- College of Food Science and Engineering, Guangdong Ocean University, Yangjiang, China
Diabetes mellitus (DM) is a global health threat. Searching for anti-diabetic components from natural resources is of intense interest to scientists. Mushroom polysaccharides have received growing attention in anti-diabetes fields due to their advantages in broad resources, structure diversity, and multiple bioactivities, which are considered an unlimited source of healthy active components potentially applied in functional foods and nutraceuticals. In this review, the current knowledge about the roles of oxidative stress in the pathogenesis of DM, the extraction method of mushroom polysaccharides, and their potential biological mechanisms associated with anti-diabetes, including antioxidant, hypolipidemic, anti-inflammatory, and gut microbiota modulatory actions, were summarized based on a variety of in vitro and in vivo studies, with aiming at better understanding the roles of mushroom polysaccharides in the prevention and management of DM and its complications. Finally, future perspectives including bridging the gap between the intervention of mushroom polysaccharides and the modulation of insulin signaling pathway, revealing structure-bioactivity of mushroom polysaccharides, developing synergistic foods, conducting well-controlled clinical trials that may be very helpful in discovering valuable mushroom polysaccharides and better applications of mushroom polysaccharides in diabetic control were proposed.
1 Introduction
Mushrooms have been a part of human diet for thousands of years for their characteristic flavor and texture as an authentic delicacy. There are approximately 150,000–160,000 mushroom species on earth, of which only 10% are known to science (1). About 700 mushroom species have pharmacological activities and are edible (1). Since the 1970s, global mushroom production has increased more than 30 times, and China has become the world’s largest producer (2). Mushrooms not only be consumed as foods, but also be widely used as medicinal materials. Accumulative evidences from animal models and human intervention studies have demonstrated their bioactivities in anti-cancer (3), anti-inflammation (4), immunomodulation (5), and their ability in the prevention and management of metabolic-related diseases, including diabetes, obesity, and cardiovascular diseases (6), and their protective action on the degenerative brain function (7). Furthermore, some researchers have also proposed the potential of mushrooms in the COVID-19 treatment of the disease COVID-19 (8).
Mushrooms are rich in protein and carbohydrates, with approximately 13–62% of crude protein and 14–75% of carbohydrates in the dry matter for wild-growing mushrooms, whereas they have low content in fat, approximately 0.1–8% (9). The primary elements in mushrooms include potassium, magnesium, calcium, and sodium, with potassium as the dominant element. Vitamins including ascorbic acid, B-group vitamins, tocopherols, and provitamin of ergocalciferol (vitamin D2), pigments, and phenolics, are also widely reported to be present in mushrooms (9). Studies have revealed many types of active components present in mushrooms, including high molecular weight components such as polysaccharides and polysaccharide-protein complexes, lectins, fungal immunomodulatory proteins (FIPs), ribosome-inactivating proteins (RIPs), ribonucleases, laccases, polyphenols, and low molecular weight components such as triterpenes, ergothioneine, alkaloids (5, 6). Among these components, polysaccharides and polysaccharide–protein complexes are highly contained in mushrooms, which primarily contribute to the bioactivities of mushrooms (10).
Diabetes mellitus (DM) is a non-communicable disease characterizing chronic hyperglycemia caused by impaired functions in insulin secretion and/or insulin action (11). Clinically, DM is classified into type 1 diabetes mellitus (T1DM), type 2 diabetes mellitus (T2DM), gestational DM, and specific types of DM due to gene, drug, or disease (12). T2DM is due to a progressive insulin insufficiency on the background of peripheral insulin resistance, accounting for more than 90% of all diabetes (12). DM is a major reason for the development of cardiovascular diseases, blindness, kidney failure, and amputations of limbs worldwide, which are called complications (13). In 2017, 6.28% of the world’s population was affected by T2DM, and the global prevalence rate of T2DM is estimated to be approximately 7.08% by 2030 (14). The high prevalence of DM and its complications place a tremendous burden on the healthcare system worldwide. The pharmacological mechanisms of drugs for the management of DM include inhibition of glucose absorption from diets, reduction of hepatic glucose production, and increased insulin sensitivity in tissues (15). However, currently available most of the synthetic drugs in the long-term management of DM have side effects such as weight gain and hypoglycemia along with their therapeutic potential (15). Natural components derived from food materials and traditional herbal medicines, such as mushrooms, and medicinal plants, have emerged as safe and relatively economical approaches (16, 17).
Interdisciplinary studies focusing on mushrooms have documented much of the knowledge on mushroom polysaccharides and increasingly demonstrate valuably pharmaceutical properties of mushroom polysaccharides extracted from a number of species. As a result, several reviews have been conducted in the field of mushroom polysaccharides related to DM. Khursheed et al. (18) have summarized mushroom polysaccharides with anti-diabetic activity mainly derived from Hericium erinaceus, Phellinus linteus, Inonotus obliquus, Catathelasma ventricosum, and Ganoderma lucidum from the view of modulating gut microbiota. The potential applications of mushroom polysaccharides in diabetic complications have also been proposed by researchers (19). Additionally, mushroom polysaccharides ameliorating oxidative stress, beta-cell dysfunction, and insulin resistance, which are closely associated with DM, through antioxidant action have been discussed recently (20). In this review, the extraction methods of mushroom polysaccharides, and mushroom polysaccharides with potentials in the management of DM reported in recent 3 years were summarized; the biological mechanisms including anti-oxidation, hypolipidemia, anti-inflammation, and modulating gut microbiota in anti-diabetes, and future perspectives were also discussed for a better understanding the current status of knowledge in such fields and providing a valuable reference for the development and application of mushrooms polysaccharides in functional foods and nutraceuticals for DM and its complications management.
2 Pathogenesis of diabetes mellitus and its complications
Reactive oxygen species (ROS) and reactive nitrogen species (RNS) are vital for normal physiology in human body. The ROS/RNS participate in normal physiology, including reversible protein modifications, adaptive mitogen-activated protein kinase (MAPK) signaling activation, and modulation of gene regulation, and play essential roles in a number of physiological processes. Therefore, cells keep a physiological content of ROS/RNS to maintain homeostasis (21). Endogenous factors, such as hormones, and pro-inflammatory cytokines, and exogenous factors, such as nutrients, and ultraviolet irradiation, trigger ROS/RNS generation through multiple mechanisms, including mitochondria, NADPH oxidase, nitric oxide synthase, etc. (22). High metabolic load such as elevated glucose and/or free fatty acid levels, inflammation, ER stress, and endocrine dysregulation, increase the production of ROS/RNS in cells (22). Oxidative stress is a result of the overproduction of ROS/RNS mainly produced by mitochondria, leading to an imbalance between antioxidants and pro-oxidants. This means excessive production and/or incapable removal of ROS/RNS within cells (23). A growing number of in vitro and in vivo studies have proposed that oxidative stress plays a central role in the pathogenesis of DM and its complications (Figure 1). Oxidative stress disrupts normal insulin signaling and progressively induces insulin resistance (22). Insulin resistance is an early symptom of the pathogenesis of DM characterized by high levels of blood insulin and glucose, defined as prediabetes. High level of insulin damages to β-cell mass and/or function progressively. Moreover, abnormal insulin level modulates the expressions of key molecules such as proprotein convertase subtilisin/kexin type 9 (PCSK9), low-density lipoprotein receptor (LDLR) in lipid metabolism, and causes disorders of lipid metabolism (13). Additionally, oxidative stress-induced damages to DNA, proteins, and lipids in cells impair the structures and functions of cells and organs. Cumulative damages over time ultimately result in the pathogenesis of a variety of diseases including DM, cancer, Alzheimer’s disease, chronic renal failure, etc. (23). Furthermore, high level of glucose compromise autophagy in endothelial cells, leading to endothelial dysfunction and inducing disorder of platelets and thrombosis (13), therefore, causing cardiovascular diseases ultimately such as coronary artery disease and microvascular diseases such as nephropathy, neuropathy, and retinopathy. Therefore, once hyperglycemia occurs, endogenous and exogenous factors drive patients to develop multiple chronic complications eventually.
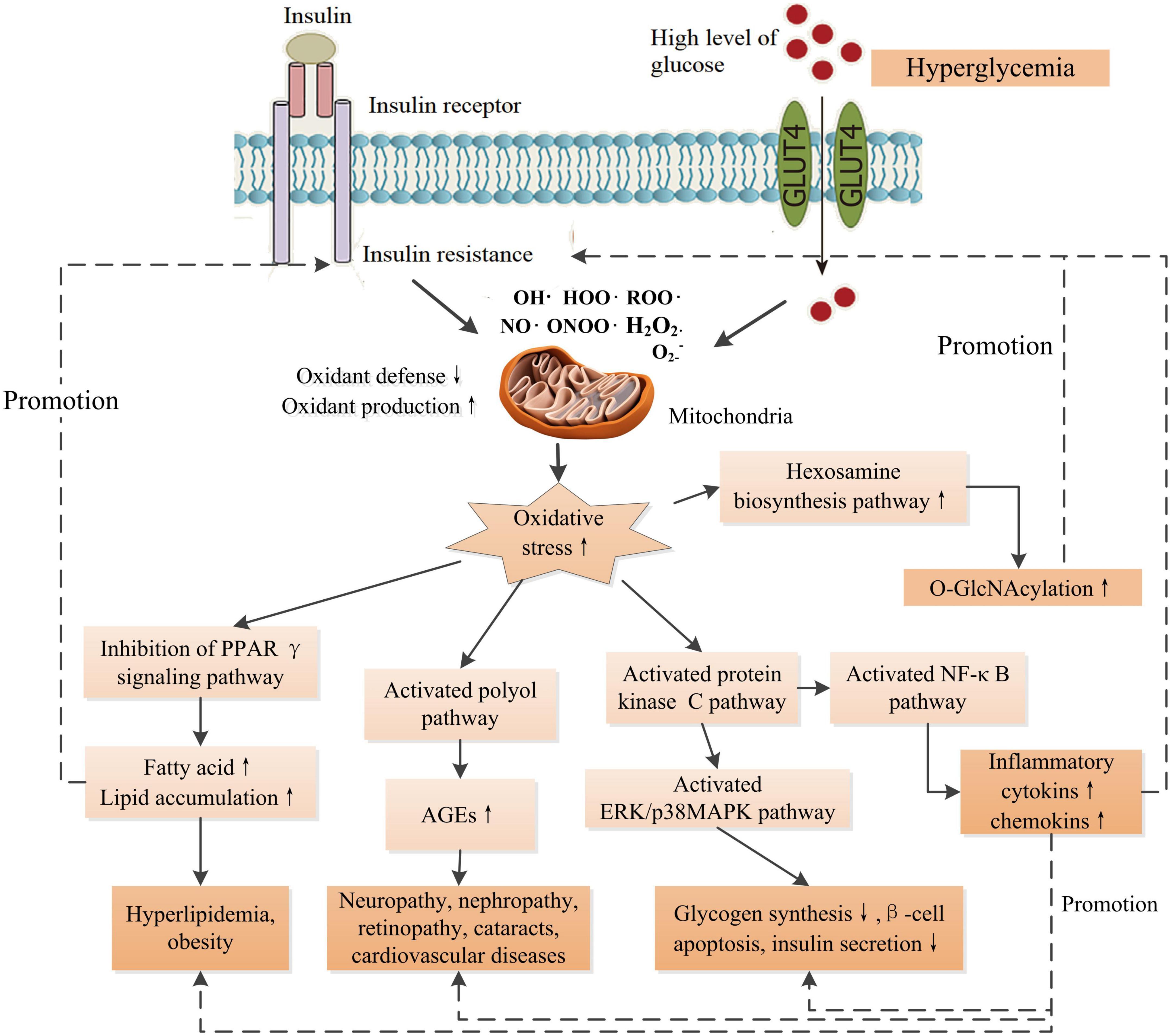
Figure 1. Schematic diagram of the effects of increased oxidative stress associated with insulin resistance and high glucose level on the signaling pathways of the development of DM and its complications. O-GlcNAcylation could promote the production of AGEs. AGEs contribute to inflammation through NF-κB signaling pathway. There are intricate links between oxidative stress and the development of complications. PPARγ, peroxisome proliferator activated receptor-γ; AGEs, advanced glycation end-products; ERK, extracellular-signal-regulated kinase; MAPK, mitogen-activated protein kinase; O-GlcNAcylation, O-linked-acetylglucosamine. ↑ Indicates increase and ↓ indicates decrease.
3 Polysaccharides from mushrooms with anti-diabetes potentials
Polysaccharides from medicinal plants, grains, fruits, vegetables, edible mushrooms, and medicinal foods have a wide range of bioactivities such as hypoglycemic, hypolipidemic, antioxidant, anti-inflammatory, and prebiotic effects, which are associated with anti-diabetes (24). Mushroom polysaccharides are naturally active components consumed frequently in our lives. Due to their low toxicity, easy availability, and multiple bioactivities, the evaluation of mushroom polysaccharides in anti-diabetic potential has attracted extensive interest from researchers (18, 24). The frequently investigated mushrooms include Cordyceps militaris, H. erinaceus, P. linteus, I. obliquus, C. ventricosum, G. lucidum, and Grifola frondosa. In recent years, a number of novel polysaccharides derived from a variety of mushrooms such as Auricularia auricula, Auricularia polytricha, and Dictyophora indusiata have been documented to have multiple pharmacological properties associated with anti-diabetic potentials, including improving lipid metabolism, increasing hepatic glycogen synthesis, anti-inflammation, and reducing insulin resistance, etc. (Table 1).
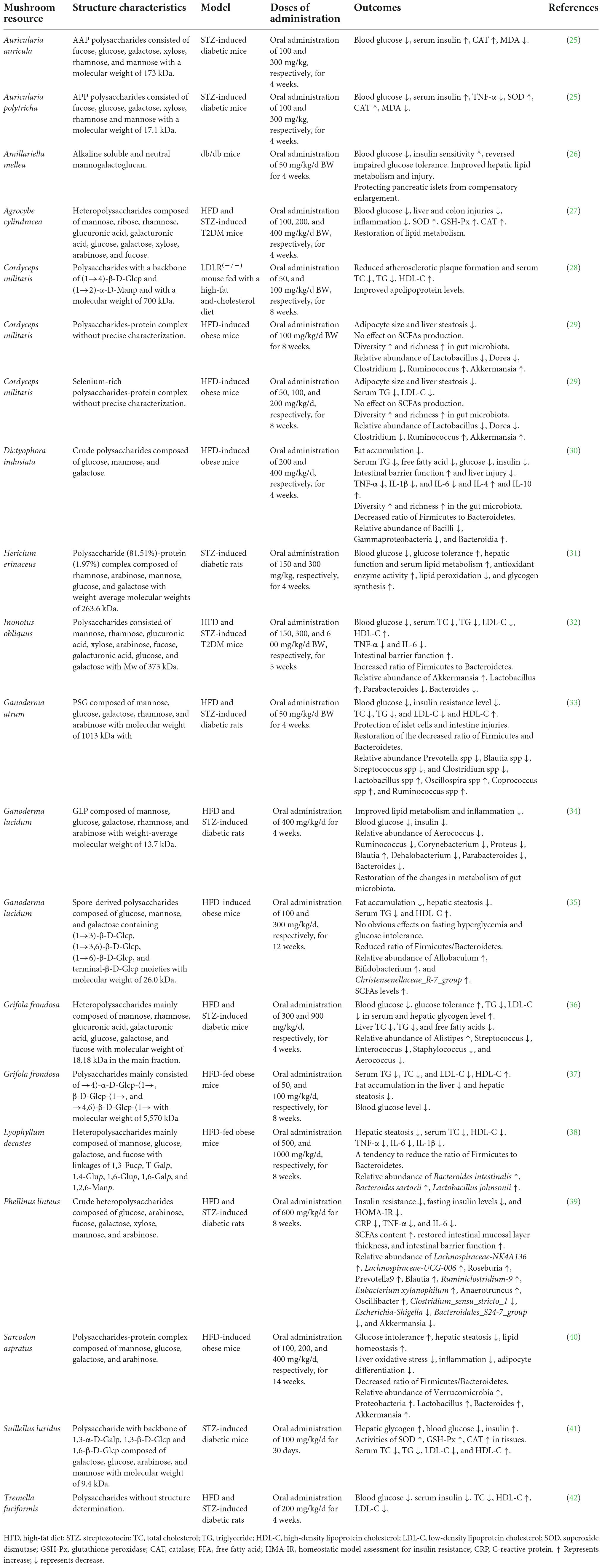
Table 1. Polysaccharides derived from mushrooms with anti-diabetic potentials reported in recent years.
4 Biological mechanisms of mushroom polysaccharides on anti-diabetes effects
4.1 Antioxidant action
Oxidative stress arising from the overproduction of ROS/RNS within cells plays a crucial role in the pathogenesis of many chronic diseases, including DM, cancer, cardiovascular diseases, Alzheimer’s disease, and so on. A large number of studies have documented that mushroom polysaccharides have notable activities in scavenging against 1,1-Diphenyl-2-picrylhydrazyl (DPPH), hydroxyl radicals, superoxide radicals, hydrogen peroxide, nitric oxide, and peroxynitrite, and inhibiting lipid peroxidation (43, 44). Among the active components contained in mushrooms, including flavonoids, polysaccharides, phenols, phenolic compounds, tocopherols, ascorbic acid, and terpenes, polysaccharides exhibit relatively low antioxidant activities in terms of scavenging activities against free radicals using in vitro models (43). However, the evaluation of scavenging activities in vitro is not a fully valid proof to claim the antioxidant effects of the polysaccharides because the scavenging activities investigations cannot simulate the interaction of antioxidants with chemical free radicals in the internal conditions of cells in a complex organism (45). Interestingly, numerous in vivo studies have shown that mushroom polysaccharides are capable of protecting the antioxidant activities of enzymes such as superoxide dismutase (SOD), catalase (CAT), and glutathione peroxidase (GSH-Px), which are major components of the defense system against ROS within body (20). For instance, oral administration of Tremella fuciformis polysaccharides (TFPS) markedly improved the SOD and GSH-Px activities in serum, liver, and heart tissues in D-galactose-induced aging mice (46). G. lucidum polysaccharides showed protective effects against acute liver injury induced by restraint stress in mice, evidenced by the improvement of GSH-Px, CAT, and SOD activities and a decrease in the activities of ALT and AST (47). Agaricus blazei Murill polysaccharides alleviated liver and lung damages by improving the activities of SOD, GSH-Px, and CAT to relieve oxidative stress in organ dysfunction syndrome (MODS) mice (48). Moreover, many studies have also revealed that mushroom polysaccharides enhance the activities of antioxidant enzymes including SOD, GSH-Px, and CAT in diabetes models (Table 1). However, the molecular mechanisms by which mushroom polysaccharides modulate the activity of antioxidant enzymes in vivo are still unclear. Natural macromolecules such as polyphenols can provide antioxidant protection against oxidative damage by regulating complicated intracellular signals for the induction of antioxidant enzymes (49). Plant-derived polysaccharides have been reported to promote the expression of antioxidant genes in Drosophila and cell models (50, 51). Polysaccharides derived from Suillellus luridus have been supposed to relieve oxidative stress in STZ-induced diabetic mice by regulating the nuclear factor erythroid 2-related factor 2 (Nrf2)/heme oxygenase-1 (HO-1) pathway (41). Nrf2 modulates the expression of antioxidant and cyto-protective related genes (52). Therefore, more studies would be needed to uncover the molecular mechanism of how mushroom polysaccharides regulate the expression profiles of antioxidant genes in the mammalian models as well as the Nrf2/HO-1 pathway.
4.2 Anti-hyperlipidemic action
A high load of free fatty acids can result in reduced glucose uptake and insulin resistance in cells (53), which is a critical factor in the pathogenesis of obesity and type 2 diabetes. Ectopic lipid deposition within cells deteriorates insulin resistance and ultimately accelerates the progression of DM and various chronic complications (54). Intracellular molecules such as peroxisome proliferator activated receptors (PPARs), sterol regulatory element binding protein-1c (SREBP-1c), adenosine (AMP)-protein kinase (AMPK), acetyl-CoA carboxylase (ACC), and 3-hydroxy-3-methylglutaryl-CoA (HMG-CoA) reductase, fatty acid synthase (FAS), cytochrome P450 family 7 subfamily a member 1 (CYP7A1), and carnitine palmitoyltransferase-1 (CPT1) are critical mediators in the regulation of the biosynthesis of fatty acid, triglyceride, and cholesterol, and fatty acid oxidation within cells (55). Experiment evidences have proven that insulin resistance regulates these key mediators and results in abnormal lipid metabolism, progressively leading to obesity and hyperlipidemia, which in turn deteriorate insulin resistance, and therefore accelerate the progression of DM and its complications (Figure 2) (55–57).
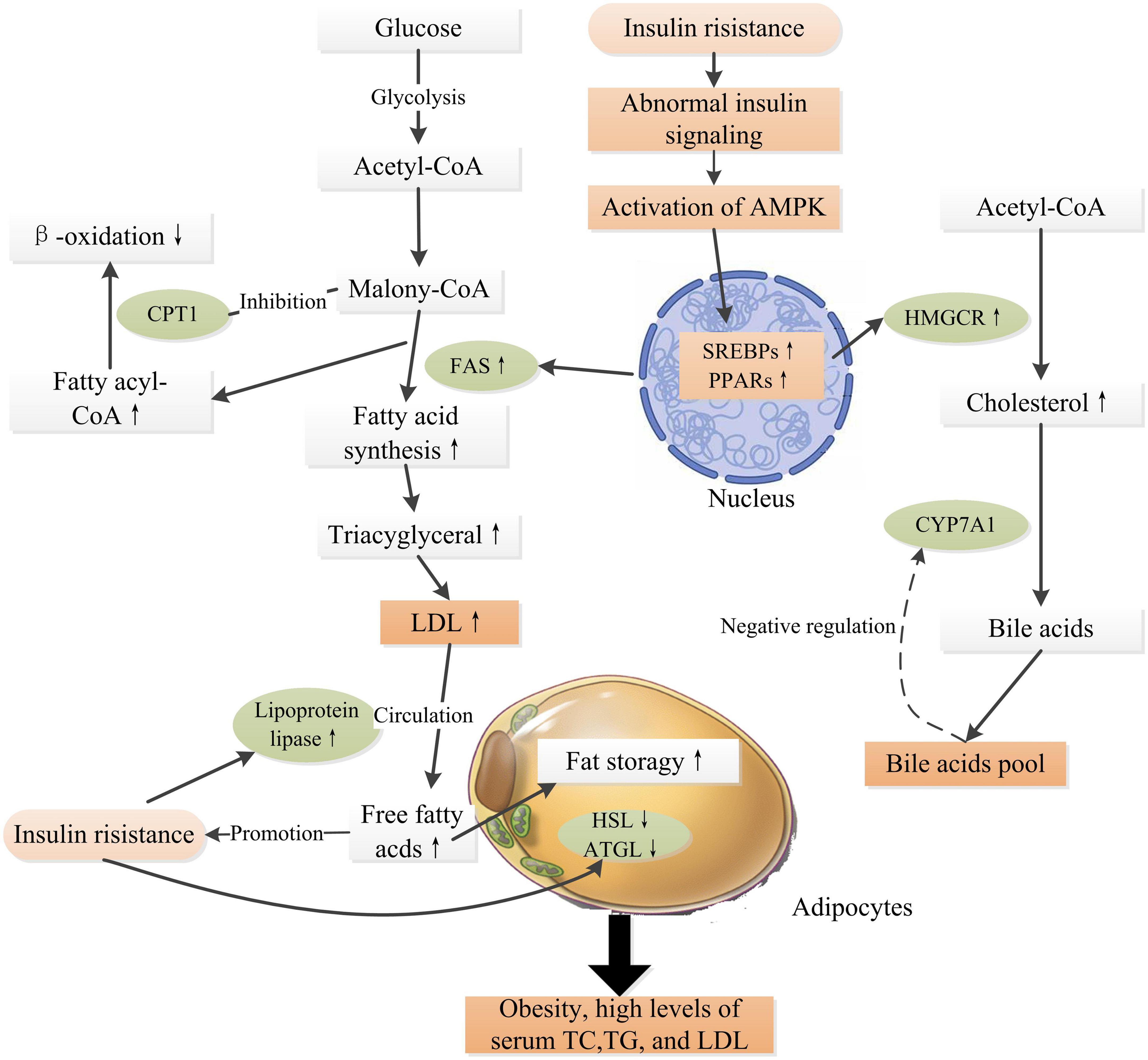
Figure 2. Schematic representation of insulin resistance and high glucose level inducing obesity and hyperlipidemia. Insulin resistance induces abnormal insulin signaling, activating transcription factors such as SREBPs, PPARs, leading to up-expressions of FAS and HMGCR, which promote fatty acid synthesis and cholesterol synthesis. CYP7A1 is a rate limiting enzyme in the pathway of transforming cholesterol into bile acids, which is regulated by the “bile acid pool” within human body. The β-oxidation of long-chain fatty acids is regulated by the activity of CPT1, which is highly sensitive to inhibition by malonyl-CoA (55). In adipose tissue, insulin is an anti-lipolytic hormone that inhibits the actions of HSL and ATGL, thus increasing fatty acid storage in adipocytes. Meanwhile, insulin increases LPL activity, releasing free fatty acids into the circulation from LDL (56). High levels of fatty acids in the circulation further, in turn, deteriorate insulin resistance. Obesity is an independent risk factor for the pathogenesis of DM and related complications. PPARs, peroxisome proliferator activated receptors; AMPK, adenosine (AMP)-protein kinase; SREBPs, sterol regulatory element binding proteins; ACC, acetyl-CoA carboxylase; HMGCR, 3-hydroxy-3-methylglutaryl-CoA reductase; FAS, fatty acid synthase; CYP7A1, cytochrome P450 family 7 subfamily a member 1; CPT1, carnitine palmitoyltransferase-1; HSL, hormone-sensitive lipase; ATGL, adipose triglyceride lipase. ↑ Indicates increase and ↓ indicates decrease.
Low-density lipoprotein (LDL) receptor-involved endocytosis is vital for cholesterol homeostasis, and upregulation of the LDL receptor results in a decrease in blood LDL-C level. However, in an LDLR(–/–) mouse model, C. militaris derived polysaccharides (CM) could decrease plasma total cholesterol (TC) and triglyceride (TG), and increase the high-density lipoprotein cholesterol (HDL-C) and improve apolipoprotein levels. Further molecular mechanical analysis revealed that CM markedly enhanced VLDLR expression and decreased SREBP-1c and ApoB expression at the protein level in the liver, and significantly increased the protein expression of LXRα/ABCG5 in the small intestine, as well as downregulation of the protein expression of PPARγ and ATGL in the epididymal fat (28). This experiment demonstrated a non-LDL receptor-involved cholesterol lowering pathway.
Pleurotus ostreatus polysaccharides fraction reduced liver triglyceride level through modulating the expressions of Dgat1, Ldlr, Nr1h4, Acat1, Srebf1, and Srebf2 in diet-induced hypercholesterolemic mice (58). Treatment with Trametes versicolor polysaccharide (EVP) dramatically reduced serum TC, TG, LDL-C, and atherosclerosis index in a dose-dependent manner in high-fat diet-induced hyperlipidemic mice. A significant increase in serum lipoprotein lipase (LPL) activity and a notable decrease in protein expression of hepatic 3-hydroxy-3-methylglutaryl-CoA reductase (HMGR) were observed, demonstrated that the anti-lipidemic properties of EVP probably via regulation of hepatic LPL and HMGR (59). Grifola frondosa polysaccharides (GFP) were proven to be capable of decreasing the levels of fasting blood glucose (FBG), oral glucose tolerance (OGT), TC, TG, and LDL-C in serum, and reducing the hepatic levels of TC, TG, and free fatty acids through inhibiting the expressions of SREBP-1c, ACC, Cd36, and HMGCR, and promoting the expressions of Acox1, Ldlr, CYP7A1, and BSEP in gene levels in high fat diet (HFD) and STZ-induced diabetic mice (36). Dictyophora indusiate crude polysaccharides displayed hypolipidemic actions, including a decrease in fat accumulation and serum TG and free fatty acid levels by inhibiting the expressions of lipogenic genes, such as PPAR-γ, C/EBPα, SREBP-1c, acetyl-CoA carboxylase-1 (ACC-1), and FAS (30). The polysaccharide-protein complex obtained from Sarcodon aspratus could improve lipid metabolism in HFD-induced obese mice by regulating lipogenesis meditators (ACC-1 and PGC-1α) (40). The current results suggested that different mushroom polysaccharides might play a role in hypolipidemic action through differential molecular mechanisms. Taken together, these observations demonstrated that mushroom polysaccharides could exert anti-lipidemic action by multiple pathways, including reducing TG and TC synthesis, decreasing lipid storage in adipocytes, elevating energy production, and promoting TC secretions from the liver and small intestine. Further studies are needed to illustrate the detailed mechanism of hypolipidemia of mushroom polysaccharides with high purity and precise structure characterization.
Numerous animal and human clinical trial studies have demonstrated that dietary polysaccharides, such as plant polysaccharides, mushroom polysaccharides, and alga polysaccharides, have potential anti-hyperlipidemic effects (60). It is commonly recognized that oat or barley β-glucan greater than 3.0 g/d is effective in lipid-lowering function in humans, and the high molecular weight of β-glucan is more effective than those with low molecular weight (60). Contrary to intensive investigations of oat or barley β-glucan in clinical trials, the investigation of anti-lipidemic effects of mushroom polysaccharides is mainly carried out in animal models. Thus, clinical trials to assess the efficacy of mushroom polysaccharides in metabolic disorders management are necessary for their successful applications (61). Additionally, structure-bioactivity relationship of mushroom polysaccharides is also an exciting topic, which provides some guides for exploring highly active polysaccharide. For example, degraded polysaccharides obtained from G. lucidum with a molecular weight of 13.6 kDa exhibited higher anti-lipidemic effects than the original ones with a higher molecular weight of 3.06 × 103 kDa, including atherosclerosis index, TC, TG, low-density lipoprotein cholesterol (LDL-C), and HDL-C in HFD-induced hyperlipidemia mice (62).
4.3 Anti-inflammatory action
Inflammation promotes the pathogenesis of insulin resistance and DM (63). Multiple studies have solidified a link between low-grade chronic inflammation and the development of DM, particularly T2DM, and the deterioration of cardiovascular diseases in people with DM. Obesity and insulin resistance are characterized by a chronic low-grade inflammation (64). Therefore, many factors, such as dysbiosis, intestinal barrier dysfunction, and low-fiber diets, which could aggravate inflammation, may be related to the progression of DM and its complications (65).
In the past several decades, mushroom polysaccharides have attracted growing interest from global researchers due to their outstanding immune-modulatory and anticancer properties and diversity in resources and structures (66). Mushroom polysaccharides are well known as biological response modifier (BRM) and immune-modulatory activity is one of their most important biological properties (67). The immunomodulatory mechanisms of mushroom polysaccharides to immune cells have been well recognized. Mushroom polysaccharides can interact with immune cells such as dendritic cells (DCs), macrophages, and NK cells through cell surface receptors and subsequently activate intracellular cascade signaling to induce immune responses (68). A variety of immune cell receptors, including dectin-1, scavenger receptors, complement receptor 3, lactosylceramide, and toll-like receptors (TLRs), are proposed to be involved in mushroom polysaccharide-induced immune responses, including immune modulation, anticancer, and anti-inflammation (68, 69). For example, TLR-2, TLR-4, and Dectin-1 have been identified to be involved in the activation of immune-related intracellular signaling of a novel polysaccharide from C. militaris (70). Natural polysaccharides have been documented to suppress the production of cyclooxygenase 2 (COX2) and inducible nitric oxide synthase (iNOS), which are associated with a reduction in pro-inflammatory responses, and modulate NF-κB-related signaling pathways to increase expression of anti-inflammatory cytokines like IL-10 and decrease expression of inflammatory cytokines such as TNF-α, IL-1β, leading to anti-inflammatory action (71). For instance, TFPS attenuated LPS-induced inflammation through inhibiting miR-155, a small RNA which regulates NF-κB, to inactivate NF-κB signaling pathway, leading to reduce the production of TNF-α and IL-6 in macrophages (72). Observations derived from dextran sulfate sodium (DSS)-treated animal model suggested that the anti-inflammatory effects of mushroom polysaccharides might be gut microbiota-dependent in vivo since β-glucan aggravated colitis features of DSS-treated mice which were given drinking water with antibiotics to diminish gut microbiota (73).
Intestinal epithelial cells (IECs) express TLRs and Dectin-1 receptors by which mushroom polysaccharides could activate a variety of physiological responses, including immune regulation, anti-inflammation, and enhanced barrier function (74–76), which might be important action pathways for exerting pharmacological properties of mushroom polysaccharides. In addition, as an essential part of gut-associated lymphoid tissue, Peyer’s patches distribute in the ileum region of the small intestine. Mushroom polysaccharides can be uptake into Peyer’s patches via M cells and stimulate resident immune cells such as macrophages, DCs, and T-cells (77), thus triggering a cascade of immune responses. Moreover, mushroom polysaccharides can be fermented by gut microbiota in the colon to produce SCFAs, although differently structural mushroom polysaccharides might induce differential production of SCFAs (66). SCFAs play anti-inflammatory activity through inducing the proliferation and differentiation of Foxp3+ regulatory T-cells and the secretion of IL-10 (78). In DSS-induced colitis mice, TFPS could inhibit colonic inflammation via promotion of Foxp3+ T-cells and production of anti-inflammatory cytokines (79). Therefore, mushroom polysaccharides could exert anti-inflammatory actions through multiple pathways but in concert by integrating various receptors-involved signaling within mucosal immune system (Figure 3).
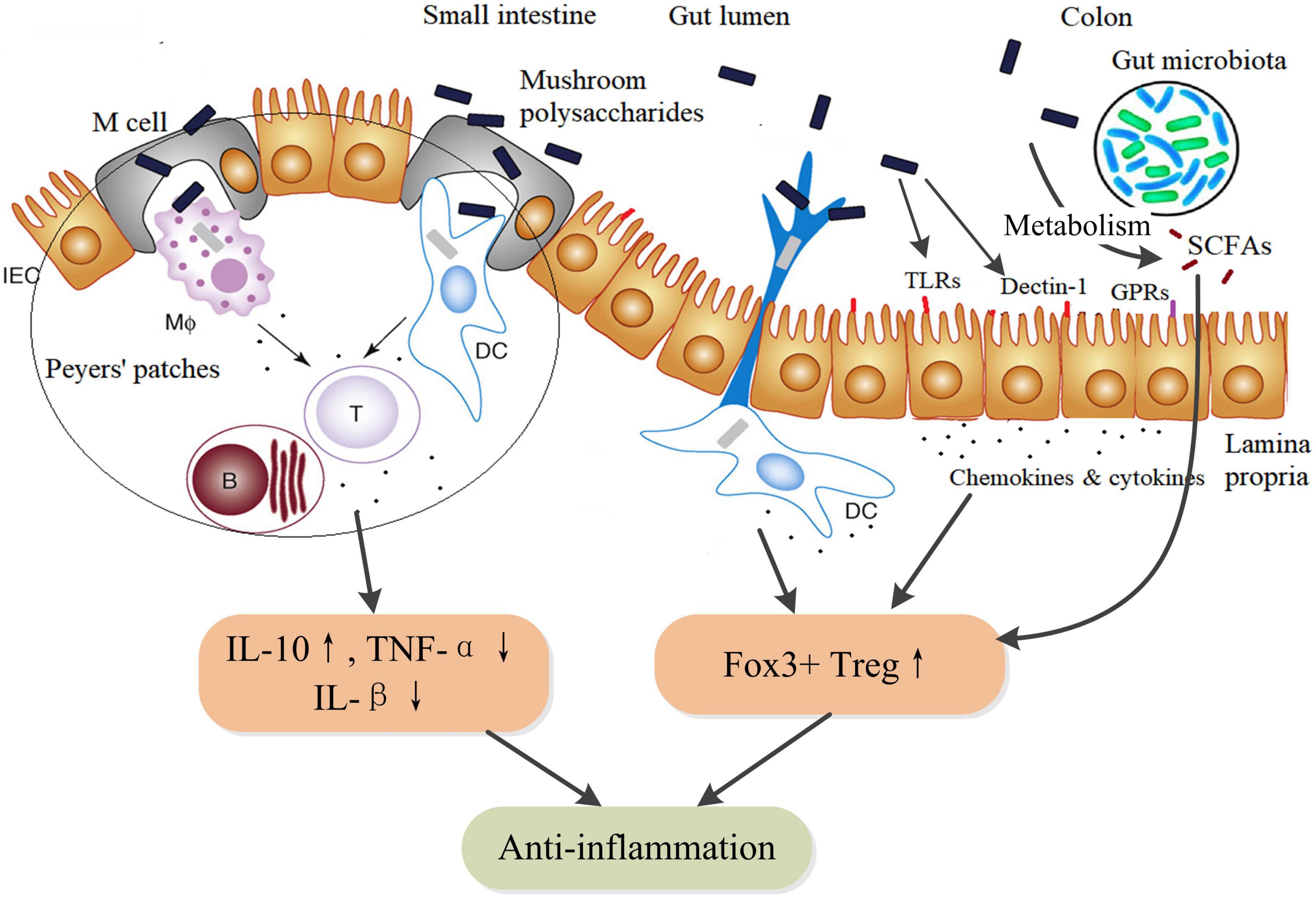
Figure 3. Schematic diagram of the anti-inflammatory action pathways of mushroom polysaccharides and their metabolites SCFAs. SCFAs, such as acetate, butyrate, and propionate, are metabolites of mushroom polysaccharides by fermentation of the gut microbiota. SCFAs facilitate the proliferation and differentiation of IL-10-producing Foxp3+ Treg by inhibiting histone deacetylase (HDAC) activity through G protein–coupled receptors (GPRs). Additionally, butyrate could directly regulate the function of macrophages and dendritic cells to induce Foxp3+ Treg (78). IEC, intestinal epithelial cell; MΦ, macrophages; DC, dendritic cell; B, B lymphocytes; T, TH lymphocytes; TLR, toll-like receptors; GPRs, G protein–coupled receptors (GPR41, GPR43, and GPR109A); SCFAs, short chain fatty acids.
A β-glucan isolated from the fruiting body of A. blazei Murill, named ABMP, with a triple-helical structure and a molecular weight of 12.26 kD, showed protective effects on acute inflammatory injury mice established by high dosage zymosan (ZY)-induced organ dysfunction syndrome (MODS) partially through activating NF-κB signaling pathway and then reducing expression of inflammatory cytokines, including TNF-α, IL-1β, IL-6, COX-2, and PGE-2 (48). The effects of a G. lucidum spore polysaccharides (BSGLP) with a molecular weight of 26.0 kDa on metabolic disorders and chronic inflammation were evaluated in a mouse model of dietary-induced obesity. BSGLP dramatically reduced serum levels of TNF-α, IL-1β, and monocyte chemoattractant protein-1 (MCP-1), and inhibited macrophage infiltration into white adipose tissues (WAT). Molecular mechanism analysis in mRNA and protein levels showed that BSGLP partially inhibits inflammation via TLR4/Myd88/NF-κB signaling pathway (35). Besides, BSGLP could increase SCFAs production, in particular acetate and butyrate production, which were associated with increased GPR43 expression and reduced inflammation in WAT. Furthermore, fecal microbiota transplantation (FMT) from HFD with BSGLP markedly decreased serum levels of LPS and TNF-α, and slightly decreased the content of IL-1β and MCP-1 in serum in the recipient mice, demonstrating that the anti-inflammatory effects of BSGLP might be in part dependent on the gut microbiota (35). These results suggested that multiple pathways may exist for each mushroom polysaccharides to exert anti-inflammatory effects, however, identifying the major action pathway is still great challenges to researchers. In recent years, a variety of mushroom polysaccharides obtained from different resources have shown anti-inflammation activities in metabolic disorder models (Table 1).
In an acute liver injury mouse model characterized by high levels of alanine aminotransferase (ALT) and aspartate aminotransferase (AST), and high levels of pro-inflammatory cytokines TNF-α, IL-2, IL-6, and MCP-1 induced by lipopolysaccharide/d-galactosamine (LPS/d-GalN), GFP inhibited inflammation level, elevated the levels of SOD and glutathione, and attenuated liver injury. Further analysis revealed that the expression levels of miR-122, a key molecule targeting Nrf2, was reduced and Nrf2/antioxidant response element (ARE) signaling pathway was regulated in liver tissue evidenced by upregulation of transcription factors Nrf2, Nqo-1, and HO-1, and downregulation of transcription factor Kelch-like ECH-associated protein 1 (Keap-1) (80). Keap-1 is vital for maintaining intracellular redox homeostasis and modulating inflammation. HO-1 plays a role in anti-inflammation (81). Thus, Nrf2/ARE signaling pathway might play key roles in modulating the antioxidant response and inflammatory response within cells. This provides an explanation that GFP exhibits anti-inflammation and anti-oxidation effects on the liver injury mouse model partially through activating the miR-122-Nrf2/ARE pathway (80, 81).
However, not all mushroom polysaccharides show consistently beneficial effects on hyperlipidemic animals. For example, Cordyceps sinensis polysaccharides (CSP) with a molecular weight of 6.5 × 104 Da could decrease serum levels of LDL-C and TC, but increase serum TG level in HFD-induced obese mice. Surprisingly, CSP deteriorated liver fibrosis and steatosis characterized by increased liver weight and fat accumulation, elevated alanine aminotransferase and infiltration of inflammatory cells in the liver. Furthermore, CSP could increase insulin resistance and inflammation. Therefore, CSP would lead to non-alcoholic steatohepatitis (NASH) and elevate the risk of type 2 diabetes. However, CSP could protect barrier function characterized by an increase in the expression of ZO-1 and occludin in HFD-induced obese mice (82). For these controversial findings, further investigations are needed to clarify the effects and its mechanisms of CSP on metabolic disorders.
4.4 Modulating of gut microbiota
Diabetes mellitus is strongly linked and associated with alterations in gut microbiota and intestinal barrier function (83). In patients with type 2 diabetes, Firmicutes and Clostridia are obviously reduced, while Proteobacteria and Bacteroidetes are increased, compared with those in healthy individuals (84). Elevated Ruminococcus and Fusobacterium, whereas decreased Roseburia and Clostridium, as well as fewer butyrate-producing bacteria, were observed in T2DM patients and prediabetes (85, 86). In animal models, continuous intake of a high-fat diet induces dysbiosis in the gut microbiota and intestinal barrier dysfunction, which is linked to the development of DM (32, 87). Gut microbiota involves in modulating insulin sensitivity and glucose tolerance through bile acids metabolism, amino acid metabolism, and SCFAs metabolism. Studies suggested that the absence of the commensal bacterium Akkermansia muciniphila damaged to integrity of intestinal barrier and increased intestinal permeability, leading to insulin resistance ultimately in mice and macaques (88). Correspondingly, supplementation of A. muciniphila restored normal insulin sensitivity in diabetic mice and macaques (88). Fecal microbiota transplantation from T2DM patients impaired insulin sensitivity and OGT in recipient mice by altering the ability of intestinal microbiota to metabolize bile acids (BAs) and regulating the BAs/glucagon-like peptide-1 (GLP-1) pathway (89). Branched-chain amino acids (BCAA) play important roles in regulating metabolism of glucose, lipid, and protein, except for being utilized as materials for synthesis of proteins (90). Increased BCAA levels contribute to the pathogenesis of insulin resistance and DM in human, whereas enhancing BCAA catabolism improves glucose homeostasis and ameliorates metabolic disorders related complications such as cardiovascular damage (91, 92). Interestingly, an intestinal microbiome possessing a higher potential for biosynthesis of BCAA was associated with increased levels of BCAA in plasma and decreased insulin sensitivity in human (93). Specifically, the gut bacteria Prevotella copri could elevate circulating levels of BCAAs and induce insulin resistance in mice (93). Thus, the downstream signaling activated by altered microbial metabolites and microbiota composition promotes the pathogenesis of metabolic diseases such as obesity and diabetes (94, 95) (Figure 4). In addition, LPS derived from the cell wall of Gram-negative bacteria in the gut activates inflammatory response through binding to toll-like receptor 4 (TLR4) on the mucosal immune cells, which is vital for maintaining gut microbiota homeostasis in normal physiology. However, dysbiosis results in elevated level of LPS in gut and therefore initiates low-grade chronic inflammation, which could induce insulin resistance to host (83).
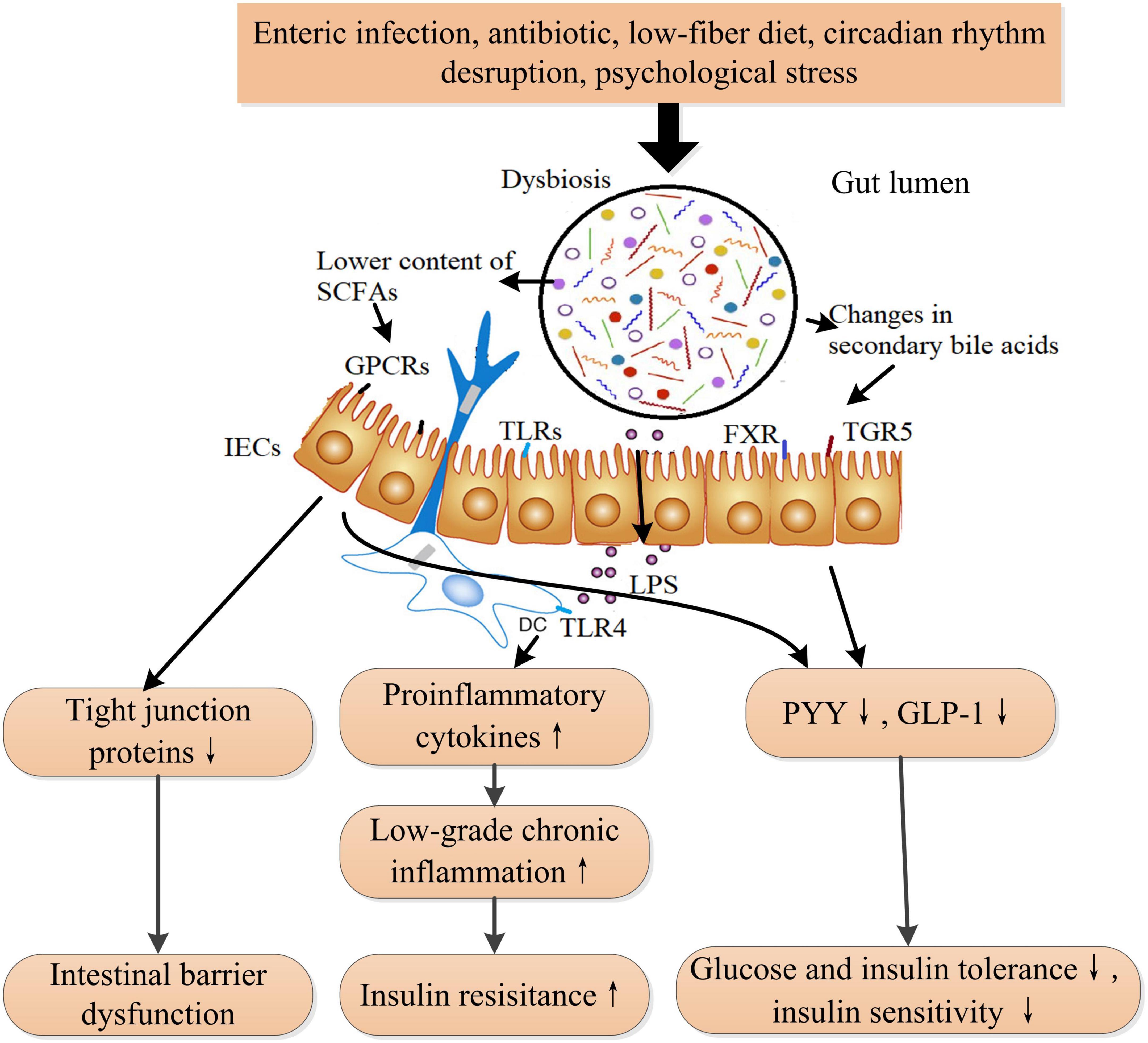
Figure 4. The proposed mechanism of dysbiosis in the gut microbiota that induces insulin resistance and ultimately DM. Gut microbiota is influenced by diet, medication, lifestyle, and stress. Dysbiosis in gut microbiota leads to increased circulating LPS, lower short-chain fatty acids (SCFAs) content, and changes in secondary bile acids. Increased LPS induces low-grade inflammation and insulin resistance through activating the toll-like receptor 4 signaling pathway. Bile acids are transformed into secondary bile acids through the metabolism of the altered gut microbiota. The alterations in secondary bile acids result in reduced GLP-1 and peptide YY secretions through TGR5 and FXR receptors-involved pathways from intestinal L cells. Lower SCFAs also reduces the secretions of GLP-1 and PYY and the expression of tight junction proteins through inactivating GPCRs (GPR41, GPR43, and GPR109A) signaling pathway, leading to intestinal barrier dysfunction and decreased insulin sensitivity. SCFAs also play a role in immune cells. LPS, lipopolysaccharide; TLRs, toll-like receptors; TLR4, toll-like receptor 4; FXR, farnesoid X receptor; TGR5, takeda G protein-coupled receptor 5; PYY, peptide YY; GLP-1, glucagon-like peptide-1; SCFAs, short-chain fatty acids; GPCRs, G-protein coupled receptors. ↑ Indicates increase and ↓ indicates decrease.
Recently, the modulatory properties of mushroom polysaccharides on gut microbiota have attracted intensive investigations because of the development of metagenome sequencing technology and genome-wide association studies. The genomes of gut microbiota encode a number of carbohydrate-active enzymes (CAZymes), which can degrade mushroom polysaccharides. Three extracellular degradation patterns of polysaccharides by gut microbiota have been illustrated to date, such as starch utilization system (Sus)-like system, ABC-transporter system, and multi-enzyme complex system (66, 96). Mushroom polysaccharides can arrive the colon, where the gut microbiota metabolizes them with mainly SCFAs as the final products through CAZymes degradation systems since gastric juices couldn’t hydrolyze them (97). According to the currently available data, different mushroom polysaccharides exhibit distinct effects on gut microbiota composition and its metabolites (66, 97), resulting in differential physiological responses to the host (98). These may be due to the diverse structures of mushroom polysaccharides (99), the physiological status of the host, and individual differences in gut microbial colonization (100). For example, two kinds of Flammulina velutipes-derived polysaccharides with different monosaccharide compositions showed differential productions of SCFAs in rats (101, 102). A heteropolysaccharides derived from Lentinus edodes reduced the diversity and evenness of gut microbiota in adult mice while increased the diversity and evenness of gut microbiota in aged mice (103, 104).
Increasing evidences have demonstrated the critical roles of gut microbiota in the prevention and treatment of DM (95). Altering the gut microbiota composition to improve insulin sensitivity and glucose tolerance has received attention from many researchers. Generally, mushroom polysaccharides decrease the ratio of Firmicutes to Bacteroidetes and increase the diversity and richness of gut microbiota along with ameliorations in blood glucose level and insulin sensitivity in obese and diabetic animal models. However, considerable inconsistent impacts on intestinal bacteria at family and genus levels were observed in different studies (Table 1). A polysaccharides from I. obliquus decreases FBG, improves intestinal barrier function, and restores the disrupted gut microbiota, characterizing upregulation of Ki-67, ZO-1, and MUC2 expression in the intestine, a restoration of the ratio of Firmicutes to Bacteroidetes, and enriched beneficial bacteria such as Akkermansia and Lactobacillus after the treatment in diabetic mice (32). However, different mushroom polysaccharides might exert differential impacts on the gut microbiota of diabetes, although exhibiting a similar alleviation in metabolic symptoms. For instance, both Ganoderma atrum polysaccharide (PGS) and white hyacinth bean polysaccharide (WHBP) ameliorated FBG and insulin resistance, as well as the levels of TC, TG, and LDL-C in T2DM rats. In common, PSG and WHBP alleviated the reduction of Firmicutes and the increase of Bacteroidetes, decreased the genus Prevotella spp, and elevated the genus Lactobacillus in T2DM rats. However, PSG and WHBP also showed their respective characteristic effects on microbial regulation. Specifically, PSG restricted the increase of Streptococcus spp and Clostridium spp, while WHBP could promote the growth of Roseburia spp and inhibit Turicibacter spp and Bacteroides spp in T2DM rats (33). Interestingly, a recent study revealed that commensal bacteria Bacteroides intestinalis and Lactobacillus johnsonii enriched by treatment of mushroom polysaccharides derived from Lyophyllum decastes were causally correlated with decreased plasma TG and LDL-C in HFD-induced obese mice through altering bile acid metabolism of gut microbiota (38), demonstrating a potential therapeutic approach of mushroom polysaccharides in the management of DM. Though many mushroom polysaccharides with structural characterization in somewhat context have been investigated in obese and diabetic models regarding the impacts on gut microbiota, the relationship of the structure of mushroom polysaccharides and the changes of gut microbiota in these models is still poorly understand due to heterogeneity in experimental protocols since environmental factors and animal species strongly affect the initial composition of gut microbiota (105). These influencing factors should be considered in future studies when uncovering the structure-function relationship in terms of mushroom polysaccharides and gut microbiota.
Mushroom polysaccharides show prebiotic properties in vitro fermentation, acting as a selective substrate for beneficial gut microbiota growth. The degree of proliferation of Bifidobacterium and Lactobacillus is commonly utilized to assess the prebiotic properties. Seven crude polysaccharides extracted from seven edible mushrooms: A. auricula-judae, L. edodes, Pleurotus citrinopileatus, Pleurotus djamor, P. ostreatus, P. ostreatus (Jacq.Fr.) Kummer and Pleurotus pulmonarius have been determined based on probiotic growth promotion (Lactobacillus acidophilus and Lactobacillus plantarum), pathogenic inhibition (Bacillus cereus, Escherichia coli, Salmonella Paratyphi, and Staphylococcus aureus). These results suggested that the monosaccharide composition of crude polysaccharides might influence their prebiotic properties (106). The indigestible residues of five strains of Macrocybe crassa mushroom varied in carbohydrate and phenolic content and showed differential impacts on the promotion of the growth of lactic acid bacteria and the inhibition of pathogenic bacteria growth (107). Polysaccharides from mushroom Clitocybe squamulosa (CSFP) were fermented in a simulated human fecal microbial model after its treatment by simulated saliva-gastrointestinal digestion. The production of total SCFAs was increased due to an increase in the content of acetic acid, propionic acid, and n-butyric acid, and the ratio of Firmicutes/Bacteroidetes was decreased. Specifically, CSFP promoted the growth of some beneficial bacteria, such as Bacteroides, and Prevotella_9, while inhibited the growth of the typical intestinal pathogen Escherichia-shigella (108). This demonstrates that simulated human intestinal fermentation in vitro model might be an appropriate tool to investigate the modulatory properties of different mushroom polysaccharides on gut microbiota (109). Variation in the molecular structure such as molecular weight, conformation, and branch degree of mushroom polysaccharides has a significant impact on their solubility, viscosity, and rheological properties (110), which might affect the accessibility of polysaccharides to degrading bacteria in the gut. However, the mechanism of how gut bacteria degrade and utilize mushroom polysaccharides is rarely illustrated, and more researches on this topic are needed.
5 Extraction method
The cell wall of mushrooms is a complex consisting mainly of glycoproteins, glucans, chitin, and less proteins; however, the assembly of these molecules has not been fully elucidated. Polysaccharides (50–60%) are the major structural components of the cell wall of mushrooms. Mushroom polysaccharides present in the cell wall can mainly be classified into three layers according to their distribution and cellular localization: (1) the outside layer is glycoprotein consisting of protein and heteropolysaccharides that vary in proportion and monosaccharide composition between species and sites of mushrooms; (2) the middle layer is mainly composed of β-glucan which are insoluble; (3) the inner layer is composed of a complex of chitin and β-glucan (43, 69). With the increasing interest in exploring the structures and bioactivities of mushroom polysaccharides by global researchers, various extraction methods followed by a purification process and structural identification have been developed (111) (Figure 5). Generally, aqueous solution is widely applied to extract polysaccharides with or without pre-treatment with organic solvents (e.g., ethanol, acetone) to remove lipids, and phenols, which facilitates the separation of polysaccharides from other compounds in the cell wall (112). Hot water is the most widely used because of its low cost and ease of handling. The frequently extracted polysaccharides are heteropolysaccharides-proteins complexes present in the outer layer of the cell wall because of its low efficiency in breaking through and penetrating the outer layer of glycoprotein structures. An acid or alkaline solution is commonly used to obtain a high amount of β-glucan present in the middle layer. For example, β-glucans with globular small particle sizes were extracted from the mushrooms Lentinula edodes and P. ostreatus using an acid-base treatment followed by boiling with 0.5 M NaOH (113). β-D-glucans obtained from mushroom Macrocybe titans were consisted of (1→3)-linked β-D-Glcp by alkaline extraction method (114). To increase the yield of polysaccharides and improve the efficacy in extraction and/or explore green processes, many novel assisted extraction technologies have been developed. Novel technologies such as microwave-assisted extraction (MAE), ultrasonic-assisted extraction (UAE), enzyme-assisted extraction (EAE), subcritical water extraction (SWE), pulsed electric field-assisted extraction, high pressurized water extraction, deep eutectic solvent extraction, and combined methods such as ultrasonic-microwave synergistic extraction (UMSE), subcritical and pressurized hot water extraction, pressure-associated hot water extraction have emerged in the extraction of mushroom polysaccharides (115–118). These novel extraction technologies mainly help solvent to penetrate through and rupture the outer layer to reach the inner cell wall, contributing to the release of polysaccharides from the compact matrix. However, every extraction method has advantages and disadvantages, such as extraction time, efficiency, and risk of glycosidic bond breakage. Comprehensive information including process parameters, yields, and structural characteristics of obtained polysaccharides on traditional extraction methods, and innovative and advanced extraction methods in the extraction of polysaccharides from mushrooms can refer to relating topic reviews (117, 118). The bioactivities of mushroom polysaccharides greatly depend on their structures, such as molecular weight, monosaccharide composition, branch of degree, and conformation (119), whereas the structures of mushroom polysaccharides vary remarkably based on resources (species), extraction methods, as well as purification processes (120), even dry processes of the fruiting body (121). Thus, novel extraction methods should be evaluated in terms of efficacy, yield, and bioactivity for better assessing the practicability in industrial applications. Recent studies have shown that innovative extraction methods can not only improve the efficacy in the extraction of polysaccharides from natural materials, but also enhance the bioactivities of the polysaccharides obtained (122), which provide a promising direction for future studies.
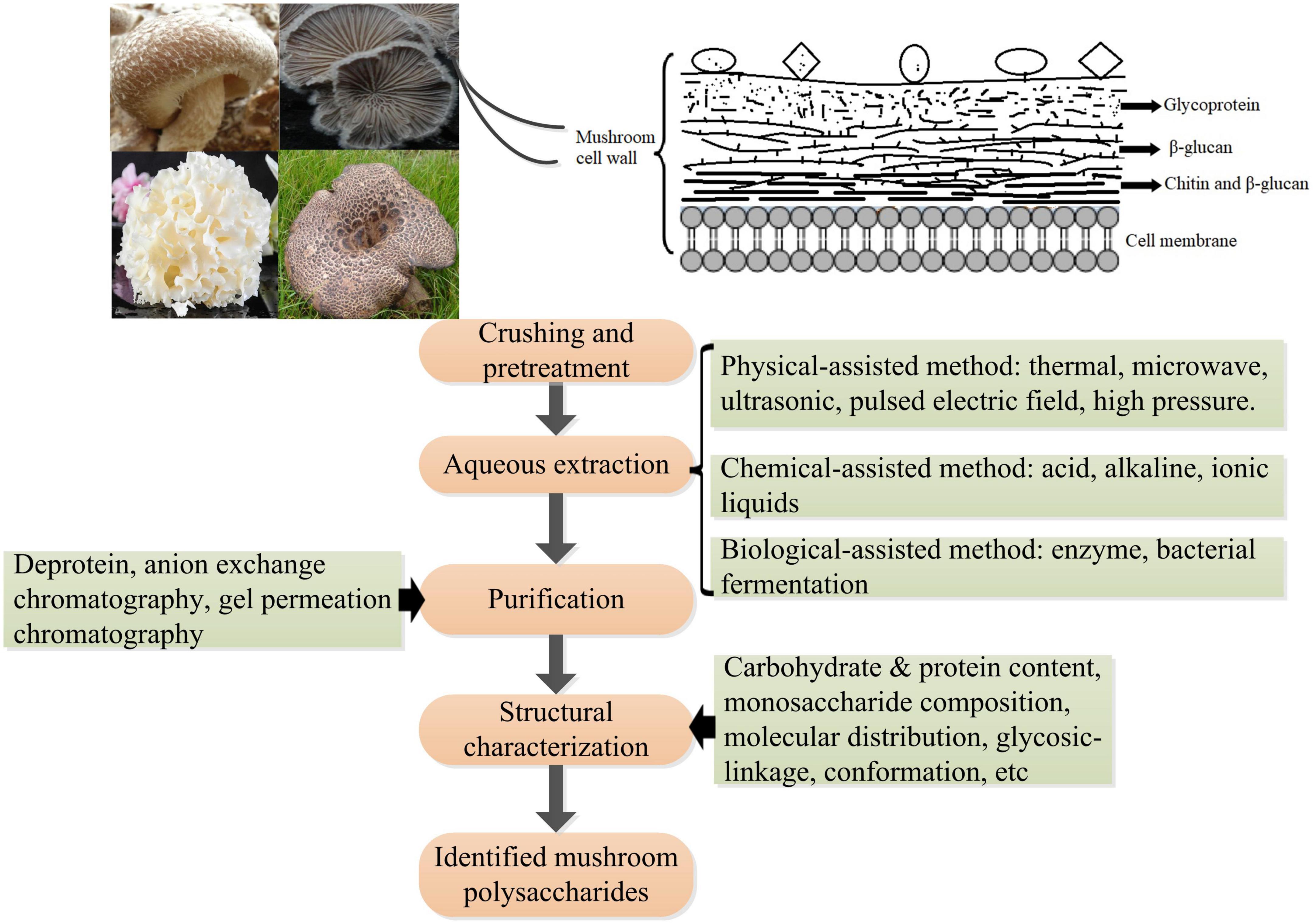
Figure 5. Schematic diagram of the polysaccharides’ location in the cell wall of mushrooms and the procedure of extraction, purification, and structural analysis of mushroom polysaccharides.
6 Future perspective
In recent decades, many studies have demonstrated that mushroom polysaccharides could modulate the insulin signaling pathway to display hypoglycemic and hypolipidemic effects. Regulation in mRNA and protein levels and phosphorylation of many key molecules such as insulin receptor substrate (IRS), protein kinase B (PKB), phosphatidylinositol-3-kinase (PI3K), protein kinase B (Akt), Jun N-terminal Kinase 1 (JNK), MAPK, and fork-head box O family proteins (FOXO) involved in insulin signaling pathway were observed in diabetic animals treated with polysaccharides extracted from a number of mushrooms such as Grifola frondosa, L. edodes, P. linteus, and Ophiocordyceps sinensis (18, 123–125). Lowered blood glucose level, increased glycogen level, and amelioration in damages to tissues were observed as results of treatments as well as improved insulin intolerance. Mushroom polysaccharides might directly interact with insulin receptor or indirectly interplay with insulin signaling through NF-κB pathway and subsequently stimulate cascade signaling to promote glucose uptake of cells. A heteropolysaccharides obtained from G. frondosa with an average molecular weight of 66.1 kDa significantly upregulate glucose transporter 4 to improve glucose uptake in insulin resistant HepG2 cell induced by dexamethasone through activating insulin receptor substrate 1 (IRS-1)–PI3K–c-JNK signaling pathway (124). Alkaline soluble polysaccharides derived from the fruiting bodies of Amillariella mellea, Gomphidius rutilus, and Agrocybe cylindracea, respectively, showed better promotion of glucose uptake than those from Hypsizygus marmoreus, Pleurotus eryngii, and P. ostreatus extracted with the same method in Hepa1–6 cells insulin resistance model through activating insulin receptor (IR)-AKT signaling pathway (26). However, there is still a great gap in understanding the detailed interrelation between the intervention of mushroom polysaccharides and the regulation of insulin signaling pathway (Figure 6). Therefore, more works are needed to bridge the gap and reveal the intricate interactions of intracellular signaling pathways within cells, including insulin signaling pathway, MAPK/NF-κB pathway, and oxidative stress-related signaling pathways. The progress in understanding the molecular mechanism of mushroom polysaccharides in modulating insulin signaling pathway would provide a basis for developing targeted therapy for the prevention and management of DM.
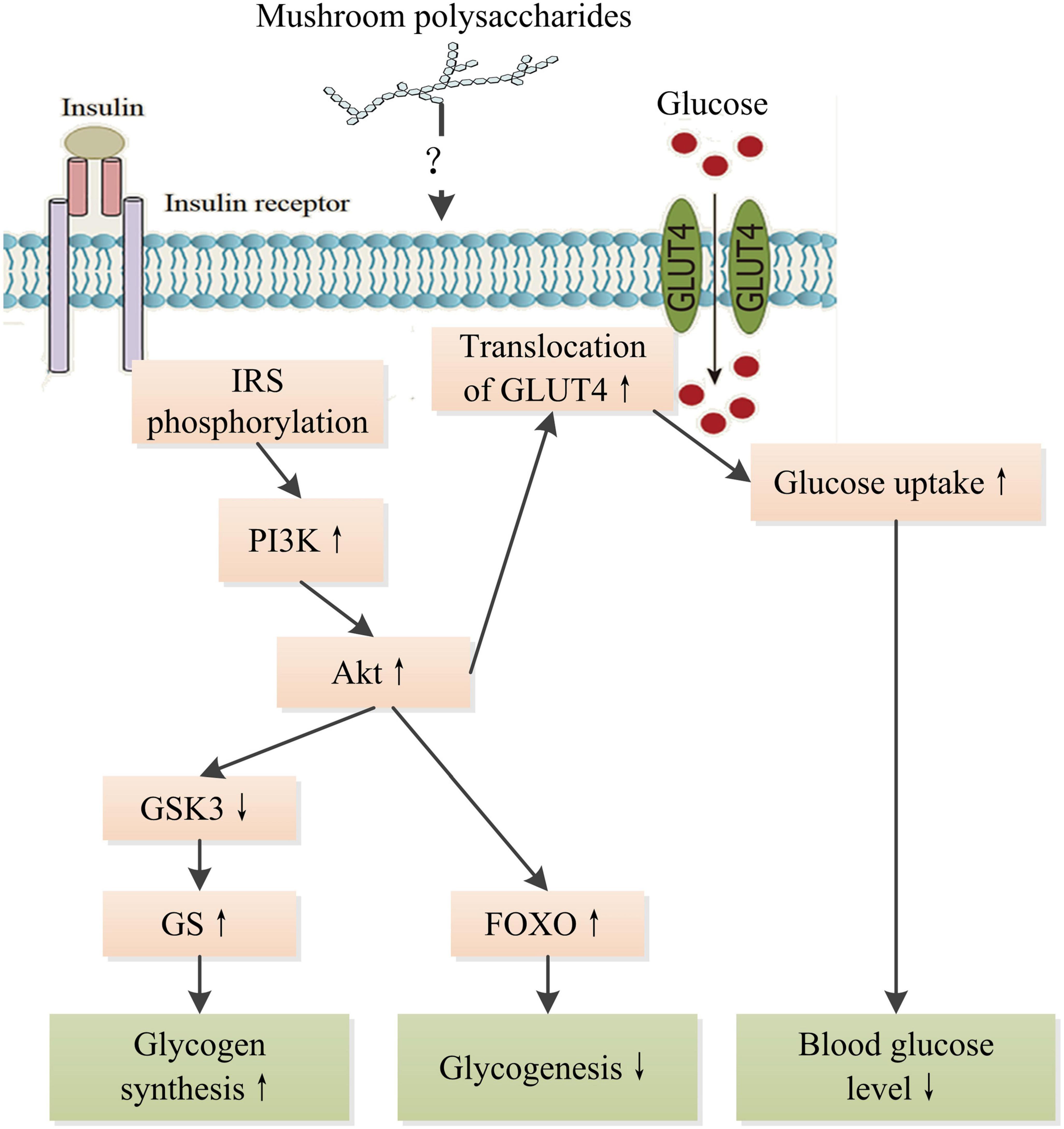
Figure 6. Schematic representation of the hypoglycemic mechanism of mushroom polysaccharides by modulating IR signaling pathway. IR, insulin receptor; IRS, insulin receptor substrate; PI3K, phosphatetidylinostitol-3-kinase; Akt, protein kinase B; GSK3, glycogen synthesis kinase 3; GS, glycogen synthase; GLUT4, glucose transporter 4; FOXO, fork-head box O family proteins. ↑ Indicates increase and ↓ indicates decrease.
The present state of knowledge on the structures of mushroom polysaccharides and their biological functions of anti-diabetes is limited. The successful development and application of mushroom polysaccharides in the anti-diabetic field requires illustrating the structure-activity relationship of mushroom polysaccharides. Considering the great variability in monosaccharide composition, molecular weight (molecule size), glycosidic linkage, and conformation of mushroom polysaccharides within individual species, not all mushroom polysaccharides contained in mushrooms exhibit equivalently therapeutic activity. For example, six alkaline soluble polysaccharides extracted from the fruiting bodies of A. mellea, G. rutilus, A. cylindracea, H. marmoreus, P. eryngii, and P. ostreatus, respectively, showed differential improvement of insulin sensitivity in the Hepa1–6 insulin resistance cell model, suggesting that the specific structure of polysaccharides could play an important role in modulating insulin sensitization (26). However, there is insufficient data to conclude which structural characteristics are key determinants of mushroom polysaccharides in anti-diabetes, and which type of mushroom polysaccharides might have better effects. With the expanding exploration of mushrooms, more and more mushroom species are found and identified. Given the great potential of mushroom diversity, and structural complicity and diversity of mushroom polysaccharides, the current lacking of internationally recognized standard protocols for the testing anti-diabetic activity would bring considerable challenges to their translational applications for scientists worldwide. Future studies assessing the efficacy of mushroom polysaccharides with precise structural characterization in well-recognized models for the prevention and/or treatment of DM are needed. Furthermore, deep uncovering the anti-diabetic mechanisms using modern technologies, including metagenomics, proteomics, metabolomics, and transcriptomics, would expand our understanding of the biological and pharmacological properties of mushroom polysaccharides. These would lead to the successful development of therapeutic agents in managing DM.
There has been an increasing interest in developing synergic foods as functional foods or nutraceuticals, which have advantages in stronger health benefits and convenience for the management of non-transmissible chronic diseases (126). Besides mushroom polysaccharides, other active substances such as minerals and trace elements also have beneficial roles in insulin resistance and DM (127). Thus, mushroom polysaccharides combined with other active components aiming at synergistic effects would provide a potentially powerful approach to preventing and treating DM and its complications. Mushroom polysaccharides show a good metal ion chelating ability (43). Some metal ions are vital for metabolism. For example, chromium (III) is an essential trace element and plays a vital role in maintaining insulin sensitivity and glucose homeostasis in the human body (128). Polysaccharides-metal ion complexes to improve the therapeutic activities in anti-diabetes have been conducted. For instance, chromium (Cr)-G. frondosa-derived polysaccharides complexes have been synthesized and evaluated in terms of their anti-hyperlipidemic and anti-hyperglycemic effects in HFD- and STZ-induced diabetic mice. The Cr-polysaccharides complex showed better activities in reducing blood glucose level, improving liver glycogen synthesis, and decreasing serum TC and TG than polysaccharides alone, suggesting outstanding therapeutic actions of the complex in improving glucose and lipid metabolism in diabetes (129). Selenium (Se) is a vital micronutrient and plays a crucial role in redox homeostasis in the body (130). Se-rich polysaccharides extracted from the fruiting body of C. militaris exhibited stronger anti-inflammation and anti-lipidemic properties than the same source of Se-deficient polysaccharides (29). Furthermore, a combination of inulin and G. lucidum polysaccharides displayed better activities in improving insulin sensitivity, increasing glycogen synthesis, and ameliorating lipid metabolism than inulin or G. lucidum polysaccharides alone in T2DM rats, demonstrating synergistic actions of inulin and G. lucidum in anti-diabetes (131).
In addition, active polysaccharides combined with nano-and micro-technological strategies or functionalized with specific molecules would improve their efficacy in anti-diabetes through targeting delivery and/or increasing intestinal permeability (132). A polysaccharide-based micelle-hydrogel containing insulin and nattokinase was developed. This synergistic therapy system not only possesses glucose-responsive insulin delivery properties, but also provides good thrombolytic capacity (133). Se-C. ventricosum-derived polysaccharides nanoparticles exhibited significantly higher anti-diabetic effects than Se nanoparticles alone (134). Therefore, a synergistic formula composed of multiple mushroom polysaccharides and/or other active components with different pharmacological properties by targeting multiple action pathways combined with efficient delivery technology would synergistically improve the outcomes in the prevention and management of DM and its complications.
Increasing studies focus on the interventions of mushroom polysaccharides in diabetic animal model. However, little attention has been paid to the pharmacological actions of mushroom polysaccharides in DM patients. Available data on human mainly use mushroom powder or mushroom extracts. In a single-blind trial, 24 T2DM patients were randomized for consumption of 50 mg/kg BW of dried and powdered P. ostreatus or Pleurotus cystidiosus, respectively (135). Both mushroom interventions effectively reduced postprandial serum glucose levels and increased postprandial serum insulin levels of diabetes patients. Twenty-two subjects with impaired glucose tolerance consumed a meal either fortified with 20 g of dried P. ostreatus powder (contained 8.1 g β-glucan) or without enrichment, a fortified meal improved the levels of postprandial GLP-1 and non-esterified free fatty acids compared to control meal in a double-blind, randomized controlled crossover trial, suggesting the beneficial effects of mushrooms β-glucan in improving postprandial metabolism for peoples with a high risk of development of T2DM (136). A possible reason might be that it is tough and time-consuming to prepare a sufficient amount of purified mushroom polysaccharide used in clinical trials. This obstacle requires cooperation between institutes and industrial companies. Further studies and well-controlled clinical trials should be conducted in this context to develop the most reliable anti-diabetic agents screened from mushroom polysaccharides.
Author contributions
XL and DL: conceptualization, data curation, investigation, visualization, and writing—original draft, review, and editing. JG and JC: writing—original draft, review, and editing. XX: conceptualization, supervision, methodology, formal analysis, project administration, funding acquisition, resources, and writing review and editing. All authors contributed to the article and approved the submitted version.
Funding
This work was partly supported by the program for scientific research start-up funds of Guangdong Ocean University.
Conflict of interest
The authors declare that the research was conducted in the absence of any commercial or financial relationships that could be construed as a potential conflict of interest.
Publisher’s note
All claims expressed in this article are solely those of the authors and do not necessarily represent those of their affiliated organizations, or those of the publisher, the editors and the reviewers. Any product that may be evaluated in this article, or claim that may be made by its manufacturer, is not guaranteed or endorsed by the publisher.
References
1. Wasser S. Medicinal mushroom science: current perspectives, advances, evidences, and challenges. Biomed J. (2014). 37:345–56. doi: 10.4103/2319-4170.138318
2. Royse D, Baars J, Tan Q. Current overview of mushroom production in the world. In: Diego CZ, Pardo-Giménez A editors. Edible and medicinal mushrooms. Hoboken, NJ: Wiley-Blackwell (2017). p. 5–13.
3. Wong J, Ng T, Chan H, Liu Q, Man G, Zhang C, et al. Mushroom extracts and compounds with suppressive action on breast cancer: evidence from studies using cultured cancer cells, tumor-bearing animals, and clinical trials. Appl Microbiol Biot. (2020) 104:4675–703. doi: 10.1007/s00253-020-10476-4
4. Muszyńska B, Grzywacz-Kisielewska A, Kała K, Gdula-Argasińska J. Anti-inflammatory properties of edible mushrooms: a review. Food Chem. (2018) 243:373–81. doi: 10.1016/j.foodchem.2017.09.149
5. Motta F, Gershwin M, Selmi C. Mushrooms and immunity. J Autoimmun. (2021) 117:102576. doi: 10.1016/j.jaut.2020.102576
6. Tung Y, Pan C, Chien Y, Huang H. Edible mushrooms: novel medicinal agents to combat metabolic syndrome and associated diseases. Curr Pharm Des. (2020) 26:4970–81. doi: 10.2174/1381612826666200831151316
7. Rahman M, Abdullah N, Aminudin N. Interpretation of mushroom as a common therapeutic agent for Alzheimer’s disease and cardiovascular diseases. Crit Rev Biotechnol. (2016) 36:1131–42. doi: 10.3109/07388551.2015.1100585
8. Shahzad F, Anderson D, Najafzadeh M. The antiviral, anti-inflammatory effects of natural medicinal herbs and mushrooms and Sars-Cov-2 infection. Nutrients. (2020) 12:2573. doi: 10.3390/nu12092573
9. Kalaè P. A review of chemical composition and nutritional value of wild-growing and cultivated mushrooms. J Sci Food Agric. (2013) 93:209–18. doi: 10.1002/jsfa.5960
10. Huang X, Nie S. The structure of mushroom polysaccharides and their beneficial role in health. Food Funct. (2015) 6:3205–17. doi: 10.1039/c5fo00678c
11. Kerner W, Brückel J. Definition, classification and diagnosis of diabetes mellitus. Exp Clin Endocr Diabetes. (2014) 122:384–6. doi: 10.1055/s-0034-1366278
12. American Diabetes Association. 2. Classification and diagnosis of diabetes: dtandards of medical care in diabetes-2021. Diabetes Care. (2021) 44(Suppl. 1):S15–33. doi: 10.2337/dc21-S002
13. Schmidt A. Highlighting diabetes mellitus: the epidemic continues. Arterioscl Throm Vasc. (2018) 38:e1–8. doi: 10.1161/atvbaha.117.310221
14. Khan M, Hashim M, King J, Govender R, Mustafa H, Al Kaabi J. Epidemiology of type 2 diabetes - global burden of disease and forecasted trends. J Epidemiol Glob Health. (2020) 10:107–11. doi: 10.2991/jegh.k.191028.001
15. Chaudhury A, Duvoor C, Reddy Dendi V, Kraleti S, Chada A, Ravilla R, et al. Clinical review of antidiabetic drugs: implications for type 2 diabetes mellitus management. Front Endocrinol. (2017) 8:6. doi: 10.3389/fendo.2017.00006
16. Chowdhury P, Paul S. The potential role of mushrooms in the prevention and treatment of diabetes: a review. J Biol Act Prod Nat. (2020) 10:429–54. doi: 10.1080/22311866.2020.1831958
17. Wu L, Gao Y, Su Y, Li J, Ren W, Wang Q, et al. Probiotics with anti-type 2 diabetes mellitus properties: targets of polysaccharides from traditional Chinese medicine. Chin J Nat Med. (2022) 20:641–55. doi: 10.1016/S1875-5364(22)60210-3
18. Khursheed R, Singh S, Wadhwa S, Gulati M, Awasthi A. Therapeutic potential of mushrooms in diabetes mellitus: role of polysaccharides. Int J Biol Macromol. (2020) 164:1194–205. doi: 10.1016/j.ijbiomac.2020.07.145
19. Jiang X, Meng W, Li L, Meng Z, Wang D. Adjuvant therapy with mushroom polysaccharides for diabetic complications. Front Pharmacol. (2020) 11:168. doi: 10.3389/fphar.2020.00168
20. Arunachalam K, Sreeja P, Yang X. The antioxidant properties of mushroom polysaccharides can potentially mitigate oxidative stress, beta-cell dysfunction and insulin resistance. Front Pharmacol. (2022) 13:874474. doi: 10.3389/fphar.2022.874474
21. Zhang B, Pan C, Feng C, Yan C, Yu Y, Chen Z, et al. Role of mitochondrial reactive oxygen species in homeostasis regulation. Redox Rep. (2022) 27:45–52. doi: 10.1080/13510002.2022.2046423
22. Bashan N, Kovsan J, Kachko I, Ovadia H, Rudich A. Positive and negative regulation of insulin signaling by reactive oxygen and nitrogen species. Physiol Rev. (2009) 89:27–71. doi: 10.1152/physrev.00014.2008
23. Asmat U, Abad K, Ismail K. Diabetes mellitus and oxidative stress—a concise review. Saudi Pharm J. (2016) 24:547–53. doi: 10.1016/j.jsps.2015.03.013
24. Ganesan K, Xu B. Anti-diabetic effects and mechanisms of dietary polysaccharides. Molecules. (2019) 24:2556.
25. Xiang H, Sun-Waterhouse D, Cui C. Hypoglycemic polysaccharides from Auricularia auricula and Auricularia polytricha inhibit oxidative stress, NF-κB signaling and proinflammatory cytokine production in streptozotocin-induced diabetic mice. Food Sci Hum Wellness. (2021) 10:87–93. doi: 10.1016/j.fshw.2020.06.001
26. Yang S, Yan J, Yang L, Meng Y, Wang N, He C, et al. Alkali-soluble polysaccharides from mushroom fruiting bodies improve insulin resistance. Int J Biol Macromol. (2019) 126:466–74. doi: 10.1016/j.ijbiomac.2018.12.251
27. Sun W, Zhang Y, Jia L. Polysaccharides from Agrocybe cylindracea residue alleviate type 2-diabetes-induced liver and colon injuries by P38 MAPK signaling pathway. Food Biosci. (2022) 47:101690. doi: 10.1016/j.fbio.2022.101690
28. Yin F, Lin P, Yu W, Shen N, Li Y, Guo S. The Cordyceps militaris-derived polysaccharide CM1 alleviates atherosclerosis in Ldlr(-/-) mice by improving hyperlipidemia. Front Mol Biosci. (2021) 8:783807. doi: 10.3389/fmolb.2021.783807
29. Yu M, Yue J, Hui N, Zhi Y, Hayat K, Yang X, et al. Anti-hyperlipidemia and gut microbiota community regulation effects of selenium-rich Cordyceps militaris polysaccharides on the high-fat diet-fed mice model. Foods. (2021) 10:2252. doi: 10.3390/foods10102252
30. Kanwal S, Aliya S, Xin Y. Anti-obesity effect of Dictyophora indusiata mushroom polysaccharide (DIP) in high fat diet-induced obesity via regulating inflammatory cascades and intestinal microbiome. Front Endocrinol. (2020) 11:558874. doi: 10.3389/fendo.2020.558874
31. Cai W, Ding Z, Wang Y, Yang Y, Zhang H, Yan J. Hypoglycemic benefit and potential mechanism of a polysaccharide from Hericium erinaceus in streptozotoxin-induced diabetic rats. Process Biochem. (2020) 88:180–8. doi: 10.1016/j.procbio.2019.09.035
32. Su L, Xin C, Yang J, Dong L, Mei H, Dai X, et al. A polysaccharide from Inonotus obliquus ameliorates intestinal barrier dysfunction in mice with type 2 diabetes mellitus. Int J Biol Macromol. (2022) 214:312–23. doi: 10.1016/j.ijbiomac.2022.06.071
33. Wu R, Wang L, Yao Y, Sang T, Wu Q, Fu W, et al. Activity fingerprinting of polysaccharides on oral, gut, pancreas and lung microbiota in diabetic rats. Biomed Pharmacother. (2022) 155:113681. doi: 10.1016/j.biopha.2022.113681
34. Chen M, Xiao D, Liu W, Song Y, Zou B, Li L, et al. Intake of Ganoderma lucidum polysaccharides reverses the disturbed gut microbiota and metabolism in type 2 diabetic rats. Int J Biol Macromol. (2020) 155:890–902. doi: 10.1016/j.ijbiomac.2019.11.047
35. Sang T, Guo C, Guo D, Wu J, Wang Y, Wang Y, et al. Suppression of obesity and inflammation by polysaccharide from sporoderm-broken spore of Ganoderma lucidum via gut microbiota regulation. Carbohydr Polym. (2021) 256:117594. doi: 10.1016/j.carbpol.2020.117594
36. Guo W, Deng J, Pan Y, Xu J, Hong J, Shi F, et al. Hypoglycemic and hypolipidemic activities of Grifola frondosa polysaccharides and their relationships with the modulation of intestinal microflora in diabetic mice induced by high-fat diet and streptozotocin. Int J Biol Macromol. (2020) 153:1231–40. doi: 10.1016/j.ijbiomac.2019.10.253
37. Jiang X, Hao J, Zhu Y, Liu Z, Li L, Zhou Y, et al. The anti-obesity effects of a water-soluble glucan from Grifola frondosa via the modulation of chronic inflammation. Front Immunol. (2022) 13:962341. doi: 10.3389/fimmu.2022.962341
38. Wang T, Han J, Dai H, Sun J, Ren J, Wang W, et al. Polysaccharides from Lyophyllum decastes reduce obesity by altering gut microbiota and increasing energy expenditure. Carbohyd Polym. (2022) 295:119862. doi: 10.1016/j.carbpol.2022.119862
39. Liu Y, Wang C, Li J, Li T, Zhang Y, Liang Y, et al. Phellinus linteus polysaccharide extract improves insulin resistance by regulating gut microbiota composition. FASEB J. (2020) 34:1065–78. doi: 10.1096/fj.201901943RR
40. Chen J, Liu J, Yan C, Zhang C, Pan W, Zhang W, et al. Sarcodon aspratus polysaccharides ameliorated obesity-induced metabolic disorders and modulated gut microbiota dysbiosis in mice fed a high-fat diet. Food Funct. (2020) 11:2588–602. doi: 10.1039/c9fo00963a
41. Liu Y, Liu Y, Zhang M, Li C, Zhang Z, Liu A, et al. Structural characterization of a polysaccharide from Suillellus luridus and its antidiabetic activity via Nrf2/Ho-1 and Nf-κB pathways. Int J Biol Macromol. (2020) 162:935–45. doi: 10.1016/j.ijbiomac.2020.06.212
42. Niu B, Feng S, Xuan S, Shao P. Moisture and caking resistant Tremella fuciformis polysaccharides microcapsules with hypoglycemic activity. Food Res Int. (2021) 146:110420. doi: 10.1016/j.foodres.2021.110420
43. Mwangi R, Macharia J, Wagara I, Bence R. The antioxidant potential of different edible and medicinal mushrooms. Biomed Pharmacother. (2022) 147:112621. doi: 10.1016/j.biopha.2022.112621
44. Yan J, Zhu L, Qu Y, Qu X, Mu M, Zhang M, et al. Analyses of active antioxidant polysaccharides from four edible mushrooms. Int J Biol Macromol. (2019) 123:945–56. doi: 10.1016/j.ijbiomac.2018.11.079
45. Heinrich M, Appendino G, Efferth T, Fürst R, Izzo A, Kayser O, et al. Best practice in research – overcoming common challenges in phytopharmacological research. J Ethnopharmacol. (2020) 246:112230. doi: 10.1016/j.jep.2019.112230
46. Xu X, Luo D, Zhao M, Zhong X. Comparison of anti-aging effects between two Tremella fuciformis polysaccharides. Acta Edulis Fungi. (2022) 38:93–102. doi: 10.16488/j.cnki.1005-9873.2022.02.011
47. Chen S, Guan X, Yong T, Gao X, Xiao C, Xie Y, et al. Structural characterization and hepatoprotective activity of an acidic polysaccharide from Ganoderma lucidum. Food Chem X. (2022) 13:100204. doi: 10.1016/j.fochx.2022.100204
48. Wang W, Liu M, Zhang M, Sun W, Zhang J, Jia L. Agaricus blazei Murill polysaccharides alleviate oxidative stress and inflammatory responses against liver and lung injury. Food Biosci. (2022) 47:101645. doi: 10.1016/j.fbio.2022.101645
49. Forman H, Zhang H. Targeting oxidative stress in disease: promise and limitations of antioxidant therapy. Nat Rev Drug Discov. (2021) 20:689–709. doi: 10.1038/s41573-021-00233-1
50. Yang F, Xiu M, Yang S, Li X, Tuo W, Su Y, et al. Extension of Drosophila lifespan by Astragalus polysaccharide through a mechanism dependent on antioxidant and insulin/Igf-1 signaling. Evid Based Complementary Altern Med. (2021) 2021:6686748. doi: 10.1155/2021/6686748
51. Zou Y, Chen M, Fu Y, Zhu Z, Zhang Y, Paulsen B, et al. Characterization of an antioxidant pectic polysaccharide from Platycodon grandiflorus. Int J Biol Macromol. (2021) 175:473–80. doi: 10.1016/j.ijbiomac.2021.02.041
52. Vomund S, Schäfer A, Parnham M, Brüne B, von Knethen A. Nrf2, the master regulator of anti-oxidative responses. Int J Mol Sci. (2017) 18:2772. doi: 10.3390/ijms18122772
53. Li X, Gong H, Yang S, Yang L, Fan Y, Zhou Y. Pectic bee pollen polysaccharide from Rosa rugosa alleviates diet-induced hepatic steatosis and insulin resistance via induction of AMPK/mTOR-mediated autophagy. Molecules. (2017) 22:699. doi: 10.3390/molecules22050699
54. Lotta L, Gulati P, Day F, Payne F, Ongen H, van de Bunt M, et al. Integrative genomic analysis implicates limited peripheral adipose storage capacity in the pathogenesis of human insulin resistance. Nat Genet. (2017) 49:17–26. doi: 10.1038/ng.3714
55. Nguyen P, Leray V, Diez M, Serisier S, Le Bloc’h J, Siliart B, et al. Liver lipid metabolism. J Anim Physiol Anim Nutr. (2008) 92:272–83. doi: 10.1111/j.1439-0396.2007.00752.x
56. Sears B, Perry M. The role of fatty acids in insulin resistance. Lipids Health Dis. (2015) 14:121. doi: 10.1186/s12944-015-0123-1
57. Yan Y, Ma R, Zhang J, He J, Ma J, Pang H, et al. Association of insulin resistance with glucose and lipid metabolism: ethnic heterogeneity in far western China. Mediators Inflamm. (2016) 2016:3825037. doi: 10.1155/2016/3825037
58. Caz V, Gil-Ramírez A, Largo C, Tabernero M, Santamaría M, Martín-Hernández R, et al. Modulation of cholesterol-related gene expression by dietary fiber fractions from edible mushrooms. J Agric Food Chem. (2015) 63:7371–80. doi: 10.1021/acs.jafc.5b02942
59. Huang Z, Zhang M, Wang Y, Zhang S, Jiang X. Extracellular and intracellular polysaccharide extracts of Trametes versicolor improve lipid profiles via serum regulation of lipid-regulating enzymes in hyperlipidemic mice. Curr Microbiol. (2020) 77:3526–37. doi: 10.1007/s00284-020-02156-3
60. Nie Y, Luo F. Dietary fiber: an opportunity for a global control of hyperlipidemia. Oxid Med Cell Longev. (2021) 2021:5542342. doi: 10.1155/2021/5542342
61. Lindequist U, Haertel B. Medicinal mushrooms for treatment of type 2 diabetes: an update on clinical trials. Int J Med Mushrooms. (2020) 22:845–54. doi: 10.1615/IntJMedMushrooms.2020035863
62. Xu Y, Zhang X, Yan X, Zhang J, Wang L, Xue H, et al. Characterization, hypolipidemic and antioxidant activities of degraded polysaccharides from Ganoderma lucidum. Int J Biol Macromol. (2019) 135:706–16. doi: 10.1016/j.ijbiomac.2019.05.166
63. He F, Huang Y, Song Z, Zhou H, Zhang H, Perry R, et al. Mitophagy-mediated adipose inflammation contributes to type 2 diabetes with hepatic insulin resistance. J Exp Med. (2021) 218:e20201416. doi: 10.1084/jem.20201416
64. Ellulu M, Patimah I, Khaza’ai H, Rahmat A, Abed Y. Obesity and inflammation: the linking mechanism and the complications. Arch Med Sci. (2017) 13:851–63. doi: 10.5114/aoms.2016.58928
65. Martel J, Chang S, Ko Y, Hwang T, Young J, Ojcius D. Gut barrier disruption and chronic disease. Trends Endocrin Metab. (2022) 33:247–65. doi: 10.1016/j.tem.2022.01.002
66. Yin C, Noratto G, Fan X, Chen Z, Yao F, Shi D, et al. The impact of mushroom polysaccharides on gut microbiota and its beneficial effects to host: a review. Carbohyd Polym. (2020) 250:116942. doi: 10.1016/j.carbpol.2020.116942
67. Zhang J, Liu D, Wen C, Liu J, Xu X, Liu G, et al. New light on Grifola frondosa polysaccharides as biological response modifiers. Trends Food Sci Technol. (2022) 119:565–78. doi: 10.1016/j.tifs.2021.11.017
68. Chakraborty N, Banerjee A, Sarkar A, Ghosh S, Krishnendu A. Mushroom polysaccharides: a potent immune-modulator. Biointerface Res Appl Chem. (2021) 11:8915–8930. doi: 10.33263/BRIAC112.89158930
69. Xu X, Yan H, Tang J, Chen J, Zhang X. Polysaccharides in Lentinus edodes: isolation, structure, immunomodulating activity and future prospective. Crit Rev Food Sci Nutr. (2014) 54:474–87. doi: 10.1080/10408398.2011.587616
70. Lee J, Kwon D, Lee K, Park J, Ha S, Hong E. Mechanism of macrophage activation induced by polysaccharide from Cordyceps militaris culture broth. Carbohyd Polym. (2015) 120:29–37. doi: 10.1016/j.carbpol.2014.11.059
71. Hou C, Chen L, Yang L, Ji X. An insight into anti-inflammatory effects of natural polysaccharides. Int J Biol Macromol. (2020) 153:248–55. doi: 10.1016/j.ijbiomac.2020.02.315
72. Ruan Y, Li H, Pu L, Shen T. Tremella fuciformis polysaccharides attenuate oxidative stress and inflammation in macrophages through mi-155. Anal Cell Pathol. (2018) 2018:5762371. doi: 10.1155/2018/5762371
73. Gudi R, Suber J, Brown R, Johnson B, Vasu C. Pretreatment with yeast-derived complex dietary polysaccharides suppresses gut inflammation, alters the microbiota composition, and increases immune regulatory short-chain fatty acid production in C57bl/6 mice. J Nutr. (2020) 150:1291–302. doi: 10.1093/jn/nxz328
74. Cohen-Kedar S, Baram L, Elad H, Brazowski E, Guzner-Gur H, Dotan I. Human intestinal epithelial cells respond to β-glucans via dectin-1 and Syk. Eur J Immunol. (2014) 44:3729–40. doi: 10.1002/eji.201444876
75. Price A, Shamardani K, Lugo K, Deguine J, Roberts A, Lee B, et al. A map of Toll-like receptor expression in the intestinal epithelium reveals distinct spatial, cell type-specific, and temporal patterns. Immunity. (2018) 49:560–75.e6. doi: 10.1016/j.immuni.2018.07.016
76. Mata-Martínez P, Bergón-Gutiérrez M, Del Fresno C. Dectin-1 signaling update: new perspectives for trained immunity. Front Immunol. (2022) 13:812148. doi: 10.3389/fimmu.2022.812148
77. Suga Y, Matsunaga Y, Satoh Y, Murata M, Suga T. The importance of size for anti-tumor effects of β-glucan. Biotherapy. (2005) 19:273–8.
78. Kayama H, Okumura R, Takeda K. Interaction between the microbiota, epithelia, and immune cells in the intestine. Annu Rev Immunol. (2020) 38:23–48. doi: 10.1146/annurev-immunol-070119-115104
79. Xu Y, Xie L, Zhang Z, Zhang W, Tang J, He X, et al. Tremella fuciformis polysaccharides inhibited colonic inflammation in dextran sulfate sodium-treated mice via Foxp3+ T cells, gut microbiota, and bacterial metabolites. Front Immunol. (2021) 12:648162. doi: 10.3389/fimmu.2021.648162
80. Meng M, Zhang R, Han R, Kong Y, Wang R, Hou L. The polysaccharides from the Grifola frondosa fruiting body prevent lipopolysaccharide/D-galactosamine-induced acute liver injury via the miR-122-Nrf2/ARE pathways. Food Funct. (2021) 12:1973–82. doi: 10.1039/d0fo03327h
81. Saha S, Buttari B, Panieri E, Profumo E, Saso L. An overview of Nrf2 signaling pathway and its role in inflammation. Molecules. (2020) 25:5474. doi: 10.3390/molecules25225474
82. Chen L, Zhang L, Wang W, Qiu W, Liu L, Ning A, et al. Polysaccharides isolated from Cordyceps sinensis contribute to the progression of NASH by modifying the gut microbiota in mice fed a high-fat diet. PLoS One. (2020) 15:e0232972. doi: 10.1371/journal.pone.0232972
83. Li W, Stirling K, Yang J, Zhang L. Gut microbiota and diabetes: from correlation to causality and mechanism. World J Diabetes. (2020) 11:293–308. doi: 10.4239/wjd.v11.i7.293
84. Larsen N, Vogensen F, van den Berg F, Nielsen D, Andreasen A, Pedersen B, et al. Gut microbiota in human adults with type 2 diabetes differs from non-diabetic adults. PLoS One. (2010) 5:e9085. doi: 10.1371/journal.pone.0009085
85. Gavin P, Hamilton-Williams E. The gut microbiota in type 1 diabetes: friend or foe? Curr Opin Endocrinol Diabetes Obes. (2019) 26:207–12. doi: 10.1097/med.0000000000000483
86. Allin K, Tremaroli V, Caesar R, Jensen B, Damgaard M, Bahl M, et al. Aberrant intestinal microbiota in individuals with prediabetes. Diabetologia. (2018) 61:810–20. doi: 10.1007/s00125-018-4550-1
87. Liu Y, Xu Z, Huang H, Xue Y, Zhang D, Zhang Y, et al. Fucoidan ameliorates glucose metabolism by the improvement of intestinal barrier and inflammatory damage in type 2 diabetic rats. Int J Biol Macromol. (2022) 201:616–29. doi: 10.1016/j.ijbiomac.2022.01.102
88. Bodogai M, O’Connell J, Kim K, Kim Y, Moritoh K, Chen C, et al. Commensal bacteria contribute to insulin resistance in aging by activating innate B1a cells. Sci Transl Med. (2018) 10:eaat4271. doi: 10.1126/scitranslmed.aat4271
89. Wang C, Wang Y, Yang H, Tian Z, Zhu M, Sha X, et al. Uygur type 2 diabetes patient fecal microbiota transplantation disrupts blood glucose and bile acid levels by changing the ability of the intestinal flora to metabolize bile acids in C57bl/6 mice. BMC Endocr Disord. (2022) 22:236. doi: 10.1186/s12902-022-01155-8
90. Nie C, He T, Zhang W, Zhang G, Ma X. Branched chain amino acids: beyond nutrition metabolism. Int J Mol Sci. (2018) 19:954. doi: 10.3390/ijms19040954
91. Vanweert F, Schrauwen P, Phielix E. Role of branched-chain amino acid metabolism in the pathogenesis of obesity and type 2 diabetes-related metabolic disturbances BCAA metabolism in type 2 diabetes. Nutr Diabetes. (2022) 12:35. doi: 10.1038/s41387-022-00213-3
92. Qiao S, Liu C, Sun L, Wang T, Dai H, Wang K, et al. Gut Parabacteroides merdae protects against cardiovascular damage by enhancing branched-chain amino acid catabolism. Nat Metab. (2022) 4:1271–86. doi: 10.1038/s42255-022-00649-y
93. Pedersen H, Gudmundsdottir V, Nielsen H, Hyotylainen T, Nielsen T, Jensen B, et al. Human gut microbes impact host serum metabolome and insulin sensitivity. Nature. (2016) 535:376–81. doi: 10.1038/nature18646
94. Pathak P, Xie C, Nichols R, Ferrell J, Boehme S, Krausz K, et al. Intestine farnesoid X receptor agonist and the gut microbiota activate G-protein bile acid receptor-1 signaling to improve metabolism. Hepatology. (2018) 68:1574–88. doi: 10.1002/hep.29857
95. Liu L, Zhang J, Cheng Y, Zhu M, Xiao Z, Ruan G, et al. Gut microbiota: a new target for T2DM prevention and treatment. Front Endocrinol. (2022) 13:958218. doi: 10.3389/fendo.2022.958218
96. Temple M, Cuskin F, Baslé A, Hickey N, Speciale G, Williams S, et al. A Bacteroidetes locus dedicated to fungal 1,6-β-glucan degradation: unique substrate conformation drives specificity of the key endo-1,6-β-glucanase. J Biol Chem. (2017) 292:10639–50. doi: 10.1074/jbc.M117.787606
97. Zheng M, Pi X, Li H, Cheng S, Su Y, Zhang Y, et al. Ganoderma spp. polysaccharides are potential prebiotics: a review. Crit Rev Food Sci Nutr. (2022). [Epub ahead of print]. doi: 10.1080/10408398.2022.2110035
98. Yin C, Qiao X, Fan X, Chen Z, Yao F, Shi D, et al. Differences of gut microbiota composition in mice supplied with polysaccharides from γ-irradiated and non-irradiated schizophyllum commune. Food Res Int. (2022) 151:110855. doi: 10.1016/j.foodres.2021.110855
99. Luan F, Peng X, Zhao G, Zeng J, Zou J, Rao Z, et al. Structural diversity and bioactivity of polysaccharides from medicinal mushroom Phellinus spp.: a review. Food Chem. (2022) 397:133731. doi: 10.1016/j.foodchem.2022.133731
100. Rashidi A, Ebadi M, Rehman T, Elhusseini H, Nalluri H, Kaiser T, et al. Gut microbiota response to antibiotics is personalized and depends on baseline microbiota. Microbiome. (2021) 9:211. doi: 10.1186/s40168-021-01170-2
101. Zhao R, Hu Q, Ma G, Su A, Xie M, Li X, et al. Effects of Flammulina velutipes polysaccharide on immune response and intestinal microbiota in mice. J Funct Foods. (2019) 56:255–64. doi: 10.1016/j.jff.2019.03.031
102. Ye J, Wang X, Wang K, Deng Y, Yang Y, Ali R, et al. A novel polysaccharide isolated from Flammulina velutipes, characterization, macrophage immunomodulatory activities and its impact on gut microbiota in rats. J Anim Physiol Anim Nutr. (2020) 104:735–48. doi: 10.1111/jpn.13290
103. Xu X, Yang J, Ning Z, Zhang X. Lentinula edodes-derived polysaccharide rejuvenates mice in terms of immune responses and gut microbiota. Food Funct. (2015) 6:2653–63. doi: 10.1039/c5fo00689a
104. Xu X, Zhang X. Lentinula edodes-derived polysaccharide alters the spatial structure of gut microbiota in mice. PLoS One. (2015) 10:e0115037. doi: 10.1371/journal.pone.0115037
105. Ericsson A, Gagliardi J, Bouhan D, Spollen W, Givan S, Franklin C. The influence of caging, bedding, and diet on the composition of the microbiota in different regions of the mouse gut. Sci Rep. (2018) 8:4065. doi: 10.1038/s41598-018-21986-7
106. Sawangwan T, Wansanit W, Pattani L, Noysang C. Study of prebiotic properties from edible mushroom extraction. Agri Nat Resour. (2018) 52:519–24. doi: 10.1016/j.anres.2018.11.020
107. Inyod T, Ayimbila F, Payapanon A, Keawsompong S. Antioxidant activities and prebiotic properties of the tropical mushroom Macrocybe crassa. Bioact Carbohydr Diet Fibre. (2022) 27:100298. doi: 10.1016/j.bcdf.2021.100298
108. Guo D, Lei J, He C, Peng Z, Liu R, Pan X, et al. In vitro digestion and fermentation by human fecal microbiota of polysaccharides from Clitocybe squamulose. Int J Biol Macromol. (2022) 208:343–55. doi: 10.1016/j.ijbiomac.2022.03.126
109. Xue Z, Ma Q, Chen Y, Lu Y, Wang Y, Jia Y, et al. Structure characterization of soluble dietary fiber fractions from mushroom Lentinula edodes (Berk.) Pegler and the effects on fermentation and human gut microbiota in vitro. Food Res Int. (2020) 129:108870. doi: 10.1016/j.foodres.2019.108870
110. Du B, Meenu M, Liu H, Xu B. A concise review on the molecular structure and function relationship of β-glucan. Int J Mol Sci. (2019) 20:4032. doi: 10.3390/ijms20164032
111. Zhang J, Wen C, Duan Y, Zhang H, Ma H. Advance in Cordyceps militaris (Linn) link polysaccharides: isolation, structure, and bioactivities: a review. Int J Biol Macromol. (2019) 132:906–14. doi: 10.1016/j.ijbiomac.2019.04.020
112. Ruthes A, Smiderle F, Iacomini M. D-glucans from edible mushrooms: a review on the extraction, purification and chemical characterization approaches. Carbohyd Polym. (2015) 117:753–61. doi: 10.1016/j.carbpol.2014.10.051
113. Shaheen T, Hussien G, Mekawey A, Ghalia H, Youssry A, El Mokadem M. Facile extraction of nanosized β-glucans from edible mushrooms and their antitumor activities. J Food Compos Anal. (2022) 111:104607. doi: 10.1016/j.jfca.2022.104607
114. da Silva Milhorini S, Simas F, Smiderle F, Inara de Jesus L, Rosado F, Longoria E, et al. β-glucans from the giant mushroom Macrocybe titans: chemical characterization and rheological properties. Food Hydrocoll. (2022) 125:107392. doi: 10.1016/j.foodhyd.2021.107392
115. Zhang H, Jiang F, Zhang J, Wang W, Li L, Yan J. Modulatory effects of polysaccharides from plants, marine algae and edible mushrooms on gut microbiota and related health benefits: a review. Int J Biol Macromol. (2022) 204:169–92. doi: 10.1016/j.ijbiomac.2022.01.166
116. Rodrigues Barbosa J, Dos Santos Freitas MM, da Silva Martins LH, de Carvalho RN Jr Polysaccharides of mushroom Pleurotus spp.: new extraction techniques, biological activities and development of new technologies. Carbohyd Polym. (2020) 229:115550. doi: 10.1016/j.carbpol.2019.115550
117. Leong Y, Yang F, Chang J. Extraction of polysaccharides from edible mushrooms: emerging technologies and recent advances. Carbohyd Polym. (2021) 251:117006. doi: 10.1016/j.carbpol.2020.117006
118. Gong P, Wang S, Liu M, Chen F, Yang W, Chang X, et al. Extraction methods, chemical characterizations and biological activities of mushroom polysaccharides: a mini-review. Carbohyd Res. (2020) 494:108037. doi: 10.1016/j.carres.2020.108037
119. Ferreira S, Passos C, Madureira P, Vilanova M, Coimbra M. Structure–function relationships of immunostimulatory polysaccharides: a review. Carbohyd Polym. (2015) 132:378–96. doi: 10.1016/j.carbpol.2015.05.079
120. Wu Y, Wei Z, Zhang F, Linhardt R, Sun P, Zhang A. Structure, bioactivities and applications of the polysaccharides from Tremella fuciformis mushroom: a review. Int J Biol Macromol. (2019) 121:1005–10. doi: 10.1016/j.ijbiomac.2018.10.117
121. Lin C, Tsai S. Differences in the moisture capacity and thermal stability of Tremella fuciformis polysaccharides obtained by various drying processes. Molecules. (2019) 24:2856. doi: 10.3390/molecules24152856
122. Wu D, He Y, Fu M, Gan R, Hu Y, Peng L, et al. Structural characteristics and biological activities of a pectic-polysaccharide from okra affected by ultrasound assisted metal-free Fenton reaction. Food Hydrocoll. (2022) 122:107085. doi: 10.1016/j.foodhyd.2021.107085
123. Chen Y, Liu D, Wang D, Lai S, Zhong R, Liu Y, et al. Hypoglycemic activity and gut microbiota regulation of a novel polysaccharide from Grifola frondosa in type 2 diabetic mice. Food Chem Toxicol. (2019) 126:295–302. doi: 10.1016/j.fct.2019.02.034
124. Chen Y, Liu Y, Sarker M, Yan X, Yang C, Zhao L, et al. Structural characterization and antidiabetic potential of a novel heteropolysaccharide from Grifola frondosa via IRS1/PI3K-JNK signaling pathways. Carbohyd Polym. (2018) 198:452–61. doi: 10.1016/j.carbpol.2018.06.077
125. Chen L, Zhang Y, Sha O, Xu W, Wang S. Hypolipidaemic and hypoglycaemic activities of polysaccharide from Pleurotus eryngii in Kunming mice. Int J Biol Macromol. (2016) 93:1206–9. doi: 10.1016/j.ijbiomac.2016.09.094
126. Natarajan T, Ramasamy J, Palanisamy K. Nutraceutical potentials of synergic foods: a systematic review. J Ethnic Foods. (2019) 6:27. doi: 10.1186/s42779-019-0033-3
127. Dubey P, Thakur V, Chattopadhyay M. Role of minerals and trace elements in diabetes and insulin resistance. Nutrients. (2020) 12:1864. doi: 10.3390/nu12061864
128. Tang H, Xiao Q, Xu H, Zhang Y. Hypoglycemic activity and acute oral toxicity of chromium methionine complexes in mice. J Trace Elem Med Biol. (2015) 29:136–44. doi: 10.1016/j.jtemb.2014.07.001
129. Guo W, Shi F, Li L, Xu J, Chen M, Wu L, et al. Preparation of a novel Grifola frondosa polysaccharide-chromium (III) complex and its hypoglycemic and hypolipidemic activities in high fat diet and streptozotocin-induced diabetic mice. Int J Biol Macromol. (2019) 131:81–8. doi: 10.1016/j.ijbiomac.2019.03.042
130. Labunskyy V, Hatfield D, Gladyshev V. Selenoproteins: molecular pathways and physiological roles. Physiol Rev. (2014) 94:739–77. doi: 10.1152/physrev.00039.2013
131. Liu Y, Li Y, Zhang W, Sun M, Zhang Z. Hypoglycemic effect of inulin combined with Ganoderma lucidum polysaccharides in T2DM rats. J Funct Foods. (2019) 55:381–90. doi: 10.1016/j.jff.2019.02.036
132. Meneguin A, Silvestre A, Sposito L, de Souza M, Sábio R, Araújo V, et al. The role of polysaccharides from natural resources to design oral insulin micro- and nanoparticles intended for the treatment of diabetes mellitus: a review. Carbohyd Polym. (2021) 256:117504. doi: 10.1016/j.carbpol.2020.117504
133. Wen N, Lü S, Xu X, Ning P, Wang Z, Zhang Z, et al. A polysaccharide-based micelle-hydrogel synergistic therapy system for diabetes and vascular diabetes complications treatment. Mater Sci Eng C Mater Biol Appl. (2019) 100:94–103. doi: 10.1016/j.msec.2019.02.081
134. Liu Y, Zeng S, Liu Y, Wu W, Shen Y, Zhang L, et al. Synthesis and antidiabetic activity of selenium nanoparticles in the presence of polysaccharides from Catathelasma ventricosum. Int J Biol Macromol. (2018) 114:632–9. doi: 10.1016/j.ijbiomac.2018.03.161
135. Jayasuriya W, Wanigatunge C, Fernando G, Abeytunga D, Suresh T. Hypoglycaemic activity of culinary Pleurotus Ostreatus and P. Cystidiosus mushrooms in healthy volunteers and type 2 diabetic patients on diet control and the possible mechanisms of action. Phytother Res. (2015) 29:303–9. doi: 10.1002/ptr.5255
136. Dicks L, Jakobs L, Sari M, Hambitzer R, Ludwig N, Simon M, et al. Fortifying a meal with oyster mushroom powder beneficially affects postprandial glucagon-like peptide-1, non-esterified free fatty acids and hunger sensation in adults with impaired glucose tolerance: a double-blind randomized controlled crossover trial. Eur J Nutr. (2022) 61:687–701. doi: 10.1007/s00394-021-02674-1
Keywords: oxidative stress, anti-oxidation, anti-lipidemia, anti-inflammation, gut microbiota, insulin resistance, structure-bioactivity relationship
Citation: Liu X, Luo D, Guan J, Chen J and Xu X (2022) Mushroom polysaccharides with potential in anti-diabetes: Biological mechanisms, extraction, and future perspectives: A review. Front. Nutr. 9:1087826. doi: 10.3389/fnut.2022.1087826
Received: 02 November 2022; Accepted: 29 November 2022;
Published: 14 December 2022.
Edited by:
Rui-Bo Jia, South China University of Technology, ChinaReviewed by:
Rongkang Hu, Anhui Normal University, ChinaZirui Huang, Shanghai Jiao Tong University, China
Copyright © 2022 Liu, Luo, Guan, Chen and Xu. This is an open-access article distributed under the terms of the Creative Commons Attribution License (CC BY). The use, distribution or reproduction in other forums is permitted, provided the original author(s) and the copyright owner(s) are credited and that the original publication in this journal is cited, in accordance with accepted academic practice. No use, distribution or reproduction is permitted which does not comply with these terms.
*Correspondence: Xiaofei Xu, eGZ4dWZlQGdkb3UuZWR1LmNu
†These authors have contributed equally to this work and share first authorship