- 1Department of Chemical and Food Engineering, Federal University of Santa Catarina, Florianópolis, Brazil
- 2Department of Nutrition, Federal University of Santa Catarina, Florianópolis, Brazil
Protein from plant sources is claimed alternatives to animal sources in the human diet. Suitable protein sources need high protein digestibility and amino acid bioavailability. In terms of protein functionality and food applications, they also need high-quality attributes, such as solubility, gelling, water- and oil-holding capacities, emulsifying, and foaming. Thermal processing can improve the nutritional quality of plants with some disadvantages, like reducing the assimilation of micronutrients (vitamins and minerals). Emerging technologies—such as ultrasound, high-pressure, ohmic heating, microwave, pulsed electric field, cold plasma, and enzymatic processes—can overcome those disadvantages. Recent studies demonstrate their enormous potential to improve protein techno-functional properties, protein quality, and decrease protein allergenicity. However, the literature lacks a broader evaluation, including protein digestibility, industrial-scale optimization, and exploring applications to these alternative protein sources.
Introduction
Proteins are vital macronutrients in human nutrition, supplying the essential amino acids. It performs relevant functional roles in food formulation, processing, storage, and consumption. They also benefit food sensory and quality attributes, depending on their functional properties, such as solubility, gelling, water- and oil-holding capacities, emulsifying, and foaming (1, 2).
The main current challenges regarding protein food sources are supply and distribution guarantees. Regarding animal protein production and consumption, there are concerns about adverse impacts on human health, natural resource depletion, climate change, and animal welfare (3). These concerns lead to a constant growing adoption of vegetarian and vegan diets. Food security awareness for the increasing world population (about 10 billion by 2050) drives the demand for sustainable protein sources (4). The most prominent new protein sources are non-conventional plants (including agro-industrial by-products), fungi, algae, and insects (5, 6). Although food-grade insects are excellent protein sources, they are not widely accepted by consumers, mainly due to cultural aspects. Fungi and algae are limited in terms of supply. Thus, plant-based proteins keep drowning the most attention among others.
In general, thermal processing improves plant protein nutritional quality. However, some disadvantages are high time- and energy-consuming procedures, large water expenditure, and losses of desirable compounds in the final product (7). The main chemical changes produced by heating are degrading heat-labile micronutrients, reducing vitamins and minerals assimilation, and when the Maillard reaction occurs, generating toxic compounds and reducing the essential amino acids bioavailability (8). Elevated processing temperatures may also induce crosslinking, protein-protein interactions, and amino acid racemization (9).
Alternatively, some emerging food processing technologies have been investigated for the best protein employment, such as ultrasound, microwave, supercritical fluids, pulsed electric field, high-pressure, ohmic heating, cold plasma, and enzymatic processes (10, 11). Figure 1 illustrates the use of these techniques for valorizing plant-based proteins (11), which may contribute to environmental preservation by reducing wastewater production, organic solvents utilization, and processing time (12). Under mild temperatures, a balance can be reached between the processing feasibility with reduced environmental impact and the increased nutritional aspects and techno-functionalities of the proteins.
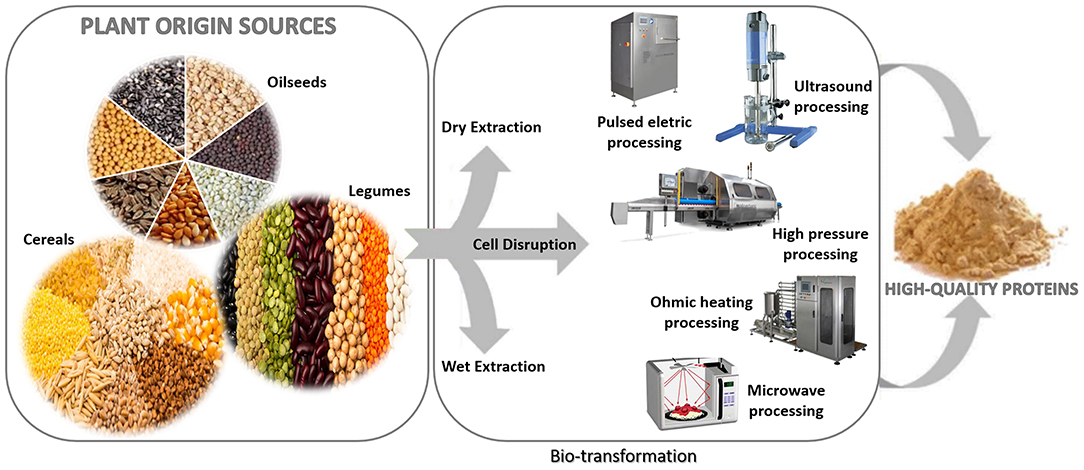
Figure 1. Emerging technologies for protein valorization of plant origin sources. Adapted from Pojić et al. (11).
Plants have been studied and used worldwide as protein sources, including legumes, cereals, pseudocereals, and seeds (6). However, plant-based proteins are negatively associated with a diminished nutritional quality due to minor components that impair protein bioavailability to the human body. These antinutritional factors are protease (trypsin) inhibitors, polyphenols (tannins), phytates, fibers, haemagglutinins, and non-starch polysaccharides (8). Thus, a proper plant protein processing selection may affect the digestibility and nutritional value by inactivating or eliminating these compounds, modifying the profile of bioactive peptides, and changing the protein structure (13). Besides, some plant proteins present food allergenicity, including nuts, wheat, and soybean (14). Those emerging technologies are also being investigated to create hypoallergenic products (15) due to changing the protein conformation and the IgE epitopes of the allergens, making them less available to antibody receptors, which declines the protein allergenicity, as well as increase the protein digestibility (16).
Therefore, this critical review aims to discuss the advances and perspectives of the emerging processing methods—non-thermal or performed at mild temperatures/short times—to improve plant protein quality and properties. It is focused on the nutritional aspects, protein digestibility, protein allergenicity, and techno-functional properties.
Techno-Functional Properties of Plant Proteins
Protein functionality is critical in determining the applicability of plant proteins flours, concentrates, and isolates (17, 18). The bio-functionality is related to protein physiological and nutritional properties (e.g., antioxidant and antibacterial activity) (11). On the other hand, techno-functionality is associated with the impact on the physicochemical characteristics of food products, affecting the texture, appearance, stability, emulsifying, solubility, foaming, gelling, water- and oil-holding capacities, cohesion-adhesion, elasticity, and viscosity (19).
Table 1 presents some protein techno-functional properties and their relationship with food sensory and physicochemical characteristics.
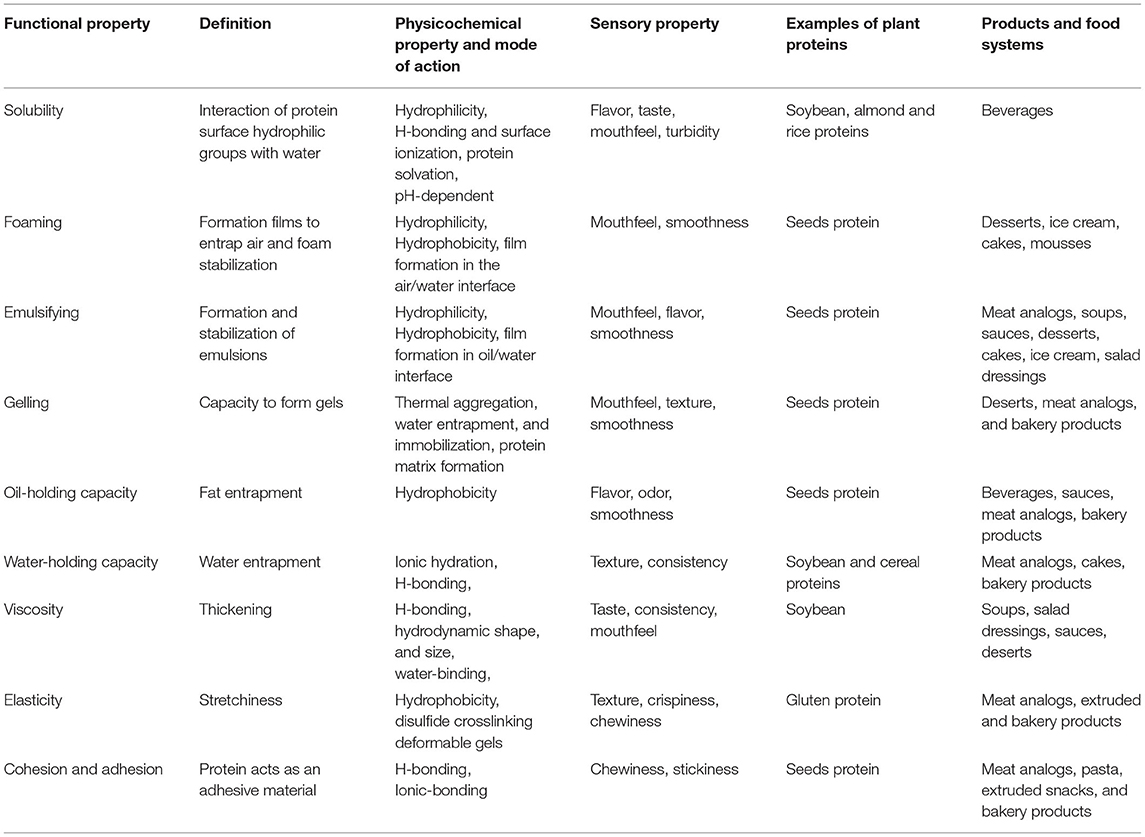
Table 1. Functional properties and their relationships with physicochemical and sensory properties of proteins (15, 49, 106, 107).
Food macromolecules (e.g., polysaccharides, lipids, and proteins) are inherently functional by their molecular structure and ability to interact and form complexes. Protein molecular structures have important roles in determining food functionality and can be used as targets to alter protein functionality (20). Intrinsic and environmental factors determine the functional properties, stability, and shelf-life of foods containing functional proteins. The main intrinsic factors are the protein structure, conformation, amino acid composition, surface functional groups, net and surface electric charge, and hydrophobicity/hydrophilicity. Extrinsic factors are the medium pH, salts and solvents, ionic strength, temperature, pressure, and shear stress (21, 22). Protein extraction and processing may change those functional properties. Thus, it is also essential to study the parameters setup impact on a diversity of functional and physicochemical properties of food products.
Most proteins are functional due to their globular component properties, especially solubility, which is attributed to the amphiphilicity of these molecules. Proteins have both inwardly bounded apolar (hydrophobic) amino acids and outwardly exposed polar (hydrophilic) side-chain amino acid residues. This arrangement allows dipole-dipole interactions with solvents by twisting and unfolding the amino acid side chains, placing the polar groups at the protein's surface. It leads to networks that can form gels and develop films, hold water, absorb fat, foam, emulsify, and dissolve under various pH conditions (20). Also, the relative amount of α-helices, random coils, and the α-helix/β-sheet ratio in protein secondary structures of soybean and corn meals were positively correlated with protein solubility, while the percentage of β-sheet structures was negatively correlated with this same ability (23).
Some studies assessed the techno-functional properties of plant proteins, such as soybean, chickpea, kidney bean (24), mung bean, pea (22, 25), cowpea, lentils (26), amaranth, quinoa (16), cashew nut (27), sorghum (28), avocado (29), and mustard (30). Few studies investigated these properties in edible oil processing by-products, such as rapeseed meal protein solubility (31, 32). The utilization of plant proteins is limited due to their extremely low solubility at neutral pH, except for the soybean, pea, canola (9), and cowpea (33).
Other studies also evaluated some plant proteins' foaming capacity and stability, like soybean, pea, chickpea, lupin, and rapeseed (34, 35). These sources have excellent foaming properties, comparable to egg protein, mostly due to high solubility, high surface hydrophobicity, low molecular weight, and net charge (36).
Some plant proteins have highlighted emulsifying properties, like the bell pepper, which formed stabilized emulsions with small oil droplet sizes (37); peas (34); chickpeas, with high emulsion activity index (EAI) at pH 10 (35); soybean, with a high emulsifying capability and emulsion stabilization against creaming during storage (38); and rapeseed, with higher emulsifying stabilities than soybean products (39).
Furthermore, few studies evaluated the water- and oil-holding capacities (WHC and OHC) of plant proteins. Bell peppers are suggested to food products requiring high WHC (37), while the OHC of peanut protein isolates was remarkably higher than commercial soybean protein isolates (40).
Although few studies evaluated the gelling properties of plant proteins, there are results about rapeseed products (flours, concentrates, and isolates) reporting poor gelation properties (41). However, soybean protein isolates have been used as gelling agents in several semi-solid food products, mainly for meat analogs (42).
Therefore, in terms of techno-functionality, there is little research reporting the solubility, emulsifying, foaming, water- and oil-holding capacities, and gelling properties for plant proteins. Potentially, plant-based proteins may be used by the food industry in formulations for protein supplements, meat analogs, beverages, snacks, desserts, bakery, whipped creams, soups, sauces, and salad dressings (22). From here, one can consider that exploring plant-based proteins aiming to develop technological alternatives for food formulation is an open field, including evaluating the required processing technologies for extraction and modulating thetechno-functionalities.
Emerging Technologies for Protein Valorization, Recovery, and Improvement of Protein Quality
The employability of plant proteins is related to the availability of their use, in addition to their intrinsic properties. The extraction yield from a food source is related to protein structure (primary to quaternary). Withal, the complexity of the food matrix influences the extraction process: proteins are generally bonded to other macromolecules (e.g., carbohydrates); the presence of salts and different pH values change proteins' charge, ionic strength, conformation, and solubility; previous matrix processing (e.g., defatting or heating) and the presence of water/solvents alters the matrix structure; and the fractionation of the plant sources (43–46). Also, the structural, functional, and sensory properties of extracted proteins are influenced by the conditions under which plant proteins are processed, e.g., temperature, time, pH, and ionic strength (44).
Protein extraction can be categorized into wet and dry methods (11). Subsequently to the extraction step, many technologies can be employed to purify or concentrate the protein of interest, aiming to obtain a food ingredient for different purposes. Figure 2 presents a diagram with the methods most used for recovering proteins from agri-food materials, which could be applied in a biorefinery concept.
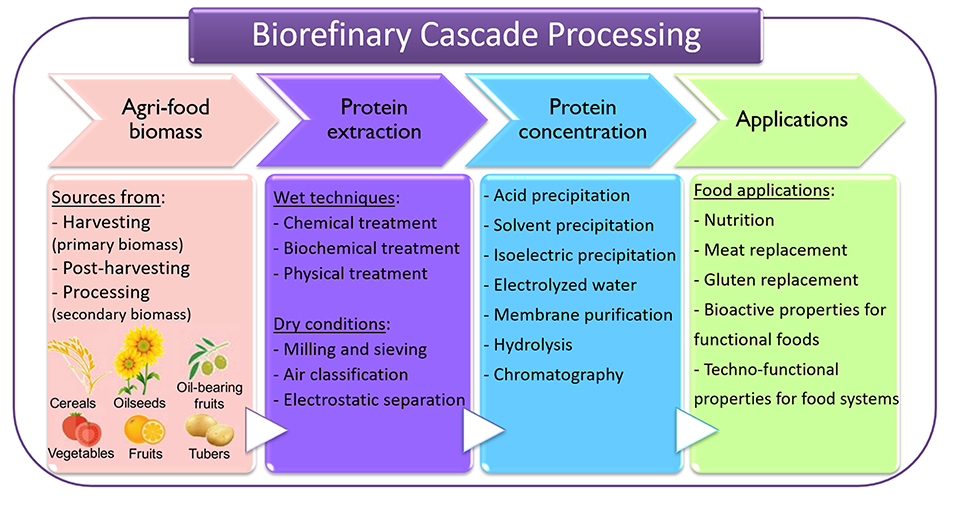
Figure 2. Flow diagram representing the extraction methods most used for recovering proteins from agri-food. Adapted from Contreras et al. (9).
The main consequence of many extraction methods is protein denaturation, which alters the proteins' secondary, tertiary, and quaternary structures due to changes in temperature, pH, and organic solvents and salts presence (47). In general, denaturation makes the protein more susceptible to digestive enzymes, improving protein digestibility (21).
Alkaline extraction (pH 8–11), followed by isoelectric precipitation (pH 4–5) of solubilized proteins, is the most usual technique employed for the extraction of plant proteins to make enriched flours (up to 65% of protein), protein concentrates (65–90%), and protein isolates (more than 90%) (48). Several studies used the alkaline technique to produce protein isolates of plants, such as seeds, cereals, and legumes (9). However, the enormous water, energy, and chemicals requirement is a significant drawback of alkaline extraction (19). Besides, high protein purity and yield are not guaranteed, which are affected by the processing conditions (e.g., equipment configuration, extraction time, temperature, pH, ionic strength, net charge, presence of salts, protein content, and protein solubility) (26, 49). Also, extreme extraction conditions (e.g., highly acid or alkaline medium and high temperature) may reduce protein nutritive value by changing the amino acid profile and degrading bioactive compounds (9, 11, 50). Furthermore, acid precipitation and neutralization tend to reduce protein solubility and negatively impact other techno-functional properties, such as gelling and foaming (51). Therefore, the alternative technique using membranes (e.g., ultrafiltration) is a less-energy consuming alternative to concentrate proteins instead of isoelectric precipitation, reaching the manufacture of protein ingredients (21, 26) and resulting in improved protein recovery yield, preserved techno-functional protein properties, and higher purity.
The existing drawbacks of conventional extraction methods (e.g., high temperature, energy consumption, wastewater, and organic solvents utilization) (19) may be overcome by emerging technologies using mild conditions. The most prominent alternatives are ultrasound, high-pressure, microwave, pulsed electric field, ohmic heating, and enzymatic processes. They potentially increase the protein extraction yield while reducing chemicals and water consumption (9–11, 50, 52, 53). However, a key question is whether these novel technologies can extract proteins from agri-food sources efficiently and cost-effectively. Data about the required energy and costs are scarce, and these approaches were mainly performed at a laboratory scale or are still in the early stage of their industrial applications; therefore, the large-scale feasibility still needs further studies.
Although these emerging processes were evaluated about protein valorization and recovery, studies concerning protein quality improvement (e.g., protein digestibility and inactivation of the so-called “antinutritional factors”) are rare for plant protein sources. Few evaluations have been made for the inactivating trypsin inhibitors in plant proteins in soybeans, chickpeas, and beans (54). However, as presented in the following, reasonable indications are that those methods are alternatives for plant-based protein processing.
High-Pressure Processing
High-pressure processing (HPP)—also known as high hydrostatic pressure (HHP) or high isostatic pressure (HIP)—is a non-thermal technology using hydrostatic pressures up to 1,000 MPa into a product in controlled temperature and time conditions (55). HPP affects the structure of the non-covalent bonds, increases the surface hydrophobicity, and causes protein denaturation, aggregation, or gelation (56). The protein secondary and tertiary structures significantly change at pressures higher than 200 MPa due to the consequent denaturation and aggregation of plant proteins with increased pressure, which changes the conformation and coagulation of their native structures because of the disruption of interactive forces, mainly hydrophobic and electrostatic bonds (57). HPP also improves protein functionality and digestibility of cereals and legumes (58), inactivating the antinutritional factors on a laboratory scale (59).
High-pressure homogenization (HPH)—also called dynamic high-pressure (DHP) or ultra-high pressure homogenization (UHPH)—imposes high-pressure conditions by pumping liquid food through a tiny gap in a valve, increasing velocity and causing high shear stresses. Consequently, it causes changes in food rheological properties. HPH utilizes the combined effectiveness of high-frequency vibration, high-velocity impact, quick pressure drop, cavitation, and intense shear stress in a short time, which causes a significant effect on proteins conformation (60). HPH was also recently applied to food products aiming at microbial inactivation and changes in the protein techno-functional properties (55).
Typical HPH pressures are moderate and usually up to 100 MPa (61), while HPP can reach ten times more. In addition to the range of applied pressures, another difference between HPP and HPH is the molecules' movements during treatment (lower in HPP and increased in HPH), leading to different protein structures after the treatments, bringing many interaction possibilities between polypeptides and protein aggregation. Also, HPP is governed by the ordering principle (33), whereas during HPH, high shear forces perturb protein structures (62).
Table 2 shows examples of high-pressure technology applied to plant proteins. The use of elevated pressure favors extracting protein from plants, decreasing solvent consumption, increasing extraction yields, and shortening the extraction time. High-pressure can modify protein techno-functional properties and reduce protein allergenicity (63, 64). HPP has also been used to extract proteins from some agri-food residues (e.g., wheat bran, grape pomace, and corn stover) (9), reduce food allergenicity, and inactivate some compounds detrimental to protein digestion.
Ultrasound
Ultrasound (US) is a non-thermal technology using high-intensity and low-frequency sound waves, ranging from 20 to 100 kHz (11). The basic principle of ultrasound technology is the cavitation phenomenon, where air bubbles are formed within the liquid phase, their volume increase, and finally explodes (19). US accelerates the mass transfer of compounds, provides high shear forces in the extractive agent (50), and improves solubility due to cellular structure's high stress and deformation (19). The mass transfer is also facilitated because microchannels may occur when bubbles collapse. The increased temperature, turbulence, and mixing effects by cavitation in the US also increase extraction efficiency (19). Consequently, the US can modify proteins by affecting H-bonds, increasing protein recovery, and reducing extraction time and protein aggregates (9). Also, improved protein functionality can occur. The cavitation bubbles on the protein surface result in micro-jetting and particle breakdown, improve solvent permeation into the food matrix and change the protein allergen conformation and reactivity (9, 11).
As a novel technology, the US appealed to environmental sustainability. High-intensity ultrasound is a quick and cost-effective technology used to modify globular proteins' structural and functional properties (25). The US was used to recover valuable proteins from food industry by-products, e.g., soybean okara (65, 66) and olive kernel (67). Table 2 summarizes the US technology application to plant proteins.
Pulsed Electric Field
Pulsed electric field (PEF) treatment consists of electric pulses of short duration (10−4 to 10−2 s) and relatively high amplitude (0.1–80 kV/cm). It induces a critical electrical potential across cell membranes, enabling an easier extraction of proteins (9, 11). PEF is a promising non-thermal food processing method that is efficient in cell disintegration (12) and microbial inactivation. PEF also induces changes in the protein hydrophobicity and structure (secondary and tertiary) and dissociates the non-covalent bondings, improving the protein functionality (68). PEF technology may cause lethal damage to cells or induce sub-lethal stress by transient permeabilization of cell membranes and electrophoretic movement of charged species between cellular compartments. Therefore, PEF application can facilitate the selective recovery of valuable compounds without deteriorating the treated matrix, favoring the subsequent separation and purification stages (69). Exposure of viable cells to PEF increases cell membrane permeability due to electroporation. This phenomenon can be used in biorefineries to extract and introduce molecules into the cells. The PEF application on plant cell tissues can positively change the membrane transport properties, facilitating the extraction of targeted molecules (e.g., proteins). Figure 3 schematically represents the impacts of PEF on any viable cells, where the possible outcomes depend on the PEF setup protocols (amplitude, number, shape, and pulses duration) and additional techniques (e.g., electrophoresis) (12).
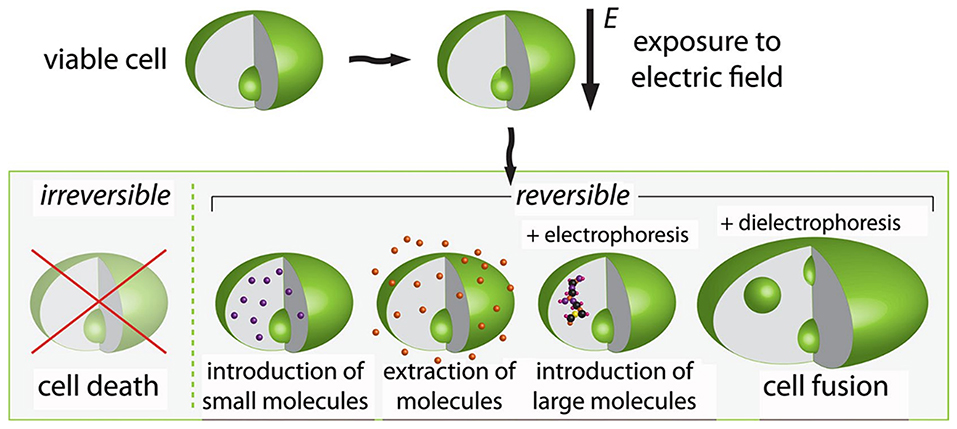
Figure 3. Schematic representation of the effect on cells exposed to pulsed electric fields. Adapted from Golberg et al. (12).
The PEF advantages are increased mass transfer, improved extraction yield, decreased processing time, reduced compounds degradation (e.g., flavors and proteins), and reduced energy costs (68). The application of PEF is a potential alternative to recover high-added value compounds from food matrixes and residues, which reduces waste disposal and extends limited resources. However, these studies were performed at the laboratory scale, and further investigations are needed to address the large-scale feasibility and energy costs.
Recent studies used PEF on the recovery of bioactive compounds from plants (e.g., betalain from red beet, beta-carotene from carrot, sucrose from sugar beetroot, inulin from chicory, and phenolics from grapes), agri-food residues, such as pomace, peels, kernels, and oilseed meals (e.g., carotenoids, chlorophylls, sterols, and polyphenols), and marine microalgae without killing the cells (12, 49, 70). However, the literature using PEF to improve protein digestibility and reduce antinutritional factors of plant proteins is scarce, and further research on this topic may be worthy. Table 2 presents applications of PEF to plant proteins.
Ohmic Heating
Ohmic heating (OH) is an advanced thermal processing method that applies electrical current to generate heat inside a food material by the well-known Joule effect (71). OH emerged as an alternative method to food thermal pasteurization and sterilization (11) and can promote higher yields and lower processing time than the conventional methods, bringing significant nutrient retention and preservation of the food quality (60). The existence of electrolytic components, such as salt and acids, allows the electric current to pass through food materials, which is the basis of the OH technique, generating heat internally (72). Besides heating, OH causes electroporation of cell membranes, increases the electrical conductivity and permeability, and positively influences the extraction rates of different biomolecules (11).
It was shown that OH inactivates trypsin inhibitors due to the electrochemical effects, depending on the electric voltage used. Studies using OH (50 Hz, 220 V, 3 min) showed more efficient inactivation of the trypsin inhibitors than the electric stove over the same processing time (59). The heat produced by the OH depends on the food material's electrical field strength and electrical conductivity. Low electrical conductivity heats slower than higher ones if the same electrical field strength is used. Food materials with 0.01–10 S/m of electrical conductivities are considered proper for the OH technique (73). OH was a pretreatment to soybean oil recovery, in which 600 V for 10 min promoted a 73% yield at 90°C (74). However, no studies using OH to assist protein extraction or improve plant proteins' functional properties and digestibility were found. Thus, there are opportunities for new applications for this emerging technology.
Microwave
Microwave heating (MH) uses non-ionizing electromagnetic waves from 300 MHz to 300 GHz (75). Microwaves cause heating due to the interaction of the alternating electromagnetic field with the food chemical constituents. MH of food materials occurs due to dipole and ionic mechanisms, and the dielectric properties and penetration depth are the most important characteristics affecting the process (76).
Microwave is mostly applied to foods for shorting cooking time, with less energy consumption. However, this technique can also influence the extraction of proteins (46) and other nutrients in food matrix (e.g., polyphenols and polysaccharides) (77). Moreover, the advanced MH use may improve the nutritional food quality by combining operating conditions that preserve more nutrients (e.g., vitamins and heat-labile amino acids like lysine, tryptophan, and sulfur amino acids) and sensory aspects (78–80). MH affects the conformational properties of food proteins (secondary structure) and accelerates their denaturation without changing their primary structure (60). The microwave processing disrupts H-bonds, increases the food matrix porosity, and allows dissolved ions migration. These food changes facilitate the extraction and the improvement of the protein functional properties (11). MH is also useful for inactivating the antinutritional factors usually present in plant proteins (59) and improves protein digestibility (8). Table 2 presents MH applications to plant proteins.
Literature shows some studies that use microwave heating to reduce the cooking time of plants, reduce the concentration of antinutritional factors, and enhance protein digestibility (8). However, there is scarce evidence of efficiently using this technology to extract proteins from plant sources and agro-industrial waste.
Cold Plasma
Plasma technology (or gas discharge plasma) involves producing and using ionized gas molecules to treat a material for superficial effects, e.g., polymers functionalization and food decontamination. The gas ionization produces reactive species, e.g., ions, electrons, excited atoms, ultraviolet (UV) photons, and free radicals (81–83), that can cause different changes on the food surfaces (solids food) and food bulk. Plasma can be generated at different temperatures and classified into thermal and non-thermal (also called cold) plasma (81, 84). Depending on the pressure condition, plasma can also be classified as low-, high-, or atmospheric-pressure plasma (85).
Cold atmospheric plasma processing (CAPP) is an emerging, sustainable, and environmentally friendly technology that has received significant attention in the food industry for the recent decade (82, 83, 86). CAPP can be generated by corona discharge, atmospheric-pressure plasma jet (APPJ), dielectric barrier discharge (DBD), microwave discharge (85, 87), and radiofrequency (RF) (88). It produces reactive oxygen species, including singlet oxygen and ozone, and exciting molecular nitrogen (81, 89). CAPP has an inactivation effect on microorganisms, such as food pathogens, spores, and viruses (84, 85, 89). Also, it is valid for surface modification (90, 91), inactivation of deteriorating enzymes (82), food packaging modification (85), and reduction of food allergenicity (83). According to processing conditions, CAPP influences food proteins' conformation and functional properties, e.g., plasma source, design, treatment power, pressure, reactive gas type, exposed time, and sample nature (84, 85).
Reactions initiated by reactive oxygen species (ROS) with the synergistic effect of reactive nitrogen species (RNS) are the leading cause of protein structure modification, triggering the cleavage of proteins into peptides (84). Also, CAPP can damage proteins, amino acids, nucleic acids, and lipids (81, 84, 89). The plasma protein denaturation mechanism is associated with reactive species interaction with amino acids and secondary structure by losing α-helix and β-sheet (85). Besides, it was observed that sulfur and aromatic amino acid content decreased after plasma exposure (84, 92).
There are few studies regarding CAPP effects on protein modification and the techno-functional properties of plant proteins and by-products. Table 2 brings some applications of CAPP technology to plant proteins present in the current literature. There is no published report on the extraction and protein quality enhancement of plant proteins in terms of protein digestibility by plasma technology. Also, no studies about the protein recovery of plant proteins from by-products using CAPP were found in the literature.
Enzymatic Processes
Enzymes are biocatalysts in many industries (e.g., food, chemical, and pharmaceutical). The enzyme-assisted extraction processing (EAEP) simultaneously extracts oil and protein from plants. EAEP has a low environmental impact due to avoiding organic solvents and can be labeled as an eco-friendly technique that produces valuable products in mild conditions without losing quality (50).
EAEP causes plant cell walls disruption by specific enzyme activity (e.g., proteases, cellulases, and pectinases). These enzymes enhance the extraction yield by decoupling proteins attached to the plant polysaccharide matrix (50). Proteases are the most used enzymes for EAEP and bring the highest protein recovery (9, 11).
Besides the high extraction yield, EAEP can also improve the functional properties of plant proteins, depending on extraction conditions, such as temperature, pH, ionic strength, and presence of salts (93). High-quality co-products (e.g., polyphenols and polysaccharides) from plants can also be obtained with good yields and preserved intrinsic qualities using EAEP (93). Table 2 shows examples of EAEP applied to plant proteins.
In addition to enzyme-assisted extraction, the enzymatic hydrolysis (EH) technique may generate protein hydrolysates, such as di- and tri-peptides (9), and bioactive peptides from plant proteins, which contribute to the production of bio-functional foods (94, 95). EH is better than conventional chemical hydrolysis (e.g., acid or alkali) due to high product quality, reduced processing time, and mild conditions (17).
The endopeptidases (e.g., Alcalase®) and the exopeptidases (e.g., Flavourzyme®) used in the EAEP process break down some peptide bonds (17, 96). The resulting carboxyl groups and free amino acids increase the isolated proteins' nutritional value, digestibility, and functional properties (9, 49, 97). EH decreases molecular weight, increases hydrophilicity, and changes protein conformation (17, 97).
Multi-enzyme cocktails may be a strategy to improve hydrolytic reactions (96). The enhanced functionality in protein hydrolysates depends on the extent of hydrolysis and process conditions (e.g., pH, temperature, and enzyme/substrate ratio) (17). The degree of hydrolysis (DH) is the percentage of peptide bonds cleaved per gram of protein over the total number of peptide bonds (98). DH is crucial for obtaining desirable results and avoiding excessive protein hydrolysis that can impair functionalities and sensory attributes (38, 98).
EH can result in hypoallergenic foods due to peptides' production that does not trigger IgE antibody binding activity, lowering protein allergenicity (99). High-quality protein hydrolysates have applications in food formulations destined for individuals with special diets (e.g., infants, elderly, allergic individuals, or medical nutrition) or those seeking a higher protein intake (e.g., athletes) (17, 49). However, there are some challenges regarding protein hydrolysate applications. The significant drawbacks of protein hydrolysates are their palatability and the bitter taste due to the release of bitter hydrophobic peptides (8). Table 2 shows examples of applications of enzymatic hydrolysis to plant proteins.
Other Emerging Technologies
Other processes commented in the literature allow the extraction and conformation changes, leading to high yield and improved protein quality. Examples are gamma irradiation (100), direct steam injection processing (DSI) (101), and refractance-window (RW) and cast-tape drying (CTD) (35).
DSI may lead to protein denaturation due to product exposure to high temperatures for short periods. DSI changes proteins' 3D conformation, like disulfide bonds and free S-H, and the protein surface becomes more hydrophilic, enhancing the functional properties (e.g., solubility) without affecting the essential amino acid composition (101).
CTD and RW are emerging techniques used for dehydrating liquid and semi-liquid foods (102, 103). The heat transfer occurs fastly to the product bottom by conduction (104). The processing time is relatively short, leading to minor changes in product nutritive value, conserving, or improving the protein functional properties (35).
Gamma irradiation (GI) may improve protein extraction and cause conformational changes (secondary structure rearrangement), crosslinking, and break covalent bonds. The protein structure and concentration and the presence of oxygen are the main factors affecting this process. However, GI may promote amino acid oxidation (8, 100).
Table 2 shows DSI, RW, and GI applications to plant proteins. There are rare studies on extracting plant proteins and evaluating their protein digestibility and techno-functional properties.
Combined Emerging Technologies
Some studies show the combined effect of some presented emerging technologies (e.g., US, HPP, MH, OH) with the enzymatic processes on enhancing protein recovery, extraction yield, and improving protein functional properties (10, 105). The improvement of protein extraction and functional properties results can be explained due to the synergistic effect of the mentioned technologies related to the increase of mass transfer and the influence of the conformational and protein structure changes, for example. Table 3 shows applications of combined processes on plant proteins. These studies showed combined techniques as more efficient in enhancing protein functional properties. Nevertheless, more research is required to validate their application to improve the nutritional quality of plant proteins.
Perspectives and Concluding Remarks
The emerging technologies for food processing are alternatives to traditional thermal processing to enhance plant proteins' nutritional quality or techno-functional properties. Protein quality is the main concern when replacing traditional sources, particularly meat by plants. It was observed that the selected processing technologies could interact with the protein structure (from primary to quaternary) and inactivate/eliminate the antinutritional factors, which indicate changes toward higher protein digestibility. This effect was demonstrated for high-pressure, ultrasound, microwave, ohmic heating, gamma irradiation, and enzymatic processes. In particular, trypsin inhibitors were inactivated by ultrasound, microwave, and ohmic heating, while gamma irradiation was suitable to inactivate the phytic acid. Protein allergenicity is also a concern for adding alternative protein sources in a diet, and it was demonstrated that enzymatic processes, cold plasma, and high-pressure were also efficient in reducing the allergenicity of these alternative proteins.
High-pressure processing is the most extensively explored process and presented many good results for techno-functional properties (foaming, emulsifying, and water- and oil-holding capacities), reducing allergenicity and antinutritional factors, and, as a consequence, improving plant protein digestibility and food applications. Those techno-functionality improvements result from changing the protein structure (from secondary to quaternary). Most of the emerging technologies discussed here can promote similar changes to the protein molecules, and, in this way, they are potential methods to impact those properties positively. In some studies, these properties were affected by ultrasound, pulsed electric field, microwave, cold plasma, direct steam injection, refractance-window drying, and enzymatic processes. In addition to the mentioned benefits, both ultrasound and enzymatic processes are suitable for increasing protein extraction yield and bioactive compounds recovery.
The promising results found about these technologies can be explored to nutritionally enrich traditional plant origin sources, such as soybean, peas, beans, and chickpeas. This work claims the broad perspective of these emerging technologies for achieving the adequate protein quality of plant origin sources. However, there are scarce studies evaluating protein digestibility and amino acid composition of plant proteins when using these emerging technologies. There is no published data on ohmic heating and cold plasma impact on plant protein quality enhancement or functional properties. Furthermore, combining some of these technologies has been indicated as a suitable strategy for better results, and many further investigations can be carried out to find optimal conditions.
Finally, the industrial application feasibility needs additional development at scales more extensive than those implemented at the laboratory. An actual industrial processing method must consider economic, environmental, and food security issues, which will supply sufficient proteins to the growing global population. Besides, the technological selection must meet human nutritional and sensory requirements and the consumer's cultural aspects. A key question is whether plant proteins can be extracted efficiently and cost-effectively and preserve nutritional value for human consumption worldwide.
Author Contributions
AS conceived and designed the work, performed the data search, figure and tables preparation, and wrote the draft. JL and YM revised the draft. BC conceived and designed the work, and revised the draft. All authors contributed to the article and approved the submitted version.
Funding
The authors thank the financial support from Brazilian Agencies CNPq (National Council for Scientific and Technological Development) and CAPES (Coordination for the Improvement of Higher Education Personnel—Financial code 001 and CAPES-PRINT Project 88887.310373/2018–00).
Conflict of Interest
The authors declare that the research was conducted in the absence of any commercial or financial relationships that could be construed as a potential conflict of interest.
Publisher's Note
All claims expressed in this article are solely those of the authors and do not necessarily represent those of their affiliated organizations, or those of the publisher, the editors and the reviewers. Any product that may be evaluated in this article, or claim that may be made by its manufacturer, is not guaranteed or endorsed by the publisher.
References
1. Jia W, Rodriguez-Alonso E, Bianeis M, Keppler JK, van der Goot AJ. Assessing functional properties of rapeseed protein concentrate versus isolate for food applications. Innov Food Sci Emerg Technol. (2021) 68:102636. doi: 10.1016/j.ifset.2021.102636
2. Ren Y, Yuan TZ, Chigwedere CM, Ai Y. A current review of structure, functional properties, and industrial applications of pulse starches for value-added utilization. Compr Rev Food Sci Food Saf . (2021) 20:1–32. doi: 10.1111/1541-4337.12735
3. Kumar M, Tomar M, Potkule J, Verma R, Punia S, Mahapatra A, et al. Advances in the plant protein extraction: mechanism and recommendations. Food Hydrocoll. (2021) 115:106595. doi: 10.1016/j.foodhyd.2021.106595
4. Boukid F, Rosell CM, Rosene S, Bover-Cid S, Castellari M. Non-animal proteins as cutting-edge ingredients to reformulate animal-free foodstuffs: present status and future perspectives. Crit Rev Food Sci Nutr. (2021) 0:1–31. doi: 10.1080/10408398.2021.1901649
5. Sá AGA, Silva DC, Pacheco MTB, Moreno YMF, Carciofi BAM. Oilseed by-products as plant-based protein sources: amino acid profile and digestibility. Futur Foods. (2021) 3:100023. doi: 10.1016/j.fufo.2021.100023
6. Sá AGA, Moreno YMF, Carciofi BAM. Plant proteins as high-quality nutritional source for human diet. Trends Food Sci Technol. (2020) 97:170–84. doi: 10.1016/j.tifs.2020.01.011
7. Córdova-Ramos JS, Glorio-Paulet P, Camarena F, Brandolini A, Hidalgo A. Andean lupin (Lupinus mutabilis Sweet): processing effects on chemical composition, heat damage, and in vitro protein digestibility. Cereal Chem. (2020) 97:827–35. doi: 10.1002/cche.10303
8. Sá AGA, Moreno YMF, Carciofi BAM. Food processing for the improvement of plant proteins digestibility. Crit Rev Food Sci Nutr. (2019) 0:1–20. doi: 10.1080/10408398.2019.1688249
9. Contreras M, Lama-Muñoz A, Gutiérrez-Pérez JM, Espínola F, Moya M, Castro E. Protein extraction from agri-food residues for integration in biorefinery: potential techniques and current status. Bioresour Technol. (2019) 280:459–77. doi: 10.1016/j.biortech.2019.02.040
10. Al-Ruwaih N, Ahmed J, Mulla MF, Arfat YA. High-pressure assisted enzymatic proteolysis of kidney beans protein isolates and characterization of hydrolysates by functional, structural, rheological and antioxidant properties. LWT Food Sci Technol. (2019) 100:231–6. doi: 10.1016/j.lwt.2018.10.074
11. Pojić M, Misan A, Tiwari B. Eco-innovative technologies for extraction of proteins for human consumption from renewable protein sources of plant origin. Trends Food Sci Technol. (2018) 75:93–104. doi: 10.1016/j.tifs.2018.03.010
12. Golberg A, Sack M, Teissie J, Pataro G, Pliquett U, Saulis G, et al. Energy-efficient biomass processing with pulsed electric fields for bioeconomy and sustainable development. Biotechnol Biofuels. (2016) 9:1–22. doi: 10.1186/s13068-016-0508-z
13. Popova A, Mihaylova D. Antinutrients in plant-based foods: a review. Open Biotechnol J. (2019) 13:68–76. doi: 10.2174/1874070701913010068
14. Fasolin LH, Pereira RN, Pinheiro AC, Martins JT, Andrade CCP, Ramos OL, et al. Emergent food proteins – Towards sustainability, health and innovation. Food Res Int. (2019) 125:1–16. doi: 10.1016/j.foodres.2019.108586
15. Nadathur SR, Wanasundara JPD, Scanlin L. Proteins in the diet: Challenges in feeding the global population. In: Nadathur SR, Wanasundara JPD, Scanlin L, editors. Sustainable Protein Sources. Amsterdam: Elsevier Inc. p. 1–20.
16. Ruiz GA. Exploring Novel Food Proteins and Processing Technologies: A Case Study on Quinoa Protein and High Pressure – High Temperature Processing. Wageningen: Wageningen University (2016).
17. Liceaga AM, Hall F. Nutritional, functional and bioactive protein hydrolysates. Encycl Food Chem. (2019) 3:456–64. doi: 10.1016/B978-0-08-100596-5.21776-9
18. Oliveira APH, Omura MH, Barbosa ÉAA, Bressan GC, Vieira ÉNR, Coimbra JSR, et al. Combined adjustment of pH and ultrasound treatments modify techno-functionalities of pea protein concentrates. Colloids Surfaces A Physicochem Eng Asp. (2020) 603: doi: 10.1016/j.colsurfa.2020.125156
19. Gençdag E, Görgüç A, Yilmaz FM. Recent advances in the recovery techniques of plant-based proteins from agro-industrial by-products. Food Rev Int. (2020) 37:1–22. doi: 10.1080/87559129.2019.1709203
20. Aryee ANA, Agyei D, Udenigwe CC. Impact of processing on the chemistry and functionality of food proteins. In: Yada RY, editor. Proteins in Food Processing: Second Edition. Sawston: Woodhead Publishing (2018).
21. Stone AK, Wang Y, Tulbek M, Nickerson MT. Plant protein ingredients. Encycl Food Chem. (2019) 1:229–34. doi: 10.1016/B978-0-08-100596-5.21601-6
22. Kyriakopoulou K, Dekkers B, van der Goot AJ. Plant-based meat analogues. In: Galanakis CM, editor. Sustainable Meat Production and Processing Amsterdam: Elsevier Inc. (2019). p. 103–26.
23. Bai M, Qin G, Sun Z, Long G. Relationship between molecular structure characteristics of feed proteins and protein in vitro digestibility and solubility. Asian Aust J Anim Sci. (2016) 29:1159–65. doi: 10.5713/ajas.15.0701
24. Byanju B, Rahman MM, Hojilla-Evangelista MP, Lamsal BP. Effect of high-power sonication pretreatment on extraction and some physicochemical properties of proteins from chickpea, kidney bean, and soybean. Int J Biol Macromol. (2020) 145:712–21. doi: 10.1016/j.ijbiomac.2019.12.118
25. Xiong T, Xiong W, Ge M, Xia J, Li B, Chen Y. Effect of high intensity ultrasound on structure and foaming properties of pea protein isolate. Food Res Int. (2018) 109:260–7. doi: 10.1016/j.foodres.2018.04.044
26. Samaranayaka A. Lentil: revival of poor man's meat. In: Nadathur SR, Wanasundara JPD, Scanlin L, editors. Sustainable Protein Sources. Amsterdam: Elsevier Inc. (2016). p. 185–96.
27. Liu C, Peng Q, Zhong J, Liu W, Zhong Y, Wang F. Molecular and functional properties of protein fractions and isolate from cashew nut (Anacardium occidentale L.). Molecules. (2018) 23:1–15. doi: 10.3390/molecules23020393
28. Bernardo CO, Ascheri JLR, Carvalho CWP, Chávez DWH, Martins IBA, Deliza R, et al. Impact of extruded sorghum genotypes on the rehydration and sensory properties of soluble beverages and the Brazilian consumers ' perception of sorghum and cereal beverage using word association. J Cereal Sci. (2019) 89:102793. doi: 10.1016/j.jcs.2019.102793
29. Wang J, Wang A, Zang X, Tan L, Xu B, Chen H, et al. Physicochemical, functional and emulsion properties of edible protein from avocado (Persea americana Mill.) oil processing by-products. Food Chem. (2019) 288:146–53. doi: 10.1016/j.foodchem.2019.02.098
30. Chakraborty P, Bhattacharyya DK, Ghosh M. Extrusion treated meal concentrates of Brassica juncea as functionally improved ingredient in protein and fiber rich breadstick preparation. LWT - Food Sci Technol. (2021) 142:111039. doi: 10.1016/j.lwt.2021.111039
31. Zhang B, Liu G, Ying D, Sanguansri L, Augustin MA. Effect of extrusion conditions on the physico-chemical properties and in vitro protein digestibility of canola meal. Food Res Int. (2017) 100:658–64. doi: 10.1016/j.foodres.2017.07.060
32. Campbell L, Rempel CB, Wanasundara JPD. Canola/Rapessed protein: future opportunities and directions - Workshop proceedings of IRC 2015. Plants. (2016) 5:1–7. doi: 10.3390/plants5020017
33. Peyrano F, Lamballerie M, Avanza M V., Speroni F. Gelation of cowpea proteins induced by high hydrostatic pressure. Food Hydrocoll. (2021) 111:106191. doi: 10.1016/j.foodhyd.2020.106191
34. Barac MB, Pesic MB, Stanojevic S, Kostic AZ, Bivolarevic V. Comparative study of the functional properties of three legume seed isolates: adzuki, pea and soybean. J Food Sci. (2015) 52:2779–87. doi: 10.1007/s13197-014-1298-6
35. Tontul I, Kasimoglu Z, Asik S, Atbakan T, Topuz A. Functional properties of chickpea protein isolates dried by refractance window drying. Int J Biol Macromol. (2018) 109:1253–9. doi: 10.1016/j.ijbiomac.2017.11.135
36. Sun-Waterhouse D, Zhao M, Waterhouse GIN. Protein modification during ingredient preparation and food processing: approaches to improve food processability and nutrition. Food Bioprocess Technol. (2014) 7:1853–93. doi: 10.1007/s11947-014-1326-6
37. Li M, Wen X, Peng Y, Wang Y, Wang K, Ni Y. Functional properties of protein isolates from bell pepper (Capsicum annuum L. var. annuum) seeds. LWT Food Sci Technol. (2018) 97:802–10. doi: 10.1016/j.lwt.2018.07.069
38. Chen L, Chen J, Ren J, Zhao M. Effects of ultrasound pretreatment on the enzymatic hydrolysis of soy protein isolates and on the emulsifying properties of hydrolysates. J Agric Food Chem. (2011) 59:2600–9. doi: 10.1021/jf103771x
39. Aider M, Barbana C. Canola proteins: composition, extraction, functional properties, bioactivity, applications as a food ingredient and allergenicity - a practical and critical review. Trends Food Sci Technol. (2011) 22:21–39. doi: 10.1016/j.tifs.2010.11.002
40. He X, Liu H, Liu L, Zhao G, Wang Q, Chen Q. Effects of high pressure on the physicochemical and functional properties of peanut protein isolates. Food Hydrocoll. (2014) 36:123–9. doi: 10.1016/j.foodhyd.2013.08.031
41. Tan SH, Mailer RJ, Blanchard CL, Agboola SO. Canola proteins for human consumption: extraction, profile, and functional properties. J Food Sci. (2011) 76:R16–28. doi: 10.1111/j.1750-3841.2010.01930.x
42. Bessada SMF, Barreira JCM, Oliveira MBPP. Pulses and food security: dietary protein, digestibility, bioactive and functional properties. Trends Food Sci Technol. (2019) 93:53–68. doi: 10.1016/j.tifs.2019.08.022
43. Rezig L, Chibani F, Chouaibi M, Dalgalarrondo M, Hessini K, Guéguen J, et al. Pumpkin (cucurbita maxima) seed proteins: sequential extraction processing and fraction characterization. J Agric Food Chem. (2013) 61:7715–21. doi: 10.1021/jf402323u
44. Gao Z, Shen P, Lan Y, Cui L, Ohm JB, Chen B, et al. Effect of alkaline extraction pH on structure properties, solubility, and beany flavor of yellow pea protein isolate. Food Res Int. (2020) 131:109045. doi: 10.1016/j.foodres.2020.109045
45. Esaka M, Suzuki K, Kubota K. Inactivation of lipoxygenase and trypsin inhibitor in soybeans on microwave irradiation. Agric Biol Chem. (1986) 50:2395–6. doi: 10.1080/00021369.1986.10867755
46. Wen L, Álvarez C, Zhang Z, Poojary MM, Lund MN, Sun D, Tiwari BK. Optimisation and characterisation of protein extraction from coffee silverskin assisted by ultrasound or microwave techniques. Biomass Convers Biorefinery. (2020) 11:1575–85. doi: 10.1007/s13399-020-00712-2
47. Tanger C, Engel J, Kulozik U. Influence of extraction conditions on the conformational alteration of pea protein extracted from pea flour. Food Hydrocoll. (2020) 107:1–15. doi: 10.1016/j.foodhyd.2020.105949
48. Xing Q, Utami DP, Demattey MB, Kyriakopoulou K, de Wit M, Boom RM, et al. A two-step air classification and electrostatic separation process for protein enrichment of starch-containing legumes. Innov Food Sci Emerg Technol. (2020) 66:102480. doi: 10.1016/j.ifset.2020.102480
49. Voudouris P, Tenorio AT, Lesschen JP, Mulder WJ, Kyriakopoulou K, Sanders JPM, et al. Sustainable protein technology: an evaluation on the STW protein programme and an outlook for the future. Sustainable Protein. (2017) 1786:1–60. doi: 10.18174/429443
50. Görgüç A, Bircan C, Yilmaz FM. Sesame bran as an unexploited by-product: effect of enzyme and ultrasound-assisted extraction on the recovery of protein and antioxidant compounds. Food Chem. (2019) 283:637–45. doi: 10.1016/j.foodchem.2019.01.077
51. Berghout JAM, Venema P, Boom RM, van der Goot AJ. Comparing functional properties of concentrated protein isolates with freeze-dried protein isolates from lupin seeds. Food Hydrocoll. (2015) 51:346–54. doi: 10.1016/j.foodhyd.2015.05.017
52. Júnior LM, Anjos CAR. Effect of high-pressure processing on characteristics of flexible packaging for foods and beverages. Food Res Int. (2018) 119:1–11. doi: 10.1016/j.foodres.2018.10.078
53. Khanra S, Mondal M, Halder G, Tiwari ON, Gayen K, Bhowmick TK. Downstream processing of microalgae for pigments, protein and carbohydrate in industrial application: a review. Food Bioprod Process. (2018) 110:60–84. doi: 10.1016/j.fbp.2018.02.002
54. Vagadia BH, Vanga SK, Singh A, Gariepy Y, Raghavan V. Comparison of conventional and microwave treatment on soymilk for inactivation of trypsin inhibitors and in vitro protein digestibility. Foods. (2018) 7:1–14. doi: 10.3390/foods7010006
55. Júnior BRCL, Tribst AAL, Cristianini M. Effect of high-pressure Technologies on enzymes applied in food processing. In: Şentürk M, editor. Enzyme Inhibitors and Activators. London: IntechOpen (2017). p. 49–72.
56. Zhao Z, Mu T, Zhang M, Richel A. Effects of sulfur-containing amino acids and high hydrostatic pressure on structure and gelation properties of sweet potato protein. Food Bioprocess Technol. (2019) 12:1863–73. doi: 10.1007/s11947-019-02343-6
57. Gharibzahedi SMT, Smith B. Effects of high hydrostatic pressure on the quality and functionality of protein isolates, concentrates, and hydrolysates derived from pulse legumes: a review. Trends Food Sci Technol. (2021) 107:466–79. doi: 10.1016/j.tifs.2020.11.016
58. Belmiro RH, Artigiani A, Tribst L, Cristianini M. Impact of high pressure in hydration and drying curves of common beans (Phaseolus vulgaris L.). Innov Food Sci Emerg Technol. (2018) 28:279–85. doi: 10.1016/j.ifset.2018.03.013
59. Vagadia BH, Vanga SK, Raghavan V. Inactivation methods of soybean trypsin inhibitor - a review. Trends Food Sci Technol. (2017) 64:115–25. doi: 10.1016/j.tifs.2017.02.003
60. Leite TS, Sastry SK, Cristianini M. Effect of concentration and consistency on ohmic heating. J Food Process Eng. (2018) 41:1–9. doi: 10.1111/jfpe.12883
61. Kubo MTK, Augusto PED, Cristianini M. Effect of high pressure homogenization (HPH) on the physical stability of tomato juice. Food Res Int. (2013) 51:170–9. doi: 10.1016/j.foodres.2012.12.004
62. Keerati-U-Rai M, Corredig M. Effect of dynamic high pressure homogenization on the aggregation state of soy protein. J Agric Food Chem. (2009) 57:3556–62. doi: 10.1021/jf803562q
63. Mirmoghtadaie L, Aliabadi SS, Hosseini SM. Recent approaches in physical modification of protein functionality. Food Chem. (2016) 199:619–27. doi: 10.1016/j.foodchem.2015.12.067
64. Li H, Zhu K, Zhou H, Peng W, Guo X. Comparative study of four physical approaches about allergenicity of soybean protein isolate for infant formula. Food Agric Immunol. (2016) 27:604–23. doi: 10.1080/09540105.2015.1129602
65. Preece KE, Hooshyar N, Krijgsman AJ, Fryer PJ, Zuidam NJ. Intensification of protein extraction from soybean processing materials using hydrodynamic cavitation. Innov Food Sci Emerg Technol. (2017) 41:47–55. doi: 10.1016/j.ifset.2017.01.002
66. Preece KE, Hooshyar N, Krijgsman A, Fryer PJ, Zuidam NJ. Intensified soy protein extraction by ultrasound. Chem Eng Process Process Intensif. (2016) 113:94–101. doi: 10.1016/j.cep.2016.09.003
67. Roselló-Soto E, Barba FJ, Parniakov O, Galanakis CM, Lebovka N, Grimi N, et al. High voltage electrical discharges, pulsed electric field, and ultrasound assisted extraction of protein and phenolic compounds from olive kernel. Food Bioprocess Technol. (2015) 8:885–94. doi: 10.1007/s11947-014-1456-x
68. Melchior S, Calligaris S, Bisson G, Manzocco L. Understanding the impact of moderate-intensity pulsed electric fields (MIPEF) on structural and functional characteristics of pea, rice and gluten concentrates. Food Bioprocess Technol. (2020) 13:2145–55. doi: 10.1007/s11947-020-02554-2
69. Barba FJ, Galanakis CM, Esteve MJ, Frigola A, Vorobiev E. Potential use of pulsed electric technologies and ultrasounds to improve the recovery of high-added value compounds from blackberries. J Food Eng. (2015) 167:38–44. doi: 10.1016/j.jfoodeng.2015.02.001
70. Barba FJ, Parniakov O, Pereira A, Wiktor A, Grimi N, Boussetta N, et al. Current applications and new opportunities for the use of pulsed electric fields in food science and industry. Food Res Int. (2015) 77:773–98. doi: 10.1016/j.foodres.2015.09.015
71. Nazari B, Mohammadifar MA, Shojaee-Aliabadi S, Feizollahi E, Mirmoghtadaie L. Effect of ultrasound treatments on functional properties and structure of millet protein concentrate. Ultrason Sonochem. (2018) 41:382–8. doi: 10.1016/j.ultsonch.2017.10.002
72. Ramaswamy HS, Marcotte M, Sastry S, Abdelrahim K. Ohmic Heating in Food Processing. Boca Raton, FL: CRC Press (2014).
73. Jittanit W, Khuenpet K, Kaewsri P, Dumrongpongpaiboon N, Hayamin P, Jantarangsri K. Ohmic heating for cooking rice: electrical conductivity measurements, textural quality determination and energy analysis. Innov Food Sci Emerg Technol. (2017) 42:16–24. doi: 10.1016/j.ifset.2017.05.008
74. Pare A, Nema A, Singh VK, Mandhyan BL. Combined effect of ohmic heating and enzyme assisted aqueous extraction process on soy oil recovery. J Food Sci Technol. (2014) 51:1606–11. doi: 10.1007/s13197-012-0685-0
75. Datta AK, Anastheswaran RC. Handbook of Microwave Technology for Food Applications. 1st ed. New York, NY: CRC Press (2001).
76. Divekar MT, Karunakaran C, Lahlali R, Kumar S, Chelladurai V, Liu X, et al. Effect of microwave treatment on the cooking and macronutrient qualities of pulses. Int J Food Prop. (2017) 20:409–22. doi: 10.1080/10942912.2016.1163578
77. He Y, Lu Q, Liviu G. Effects of extraction processes on the antioxidant activity of apple polyphenols. CYTA J Food. (2015) 13:603–6. doi: 10.1080/19476337.2015.1026403
78. Monteiro RL, Moraes JO, Domingos JD, Carciofi BAM, Laurindo JB. Evolution of the physicochemical properties of oil-free sweet potato chips during microwave vacuum drying. Innov Food Sci Emerg Technol. (2020) 63:102317. doi: 10.1016/j.ifset.2020.102317
79. Monteiro RL, Link J V., Tribuzi G, Carciofi BAM, Laurindo JB. Microwave vacuum drying and multi-flash drying of pumpkin slices. J Food Eng. (2018) 232:1–10. doi: 10.1016/j.jfoodeng.2018.03.015
80. Barreto IMA, Tribuzi G, Marsaioli Junior A, Carciofi BAM, Laurindo JB. Oil–free potato chips produced by microwave multiflash drying. J Food Eng. (2019) 261:133–9. doi: 10.1016/j.jfoodeng.2019.05.033
81. Sharifian A, Soltanizadeh N, Abbaszadeh R. Effects of dielectric barrier discharge plasma on the physicochemical and functional properties of myofibrillar proteins. Innov Food Sci Emerg Technol. (2019) 54:1–8. doi: 10.1016/j.ifset.2019.03.006
82. Han Y, Cheng J, Sun D. Changes in activity, structure and morphology of horseradish peroxidase induced by cold plasma. Food Chem. (2019) 301:125240. doi: 10.1016/j.foodchem.2019.125240
83. Zhang Q, Cheng Z, Zhang J, Nasiru MM, Wang Y, Fu L. Atmospheric cold plasma treatment of soybean protein isolate: insights into the structural, physicochemical, and allergenic characteristics. J Food Sci. (2021) 86:68–77. doi: 10.1111/1750-3841.15556
84. Tolouie H, Mohammadifar MA, Ghomi H, Hashemi M. Cold atmospheric plasma manipulation of proteins in food systems. Crit Rev Food Sci Nutr. (2018) 58:2583–97. doi: 10.1080/10408398.2017.1335689
85. Pankaj SK, Wan Z, Keener KM. Effects of cold plasma on food quality: a review. Foods. (2018) 7:1–21. doi: 10.3390/foods7010004
86. Chaple S, Sarangapani C, Jones J, Carey E, Causeret L, Genson A, et al. Effect of atmospheric cold plasma on the functional properties of whole wheat (Triticum aestivum L.) grain and wheat flour. Innov Food Sci Emerg Technol. (2020) 66:102529. doi: 10.1016/j.ifset.2020.102529
87. Misra NN, Pankaj SK, Segat A, Ishikawa K. Cold plasma interactions with enzymes in foods and model systems. Trends Food Sci Technol. (2016) 55:39–47. doi: 10.1016/j.tifs.2016.07.001
88. Bußler S, Steins V, Ehlbeck J, Schlüter O. Impact of thermal treatment versus cold atmospheric plasma processing on the techno-functional protein properties from Pisum sativum “Salamanca.” J Food Eng. (2015) 167:166–74. doi: 10.1016/j.jfoodeng.2015.05.036
89. Yang R, Liu Y, Meng D, Wang D, Blanchard CL, Zhou Z. Effect of atmospheric cold plasma on structure, activity, and reversible assembly of the phytoferritin. Food Chem. (2018) 264:41–8. doi: 10.1016/j.foodchem.2018.04.049
90. Pérez-Andrés JM, Álvarez C, Cullen PJ, Tiwari BK. Effect of cold plasma on the techno-functional properties of animal protein food ingredients. Innov Food Sci Emerg Technol. (2019) 102205:1–7. doi: 10.1016/j.ifset.2019.102205
91. Heidemann HM, Dotto MER, Laurindo JB, Carciofi BAM, Costa C. Cold plasma treatment to improve the adhesion of cassava starch films onto PCL and PLA surface. Colloids Surfaces A Physicochem Eng Asp. (2019) 580:1–9. doi: 10.1016/j.colsurfa.2019.123739
92. Takai E, Kitamura T, Kuwabara J, Ikawa S, Yoshizawa S, Shiraki K, et al. Chemical modification of amino acids by atmospheric-pressure cold plasma in aqueous solution. J Phys D Appl Phys. (2014) 47:1–15. doi: 10.1088/0022-3727/47/28/285403
93. Liu J, Gasmalla MAAG, Li P, Yang R. Enzyme-assisted extraction processing from oilseeds: principle, processing and application. Innov Food Sci Emerg Technol. (2016) 35:184–93. doi: 10.1016/j.ifset.2016.05.002
94. Hartmann R, Meisel H. Food-derived peptides with biological activity: from research to food applications. Curr Opin Biotechnol. (2007) 18:163–9. doi: 10.1016/j.copbio.2007.01.013
95. Nardo AE, Suárez S, Quiroga A V., Añón MC. Amaranth as a source of antihypertensive peptides. Front Plant Sci. (2020) 11:1–15. doi: 10.3389/fpls.2020.578631
96. Patto MC V, Amarowicz R, Aryee ANA, Boye JI, Chung H, Martín-Cabrejas MA, et al. Achievements and challenges in improving the nutritional quality of food legumes. CRC Crit Rev Plant Sci. (2015) 34:105–43. doi: 10.1080/07352689.2014.897907
97. Chen L, Chen J, Yu L, Wu K, Zhao M. Emulsification performance and interfacial properties of enzymically hydrolyzed peanut protein isolate pretreated by extrusion cooking. Food Hydrocoll. (2018) 77:607–16. doi: 10.1016/j.foodhyd.2017.11.002
98. Wouters AGB, Rombouts I, Fierens E, Brijs K, Delcour JA. Relevance of the functional properties of enzymatic plant protein hydrolysates in food systems. Compr Rev Food Sci Food Saf. (2016) 15:786–800. doi: 10.1111/1541-4337.12209
99. Schlegel K, Sontheimer K, Eisner P, Schweiggert-Weisz U. Effect of enzyme-assisted hydrolysis on protein pattern, technofunctional, and sensory properties of lupin protein isolates using enzyme combinations. Food Sci Nutr. (2020) 8:3041–51. doi: 10.1002/fsn3.1286
100. Arvanitoyannis IS, Tziatzios G. Proteins. In: Arvanitoyannis IS, editor. Irradiation of Food Commodities. Amsterdam: Elsevier Inc. (2010). p. 367–447.
101. Pietrysiak E, Smith DM, Smith BM, Ganjyal GM. Enhanced functionality of pea-rice protein isolate blends through direct steam injection processing. Food Chem. (2018) 243:338–44. doi: 10.1016/j.foodchem.2017.09.132
102. Simão RS, Moraes JO, Souza PG, Carciofi BAM, Laurindo JB. Production of mango leathers by cast-tape drying: product characteristics and sensory evaluation. LWT Food Sci Technol. (2018) 99:445–52. doi: 10.1016/j.lwt.2018.10.013
103. Durigon A, Parisotto EIB, Carciofi BAM, Laurindo JB. Heat transfer and drying kinetics of tomato pulp processed by cast-tape drying. Dry Technol. (2017) 36:160–8. doi: 10.1080/07373937.2017.1304411
104. Parisotto EIB, Teleken JT, Laurindo JB, Carciofi BAM. Mathematical modeling and experimental assessment of the cast-tape drying. Dry Technol. (2019) 0:1–12. doi: 10.1080/07373937.2019.1610768
105. Ding Q, Zhang T, Niu S, Cao F, Wu-Chen RA, Luo L, et al. Impact of ultrasound pretreatment on hydrolysate and digestion products of grape seed protein. Ultrason Sonochem. (2018) 42:704–13. doi: 10.1016/j.ultsonch.2017.11.027
106. Lusas EW, Rhee KC. Soy protein processing and utilization. In: Erickson DR, editor. Practical Handbook of Soybean Processing and Utilization (Urbana, IL: AOCS Press) (1995). p. 117–60.
107. Arntfield SD, Maskus HD. Peas and other legume proteins. In: Phillips GO, Williams PA, editors. Handbook of Food Proteins. Sawston: Woodhead Publishing (2011). p. 233–66.
108. Torrezan R, Frazier RA, Cristianini M. Efeito do tratamento sob alta pressão isostática sobre os teores de fitato e inibidor de tripsina de soja. Bol Cent Pesqui Process Aliment. (2010) 28:179–86. doi: 10.5380/cep.v28i2.20400
109. Su D, Li S, Laurie HM, Zhao F, Zhang L, Zhao X, et al. Effects of high hydrostatic pressure on in vitro digestion of soy protein. Int Agric Eng J. (2010) 19:49–58.
110. Martínez KD, Ganesan V, Pilosof AMR, Harte FM. Effect of dynamic high-pressure treatment on the interfacial and foaming properties of soy protein isolate - hydroxypropylmethylcelluloses systems. Food Hydrocoll. (2011) 25:1640–5. doi: 10.1016/j.foodhyd.2011.02.013
111. Sun M, Mu T, Sun H, Zhang M. Digestibility and structural properties of thermal and high hydrostatic pressure treated sweet potato (Ipomoea batatas L.) protein. Plant Foods Hum Nutr. (2014) 69:270–5. doi: 10.1007/s11130-014-0426-9
112. Zhao Z, Mu T, Zhang M, Richel A. Chemical forces, structure, and gelation properties of sweet potato protein as affected by pH and high hydrostatic pressure. Food Bioprocess Technol. (2018) 11:1719–32. doi: 10.1007/s11947-018-2137-y
113. Adebowale YA, Adeyemi IA, Oshodi AA, Niranjan K. Isolation, fractionation and characterisation of proteins from Mucuna bean. Food Chem. (2007) 104:287–99. doi: 10.1016/j.foodchem.2006.11.050
114. Chao D, Jung S, Aluko RE. Physicochemical and functional properties of high pressure-treated isolated pea protein. Innov Food Sci Emerg Technol. (2018) 45:179–85. doi: 10.1016/j.ifset.2017.10.014
115. Yang J, Liu G, Zeng H, Chen L. Effects of high pressure homogenization on faba bean protein aggregation in relation to solubility and interfacial properties. Food Hydrocoll. (2018) 83:275–86. doi: 10.1016/j.foodhyd.2018.05.020
116. Primozic M, Duchek A, Nickerson M, Ghosh S. Formation, stability and in vitro digestibility of nanoemulsions stabilized by high-pressure homogenized lentil proteins isolate. Food Hydrocoll. (2018) 77:126–41. doi: 10.1016/j.foodhyd.2017.09.028
117. Saricaoglu FT, Gul O, Besir A, Atalar I. Effect of high pressure homogenization (HPH) on functional and rheological properties of hazelnut meal proteins obtained from hazelnut oil industry by-products. J Food Eng. (2018) 233:98–108. doi: 10.1016/j.jfoodeng.2018.04.003
118. Sim SYJ, Hua XY, Henry CJ. A novel approach to structure plant-based yogurts using high pressure processing. Foods. (2020) 9:1–10. doi: 10.3390/foods9081126
119. Katzav H, Chirug L, Okun Z, Davidovich-pinhas M, Shpigelman A. Comparison of thermal and high-pressure gelation of potato protein isolates. Foods. (2020) 9:1–19. doi: 10.3390/foods9081041
120. Huang H, Kwok KC, Liang HH. Inhibitory activity and conformation changes of soybean trypsin inhibitors induced by ultrasound. Ultrason Sonochem. (2008) 15:724–30. doi: 10.1016/j.ultsonch.2007.10.007
121. Cui Q, Wang X, Wang G, Li R, Wang X, Chen S, et al. Effects of ultrasonic treatment on the gel properties of microbial transglutaminase crosslinked soy, whey and soy–whey proteins. Food Sci Biotechnol. (2019) 28:1455–64. doi: 10.1007/s10068-019-00583-y
122. Vanga SK, Wang J, Raghavan V. Effect of ultrasound and microwave processing on the structure, in-vitro digestibility and trypsin inhibitor activity of soymilk proteins. LWT Food Sci Technol. (2020) 131:109708. doi: 10.1016/j.lwt.2020.109708
123. Omura MH, Oliveira APH, Soares LS, Coimbra JSR, Barros FAR, Vidigal MCTR, et al. Effects of protein concentration during ultrasonic processing on physicochemical properties and techno-functionality of plant food proteins. Food Hydrocoll. (2021) 113:106457. doi: 10.1016/j.foodhyd.2020.106457
124. Mao C, Wu J, Zhang X, Ma F, Cheng Y. Improving the solubility and digestibility of potato protein with an online ultrasound-assisted pH shifting treatment at medium temperature. Foods. (2020) 9:1908. doi: 10.3390/foods9121908
125. Silventoinen P, Sozer N. Impact of ultrasound treatment and pH-shifting on physicochemical properties of protein-enriched barley fraction and barley protein isolate. Foods. (2020) 9:1–14. doi: 10.3390/foods9081055
126. Malik MA, Sharma HK, Saini CS. High Intensity Ultrasound Treatment of Protein Isolate Extracted From Dephenolized Sunflower Meal: Effect on Physicochemical and functional properties. Amsterdam: Elsevier B.V. (2017).
127. Biswas B, Sit N. Effect of ultrasonication on functional properties of tamarind seed protein isolates. J Food Sci Technol. (2020) 57:2070–8. doi: 10.1007/s13197-020-04241-8
128. Jiang S, Ding J, Andrade J, Rababah TM, Almajwal A, Abulmeaty MM, et al. Modifying the physicochemical properties of pea protein by pH-shifting and ultrasound combined treatments. Ultrason Sonochem. (2017) 38:835–42. doi: 10.1016/j.ultsonch.2017.03.046
129. Garde-Cerdán T, Arias-Gil M, Marsellés-Fontanet AR, Ancín-Azpilicueta C, Martín-Belloso O. Effects of thermal and non-thermal processing treatments on fatty acids and free amino acids of grape juice. Food Control. (2007) 18:473–9. doi: 10.1016/j.foodcont.2005.12.004
130. Yu X, Bals O, Grimi N, Vorobiev E. A new way for the oil plant biomass valorization: polyphenols and proteins extraction from rapeseed stems and leaves assisted by pulsed electric fields. Ind Crops Prod. (2015) 74:309–18. doi: 10.1016/j.indcrop.2015.03.045
131. Chiesa S, Gnansounou E. Protein extraction from biomass in a bioethanol refinery – possible dietary applications: use as animal feed and potential extension to human consumption. Bioresour Technol. (2011) 102:427–36. doi: 10.1016/j.biortech.2010.07.125
132. Jourdan GA, Norea CPZ, Brandelli A. Inactivation of trypsin inhibitor activity from Brazilian varieties of beans (Phaseolus vulgaris L.). Food Sci Technol Int. (2007) 13:195–8. doi: 10.1177/1082013207079898
133. Varghese T, Pare A. Effect of microwave assisted extraction on yield and protein characteristics of soymilk. J Food Eng. (2019) 262:92–9. doi: 10.1016/j.jfoodeng.2019.05.020
134. Sadeghi AA, Shawrang P. Effects of microwave irradiation on ruminal degradability and in vitro digestibility of canola meal. Anim Feed Sci Technol. (2006) 127:45–54. doi: 10.1016/j.anifeedsci.2005.08.016
135. Zhang H, Yu L, Yang Q, Sun J, Bi J, Liu S, et al. Optimization of a microwave-coupled enzymatic digestion process to prepare peanut peptides. Molecules. (2012) 17:5661–74. doi: 10.3390/molecules17055661
136. Alajaji SA, El-Adawy TA. Nutritional composition of chickpea (Cicer arietinum L.) as affected by microwave cooking and other traditional cooking methods. J Food Compos Anal. (2006) 19:806–12. doi: 10.1016/j.jfca.2006.03.015
137. Ji H, Dong S, Han F, Li Y, Chen G, Li L, et al. Effects of dielectric barrier discharge (DBD) cold plasma treatment on physicochemical and functional properties of peanut protein. Food Bioprocess Technol. (2018) 11:344–54. doi: 10.1007/s11947-017-2015-z
138. Bahrami N, Bayliss D, Chope G, Penson S, Perehinec T, Fisk ID. Cold plasma: a new technology to modify wheat flour functionality. Food Chem. (2016) 202:247–53. doi: 10.1016/j.foodchem.2016.01.113
139. Pal P, Kaur P, Singh N, Kaur AP, Misra NN, Tiwari BK, et al. Effect of nonthermal plasma on physico-chemical, amino acid composition, pasting and protein characteristics of short and long grain rice flour. Food Res Int. (2016) 81:50–7. doi: 10.1016/j.foodres.2015.12.019
140. Lu W, Chen XW, Wang JM, Yang XQ, Qi JR. Enzyme-assisted subcritical water extraction and characterization of soy protein from heat-denatured meal. J Food Eng. (2016) 169:250–8. doi: 10.1016/j.jfoodeng.2015.09.006
141. Jiang L, Hua D, Wang Z, Xu S. Aqueous enzymatic extraction of peanut oil and protein hydrolysates. Food Bioprod Process. (2010) 88:233–8. doi: 10.1016/j.fbp.2009.08.002
142. Zhang HJ, Zhang H, Wang L, Guo XN. Preparation and functional properties of rice bran proteins from heat-stabilized defatted rice bran. Food R. (2012) 47:359–63. doi: 10.1016/j.foodres.2011.08.014
143. Prosekov A, Babich O, Kriger O, Ivanova S, Pavsky V, Sukhikh S, et al. Functional properties of the enzyme-modified protein from oat bran. Food Biosci. (2018) 24:46–9. doi: 10.1016/j.fbio.2018.05.003
144. Latif S, Anwar F, Hussain AI, Shahid M. Aqueous enzymatic process for oil and protein extraction from Moringa oleifera seed. Eur J Lipid Sci Technol. (2011) 113:1012–8. doi: 10.1002/ejlt.201000525
145. Niu YX, Li W, Zhu J, Huang Q, Jiang M, Huang F. Aqueous enzymatic extraction of rapeseed oil and protein from dehulled cold-pressed double-low rapeseed cake. Int J Food Eng. (2012) 8:1–14. doi: 10.1515/1556-3758.2530
146. Souza TSP, Dias FFG, Oliveira JPS, Bell JMLNM, Koblitz MGB. Biological properties of almond proteins produced by aqueous and enzyme-assisted aqueous extraction processes from almond cake. Sci Rep. (2020) 10:1–12. doi: 10.1038/s41598-020-67682-3
147. Sathitkowitchai W, Nitisinprasert S, Keawsompong S. Improving palm kernel cake nutrition using enzymatic hydrolysis optimized by Taguchi method. 3 Biotech. (2018) 8:407. doi: 10.1007/s13205-018-1433-6
148. Mokni Ghribi A, Maklouf Gafsi I, Sila A, Blecker C, Danthine S, Attia H, et al. Effects of enzymatic hydrolysis on conformational and functional properties of chickpea protein isolate. Food Chem. (2015) 187:322–30. doi: 10.1016/j.foodchem.2015.04.109
149. Dias DR, Abreu CMP, Silvestre MPC, Schwan RF. In vitro protein digestibility of enzymatically pre-treated bean (Phaseolus vulgaris L.) flour using commercial protease and Bacillus sp. protease. Food Sci Technol. (2010) 30:94–9. doi: 10.1590/S0101-20612010005000010
Keywords: plant-based proteins, food processing, eco-friendly technologies, nutritional quality, in vitro protein digestibility, food safety
Citation: Sá AGA, Laurindo JB, Moreno YMF and Carciofi BAM (2022) Influence of Emerging Technologies on the Utilization of Plant Proteins. Front. Nutr. 9:809058. doi: 10.3389/fnut.2022.809058
Received: 04 November 2021; Accepted: 18 January 2022;
Published: 11 February 2022.
Edited by:
Emmanuel Purlis, Consejo Nacional de Investigaciones Científicas y Técnicas (CONICET), ArgentinaReviewed by:
Francisco Speroni, National University of La Plata, ArgentinaYukiharu Ogawa, Chiba University, Japan
Vijaya G. S. Raghavan, McGill University, Canada
Copyright © 2022 Sá, Laurindo, Moreno and Carciofi. This is an open-access article distributed under the terms of the Creative Commons Attribution License (CC BY). The use, distribution or reproduction in other forums is permitted, provided the original author(s) and the copyright owner(s) are credited and that the original publication in this journal is cited, in accordance with accepted academic practice. No use, distribution or reproduction is permitted which does not comply with these terms.
*Correspondence: Bruno Augusto Mattar Carciofi, YnJ1bm8uY2FyY2lvZmlAdWZzYy5icg==