- 1Division of Nephrology, Department of Medicine, University of Texas Southwestern, Dallas, TX, United States
- 2Division of Pediatric Nephrology, Department of Pediatrics, University of Texas Southwestern, Dallas, TX, United States
- 3Department of Pharmacology, University of Texas Southwestern, Dallas, TX, United States
Introduction: Quinolinic acid is an intermediate compound derived from the metabolism of dietary tryptophan. Its accumulation has been reported in patients suffering a broad spectrum of diseases and conditions. In this manuscript, we present the results of a systematic review of research studies assessing urinary quinolinic acid in health and disease.
Methods: We performed a literature review using PubMed, Cochrane, and Scopus databases of all studies reporting data on urinary quinolinic acid in human subjects from December 1949 to January 2022.
Results: Fifty-seven articles met the inclusion criteria. In most of the reported studies, compared to the control group, quinolinic acid was shown to be at increased concentration in urine of patients suffering from different diseases and conditions. This metabolite was also demonstrated to correlate with the severity of certain diseases including juvenile idiopathic inflammatory myopathies, graft vs. host disease, autism spectrum disorder, and prostate cancer. In critically ill patients, elevated quinolinic acid in urine predicted a spectrum of adverse outcomes including hospital mortality.
Conclusion: Quinolinic acid has been implicated in the pathophysiology of multiple conditions. Its urinary accumulation appears to be a feature of acute physiological stress and several chronic diseases. The exact significance of these findings is still under investigation, and further studies are needed to reveal the subsequent implications of this accumulation.
Introduction
Quinolinic acid, or pyridine-2,3-dicarboxylic acid, was originally described in 1949 by Henderson et al. as a normal component of urine and a possible substrate of tryptophan (1). More recently, quinolinic acid has been recognized as a metabolite of the kynurenine pathway, also known as the de novo nicotinamide adenine dinucleotide (NAD+) synthesis pathway. This pathway catabolizes dietary tryptophan into NAD+ and is one of the three independent pathways for NAD+ biosynthesis (Figures 1, 2). In times of stress and inflammation, quinolinic acid accumulates (3, 4). This accumulation was hypothesized to result from two phenomena. First, pro-inflammatory cytokines activate the first and rate-limiting enzyme of the kynurenine pathway, indoleamine 2,3-dioxygenase (IDO), inducing the accumulation of downstream metabolites (5). Second, the quinolinate phosphoribosyl transferase (QPRT) enzyme which catalyzes quinolinic acid and commits the pathway to NAD+ biosynthesis declines or saturates with inflammation (6, 7).
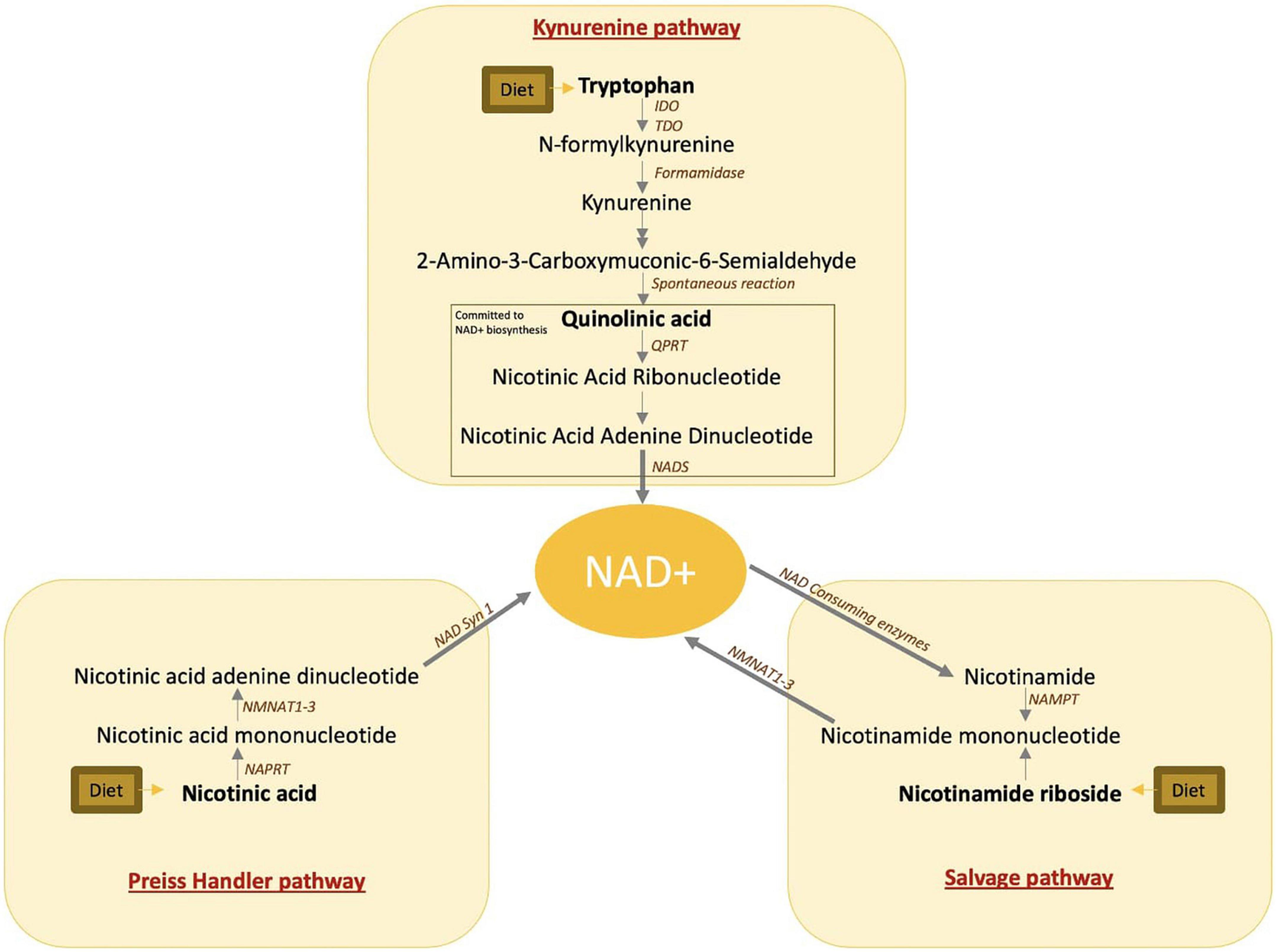
Figure 1. NAD+ biosynthetic pathways. IDO, indoleamine-pyrrole 2,3-dioxygenase; TDO, tryptophan 2,3-dioxygenase; QPRT, quinolinate phosphoribosyltransferase; NAD, nicotinamide adenine dinucleotide; NADS, nicotinamide adenine dinucleotide synthetase 1; NMNAT, nicotinamide mononucleotide adenylyl transferase; NAPRT, nicotinate phosphoribosyltransferase; NAMPT, nicotinamide phosphoribosyltransferase.
The redox cofactor nicotinamide adenine dinucleotide (NAD+) plays a fundamental role in cellular energy production by carrying high energy electrons and driving oxidative phosphorylation (8). In addition to the de novo NAD+ biosynthesis pathway, NAD+ is biosynthesized from two additional pathways including the Preiss–Handler pathway, which makes NAD+ from dietary niacin, and the NAD+ salvage pathway which makes NAD+ from dietary or recycled niacinamide (Figures 1, 2). Despite the alternative pathways of NAD+ production, quinolinic acid accumulation is frequently accompanied by NAD+ reduction (9).
Over the past decade, a growing number of reports has described quinolinic acid accumulation during inflammation and has hypothesized that quinolinic acid may play a role in many disease processes. Inflammation triggers a complex cascade of cytokines which can activate the kynurenine pathway and cause quinolinic acid accumulation. In fact, IDO, the enzyme responsible for the first and rate-limiting step of this pathway, is induced by many inflammatory cytokines (10, 11). Interferon-γ (IFN-γ), specifically, is a powerful IDO-activating cytokine (12). Quinolinic acid seems to have a controversial and unclear role during inflammation. In some instances, quinolinic acid appears to have an anti-inflammatory role by reducing Th1-like cells and increasing the Th2-like cells, which limits adaptive immunity overactivation (13). However, quinolinic acid accumulation has also been considered a deleterious feature of inflammation, and its increased concentration can be responsible for cytotoxicity, particularly in neurologic diseases through numerous proposed mechanisms (2).
Quinolinic acid has been noted to accumulate in several organs, blood, cerebrospinal fluid (CSF), and urine during pathological conditions. This accumulation could be interpreted as either evidence of a high-flux state or as suppression of pathway enzymes, including QPRT activity, during inflammation and stress, leading to altered NAD+ biosynthesis (7, 14). Quinolinic acid accumulation has been associated with a spectrum of diseases including neurodegenerative conditions, psychiatric diseases, acute illness, kidney failure, and liver failure. The exact implication of quinolinic acid accumulation as well as its correlation with the disease severity is still uncertain. Urinary measurement of quinolinic acid is a convenient diagnostic test of this metabolite as it is a non-invasive and easily collected. Additionally, there is evidence that urinary quinolinic acid levels correspond with systemic levels (15). Several studies have assessed urinary quinolinic acid levels as markers of various pathologies. However, until now, a broad examination of urinary quinolinic acid excretion has not been undertaken. In this review, we categorize and summarize primary research that has reported quinolinic acid in human urine.
Materials and methods
Literature search
This study was guided by the Preferred Reported Items for Systematic Reviews and Meta-Analysis (PRISMA) statement issued in 2020. The search was restricted to English language journal articles with human subjects, published between December 1949 and January 2022.
The search of the literature was conducted using electronic databases PubMed, Cochrane, and Scopus. The following keywords were used to perform the search: ((Quinolinic Acid) OR (Quinolinate)) AND ((Urine) OR (Urinary) OR (Urine analysis)).
Inclusion and exclusion criteria
Eligible studies were included if they were (i) peer reviewed primary scientific articles and (ii) reported quinolinic acid measurement in the urine of human subjects irrespective of study aim. Studies were excluded if they were (i) reviews or meta-analyses, (ii) articles written in languages other than English, (iii) assessments of quinolinic acid in body fluids other than urine, and (iv) studies performed exclusively on animals.
Study selection and data extraction
Two investigators performed the literature search and selected qualified studies according to the inclusion and exclusion criteria. The study selection was performed in a two-step process, beginning with a title and abstract screening followed by full-text screening. The data extracted from each study included the general aim of the study, study method and design for measuring metabolites, and the results concerning quinolinic acid measurement in urine. Included studies were categorized in a system-based classification: endocrinology, gastroenterology, hematology, infectious diseases, nephrology, neurology, obstetrics and gynecology, oncology, psychiatry, rheumatology, and other conditions. A descriptive analysis of the results was presented.
Results
The electronic database search yielded a total of 512 articles. Based on title and/or abstract, 90 articles were deemed potentially relevant, and after the full text viewing, 54 articles were included in the review (Figure 2).
Studies measuring urinary quinolinic acid in humans are shown in Table 1. Various methods were used to measure urinary quinolinic acid. However, most studies described chromatography-spectrometry techniques. Additionally, human urine exhibits significant variability in concentration and composition within an individual arising from various external and internal factors such as hydration, solute intake, and kidney function. To avoid false data interpretation, several normalization methods exist for urinary metabolomic studies (16). We specified for each study which normalization technique was used (if any) to insist on the validity of the results and to facilitate the interpretation of the data.
Urinary quinolinic acid was measured in multiple pathological conditions. Compared to control groups, this metabolite was shown to be higher in urine of patients suffering from premature rupture of membranes during labor (17), juvenile idiopathic inflammatory myopathies (18), Sjögren’s Syndrome (19), mitochondrial oxodicarboxylate carrier deficiency (20), kidney cancer (21), breast cancer (22), prostate cancer (23), graft versus host disease (GVHD) (24), major depressive disorder (25), autism spectrum disorder (26), attention-deficit/hyperactivity disorder (ADHD) (27), tuberculous meningitis (28), kidney transplant rejection, acute kidney injury (AKI) (6), and metabolic syndrome including hyperlipidemia, obesity, hypertension, and diabetes (29, 30). In some cases, urinary quinolinic acid was even demonstrated to correlate with disease severity. In juvenile idiopathic inflammatory myopathies, urinary quinolinic acid was shown to increase with several aspects of disease activity including physician and parent global assessments of disease activity, manual muscle testing total score, Childhood Myositis Assessment Scale, and Childhood Health Assessment Questionnaire (18). In GVHD, urinary quinolinic acid was significantly higher in patients with GVHD grade 3 or 4 compared to those with grade 1 and 2 (24). Moreover, in a critically ill population, urinary quinolinic acid to tryptophan ratio was not only associated with higher risk of AKI development, but also increased probability of global adverse outcomes, including hospital mortality (6). In a study on patients with autism spectrum disorder comparing urine metabolomics of patients with severe autism spectrum disorder symptoms to those with mild-to-moderate symptoms, quinolinic acid was significantly higher in patients with severe symptoms (31). In patients with prostate cancer, quinolinic acid to tryptophan ratio level in urine significantly correlated with their Gleason score.
Conversely, Heyes et al. found no significant difference in urinary quinolinic acid in patients with Huntington’s disease compared to the control group (32). Patients suffering from acute on chronic liver failure showed no significant difference in their urinary quinolinic acid during acute kidney failure (33). And, one study investigating patients with bladder cancer showed a lower ratio of urinary tryptophan to quinolinic acid compared to the healthy control group (34).
Urinary quinolinic acid is increased in several non-disease settings as well. Quinolinic acid is reportedly higher in urine of patients who were administered deoxypyridoxine (a vitamin B6-antagonist), oral contraceptive (35), tryptophan (1, 36–38), high-protein diet (39), and those who were exposed to phthalate (an industrial chemical) (40; Table 1).
Discussion
To our knowledge, this is the first systematic review evaluating urinary quinolinic acid in humans. There is abundant evidence that urinary quinolinic acid is increased in various disease states including cancer, infections, autoimmune diseases, metabolic syndrome, and psychiatric conditions, and in many instances quinolinic acid levels correlate with disease severity (41). While this review focused primarily on human urine, given its ease of measurement, this metabolite has also been investigated in blood, CSF, and tissue biopsies and has been implicated in the pathophysiology of different diseases in animals as well.
The included articles described a wide spectrum of settings in which quinolinic acid is increased with different methods and units of measurement. These variations limited our ability to compare findings. Another limitation to our systematic review is the difficulty of assessing bias in the included studies since the quinolinic acid data is not always primary outcome of the studies presented. We recognize that undetected biases may have been present in the articles described here. We also acknowledge that focusing on urinary-based quinolinic acid studies and excluding plasma and tissue-based quinolinic acid studies is an additional limitation to our results, but this was an indispensable filter for the purpose and feasibility of our study.
Overall, we were able to demonstrate build-up of quinolinic acid in urine in a wide spectrum of settings. Quinolinic acid increase during inflammation cannot be analyzed independently from its biochemical pathway, and the exact implication of its build-up is yet unrevealed. Acting as a double-edged sword, quinolinic acid is both an essential precursor for nicotinamide adenine dinucleotide (NAD+) biosynthesis and a potentially toxic metabolite at high concentrations. Quinolinic acid accumulation has been observed in numerous pathologic conditions. While most of the reported studies are observational in nature, they have highlighted that urinary measurement of quinolinic acid is an easy value to obtain that may reveal useful information about systemic disease processes. The true significance of its accumulation and its correlation with the severity of disease are still uncertain. Further studies are needed to investigate potential diagnostic, prognostic, and therapeutic implications of quinolinic acid.
Data availability statement
The original contributions presented in this study are included in the article/supplementary material, further inquiries can be directed to the corresponding author.
Author contributions
SP planned, designed, and supervised the project. AC and MS performed the literature search and collected the qualified studies according to the inclusion and exclusion criteria. All authors discussed the results, wrote the manuscript, and approved the submitted version.
Conflict of interest
The authors declare that the research was conducted in the absence of any commercial or financial relationships that could be construed as a potential conflict of interest.
Publisher’s note
All claims expressed in this article are solely those of the authors and do not necessarily represent those of their affiliated organizations, or those of the publisher, the editors and the reviewers. Any product that may be evaluated in this article, or claim that may be made by its manufacturer, is not guaranteed or endorsed by the publisher.
References
1. Henderson L, Ramasarma G, Johnson B. Quinolinic acid metabolism: IV. Urinary excretion by man and other mammals as affected by the ingestion of tryptophan. J Biol Chem. (1949) 181:731–8. doi: 10.1016/S0021-9258(18)56596-0
2. Lugo-Huitrón R, Ugalde Muñiz P, Pineda B, Pedraza-Chaverrí J, Ríos C, Pérez-de la Cruz V. Quinolinic acid: an endogenous neurotoxin with multiple targets. Oxid Med Cell Longev. (2013) 2013:104024. doi: 10.1155/2013/104024
3. Heyes M, Saito K, Major E, Milstien S, Markey S, Vickers JH. A mechanism of quinolinic acid formation by brain in inflammatory neurological disease: attenuation of synthesis from l-tryptophan by 6-chlorotryptophan and 4-chloro-3-hydroxyanthranilate. Brain. (1993) 116:1425–50. doi: 10.1093/brain/116.6.1425
4. Badawy A. Hypothesis kynurenic and quinolinic acids: the main players of the kynurenine pathway and opponents in inflammatory disease. Med Hypotheses. (2018) 118:129–38. doi: 10.1016/j.mehy.2018.06.021
5. Kim S, Miller B, Stefanek M, Miller A. Inflammation-induced activation of the indoleamine 2,3-dioxygenase pathway: relevance to cancer-related fatigue. Cancer. (2015) 121:2129–36. doi: 10.1002/cncr.29302
6. Poyan Mehr A, Tran M, Ralto K, Leaf D, Washco V, Messmer J, et al. De novo NAD+ biosynthetic impairment in acute kidney injury in humans. Nat Med. (2018) 24:1351–9. doi: 10.1038/s41591-018-0138-z
7. Bignon Y, Rinaldi A, Nadour Z, Poindessous V, Nemazanyy I, Lenoir O, et al. Cell stress response impairs de novo NAD+ biosynthesis in the kidney. JCI Insight. (2022) 7:e153019. doi: 10.1172/jci.insight.153019
8. Pollak N, Dölle C, Ziegler M. The power to reduce: pyridine nucleotides – small molecules with a multitude of functions. Biochem J. (2007) 402:205–18. doi: 10.1042/BJ20061638
9. Ralto K, Rhee E, Parikh SM. NAD + homeostasis in renal health and disease. Nat Rev Nephrol. (2020) 16:99–111. doi: 10.1038/s41581-019-0216-6
10. Baumgartner R, Forteza M, Ketelhuth D. The interplay between cytokines and the kynurenine pathway in inflammation and atherosclerosis. Cytokine. (2019) 122:154148. doi: 10.1016/j.cyto.2017.09.004
11. Lovelace M, Varney B, Sundaram G, Franco N, Ng M, Pai S, et al. Current evidence for a role of the kynurenine pathway of tryptophan metabolism in multiple sclerosis. Front Immunol. (2016) 7:246. doi: 10.3389/fimmu.2016.00246
12. Sorgdrager F, Naudé P, Kema I, Nollen E, Deyn P. Tryptophan metabolism in inflammaging: from biomarker to therapeutic target. Front Immunol. (2019) 10:2565. doi: 10.3389/fimmu.2019.02565
13. Orihara K, Odemuyiwa S, Stefura W, Ilarraza R, HayGlass K, Moqbel R. Neurotransmitter signalling via NMDA receptors leads to decreased T helper type 1-like and enhanced T helper type 2-like immune balance in humans. Immunology. (2018) 153:368–79. doi: 10.1111/imm.12846
14. Braidy N, Berg J, Clement J, Khorshidi F, Poljak A, Jayasena T, et al. Role of nicotinamide adenine dinucleotide and related precursors as therapeutic targets for age-related degenerative diseases: rationale, biochemistry, pharmacokinetics, and outcomes. Antioxid Redox Signal. (2019) 30:251–94. doi: 10.1089/ars.2017.7269
15. Moffett J, Arun P, Puthillathu N, Vengilote R, Ives J, Badawy A, et al. Quinolinate as a marker for kynurenine metabolite formation and the unresolved question of NAD+ synthesis during inflammation and infection. Front Immunol. (2020) 11:31. doi: 10.3389/fimmu.2020.00031
16. Mervant L, Tremblay-Franco M, Jamin E, Kesse-Guyot E, Galan P, Martin J, et al. Osmolality-based normalization enhances statistical discrimination of untargeted metabolomic urine analysis: results from a comparative study. Metabolomics. (2021) 17:2. doi: 10.1007/s11306-020-01758-z
17. Meloni A, Palmas F, Barberini L, Mereu R, Deiana S, Fais M, et al. PROM and labour effects on urinary metabolome: a pilot study. Dis Markers. (2018) 2018:e1042479. doi: 10.1155/2018/1042479
18. Rider L, Schiffenbauer A, Zito M, Lim K, Ahmed A, Zemel L, et al. Neopterin and quinolinic acid are surrogate measures of disease activity in the juvenile idiopathic inflammatory myopathies. Clin Chem. (2002) 48:1681–8.
19. Fernández-Ochoa Á, Borrás-Linares I, Quirantes-Piné R, Alarcón-Riquelme M, Beretta L, Segura-Carretero A. Discovering new metabolite alterations in primary sjögren’s syndrome in urinary and plasma samples using an HPLC-ESI-QTOF-MS methodology. J Pharm Biomed Anal. (2020) 179:112999. doi: 10.1016/j.jpba.2019.112999
20. Boczonadi V, King M, Smith A, Olahova M, Bansagi B, Roos A, et al. Mitochondrial oxodicarboxylate carrier deficiency is associated with mitochondrial DNA depletion and spinal muscular atrophy-like disease. Genet Med. (2018) 20:1224–35.
21. Kim K, Taylor S, Ganti S, Guo L, Osier M, Weiss R. Urine metabolomic analysis identifies potential biomarkers and pathogenic pathways in kidney cancer. Omics J Integr Biol. (2011) 15:293–303. doi: 10.1089/omi.2010.0094
22. Zimmer P, Schmidt M, Prentzell M, Berdel B, Wiskemann J, Kellner K, et al. Resistance exercise reduces kynurenine pathway metabolites in breast cancer patients undergoing radiotherapy. Front Oncol. (2019) 9:962. doi: 10.3389/fonc.2019.00962
23. Thüring M, Knuchel R, Picchetta L, Keller D, Schmidli T, Provenzano M. The prognostic value of indoleamine-2,3-dioxygenase gene expression in urine of prostate cancer patients undergoing radical prostatectomy as first treatment of choice. Front Immunol. (2020) 11:1244. doi: 10.3389/fimmu.2020.01244
24. Landfried K, Zhu W, Waldhier M, Schulz U, Ammer J, Holler B, et al. Tryptophan catabolism is associated with acute GVHD after human allogeneic stem cell transplantation and indicates activation of indoleamine 2,3-dioxygenase. Blood. (2011) 118:6971–4. doi: 10.1182/blood-2011-06-357814
25. Zheng P, Chen J, Huang T, Wang M, Wang Y, Dong M, et al. A novel urinary metabolite signature for diagnosing major depressive disorder. J Proteome Res. (2013) 12:5904–11. doi: 10.1021/pr400939q
26. Gevi F, Zolla L, Gabriele S, Persico A. Urinary metabolomics of young Italian autistic children supports abnormal tryptophan and purine metabolism. Mol Autism. (2016) 7:47. doi: 10.1186/s13229-016-0109-5
27. Molina-Carballo A, Cubero-Millán I, Fernández-López L, Checa-Ros A, Machado-Casas I, Jerez-Calero A, et al. Methylphenidate ameliorates the homeostatic balance between levels of kynurenines in ADHD children. Psychiatry Res. (2021) 303:114060. doi: 10.1016/j.psychres.2021.114060
28. Mason S, Furth A, Solomons R, Wevers R, Reenen M, Reinecke CJ. A putative urinary biosignature for diagnosis and follow-up of tuberculous meningitis in children: outcome of a metabolomics study disclosing host–pathogen responses. Metabolomics. (2016) 7:1–16. doi: 10.1007/s11306-016-1053-2
29. Oh J, Seo H, Kim K, Pyo H, Chung B, Lee J. Urinary profiling of tryptophan and its related metabolites in patients with metabolic syndrome by liquid chromatography-electrospray ionization/mass spectrometry. Anal Bioanal Chem. (2017) 409:5501–12. doi: 10.1007/s00216-017-0486-4
30. Haam J, Lee Y, Suh E, Choi S, Chun H, Kim Y. Urine organic acids may be useful biomarkers for metabolic syndrome and its components in Korean adults. Clin Chem Lab Med. (2021) 59:1824–31. doi: 10.1515/cclm-2021-0598
31. Mussap M, Siracusano M, Noto A, Fattuoni C, Riccioni A, Rajula H, et al. The urine metabolome of young autistic children correlates with their clinical profile severity. Metabolites. (2020) 10:1–21. doi: 10.3390/metabo10110476
32. Heyes M, Garnett E, Brown R. Normal excretion of quinolinic acid in Huntington’s disease. Life Sci. (1985) 37:1811–6. doi: 10.1016/0024-3205(85)90223-1
33. Clària J, Moreau R, Fenaille F, Amorós A, Junot C, Gronbaek H, et al. Orchestration of tryptophan-kynurenine pathway, acute decompensation, and acute-on-chronic liver failure in cirrhosis. Hepatology. (2019) 69:1686–701. doi: 10.1002/hep.30363
34. Pasikanti K, Esuvaranathan K, Hong Y, Ho P, Mahendran R, Raman Nee Mani L, et al. Urinary metabotyping of bladder cancer using two-dimensional gas chromatography time-of-flight mass spectrometry. J Proteome Res. (2013) 12:3865–73. doi: 10.1021/pr4000448
35. Rose D, Toseland P. Urinary excretion of quinolinic acid and other tryptophan metabolites after deoxypyridoxine or oral contraceptive administration. Metabolism. (1973) 22:165–71. doi: 10.1016/0026-0495(73)90267-9
36. Toseland P. The determination of urinary quinolinic acid by gas-liquid chromatography. Clin Chim Acta. (1969) 25:185. doi: 10.1016/0009-8981(69)90250-2
37. Crawford M, Hansen I, Lopez A. The excretion of 3-hydroxyanthranilic and quinolinic acid in Uganda Africans. Br J Cancer. (1969) 23:644–54. doi: 10.1038/bjc.1969.80
38. Hiratsuka C, Sano M, Fukuwatari T, Shibata K. Time-dependent effects of L-tryptophan administration on urinary excretion of L-tryptophan metabolites. J Nutr Sci Vitaminol. (2014) 60:255–60. doi: 10.3177/jnsv.60.255
39. Poesen R, Mutsaers H, Windey K, van den Broek P, Verweij V, Augustijns P, et al. The influence of dietary protein intake on mammalian tryptophan and phenolic metabolites. PLoS One. (2015) 10:e0140820. doi: 10.1371/journal.pone.0140820
40. Nassan F, Gunn J, Hill M, Coull B, Hauser R. High phthalate exposure increased urinary concentrations of quinolinic acid, implicated in the pathogenesis of neurological disorders: is this a potential missing link? Environ Res. (2019) 172:430–6. doi: 10.1016/j.envres.2019.02.034
41. Wang Q, Liu D, Song P, Zou M. Tryptophan-kynurenine pathway is dysregulated in inflammation, and immune activation. Front Biosci. (2015) 20:1116–43. doi: 10.2741/4363
42. Ney D, Murali S, Stroup B, Nair N, Sawin E, Rohr F, et al. Metabolomic changes demonstrate reduced bioavailability of tyrosine and altered metabolism of tryptophan via the kynurenine pathway with ingestion of medical foods in phenylketonuria. Mol Genet Metab. (2017) 121:96–103.
43. Suhre K, Schwartz J, Sharma V, Chen Q, Lee J, Muthukumar T, et al. Urine metabolite profiles predictive of human kidney allograft status. J Am Soc Nephrol. (2016) 27:626–36. doi: 10.1681/ASN.2015010107
44. Bajaj J, Garcia-Tsao G, Reddy K, O’Leary J, Vargas H, Lai J, et al. Admission urinary and serum metabolites predict renal outcomes in hospitalized patients with cirrhosis. Hepatology. (2021) 74:2699–713. doi: 10.1002/hep.31907
45. Raines N, Cheung M, Wilson L, Edberg J, Erdmann N, Schmaier A, et al. Nicotinamide adenine dinucleotide biosynthetic impairment and urinary metabolomic alterations observed in hospitalized adults with COVID-19–related acute kidney injury. Kidney Int Rep. (2021) 6:3002–13. doi: 10.1016/j.ekir.2021.09.001
46. Suhre K, Dadhania D, Lee J, Muthukumar T, Chen Q, Gross S, et al. Kidney allograft function is a confounder of urine metabolite profiles in kidney allograft recipients. Metabolites. (2021) 11:533. doi: 10.3390/metabo11080533
47. Amirdelfan K, Pope J, Gunn J, Hill M, Cotten B, Beresh J, et al. Clinical validation of a multi-biomarker assay for the evaluation of chronic pain patients in a cross-sectional, observational study. Pain Ther. (2020) 9:511–29. doi: 10.1007/s40122-020-00175-3
48. Harutyunyan A, Harutyunyan H, Yenkoyan K. Novel probable glance at inflammatory scenario development in autistic pathology. Front Psychiatry. (2021) 12:788779. doi: 10.3389/fpsyt.2021.788779
49. Drago D, Andolfo A, Mosca E, Orro A, Nocera L, Cucchiara V, et al. A novel expressed prostatic secretion (EPS)-urine metabolomic signature for the diagnosis of clinically significant prostate cancer. Cancer Biol Med. (2021) 18:604–15. doi: 10.20892/j.issn.2095-3941.2020.0617
50. Esturau-Escofet N, Rodríguez de San Miguel E, Vela-Amieva M, García-Aguilera M, Hernández-Espino C, Macias-Kauffer L, et al. A longitudinal 1H NMR-based metabolic profile analysis of urine from hospitalized premature newborns receiving enteral and parenteral nutrition. Metabolites. (2022) 12:255. doi: 10.3390/metabo12030255
51. Banerjee S, Agarwal P. Tryptophan-nicotinic acid metabolism in schizophrenia. Proc Soc Exp Biol Med. (1958) 97:657–9. doi: 10.3181/00379727-97-23837
52. Chojnacki C, Popławski T, Chojnacki J, Fila M, Konrad P, Blasiak J. Tryptophan intake and metabolism in older adults with mood disorders. Nutrients. (2020) 12:3183.
53. Hankes L, De Bruin E, Jansen C, Vorster L, Schmaeler M. Metabolism of 14C-labelled L-tryptophan, L-kynurenine and hydroxy-L-kynurenine in miners with scleroderma. South Afr Med J. (1977) 51:383–90.
54. Noakes R. Effects of tranilast on the urinary excretion of kynurenic and quinolinic acid under conditions of L tryptophan loading. Int J Tryptophan Res. (2013) 6:67–71. doi: 10.4137/IJTR.S12797
55. Heeley A, McCubbing D, Shepherd J. Effect of pyridoxine on the metabolism of tryptophan and branched-chain amino acids in two mentally retarded sibs. Arch Dis Child. (1966) 41:652–7. doi: 10.1136/adc.41.220.652
56. Brown R, Yess N, Price J, Linkswiler H, Swan P, Hankes L. Vitamin B6 depletion in man: urinary excretion of quinolinic acid and niacin metabolites. J Nutr. (1965) 87:419–23. doi: 10.1093/jn/87.4.419
57. Hankes L, Brown R, Schmaeler M. Metabolism of isomers of 3-hydroxykynureninec14 to quinolinic acid, niacin metabolites and carbon dioxide. Proc Soc Exp Biol Med. (1966) 121:253–9. doi: 10.3181/00379727-121-30750
58. Nakagawa I, Oguri S, Sasaki A, Kajimoto M, Sasaki M. Effects of excess intake of leucine and valine deficiency on tryptophan and niacin metabolites in humans. J Nutr. (1975) 105:1241–52. doi: 10.1093/jn/105.10.1241
59. Krishnaswamy K, Rao S, Raghuram T, Srikantia S. Effect of vitamin B6 on leucine-induced changes in human subjects. Am J Clin Nutr. (1976) 29:177–81. doi: 10.1093/ajcn/29.2.177
60. Yeh J, Brown R. Effects of vitamin B-6 deficiency and tryptophan loading on urinary excretion of tryptophan metabolites in mammals. J Nutr. (1977) 107:261–71. doi: 10.1093/jn/107.2.261
61. Patterson J, Brown R, Linkswiler H, Harper A. Excretion of tryptophan-niacin metabolites by young men: effects of tryptophan, leucine, and vitamin B6 intakes. Am J Clin Nutr. (1980) 33:2157–67. doi: 10.1093/ajcn/33.10.2157
62. Hankes L, Schmaeler M, Jansen C, Brown R. Vitamin effects on tryptophan-niacin metabolism in primary hepatoma patients. Adv Exp Med Biol. (1999) 467:283–7. doi: 10.1007/978-1-4615-4709-9_36
63. Fukuwatari T, Shibata K. Effect of nicotinamide administration on the tryptophan-nicotinamide pathway in humans. Int J Vitam Nutr Res. (2007) 77:255–62. doi: 10.1024/0300-9831.77.4.255
64. Hiratsuka C, Fukuwatari T, Shibata K. Fate of dietary tryptophan in young Japanese women. Int J Tryptophan Res. (2012) 5:33–47. doi: 10.4137/IJTR.S10497
65. Shibata K, Hirose J, Fukuwatari T. Method for evaluation of the requirements of B-group vitamins using tryptophan metabolites in human urine. Int J Tryptophan Res. (2015) 8:31–9. doi: 10.4137/IJTR.S24412
66. Cao B, Liu S, Yang L, Chi A. Changes of differential urinary metabolites after high-intensive training in teenage football players. BioMed Res Int. (2020) 2020:e2073803. doi: 10.1155/2020/2073803
67. Nassan F, Gunn J, Hill M, Williams P, Hauser R. Association of urinary concentrations of phthalate metabolites with quinolinic acid among women: a potential link to neurological disorders. Environ Int. (2020) 138:105643. doi: 10.1016/j.envint.2020.105643
Keywords: quinolinic acid (QA), tryptophan, urine, NAD+, inflammation, NAD+ biosynthesis, metabolism, quinolinate phosphoribosyl transferase (QPRT)
Citation: Saade MC, Clark AJ and Parikh SM (2022) States of quinolinic acid excess in urine: A systematic review of human studies. Front. Nutr. 9:1070435. doi: 10.3389/fnut.2022.1070435
Received: 17 October 2022; Accepted: 28 November 2022;
Published: 16 December 2022.
Edited by:
Hui-Xin Liu, China Medical University, ChinaReviewed by:
Shayne Mason, North-West University, South AfricaIman Zarei, University of Eastern Finland, Finland
Copyright © 2022 Saade, Clark and Parikh. This is an open-access article distributed under the terms of the Creative Commons Attribution License (CC BY). The use, distribution or reproduction in other forums is permitted, provided the original author(s) and the copyright owner(s) are credited and that the original publication in this journal is cited, in accordance with accepted academic practice. No use, distribution or reproduction is permitted which does not comply with these terms.
*Correspondence: Samir M. Parikh, ✉ c2FtaXIucGFyaWtoQHV0c291dGh3ZXN0ZXJuLmVkdQ==