, high carbohydrate diet. J Clin Invest. (1996) 97:2081–91. doi: 10.1172/JCI118645
64. Cnop M, Hannaert JC, Hoorens A, Eizirik DL, Pipeleers DG. Inverse relationship between cytotoxicity of free fatty acids in pancreatic islet cells and cellular triglyceride accumulation. Diabetes. (2001) 50:1771–7. doi: 10.2337/diabetes.50.8.1771
65. Listenberger LL, Han X, Lewis SE, Cases S, Farese RV Jr, Ory DS, et al. Triglyceride accumulation protects against fatty acid-induced lipotoxicity. Proc Natl Acad Sci USA. (2003) 100:3077–82. doi: 10.1073/pnas.0630588100
66. Cao H, Gerhold K, Mayers JR, Wiest MM, Watkins SM, Hotamisligil GS. Identification of a lipokine, a lipid hormone linking adipose tissue to systemic metabolism. Cell. (2008) 134:933–44. doi: 10.1016/j.cell.2008.07.048
67. Li Y, Xu S, Mihaylova MM, Zheng B, Hou X, Jiang B, et al. AMPK phosphorylates and inhibits SREBP activity to attenuate hepatic steatosis and atherosclerosis in diet-induced insulin-resistant mice. Cell Metab. (2011) 13:376–88. doi: 10.1016/j.cmet.2011.03.009
68. Yore MM, Syed I, Moraes-Vieira PM, Zhang T, Herman MA, Homan EA, et al. Discovery of a class of endogenous mammalian lipids with anti-diabetic and anti-inflammatory effects. Cell. (2014) 159:318–32. doi: 10.1016/j.cell.2014.09.035
69. Aarsland A, Chinkes D, Wolfe RR. Hepatic and whole-body fat synthesis in humans during carbohydrate overfeeding. Am J Clin Nutr. (1997) 65:1774–82. doi: 10.1093/ajcn/65.6.1774
70. Ameer F, Scandiuzzi L, Hasnain S, Kalbacher H, Zaidi N. De novo lipogenesis in health and disease. Metabolism. (2014) 63:895–902. doi: 10.1016/j.metabol.2014.04.003
71. Bjorntorp P, Sjostrom L. Carbohydrate storage in man: speculations and some quantitative considerations. Metabolism. (1978) 27(Suppl. 2):1853–65. doi: 10.1016/S0026-0495(78)80004-3
72. Letexier D, Pinteur C, Large V, Frering V, Beylot M. Comparison of the expression and activity of the lipogenic pathway in human and rat adipose tissue. J Lipid Res. (2003) 44:2127–34. doi: 10.1194/jlr.M300235-JLR200
73. Minehira K, Vega N, Vidal H, Acheson K, Tappy L. Effect of carbohydrate overfeeding on whole body macronutrient metabolism and expression of lipogenic enzymes in adipose tissue of lean and overweight humans. Int J Obes Relat Metab Disord. (2004) 28:1291–8. doi: 10.1038/sj.ijo.0802760
74. Schwarz JM, Linfoot P, Dare D, Aghajanian K. Hepatic de novo lipogenesis in normoinsulinemic and hyperinsulinemic subjects consuming high-fat, low-carbohydrate and low-fat, high-carbohydrate isoenergetic diets. Am J Clin Nutr. (2003) 77:43–50. doi: 10.1093/ajcn/77.1.43
75. Strawford A, Antelo F, Christiansen M, Hellerstein MK. Adipose tissue triglyceride turnover, de novo lipogenesis, and cell proliferation in humans measured with 2H2O. Am J Physiol Endocrinol Metab. (2004) 286:E577–88. doi: 10.1152/ajpendo.00093.2003
76. Marques-Lopes I, Ansorena D, Astiasaran I, Forga L, Martinez JA. Postprandial de novo lipogenesis and metabolic changes induced by a high-carbohydrate, low-fat meal in lean and overweight men. Am J Clin Nutr. (2001) 73:253–61. doi: 10.1093/ajcn/73.2.253
77. Dirlewanger M, di Vetta V, Guenat E, Battilana P, Seematter G, Schneiter P, et al. Effects of short-term carbohydrate or fat overfeeding on energy expenditure and plasma leptin concentrations in healthy female subjects. Int J Obes Relat Metab Disord. (2000) 24:1413–8. doi: 10.1038/sj.ijo.0801395
78. Minehira K, Bettschart V, Vidal H, Vega N, Di Vetta V, Rey V, et al. Effect of carbohydrate overfeeding on whole body and adipose tissue metabolism in humans. Obes Res. (2003) 11:1096–103. doi: 10.1038/oby.2003.150
79. Diraison F, Dusserre E, Vidal H, Sothier M, Beylot M. Increased hepatic lipogenesis but decreased expression of lipogenic gene in adipose tissue in human obesity. Am J Physiol Endocrinol Metab. (2002) 282:E46–51. doi: 10.1152/ajpendo.2002.282.1.E46
80. Eissing L, Scherer T, Todter K, Knippschild U, Greve JW, Buurman WA, et al. De novo lipogenesis in human fat and liver is linked to ChREBP-beta and metabolic health. Nat Commun. (2013) 4:1528. doi: 10.1038/ncomms2537
81. Salans LB, Knittle JL, Hirsch J. The role of adipose cell size and adipose tissue insulin sensitivity in the carbohydrate intolerance of human obesity. J Clin Invest. (1968) 47:153–65. doi: 10.1172/JCI105705
82. Hudgins LC, Seidman CE, Diakun J, Hirsch J. Human fatty acid synthesis is reduced after the substitution of dietary starch for sugar. Am J Clin Nutr. (1998) 67:631–9. doi: 10.1093/ajcn/67.4.631
83. Neese RA, Benowitz NL, Hoh R, Faix D, LaBua A, Pun K, et al. Metabolic interactions between surplus dietary energy intake and cigarette smoking or its cessation. Am J Physiol. (1994) 267:E1023–34. doi: 10.1152/ajpendo.1994.267.6.E1023
84. Parks EJ, Krauss RM, Christiansen MP, Neese RA, Hellerstein MK. Effects of a low-fat, high-carbohydrate diet on VLDL-triglyceride assembly, production, and clearance. J Clin Invest. (1999) 104:1087–96. doi: 10.1172/JCI6572
85. Strable MS, Ntambi JM. Genetic control of de novo lipogenesis: role in diet-induced obesity. Crit Rev Biochem Mol Biol. (2010) 45:199–214. doi: 10.3109/10409231003667500
86. Timlin MT, Parks EJ. Temporal pattern of de novo lipogenesis in the postprandial state in healthy men. Am J Clin Nutr. (2005) 81:35–42. doi: 10.1093/ajcn/81.1.35
87. Cai D, Yuan M, Jia Y, Liu H, Hu Y, Zhao R. Maternal gestational betaine supplementation-mediated suppression of hepatic cyclin D2 and presenilin1 gene in newborn piglets is associated with epigenetic regulation of the STAT3-dependent pathway. J Nutr Biochem. (2015) 26:1622–31. doi: 10.1016/j.jnutbio.2015.08.007
88. Choi SH, Ginsberg HN. Increased very low density lipoprotein (VLDL) secretion, hepatic steatosis, and insulin resistance. Trends Endocrinol Metab. (2011) 22:353–63. doi: 10.1016/j.tem.2011.04.007
89. Donnelly KL, Smith CI, Schwarzenberg SJ, Jessurun J, Boldt MD, Parks EJ. Sources of fatty acids stored in liver and secreted via lipoproteins in patients with nonalcoholic fatty liver disease. J Clin Invest. (2005) 115:1343–51. doi: 10.1172/JCI23621
90. Grefhorst A, Elzinga BM, Voshol PJ, Plosch T, Kok T, Bloks VW, et al. Stimulation of lipogenesis by pharmacological activation of the liver X receptor leads to production of large, triglyceride-rich very low density lipoprotein particles. J Biol Chem. (2002) 277:34182–90. doi: 10.1074/jbc.M204887200
91. Ma W, Wu JH, Wang Q, Lemaitre RN, Mukamal KJ, Djousse L, et al. Prospective association of fatty acids in the de novo lipogenesis pathway with risk of type 2 diabetes: the Cardiovascular Health Study. Am J Clin Nutr. (2015) 101:153–63. doi: 10.3945/ajcn.114.092601
92. Herman MA, Peroni OD, Villoria J, Schon MR, Abumrad NA, Bluher M, et al. A novel ChREBP isoform in adipose tissue regulates systemic glucose metabolism. Nature. (2012) 484:333–8. doi: 10.1038/nature10986
93. Kersten S. Mechanisms of nutritional and hormonal regulation of lipogenesis. EMBO Rep. (2001) 2:282–6. doi: 10.1093/embo-reports/kve071
94. Oosterveer MH, Schoonjans K. Hepatic glucose sensing and integrative pathways in the liver. Cell Mol Life Sci. (2014) 71:1453–67. doi: 10.1007/s00018-013-1505-z
95. Shao W, Espenshade PJ. Expanding roles for SREBP in metabolism. Cell Metab. (2012) 16:414–9. doi: 10.1016/j.cmet.2012.09.002
96. Jump DB, Clarke SD, Thelen A, Liimatta M. Coordinate regulation of glycolytic and lipogenic gene expression by polyunsaturated fatty acids. J Lipid Res. (1994) 35:1076–84. doi: 10.1016/S0022-2275(20)40103-8
97. Swierczynski J. Leptin and age-related down-regulation of lipogenic enzymes genes expression in rat white adipose tissue. J Physiol Pharmacol. (2006) 57(Suppl. 6):85–102. Available online at: https://www.jpp.krakow.pl/journal/archive/11_06_s6/pdf/85_11_06_s6_article.pdf
98. Fajas L, Schoonjans K, Gelman L, Kim JB, Najib J, Martin G, et al. Regulation of peroxisome proliferator-activated receptor gamma expression by adipocyte differentiation and determination factor 1/sterol regulatory element binding protein 1: implications for adipocyte differentiation and metabolism. Mol Cell Biol. (1999) 19:5495–503. doi: 10.1128/MCB.19.8.5495
99. Vidal-Puig AJ, Considine RV, Jimenez-Linan M, Werman A, Pories WJ, Caro JF, et al. Peroxisome proliferator-activated receptor gene expression in human tissues. Effects of obesity, weight loss, and regulation by insulin and glucocorticoids. J Clin Invest. (1997) 99:2416–22. doi: 10.1172/JCI119424
100. Feige JN, Gelman L, Michalik L, Desvergne B, Wahli W. From molecular action to physiological outputs: peroxisome proliferator-activated receptors are nuclear receptors at the crossroads of key cellular functions. Prog Lipid Res. (2006) 45:120–59. doi: 10.1016/j.plipres.2005.12.002
101. Wu Z, Xie Y, Morrison RF, Bucher NL, Farmer SR. PPARgamma induces the insulin-dependent glucose transporter GLUT4 in the absence of C/EBPalpha during the conversion of 3T3 fibroblasts into adipocytes. J Clin Invest. (1998) 101:22–32. doi: 10.1172/JCI1244
102. Rosen ED, Spiegelman BM. PPARgamma: a nuclear regulator of metabolism, differentiation, and cell growth. J Biol Chem. (2001) 276:37731–4. doi: 10.1074/jbc.R100034200
103. Motojima K, Passilly P, Peters JM, Gonzalez FJ, Latruffe N. Expression of putative fatty acid transporter genes are regulated by peroxisome proliferator-activated receptor alpha and gamma activators in a tissue- and inducer-specific manner. J Biol Chem. (1998) 273:16710–4. doi: 10.1074/jbc.273.27.16710
104. Edmond J, Higa TA, Korsak RA, Bergner EA, Lee WN. Fatty acid transport and utilization for the developing brain. J Neurochem. (1998) 70:1227–34. doi: 10.1046/j.1471-4159.1998.70031227.x
105. Li B, Leung JCK, Chan LYY, Yiu WH, Tang SCW. A global perspective on the crosstalk between saturated fatty acids and Toll-like receptor 4 in the etiology of inflammation and insulin resistance. Prog Lipid Res. (2020) 77:101020. doi: 10.1016/j.plipres.2019.101020
106. Turpin-Nolan SM, Bruning JC. The role of ceramides in metabolic disorders: when size and localization matters. Nat Rev Endocrinol. (2020) 16:224–33. doi: 10.1038/s41574-020-0320-5
107. Calder PC. Lipids: a hole in the diet-heart hypothesis? Nat Rev Cardiol. (2016) 13:385–6. doi: 10.1038/nrcardio.2016.78
108. Hooper L, Martin N, Jimoh OF, Kirk C, Foster E, Abdelhamid AS. Reduction in saturated fat intake for cardiovascular disease. Cochrane Database Syst Rev. (2020) 5:CD011737. doi: 10.1002/14651858.CD011737.pub2
109. Krauss RM. All low-density lipoprotein particles are not created equal. Arterioscler Thromb Vasc Biol. (2014) 34:959–61. doi: 10.1161/ATVBAHA.114.303458
110. Bernstein AM, Sun Q, Hu FB, Stampfer MJ, Manson JE, Willett WC. Major dietary protein sources and risk of coronary heart disease in women. Circulation. (2010) 122:876–83. doi: 10.1161/CIRCULATIONAHA.109.915165
111. Mensink RP, Zock PL, Kester AD, Katan MB. Effects of dietary fatty acids and carbohydrates on the ratio of serum total to HDL cholesterol and on serum lipids and apolipoproteins: a meta-analysis of 60 controlled trials. Am J Clin Nutr. (2003) 77:1146–55. doi: 10.1093/ajcn/77.5.1146
112. Xu L, Wang W, Zhang X, Ke H, Qin Y, You L, et al. Palmitic acid causes insulin resistance in granulosa cells via activation of JNK. J Mol Endocrinol. (2019) 62:197–206. doi: 10.1530/JME-18-0214
113. Pascual G, Dominguez D, Elosua-Bayes M, Beckedorff F, Laudanna C, Bigas C, et al. Dietary palmitic acid promotes a prometastatic memory via Schwann cells. Nature. (2021) 599:485–90. doi: 10.1038/s41586-021-04075-0
114. Nakatani T, Kim HJ, Kaburagi Y, Yasuda K, Ezaki O. A low fish oil inhibits SREBP-1 proteolytic cascade, while a high-fish-oil feeding decreases SREBP-1 mRNA in mice liver: relationship to anti-obesity. J Lipid Res. (2003) 44:369–79. doi: 10.1194/jlr.M200289-JLR200
115. Imamura F, Micha R, Wu JH, de Oliveira Otto MC, Otite FO, Abioye AI, et al. Effects of saturated fat, polyunsaturated fat, monounsaturated fat, and carbohydrate on glucose-insulin homeostasis: a systematic review and meta-analysis of randomised controlled feeding trials. PLoS Med. (2016) 13:e1002087. doi: 10.1371/journal.pmed.1002087
116. Hamazaki T, Okuyama H. The Japan Society for Lipid Nutrition recommends to reduce the intake of linoleic acid. A review and critique of the scientific evidence. World Rev Nutr Diet. (2003) 92:109–32. doi: 10.1159/000073796
117. Simopoulos AP. The importance of the omega-6/omega-3 fatty acid ratio in cardiovascular disease and other chronic diseases. Exp Biol Med. (2008) 233:674–88. doi: 10.3181/0711-MR-311
118. Trumbo P, Schlicker S, Yates AA, Poos M. Food, Nutrition Board of the Institute of Medicine TNA. Dietary reference intakes for energy, carbohydrate, fiber, fat, fatty acids, cholesterol, protein and amino acids. J Am Diet Assoc. (2002) 102:1621–30. doi: 10.1016/S0002-8223(02)90346-9
119. Ailhaud G, Massiera F, Weill P, Legrand P, Alessandri JM, Guesnet P. Temporal changes in dietary fats: role of n-6 polyunsaturated fatty acids in excessive adipose tissue development and relationship to obesity. Prog Lipid Res. (2006) 45:203–36. doi: 10.1016/j.plipres.2006.01.003
120. Ruiz R, Jideonwo V, Ahn M, Surendran S, Tagliabracci VS, Hou Y, et al. Sterol regulatory element-binding protein-1 (SREBP-1) is required to regulate glycogen synthesis and gluconeogenic gene expression in mouse liver. J Biol Chem. (2014) 289:5510–7. doi: 10.1074/jbc.M113.541110
121. Muzsik A, Jelen HH, Chmurzynska A. Metabolic syndrome in postmenopausal women is associated with lower erythrocyte PUFA/MUFA and n-3/n-6 ratio: a case-control study. Prostaglandins Leukot Essent Fatty Acids. (2020) 159:102155. doi: 10.1016/j.plefa.2020.102155
122. Melis M, Carta G, Pintus S, Pintus P, Piras CA, Murru E, et al. Polymorphism rs1761667 in the CD36 gene is associated to changes in fatty acid metabolism and circulating endocannabinoid levels distinctively in normal weight and obese subjects. Front Physiol. (2017) 8:1006. doi: 10.3389/fphys.2017.01006
123. Clarke SD. Polyunsaturated fatty acid regulation of gene transcription: a molecular mechanism to improve the metabolic syndrome. J Nutr. (2001) 131:1129–32. doi: 10.1093/jn/131.4.1129
124. Linden AG, Li S, Choi HY, Fang F, Fukasawa M, Uyeda K, et al. Interplay between ChREBP and SREBP-1c coordinates postprandial glycolysis and lipogenesis in livers of mice. J Lipid Res. (2018) 59:475–87. doi: 10.1194/jlr.M081836
125. Xu J, Cho H, O'Malley S, Park JH, Clarke SD. Dietary polyunsaturated fats regulate rat liver sterol regulatory element binding proteins-1 and−2 in three distinct stages and by different mechanisms. J Nutr. (2002) 132:3333–9. doi: 10.1093/jn/132.11.3333
126. Zinocker MK, Svendsen K, Dankel SN. The homeoviscous adaptation to dietary lipids (HADL) model explains controversies over saturated fat, cholesterol, and cardiovascular disease risk. Am J Clin Nutr. (2021) 113:277–89. doi: 10.1093/ajcn/nqaa322
127. Reeves PG. Components of the AIN-93 diets as improvements in the AIN-76A diet. J Nutr. (1997) 127(Suppl. 5):838S−41S. doi: 10.1093/jn/127.5.838S
128. Speakman JR. Use of high-fat diets to study rodent obesity as a model of human obesity. Int J Obes. (2019) 43:1491–2. doi: 10.1038/s41366-019-0363-7
129. Carta G, Murru E, Banni S, Manca C. Palmitic acid: physiological role, metabolism and nutritional implications. Front Physiol. (2017) 8:902. doi: 10.3389/fphys.2017.00902
Keywords: palmitic acid, de novo lipogenesis, fatty acid metabolism, dietary fatty acids, saturated/unsaturated ratio
Citation: Murru E, Manca C, Carta G and Banni S (2022) Impact of Dietary Palmitic Acid on Lipid Metabolism. Front. Nutr. 9:861664. doi: 10.3389/fnut.2022.861664
Received: 24 January 2022; Accepted: 07 February 2022;
Published: 23 March 2022.
Edited by:
Carlo Virginio Agostoni, University of Milan, ItalyReviewed by:
Giuseppe Poli, University of Turin, ItalyCopyright © 2022 Murru, Manca, Carta and Banni. This is an open-access article distributed under the terms of the Creative Commons Attribution License (CC BY). The use, distribution or reproduction in other forums is permitted, provided the original author(s) and the copyright owner(s) are credited and that the original publication in this journal is cited, in accordance with accepted academic practice. No use, distribution or reproduction is permitted which does not comply with these terms.
*Correspondence: Sebastiano Banni, YmFubmlAdW5pY2EuaXQ=
†These authors have contributed equally to this work