- 1Saisei Mirai Cell Processing Center, Osaka, Japan
- 2Kobe Saisei Mirai Clinic, Kobe, Japan
- 3Inui Immunotherapy Clinic, Osaka, Japan
- 4Drug Discovery of Next-Generation GcMAF, Kagoshima University Graduate School of Medical and Dental Sciences, Kagoshima, Japan
- 5Pharmacological Department of Herbal Medicine, Kagoshima University Graduate School of Medical and Dental Sciences, Kagoshima, Japan
The effects of degalactosylated whey protein on lipopolysaccharide (LPS)-induced inflammatory responses in mice were observed in comparison with intact whey protein. Intraperitoneal administration of both intact and degalactosylated whey proteins for 5 days did not affect body weight and food intake in mice. On day 6, intraperitoneal administration of LPS induced a marked decrease in body weight 4 h later. The LPS-induced decrease in body weight was significantly suppressed by the administration of degalactosylated whey protein, but not intact whey protein. Administration of LPS also significantly increase plasma tumor necrosis factor-α (TNF-α) and interleukin-1β (IL-1β) levels, which were significantly suppressed by the administration of degalactosylated whey protein, but not intact whey protein. Moreover, the application of degalactosylated whey protein to RAW264.7 cells significantly reduced mRNA expression of toll-like receptor 4 (TLR4) and significantly increased mRNA expression of mitogen-activated protein kinase phosphatase-1 (MKP-1). The marked increased expression of TNF-α and IL-1β in response to LPS in RAW264.7 cells was significantly suppressed by the application of degalactosylated whey protein. These results suggest that degalactosylated whey protein suppresses the effects of LPS in part by decreasing in TLR4 and increasing in MKP-1.
Introduction
The protein fraction of milk contains many valuable nutritional components and biologically active substances (1). Milk contains two primary sources of protein, casein and whey (2). Whey is the liquid remaining after precipitation and removal of milk casein curd during the manufacture of cheese, and recognized as a functional food with nutritional applications and health benefits, including disease prevention and treatment (2, 3). Whey comprises beta-lactoglobulin, alpha-lactalbumin, bovine serum albumin, lactoferrin, immunoglobulins, lactoperoxidase enzymes, glycomacropeptides, lactose, and minerals (3), and has antioxidant, antihypertensive, antitumor, antiviral, hypolipidemic, and anti-bacterial effects in vitro and in vivo (2). Several lines of evidence suggest that whey protein has immunoregulatory effects (4–7). For example, animal studies revealed that whey protein reduces the levels of proinflammatory cytokines, such as tumor necrosis factor-α (TNF-α) and interleukin-1β (IL-1β), and increases the levels of anti-inflammatory cytokines, such as interleukin-4 and interleukin-6 (8–11), making whey protein a promising therapeutic candidate for immunomodulation in different diseases (11). The precise mechanisms underlying the immunomodulatory effects of whey protein, however, are not clear. Moreover, the effects of whey protein on the actions of lipopolysaccharide (LPS) are not examined.
Protein glycosylation is involved in multiple biological processes, such as cell recognition, differentiation, development, signal transduction, and immune responses (12). Glycoproteins are present in various forms, e.g., enzymes (13, 14), peptide hormones, antibodies, lectins, membrane bound proteins, collagen, and fibronectin, to induce biological activities (15–20). Deglycosylation converts biologically inactive proteins to biologically active proteins. For example, deglycosylated melanin-concentrating hormone receptor is fully active for ligand binding and signal transduction (21).
In the present study, we examined the immunomodulatory effects of degalactosylated whey protein on the inflammatory responses to LPS in mice. Our findings revealed that the functional benefits of the degalactosylation of whey protein in converting inactive protein to active protein in suppressing the inflammatory responses to LPS, of which possible mechanisms are partially attributed to decrease in toll-like receptor 4 (TLR4) and increase in mitogen-activated protein kinase phosphatase-1 (MKP-1).
Materials and Methods
Experimental Animals
Male C57BL/6J mice (6 weeks old) were obtained from CLEA Japan, Inc. (Tokyo, Japan) and individually housed in plastic cages under a 12:12 h light/dark cycle (lights turned on at 7 am) at room temperature (23 ± 1°C). The animals were given ad libitum access to water and food (CE-2; CLEA Japan, Inc.). Animals were used in the experiments at 10 weeks of age. Eight to twelve mice were used in each group. All experiments were performed in accordance with the guidelines established by the Institute of Laboratory Animal Science Research Support Center at Kagoshima University and the United States National Institutes of Health Guide, and approved by the Kagoshima University Institutional Animal Care and Use Committee for the care and use of laboratory animals. Every effort was made to optimize the comfort of the animals and to minimize their use.
Preparation of Degalactosylated Whey Protein
Whey protein was obtained from Yotsuba Milk Products Co., Ltd. (Sapporo, Japan). The whey protein was first treated with enzyme as described previously (22). Briefly, 1 mg of whey protein was dissolved in 1 ml of 50 mM sodium phosphate buffer (pH 7.0) and incubated with 65 mU of β-D-galactosidase (from Escherichia coli; WAKO Pure Chemical Industries, Ltd., Osaka, Japan) at 37°C for 1 h. The reaction mixture was heated at 60°C for 10 min to inactivate the enzyme. The protein concentrations were determined using a Pierce® BCA protein assay kit (Thermo Fisher Scientific Inc., Waltham, MA, United States).
In vivo Experimental Schedule in Mice
Intact and degalactosylated whey proteins (10 mg/kg) were intraperitoneally administered twice a day at 11 a.m. and 5 p.m. for 5 days in mice (Figure 1), according to a previous report (9, 23). Body weight and food intake were measured every day for 5 days as same as our previous reports (24, 25). On day 6, the intact and degalactosylated whey proteins were intraperitoneally administered with LPS (0.33 mg/kg). According to the previous report showing that LPS-induced sickness behavior and elevation of plasma levels of pro-inflammatory cytokines, IL-1β and TNF-α, in mice appears at 2 h after LPS injection and persists for 7 h (26–28), blood samples were collected from the retroorbital vein 4 h later under isoflurane anesthesia and immediately transferred to tubes containing EDTA (10 μl of 0.2 M EDTA/tube) and aprotinin (0.1 mg/tube, Merck KGaA, Darmstadt, Germany). The blood samples were centrifuged at 3,000 × g for 5 min at 4°C, and the plasma was separated and stored at –80°C until assayed. Body weight changes were also measured at 4 h after LPS administration.
Enzyme-Linked Immunosorbent Assay
Using 100 μl of plasma, plasma levels of tumor necrosis factor-α (TNF-α) and interleukin-1β (IL-1β) were measured using commercially available kits, TNF-α kit (Mouse TNF-alpha Quantikine ELISA Kit, R&D Systems, MN, United States) and IL-1β kit (Mouse IL-1 beta/IL-1F2 Quantikine ELISA Kit, R&D Systems), respectively.
Cell Cultures
The mouse macrophage cell line RAW264.7 (ECACC 91062702, European Tissue Culture Collection, London, United Kingdom) was cultured in Dulbecco’s Modified Eagle Medium (DMEM; Thermo Fisher Scientific Inc.) containing 10% fetal bovine serum (Thermo Fisher Scientific Inc.) and 1% antibiotic-antimycotic solution (Thermo Fisher Scientific Inc.), seeded in 6-well plates (5 × 105 cells/ml/well), and maintained in 95% humidified air and 5% CO2 at 37°C for 3–4 days, as described previously (29). Cells were incubated with degalactosylated whey protein (100 μg mg/ml) for 3 h to evaluate the effects of degalactosylated whey protein on the mRNA expression of TLR4 and MKP-1. Moreover, to examine the effects of degalactosylated whey protein on the LPS-induced increase in the mRNA expression of TNF-α and IL-1β, cells were first incubated with degalactosylated whey protein (100 μg mg/ml) for 3 h, and then with LPS (5 ng/ml) and degalactosylated whey protein (100 μg mg/ml) for another 3 h.
Reverse Transcription-Polymerase Chain Reaction
The mRNA levels were measured by quantitative real-time reverse transcription-polymerase chain reaction (RT-PCR) (29, 30). Following the treatment, the cells were lysed and total RNA was isolated using RNeasy Mini Kit (QIAGEN, Hilden, Germany), and cDNA was synthesized using Verso cDNA Synthesis Kit (Thermo Fisher Scientific Inc.). Aliquots of diluted cDNA were amplified with FastStart SYBR Green Master (Roche Applied Science, Mannheim, Germany) in a final volume of 25 μl on Thermal Cycler Dice Real Time System (TAKARA BIO INC., Shiga, Japan). The RT-PCR schedule was 95°C for 10 min followed by 40 cycles of 5 s at 95°C, 30 s at 60°C and 1 cycle of 15 s at 95°C, 30 s at 60°C and 15 s at 95°C. The samples were run in duplicate. All gene-specific mRNA expression values were normalized against the internal housekeeping gene, glyceraldehyde-3-phosphate dehydrogenase (GAPDH). Primers were as follows: GAPDH (sense TGCACCACCAACTGCTTAGC, antisense GGATGCAGGGATGATGTTCTG); TLR4 (sense GCTTTCA CCTCTGCCTTCAC, antisense AGGCGATACAATTCCACC TG); MKP-1 (sense GGCCAGCTGCTGCAGTTTGAGT, antisense AGGTGCCCCGGTCAAGGACA); TNF-α (sense GTG GAACTGGCAGAAGAG, antisense CCATAGAACTGATG AGAGG); IL-1β (sense CTGTGTCTTTCCCGTGGACC, antisense CAGCTCATATGGGTCCGACA).
Statistical Analysis
Data are expressed as mean ± SEM. Statistical analysis of the data was performed by ANOVA followed by the Tukey-Kramer test. Statistical significance was defined as P < 0.05.
Results
Effects of Intraperitoneal Administration of Degalactosylated Whey Protein for 5 Days on Body Weight and Food Intake in Mice
Intraperitoneal administration of both intact and degalactosylated whey proteins (10 mg/kg) for 5 days did not affect body weight in mice (Figure 2A). In addition, intraperitoneal administration of both intact and degalactosylated whey proteins for 5 days did not influence cumulative food intake for 5 days in mice (Figure 2B).
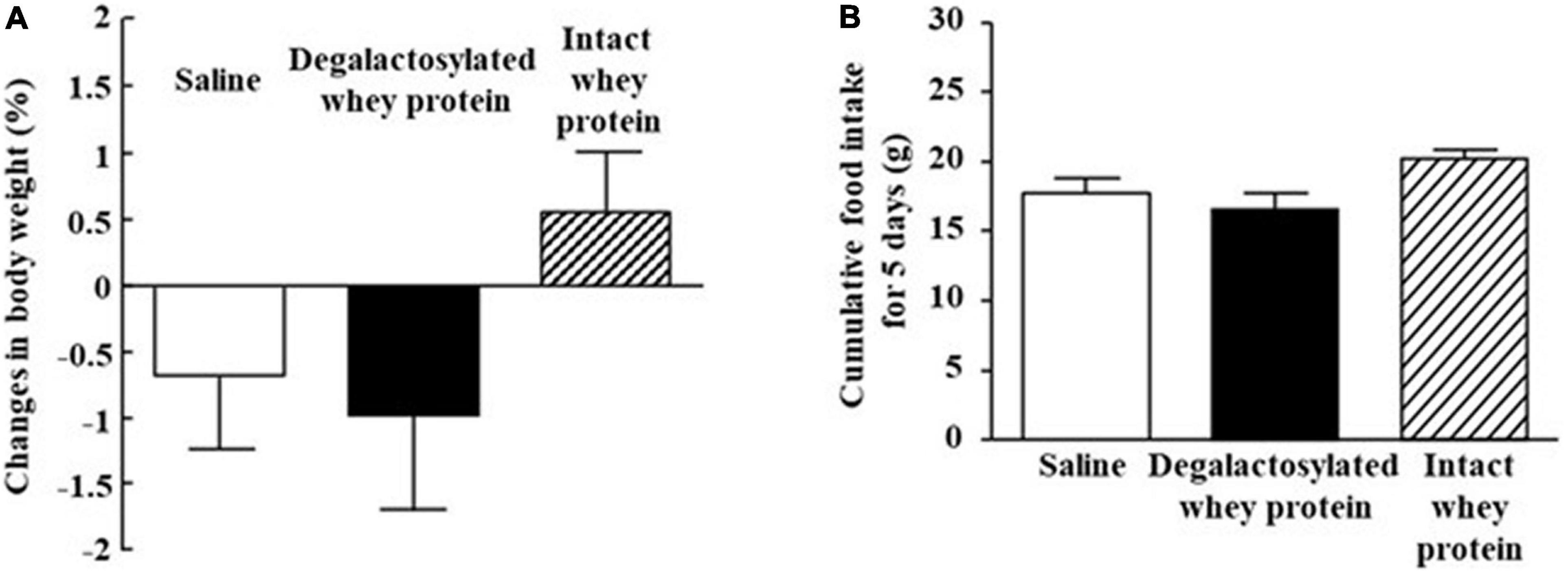
Figure 2. Effects of intraperitoneal administration of degalactosylated whey protein for 5 days on body weight and food intake in mice. (A) Body weight changes (%) in mice after intraperitoneal treatment of intact and degalactosylated whey proteins (10 mg/kg) for 5 days. (B) Cumulative food intake in mice intraperitoneally treated with intact and degalactosylated whey proteins (10 mg/kg) for 5 days. Results are expressed as mean ± SE for 8–12 mice.
Effects of Intraperitoneal Administration of Degalactosylated Whey Protein for 5 Days on Body Weight Loss Induced by Intraperitoneal Administration of Lipopolysaccharide in Mice
Intraperitoneal administration of LPS (0.33 mg/kg) induced a marked decrease in body weight 4 h later, which was significantly suppressed by intraperitoneal administration of degalactosylated whey protein (10 mg/kg) in mice pretreated with degalactosylated whey protein to 62% of the body weight loss induced by LPS treatment in saline-pretreated mice (Figure 3). Intact whey protein did not influence LPS-induced body weight loss in mice (Figure 3).
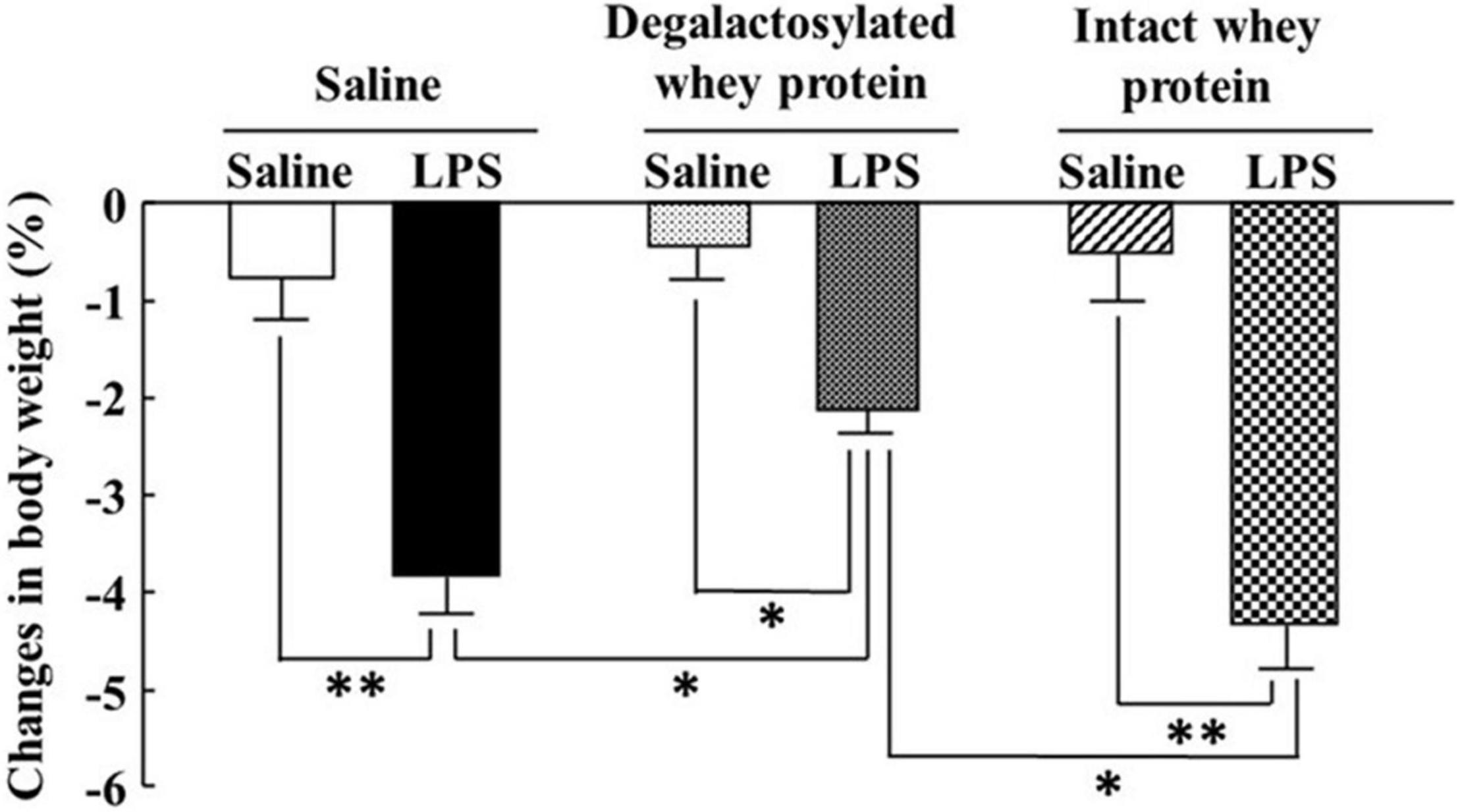
Figure 3. Effects of intraperitoneal administration of degalactosylated whey protein for 5 days on body weight loss induced by intraperitoneal administration of LPS in mice. Body weight changes (%) in mice at 4 h after intraperitoneal administration of LPS (0.33 mg/kg) following intraperitoneal administration of intact and degalactosylated whey proteins (10 mg/kg) for 5 days. Results are expressed as mean ± SE for 8–12 mice. *p < 0.05, **p < 0.01.
Effects of Intraperitoneal Administration of Degalactosylated Whey Protein for 5 Days on the Increase in Plasma Tumor Necrosis Factor-α and Interleukin-1β Levels Induced by Intraperitoneal Administration of Lipopolysaccharide in Mice
Intraperitoneal administration of LPS (0.33 mg/kg) in mice induced a marked increase in plasma TNF-α and IL-1β levels 4 h later (Figures 4A,B). The increase in the plasma TNF-α and IL-1β levels induced by LPS was significantly suppressed by intraperitoneal administration of degalactosylated whey protein (10 mg/kg) in mice pretreated with degalactosylated whey protein (10 mg/kg) to 54 and 10%, respectively, of the levels induced by LPS treatment in saline-pretreated mice, respectively (Figures 4A,B). Intact whey protein (10 mg/kg) did not suppress the increase in these plasma cytokine levels induced by intraperitoneal administration of LPS (Figures 4A,B).
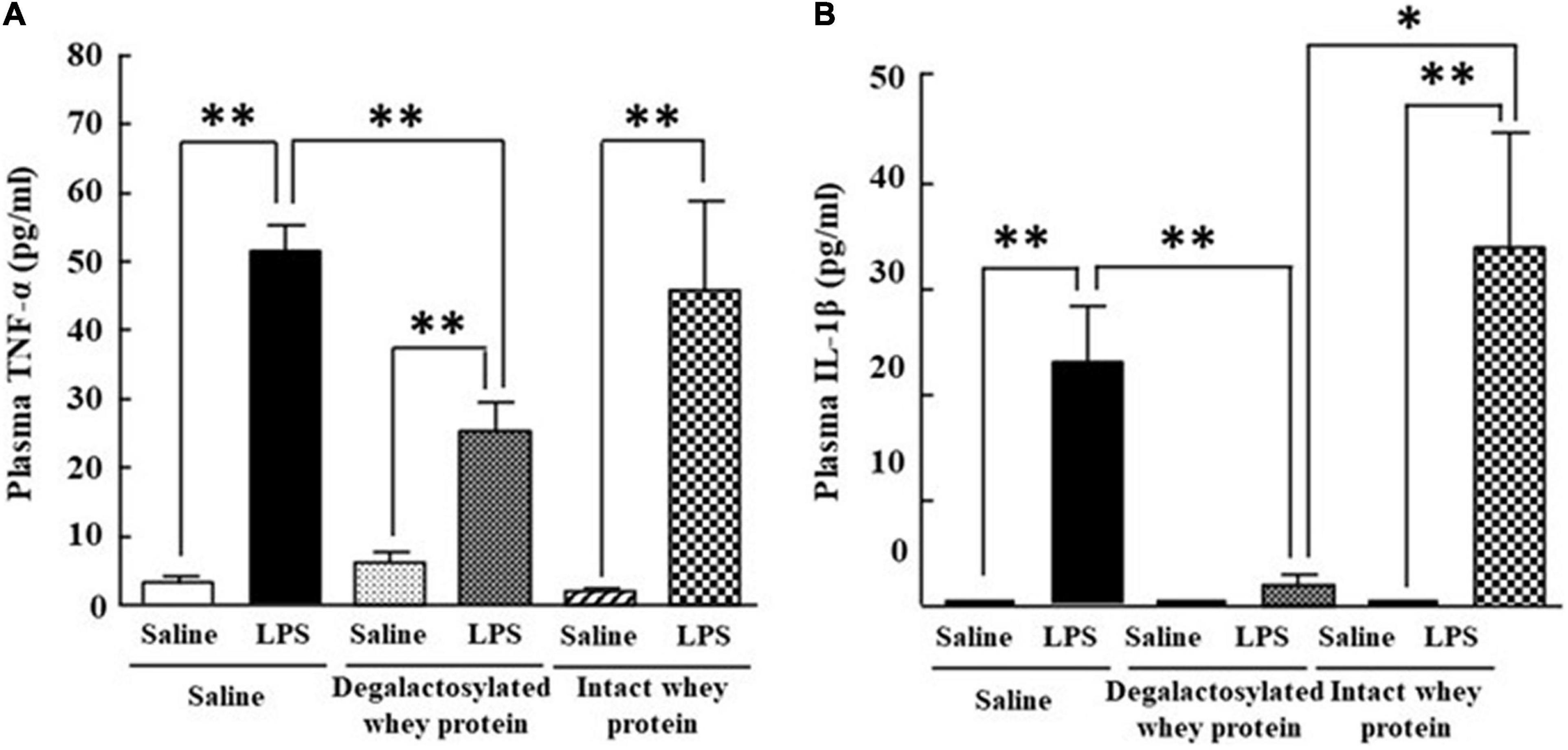
Figure 4. Effects of intraperitoneal administration of degalactosylated whey protein for 5 days on increases in plasma TNF-α and IL-1β levels induced by intraperitoneal administration of LPS in mice. (A) Changes in plasma TNF-α levels at 4 h after intraperitoneal administration of LPS (0.33 mg/kg) following intraperitoneal administration of intact and degalactosylated whey proteins (10 mg/kg) for 5 days in mice. (B) Changes in plasma IL-1β levels at 4 h after intraperitoneal administration of LPS (0.33 mg/kg) following intraperitoneal administration of intact and degalactosylated whey proteins (10 mg/kg) for 5 days. Results are expressed as mean ± SE for 8–12 mice. *p < 0.05, **p < 0.01.
Effects of Degalactosylated Whey Protein on the mRNA Expression of Toll-Like Receptor 4 and Mitogen-Activated Protein Kinase Phosphatase-1, and Lipopolysaccharide-Induced mRNA Expression of Tumor Necrosis Factor-α and Interleukin-1β in RAW264.7 Cells
The increased mRNA expression of TNF-α and IL-1β in response to LPS (5 ng/ml) in RAW264.7 cells was significantly suppressed by the application of degalactosylated whey protein (100 μg/ml) to 75 and 79%, respectively, of the levels of cells treated with LPS alone, respectively, while degalactosylated whey protein itself did not change the mRNA expression of TNF-α and IL-1β in RAW264.7 cells (Figures 5A,B). In addition, the application of degalactosylated whey protein (100 μg/ml) to RAW264.7 cells induced a significant reduction in the mRNA expression of TLR4 to 80% of the control group (Figure 5C). Moreover, the application of degalactosylated whey protein (100 μg/ml) to RAW264.7 cells induced a significant increase in the mRNA expression of MKP-1 to 122% of the control group (Figure 5D).
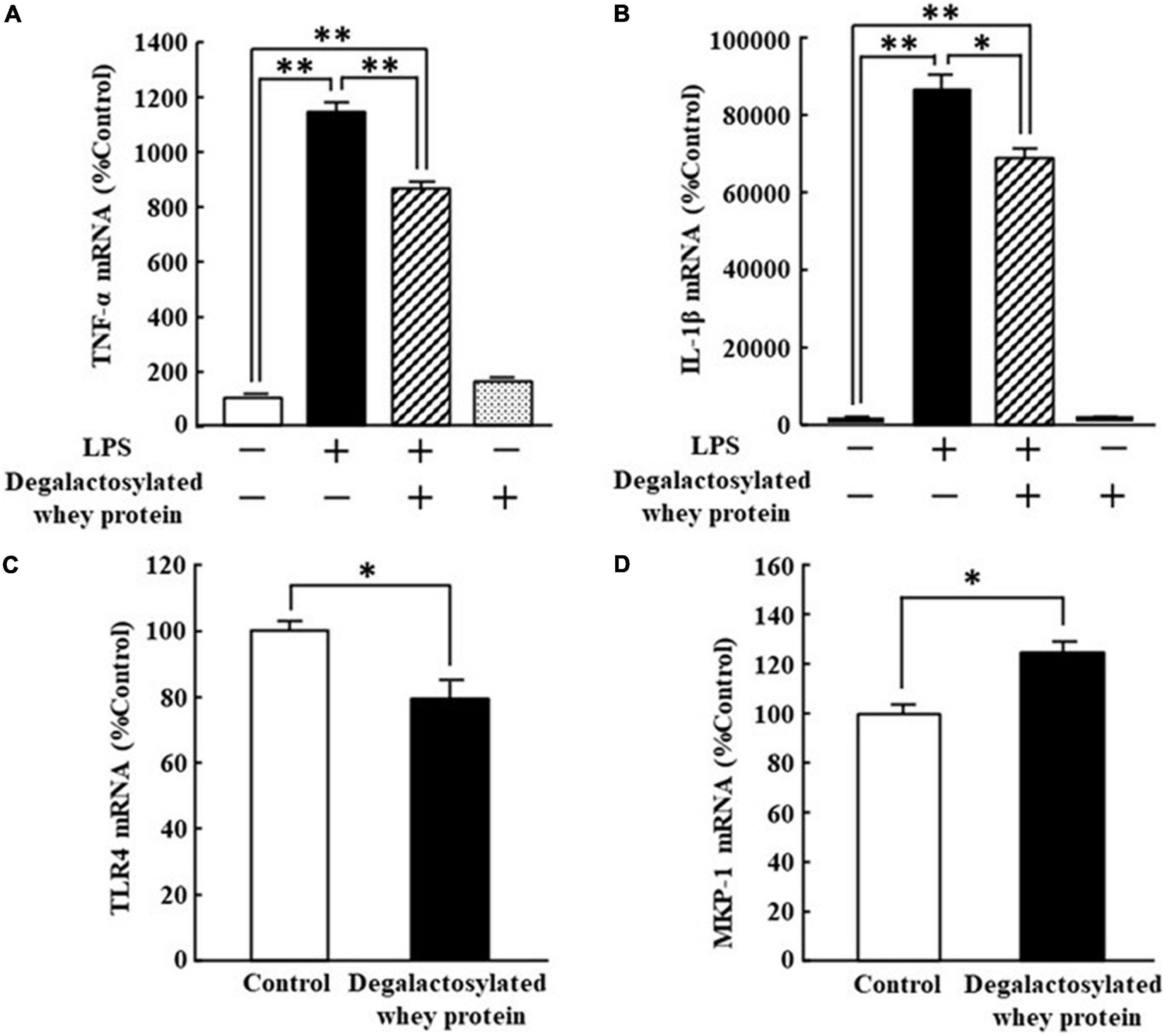
Figure 5. Effects of degalactosylated whey protein on mRNA expression of TLR4 and MKP-1, and LPS-induced mRNA expression of TNF-α and IL-1β in RAW264.7 cells. (A) Effects of 3-h preincubation with degalactosylated whey protein (100 μg mg/ml) on mRNA expression of TNF-α induced by LPS (5 ng/ml) after incubation for another 3 h. (B) Effects of 3-h preincubation with degalactosylated whey protein (100 μg mg/ml) on mRNA expression of IL-1β induced by LPS (5 ng/ml) after incubation for another 3 h. (C) Effects of 3-h incubation with degalactosylated whey protein (100 μg mg/ml) on mRNA expression of TRL-4. (D) Effects of 3-h incubation with degalactosylated whey protein (100 μg mg/ml) on mRNA expression of MKP-1. Results are expressed as mean ± SE for 8–12. *p < 0.05, **p < 0.01.
Discussion
The findings of the present study demonstrated that degalactosylated whey protein, but not intact whey protein, significantly suppressed the increase in plasma TNF-α and IL-1β levels following LPS administration in mice. In addition, following in vitro application of degalactosylated whey protein to mouse macrophage Raw264.7 cells, TLR4 mRNA was significantly decreased and MKP-1 mRNA was significantly increased. Moreover, degalactosylated whey protein significantly suppressed the increase in TNF-α and IL-1β mRNA induced by LPS application in Raw264.7 cells. These findings suggest that degalactosylated whey protein has anti-inflammatory activity.
Whey protein intake is beneficial for exercise performance and exercise enhancement. Whey protein also appears to be very promising as a functional food with several health benefits, such as antioxidant, antihypertensive, antitumor, antiviral, hypolipidemic, and antibacterial activity (2, 31). Further, whey protein is reported to exhibit several immunoregulatory actions (4–7). Animal studies demonstrated that whey protein reduces the production of proinflammatory cytokines like TNF-a, IL-6, and IL-1β, and increases the production of anti-inflammatory cytokines like IL-4 (8–11). Our findings of this study revealed that degalactosylated whey protein, but not intact whey protein, significantly reduced the increased plasma levels of TNF-α and IL-1β following LPS administration in mice. Protein glycosylation is a common post-translational modification of numerous important biological processes (12). Protein glycosylation plays a significant role in protein folding, targeted transport, cellular localization, and activity (32). On the other hand, deglycosylation converts biologically inactive proteins to biologically active proteins. For example, non-glycosylated V2 vasopressin receptors are fully active for ligand binding and signal transduction (21). Similarly, in the case of whey protein, the present study showed that galactosidase treatment converts inactive protein to active protein to suppress LPS responses. LPS has complex interactions with TLR4 on host immune cells including macrophages and microglia, where it induces the production of pro-inflammatory cytokines (33). These cytokines invoke a constellation of motivated behavioral adaptations, so-called sickness behavior (34). Sickness behaviors include loss of appetite and body weight, fatigue, withdrawal from normal social activities, altered cognition, hyperalgesia, and fever (35, 36). Sickness behaviors are well known to be due to the central actions of proinflammatory cytokines, such as TNF-α and IL-1β (37). In the present study, consistent with the previous reports (27, 34), LPS induced a marked decrease in body weight at 4 h after its administration in mice, which was significantly suppressed by the administration of degalactosylated whey protein, but not intact whey protein. Moreover, the present study showed that degalactosylated whey protein, but not intact whey protein, significantly decreased the enhancement of TNF-α and IL-1β production in response to LPS in in vivo and in vitro studies. Our findings suggest that degalactosylated whey protein attenuates sickness behavior.
We next tried to confirm the suppressive effects of degalactosylated whey protein on the mRNA expression of TNF-α and IL-1β using mouse macrophage Raw264 cells. Moreover, we examined the effects of degalactosylated whey protein on the expression of TLR4 and MKP-1 to elucidate the mechanisms underlying the anti-inflammatory activities of degalactosylated whey protein. Stimulation of TLR4 activates proinflammatory pathways and induces cytokine expression in a variety of cell types (38). Accordingly, suppression of TLR4 signaling could be therapeutically useful for suppressing an inappropriately overactive immune system (33). TLR4 antagonists decrease the production of pro-inflammatory cytokines in LPS-stimulated cultured human monocytes (33). The signaling transduction resulting from interactions between TLR4 and the mitogen-activated protein kinase (MAPK) family including the extracellular signal-regulated kinase 1/2 (ERK1/2), Jun N-terminal kinase (JNK), and p38 subfamilies, plays a pivotal role in the biosynthesis of proinflammatory cytokines in response to LPS in macrophages (38–42). MAPK phosphatase-1 (MKP-1) is an archetypal member of the dual specificity protein phosphatase family that dephosphorylates MAPK. MKP-1 is an anti-inflammatory phosphatase that negatively regulates MAPK activities (43, 44). Similarly, MKP-1-knockout mice showed the enhancement of plasma proinflammatory cytokine levels in response to LPS compared with wild-type mice, and, moreover, MKP-1 deficient macrophages exhibited enhanced production of proinflammatory cytokines compared with wild-type cells (45). MKP-1 upregulation in the central nervous system is also associated with reduced inflammation via endocannabinoid-mediated microglial expression (46). In the present study, in Raw264 cells, degalactosylated whey protein significantly suppressed TNF-α and IL-1β mRNA expression increased after LPS stimulation. Moreover, degalactosylated whey protein significantly decreased TLR4 expression and increased MKP-1 expression in Raw264 cells. Based on these findings, the anti-inflammatory actions of degalactosylated whey protein are attributed to deceased TLR4 expression and increased MKP-1 expression. These findings indicate that degalactosylated whey protein has potent immunoregulatory effects. However, the study on oral administration of whey proteins should be necessary to elucidate the role of whey protein supplementation in suppressing inappropriate overactivation of the immune response.
Conclusion
The present findings revealed that degalactosylated whey protein has an anti-inflammatory effect in comparison to intact whey protein. The present data expand our knowledge about the role of degalactosylated whey protein in suppressing inappropriate overactivation of the immune response.
Data Availability Statement
The original contributions presented in the study are included in the article/supplementary material, further inquiries can be directed to the corresponding author/s.
Ethics Statement
The animal study was reviewed and approved by Akira Sano, President of Kagoshima University.
Author Contributions
TI, NK, and GK performed the experiments, contributed to discussions, and wrote the manuscript. RN performed the experiments. AI contributed to discussions, reviewed, and edited the manuscript. NK was the guarantor of this work and, as such, had full access to all the data in this study and took responsibility for the integrity of the data and the accuracy of the data analysis. All authors contributed to the article and approved the submitted version.
Funding
This work was supported by the Institute of Laboratory Animal Science Research Support Center Kagoshima University.
Conflict of Interest
The authors declare that the research was conducted in the absence of any commercial or financial relationships that could be construed as a potential conflict of interest.
Publisher’s Note
All claims expressed in this article are solely those of the authors and do not necessarily represent those of their affiliated organizations, or those of the publisher, the editors and the reviewers. Any product that may be evaluated in this article, or claim that may be made by its manufacturer, is not guaranteed or endorsed by the publisher.
Acknowledgments
We wish to thank Joint Research Laboratory, Kagoshima University Graduate School of Medical and Dental Sciences, for the use of their facilities.
References
1. Meisel H. Overview on milk protein-derived peptides. Int Dairy J. (1998) 363:363–73. doi: 10.1016/S0958-6946(98)00059-4
3. Walzem RL, Dillard CJ, German JB. Whey components: millennia of evolution create functionalities for mammalian nutrition: what we know and what we may be overlooking. Crit Rev Food Sci Nutr. (2002) 42:353–75. doi: 10.1080/10408690290825574
4. Hakansson A, Andreasson J, Zhivotovsky B, Karpman D, Orrenius S, Svanborg C. Multimeric alpha-lactalbumin from human milk induces apoptosis through a direct effect on cell nuclei. Exp Cell Res. (1999) 246:451–60. doi: 10.1006/excr.1998.4265
5. Beaulieu J, Dupont C, Lemieux P. Whey proteins and peptides: beneficial effects on immune health. Therapy. (2006) 3:1–10. doi: 10.1586/14750708.3.1.69
6. Rusu D, Drouin R, Pouliot Y, Gauthier S, Poubelle PE. A bovine whey protein extract stimulates human neutrophils to generate bioactive IL-1Ra through a NF-kappaB- and MAPK-dependent mechanism. J Nutr. (2010) 140:382–91. doi: 10.3945/jn.109.109645
7. Castro GA, Maria DA, Bouhallab S, Sgarbieri VC. In vitro impact of a whey protein isolate (WPI) and collagen hydrolysates (CHs) on B16F10 melanoma cells proliferation. J Dermatol Sci. (2009) 56:51–7. doi: 10.1016/j.jdermsci.2009.06.016
8. Ebaid H, Salem A, Sayed A, Metwalli A. Whey protein enhances normal inflammatory responses during cutaneous wound healing in diabetic rats. Lipids Health Dis. (2011) 10:235–44. doi: 10.1186/1476-511X-10-235
9. Kume H, Okazaki K, Sasaki H. Hepatoprotective effects of whey protein on D-galactosamine-induced hepatitis and liver fibrosis in rats. Biosci Biotechnol Biochem. (2006) 70:1281–5. doi: 10.1271/bbb.70.1281
10. Takayanagi T, Sasaki H, Kawashima A, Mizuochi Y, Hirate H, Sugiura T, et al. A new enteral diet, MHN-02, which contains abundant antioxidants and whey peptide, protects against carbon tetrachloride-induced hepatitis. J Parenter Enteral Nutr. (2011) 35:516–22. doi: 10.1177/0148607110381599
11. Badr G, Ebaid H, Mohany M, Abuelsaad AS. Modulation of immune cell proliferation and chemotaxis towards CC chemokine ligand (CCL)-21 and CXC chemokine ligand (CXCL)-12 in undenatured whey protein treated mice. J Nutr Biochem. (2012) 23:1640–6. doi: 10.1016/j.jnutbio.2011.11.006
12. Lin B, Qing X, Liao J, Zhuo K. Role of protein glycosylation in host-pathogen interaction. Cells. (2020) 9:1022. doi: 10.3390/cells9041022
13. Pagano MR, Mendieta JR, Munoz FF, Daleo GR, Guevara MG. Roles of glycosylation on the antifungal activity and apoplast accumulation of StAPs (Solanum tuberosum aspartic proteases). Int J Biol Macromol. (2007) 41:512–20. doi: 10.1016/j.ijbiomac.2007.07.009
14. Yan QJ, Qi XW, Jiang ZQ, Yang SQ, Han LJ. Characterization of a pathogenesis-related class 10 protein (PR-10) from Astragalus mongholicus with ribonuclease activity. Plant Physiol Biochem. (2008) 46:93–9. doi: 10.1016/j.plaphy.2007.10.002
15. Park JI, Semyonov J, Chang CL, Hsu SYT. Conservation of the heterodimeric glycoprotein hormone subunit family proteins and the LGR signaling system from nematodes to humans. Endocrine. (2005) 26:267–76. doi: 10.1385/ENDO:26:3:267
16. Shinkawa T, Nakamura K, Yamane N, Shoji-Hosaka E, Kanda Y, Sakurada M, et al. The absence of fucose but not the presence of galactose or bisecting N-acetylglucosamine of human IgG1 complex-type oligosaccharides shows the critical role of enhancing antibody-dependent cellular cytotoxicity. J Biol Chem. (2003) 278:3466–73. doi: 10.1074/jbc.M210665200
17. Su YP, Bakker T, Harris J, Tsang C, Brown GD, Wormald MR. Glycosylation influences the lectin activities of the macrophage mannose receptor. J Biol Chem. (2005) 280:32811–20. doi: 10.1074/jbc.M503457200
18. Tytgat HLP, de Vos WM. Sugar coating the envelope: glycoconjucates for microbe-host crosstalk. Trends Microbiol. (2016) 24:853–61. doi: 10.1016/j.tim.2016.06.004
19. Bann JG, Peyton DH, Bachinger HP. Sweet is stable: glycosylation stabilizes collagen. FEBS Lett. (2000) 473:237–40. doi: 10.1016/s0014-5793(00)01493-9
20. Sano K, Asahi M, Yanagibashi M, Hashii N, Itoh S, Kawasaki N, et al. Glycosylation and ligand-binding activities of rat plasma fibronectin during liver regeneration after partial hepatectomy. Carbohydr Res. (2008) 343:2329–35. doi: 10.1016/j.carres.2008.03.027
21. Innamorati G, Sadeghi H, Birnbaumer M. A fully active nonglycosylated V2 vasopressin receptor. Mol Pharmacol. (1996) 50:467–73.
22. Uto Y, Kawai T, Sasaki T, Hamada K, Yamada H, Kuchiike D, et al. Degalactosylated/desialylated bovine colostrum induces macrophage phagocytic activity independently of inflammatory cytokine production. Anticancer Res. (2015) 35:4487–92.
23. Maryniak NZ, Hansen EB, Ballegaard ASR, Sancho AI, Bøgh KL. Comparison of the allergenicity and immunogenicity of camel and Cow’s milk-a study in brown Norway rats. Nutrients. (2018) 10:1903. doi: 10.3390/nu10121903
24. Satoh N, Ogawa Y, Katsuura G, Numata Y, Masuzaki H, Yoshimasa Y, et al. Satiety effect and sympathetic activation of leptin are mediated by hypothalamic melanocortin system. Neurosci Lett. (1998) 249:107–10. doi: 10.1016/s0304-3940(98)00401-7
25. Yamada-Goto N, Katsuura G, Ebihara K, Inuzuka M, Ochi Y, Yamashita Y, et al. Intracerebroventricular administration of C-type natriuretic peptide suppresses food intake via activation of the melanocortin system in mice. Diabetes. (2013) 62:1500–4. doi: 10.2337/db12-0718
26. MacDonald L, Radler M, Paolini AG, Kent S. Calorie restriction attenuates LPS-induced sickness behavior and shifts hypothalamic signaling pathways to an anti-inflammatory bias. Am J Physiol Regul Integr Comp Physiol. (2011) 301:R172–84. doi: 10.1152/ajpregu.00057.2011
27. Iwasa T, Matsuzaki T, Kinouchi R, Gereltsetseg G, Murakami M, Munkhzaya M, et al. Changes in central and peripheral inflammatory responses to lipopolysaccharide in ovariectomized female rats. Cytokine. (2014) 65:65–73. doi: 10.1016/j.cyto.2013.10.004
28. Bluthé R-M, Michaud B, Poli V, Dantzer R. Role of IL-6 in cytokine-induced sickness behavior: a study with IL-6 deficient mice. Physiol Behav. (2000) 70:367–73. doi: 10.1016/s0031-9384(00)00269-9
29. Novianti E, Katsuura G, Kawamura N, Asakawa A, Inui A. Atractylenolide-III suppresses lipopolysaccharide-induced inflammation via downregulation of toll-like receptor 4 in mouse microglia. Heliyon. (2021) 7:e08269. doi: 10.1016/j.heliyon.2021.e08269
30. Kawamura N, Katsuura G, Yamada-Goto N, Novianti E, Inui A, Asakawa A. Impaired brain fractalkine-CX3CR1 signaling is implicated in cognitive dysfunction in diet-induced obese mice. BMJ Open Diab Res Care. (2021) 9:e001492. doi: 10.1136/bmjdrc-2020-001492
31. Flaim C, Kob M, Di Pierro AM, Herrmann M, Lucchin L. Effects of a whey protein supplementation on oxidative stress, body composition and glucose metabolism among overweight people affected by diabetes mellitus or impaired fasting glucose: a pilot study. J Nutr Biochem. (2017) 50:95–102. doi: 10.1016/j.jnutbio.2017.05.003
32. Struwe WB, Robinson CV. Relating glycoprotein structural heterogeneity to function – insights from native mass spectrometry. Curr Opin Struc Biol. (2019) 58:241–8. doi: 10.1016/j.sbi.2019.05.019
33. Hennessy EJ, Parker AE, O’Neill LAJ. Targeting Toll-like receptors: emerging therapeutics? Nat Rev Drug Discov. (2010) 9:293–307. doi: 10.1038/nrd3203
34. Martin SA, Pence BD, Greene RM, Johnson SJ, Dantzer R, Kelley KW, et al. Effects of voluntary wheel running on LPS-induced sickness behavior in aged mice. Brain Behav Immun. (2013) 29:113–23. doi: 10.1016/j.bbi.2012.12.014
35. Dantzer R, Kelley KW. Twenty years of research on cytokine-induced sickness behavior. Brain Behav Immun. (2007) 21:153–60. doi: 10.1016/j.bbi.2006.09.006
36. Dantzer R, O’Connor JC, Freund GG, Johnson RW, Kelley KW. From inflammation to sickness and depression: when the immune system subjugates the brain. Nat Rev Neurosci. (2008) 9:46–56. doi: 10.1038/nrn2297
37. Dantzer R. Cytokine-induced sickness behaviour: a neuroimmune response to activation of innate immunity. Eur J Pharmacol. (2004) 500:399–411. doi: 10.1016/j.ejphar.2004.07.040
38. Taylor DM, Moser R, Régulier E, Breuillaud L, Dixon M, Beesen AA, et al. MAP kinase phosphatase 1 (MKP-1/DUSP1) is neuroprotective in Huntington’s disease via additive effects of JNK and p38 inhibition. J Neurosci. (2013) 33:2313–25. doi: 10.1523/JNEUROSCI.4965-11.2013
39. Dong C, Davis RJ, Flavell RA. MAP kinases in the immune response. Annu. Rev Immunol. (2002) 20:55–72. doi: 10.1146/annurev.immunol.20.091301.131133
40. Lee JC, Kassis S, Kumar S, Badger A, Adams JL. p38 mitogen-activated protein kinase inhibitors – mechanisms and therapeutic potentials. Pharmacol Ther. (1999) 82:389–97. doi: 10.1016/s0163-7258(99)00008-x
41. Kontoyiannis D, Pasparakis M, Pizarro TT, Cominelli F, Kollias G. Impaired on/off regulation of TNF biosynthesis in mice lacking TNF AU-rich elements: implications for joint and gut-associated immunopathologies. Immunity. (1999) 10:387–98. doi: 10.1016/s1074-7613(00)80038-2
42. Dumitru CD, Ceci JD, Tsatsanis C, Kontoyiannis D, Stamatakis K, Lin JH, et al. TNF-α induction by LPS is regulated posttranscriptionally via a Tpl2/ERK-dependent pathway. Cell. (2000) 103:1071–83. doi: 10.1016/s0092-8674(00)00210-5
43. Grönwall C, Chen Y, Vas J, Khanna S, Thiel S, Corr M, et al. MAPK phosphatase-1 is required for regulatory natural autoantibody-mediated inhibition of TLR responses. Proc Natl Acad Sci USA. (2012) 109:19745–50. doi: 10.1073/pnas.1211868109
44. Wang Z, Cao N, Nantajit D, Fan M, Liu Y, Li JJ. Mitogen-activated protein kinase phosphatase-1 represses c-Jun NH2-terminal kinase-mediated apoptosis via NF-κB regulation. J Biol Chem. (2008) 283:21011–23. doi: 10.1074/jbc.M802229200
45. Zhao Q, Wang X, Nelin LD, Yao Y, Matta R, Manson ME, et al. MAP kinase phosphatase 1 controls innate immune responses and suppresses endotoxic shock. J Exp Med. (2006) 203:131–40. doi: 10.1084/jem.20051794
Keywords: whey protein, degalactosylation, lipopolysaccharide, cytokines, mice, inflammation, macrophage
Citation: Inui T, Kawamura N, Nakama R, Inui A and Katsuura G (2022) Degalactosylated Whey Protein Suppresses Inflammatory Responses Induced by Lipopolysaccharide in Mice. Front. Nutr. 9:852355. doi: 10.3389/fnut.2022.852355
Received: 11 January 2022; Accepted: 11 April 2022;
Published: 29 April 2022.
Edited by:
Gabriel Nasri Marzuca-Nassr, University of La Frontera, ChileReviewed by:
Brijeshkumar Patel, Mayo Clinic, United StatesLaureane Nunes Masi, Universidade Cruzeiro do Sul, Brazil
Copyright © 2022 Inui, Kawamura, Nakama, Inui and Katsuura. This is an open-access article distributed under the terms of the Creative Commons Attribution License (CC BY). The use, distribution or reproduction in other forums is permitted, provided the original author(s) and the copyright owner(s) are credited and that the original publication in this journal is cited, in accordance with accepted academic practice. No use, distribution or reproduction is permitted which does not comply with these terms.
*Correspondence: Toshio Inui, dG9zaGlvLmludWkxMDAzQGdtYWlsLmNvbQ==