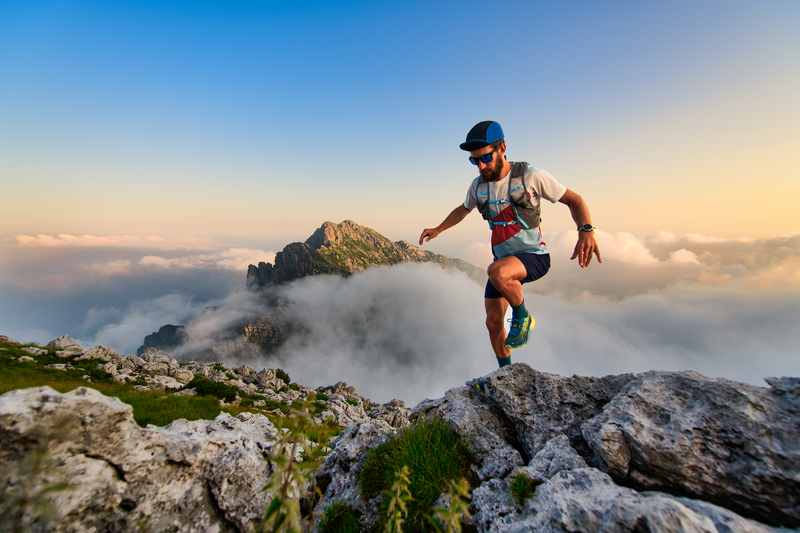
95% of researchers rate our articles as excellent or good
Learn more about the work of our research integrity team to safeguard the quality of each article we publish.
Find out more
ORIGINAL RESEARCH article
Front. Nutr. , 03 May 2022
Sec. Nutrition and Food Science Technology
Volume 9 - 2022 | https://doi.org/10.3389/fnut.2022.849928
This article is part of the Research Topic Selenium in Soil-Plant-Animal Systems and its Essential Role for Human Health View all 16 articles
Selenium (Se) biofortification during germination is an efficient method for producing Se-enriched soybean sprouts; however, few studies have investigated Se distribution in different germinated soybean proteins and its effects on protein fractions. Herein, we examined Se distribution and speciation in the dominant proteins 7S and 11S of raw soybean (RS), germinated soybean (GS), and germinated soybean with Se biofortification (GS-Se). The effects of germination and Se treatment on protein structure, functional properties, and antioxidant capacity were also determined. The Se concentration in GS-Se was 79.8-fold higher than that in GS. Selenomethionine and methylselenocysteine were the dominant Se species in GS-Se, accounting for 41.5–80.5 and 19.5–21.2% of the total Se with different concentrations of Se treatment, respectively. Se treatment had no significant effects on amino acids but decreased methionine in 11S. In addition, the α-helix contents decreased as the Se concentration increased; the other structures showed no significant changes. The Se treatment also had no significant effects on the water and oil-holding capacities in protein but increased the foaming capacity and emulsion activity index (EAI) of 7S, but only the EAI of 11S. The Se treatment also significantly increased the antioxidant capacity in 7S but not in 11S. This study indicates that the dominant proteins 7S and 11S have different Se enrichment abilities, and the protein structures, functional properties, and antioxidant capacity of GS can be altered by Se biofortification.
Soybean is a major source of high-quality plant protein, providing essential amino acids and proteins with a variety of functional properties at low cost (1, 2). Soybeans also have multiple roles in food processing due to their unique protein-related food texture (3), high water-holding capacities, and foaming properties. Thus, the soybean has been widely used in the processing of sausages (4), beverages (5), bread, and cakes (6) to modify the food texture. The structure and functionality of soybean proteins are also important for the nutrition and quality of food products (7).
Selenium (Se) is an important micronutrient for both animal and human health, and Se intake should meet the recommended adult dietary allowance of 50–60 μg/day (8). However, the Se contents in agricultural soils are considerably low in some regions, such as China, New Zealand, and parts of Europe, where insufficient Se intake from plant-derived food has become a public health issue because of low Se levels in the environment (9). Selenoproteins play important roles in both human and animal health. For example, glutathione peroxidase (GPx) can protect the human body from oxidative stress (10). Indeed, the antioxidant activity of Se has attracted increasing research interest (11, 12), and the enrichment of foods through Se biofortification has become a popular strategy to promote adequate dietary intake of Se.
Excessive Se intake can result in Se toxicity, which largely depends on both the concentration of total Se and the Se speciation in dietary materials. Different Se species are associated with different metabolism pathways and further result in different levels of Se mobility, bioavailability, and toxicity (13, 14). In general, selenite () is known to be more toxic to organisms than selenate and other organic Se compounds, such as selenomethionine (SeMet), selenocystine (SeCys2), and methylselenocysteine (MeSeCys). Previous studies also found that these five Se compounds are the dominant chemical compounds of Se accumulated in Se-enriched soybean (7). Organic Se compounds have relatively low toxicity and high nutritional value compared with inorganic Se compounds (15), and those seleno-amino acids are incorporated into proteins, namely selenoproteins. Chan et al. found that over 80% of the total Se is bonded to high-molecular-weight proteins in Se-enriched soybean (16). Se-containing proteins in food products are ideal dietary sources of Se intake and are used as food supplement products via appropriate processing procedures in the agri-food industry (17).
However, Se is distributed unequally in protein fractions. Soybean proteins mostly consist of globulins, and four kinds, namely, 2S, 7S, 11S, and 15S, account for 15, 34, 41.9, and 9.1% of all soybean globulins, respectively. 7S and 11S account for 75.9% of the total globulins (18), as the dominant proteins, they have been the focus in regards to soybean and protein processing in previous studies (19, 20). A previous study found that the abilities of different protein fractions to enrich Se differ from each other: the concentration of Se in 11S was 38% higher than that in 7S in soybeans cultivated by Se foliar spray, and 11S had a higher Se enrichment ability than 7S (7). However, there is less information on the distribution and species of Se in Se biofortification-germinated soybean proteins, and the Se distribution and these species should be clarified.
To better utilize Se-enriched germinated soybean protein, the structure of the protein should be known. Previous studies have indicated that protein structure, functional properties, and antioxidant capacity are changed during germination (21–23). Simultaneously, Se can bind to soybean proteins by S–S, Se–S, and Se–Se bonds; therefore, parts of the secondary structure, such as the α-helix, β-sheet, and random coil, are influenced (24). A previous study found that Se could influence the secondary structures of 7S and 11S in raw soybeans (25), but Deng et al. found that there was no significant effect on protein secondary structure in soybeans enriched through Se foliar spraying (7). The influence of Se on the secondary structure of raw soybean protein has not yet been clarified, and to the best of our knowledge, Se-enriched germinated soybean proteins have not been studied.
Changes in protein components and structure can affect their functional properties and antioxidant capacity. The functionality of soybean proteins, including their water-holding capacity (WHC), oil-holding capacity (OHC), foaming capacity (FC), and emulsion activity index (EAI), will influence how soybean proteins work in food processing. Germination can cause the breakdown of macro-molecular proteins, and the secondary structure of the protein changes after germination, affecting both the FC and EAI of proteins (26). Previous studies have focused on the functionality of Se-enriched soybean (7, 25), but less is known about germinated Se biofortification soybeans. Previous studies investigated the antioxidant capacity of germinated soybean (22, 27) and found that germination could improve the antioxidant capacity. The antioxidant capacity of Se-containing protein from Ganoderma lucidum was three times higher than that of the proteins without Se treatment. In addition, the capacity was correlated with the Se content in protein (28). However, the effect of Se biofortification on soybean sprout proteins remains unknown.
As the application of Se biofortification during germination in the food industry has increased, Se distribution and its effects on protein structure and functionality need to be clarified. Previous studies have confirmed that Se biofortification can promote the content of Se and influence the protein structure and functional ability of Se-enriched soybeans; however, its effects on germinated soybean proteins remain unclear. In addition, few studies have assessed Se distribution and speciation in biofortified germinated soybean proteins and their effects on the structure of different soybean protein fractions. Therefore, the purpose of our study was to investigate the effects of Se on the structure, functional properties, and antioxidant capacity of germinated soybean proteins.
Soybean (Zhonghuang 13) seeds were provided by the Institute of Crop Science of CAAS (Beijing, China). The soybean seeds were surface-sterilized with 0.1% NaClO solution for 5 min, then washed five times with deionised water (Milli-RO Plus; MilliporeSigma, Burlington, MA, USA), and soaked in different concentrations of Se solution (0, 5, 30, and 60 mg/L of sodium selenite solution) at a ratio of 1:5 (w/v) for 6 h. Later, the seeds were placed in plant tissue culture containers with two layers of gauze and then placed in an incubator in the dark with a controlled temperature of 25°C. The seeds were sprayed with deionised water (~350 ml) for 5 s every 10 h during germination, and soybean sprouts were harvested every 24 h until 120 h. Raw soybean (RS), germinated soybean in the control (GS), and germinated soybean under Se treatment (GS-Se5/30/60) were freeze-dried and ground with a grinder to pass through a 40 mesh sieve. The powders were sealed in bags until use. 7S and 11S were prepared according to the previous methods (7, 29), which are presented in the Supplementary Material.
Approximately 0.5 g of soybean sprout powder was mixed with 6 ml of HNO3 and 2 ml of H2O2 (30%) in 50-ml polypropylene tubes. The mixture was digested at 120°C until white fumes appeared. Concentrated HCl (5 ml) was added as a reductant to reduce selenate to selenite. The solution was diluted with deionised water to 25 ml, and Se was detected using hydride generation atomic fluorescence spectrometry (HG-AFS 9230; Beijing Titan Instruments, Beijing, China). The limits of quantification and detection for Se based on plant dry weight for the entire procedure were estimated to be 0.48 and 0.36 μg/kg, respectively.
Samples were prepared according to a previous study (30), with some modifications. A powdered sample (0.1 g) was transferred into a 15-ml plastic tube, and 10 ml of Tris-HCl (75 mmol/L, pH 7.5) and 10 mg protease XIV (Sigma-Aldrich, St. Louis, MO, USA) were added. The mixture was homogenized using ultrasound at 37°C for 18 h. The supernatant was collected after being centrifuged, and the filtrate was obtained by a 0.22-μm hydrophilic filter and stored at 4°C until Se speciation analysis.
Instrumental analysis was conducted according to a previous study (31), with some modifications. The Se species were determined using a high-performance liquid chromatography (HPLC) system (U3000; Thermo Fisher Scientific, Waltham, MA, USA) equipped with a ZORBAX SB-Aq column (4.6 × 250 mm, particle size, 5 μm; Thermo Fisher Scientific). The flow rate of mobile phase (10 mM citric acid, 0.5 mM sodium 1-hexanesulfonate, 2% methanol, pH 5.5) was 0.8 ml/min. The outlet of the HPLC system was coupled to an ICP-MS instrument (X Series 2; Thermo Fisher Scientific; USA). The column outlet of the HPLC system was connected to a Micro-mist nebuliser using PEEK tubing (0.25 mm i.d. × 104 cm length).
Amino acids in samples were determined as previously reported (7) and are presented in the Supplementary Material. The protein subunit was analyzed by sodium dodecyl sulfate-polyacrylamide gel electrophoresis (SDS-PAGE) according to a previous study (26), with minor modifications. Then, 2 mg/ml soybean protein sample solution was first prepared, and the buffer was 12% separating gel and stacking gel. After being stained, gels were decolorised until the background was clear. The standard marker was a 10–1,000 kDa molecular weight protein.
Fourier transform infrared (FTIR) spectroscopy analysis of protein was performed according to a previous study (30). Approximately 1 mg dried sample was mixed with 100 mg KBr and pressed into a pellet. The pellet was analyzed by a Nicolet 5700 FTIR spectrometer (400–4,000 cm−1 wavenumber) with a 4 cm−1 resolution and an accumulation of 32 scans. Data were acquired and processed using Omnic 8.0 software (Thermo Fisher Scientific Inc., Madison, WI, USA) and Peakfit 4.12 (Systat Software, San Jose, CA, USA).
The WHC and OHC were determined according to a previous report (7). About 0.5 g of sample (W0) was placed into a centrifuge tube (25 ml) and then weighed (W1). Then, 10 ml water or oil was added, and the sample was allowed to sit for 30 min before centrifuging for 10 min (6,000 × g). The samples together with the centrifuge tube were weighed after removing the upper layer of water or oil (W2). WHC and OHC were calculated using the following equation:
FC and FS were assessed according to a previous report (32). A 30 ml sample of 1% (w/v) protein solution (pH 7.0) was homogenized in a mechanical homogeniser at 13,000 rpm for 3 min. FC was calculated using the following equation:
where V0 and V1 are the volumes before and after whipping, respectively, and V2 is the volume after standing for 30 min.
EAI and ESI were assessed according to a previous report (33) and calculated using Equations 4, 5. Aqueous emulsifier solution (9 ml, containing 1% protein) and 3 ml soybean oil were added into a tube and blended. Then, 20 μl of the emulsion was pipetted into 5 ml of 0.1% sodium dodecyl sulfate aqueous solution. Then, the absorbance was read at 500 nm at 0 (A0) and 30 (A30) min.
where T is 2.303, A0 and A30 are the absorbances at 0 and 30 min, respectively, N is 250, C is the initial protein concentration, and Φ is the volume fraction of the emulsion (0.2).
The 1,1-diphenyl-2-picrylhydrazyl (DPPH) radical-scavenging ability of 7S and 11S were analyzed according to a previous study (34). Briefly, 2.0 ml of a water solution of the samples at 1.0, 2.0, 3.0, 4.0, and 5.0 mg/ml was mixed with 2 ml alcoholic solution of DPPH (1.0 × 10−4 M) and then measured after the reaction. The DPPH radical-scavenging ability was calculated using the following equation:
where A0 is the absorbance of the deionised water; A1 and A2 are the absorbances of the sample with ethanol solution and DPPH, respectively.
The •OH radical-scavenging ability of 7S and 11S was analyzed according to a previous study (35). Briefly, 2.0 ml of a water solution of the samples at 1.0, 2.0, 3.0, 4.0, and 5.0 mg/ml were mixed with 1.0 ml of 9.0 mmol/L salicylic acid ethanol solution and 1.0 ml of 9.0 mmol/L FeSO4 and H2O2 aqueous solution and then measured after the reaction. The •OH radical-scavenging ability was calculated by the following equation:
where A0 is the absorbance of deionised water, and A1 and A2 are the absorbances of the sample with deionised water and H2O2, respectively.
Analysis of variance was performed followed by Duncan's test (p < 0.05) in the SPSS 19 software (IBM Corp., Armonk, NY, USA). For each tested group, the sample sizes in “amino acid concentration” are 2, and the other sample sizes are 3, respectively. Figures were drawn by Origin 2018 (OriginLab Inc., Northampton, MA, USA).
The total Se of soybean/sprout powder (SP), 7S, and 11S in RS, GS, and GS-Se with different concentrations of Se treatment is summarized in Table 1. There was an increase in total Se content, as the Se concentration in the solution increased from 5 to 60 mg/L; the total Se in SP, 7S, and 11S of GS-Se60 was 2,882, 2,035, and 4,301 μg/kg, and elevated by 79.8, 60.2, and 73.9 times, respectively, compared with the control group. The total Se in 11S was significantly higher than that in 7S by 69.6, 72.1, and 111% in RS, GS, and GS-Se30, respectively.
Table 1. Total selenium (Se) concentration in raw soybean (RS), germinated soybean (GS), and germinated soybean with Se biofortification (GS-Se) with different Se treatment concentrations (μg/kg).
Five Se species were separated and identified by HPLC-inductively coupled plasma mass spectrometry; the chromatograms are shown in Figure 1 (A, standard solution; B, sample). The retention times of standard SeCys2, MeSeCys, SeMet, selenite, and selenate were 2.694, 3.146, 3.832, 4.937, and 13.019 min, respectively, and the recoveries were calculated through the sum of different Se species to total Se ranging from 77.7 to 97.5%. Of the five Se species, SeMet and MeSeCys were the dominant Se species (Figure 2), accounting for 41.5–80.5 and 19.5–21.2% in GS-Se with different concentrations of Se treatment, respectively. However, SeCys2 was only found in the germinated soybean with Se treatment above 30 mg/L, and selenate was not found in any of the treatments. The proportion of organic Se (sum of SeCys2, MeSeCys, and SeMet) decreased from 100 to 67.8%, as the Se treatment increased from 0 to 60 mg/L, and the proportion in 7S and 11S was higher than that in SP; however, the percentage of organic Se in 7S and 11S was 76.2 ± 4.9% and 77.7 ± 6.0%, respectively. There was no significant difference in the proportion of organic Se between the two protein fractions at the same level of Se treatment. Furthermore, the concentrations of different species were all higher in 11S than those in 7S by 31.5–150%.
Figure 1. Chromatograms of different selenium (Se) species in standard solution (10 μg/L) (A) and Se-enriched germinated soybean sprouts (B). Selenocystine (1), methylselenocysteine (2), selenomethionine (3), selenite (4), and selenate (5).
Figure 2. Concentration (A) and proportions (B) of Se species in soybean and proteins under different treatments. Different letters are used to show significant differences at various treatments (p < 0.05).
The amino acid composition of soybean sprouts subjected to Se biofortification is listed in Table 2. The content of glutamic acid was the highest in all treatment groups (17.42–23.70%), followed by aspartic acid (10.71–12.41%), while the contents of cysteine (0.82–1.09%) and methionine were the lowest (0.72–1.45%). Isoleucine and leucine showed an increasing trend after germination, while most of the other amino acids increased slightly, without significant differences. The total amino acid content increased significantly after germination in both 7S and 11S. Se treatment had no significant effects on amino acids but decreased methionine in 11S. The amino acid content in 7S was significantly higher than that in 11S.
Table 2. Total amino acid concentration during soybean germination with different concentrations of Se treatment (g/100 g).
The subunits of different protein fractions (7S and 11S) from RS, GS, and GS-Se60 were analyzed by SDS-PAGE, and the results are shown in Figure 3. The molecular weight of 7S was mainly distributed at ~70, 40, 35, and 15 kDa, and the molecular weight of 11S was mainly distributed at ~37, 34, and 17 kDa. Both 7S and 11S shared a similar subunit composition in RS, GS, and GS-Se60, and there was no disappearance of the protein bands or appearance of new protein bands under Se treatment. Moreover, 7S and 11S were partially degraded into peptides with a low molecular weight due to germination, and the molecular weights of some proteins were lower than 15–25 kDa.
Figure 3. Sodium dodecyl sulfate-polyacrylamide gel electrophoresis patterns of different soybean proteins.
The percentages of α-helix, β-sheet, β-turn, and random coil secondary structures of different protein fractions determined by FTIR spectroscopy in all the samples are shown in Table 3. The order of the secondary structure of proteins in all treatments was as follows: β-sheet, β-turn, random coil, and α-helix. Compared to those in RS, germination increased secondary structural components of different proteins in α-helix and β-turn and decreased them in β-sheet and random coil in 7S. Conversely, germination increased secondary structural components of proteins in random coil and decreased them in α-helix, β-sheet, and β-turn in 11S compared with those in RS. Comparing the structural composition percentages in the control and Se biofortification samples, the α-helix decreased with an increase in the Se concentration, while the other structural compositions were not significantly different, and the secondary structures of 11S showed no significant differences between the control and Se biofortification samples.
The WHC and OHC of proteins from GS were significantly higher than those from RS, increasing by 18.17–20.28% in WHC and 10.40–27.32% in OHC, while Se treatment showed no significant effects on either WHC or OHC within the same treatment. The WHC of 7S and 11S from GS-Se60 was not significantly different; however, the OHC of 7S was significantly higher than that of 11S, increasing by 23.28–42.17% (Figures 4A,B). The FC of 7S increased with germination, and Se treatment promoted the FC of 7S (Figure 4C); however, there were no significant differences in 11S. Germination showed no significant effects on FS in both 7S and 11S (Figure 4D); the FS in 7S increased under Se treatment but showed no significant differences in 11S. The EAI of 7S and 11S decreased after germination, whereas Se biofortification had no significant effects on either 7S or 11S (Figure 4E). The ESI increased significantly under Se biofortification, whereas Se had no significant effects on 11S (Figure 4F).
Figure 4. Functional properties of different soybean proteins treated with or without Se. (A) Water holding capacity (WHC), (B) oil holding capacity (OHC), (C) foaming capacity (FC), (D) foam stability (FS), (E) emulsion activity index (EAI), and (F) emulsifying stability index (ESI). Different letters are used to show significant differences at various treatments (p < 0.05).
Germination promoted the ability of soybean protein to scavenge DPPH free radicals and hydroxyl radicals in both 7S and 11S, and the DPPH and hydroxyl radical-scavenging activities of the samples increased in a concentration-dependent manner (Figure 5). Se improved the ability of soybean protein to scavenge DPPH free radicals and hydroxyl radicals in 7S; however, this ability decreased in 11S. The order of DPPH scavenging ability among different proteins at 5 mg/ml was as follows: 11S-GS (51.8 ± 1.69), 7S-GS-Se60 (48.1 ± 0.99), 11S- GS-Se60 (40.1 ± 0.94), 7S-GS (37.0 ± 0.49), 11S-RS (28.3 ± 2.16), and 7S-RS (14.5 ± 0.85). The order of hydroxyl radical-scavenging ability among different proteins at 5 mg/ml was as follows: 11S-GS (35.74 ± 1.81), 7S-GS-Se60 (34.1 ± 1.40), 11S-GS-Se60 (34.08 ± 1.40), 11S-RS (33.18 ± 1.13), 7S-GS (30.1 ± 1.40), and 7S-RS (22.5 ± 1.13).
Figure 5. 1,1-Diphenyl-2-picrylhydrazyl (DPPH) radical scavenging activity (A) and hydroxyl free (·OH) radical scavenging ability (B).
The results indicated that germination in the presence of a selenite solution is an efficient method for Se biofortification of germinated soybeans, similar to a previous study (36) in which the Se concentration in soybean increased from 4.6 to 10,100 μg/kg. Se is beneficial at low levels but toxic at high levels, and the margin between deficiency and excess is narrow (13). To achieve health benefits, it has been suggested that Se intake should exceed the adult recommended dietary allowance of 50–60 μg/day and stay below the tolerable upper intake level of 400 μg/day (37). In our study, the concentration of total Se in GS-Se60 was 2,822 μg/kg, which was 79.8 times higher than that in RS. The ability of 11S to enrich Se was markedly higher than that of 7S in all treatment groups during germination. To the best of our knowledge, there has been no study investigating Se distribution in different germinated soybean proteins, and the results were consistent with those of a study on soybeans cultivated on farms. Deng et al. (7) found that the concentrations of Se in 11S are significantly higher (38.6%) than those in 7S; Wang et al. (38) also found that the concentration of Se in 11S is considerably higher (12.1%) than that in 7S in natural Se-enriched raw soybeans in Enshi, indicating that the 11S can bind Se more efficiently than 7S. However, Zhao et al. found that the Se content of 7S and 11S from Se biofortification soybeans is about 9.9-fold higher compared with ordinary soybeans, and there is no significant difference between 7S and 11S (25), which may be due to differences in the methods of soybean cultivation, extraction, and analysis. Above all, the ability of 11S to enrich Se in raw soybeans compared that of 7S was not greater than that of Se-enriched soybeans during germination, indicating that it is much easier to combine Se with 11S than with 7S during germination.
SeMet and MeSeCys were the dominant Se species in the germinated soybeans, indicating that selenite is easily converted to organic species during germination and can be efficiently incorporated into proteins (39). The decreased proportion of organic Se with increasing Se treatment concentrations was in line with a previous study, in which the proportion of organic Se in plants decreased from 88 to 80%, indicating that an increased concentration of Se in fertilizer will reduce the efficiency of conversion from inorganic to organic Se (30). Similar to the results for the total Se contents, the concentrations of different species were far higher in 11S than those in 7S. A plausible reason for this phenomenon is that S-containing amino acids in 7S were lower than those in 11S, and a previous study found that Se is incorporated into proteins mainly through taking the place of S in the S-containing amino acids (7). Previous studies also found that Se is mainly incorporated into proteins with low-molecular-weight compounds, such as in selenium-enriched mushrooms (not more than 16 kDa), soybean (15–20 kDa), Se-enriched rice (<36.3 kDa), and Se-enriched Tenebrio molitor larvae (<40 kDa) (11, 40–42). The contents of low-molecular-weight subunits in 11S were higher than those in 7S, and this may also be the reason for higher Se content in 11S.
Germination had no significant effects on most of the amino acids, with amino acid contents increasing slightly without significant differences; however, germination led to a significant increase in the total amino acids. Yang et al. found that the germination process leads to a significant decrease in some amino acids (23). However, Gao et al. found that germination increased the amino acid concentration of soybeans (22). The differences between the previous results and our work could be due to differences in the varieties of soybean and conditions during germination. Se treatment had no significant effect on amino acids but decreased methionine in 11S. A similar phenomenon has also been reported by Zhao et al. (25), who found that Se had no significant effect on the concentrations of most amino acids in raw soybean and only caused a reduction in concentrations of cysteine and methionine. This result could be due to Se taking the place of S in these two amino acids so that parts of methionine and cysteine are converted to SeMet and SeCys (43).
The subunits of 7S and 11S extracted from RS, GS, and GS-Se samples were analyzed by SDS-PAGE, and there were no new bands formed and existing bands did not disappear, indicating that Se biofortification did not make protein subunits degrade or aggregate. However, 7S and 11S were partially degraded into low-molecular-weight peptides due to germination, which is consistent with previous research (23, 44). Previous studies have found that 7S and 11S share the same bands between Se-enriched and ordinary raw soybeans (7, 11, 25). These studies indicate that the Se incorporated into proteins does not significantly affect the subunit composition of soybean proteins. However, Luo et al. (30) found that as Se fertilizer increases to 100 g/ha, the protein subunit of molecular weight of ~30 kDa moves upward, because the high Se content leads to protein subunit binding. The different phenomena observed in these studies may be due to the concentration of Se. A low concentration of Se does not change the protein subunit distribution, and a high concentration of Se can influence the molecular weight of proteins.
Based on the results detailed in Table 3, it can be concluded that Se influenced the secondary structures of proteins to some extent. Se is bound to amino acids and then incorporated into soybean proteins, which may influence the protein structure and also the secondary structure (17). It has been speculated that the decrease in the concentration of Cys and Met is caused by the replacement of S with Se in these two amino acids, both being hydrophobic (30). If Se converts S–S into Se–Se, indicating that the disulphide bond has changed, together with the atomic size and ionization of Se, then the secondary structure will be influenced (45). Zhao et al. (25) speculated that Se could influence the secondary structures of 7S and 11S in raw soybeans. However, Deng et al. (7) found a different phenomenon that Se has no significant effects on the secondary structure of 7S and 11S in raw soybean. It is possible that the interference of Se species with the contents of sulfhydryl groups and disulfide bonds is negligible because the Se content in the protein is much lower than that of S (30). Therefore, the specific reasons for the effect of Se on protein secondary structure need to be further investigated.
The improvement in protein WHC of 7S and 11S by germination was consistent with the results of SDS-PAGE experiments, in which 7S and 11S are hydrolysed by enzymes during germination (44), and the high WHC of proteins could be attributed to the high hydrophilicity of the soybean proteins (3). It seems that with the increased grade of hydrolysis, the protein may easily hold more water, and there is a higher WHC. The present study found that Se had no significant effect on the WHC and OHC, which is consistent with the study conducted by Deng et al. (7). However, Lazo-Velez et al. found that germinated soybeans enriched with Se promote WHC (46). The water-holding ability is an important functional property in food processing, such as in the preparation of sausages, pumped meats, and confections, as it increases product yields (47). Higher oil absorption can be useful for ingredients in meat, sausages, and dairy products, where the oil-holding ability affects the texture of the food (48).
Germination increased the FC of 7S and 11S and decreased the FS, which is consistent with previous studies (26, 49). This may be because the increased concentration of polypeptide, which is produced during soybean germination, could promote FC by incorporating more air (23). According to a previous study, a reduction in FS may be due to the low strength of micropeptides that maintain the stability of the foam (49). Therefore, the increased FC and decreased FS of GS in this study could be caused by the high solubility and low molecular weight of peptides. These low-molecular-weight peptides do not promote stable foams because of the reduced interactions between proteins (26, 50). The low FS is caused by weak interfacial surface films between bubbles, and the bubbles tend to collapse (51). The FC and FS of protein are important for processing cakes, whipped desserts, and ice cream (47). Interestingly, the 7S of GS-Se had both high FA and FS, and studies have shown that the FS depends on the rheological properties of the protein-membrane, as well as the protein-protein interactions and environmental factors (52). Thus, this result may be due to Se producing greater electrostatic repulsion, thus stabilizing the foams.
Previous studies have reported that the composition and structure of protein would influence emulsifying properties (53). In the present study, germination increased the EAI and ESI of 7S and 11S, and Se treatment increased EAI but decreased ESI. A similar phenomenon in germination was found in some previous studies, in which EAI and ESI were influenced by germination time (22, 44). Yang et al. found that germination can significantly increase the EAI of soybean protein but has no effect on ESI (23). Enzymatic hydrolysis could promote EAI and ESI by increasing the solubility of protein, which is important for emulsifying properties (26). A high solubility allows proteins to rapidly diffuse and adsorb at the interface between water and oil (3). Germination improved the EAI and ESI compared with those of raw soybean, which may also be caused by the increased solubility, so proteins can migrate to the interface rapidly (54). Overall, the changes in the protein content, amino acid composition, and protein structure might affect the functional properties of soybean proteins (17).
Previous studies have shown that germination can improve the antioxidant capacity of many products, such as soybean (55), kale, kohlrabi (56), and wheat (57), but these studies have mainly focused on the whole product powder; they attributed the improved antioxidant capacity to phenolics and flavonoids. This has also been verified by other studies, where higher antioxidant capacities are observed in germinated soybean mainly because of an increase in the concentration of total isoflavones (58, 59). Gao et al. found that soybean sprout protein effectively eliminates DPPH and •OH free radicals, showing that germination can strengthen the antioxidant activity of soy proteins (22). In the present study, we further investigated 7S and 11S, the two main protein fractions, and the antioxidant capacities of both proteins were improved through germination; the plausible reason is that germination can transform protein into small peptides, which have strong radical-scavenging abilities (60).
Selenium improved the ability of 7S to scavenge DPPH-free radicals and hydroxyl radicals in our study, which has also been found in previous studies (12, 61, 62); Se-containing proteins exhibit significantly higher antioxidant ability in vitro than proteins without Se (63). Se can promote both enzymatic and non-enzymatic antioxidant systems, such as GPx and glutathione (64). Se also increases some hydrophobic amino acid content in proteins, thereby enhancing the antioxidant capacity (40). A diselenide bridge, formed during the oxidation of two neighboring Cys residues, is longer than a disulfide bridge and has a lower redox potential (65). Therefore, a plausible reason is that 7S-containing Se–Se bonds tend to have higher antioxidant capacity than that containing S-S bonds.
However, Se had opposite effects on the antioxidant activities of 11S in our study, which cannot be reasonably explained, as the antioxidant mechanism of Se-enriched soy protein may be related to one or more of these mechanisms. Antioxidant properties are promoted with an increased concentration of Se in proteins (11). The antioxidant ability is affected not only by Se content and species but also by other factors, such as protein and amino acids (66), or by reducing sugars, ascorbic acid, and organic acids, among others, which may influence the evaluation of the antioxidant ability (64). Because the antioxidant activity is influenced by so many factors, the specific mechanism by which Se enhances the antioxidant activity of the protein is still uncertain, and the changes in antioxidant activity need to be further studied to clarify how Se affects the antioxidant activity. In addition, in vitro experiments are widely used to evaluate antioxidant capacity. However, these reactions are not just involved in the antioxidant enzymes in organisms, and evaluation methods should simulate real physiological conditions in organisms or involve tests using animal models.
SeMet and MeSeCys were the main Se species in the germinated soybeans, and dominant proteins 7S and 11S had different Se enrichment abilities. Se treatment had no significant effects on amino acids but decreased methionine in 11S. Moreover, the contents of α-helix decreased with increasing Se concentration, while the other structures were not significantly different. Se treatment had no significant effects on WHC and OHC but increased the FC and EAI of 7S, but only the EAI of 11S. Furthermore, Se treatment increased the antioxidant capacity in 7S but had no significant effects on that of 11S. The present study provides initial insight into the Se distribution in different germinated soybean dominant proteins and its effects on protein structure, functional properties, and antioxidant activity. The results provide important evidence for the development of efficient natural Se-enriched food supplements and the processing of Se-enriched germinated soybean protein.
The original contributions presented in the study are included in the article/Supplementary Material, further inquiries can be directed to the corresponding authors.
YH contributed to methodology, study design, and manuscript writing. NL, YX, and YL investigated the study. BF and LT contributed to data analysis. FW, CB, and PM contributed to study design, manuscript review, and funding acquisition. All authors have read and agreed to the published version of the manuscript.
This study was supported by the Special National Key Research and Development Plan (2021YFD1600101), the Quality and Safety of Agricultural Products (Risk Assessment) from the Chinese Ministry of Agriculture and Rural Affairs (Grant No. GJFP2019001), and the China Agriculture Research System (CARS-04).
The authors declare that the research was conducted in the absence of any commercial or financial relationships that could be construed as a potential conflict of interest.
All claims expressed in this article are solely those of the authors and do not necessarily represent those of their affiliated organizations, or those of the publisher, the editors and the reviewers. Any product that may be evaluated in this article, or claim that may be made by its manufacturer, is not guaranteed or endorsed by the publisher.
The Supplementary Material for this article can be found online at: https://www.frontiersin.org/articles/10.3389/fnut.2022.849928/full#supplementary-material
1. Day L. Proteins from land plants–potential resources for human nutrition and food security. Trends Food Sci Technol. (2013) 32:25–42. doi: 10.1016/j.tifs.2013.05.005
2. Patil G, Mian R, Vuong T, Pantalone V, Song Q, Chen P, et al. Molecular mapping and genomics of soybean seed protein: a review and perspective for the future. Theor Appl Genet. (2017) 130:1975–91. doi: 10.1007/s00122-017-2955-8
3. Makeri MU, Mohamed SA, Karim R, Ramakrishnan Y, Muhammad K. Fractionation, physicochemical, and structural characterization of winged bean seed protein fractions with reference to soybean. Int J Food Prop. (2017) 2017:1–17. doi: 10.1080/10942912.2017.1369101
4. Sha L, Liu S, Liu D. Effects of soybean protein isolate on protein structure, batter rheology, and water migration in emulsified sausage. J Food Proces Preserv. (2020) 44:14711. doi: 10.1111/jfpp.14711
5. Liang Y, Chen B, Li X, Dimler R. Fortification of concentrated milk protein beverages with soy proteins: impact of divalent cations and heating treatment on the physical stability. J Food Process Technol. (2018) 9:735. doi: 10.4172/2157-7110.1000735
6. Wang C, Yin H, Zhao Y, Zheng Y, Xu X, Yue J. Optimization of high hydrostatic pressure treatments on soybean protein isolate to improve its functionality and evaluation of its application in yogurt. Foods. (2021) 10:30667. doi: 10.3390/foods10030667
7. Deng X, Liao J, Zhao Z, Qin Y, Liu X. Distribution and speciation of selenium in soybean proteins and its effect on protein structure and functionality. Food Chem. (2021) 370:130982. doi: 10.1016/j.foodchem.2021.130982
8. Hatfield DL, Tsuji PA, Carlson BA, Gladyshev VN. Selenium and selenocysteine: roles in cancer, health, and development. Trends Biochem Sci. (2014) 39:112–20. doi: 10.1016/j.tibs.2013.12.007
9. Kipp AP, Strohm D, Brigelius-Flohe R, Schomburg L, Bechthold A, Leschik-Bonnet E, et al. Revised reference values for selenium intake. J Trace Elem Med Biol. (2015) 32:195–9. doi: 10.1016/j.jtemb.2015.07.005
10. Ingold I, Berndt C, Schmitt S, Doll S, Poschmann G, Buday K, et al. Selenium utilization by GPX4 is required to prevent hydroperoxide-induced ferroptosis. Cell. (2018) 172:409–22. e21. doi: 10.1016/j.cell.2017.11.048
11. Hu J, Zhao Q, Cheng X, Selomulya C, Bai C, Zhu X, et al. Antioxidant activities of Se-SPI produced from soybean as accumulation and biotransformation reactor of natural selenium. Food Chem. (2014) 146:531–7. doi: 10.1016/j.foodchem.2013.09.087
12. Zhao X, Gao J, Hogenkamp A, Knippels LM, Garssen J, Bai J, et al. Selenium-enriched soy protein has antioxidant potential via modulation of the NRF2-HO1 signaling pathway. Foods. (2021) 10:2542. doi: 10.3390/foods10112542
13. Li Y, Zhu N, Liang X, Zheng L, Zhang C, Li YF, et al. A comparative study on the accumulation, translocation and transformation of selenite, selenate, and SeNPs in a hydroponic-plant system. Ecotoxicol Environ Saf. (2020) 189:109955. doi: 10.1016/j.ecoenv.2019.109955
14. Umysova D, Vitova M, Douskova I, Bisova K, Hlavova M, Cizkova M, et al. Bioaccumulation and toxicity of selenium compounds in the green alga Scenedesmus quadricauda. BMC Plant Biol. (2009) 9:58. doi: 10.1186/1471-2229-9-58
15. Adadi P, Barakova NV, Muravyov KY, Krivoshapkina EF. Designing selenium functional foods and beverages: a review. Food Res Int. (2019) 120:708–25. doi: 10.1016/j.foodres.2018.11.029
16. Chan Q, Afton SE, Caruso JA. Selenium speciation profiles in selenite-enriched soybean (Glycine Max) by HPLC-ICPMS and ESI-ITMS. Metallomics. (2010) 2:147–53. doi: 10.1039/B916194E
17. Zhang X, He H, Xiang J, Yin H, Hou T. Selenium-containing proteins/peptides from plants: a review on the structures and functions. J Agric Food Chem. (2020) 68:15061–73. doi: 10.1021/acs.jafc.0c05594
18. Fukushima D. Recent progress of soybean protein foods: chemistry, technology, and nutrition. Food Rev Int. (1991) 7:323–51. doi: 10.1080/87559129109540915
19. Ju Q, Wu C, Yuan Y, Hu Y, Zhou S, Luan G. Insights into the mechanism on Glucono-delta-lactone induced gelation of soybean protein at subunit level. Food Hydrocolloids. (2022) 125:107402. doi: 10.1016/j.foodhyd.2021.107402
20. Nishinari K, Fang Y, Guo S, Phillips G. Soy proteins: a review on composition, aggregation and emulsification. Food Hydrocoll. (2014) 39:301–18. doi: 10.1016/j.foodhyd.2014.01.013
21. Hu M, Du X, Liu G, Zhang S, Wu H, Li Y. Germination improves the functional properties of soybean and enhances soymilk quality. Int J Food Sci Technol. (2021). doi: 10.1111/ijfs.15461
22. Gao C, Wang F, Yuan L, Liu J, Sun D, Li X. Physicochemical property, antioxidant activity, and cytoprotective effect of the germinated soybean proteins. Food Sci Nutr. (2019) 7:120–31. doi: 10.1002/fsn3.822
23. Yang H, Li X, Gao J, Tong P, Yang A, Chen H. Germination-assisted enzymatic hydrolysis can improve the quality of soybean protein. J Food Sci. (2017) 82:1814–9. doi: 10.1111/1750-3841.13782
24. Fang G, Lv Q, Liu C, Huo M, Wang S. An ionic liquid improved HPLC-ICP-MS method for simultaneous determination of arsenic and selenium species in animal/plant-derived foodstuffs. Analyt Methods. (2015) 7:8617–25. doi: 10.1039/C5AY02021B
25. Zhao X, Zhao Q, Chen H, Xiong H. Distribution and effects of natural selenium in soybean proteins and its protective role in soybean beta-conglycinin (7S globulins) under AAPH-induced oxidative stress. Food Chem. (2019) 272:201–9. doi: 10.1016/j.foodchem.2018.08.039
26. Di Y, Li X, Chang X, Gu R, Duan X, Liu F, et al. Impact of germination on structural, functional properties and in vitro protein digestibility of sesame (Sesamum indicum L.) protein. LWT. (2022) 154:112651. doi: 10.1016/j.lwt.2021.112651
27. Xu M, Jin Z, Peckrul A, Chen B. Pulse seed germination improves antioxidative activity of phenolic compounds in stripped soybean oil-in-water emulsions. Food Chem. (2018) 250:140–7. doi: 10.1016/j.foodchem.2018.01.049
28. Du M, Zhao L, Li C, Zhao G, Hu X. Purification and characterization of a novel fungi Se-containing protein from Se-enriched Ganoderma Lucidum mushroom and its Se-dependent radical scavenging activity. Eur Food Res Technol. (2007) 224:659–65. doi: 10.1007/s00217-006-0355-4
29. Zhang X, He H, Hou T. Molecular mechanisms of selenium-biofortified soybean protein and polyphenol conjugates in protecting mouse skin damaged by UV-B. Food Funct. (2020) 11:3563–73. doi: 10.1039/C9FO02560J
30. Luo L, Zhang J, Zhang K, Wen Q, Ming K, Xiong H, et al. Peanut selenium distribution, concentration, speciation, and effects on proteins after exogenous selenium biofortification. Food Chem. (2021) 354:129515. doi: 10.1016/j.foodchem.2021.129515
31. Zhang K, Guo X, Zhao Q, Han Y, Zhan T, Li Y, et al. Development and application of a HPLC-ICP-MS method to determine selenium speciation in muscle of pigs treated with different selenium supplements. Food Chem. (2020) 302:125371. doi: 10.1016/j.foodchem.2019.125371
32. Zhang H-J, Zhang H, Wang L, Guo X-N. Preparation and functional properties of rice bran proteins from heat-stabilized defatted rice bran. Food Res Int. (2012) 47:359–63. doi: 10.1016/j.foodres.2011.08.014
33. Zhang Q, Yang L, Hu S, Liu X, Duan X. Consequences of ball-milling treatment on the physicochemical, rheological and emulsifying properties of egg phosvitin. Food Hydrocoll. (2019) 95:418–25. doi: 10.1016/j.foodhyd.2019.04.064
34. Alam MN, Bristi NJ, Rafiquzzaman M. Review on in vivo and in vitro methods evaluation of antioxidant activity. Saudi Pharmaceut J. (2013) 21:143–52. doi: 10.1016/j.jsps.2012.05.002
35. Zeng R, Farooq MU, Zhang G, Tang Z, Zheng T, Su Y, et al. Dissecting the potential of selenoproteins extracted from selenium-enriched rice on physiological, biochemical and anti-ageing effects in vivo. Biol Trace Elem Res. (2020) 196:119–30. doi: 10.1007/s12011-019-01896-z
36. Deng B, Tian S, Li S, Guo M, Liu H, Li Y, et al. A simple, rapid and efficient method for essential element supplementation based on seed germination. Food Chem. (2020) 325:126827. doi: 10.1016/j.foodchem.2020.126827
37. Pyrzynska K, Sentkowska A. Selenium in plant foods: speciation analysis, bioavailability, and factors affecting composition. Crit Rev Food Sci Nutr. (2021) 61:1340–52. doi: 10.1080/10408398.2020.1758027
38. Wang Z, Xie S, Peng A. Distribution of Se in soybean samples with different Se concentration. J Agric Food Chem. (1996) 44:2754–9. doi: 10.1021/jf950133h
39. White PJ. Selenium metabolism in plants. Biochim Biophys Acta. (2018) 1862:2333–42. doi: 10.1016/j.bbagen.2018.05.006
40. Dong Z, Lin Y, Wu H, Zhang M. Selenium accumulation in protein fractions of Tenebrio molitor larvae and the antioxidant and immunoregulatory activity of protein hydrolysates. Food Chem. (2021) 334:127475. doi: 10.1016/j.foodchem.2020.127475
41. Liu K, Chen F, Zhao Y, Gu Z, Yang H. Selenium accumulation in protein fractions during germination of Se-enriched brown rice and molecular weights distribution of Se-containing proteins. Food Chem. (2011) 127:1526–31. doi: 10.1016/j.foodchem.2011.02.010
42. Zhang B, Zhou K, Zhang J, Chen Q, Liu G, Shang N, et al. Accumulation and species distribution of selenium in Se-enriched bacterial cells of the Bifidobacterium animalis 01. Food Chem. (2009) 115:727–34. doi: 10.1016/j.foodchem.2008.12.006
43. Wrobel K, Esperanza MG, Barrientos EY, Escobosa ARC, Wrobel K. Different approaches in metabolomic analysis of plants exposed to selenium: a comprehensive review. Acta Physiologiae Plantarum. (2020) 42:1–20. doi: 10.1007/s11738-020-03113-0
44. Aijie L, Shouwei Y, Li L. Structure, trypsin inhibitor activity and functional properties of germinated soybean protein isolate. Int J Food Sci Technol. (2014) 49:911–9. doi: 10.1111/ijfs.12386
45. Bañuelos GS, Walse SS, Yang SI, Pickering IJ, Fakra SC, Marcus MA, et al. Quantification, localization, and speciation of selenium in seeds of canola and two mustard species compared to seed-meals produced by hydraulic press. Anal Chem. (2012) 84:6024–30. doi: 10.1021/ac300813e
46. Lazo-Velez MA, Mata-Ramírez D, Serna-Saldivar SO, Chuck-Hernandez C. Functional effects of soybean concentrates obtained from sprouted seeds enriched in selenium in wheat breadmaking. Cereal Chem J. (2017) 94:740–5. doi: 10.1094/CCHEM-12-16-0295-R
47. De Wit J. Nutritional and functional characteristics of whey proteins in food products. J Dairy Sci. (1998) 81:597–608. doi: 10.3168/jds.S0022-0302(98)75613-9
48. Kaur M, Singh N. Characterization of protein isolates from different Indian chickpea (Cicer arietinum L.) cultivars. Food Chem=. (2007) 102:366–74. doi: 10.1016/j.foodchem.2006.05.029
49. Shahidi F, Han X-Q, Synowiecki J. Production and characteristics of protein hydrolysates from capelin (Mallotus villosus). Food Chem. (1995) 53:285–93. doi: 10.1016/0308-8146(95)93934-J
50. Hu Z, Tang X, Liu J, Zhu Z, Shao Y. Effect of parboiling on phytochemical content, antioxidant activity and physicochemical properties of germinated red rice. Food Chem. (2017) 214:285–92. doi: 10.1016/j.foodchem.2016.07.097
51. Adebowale K, Lawal O. Comparative study of the functional properties of bambarra groundnut (Voandzeia subterranean), jack bean (Canavalia ensiformis) and mucuna bean (Mucuna pruriens) flours. Food Res Int. (2004) 37:355–65. doi: 10.1016/j.foodres.2004.01.009
52. Vinayashree S, Vasu P. Biochemical, nutritional and functional properties of protein isolate and fractions from pumpkin (Cucurbita moschata var. Kashi Harit) seeds. Food Chem. (2021) 340:128177. doi: 10.1016/j.foodchem.2020.128177
53. Zamani Z, Razavi SM. Physicochemical, rheological and functional properties of Nettle seed (Urtica pilulifera) gum. Food Hydrocoll. (2021) 112:106304. doi: 10.1016/j.foodhyd.2020.106304
54. Nir I, Feldman Y, Aserin A, Garti N. Surface properties and emulsification behavior of denatured soy proteins. J Food Sci. (1994) 59:606–10. doi: 10.1111/j.1365-2621.1994.tb05573.x
55. Sun W-X, Zhang R-J, Fan J, He Y, Mao X-H. Comprehensive transformative profiling of nutritional and functional constituents during germination of soybean sprouts. J Food Measur Characterization. (2018) 12:1295–302. doi: 10.1007/s11694-018-9743-2
56. Zagrodzki P, Pasko P, Galanty A, Tyszka-Czochara M, Wietecha-Posluszny R, Rubio PS, et al. Does selenium fortification of kale and kohlrabi sprouts change significantly their biochemical and cytotoxic properties? J Trace Elem Med Biol. (2020) 59:126466. doi: 10.1016/j.jtemb.2020.126466
57. Islam MZ, Park BJ, Kang HM, Lee YT. Influence of selenium biofortification on the bioactive compounds and antioxidant activity of wheat microgreen extract. Food Chem. (2020) 309:125763. doi: 10.1016/j.foodchem.2019.125763
58. Guzmán-Ortiz FA, San Martín-Martínez E, Valverde ME, Rodríguez-Aza Y, Berríos JDJ, Mora-Escobedo R. Profile analysis and correlation across phenolic compounds, isoflavones and antioxidant capacity during germination of soybeans (Glycine max L) CyTA. J Food. (2017) 15:516–24. doi: 10.1080/19476337.2017.1302995
59. Lazo-Vélez MA, Guardado-Félix D, Avilés-González J, Romo-López I, Serna-Saldívar SO. Effect of germination with sodium selenite on the isoflavones and cellular antioxidant activity of soybean (Glycine max). LWT. (2018) 93:64–70. doi: 10.1016/j.lwt.2018.01.060
60. Beermann C, Euler M, Herzberg J, Stahl B. Anti-oxidative capacity of enzymatically released peptides from soybean protein isolate. Eur Food Res Technol. (2009) 229:637–44. doi: 10.1007/s00217-009-1093-1
61. Liu K, Zhao Y, Chen F, Fang Y. Purification and identification of Se-containing antioxidative peptides from enzymatic hydrolysates of Se-enriched brown rice protein. Food Chem. (2015) 187:424–30. doi: 10.1016/j.foodchem.2015.04.086
62. Zhang H, Chen T, Jiang J, Wong YS, Yang F, Zheng W. Selenium-containing allophycocyanin purified from selenium-enriched Spirulina platensis attenuates AAPH-induced oxidative stress in human erythrocytes through inhibition of ROS generation. J Agric Food Chem. (2011) 59:8683–90. doi: 10.1021/jf2019769
63. Ye Q, Wu X, Zhang X, Wang S. Organic selenium derived from chelation of soybean peptide-selenium and its functional properties: in vitro and in vivo. Food Function. (2019) 10:4761–70. doi: 10.1039/C9FO00729F
64. Gobi N, Vaseeharan B, Rekha R, Vijayakumar S, Faggio C. Bioaccumulation, cytotoxicity and oxidative stress of the acute exposure selenium in Oreochromis mossambicus. Ecotoxicol Environ Saf. (2018) 162:147–59. doi: 10.1016/j.ecoenv.2018.06.070
65. Besse D, Siedler F, Diercks T, Kessler H, Moroder L. The redox potential of selenocystine in unconstrained cyclic peptides. Angewandte Chemie Int. (1997) 36:883–5. doi: 10.1002/anie.199708831
Keywords: selenium biofortification, germinated soybean, protein structure, functional properties, antioxidant capacity
Citation: Huang Y, Fan B, Lei N, Xiong Y, Liu Y, Tong L, Wang F, Maesen P and Blecker C (2022) Selenium Biofortification of Soybean Sprouts: Effects of Selenium Enrichment on Proteins, Protein Structure, and Functional Properties. Front. Nutr. 9:849928. doi: 10.3389/fnut.2022.849928
Received: 06 January 2022; Accepted: 04 April 2022;
Published: 03 May 2022.
Edited by:
Zhi-Qing Lin, Southern Illinois University Edwardsville, United StatesReviewed by:
Baiyi Lu, Zhejiang University, ChinaCopyright © 2022 Huang, Fan, Lei, Xiong, Liu, Tong, Wang, Maesen and Blecker. This is an open-access article distributed under the terms of the Creative Commons Attribution License (CC BY). The use, distribution or reproduction in other forums is permitted, provided the original author(s) and the copyright owner(s) are credited and that the original publication in this journal is cited, in accordance with accepted academic practice. No use, distribution or reproduction is permitted which does not comply with these terms.
*Correspondence: Fengzhong Wang, d2FuZ2Zlbmd6aG9uZ0BzaW5hLmNvbQ==; Philippe Maesen, cGhpbGlwcGUubWFlc2VuQHVsaWVnZS5iZQ==; Christophe Blecker, Y2hyaXN0b3BoZS5ibGVja2VyQHVsaWVnZS5iZQ==
Disclaimer: All claims expressed in this article are solely those of the authors and do not necessarily represent those of their affiliated organizations, or those of the publisher, the editors and the reviewers. Any product that may be evaluated in this article or claim that may be made by its manufacturer is not guaranteed or endorsed by the publisher.
Research integrity at Frontiers
Learn more about the work of our research integrity team to safeguard the quality of each article we publish.