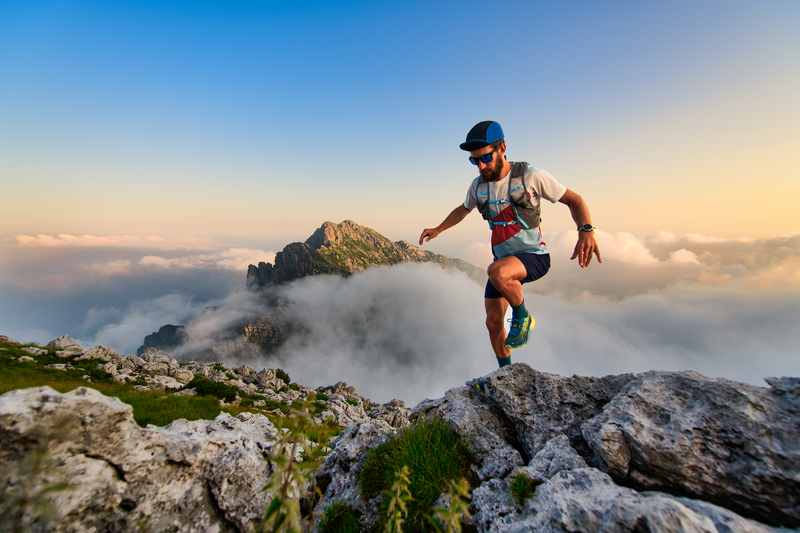
94% of researchers rate our articles as excellent or good
Learn more about the work of our research integrity team to safeguard the quality of each article we publish.
Find out more
MINI REVIEW article
Front. Nutr. , 24 November 2022
Sec. Clinical Nutrition
Volume 9 - 2022 | https://doi.org/10.3389/fnut.2022.1067647
This article is part of the Research Topic Environmental Factors Implicated in Obesity View all 11 articles
Studies in human microbiota dysbiosis have shown that short-chain fatty acids (SCFAs) like propionate, acetate, and particularly butyrate, positively affect energy homeostasis, behavior, and inflammation. This positive effect can be demonstrated in the reduction of butyrate-producing bacteria observed in the gut microbiota of individuals with type 2 diabetes (T2DM) and other energy-associated metabolic alterations. Butyrate is the major end product of dietary fiber bacterial fermentation in the large intestine and serves as the primary energy source for colonocytes. In addition, it plays a key role in reducing glycemia and improving body weight control and insulin sensitivity. The major mechanisms involved in butyrate regulation include key signaling pathways such as AMPK, p38, HDAC inhibition, and cAMP production/signaling. Treatment strategies using butyrate aim to increase its intestine levels, bioavailability, and improvement in delivery either through direct supplementation or by increasing dietary fiber in the diet, which ultimately generates a higher production of butyrate in the gut. In the final part of this review, we present a summary of the most relevant studies currently being carried out in humans.
The World Health Organization (WHO) defines obesity as an abnormal fat accumulation that may impair health (1). The evidence of the relationship between the gut microbiome and the development of obesity and type 2 diabetes mellitus (T2DM) has been rising for the last decade (2). The gut microbiota plays a pivotal role in regulating energy homeostasis. Among other host factors, this balance between energy intake and expenditure relies on the microorganisms and their metabolites, helping in nutrient processing, nutrient access regulation, and storage in the body by secreting hormones and mediators of energy homeostasis (3). Some glycemic alterations that cause a detrimental cascade effect have also been linked to chronic inflammation, cardiovascular diseases, and gut dysbiosis, where the loss of several butyrate-producing bacteria has been observed (4).
The short-chain fatty acids (SCFAs) are ingested through diet or produced by fermentation of non-digestible fiber by gut microbiota; the three major SCFAs produced are butyrate, propionate, and acetate (5). After production or consumption, butyrate, the SCFA with the most important systemic effects, is absorbed rapidly in the gut and acts as a source of energy and a signaling molecule in numerous cell types (6). It has been reported to have metabolic effects on obesity and glucose homeostasis. In a recent study, individuals with obesity and T2DM showed a decreased abundance of butyrate-producing bacteria and downregulation of genes related to butyrate production (7). Despite these findings, its exact role remains unclear since obese individuals present higher fecal butyrate concentrations than the control group, with similar diet consumption (8, 9). Restoration of butyrate-producing bacteria and butyrate levels by ingesting butyrate-rich foods or dietary fibers that lead to butyrate production might provide new treatment options for T2DM and obesity-related metabolic diseases (5). Here we review the basic mechanisms that explain the role of butyrate in this context.
SCFAs, including butyrate, are present in high amounts in milk and milk derivatives from different mammals. Bovine fat milk and its derivatives are a great source of butyric acid, e.g., butter (∼3 g/100 g), goat’s cheese (∼1–1.8 g/100 g), parmesan (∼1.5 g/100 g), whole cow’s milk (∼0.1 g/100 g) (10). Human milk (HM) has also been reported as a source of butyrate, where the concentration measured in HM samples from healthy women fluctuates between 0.15 and 1.93 mM in colostrum and 0.16–1.97 mM in mature milk. Considering a median butyrate concentration of 0.75 mM in mature HM, a breastfed infant could receive a daily dose of butyrate of approximately 30 mg/kg body weight (11).
Salatrims, a fat calorie replacer commonly used in the food industry, is also a source of dietary butyrate. Salatrim has triglyceride mixtures in which butyric acid is inter-esterified with a long-chain fatty acid moiety such as stearic acid (12, 13). Since butyrate is esterified at the α(sn-3) position (14, 15), pancreatic lipase can cleave triacylglycerols releasing free fatty acids (FFA) in the small intestine (16, 17). To prevent digestion -and absorption- in the upper part of the gastrointestinal tract, butyrate might be esterified to a dietary fiber such as butyrylated or tributyrin, in which butyrate is esterified to triglycerides; as a result, these esterificated form of butyrate have also been shown to increase colonic butyrate concentrations (18, 19).
In most human clinical and rodent studies focused on obesity and diabetes, butyrate is supplied orally as sodium butyrate, which has an unpalatable flavor and odor. Novel strategies have been developed recently to improve palatability and the release and absorption of butyrate in the digestive tract (20). Coating sodium butyrate with cellulose-based capsules has been one approach to delay the release in the intestinal tract (21). According to pharmacological and clinical data from the literature, butyric acid is considered a safe drug. Therapeutic doses (150–300 mg) have shown no clinical side effects (22). Even when higher doses up to 2,000 mg/day, no adverse reactions have been observed (22, 23)
On the other hand, dietary fiber is used by some bacteria in the gut microbiota for butyrate production in two different ways: (I) Direct, where fiber acts as a substrate for bacterial fermentation producing butyrate, (II) Indirect since bifidogenic fibers help to increase the abundance of bifidobacteria, which increase butyrate production indirectly (24).
Maybe, the most efficient way to influence the composition of intestinal and colon microbiota is by ingestion of dietary fibers such as inulin-type fructans, xylooligosaccharides, arabinoxylans, arabinoxylan oligosaccharides, β-glucans, and oligofructose (25–28). Important foods to increase intestinal butyrate are complex polysaccharides not easily digested by saliva and pancreatic amylases. For instance, resistant starch, a group of molecules that resist digestion, may be added or fortified into bread and cereals (29) but is also found naturally occurring in some legumes, cooked potatoes, and unripened bananas. Studies have shown that resistant starch potentiates butyrate production and yields more butyrate than non-starch polysaccharides (30, 31). Other non-easily digested polysaccharides producing butyrate include cereal breakfasts, such as barley and oats, containing β-glucans, which are also naturally present in edible mushrooms and seaweed (32). Finally, inulin, on the other hand, is mainly found in artichokes, onion, and chicory roots (33) are also good foods to increase intestinal butyrate.
A high-fiber diet can cause gastrointestinal discomforts, such as gas, bloating, and constipation in patients with Crohn’s disease, irritable bowel syndrome, or ulcerative colitis. This is why a gradual increase in fiber intake is recommended for everyone (34).
Although butter is the most abundant source of dietary butyrate (up to 3 g per 100 g), the best way to increase the amount of intestinal butyrate is by consuming non-digestible carbohydrates (complex polysaccharides) to increase in situ production by human gut microbiota (35). Intestinal butyrate is produced by obligate anaerobic bacteria through fermentation (36). Most human butyrate producers belong to the Firmicutes phylum including species such as Clostridium butyricum, Clostridium kluyveri, Faecalibacterium prausnitzii, Butyrivibrio fibrisolvens, Eubacterium limosum (37–39).
Although several routes for the production of butyrate have been described, in human gut microbiota, butyrate is mainly synthesized from acetyl-coenzyme A (Ac-CoA) obtained from the breakdown of complex carbohydrates (e.g., xylan, starch) as a precursor (18, 37). Subsequently, two molecules of AcCoA condense into acetoacetyl CoA, and after several consecutive steps, it is transformed into butyrate (40). The final step in butyrogenesis is the conversion of butyryl-phosphate into butyrate by butyrate kinase encoded by the buk gene or butyryl-CoA to butyrate by butyryl-CoA: acetate-CoA transferase, encoded by the but gene (41). In addition to the colonization of the colon by butyrogenic bacteria, it has been proposed that cross-feeding interactions between Bifidobacterial strains and F. prausnitzii may ultimately enhance butyrate production (42).
Whether butyrate is ingested through the diet or produced locally in the intestine from dietary fiber, it is absorbed into the enterocytes by diffusion and delivered through the portal vein into the liver and systemic circulation (43, 44). Due to its size and hydrophobicity, butyrate, like propionate and acetate, are absorbed through a non-ionic diffusion across the apical membrane of colonocytes (45, 46). Sodium-coupled monocarboxylate transporter 1 (SCMT1) utilizes colonic concentration of Na+ to internalize SCFAs within colonocytes. It has been described that SCMT1 transports propionate acetate at a slower rate compared to butyrate transport. The solute carrier family 5 member 8 (SLC5A8) is considered the primary butyrate transporter across the apical membrane of the colonocytes (47, 48).
The liver is the master organ for regulating energy homeostasis, particularly for lipid and glucose metabolism regulation. The liver plays a central role in the development of obesity-associated metabolic alterations. It is, therefore, highly relevant to outline the effect of butyrate on regulating lipid metabolism and liver function. Recent studies showed that butyrate supplementation reduced serum triglyceride levels and the respiratory exchange ratio in high-fat diet (HFD)-fed animals compared to controls with HFD only, suggesting that butyrate may exert its effect by promoting fatty acid oxidation (49–53). In addition, butyrate also reduced lipid content in brown adipose tissue (BAT) and, to a lesser extent, in the liver and muscle (51). Additionally, demonstrating its protective role, butyrate supplementation in animals with HFD resulted in a significant reduction of proinflammatory serum markers (TNF-α, MCP-1, and IL-1β) compared to the markers of animals with HFD only (50).
Although butyrate mechanisms of action are unclear, previous studies show that butyrate modulates the AMP/ATP ratio activating the AMPK pathway to promote oxidative metabolism (decrease lipid synthesis and increase lipid oxidation) in the liver and adipose tissue (53). In addition, butyrate increases the percentage of oxidative type fibers (actively using lipid oxidation for ATP biosynthesis) in skeletal muscle by activating AMPK and p38 (54) and increases mitochondrial function in skeletal muscle (49) and liver (50).
In addition to its well-known effects on intestine function (55), butyrate is a critical link between gut microbiota and the regulation of host energy homeostasis. Results from several groups have provided evidence supporting this role. For instance, chronic sodium butyrate supplementation in food (49, 51, 53, 54, 56) or orally delivered via gavage (50, 57–59) reduces body weight gain and fat mass of mice fed with a HFD compared to mice fed with an HFD alone, suggesting that butyrate can prevent or contribute for the treatment of diet-induced obesity (DIO). Also in agreement with this regulating role, intraperitoneal (IP) injection of sodium butyrate for ten consecutive weeks reduced body weight gain of rats treated with butyrate (60). A mechanism explaining the effect of butyrate on reducing body weight and fat mass is the activation of lipid oxidation initiated by butyrate in BAT and the liver (51). In addition to the increased energy expenditure and lipid oxidation, reduced food intake, also described as an effect of oral butyrate supplementation but not an intravenous injection, may contribute to decreased body weight gain induced by butyrate and reduced fat mass (51). The lack of effect of intravenous injection of butyrate suggests a role of this SCFA in regulating the gut-brain circuit.
Although there is evidence that sodium butyrate supplementation in standard diet-fed rats reduced weight gain (55), the effects of butyrate under chow diet-fed conditions remain controversial. There are contradicting results from other studies claimed that a chow diet plus 1% or 5% butyrate did not significantly affect body weight in mice (50, 61) and even that offspring rats of mothers fed a 1% butyrate in a chow diet had higher body weight (62). Major milestones of butyrate action and results from key therapeutic trials are summarized in Table 1.
As it has been extensively documented in the literature, insulin resistance can be attributed to a decrease in receptor sensitivity together with the functional impairment of β-cells in the pancreatic islets (63). Histological studies of human islet tissue have shown that butyrate has a protective effect against oxidative and mitochondrial stress promoting the survival of β-cells in vitro (64, 65). Remarkably, initial analysis of the conditions associated with β-cells autoimmunity in children at risk of type-1 diabetes mellitus (T1DM) found a reduction in the average population of butyrate-producing bacteria (66, 67). There is evidence that butyrate is involved in the metabolism of β-cells in the pancreatic islets due to its interaction with G-protein coupled receptors (GPR) like free fatty acid receptors FFAR3 (GPR41) and FFAR2 (GPR43), as seen in Figure 1 (64, 68, 69). In light of this association, one study suggested that butyrate could be responsible for a proliferative effect during in vitro mouse intestinal organoid development due to its interactions with GPR41 and 43 receptors (70).
Figure 1. Butyrate regulation pathways in β-cells (pancreatic islets). Butyrate is involved in β-cells metabolism via G protein-coupled receptors (GPRs) GPR41 and GPR43. Butyrate can also enter the cells via monocarboxylate transporters and inhibit histone deacetylases (HDAC) which are thought to be involved in gluconeogenesis signaling. Butyrate can also regulate the cAMP signaling for insulin secretion via glucagon-like peptide-1 receptors (GLP-1R).
Animal data in obese mice have shown that butyrate administration rapidly decreased fasting insulin levels (50, 71). As a result, aside from its protective role in β-cells, butyrate has been proposed as a direct regulator of insulin secretion via GPR-mediated signaling. However, this direct role remains unconfirmed and controversial (72). Nevertheless, recent findings suggest sodium-butyrate treatment can indirectly enhance insulin secretion by repressing β-cell key functional genes in rat islets (73). More evidence about the indirect role of butyrate during insulin secretion has emerged after studies reported its involvement with glucagon-like peptide-1 (GLP-1) secretion from intestinal L-cells (74, 75). Activation of the GLP-1R (receptor) genes, also present in β-cell, and subsequent GLP-1 release can also be induced by butyrate (76). GLP-1 has the potential to minimize apoptotic events and induce neogenesis and regeneration of β-cells via cAMP (cyclic adenosine monophosphate) upregulation (77). Production of cAMP can also elicit postprandial-like insulin secretion by accelerating the glucose-dependent closure of ATP-regulated potassium channels (77–79).
On the other hand, the inhibitory activity of butyrate toward histone deacetylases (HDAC) has also been extensively described in literature due to HDAC involvement in transcriptional regulation, metabolism, metastasis, oncogenesis, and ischemic brain events (80, 81). HDACs have also been linked to hyperglycemia by promoting gluconeogenesis in the liver; therefore, becoming an important target to regulate glucose levels via the administration of HDAC inhibitors like metformin (a first-line antihyperglycemic drug for T2DM treatment) (82, 83). Type-2 diabetic animal studies have revealed that butyrate has similar effects to metformin in reducing insulin resistance and other T2DM-associated conditions (60). In addition, the role of butyrate-mediated HDAC inhibition has been described as an enhancer in the differentiation and maturation of β-cells in neonatal porcine islets (84). Altogether, there is strong evidence about the crucial role of butyrate and its interactions with insulin-secreting β-cells. The potential regulation of gluconeogenesis via HDAC and the robust induction of insulin secretion via GLP-1 appoint butyrate administration as a potential target for research in diabetes treatment.
Consequently, several therapeutic interventions have already been conducted to assess the effects of butyrate supplementation in diabetic patients and patients with obesity and metabolic syndrome (Table 1). Some trials reported a positive outcome after treatment with oral butyrate with a reduction in the patient’s blood pressure and blood sugar levels (85, 86). In addition, other trials also found significant reductions in HbA1c (hemoglobin-A1c: glucose linked to hemoglobin in red blood cells), total cholesterol, and triglycerides (87). On the other hand, several studies contradict some of these findings and report no significant effects on sugar levels, insulin sensitivity, and secretion (87, 88). One study suggests that butyrate therapy benefits healthy individuals, but this outcome is not reflected in patients with metabolic syndrome (89). Overall, there are still some limitations on these data, which may prevent direct comparisons and conclusions. Some of these trials may be conditioned by the short duration of butyrate administration, small sample size, lack of placebo control, and variability within the target population. Moreover, butyrate combination therapies with other potential antidiabetic drugs are still unexplored, so further research is encouraged.
In addition to the described effects on intestinal function and metabolic control, the system-level impacts of butyrate remain elusive, and the detailed molecular mechanisms responsible for butyrate action in host and microbial cells are still a very active research field. On the other hand, to detail the effects of butyrate on host metabolism and further promote butyrate as a therapeutic approach for designing novel clinical trials, it is critical to identify its effects on different animals and humans and evaluate different doses, treatment times and delivery methods. In addition, although many studies support the role of butyrate as an essential mediator in host metabolic control, some of its effects remain controversial.
Highly relevant questions in this exciting field are still awaiting elucidation. They should be decoded, including but not limited to determining the best conditions and food sources for butyrate production by gut microbiota in situ, absorption of dietary and microbially produced butyrate under different physiological and pathological conditions, the regulatory mechanisms of butyrate at a cellular and systemic levels and the potential of using it as a therapeutic alternative in some obesity-associated metabolic alterations.
CB-O and LG: conceptualization and research/investigation. AM-R, DS-R, and CB-O: writing—original draft preparation. LG and DS-R: writing—review and editing. LG: supervision. All authors contributed to the article and approved the submitted version.
We thank the Corporación Ecuatoriana para la Investigación y la Academia (CEDIA) and its funds from CEPRA XII-2018-10 granted to LG.
The authors declare that the research was conducted in the absence of any commercial or financial relationships that could be construed as a potential conflict of interest.
All claims expressed in this article are solely those of the authors and do not necessarily represent those of their affiliated organizations, or those of the publisher, the editors and the reviewers. Any product that may be evaluated in this article, or claim that may be made by its manufacturer, is not guaranteed or endorsed by the publisher.
2. Ley RE. Obesity and the human microbiome. Curr Opin Gastroenterol. (2010) 26:5–11. doi: 10.1097/MOG.0b013e328333d751
3. Cani PD. Human gut microbiome: hopes, threats and promises. Gut. (2018) 67:1716–25. doi: 10.1136/gutjnl-2018-316723
4. Noureldein MH, Bitar S, Youssef N, Azar S, Eid AA. Butyrate modulates diabetes-linked gut dysbiosis: epigenetic and mechanistic modifications. J Mol Endocrinol. (2020) 64:29–42. doi: 10.1530/JME-19-0132
5. Arora T, Tremaroli V. Therapeutic potential of butyrate for treatment of type 2 diabetes. Front Endocrinol. (2021) 12:761834. doi: 10.3389/fendo.2021.761834
6. Valdes AM, Walter J, Segal E, Spector TD. Role of the gut microbiota in nutrition and health. BMJ. (2018) 361:k2179. doi: 10.1136/bmj.k2179
7. Brahe LK, Astrup A, Larsen LH. Is butyrate the link between diet, intestinal microbiota and obesity-related metabolic diseases? Obes Rev. (2013) 14:950–9. doi: 10.1111/obr.12068
8. Patil DP, Dhotre DP, Chavan SG, Sultan A, Jain DS, Lanjekar VB, et al. Molecular analysis of gut microbiota in obesity among Indian individuals. J Biosci. (2012) 37:647–57. doi: 10.1007/s12038-012-9244-0
9. Schwiertz A, Taras D, Schäfer K, Beijer S, Bos NA, Donus C, et al. Microbiota and SCFA in lean and overweight healthy subjects. Obesity. (2010) 18:190–5. doi: 10.1038/oby.2009.167
10. U.S. Department Of Agriculture. USDA National Nutrient Database for Standard Reference. USDA National Nutrient Database for Standard Reference. (2019). Available online at: https://fdc.nal.usda.gov/ndb/ (accessed October 1, 2022).
11. Paparo L, Nocerino R, Ciaglia E, Di Scala C, De Caro C, Russo R, et al. Butyrate as a bioactive human milk protective component against food allergy. Allergy. (2021) 76:1398–415. doi: 10.1111/all.14625
12. Moossavi S, Sepehri S, Robertson B, Bode L, Goruk S, Field CJ, et al. Composition and variation of the human milk microbiota are influenced by maternal and early-life factors. Cell Host Microbe. (2019) 25:324–35.e4. doi: 10.1016/j.chom.2019.01.011
13. Smith RE, Finley JW, Leveille GA. Overview of SALATRIM: a family of low-calorie fats. J Agric Food Chem. (1994) 42:432–4. doi: 10.1021/jf00038a036
14. Wang M, Li M, Wu S, Lebrilla CB, Chapkin RS, Ivanov I, et al. Fecal microbiota composition of breast-fed infants is correlated with human milk oligosaccharides consumed. J Pediatr Gastroenterol Nutr. (2015) 60:825–33. doi: 10.1097/MPG.0000000000000752
15. Iqbal J, Hussain MM. Intestinal lipid absorption. Am J Physiol Endocrinol Metab. (2009) 296:E1183–94. doi: 10.1152/ajpendo.90899.2008
16. Walker WA, Iyengar RS. Breast milk, microbiota, and intestinal immune homeostasis. Pediatr Res. (2015) 77:220–8. doi: 10.1038/pr.2014.160
17. Padilha M, Danneskiold-Samsøe NB, Brejnrod A, Hoffmann C, Cabral VP, Iaucci JM, et al. The human milk microbiota is modulated by maternal diet. Microorganisms. (2019) 7:502. doi: 10.3390/microorganisms7110502
18. Clarke JM, Topping DL, Christophersen CT, Bird AR, Lange K, Saunders I, et al. Butyrate esterified to starch is released in the human gastrointestinal tract. Am J Clin Nutr. (2011) 94:1276–83. doi: 10.3945/ajcn.111.017228
19. West NP, Christophersen CT, Pyne DB, Cripps AW, Conlon MA, Topping DL, et al. Butyrylated starch increases colonic butyrate concentration but has limited effects on immunity in healthy physically active individuals. Exerc Immunol Rev. (2013) 19:102–19.
20. Roda A, Simoni P, Magliulo M, Nanni P, Baraldini M, Roda G, et al. A new oral formulation for the release of sodium butyrate in the ileo-cecal region and colon. World J Gastroenterol. (2007) 13:1079–84. doi: 10.3748/wjg.v13.i7.1079
21. Weng H, Endo K, Li J, Kito N, Iwai N. Induction of peroxisomes by butyrate-producing probiotics. PLoS One. (2015) 10:e0117851. doi: 10.1371/journal.pone.0117851
22. Banasiewicz T, Domagalska D, Borycka-Kiciak K, Rydzewska G. Determination of butyric acid dosage based on clinical and experimental studies - a literature review. Prz Gastroenterol. (2020) 15:119–25. doi: 10.5114/pg.2020.95556
23. Karimi G, Vahabzadeh M. Butyric acid. In: Encyclopedia of Toxicology, ed. Elsevier. Amsterdam: Elsevier (2005). p. 597–601. doi: 10.1016/B978-0-12-386454-3.00591-1
24. van Deuren T, Blaak EE, Canfora EE. Butyrate to combat obesity and obesity-associated metabolic disorders: current status and future implications for therapeutic use. Obes Rev. (2022) 23:e13498. doi: 10.1111/obr.13498
25. Rivière A, Gagnon M, Weckx S, Roy D, De Vuyst L. Mutual Cross-Feeding Interactions between Bifidobacterium longum subsp. longum NCC2705 and Eubacterium rectale ATCC 33656 explain the bifidogenic and butyrogenic effects of arabinoxylan oligosaccharides. Appl Environ Microbiol. (2015) 81:7767–81. doi: 10.1128/AEM.02089-15
26. Bach Knudsen KE. Microbial degradation of whole-grain complex carbohydrates and impact on short-chain fatty acids and health. Adv Nutr. (2015) 6:206–13. doi: 10.3945/an.114.007450
27. Sajilata MG, Singhal RS, Kulkarni PR. Resistant starch-a review. Comp Rev Food Sci Food Safety. (2006) 5:1–17. doi: 10.1111/j.1541-4337.2006.tb00076.x
28. Ahmed W, Rashid S. Functional and therapeutic potential of inulin: a comprehensive review. Crit Rev Food Sci Nutr. (2019) 59:1–13. doi: 10.1080/10408398.2017.1355775
29. Amini Khoozani A, Kebede B, Birch J, Bekhit AE-DA. The effect of bread fortification with whole green banana flour on its physicochemical, nutritional and in vitro digestibility. Foods. (2020) 9:152. doi: 10.3390/foods9020152
30. Harvard TH. Chan School of Public Health TNS. Legumes and Resistant Starch. Legumes and Resistant Starch. (2015). Available online at: https://www.hsph.harvard.edu/nutritionsource/2015/11/16/ask-the-expert-legumes-and-resistant-starch/ (accessed October 10, 2022).
31. Birkett AM, Brown IL. Resistant starch. In: CJK Henry editor. Novel food Ingredients for Weight Control. Amsterdam: Elsevier (2007). p. 174–97. doi: 10.1533/9781845693114.2.174
32. Mirończuk-Chodakowska I, Kujawowicz K, Witkowska AM. Beta-glucans from fungi: biological and health-promoting potential in the COVID-19 pandemic era. Nutrients. (2021) 13:3960. doi: 10.3390/nu13113960
33. Zeaiter Z, Regonesi ME, Cavini S, Labra M, Sello G, Di Gennaro P. Extraction and characterization of inulin-type fructans from artichoke wastes and their effect on the growth of intestinal bacteria associated with health. Biomed Res Int. (2019) 2019:1083952. doi: 10.1155/2019/1083952
34. Ho K-S, Tan CYM, Mohd Daud MA, Seow-Choen F. Stopping or reducing dietary fiber intake reduces constipation and its associated symptoms. World J Gastroenterol. (2012) 18:4593–6. doi: 10.3748/wjg.v18.i33.4593
35. Stilling RM, van de Wouw M, Clarke G, Stanton C, Dinan TG, Cryan JF. The neuropharmacology of butyrate: The bread and butter of the microbiota-gut-brain axis? Neurochem Int. (2016) 99:110–32. doi: 10.1016/j.neuint.2016.06.011
36. Koh A, De Vadder F, Kovatcheva-Datchary P, Bäckhed F. From dietary fiber to host physiology: short-chain fatty acids as key bacterial metabolites. Cell. (2016) 165:1332–45. doi: 10.1016/j.cell.2016.05.041
37. Louis P, Flint HJ. Diversity, metabolism and microbial ecology of butyrate-producing bacteria from the human large intestine. FEMS Microbiol Lett. (2009) 294:1–8. doi: 10.1111/j.1574-6968.2009.01514.x
38. Van den Abbeele P, Belzer C, Goossens M, Kleerebezem M, De Vos WM, Thas O, et al. Butyrate-producing Clostridium cluster XIVa species specifically colonize mucins in an in vitro gut model. ISME J. (2013) 7:949–61. doi: 10.1038/ismej.2012.158
39. Vital M, Howe AC, Tiedje JM. Revealing the bacterial butyrate synthesis pathways by analyzing (meta)genomic data. mBio. (2014) 5:e00889. doi: 10.1128/mBio.00889-14
40. Bennett G. The central metabolic pathway from acetyl-CoA to butyryl-CoA in Clostridium acetobutylicum. FEMS Microbiol Rev. (1995) 17:241–9. doi: 10.1016/0168-6445(95)00011-Z
41. Vital M, Penton CR, Wang Q, Young VB, Antonopoulos DA, Sogin ML, et al. A gene-targeted approach to investigate the intestinal butyrate-producing bacterial community. Microbiome. (2013) 1:8. doi: 10.1186/2049-2618-1-8
42. Kim H, Jeong Y, Kang S, You HJ, Ji GE. Co-culture with bifidobacterium catenulatum improves the growth, gut colonization, and butyrate production of Faecalibacterium prausnitzii: in vitro and in vivo studies. Microorganisms. (2020) 8:788. doi: 10.3390/microorganisms8050788
43. Bedford A, Gong J. Implications of butyrate and its derivatives for gut health and animal production. Anim Nutr. (2018) 4:151–9. doi: 10.1016/j.aninu.2017.08.010
44. Salvi PS, Cowles RA. Butyrate and the intestinal epithelium: modulation of proliferation and inflammation in homeostasis and disease. Cells. (2021) 10:1775. doi: 10.3390/cells10071775
45. Inagaki A, Hayashi M, Andharia N, Matsuda H. Involvement of butyrate in electrogenic K+ secretion in rat rectal colon. Pflugers Arch. (2019) 471:313–27. doi: 10.1007/s00424-018-2208-y
46. Charney AN, Micic L, Egnor RW. Nonionic diffusion of short-chain fatty acids across rat colon. Am J Physiol. (1998) 274:G518–24. doi: 10.1152/ajpgi.1998.274.3.G518
47. Rackwitz R, Dengler F, Gäbel G. Butyrate permeation across the isolated ovine reticulum epithelium. Animals. (2020) 10:2198. doi: 10.3390/ani10122198
48. Ganapathy V, Thangaraju M, Gopal E, Martin PM, Itagaki S, Miyauchi S, et al. Sodium-coupled monocarboxylate transporters in normal tissues and in cancer. AAPS J. (2008) 10:193–9. doi: 10.1208/s12248-008-9022-y
49. Gao Z, Yin J, Zhang J, Ward RE, Martin RJ, Lefevre M, et al. Butyrate improves insulin sensitivity and increases energy expenditure in mice. Diabetes. (2009) 58:1509–17. doi: 10.2337/db08-1637
50. Mollica MP, Mattace Raso G, Cavaliere G, Trinchese G, De Filippo C, Aceto S, et al. Butyrate regulates liver mitochondrial function, efficiency, and dynamics in insulin-resistant obese mice. Diabetes. (2017) 66:1405–18. doi: 10.2337/db16-0924
51. Li Z, Yi C-X, Katiraei S, Kooijman S, Zhou E, Chung CK, et al. Butyrate reduces appetite and activates brown adipose tissue via the gut-brain neural circuit. Gut. (2018) 67:1269–79. doi: 10.1136/gutjnl-2017-314050
52. Lu Y, Fan C, Li P, Lu Y, Chang X, Qi K. Short chain fatty acids prevent high-fat-diet-induced obesity in mice by regulating G protein-coupled receptors and gut microbiota. Sci Rep. (2016) 6:37589. doi: 10.1038/srep37589
53. den Besten G, Bleeker A, Gerding A, van Eunen K, Havinga R, van Dijk TH, et al. Short-chain fatty acids protect against high-fat diet-induced obesity via a PPARγ-dependent switch from lipogenesis to fat oxidation. Diabetes. (2015) 64:2398–408. doi: 10.2337/db14-1213
54. Henagan TM, Stefanska B, Fang Z, Navard AM, Ye J, Lenard NR, et al. Sodium butyrate epigenetically modulates high-fat diet-induced skeletal muscle mitochondrial adaptation, obesity and insulin resistance through nucleosome positioning. Br J Pharmacol. (2015) 172:2782–98. doi: 10.1111/bph.13058
55. De Vadder F, Kovatcheva-Datchary P, Goncalves D, Vinera J, Zitoun C, Duchampt A, et al. Microbiota-generated metabolites promote metabolic benefits via gut-brain neural circuits. Cell. (2014) 156:84–96. doi: 10.1016/j.cell.2013.12.016
56. Lin HV, Frassetto A, Kowalik EJ, Nawrocki AR, Lu MM, Kosinski JR, et al. Butyrate and propionate protect against diet-induced obesity and regulate gut hormones via free fatty acid receptor 3-independent mechanisms. PLoS One. (2012) 7:e35240. doi: 10.1371/journal.pone.0035240
57. Wang D, Liu C-D, Li H-F, Tian M-L, Pan J-Q, Shu G, et al. LSD1 mediates microbial metabolite butyrate-induced thermogenesis in brown and white adipose tissue. Metab Clin Exp. (2020) 102:154011. doi: 10.1016/j.metabol.2019.154011
58. Fang W, Xue H, Chen X, Chen K, Ling W. Supplementation with sodium butyrate modulates the composition of the gut microbiota and ameliorates high-fat diet-induced obesity in mice. J Nutr. (2019) 149:747–54. doi: 10.1093/jn/nxy324
59. Hong J, Jia Y, Pan S, Jia L, Li H, Han Z, et al. Butyrate alleviates high fat diet-induced obesity through activation of adiponectin-mediated pathway and stimulation of mitochondrial function in the skeletal muscle of mice. Oncotarget. (2016) 7:56071–82. doi: 10.18632/oncotarget.11267
60. Khan S, Jena G. Sodium butyrate reduces insulin-resistance, fat accumulation and dyslipidemia in type-2 diabetic rat: a comparative study with metformin. Chem Biol Interact. (2016) 254:124–34. doi: 10.1016/j.cbi.2016.06.007
61. Aguilar EC, da Silva JF, Navia-Pelaez JM, Leonel AJ, Lopes LG, Menezes-Garcia Z, et al. Sodium butyrate modulates adipocyte expansion, adipogenesis, and insulin receptor signaling by upregulation of PPAR-γ in obese Apo E knockout mice. Nutrition. (2018) 47:75–82. doi: 10.1016/j.nut.2017.10.007
62. Huang Y, Gao S, Chen J, Albrecht E, Zhao R, Yang X. Maternal butyrate supplementation induces insulin resistance associated with enhanced intramuscular fat deposition in the offspring. Oncotarget. (2017) 8:13073–84. doi: 10.18632/oncotarget.14375
63. Javeed N, Matveyenko AV. Circadian etiology of type 2 diabetes mellitus. Physiology. (2018) 33:138–50. doi: 10.1152/physiol.00003.2018
64. Hu S, Kuwabara R, de Haan BJ, Smink AM, de Vos P. Acetate and butyrate improve β-cell metabolism and mitochondrial respiration under oxidative stress. Int J Mol Sci. (2020) 21:1542. doi: 10.3390/ijms21041542
65. Hu Y, Liu J, Yuan Y, Chen J, Cheng S, Wang H, et al. Sodium butyrate mitigates type 2 diabetes by inhibiting PERK-CHOP pathway of endoplasmic reticulum stress. Environ Toxicol Pharmacol. (2018) 64:112–21. doi: 10.1016/j.etap.2018.09.002
66. de Goffau MC, Luopajärvi K, Knip M, Ilonen J, Ruohtula T, Härkönen T, et al. Fecal microbiota composition differs between children with β-cell autoimmunity and those without. Diabetes. (2013) 62:1238–44. doi: 10.2337/db12-0526
67. Honkanen J, Vuorela A, Muthas D, Orivuori L, Luopajärvi K, Tejesvi MVG, et al. Fungal dysbiosis and intestinal inflammation in children with beta-cell autoimmunity. Front Immunol. (2020) 11:468. doi: 10.3389/fimmu.2020.00468
68. McNelis JC, Lee YS, Mayoral R, van der Kant R, Johnson AMF, Wollam J, et al. GPR43 potentiates β-cell function in obesity. Diabetes. (2015) 64:3203–17. doi: 10.2337/db14-1938
69. Priyadarshini M, Villa SR, Fuller M, Wicksteed B, Mackay CR, Alquier T, et al. An acetate-specific GPCR, FFAR2, regulates insulin secretion. Mol Endocrinol. (2015) 29:1055–66. doi: 10.1210/me.2015-1007
70. Park J-H, Kotani T, Konno T, Setiawan J, Kitamura Y, Imada S, et al. Promotion of intestinal epithelial cell turnover by commensal bacteria: role of short-chain fatty acids. PLoS One. (2016) 11:e0156334. doi: 10.1371/journal.pone.0156334
71. Gao F, Lv Y-W, Long J, Chen J-M, He J-M, Ruan X-Z, et al. Butyrate improves the metabolic disorder and gut microbiome dysbiosis in mice induced by a high-fat diet. Front Pharmacol. (2019) 10:1040. doi: 10.3389/fphar.2019.01040
72. Liu J-L, Segovia I, Yuan X-L, Gao Z-H. Controversial roles of gut microbiota-derived short-chain fatty acids (SCFAs) on pancreatic β-cell growth and insulin secretion. Int J Mol Sci. (2020) 21:910. doi: 10.3390/ijms21030910
73. Wang S, Yuan M, Zhang L, Zhu K, Sheng C, Zhou F, et al. Sodium butyrate potentiates insulin secretion from rat islets at the expense of compromised expression of β cell identity genes. Cell Death Dis. (2022) 13:67. doi: 10.1038/s41419-022-04517-1
74. Yadav H, Lee J-H, Lloyd J, Walter P, Rane SG. Beneficial metabolic effects of a probiotic via butyrate-induced GLP-1 hormone secretion. J Biol Chem. (2013) 288:25088–97. doi: 10.1074/jbc.M113.452516
75. Christiansen CB, Gabe MBN, Svendsen B, Dragsted LO, Rosenkilde MM, Holst JJ. The impact of short-chain fatty acids on GLP-1 and PYY secretion from the isolated perfused rat colon. Am J Physiol Gastrointest Liver Physiol. (2018) 315:G53–65. doi: 10.1152/ajpgi.00346.2017
76. Zhou D, Chen Y-W, Zhao Z-H, Yang R-X, Xin F-Z, Liu X-L, et al. Sodium butyrate reduces high-fat diet-induced non-alcoholic steatohepatitis through upregulation of hepatic GLP-1R expression. Exp Mol Med. (2018) 50:1–12. doi: 10.1038/s12276-018-0183-1
77. Tomas A, Jones B, Leech C. New insights into beta-cell GLP-1 receptor and cAMP signaling. J Mol Biol. (2020) 432:1347–66. doi: 10.1016/j.jmb.2019.08.009
78. Meloni AR, DeYoung MB, Lowe C, Parkes DG. GLP-1 receptor activated insulin secretion from pancreatic β-cells: mechanism and glucose dependence. Diabetes Obes Metab. (2013) 15:15–27. doi: 10.1111/j.1463-1326.2012.01663.x
79. Shuai H, Xu Y, Ahooghalandari P, Tengholm A. Glucose-induced cAMP elevation in β-cells involves amplification of constitutive and glucagon-activated GLP-1 receptor signalling. Acta Physiol (Oxf). (2021) 231:e13611. doi: 10.1111/apha.13611
80. Li G, Tian Y, Zhu W-G. The roles of histone deacetylases and their inhibitors in cancer therapy. Front Cell Dev Biol. (2020) 8:576946. doi: 10.3389/fcell.2020.576946
81. Kim HJ, Leeds P, Chuang D-M. The HDAC inhibitor, sodium butyrate, stimulates neurogenesis in the ischemic brain. J Neurochem. (2009) 110:1226–40. doi: 10.1111/j.1471-4159.2009.06212.x
82. Makkar R, Behl T, Arora S. Role of HDAC inhibitors in diabetes mellitus. Curr Res Transl Med. (2020) 68:45–50. doi: 10.1016/j.retram.2019.08.001
83. Bridgeman SC, Ellison GC, Melton PE, Newsholme P, Mamotte CDS. Epigenetic effects of metformin: from molecular mechanisms to clinical implications. Diabetes Obes Metab. (2018) 20:1553–62. doi: 10.1111/dom.13262
84. Zhang Y, Bürck LW, Lei Y, Honarpisheh M, Kemter E, Wolf E, et al. Butyrate Enhances Differentiation and Maturation of Neonatal Porcine Islets by Inhibiting Class I Histone Deacetylase in Hand in Hand Zum Ziel – Einfach.Besser.Messbar. New York, NY: Georg Thieme Verlag KG (2022). doi: 10.1055/s-0042-1746353
85. Khosravi Z, Hadi A, Tutunchi H, Asghari-Jafarabadi M, Naeinie F, Roshanravan N, et al. The effects of butyrate supplementation on glycemic control, lipid profile, blood pressure, nitric oxide level and glutathione peroxidase activity in type 2 diabetic patients: a randomized triple -blind, placebo-controlled trial. Clin Nutr ESPEN. (2022) 49:79–85. doi: 10.1016/j.clnesp.2022.03.008
86. Roshanravan N, Mahdavi R, Alizadeh E, Jafarabadi MA, Hedayati M, Ghavami A, et al. Effect of butyrate and inulin supplementation on glycemic status, lipid profile and glucagon-like peptide 1 level in patients with type 2 diabetes: a randomized double-blind, placebo-controlled trial. Horm Metab Res. (2017) 49:886–91. doi: 10.1055/s-0043-119089
87. Hartstra AV, Schüppel V, Imangaliyev S, Schrantee A, Prodan A, Collard D, et al. Infusion of donor feces affects the gut-brain axis in humans with metabolic syndrome. Mol Metab. (2020) 42:101076. doi: 10.1016/j.molmet.2020.101076
88. de Groot PF, Nikolic T, Imangaliyev S, Bekkering S, Duinkerken G, Keij FM, et al. Oral butyrate does not affect innate immunity and islet autoimmunity in individuals with longstanding type 1 diabetes: a randomised controlled trial. Diabetologia. (2020) 63:597–610. doi: 10.1007/s00125-019-05073-8
89. Bouter K, Bakker GJ, Levin E, Hartstra AV, Kootte RS, Udayappan SD, et al. Differential metabolic effects of oral butyrate treatment in lean versus metabolic syndrome subjects. Clin Transl Gastroenterol. (2018) 9:155. doi: 10.1038/s41424-018-0025-4
90. Coppola S, Nocerino R, Paparo L, Di Scala C, Voto L, Luzzetti A, et al. Butyrate against paediatric obesity: results of the BAPO trial. Nutr Metab Cardiovasc Dis. (2021) 31:3249. doi: 10.1016/j.numecd.2021.08.003
91. Cleophas MCP, Ratter JM, Bekkering S, Quintin J, Schraa K, Stroes ES, et al. Effects of oral butyrate supplementation on inflammatory potential of circulating peripheral blood mononuclear cells in healthy and obese males. Sci Rep. (2019) 9:775. doi: 10.1038/s41598-018-37246-7
92. Tougaard NH, Frimodt-Møller M, Salmenkari H, Stougaard EB, Zawadzki AD, Mattila IM, et al. Effects of butyrate supplementation on inflammation and kidney parameters in type 1 diabetes: a randomized, double-blind, placebo-controlled trial. J Clin Med. (2022) 11:3573. doi: 10.3390/jcm11133573
93. Roshanravan N, Alamdari NM, Jafarabadi MA, Mohammadi A, Shabestari BR, Nasirzadeh N, et al. Effects of oral butyrate and inulin supplementation on inflammation-induced pyroptosis pathway in type 2 diabetes: a randomized, double-blind, placebo-controlled trial. Cytokine. (2020) 131:155101. doi: 10.1016/j.cyto.2020.155101
Keywords: butyrate, obesity, fiber, short-chain fatty acid (SCFA), diabetes
Citation: Mayorga-Ramos A, Barba-Ostria C, Simancas-Racines D and Guamán LP (2022) Protective role of butyrate in obesity and diabetes: New insights. Front. Nutr. 9:1067647. doi: 10.3389/fnut.2022.1067647
Received: 12 October 2022; Accepted: 07 November 2022;
Published: 24 November 2022.
Edited by:
Evelyn Frias-Toral, Catholic University of Santiago de Guayaquil, EcuadorReviewed by:
Beatriz Quintero, Universidad de Los Andes, VenezuelaCopyright © 2022 Mayorga-Ramos, Barba-Ostria, Simancas-Racines and Guamán. This is an open-access article distributed under the terms of the Creative Commons Attribution License (CC BY). The use, distribution or reproduction in other forums is permitted, provided the original author(s) and the copyright owner(s) are credited and that the original publication in this journal is cited, in accordance with accepted academic practice. No use, distribution or reproduction is permitted which does not comply with these terms.
*Correspondence: Linda P. Guamán, bGluZGEuZ3VhbWFuQHV0ZS5lZHUuZWM=
†These authors have contributed equally to this work
Disclaimer: All claims expressed in this article are solely those of the authors and do not necessarily represent those of their affiliated organizations, or those of the publisher, the editors and the reviewers. Any product that may be evaluated in this article or claim that may be made by its manufacturer is not guaranteed or endorsed by the publisher.
Research integrity at Frontiers
Learn more about the work of our research integrity team to safeguard the quality of each article we publish.