- 1Department of Nutrition and Toxicology, School of Public Health, Hangzhou Normal University, Hangzhou, China
- 2Key Laboratory of Elemene Class Anti-Cancer Chinese Medicines of Zhejiang Province, Hangzhou Normal University, Hangzhou, China
Background: Mental disorders account for an enormous global burden of disease, and has been associated with disturbed iron metabolism in observational studies. However, such associations are inconsistent and may be attributable to confounding from environmental factors. This study uses a two-sample Mendelian randomization (MR) analysis to investigate whether there is any causal effect of systemic iron status on risk of 24 specific mental disorders.
Methods: Genetic variants with concordant relations to 4 biomarkers of iron status (serum iron, ferritin, transferrin saturation, and transferrin) were obtained from a genome-wide association study performed by the Genetics of Iron Status (GIS) consortium. Summary-level data for mental disorders were obtained from the UK Biobank. An inverse-variance weighted (IVW) approach was used for the main analysis, and the simple median, weighted median and MR-Egger methods were used in sensitivity analyses.
Results: Genetically predicted serum iron, ferritin, and transferrin saturation were positively associated with depression and psychogenic disorder, and inversely associated with gender identity disorders. A higher transferrin, indicative of lower iron status, was also associated with increased risk of gender identity disorders and decreased risk of psychogenic disorder. Results were broadly consistent when using multiple sensitivity analyses to account for potential genetic pleiotropy.
Conclusion: Our findings offer a novel insight into mental health, highlighting a detrimental effect of higher iron status on depression and psychogenic disorder as well as a potential protective role on risk of gender identity disorders. Further studies regarding the underlying mechanisms are warranted for updating preventative strategies.
Introduction
The essential element iron is closely involved in diverse fundamental biological processes, such as energy metabolism, oxygen transport, redox balance, and immunological modulation (1). Iron deficiency causes a number of pathological consequences, the most prominent being anemia. More than 2 billion individuals suffer from iron deficiency anemia worldwide, which may contribute to cognitive developmental defects in children (2). However, iron overload is also common and equally detrimental, as excess labile iron catalyzes the generation of reactive oxygen species (ROS) via the Fenton reaction and can trigger non-apoptotic cell death (3).
Disturbed iron homeostasis is related to a wide variety of chronic diseases, including mental disorders. In specific brain regions of patients with Alzheimer’s disease (AD), significant iron accumulation has been clearly observed and correlated well with a decline in cognitive function (4, 5). Increased iron concentrations were also reported as characteristic of the degenerating substantia nigra of Parkinson’s disease (PD), the second most prevalent neurodegenerative disease after AD (6). However, limited epidemiological evidence on the relationship between iron status and risk of other mental disorders is available.
Here, we conducted a Mendelian randomization (MR) study to robustly evaluate whether systemic iron status affects risk of multiple mental disorders. By exploiting genetic variants as instrumental variables of systemic iron status, the approach greatly avoided the risk of residual confounding as genetic variants are randomly allocated at conception and therefore irrelevant to environmental factors (7). Since disease development typically cannot alter the germline nuclear sequences, it may also overcome the problem of reverse causation (8). In this way, we employ MR to investigate the causal role for iron status in mental disorders, which would have important public health implications.
Materials and methods
In this present two-sample MR study, we used data from two different genome-wide association studies (GWASs)—one for the exposures and one for the outcome—to estimate the effects of exposure on outcome. Only summary-level data from these published studies were used. Appropriate patient consent and ethical approval were obtained in the original studies.
Genetic instrument selection
For unbiased detection of causal effects, the genetic variants used as genetic variants in this MR analysis must satisfy three key assumptions: (1) the used genetic variants should be robustly associated with iron status, (2) the used should not be associated with any confounders and (3) the genetic variants should influence the outcome (risk of specific mental disorder) only through iron status, rather than alternative pathways. The second and third assumptions are collectively known as independence from pleiotropy.
A large-scale meta-analysis of GWASs by the Genetics of Iron Status (GIS) Consortium was used to obtain single nucleotide polymorphisms (SNPs) proposed to be associated with systemic iron status. Data from 11 discovery cohorts and 8 replication cohorts were used in the meta-analysis, consisting of 48,972 individuals of European ancestry. It identified that 11 genome-wide-significant SNPs related to biomarkers of iron status, including increased serum iron, ferritin, transferrin saturation and decreased transferrin. Of these, three SNPs [rs1800562 and rs1799945 in the hemochromatosis (HFE) gene and rs855791 in the transmembrane serine protease 6 (TMPRSS6) gene] were robustly associated with concordant variance for each biomarker (Supplementary Table 1) (9). In addition, these SNPs are not in linkage disequilibrium and have been used as instrumental variables for systemic iron status in previous MR studies (10–12). Therefore, we included them in our MR analysis to predict systemic iron status.
F statistic of first-stage regression was employed to evaluate the strength of the instruments and was calculated using the following equation: F = (R2/k)/([1-R2]/[n-k-1]), where R2 is the proportion of the iron status variability accounted for by the SNP, k is the number of instruments used in the model and n is the sample size (13). To minimize weak instrument bias, only SNPs with an F statistic > 10 were included in subsequent analyses.
Outcome data sources
Summary-level GWAS statistics for 24 specific mental disorders were extracted from the Pan-ancestry genetic analysis of the UK Biobank (14). This UK-based prospective cohort study was approved by the North West Multicentre Research Ethics Committee, and recruited around 500,000 genotyped adults aged 40–69 years. Detailed characteristics of cases and controls can be found in Supplementary Table 2. The summary-level data are publicly available at https://pan.ukbb.broadinstitute.org.
Statistical analysis
An inverse-variance weighted (IVW) method with random-effects was used to generate the main MR estimates for the causal associations between each measure of iron status and risk of mental disorders. A threshold of P < 0.05 was used to determine statistical significance. Odds ratios (ORs) with 95% confidence intervals (CIs) of mental disorders are per one standard deviation (SD) increase in genetically predicted serum iron, log10 ferritin, transferrin saturation and transferrin levels. Cochran’s Q test was applied to evaluate heterogeneity in IVW estimates across different instruments (interpreting P < 0.05 as evidence of heterogeneity) (15).
Pleiotropy refers to a genetic variant influencing the outcome of interest through pathways independent of the risk factor. We then conducted a range of sensitivity analyses, including the simple median, weighted median and MR-Egger methods, to address potential pleiotropy. The simple and weighted median methods provide consistent MR estimates even when up to 50% of the information comes from invalid instrumental variables (16). The MR-Egger method is able to assess whether genetic variants have pleiotropic effects on the outcome and provides a consistent estimate of the causal effect even if all genetic variants are invalid (17). Since the relatively low statistical power of these methods compared with the main IVW analysis, they were used solely to confirm a consistent effect estimate, rather than to ascertain statistical significance itself via a p-value threshold. We also searched the PhenoScanner database1 to detect secondary phenotypes for the SNPs associated with the iron status biomarkers (18).
All MR analyses were performed using the TwoSampleMR package by R 4.0.2 software.2
Results
Three SNPs including rs1800562 and rs1799945 in the HFE gene and rs855791 in the TMPRSS6 gene were employed for our main analysis. The F statistics for the three SNPs ranged from 47 for 2,127 across all four biomarkers of systematic iron status, as described previously (10, 12), making significant bias from use of weak instruments unlikely (13).
Genetically predicted high serum iron levels were positively associated with depression (OR: 1.41; 95%CI: 1.02, 1.97; P = 0.04) and psychogenic disorder (OR: 1.39; 95%CI: 1.05, 1.84; P = 0.02), but inversely associated with gender identity disorders (OR: 0.65; 95%CI: 0.56, 0.75; P < 0.001). No association was found in the other 21 mental disorders. We detected significant heterogeneity only in the analyses of hallucinations (P = 0.03) and tobacco use disorder (P = 0.04). Pooled MR estimates for the effect of increased serum iron on risk of different mental disorders are shown graphically in Figure 1.
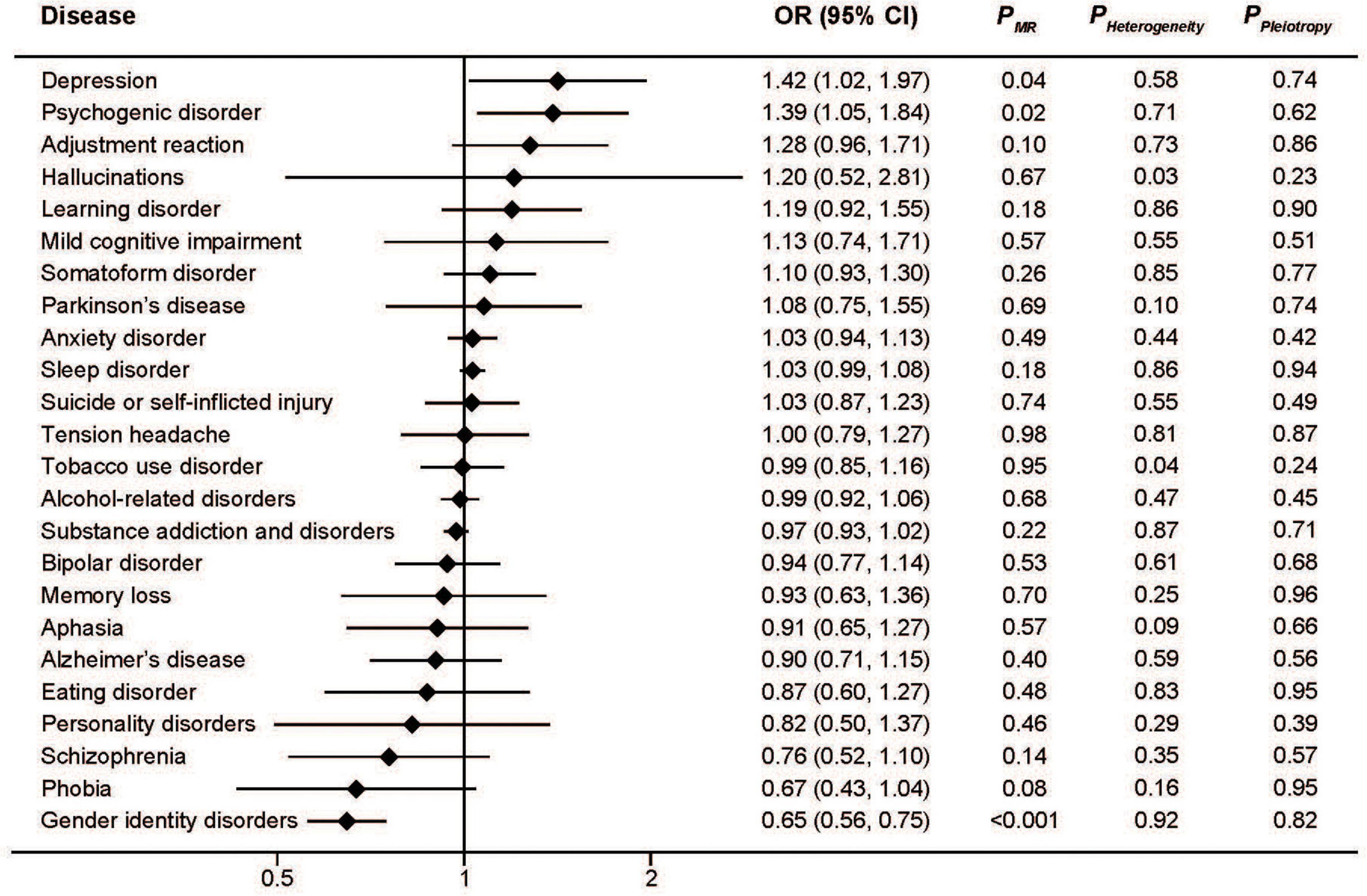
Figure 1. Associations between genetically predicted serum iron and risk of 24 specific mental disorders.
Similar to serum iron, the MR analysis showed a protective effect of ferritin on gender identity disorders as well as a detrimental effect on depression and psychogenic disorder (Figure 2). The pooled ORs for per SD unit increase of log10 (ferritin) on psychogenic disorder, depression, and gender identity disorders were 2.23 (95%CI: 1.54, 3.22; P < 0.001), 2.19 (95%CI: 1.12, 4.28; P = 0.02), and 0.39 (95%CI: 0.29, 0.52; P < 0.001), respectively. Consistent associations were also found with transferrin saturation (Figure 3). The pooled ORs for per SD unit increase of transferrin on psychogenic disorder, depression, and gender identity disorders were 1.30 (95%CI: 1.13, 1.48); P < 0.001, 1.29 (95%CI: 1.03, 1.62; P = 0.03), and 0.73 (95%CI: 0.68, 0.78; P < 0.001), respectively.
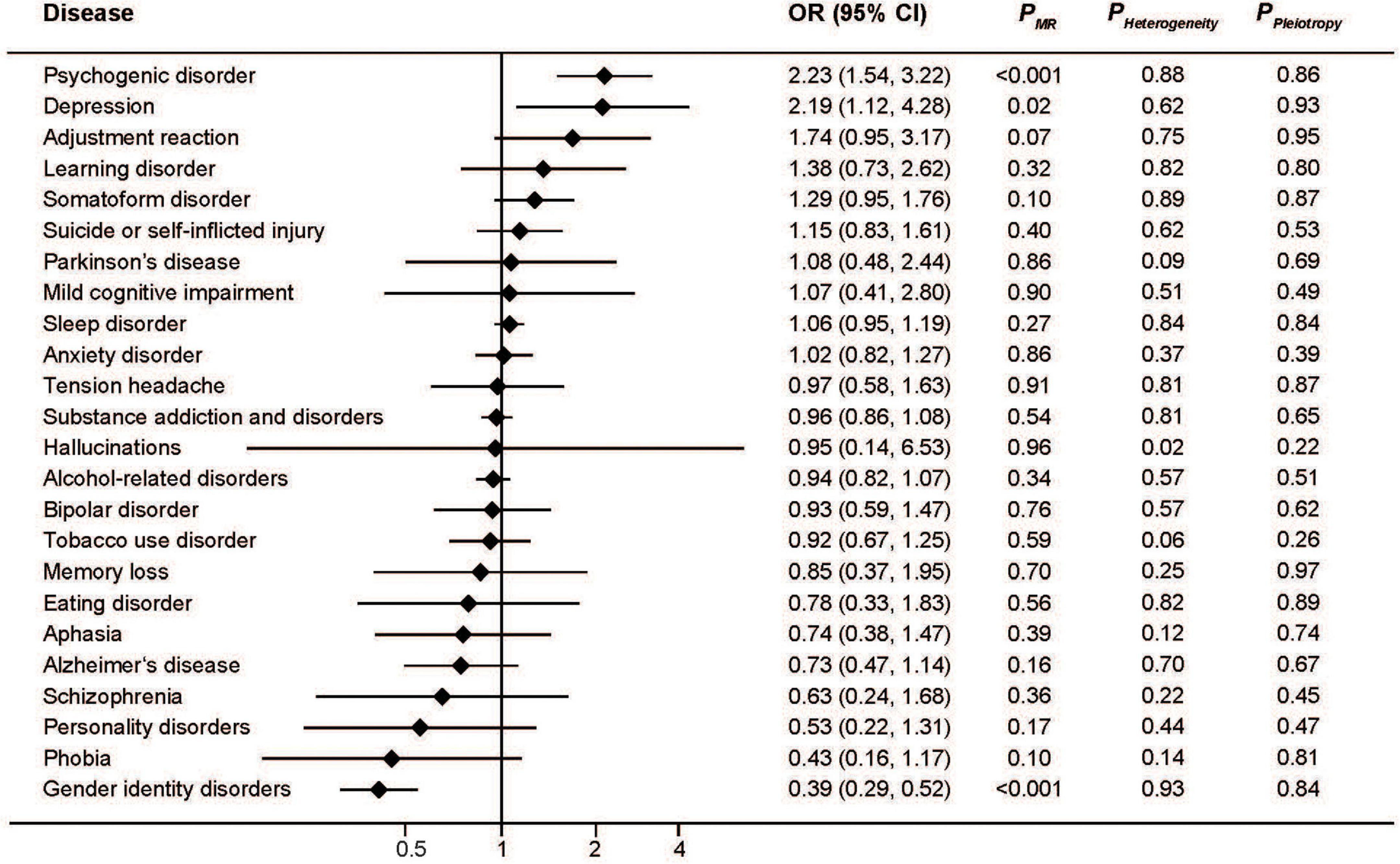
Figure 2. Associations between genetically predicted serum ferritin and risk of 24 specific mental disorders.
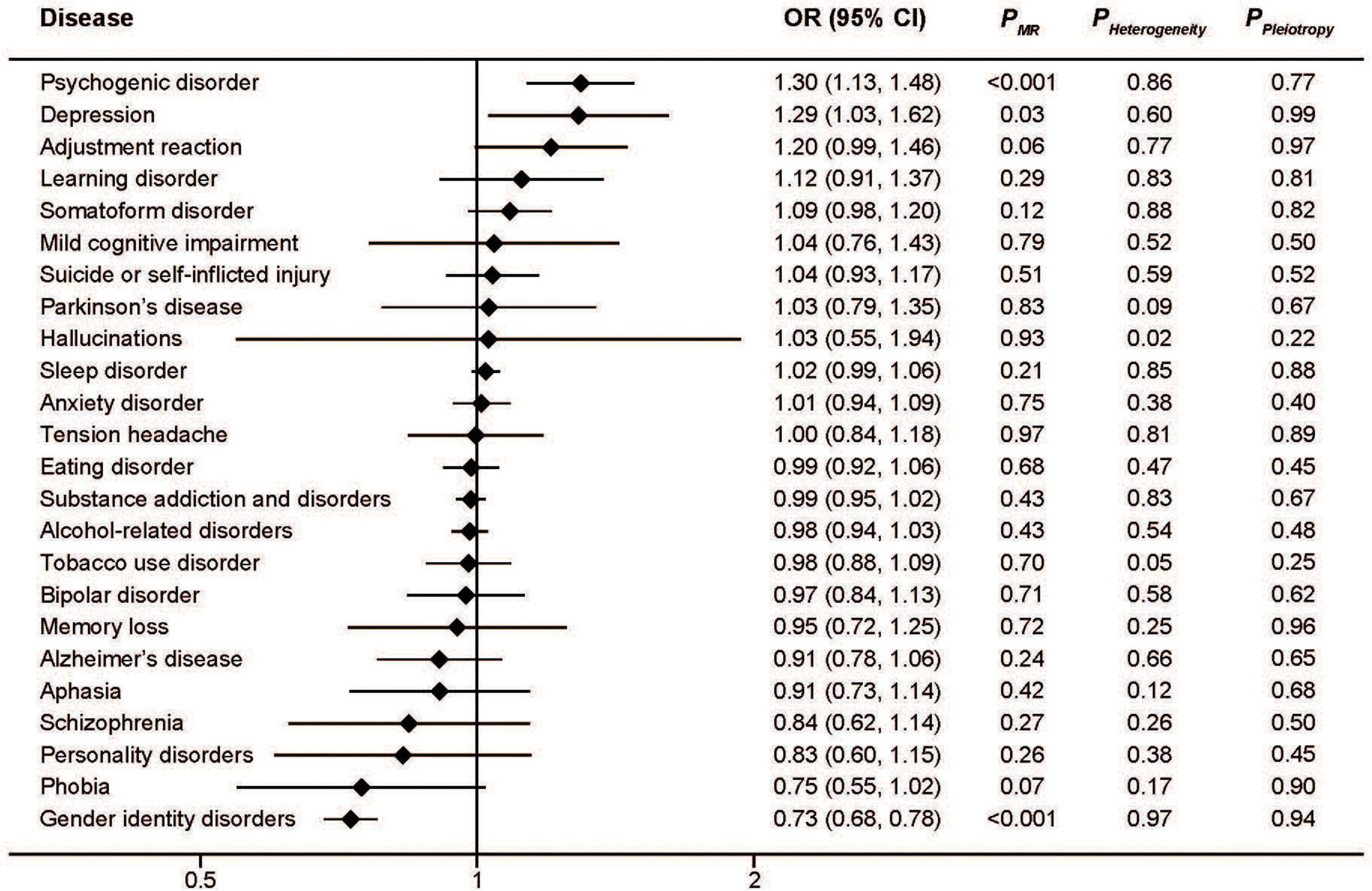
Figure 3. Associations between genetically predicted transferrin saturation and risk of 24 specific mental disorders.
In addition, higher transferrin levels, which are indicative of lower iron status, were associated with increased risk of gender identity disorders (OR: 1.53; 95%CI: 1.17, 2.00; P < 0.005) as well as decreased risk of somatoform disorder (OR: 0.87; 95%CI: 0.78, 0.98; P = 0.02) and psychogenic disorder (OR: 0.68; 95%CI: 0.59, 0.77; P < 0.001) (Figure 4). The effect of transferrin on depression seemed protective, but did not reach statistical significance (OR: 0.72; 95%CI: 0.48, 1.07; P = 0.10).
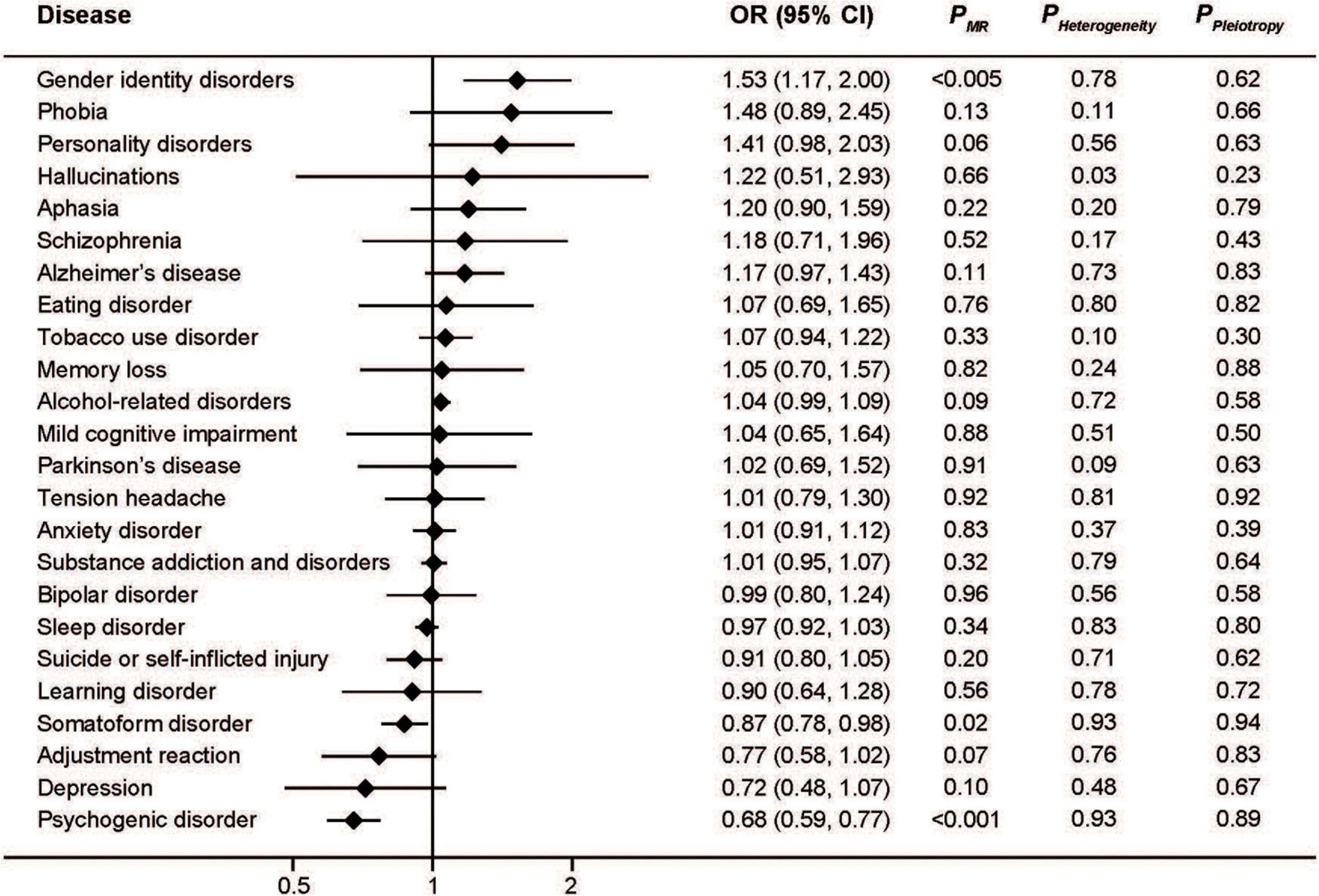
Figure 4. Associations between genetically predicted serum transferrin and risk of 24 specific mental disorders.
The MR-Egger approach did not produce evidence of pleiotropy in any of our analyses (Figures 1–4). We further examined potential pleiotropy using the PhenoScanner database. A potentially pleiotropic effect on risk of these mental disorders may be contributed by rs1800562 because of its association with reduced total and low-density lipoprotein cholesterol levels (19). Moreover, sensitivity analyses using simple median, weighted median, and MR Egger methods produced directionally consistent effects as the IVW estimates, supporting the consistency and robustness of our findings (Table 1).
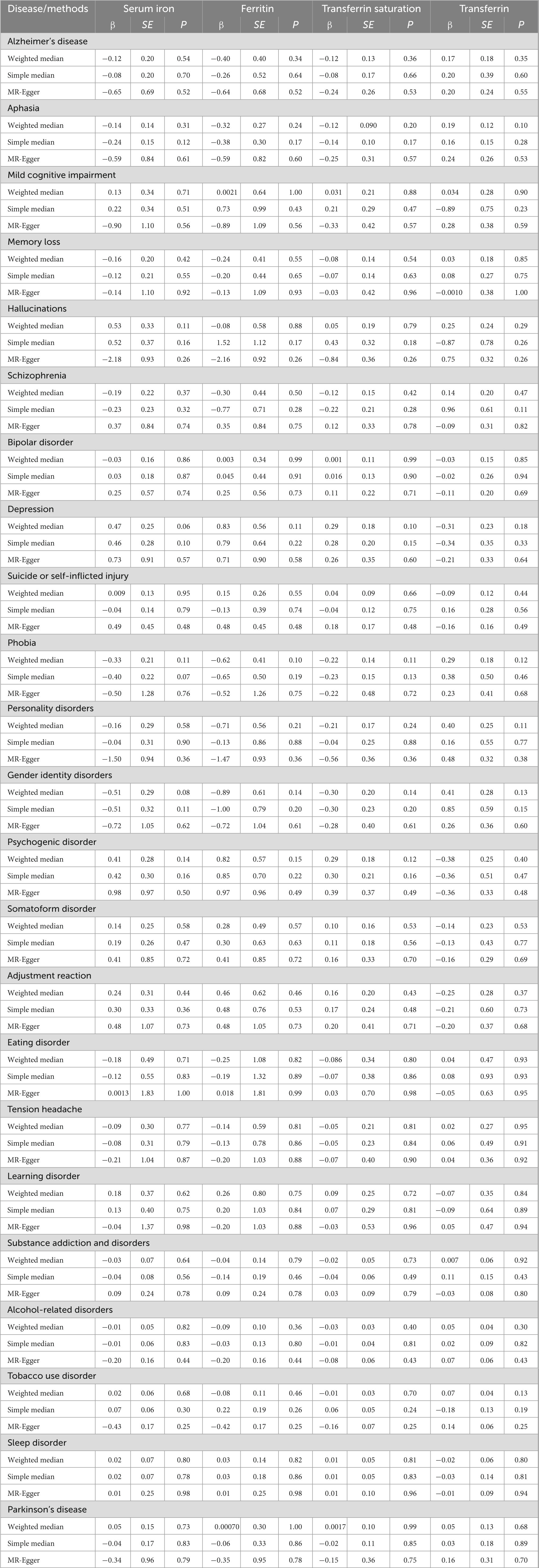
Table 1. Sensitivity analyses of the associations between genetically predicted iron status and mental disorders.
Discussion
Mental disorders affected more than 1 billion people globally and lead to 19% of all years lived with disability (20). Observational epidemiological studies have associated altered iron levels with risk of AD or PD; however, these studies are susceptible to confounding and reverse causation, and thus it remains unclear whether these connections are true. More importantly, the effect of iron on most other mental disorders remains unknown. Therefore, it is necessary to better understand the causal relationship between iron status and mental disorders in humans, in order to provide evidence to support further preclinical or clinical studies.
Iron is generally assumed to be a risk factor for AD, in line with the well-known phenomenon of iron accumulation in specific brain regions of AD patients (4), but a number of epidemiological studies have questioned this conclusion (21–24). In this study, our results showed no causal association between genetically determined markers of systemic iron status and AD. One explanation for the lack of clear relationship between serum iron status and cognitive decline is that circulating iron/ferritin levels may not be a reliable indicator of brain iron in AD patients (25).
One previous MR study indicated that increased serum iron levels are causally associated with a decreased risk of developing PD (26). Epidemiological studies, however, provided strong evidence about significantly higher serum iron levels in PD patients than health controls (27). Our study failed to replicate any of these findings, as no significant associations between iron biomarkers and PD risk were found. These conflicting results illustrate the complexity of the problem, and more investigation is required.
Our study increases the probability that iron overload has a clinically relevant impact on the risk of depression. This finding is important since evidence on the association between serum iron levels and depression risk collected so far has been controversial. Iron deficiency was generally thought of as a risk factor for depression. Stewart and Hirani once studied about 2,000 elderly individuals, and found that anemia and low serum ferritin levels were linked with depressive symptoms (28). A meta-analysis also indicated that dietary iron intake is inversely associated with risk of depression, but its result is difficult to interpret owing to limited number of included studies and high heterogeneity (29). However, recent studies have shown effects of iron in opposite directions (30, 31). These epidemiological studies suffer from reverse causation, and their conclusions may be confounded by disease status. For instance, inflammation influences iron metabolism resulting in an increase in serum ferritin and a decrease in serum iron at the same time. Given neuroinflammation has been considered to impact the development of depression (32), it may drive both higher iron status and greater depression risk. Based on MR approach, we have used genetic variants as instrumental variables for iron status to overcome these limitations of observational studies and strengthen the evidence for a detrimental effect of iron status on depression risk. We also found that genetically high iron status was associated with higher risk of psychogenic disorder and lower risk of gender identity disorders. To the best of our knowledge, this is the first evidence linking iron status to these mental disorders.
There are very few studies investigating the molecular mechanisms involved in psychogenic disorder as well as gender identity disorders. And there’s still no direct evidence that shows exactly how iron metabolism affects risk of these mental disorders. However, brain capture of blood iron are necessary for an appropriate synthesis of neurotransmitters, such as serotonin, dopamine, and noradrenaline. In addition, these neurotransmitters, involved in emotional behaviors, depend on neuron aromatic hydoxylases functioning with iron as essential cofactor (33). Iron deficient animals had significantly lower dopamine D2 receptor densities in the frontal cortex and caudate putamen. Meanwhile, serotonin (5-HT) transporter densities increased in the nucleus accumbens (34). Noradrenaline also has impact on neuroplasticity via brain-derived neurotrophic factor, which is key for prefrontal and hippocampus neurons playing a role in depression (35). Thus, abnormality of iron metabolism may cause changes in these mental disorders by affecting neurotransmitters. More population studies are needed to confirm the association; meanwhile, further mechanism studies are warranted to examine the biological connection between iron homeostasis and the diseases.
Key strengths of the study are primarily attributed to the MR design, which overcomes many unavoidable limitations introduced in observational studies. In addition, we systematically assessed the associations between four individual iron biomarkers and 24 mental disorders using summary-level data from large-scale genetic consortia and cohorts. Another strength is that the consistency between different MR approaches suggests the robustness of our findings.
There are also several limitations to this study. First, the statistical power was low in several analyses due to limited case number for some types of mental disorders. And it is also hard to perform stratified analyses by gender, age, or other categories. In addition, potential pleiotropy could not be completely ruled out. Although no evidence was found by the MR-Egger method, residual bias may still exist because the exact biological function of the SNPs associated with iron status is not entirely clear. Last, we investigated the association between iron status within the normal range and health risk. Therefore, our findings may not be used to make inferences regarding the effect of pathological iron overload caused by primary or secondary hemochromatosis.
In summary, the present two-sample MR study is the first to systematically evaluate the causal role of iron status for a wide range of mental disorders. Our findings may highlight the potential therapeutic targets and preventative strategies. Future research is required to examine the causal associations in various populations with different ethnic backgrounds based on individual-level data, as well as the possible underlying mechanisms.
Data availability statement
The original contributions presented in this study are included in the article/Supplementary material, further inquiries can be directed to the corresponding author.
Ethics statement
The studies involving human participants were reviewed and approved by the Ethics Committee of Hangzhou Normal University. The patients/participants provided their written informed consent to participate in this study.
Author contributions
XF designed the research and wrote the manuscript. JQ and FL performed the statistical analyses. All authors read and approved the final manuscript.
Funding
This study was supported by the National Natural Science Foundation of China (Grant nos. 82170644 and 31900835 to XF) and the Natural Science Foundation of Zhejiang Province (Grant no. LY22C110002 to XF).
Acknowledgments
We thank all the participants and the coordinating team of the UK Biobank for their generosity and contributions.
Conflict of interest
The authors declare that the research was conducted in the absence of any commercial or financial relationships that could be construed as a potential conflict of interest.
Publisher’s note
All claims expressed in this article are solely those of the authors and do not necessarily represent those of their affiliated organizations, or those of the publisher, the editors and the reviewers. Any product that may be evaluated in this article, or claim that may be made by its manufacturer, is not guaranteed or endorsed by the publisher.
Supplementary material
The Supplementary Material for this article can be found online at: https://www.frontiersin.org/articles/10.3389/fnut.2022.1084860/full#supplementary-material
Footnotes
References
1. Muckenthaler MU, Rivella S, Hentze MW, Galy B. A red carpet for iron metabolism. Cell. (2017) 168:344–61. doi: 10.1016/j.cell.2016.12.034
2. Camaschella C. Iron-deficiency anemia. N Engl J Med. (2015) 372:1832–43. doi: 10.1056/NEJMra1401038
3. Dixon SJ, Lemberg KM, Lamprecht MR, Skouta R, Zaitsev EM, Gleason CE, et al. Ferroptosis: an iron-dependent form of nonapoptotic cell death. Cell. (2012) 149:1060–72. doi: 10.1016/j.cell.2012.03.042
4. Tao Y, Wang Y, Rogers JT, Wang F. Perturbed iron distribution in Alzheimer’s disease serum, cerebrospinal fluid, and selected brain regions: a systematic review and meta-analysis. J Alzheimers Dis. (2014) 42:679–90. doi: 10.3233/JAD-140396
5. Du L, Zhao Z, Cui A, Zhu Y, Zhang L, Liu J, et al. Increased iron deposition on brain quantitative susceptibility mapping correlates with decreased cognitive function in Alzheimer’s disease. ACS Chem Neurosci. (2018) 9:1849–57. doi: 10.1021/acschemneuro.8b00194
6. Genoud S, Senior AM, Hare DJ, Double KL. Meta-analysis of copper and iron in Parkinson’s disease brain and biofluids. Mov Disord. (2020) 35:662–71. doi: 10.1002/mds.27947
7. Emdin CA, Khera AV, Kathiresan S. Mendelian randomization. JAMA. (2017) 318:1925–6. doi: 10.1001/jama.2017.17219
8. Davey Smith G, Hemani G. Mendelian randomization: genetic anchors for causal inference in epidemiological studies. Hum Mol Genet. (2014) 23:R89–98. doi: 10.1093/hmg/ddu328
9. Benyamin B, Esko T, Ried JS, Radhakrishnan A, Vermeulen SH, Traglia M, et al. Novel loci affecting iron homeostasis and their effects in individuals at risk for hemochromatosis. Nat Commun. (2014) 5:4926. doi: 10.1038/ncomms5926
10. Gill D, Monori G, Tzoulaki I, Dehghan A. Iron status and risk of stroke. Stroke. (2018) 49:2815–21. doi: 10.1161/STROKEAHA.118.022701
11. Yuan S, Carter P, Vithayathil M, Kar S, Giovannucci E, Mason AM, et al. Iron status and cancer risk in UK biobank: a two-sample mendelian randomization study. Nutrients. (2020) 12:526. doi: 10.3390/nu12020526
12. Wang X, Fang X, Zheng W, Zhou J, Song Z, Xu M, et al. Genetic support of a causal relationship between iron status and type 2 diabetes: a mendelian randomization study. J Clin Endocrinol Metab. (2021) 106:e4641–51. doi: 10.1210/clinem/dgab454
13. Palmer TM, Lawlor DA, Harbord RM, Sheehan NA, Tobias JH, Timpson NJ, et al. Using multiple genetic variants as instrumental variables for modifiable risk factors. Stat Methods Med Res. (2012) 21:223–42. doi: 10.1177/0962280210394459
14. Sudlow C, Gallacher J, Allen N, Beral V, Burton P, Danesh J, et al. UK biobank: an open access resource for identifying the causes of a wide range of complex diseases of middle and old age. PLoS Med. (2015) 12:e1001779. doi: 10.1371/journal.pmed.1001779
15. Burgess S, Bowden J, Fall T, Ingelsson E, Thompson SG. Sensitivity analyses for robust causal inference from mendelian randomization analyses with multiple genetic variants. Epidemiology. (2017) 28:30–42. doi: 10.1097/EDE.0000000000000559
16. Bowden J, Davey Smith G, Haycock PC, Burgess S. Consistent estimation in mendelian randomization with some invalid instruments using a weighted median estimator. Genet Epidemiol. (2016) 40:304–14. doi: 10.1002/gepi.21965
17. Burgess S, Thompson SG. Interpreting findings from Mendelian randomization using the MR-Egger method. Eur J Epidemiol. (2017) 32:377–89. doi: 10.1007/s10654-017-0255-x
18. Staley JR, Blackshaw J, Kamat MA, Ellis S, Surendran P, Sun BB, et al. PhenoScanner: a database of human genotype-phenotype associations. Bioinformatics. (2016) 32:3207–9. doi: 10.1093/bioinformatics/btw373
19. Willer CJ, Schmidt EM, Sengupta S, Peloso GM, Gustafsson S, Kanoni S, et al. Discovery and refinement of loci associated with lipid levels. Nat Genet. (2013) 45:1274–83. doi: 10.1038/ng.2797
20. Rehm J, Shield KD. Global burden of disease and the impact of mental and addictive disorders. Curr Psychiatry Rep. (2019) 21:10. doi: 10.1007/s11920-019-0997-0
21. Huang CW, Wang SJ, Wu SJ, Yang CC, Huang MW, Lin CH, et al. Potential blood biomarker for disease severity in the Taiwanese population with Alzheimer’s disease. Am J Alzheimers Dis Other Demen. (2013) 28:75–83. doi: 10.1177/1533317512467674
22. Siotto M, Simonelli I, Pasqualetti P, Mariani S, Caprara D, Bucossi S, et al. Association between serum ceruloplasmin specific activity and risk of Alzheimer’s disease. J Alzheimers Dis. (2016) 50:1181–9.
23. Lupton MK, Benyamin B, Proitsi P, Nyholt DR, Ferreira MA, Montgomery GW, et al. No genetic overlap between circulating iron levels and Alzheimer’s disease. J Alzheimers Dis. (2017) 59:85–99. doi: 10.3233/JAD-170027
24. Milward EA, Bruce DG, Knuiman MW, Divitini ML, Cole M, Inderjeeth CA, et al. A cross-sectional community study of serum iron measures and cognitive status in older adults. J Alzheimers Dis. (2010) 20:617–23.
25. House MJ, St Pierre TG, Milward EA, Bruce DG, Olynyk JK. Relationship between brain R(2) and liver and serum iron concentrations in elderly men. Magn Reson Med. (2010) 63:275–81. doi: 10.1002/mrm.22263
26. Pichler I, Del Greco MF, Gogele M, Lill CM, Bertram L, Do CB, et al. Serum iron levels and the risk of Parkinson disease: a Mendelian randomization study. PLoS Med. (2013) 10:e1001462. doi: 10.1371/journal.pmed.1001462
27. Jiao J, Guo H, He Y, Wang J, Yuan J, Hu W. Meta-analysis of the association between serum iron levels and Parkinson’s disease: evidence from 11 publications. Brain Res. (2016) 1646:490–3. doi: 10.1016/j.brainres.2016.06.044
28. Stewart R, Hirani V. Relationship between depressive symptoms, anemia, and iron status in older residents from a national survey population. Psychosom Med. (2012) 74:208–13. doi: 10.1097/PSY.0b013e3182414f7d
29. Li Z, Li B, Song X, Zhang D. Dietary zinc and iron intake and risk of depression: a meta-analysis. Psychiatry Res. (2017) 251:41–7. doi: 10.1016/j.psychres.2017.02.006
30. Richardson AC, Heath AL, Haszard JJ, Polak MA, Houghton LA, Conner TS. Higher body iron is associated with greater depression symptoms among young adult men but not women: observational data from the daily life study. Nutrients. (2015) 7:6055–72. doi: 10.3390/nu7085270
31. Al-Hakeim HK, Najm AH, Al-Dujaili AH, Maes M. Major depression in children with transfusion-dependent thalassemia is strongly associated with the combined effects of blood transfusion rate, iron overload, and increased pro-inflammatory cytokines. Neurotox Res. (2020) 38:228–41. doi: 10.1007/s12640-020-00193-1
32. Chu AL, Hickman M, Steel N, Jones PB, Davey Smith G, Khandaker GM. Inflammation and depression: a public health perspective. Brain Behav Immun. (2021) 95:1–3.
33. Berthou C, Iliou JP, Barba D. Iron, neuro-bioavailability and depression. eJHaem. (2022) 3:263–75. doi: 10.1002/jha2.321
34. Morse AC, Beard JL, Azar MR, Jones BC. Sex and genetics are important cofactors in assessing the impact of iron deficiency on the developing mouse brain. Nutr Neurosci. (1999) 2:323–35. doi: 10.1080/1028415X.1999.11747287
Keywords: iron, ferritin, transferrin, mental disorders, UK Biobank, Mendelian randomization
Citation: Qiu J, Lian F and Fang X (2022) Iron status and mental disorders: A Mendelian randomization study. Front. Nutr. 9:1084860. doi: 10.3389/fnut.2022.1084860
Received: 31 October 2022; Accepted: 01 December 2022;
Published: 15 December 2022.
Edited by:
Peng An, China Agricultural University, ChinaReviewed by:
Xinhui Wang, Zhejiang University, ChinaZijun Song, Zhejiang University, China
Ding Ye, Zhejiang Chinese Medical University, China
Copyright © 2022 Qiu, Lian and Fang. This is an open-access article distributed under the terms of the Creative Commons Attribution License (CC BY). The use, distribution or reproduction in other forums is permitted, provided the original author(s) and the copyright owner(s) are credited and that the original publication in this journal is cited, in accordance with accepted academic practice. No use, distribution or reproduction is permitted which does not comply with these terms.
*Correspondence: Xuexian Fang, ✉ eGZhbmdAaHpudS5lZHUuY24=