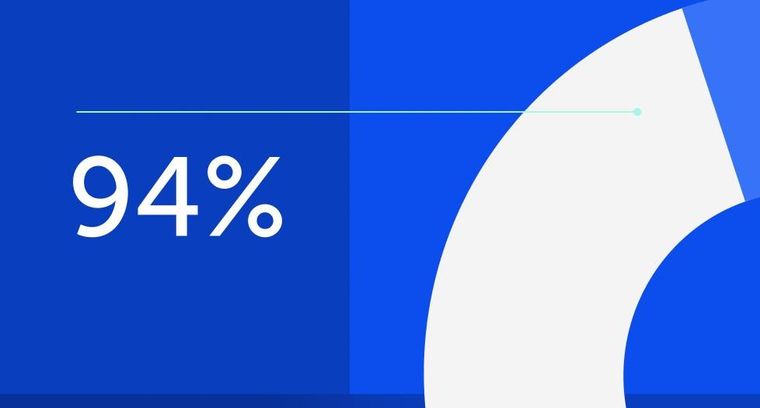
94% of researchers rate our articles as excellent or good
Learn more about the work of our research integrity team to safeguard the quality of each article we publish.
Find out more
REVIEW article
Front. Nutr., 22 December 2022
Sec. Nutrition and Food Science Technology
Volume 9 - 2022 | https://doi.org/10.3389/fnut.2022.1056445
This article is part of the Research TopicBiodigestibility, Antioxidant, and Bioactive Properties of Cereal, Legume, and Fruit Flour PolymersView all 3 articles
Finger millet (Eleusine coracana) is a staple food in several parts of the world because of its high nutritional value. In addition to its high nutrient content, finger millet contains numerous bioactive compounds, including polyphenol (10.2 mg/g TAE), flavonoid (5.54 mg/g CE), phytic acid (0.48%), and dietary fiber (15–20%). Polyphenols are known for their anti-oxidant and anti-diabetic role. Phytic acid, previously considered an anti-nutritive substance, is now regarded as a nutraceutical as it reduces carbohydrate digestibility and thus controls post-prandial glucose levels and obesity. Thus, finger millet is an attractive diet for patients with diabetes. Recent findings have revealed that the anti-oxidant activity and bio-accessibility of finger millet polyphenols increased significantly (P < 0.05) in the colon, confirming the role of the gut microbiota. The prebiotic content of finger millet was also utilized by the gut microbiota, such as Faecalibacterium, Eubacterium, and Roseburia, to generate colonic short-chain fatty acids (SCFAs), and probiotic Bifidobacterium and Lactobacillus, which are known to be anti-diabetic in nature. Notably, finger millet-induced mucus-degrading Akkermansia muciniphila can also help in alleviate diabetes by releasing propionate and Amuc_1100 protein. Various millet bio-actives effectively controlled pathogenic gut microbiota, such as Shigella and Clostridium histolyticum, to lower gut inflammation and, thus, the risk of diabetes in the host. In the current review, we have meticulously examined the role of gut microbiota in the bio-accessibility of millet compounds and their impact on diabetes.
Diabetes, one of the most common metabolic diseases, results in hyperglycemic conditions caused by insufficient insulin secretion and reduced insulin sensitivity. Diabetes can be divided into two types: type 1 and type 2. Type 1 diabetes is characterized by little or no insulin production owing to complete or partial autoimmune destruction of the insulin-producing pancreatic β-cells (1). However, Type 2 diabetes is more complex, with either insulin resistance developing or reduced sensitivity toward insulin levels, thus resulting in high glycemic conditions (2). Diabetes is a chronic metabolic disease associated with numerous complications, including obesity and lipid metabolism dysfunction, leading to higher triglyceride levels, cardiovascular diseases, and neuropathy owing to higher glucose levels (3, 4). According to WHO data, approximately 422 million people have diabetes, which is responsible for approximately 1.5 million deaths yearly (5).1
The etiology of diabetes includes a higher glycemic index, low-grade systemic inflammation, obesity, and higher oxidative stress, which further exacerbates the severity of diabetes. Various dietary strategies (probiotic, prebiotic, and synbiotic) (6, 7) and dietary substances rich in polyphenols efficiently controlled some of the adverse conditions of diabetes (8). Finger millet (Eleusine coracana) is a naturally gluten-free cereal grain rich in nutrients. It contains carbohydrates (65–75%), proteins (5–8%), dietary fiber (15–20%), minerals (2.5–3.5%), and Vitamin B complex (9, 10). Among all the cereals, finger millet contains the highest calcium content (337.53–353.41 mg/100 g), together with high phosphorus (314.46–341.70 mg/100 g), zinc (1.96–1.93 mg/100 g), and iron (3.28–3.86 mg/100 g) contents (11). According to the national health service-UK, the daily requirement of above mentioned micro nutrients are 700 mg/Day (Ca), 550 mg/day (phosphorus), 7–9.5 mg/day (Zn), and 8.7–14.8 mg/day (Fe) (12).2 Furthermore, finger millet is balanced in its protein content, as it is high in essential amino acids such as methionine, lysine, threonine, and valine, compared with that in other millets (13). Finger millet is also rich in dietary polyphenols (10.2 mg/g GAE), flavonoids (5.54 mg/g CE) (14), and tannins (0.04–3.47%) (15). Bioactive compounds of finger millet are anti-diabetic owing to their high fiber, flavonoid, and polyphenol contents, control hyperglycemic levels, and reduce oxidative stress by acting as an anti-oxidant. Finger millet grains are also rich in phytic acid (0.48%) and trypsin inhibitory factors, adversely affecting the availability of carbohydrates and proteins in the intestine, thereby lowering the glycemic level (9). Gut microbiota increased the catabolism of dietary polyphenols in the gut, and various simple bioactive derivatives were produced, improving bioavailability (16). Additionally, the gut microbiota utilizes dietary fibers to produce short-chain fatty acids (SCFAs; acetic acid, propionic acid, and butyric acid) that modulate insulin sensitivity, low-grade systemic inflammation, and glucose-lipid metabolism (17). Moreover, studies confirmed the synergistic roles of butyric acid and phenolic acids (benzoic acid, phenylacetic acid, and phenylpropionic acid) resulted in strong anti-inflammatory effects (18).
Finger millet, a rich source of phenolic acids and prebiotic dietary fibers, can be readily utilized by the gut microbiota to generate SCFAs, specifically butyrate (19). Numerous other finger millet bio-actives also modulated the gut communities by enhancing certain gut microbes and inhibiting others, possibly inducing an anti-diabetic effect (20). Therefore, the present review sought to understand the health benefits of finger millets and their role in gut microbiota, specifically against diabetes (Figure 1).
Figure 1. Role of finger millet bio-active molecule induced gut microbes in alleviation of diabetes; butyrate producers Faecalibacterium, Roseburia, and Eubacterium play a crucial role in reducing systemic inflammation by maintaining the gut barrier function to avoid the gut invasion by pathogenic microbes and toxins. Mucus degrading Akkermansia, participates in glucose-homeostasis by means of Amuc_1100 protein and regulates the blood glucose and lowers the gut inflammatory load by releasing propionate. Probiotics (Bifidobacterium and Lactobacillus) also improves the toxin level by modulating the gut community, i.e., by lowering the various possible opportunistic pathogen, and simultaneously enhancing the beneficial microbes.
Finger millet is a staple diet in various countries, including India, Nepal, Sri Lanka, and parts of Africa. However, consumption of finger millet is increasing in other parts of the world, including the Middle East and Europe, owing to public health awareness. In addition to their high nutritional content, finger millet grains are rich in dietary bioactive compounds such as polyphenols and dietary fibers (21). These molecules are further metabolized in the colon by the gut microbiota into bioactive molecules such as SCFAs and small-chain phenolic acids (21).
Dietary fibers are indigestible oligosaccharides or polysaccharides that cannot be digested by the human digestive system and act as roughage maintaining stool movement and cleaning the digestive tract. By nature, dietary fibers can also be prebiotic if they are metabolized by the gut microbiota, such as Faecalibacterium, Roseburia, Anaerostipes, and Ruminococcus, members of the family Lachnospiraceae, and members of the Clostridium IV cluster, in the colon to produce different SCFA including acetic acid, propionic acid, and butyric acid (22). In colonic fermentation, gut-microbiota hydrolyzes non-digestible dietary fiber into constituent monosaccharides through an exclusive set of enzymes, that are not present in humans. These enzymes belong to the carbohydrate-active enzyme (CAZyme), which hydrolyzes the complex fiber into respective monosaccharides (23). These hydrolyzed monosaccharides were further utilized by the microbes to generate different SCFAs via different pathways (Figure 1). For instance, gut microbes can produce acetate from the pyruvate via acetyl-CoA, propionic acid from succinate pathway, acrylate pathway, and propanediol pathway (24). While butyric acid is produced by condensing the two molecules of acetyl-CoA with simultaneous reduction to butyryl-CoA, which will be converted into butyric acid by microbial phosphotransbutyrylase, and butyrate kinase (24). Normally, the gut SCFAs (acetic acid, propionic acid, and butyric acid) are in a molar ratio of 60:20:20 (25). SCFA’s also reduces gut permeability to lower the toxin flow-through from the gut lumen to colonic tissues and liver (26), as they enhance the tight junction protein such as claudin-2, claudin-7, zonula occludens-1 (ZO-1), and occludin (27, 28).
In the gut, only a small fraction of SCFAs is metabolized by the microbes, which distinctly affects the gut microbiome, and majority of SCFAs are passively diffused to colonocyte cells via monocarboxylate transporter. Colonocyte cells are the intestinal enterocyte which utilizes SCFAs, specifically butyric acid to generate energy and stabilizes hypoxia-inducible factor (HIF) to maintain the anaerobic condition in gut, and the remaining SCFAs are transported to the portal system. In the liver also, butyric acid is utilized by the hepatocyte cells to produce energy, and the remaining SCFAs were transported to peripheral tissues. Other than that, SCFAs also act as signaling molecules and activate G-protein coupled receptors (GPR41 and GPR43) in colonocytes and adipocytes. Upon activation, GPR41 and GPR43 stimulate the release of glucagon-like peptide-1 (GLP-1) and peptide yy (PYY) hormones from the colonocyte, which induces satiety, stimulates insulin secretion, and decreases glucose level by decreasing glucagon secretion from the pancreas which generates the glucose from glycogen, to alleviate diabetes. In adipocytes, SCFA activated GPR41 and GPR43 induce higher levels of leptin hormone that also regulates glucose-energy homeostasis by increasing triglyceride hydrolysis and oxidation of free fatty acids in adipose tissue, thus promoting adipogenesis and inhibiting low-grade inflammation (29, 30). In addition, leptin also inhibits hunger to control the excess food intake and thus postprandial blood sugar level (31).
Other than that, SCFA-activated GPRs (GPR41, GPR43, and GPR109) also limit colonic inflammation, as butyric acid inhibits lipopolysaccharide (LPS) induced NF-kB activation through GPR109 (32). Additionally, butyric acid downregulates the LPS-induced pro-inflammatory cytokines such as IL-6, IL-12 (33), and IL-8 by influencing the macrophages and neutrophils, respectively (34). Moreover, butyric acid also helps in mucosal healing by stimulating the migration of epithelial cells (35). Thus, SCFAs maintain gut homeostasis by alleviating gut inflammation, limiting the prevalence of pathogenic microbes, and controlling gut permeability. They also participate in metabolic reactions, particularly butyric acid, which is utilized by the gut colonocyte cells to render the gut environment strictly anaerobic and thus limit the abundance and growth of possible pathogenic microbes such as Escherichia coli and Salmonella (36). As mentioned before, SCFAs can also regulate the host-energy metabolism and thus diet-induced obesity (30), which is a major risk factor for diabetes (37). Previous studies have demonstrated that SCFAs are also effective in alleviating chronic inflammation.
In finger millet, the main constituent of the carbohydrates is starch (65–75%), and the non-starchy polysaccharides, arabinoxylan, and β-glucan constitute the dietary fiber (15–20%) (38). According to their solubility in water, dietary fiber can be divided into soluble and insoluble categories. The soluble and insoluble dietary fiber fractions vary in finger millet. For example, Chethan and Malleshi (39) reported that the dietary fiber in finger millet was 15.7% insoluble and 1.4% soluble, whereas Shobana and Malleshi (40) reported 19.7% insoluble and 2.5% soluble dietary fiber in finger millet. The formation of resistant starch, another form of soluble fiber, which is a product of partial/least hydrolysis of starch during gastric digestion (41), also contributed to the overall dietary fiber content of finger millet (40). Soluble dietary fiber exhibited gel-forming properties by absorbing water and thus increasing food-viscosity that inhibited macronutrient absorption, reduced dyslipidemia, reduced the postprandial glucose response, and could be metabolized by the gut microflora (Bacteroides and Bifidobacterium) during colonic fermentation to generate SCFAs (42). Soluble dietary fibers decrease the absorption of bile acid in the small intestine (ileum) and increase the loss of bile acid, resulting in increased hepatic bile synthesis using intracellular cholesterol, which lowers the serum cholesterol level (43). However, insoluble dietary fibers, including cellulose and hemicellulose, were more prone to colonic fermentation by the gut microbiota and affects insulin sensitivity positively (44). In human gut, cellulose and hemicellulose are specifically metabolized by the member of the genus Ruminococcus, Prevotella, and Clostridium (45, 46). Insoluble fibers also aid in removing toxins from the intestinal tract through several mechanisms (47). They increase intestinal transit and enhance fecal bulk by increasing its water-holding capacity (48). However, prebiotic-resistant starch, that escapes enzymatic digestion, can be utilized by the gut microbiota to enhance the proportion of beneficial microbes such as probiotic Bifidobacterium, Lactobacillus, and Akkermansia (49). Studies have reported that millet-based preparations with a high dietary fiber content lower the glycemic responses and are therefore recommended for diabetic individuals (50). Several mechanisms have been proposed to promote the beneficial metabolic effects of finger millet dietary fiber, including altered lipid metabolism, altered bile acid metabolism, and improvement in glucose levels (9). In addition, being high in microbiota-accessible dietary fiber, the gut microbiota is considered to be involved in the anti-diabetic and anti-obesity effects of finger millet.
Only 5–10% of polyphenols are absorbed in the small intestine, with the remaining polyphenols (approximately 90%) reaching the large intestine (51). In the colon, accumulated polyphenols are actively metabolized by the gut microbiota (Akkermansia, Lactobacillus sp., and Eubacterium sp.) into smaller phenolic metabolites such as urolithins from ellagic acid (52), and protocatechuic acid from quercetin (53), which has health-benefiting effects. The gut microbes hydrolyze the polyphenols using (poly)phenol-associated enzymes (PAZymes) (54), cleaving glycosidic bonds and breaking complex heterocyclic compounds into smaller ones that are more active than the parent compound (55). In addition to the smaller phenolic compounds, polyphenols are prebiotic, and produce SCFA upon metabolization by the gut microbiota such as Faecalibacterium, Eubacterium, Roseburia, and members of the family Ruminococcaceae (51, 56). Studies have confirmed that different polyphenols such as catechin, ellagic acid, and ferulic acid affected the Bacteroides/Firmicutes ratio and altered the gut communities differently (57). For example, catechin (150 mg/L) significantly inhibited (P < 0.05) the pathogenic Clostridium histolyticum, while encouraging the growth of the probiotic, Bifidobacterium, and the microbes of the butyrate-producing bacteria, Clostridium coccoides-Eubacterium rectale group (58). Further, upon diffusion through colonic epithelial, they reach the liver via the portal system and can downregulate the liver genes associated with obesity and lipid metabolism (51). In particular, expression of sterol regulatory element binding protein 1 (SREBP1), fatty acid synthase (FAS), and stearyl CoA desaturase 1 is downregulated in the liver due to dietary polyphenols (55). In addition, ferulic acid; an abundant polyphenol in millets, also improves glucose and lipid homeostasis by inhibiting expression of the protein associated with gluconeogenesis such as phosphoenolpyruvate carboxylase (PEPCK) and glucose-6-phosphatase (G6P), to lower blood glucose (59).
Finger millet is a rich source of polyphenol (3.05–10.20 mg/g GAE; GAE, gallic acid equivalent) (14, 60) and flavonoids (2–5.54 mg/g CE; CE, catechin equivalent) (14, 61). However, different polyphenol levels have been reported in different finger millet varieties (15). Even in finger millet grain, the distribution of polyphenols was not uniform and was reported to be the highest in the seed coat, which also varied among different varieties (0.3 ± 0.1–2.3 ± 0.2% g) (39). The polyphenols present in finger millet are listed in Table 1. In finger millet grain, phenolic compounds are present in free, soluble-conjugated, and insoluble forms. Among these, ferulic acid, caffeic acid, and p-coumaric acid were the major insoluble bound polyphenols, whereas protocatechuic acid, gallic acid, and caffeic acid were the major free polyphenols of finger millet (62). Polyphenols (250 mg/day in diet) are crucial in controlling diabetes as they effectively reduce insulin resistance (63, 64) and regulate glucose and lipid metabolism (65). Similarly, finger millet polyphenols helped maintain the lipid metabolism as ferulic acid, a major polyphenol in finger millet (35.19–67.36 mg/100 g of dry weight), decreased the total cholesterol level (9). Gallic acid exhibited various biological properties, such as antioxidant (50–200 μM) (66), anti-inflammatory (25–100 μM) (67), and anti-microbial properties (2–8 mg/ml) (68). Catechin-rich (582.8 mg/340 ml) beverages reportedly improve obesity and blood glucose levels (69). This was achieved by enhancing the expression of adiponectin, the anti-diabetic protein, which increases insulin sensitivity and adipocyte differentiation, thereby lowering plasma glucose and free fatty acid levels (70). In an induced high-fat diet murine study, the anti-obesity effect of ferulic acid (50 mg/kg of body weight) was also confirmed (71). Finger millet polyphenols can also be metabolized by gut microbes (72) and could thus participate in the anti-diabetic effects of finger millet, as will be discussed later.
Table 1. Various polyphenolic compounds present in finger millet extract (n-hexane) (123).
The portion of dietary fiber and polyphenols released after gastrointestinal digestion (Table 2) or by the action of the gut microbiome is bio-accessible and metabolically bioactive (14). Generally, the gastrointestinal release of bioactive molecules, such as phenolic acid, from digested finger millet is low owing to the formation of complexes with the dietary fiber (arabinoxylan) (9). Furthermore, the presence of anti-nutritional factors in finger millet, such as phytic acid and tannin, hinders the availability of nutrients and decreases nutritional availability and the digestibility of starch and protein (73). In the body, enzymatic digestion of easily digestible starch and protein results in a sudden increase in blood glucose, however, owing to the presence of anti-nutritional factors, finger millet flour has a lower glycemic index (42.24) than wheat flour (62.59), proso millet flour (52.49), foxtail millet flour (49.28), and barnyard millet flour (47.95) (74), thereby indicating its nutraceutical potential (75). Although finger millet contains 22 polyphenols, only 13 are bio-accessible (14). The bio-accessible content of finger millet polyphenol and flavonoid is around 2.65 and 1.09 mg/g, respectively, which does not change with roasting (14). However, gut microbiota can enhance the availability of high molecular weight polyphenolic compounds by degrading them into simpler forms (72). In addition, studies reported that polyphenolic and antioxidant levels of finger millet increase significantly (P < 0.05) after digestion and increases after fermentation by gut microbes in the colon (76). Another study confirmed a significant increase (P < 0.05) in polyphenolic levels and anti-oxidant activity of finger millet flour upon fermentation (38). Colonic fermentation of finger millet polyphenols may favor certain microbes with antidiabetic properties to lower the risk of diabetes.
Table 2. Effect of various processing methods on bio-accessible content of finger millet polyphenols and flavonoids.
Microbial-dysbiosis in the gut of patients with diabetes and the role of the gut microbiota in regulating various diabetes-associated metabolisms, such as glucose homeostasis, oxidative stress, and T2D-associated pro-inflammatory cytokines (IL-1 and IL-6), is well documented (2). Studies have demonstrated that gut dysbiosis in diabetic patients can be alleviated by certain diets or dietary interventions such as prebiotics (77), polyphenols (78), flavonoids (79), and glucosinolates (80, 81). Fecal microbiome transplantation (FMT) therapy also effectively controls the etiology of diabetes, such as insulin resistance and inflammation, providing evidence of the significance of the gut microbiota in diabetes (82, 83). As discussed previously, finger millet is explicitly enriched in microbiota-accessible compounds (prebiotics and polyphenols) that are metabolized by the gut microbiota and benefit the health of the host. Prebiotics and polyphenols reportedly ameliorate diabetes and its associated co-morbidities, such as obesity, low-grade inflammation, and blood glucose level regulation (2). Recent studies on finger millet have demonstrated its importance in diabetes and its ability to modulate the gut microbial community (Table 3). Along with dietary fibers and polyphenols, finger millet is also rich in antioxidants and metabolically active compounds (vitamins E, A, and B), which also play a significant role in lowering oxidative stress and maintaining a healthy gut microbiome (84, 85).
Table 3. Studies showing anti-diabetic significance of finger millet along with its effect on gut microbiota.
Obesity develops as a result of excessive fat accumulation in the body and is directly associated with various metabolic disorders, including diabetes and associated comorbidities. Obesity or excessive fat deposition results in dyslipidemia, a disturbed lipid metabolism inducing excessive triglycerides and free fatty acids, which are then accumulated in non-fatty tissues and cause insulin resistance (2). Normally, obesity is defined by a body mass index (BMI) of ≥30 and is associated with a higher level of the phylum Firmicutes or a higher Firmicutes: Bacteroides ratio (86, 87). Furthermore, the dysbiotic gut of obese people was reported to be high in members of the Prevotellaceae and Enterobacteriaceae families and abundant in the endotoxin-producing genus Enterobacter (88). This can lead to gut inflammation and diabetes. Finger millet can be administered to normalize gut dysbiosis as it is a rich source of various indigestible dietary fibers such as arabinoxylan, β-glucan, lignin, cellulose, and resistant starch, that are metabolized by various beneficial gut microbes (Table 4) (9). In particular, non-starchy arabinoxylan fiber in finger millet is determined to be effective in controlling high-fat diet-induced dyslipidemia, hepatic lipid accumulation, and glucose tolerance while enhancing the levels of beneficial gut microbes such as Lactobacillus, Bifidobacterium, and Roseburia (20). Similarly, the β-glucan fiber obtained from finger millet effectively controls the post-prandial glucose levels by inhibiting α-amylase and glucosidase (89). Furthermore, β-glucan decreases cholesterol and triglyceride levels and is also metabolized by gut microbiota to produce SCFAs (90).
Table 4. Known anti-diabetic and gut-microbiota modulatory effects of different dietary fibers in finger millet.
As discussed previously, finger millet is rich in polyphenols (3.05–10.20 mg/g GAE) (14, 60) and flavonoids (2–5.54 mg/g CE) (14, 61). Finger millet polyphenols helped maintain lipid metabolism as ferulic acid, the dominant polyphenol in finger millet (35–67 mg/100 g of dry weight) (61) and more prominent in the insoluble fraction of grain (91) resulting in decreased total cholesterol levels (9). In addition, ferulic acid modulated the gut microbiome to improve lipid metabolism and alleviate diabetes (78, 92). In particular, ferulic acid increases the abundance of the mucolytic Akkermansia (78), which has anti-obesity and anti-diabetic effects. Akkermansia actively participates in glucose homeostasis in the host by releasing Amuc_1100 protein and propionate (2). The Amuc_1100 protein is immunoregulatory and promotes the secretion of serotonin, a glucose-homeostasis neurotransmitter (93). While propionate suppresses blood glucose levels by suppressing gluconeogenesis in the liver increasing the phosphorylation of AMP-activated protein kinase (AMPK) and by enhancing GLP-1 secretion in the gut, which lowers the hepatic visceral fat by reducing triglyceride synthesis (2). Similarly, tannin is also metabolized by the gut microbiota and enhances Akkermansia and SCFA-producing microbes of the Lachnospiraceae and Ruminococcaceae families (92). Caffeic acid, another polyphenolic compound in finger millet (14), regulates obesity and fat deposition in the host through gut microbes (94). Administration of caffeic acid restores gut dysbiosis in an obese host and enhances butyrate-producing microbial communities in the gut (94). Similarly, catechin, a flavonoid present in finger millet, is actively metabolized by butyrate-producing Eubacterium, probiotic Bifidobacterium longum, and Lactobacillus plantarum, that improve insulin sensitivity (95), reduces fat accumulation (96), and lowers the blood glucose levels (97), respectively. In addition, finger millet contains phytic acid, a compound of nutraceutical importance, which suppresses hepatic lipogenic gene expression and triglyceride levels, alleviating obesity and associated metabolic disorders (98). In addition, phytic acid also shifts the gut community and enhances the Bifidobacterium and Lactobacillus (98), which are negatively associated with obesity, and thus their administration can ameliorate obesity.
Low-grade or systemic inflammation is associated with insulin resistance and diabetes. This type of inflammation increases the levels of several immunomodulatory molecules, such as IL-1, IL-6, and TNF-α, inducing insulin resistance and regulating C-reactive protein (CRP) (99). CRP is a diabetes marker, and its combination with obesity is positively associated with a higher risk of diabetes (100). Alternatively, the term “oxidative stress” indicates the damaging impact of reactive oxygen species (ROS) and reactive nitrogen species (47) free radicals. Oxidative stress increases glucose overload and advanced glycation products (19, 101). AGEs generate additional ROS and activate the inflammatory cascades, which, along with glucose overload, increases insulin resistance (102).
Gut-dysbiosis also induces systemic inflammation and the risk of diabetes, as a dysbiotic gut permits pathogenic strains and microbial toxins (lipopolysaccharides and enterotoxins) to invade the gut epithelium (2). Bioactive molecules obtained from finger millet are actively metabolized by gut microbes to reduce dysbiosis, and generating metabolites of anti-diabetic significance. For example, catechin, metabolized by the probiotic L. plantarum, which is known to reduced low-grade inflammation in diabetes (97). Similarly, gallic acid, another major polyphenol in finger millet, has antioxidant and anti-inflammatory properties (68), which can be metabolized by gut microbes such as L. plantarum (103), thereby reducing gut inflammation. Caffeic acid also improves colonic inflammation (colitis) and lowers pro-inflammatory cytokines levels (IL-6, TNF-α, and IFN-γ) through Akkermansia (104). Akkermansia is a mucolytic microbe enhanced by dietary polyphenol treatment and attenuates obesity-induced metabolic syndromes (105). In addition, arabinoxylan fiber and catechin increases the proportion of Bifidobacterium in the host gut and improved high-fat diet-induced diabetes by modulating endotoxemia (106). Endotoxemia is a condition whereby microbial toxins (lipopolysaccharides and enterotoxins) cross the gut epithelial barrier into the bloodstream, causing severe inflammation. Bifidobacterium reduces the synthesis of such microbial toxins by regulating gut microbial communities and improving the gut barrier function to prevent the leakage of toxins (106). Furthermore, ferulic acid increases levels of butyrogenic Faecalibacterium (78). Faecalibacterium is the most abundant butyrate producer and is known for its anti-inflammatory role in the gut, whereby butyrate inhibits NF-κB activation and IL-8 production (107). Furthermore, butyrate produced by Faecalibacterium also maintain the integrity of the gut barrier, preventing endotoxemia (108), thereby lowering the risk of diabetes.
Selective anti-microbial activity of dietary components maintains a healthy gut environment by limiting the possible pathogens, which lowers the toxin load in the gut, thus with the lower possibility of low-grade inflammation in the gut, which increases insulin resistance and is directly associated with diabetes progression (2, 109). Finger millet modulates microbial communities through the antimicrobial activities of its bioactive molecules, such as tannin and β-glucan. Tannin, a water-soluble polyphenol, reduces the risk of diabetes by inhibiting Staphylococcus aureus (110) which causes gastrointestinal infections and releases enterotoxins that compromise gut permeability (111, 112). However, through anti-biofilm activity, β-glucan inhibits the abundance of pathogenic Enterococcus faecalis, Shigella sonnei, Proteus vulgaris, and Lysinibacillus fusiformis (89). E. faecalis is an opportunistic pathogen associated with several inflammatory diseases that suppresses host immunity (113, 114). Similarly, S. sonnei also suppress host immunity in the gut (115, 116), by producing the cytotoxic Shiga toxin-1, causes severe gastrointestinal inflammation (115, 117). L. fusiformis and P. vulgaris have been reported to cause sepsis, which is a life-threatening hyperreaction to an infection (118) and urinary inflammation (119), respectively. Polyphenolic catechin also inhibits the growth of pathogenic C. histolyticum (58). C. histolyticum releases cytotoxic toxins, causing apoptosis (120, 121), which could weaken the gut barrier, causing endotoxemia and possibly diabetes.
Millets are well known for their health benefits, specially against the obesity and diabetes, and were studied extensively in this regard, but only recently we started recognizing the relevance of gut-microbes in said metabolic conditions. Various millets, except finger millet, are also well-studied for their anti-diabetic role but further studies are still needed to elucidate the exact functional role of gut microbes in this regard. Most of the millets are rich in dietary fiber and polyphenols, yet there is a difference in the level and type of bioactive compound present in an individual millet type (122), which might lead to a slightly different microbial community than others. Although, the association of gut microbes in a millet’s anti-diabetic role is certain, owing to various microbial metabolites produced via microbial fermentation of dietary fiber and polyphenols (21). Still, based on the precise data (bio accessibility of and concentration of polyphenols, presence of beta-glucan), this study is focused on the anti-diabetic role of finger millet-induced gut-microbiota. However, other millets are also supposed to have a similar effect with varying degrees of efficacy and microbial markers. Even for finger millet, to date, very few studies have examined the relationship between finger millet and gut microbes, and a lot of things need to be explored regarding millet-induced gut microbiota and their anti-diabetic effect.
Finger millet is an excellent source of different micronutrients and is rich in complex dietary fibers, which helps to lower the glycemic level and dyslipidemia. Only recently we started recognizing relevance of gut microbes in said metabolic conditions. Therefore, presently much scientific literature is not available to elucidate the multiple aspects of the millet-induced host-microbiome interaction, and a lot of work still needs to be done to understand it. Although, gut-microbes certainly play a critical role in the anti-diabetic effects of finger millet owing to its rich prebiotic content (dietary fiber and polyphenol), which are metabolized by the gut microbiota, to produce various bioactive molecules (SCFAs and phenol) of anti-diabetic significance. In addition, finger millet contains several bioactive molecules that directly affect the beneficial microbial communities in the gut (Faecalibacterium, Lactobacillus, Eubacterium, Roseburia, and Akkermansia) and thus alleviate systemic inflammation and insulin resistance. However, these bioactive molecules also inhibit various opportunistic pathogens, which limit inflammation and toxin levels in the gut, and further reduce the risk of diabetes in the host. This accumulated evidence suggests that the gut microbiota plays a crucial role in the anti-diabetic effect of finger millet consumption in the host.
VS conceptualized the manuscript. VS, GL, and MB participated in writing and the illustrations. HS and SA participated in data collection and project management. MB and J-HS supervised, reviewed, and finalized the final draft. All authors contributed to the article and approved the submitted version.
This research was supported by the Korea Basic Science Institute National Research Facilities and Equipment Center grant funded by the Ministry of Education (2021R1A6C101A416), the project to train professional personnel in biological materials by the Ministry of Environment, and the “Leaders in Industry-University Cooperation 3.0” project, supported by the Ministry of Education and National Research Foundation of Korea.
We would like to thank the KNU NGS core facility for providing the server for data collection and additional analysis. Figure was prepared using BioRender (https://biorender.com/).
The authors declare that the research was conducted in the absence of any commercial or financial relationships that could be construed as a potential conflict of interest.
All claims expressed in this article are solely those of the authors and do not necessarily represent those of their affiliated organizations, or those of the publisher, the editors and the reviewers. Any product that may be evaluated in this article, or claim that may be made by its manufacturer, is not guaranteed or endorsed by the publisher.
2. Singh V, Park Y, Lee G, Unno T, Shin J. Dietary regulations for microbiota dysbiosis among post-menopausal women with type 2 diabetes. Crit Rev Food Sci Nutr. (2022):1–16. doi: 10.1080/10408398.2022.2076651
3. Sharma A, Mittal S, Aggarwal R, Chauhan M. Diabetes and cardiovascular disease: inter-relation of risk factors and treatment. Future J Pharm Sci. (2020) 6:130.
4. Yagihashi S, Mizukami H, Sugimoto K. Mechanism of diabetic neuropathy: where are we now and where to go? J Diabetes Invest. (2011) 2:18–32. doi: 10.1111/j.2040-1124.2010.00070.x
6. Kim YA, Keogh JB, Clifton PM. Probiotics, prebiotics, synbiotics and insulin sensitivity. Nutr Res Rev. (2018) 31:35–51.
7. Bock PM, Telo G, Ramalho R, Sbaraini M, Leivas G, Martins A, et al. The effect of probiotics, prebiotics or synbiotics on metabolic outcomes in individuals with diabetes: a systematic review and meta-analysis. Diabetologia. (2021) 64:26–41.
8. Sun C, Zhao C, Guven E, Paoli P, Simal-Gandara J, Ramkumar K, et al. Dietary polyphenols as antidiabetic agents: advances and opportunities. Food Front. (2020) 1:18–44.
9. Devi PB, Vijayabharathi R, Sathyabama S, Malleshi NG, Priyadarisini VB. Health benefits of finger millet (Eleusine coracana L.) polyphenols and dietary fiber: a review. J Food Sci Technol. (2014) 51:1021–40. doi: 10.1007/s13197-011-0584-9
10. Taylor JRN, Kruger J. Millets. In: Caballero B, Finglas PM, Toldrá F editors. Encyclopedia of Food and Health. Oxford: Academic Press (2016). p. 748–57.
11. Jayawardana SAS, Radhika Samarasekera J, Hettiarachchi G, Gooneratne J, Mazumdar S, Banerjee R. Dietary fibers, starch fractions and nutritional composition of finger millet varieties cultivated in Sri Lanka. J Food Composit Analy. (2019) 82:103249.
12. NHS. Overview Vitamins and Minerals. (2020). Available online at: https://www.nhs.uk/conditions/vitamins-and-minerals/ (accessed November 15, 2022).
13. Sripriya G, Antony U, Chandra TS. Changes in carbohydrate, free amino acids, organic acids, phytate and HCl extractability of minerals during germination and fermentation of finger millet (Eleusine coracana). Food Chem. (1997) 58:345–50.
14. Hithamani G, Srinivasan K. Effect of domestic processing on the polyphenol content and bioaccessibility in finger millet (Eleusine coracana) and pearl millet (Pennisetum glaucum). Food Chem. (2014) 164:55–62. doi: 10.1016/j.foodchem.2014.04.107
15. Panwar P, Dubey A, Verma A. Evaluation of nutraceutical and antinutritional properties in barnyard and finger millet varieties grown in Himalayan region. J Food Sci Technol. (2016) 53:2779–87. doi: 10.1007/s13197-016-2250-8
16. Selma MV, Espín JC, Tomás-Barberán FA., Interaction between phenolics and gut microbiota: role in human health. J Agric Food Chem. (2009) 57:6485–501.
17. Lau WL, Vaziri ND. Gut microbial short-chain fatty acids and the risk of diabetes. Nat Rev Nephrol. (2019) 15:389–90.
18. Zheng S, Zhang H, Liu R, Huang C, Li H, Deng Z, et al. Do short chain fatty acids and phenolic metabolites of the gut have synergistic anti-inflammatory effects? – New insights from a TNF-α-induced Caco-2 cell model. Food Res Int. (2021) 139:109833. doi: 10.1016/j.foodres.2020.109833
19. Murtaza N, Baboota RK, Jagtap S, Singh DP, Khare P, Sarma SM, et al. Finger millet bran supplementation alleviates obesity-induced oxidative stress, inflammation and gut microbial derangements in high-fat diet-fed mice. Br J Nutr. (2014) 112:1447–58. doi: 10.1017/S0007114514002396
20. Sarma SM, Singh DP, Singh P, Khare P, Mangal P, Singh S, et al. Finger millet arabinoxylan protects mice from high-fat diet induced lipid derangements, inflammation, endotoxemia and gut bacterial dysbiosis. Int J Biol Macromol. (2018) 106:994–1003. doi: 10.1016/j.ijbiomac.2017.08.100
21. Samtiya M, Aluko R, Dhaka N, Dhewa T, Puniya A. Nutritional and health-promoting attributes of millet: current and future perspectives. Nutr Rev. (2022):nuac081. doi: 10.1093/nutrit/nuac081
22. Singh V, Hwang N, Ko G, Tatsuya U. Effects of digested Cheonggukjang on human microbiota assessed by in vitro fecal fermentation. J Microbiol. (2021) 59:217–27. doi: 10.1007/s12275-021-0525-x
23. Wardman JF, Bains RK, Rahfeld P, Withers SG. Carbohydrate-active enzymes (CAZymes) in the gut microbiome. Nat Rev Microbiol. (2022) 20:542–56.
24. Alexander C, Swanson KS, Fahey GC, Garleb KA. Perspective: physiologic importance of short-chain fatty acids from nondigestible carbohydrate fermentation. Adv Nutr. (2019) 10:576–89. doi: 10.1093/advances/nmz004
25. Besten G d, Eunen K v, Groen AK, Venema K, Reijngoud D, Bakker BM. The role of short-chain fatty acids in the interplay between diet, gut microbiota, and host energy metabolism. J Lipid Res. (2013) 54:2325–40.
26. Pohl K, Moodley P, Dhanda A. The effect of increasing intestinal short-chain fatty acid concentration on gut permeability and liver injury in the context of liver disease: a systematic review. J Gastroenterol Hepatol. (2022) 37:1498–506. doi: 10.1111/jgh.15899
27. Everard A, Cani PD. Diabetes, obesity and gut microbiota. Best Pract Res Clin Gastroenterol. (2013) 27:73–83.
28. Parada Venegas D, De la Fuente M, Landskron G, González M, Quera R, Dijkstra G, et al. Short chain fatty acids (SCFAs)-mediated gut epithelial and immune regulation and its relevance for inflammatory bowel diseases. Front Immunol. (2019) 10:277. doi: 10.3389/fimmu.2019.00277
29. Xiong Y, Miyamoto N, Shibata K, Valasek MA, Motoike T, Kedzierski RM, et al. Short-chain fatty acids stimulate leptin production in adipocytes through the G protein-coupled receptor GPR41. Proc Natl Acad Sci USA. (2004) 101:1045–50. doi: 10.1073/pnas.2637002100
30. Lu Y, Fan C, Li P, Lu Y, Chang X, Qi K. Short chain fatty acids prevent high-fat-diet-induced obesity in mice by regulating g protein-coupled receptors and gut microbiota. Sci Rep. (2016) 6:37589. doi: 10.1038/srep37589
31. Obradovic M, Sudar-Milovanovic E, Soskic S, Essack M, Arya S, Stewart AJ, et al. Leptin and obesity: role and clinical implication. Front Endocrinol. (2021) 12:585887. doi: 10.3389/fendo.2021.585887
32. Thangaraju M, Cresci GA, Liu K, Ananth S, Gnanaprakasam JP, Browning DD, et al. GPR109A is a G-protein–coupled receptor for the bacterial fermentation product butyrate and functions as a tumor suppressor in colon. Cancer Res. (2009) 69:2826–32. doi: 10.1158/0008-5472.CAN-08-4466
33. Chang PV, Hao L, Offermanns S, Medzhitov R. The microbial metabolite butyrate regulates intestinal macrophage function via histone deacetylase inhibition. Proc Natl Acad Sci USA. (2014) 111:2247–52. doi: 10.1073/pnas.1322269111
34. Li G, Lin J, Zhang C, Gao H, Lu H, Gao X, et al. Microbiota metabolite butyrate constrains neutrophil functions and ameliorates mucosal inflammation in inflammatory bowel disease. Gut Microbes. (2021) 13:1968257. doi: 10.1080/19490976.2021.1968257
35. Vliet MJ, Harmsen HJ, Bont ES, Tissing WJ. The role of intestinal microbiota in the development and severity of chemotherapy-induced mucositis. PLoS Pathog. (2010) 6:e1000879. doi: 10.1371/journal.ppat.1000879
36. Singer-Englar T, Barlow G, Mathur R. Obesity, diabetes, and the gut microbiome: an updated review. Exp Rev Gastroenterol Hepatol. (2019) 13:3–15.
37. Jia R, Huang M, Qian L, Yan X, Lv Q, Ye H, et al. The depletion of carbohydrate metabolic genes in the gut microbiome contributes to the transition from central obesity to type 2 diabetes. Front Endocrinol. (2021) 12:747646. doi: 10.3389/fendo.2021.747646
38. Mutshinyani M, Mashau ME, Jideani AIO. Bioactive compounds, antioxidant activity and consumer acceptability of porridges of finger millet (Eleusine coracana) flours: effects of spontaneous fermentation. Int J Food Propert. (2020) 23:1692–710.
39. Chethan S, Malleshi N. Finger millet polyphenols: optimization of extraction and the effect of pH on their stability. Food Chem. (2007) 105:862–70.
40. Shobana S, Malleshi N. Preparation and functional properties of decorticated finger millet (Eleusine coracana). J Food Eng. (2007) 79:529–38.
41. Kaimal AM, Mujumdar AS, Thorat BN. Resistant starch from millets: recent developments and applications in food industries. Trends Food Sci Technol. (2021) 111:563–80.
42. Weickert MO, Pfeiffer AFH. Metabolic effects of dietary fiber consumption and prevention of diabetes. J Nutr. (2008) 138:439–42.
43. Jenkins DJ, Kendall CW, Axelsen M, Augustin LS, Vuksan V. Viscous and nonviscous fibres, nonabsorbable and low glycaemic index carbohydrates, blood lipids and coronary heart disease. Curr Opin Lipidol. (2000) 11:49–56. doi: 10.1097/00041433-200002000-00008
44. Mcintosh M, Miller C. A diet containing food rich in soluble and insoluble fiber improves glycemic control and reduces hyperlipidemia among patients with type 2 diabetes mellitus. Nutr Rev. (2001) 59:52–5. doi: 10.1111/j.1753-4887.2001.tb06976.x
45. Chassard C, Delmas E, Robert C, Bernalier-Donadille A. The cellulose-degrading microbial community of the human gut varies according to the presence or absence of methanogens. FEMS Microbiol Ecol. (2010) 74:205–13.
46. Basen M, Kurrer SE. A close look at pentose metabolism of gut bacteria. FEBS J. (2021) 288:1804–8. doi: 10.1111/febs.15575
47. Weisburger JH, Reddy BS., Rose DP, Cohen LA, Kendall ME, Wynder EL. Protective mechanisms of dietary fibers in nutritional carcinogenesis. In: Bronzetti G, Hayatsu H, De Flora S, Waters MD, Shankel DM, et al. Shankel DM, editors. Antimutagenesis and Anticarcinogenesis Mechanisms III. Basic Life Sciences. Boston, MA: Springer (1993). p. 45–63.
48. Eastwood MA. The physiological effect of dietary fiber: an update. Annu Rev Nutr. (1992) 12:19–35.
49. Zaman SA, Sarbini SR. The potential of resistant starch as a prebiotic. Crit Rev Biotechnol. (2016) 36:578–84.
50. Geetha K, Yankanchi GM, Hulamani S, Hiremath N. Glycemic index of millet based food mix and its effect on pre diabetic subjects. J Food Sci Technol. (2020) 57:2732–8. doi: 10.1007/s13197-020-04309-5
51. Cardona F, Andrés-Lacueva C, Tulipani S, Tinahones FJ, Queipo-Ortuño MI. Benefits of polyphenols on gut microbiota and implications in human health. J Nutr Biochem. (2013) 24:1415–22.
52. García-Villalba R, Beltrán D, Espín JC, Selma MV, Tomás-Barberán FA. Time course production of urolithins from ellagic acid by human gut microbiota. J Agric Food Chem. (2013) 61:8797–806.
53. Stevens JF, Maier CS. The chemistry of gut microbial metabolism of polyphenols. Phytochem Rev. (2016) 15:425–44.
54. Costa EM, Silva S. Chapter 2 - Impact of polyphenols on human gut microbiome and associated biomarkers. In: Pintado ME, Saraiva JMA, Alexandre EMDC editors. Technologies to Recover Polyphenols from AgroFood By-Products and Wastes. Cambridge, MA: Academic Press (2022). p. 25–40.
55. Ma J, Zheng Y, Tang W, Yan W, Nie H, Fang J, et al. Dietary polyphenols in lipid metabolism: a role of gut microbiome. Anim Nutr. (2020) 6:404–9.
56. Del Bo C, Bernardi S, Cherubini A, Porrini M, Gargari G, Hidalgo-Liberona N, et al. A polyphenol-rich dietary pattern improves intestinal permeability, evaluated as serum zonulin levels, in older subjects: the MaPLE randomised controlled trial. Clin Nutr. (2021) 40:3006–18. doi: 10.1016/j.clnu.2020.12.014
57. Rodríguez-Daza MC, Pulido-Mateos EC, Lupien-Meilleur J, Guyonnet D, Desjardins Y, Roy D. Polyphenol-mediated gut microbiota modulation: toward prebiotics and further. Front Nutr. (2021) 8:689456. doi: 10.3389/fnut.2021.689456
58. Tzounis X, Vulevic J, Kuhnle GG, George T, Leonczak J, Gibson GR, et al. Flavanol monomer-induced changes to the human faecal microflora. Br J Nutr. (2008) 99:782–92. doi: 10.1017/S0007114507853384
59. Naowaboot J, Piyabhan P, Munkong N, Parklak W, Pannangpetch P. Ferulic acid improves lipid and glucose homeostasis in high-fat diet-induced obese mice. Clin Exp Pharmacol Physiol. (2016) 43:242–50.
60. Onyango C, Luvitaa S, Unbehend G, Haase N. Physico-chemical properties of flour, dough and bread from wheat and hydrothermally-treated finger millet. J Cereal Sci. (2020) 93:102954.
61. Xiang J, Apea-Bah FB, Ndolo VU, Katundu MC, Beta T. Profile of phenolic compounds and antioxidant activity of finger millet varieties. Food Chem. (2019) 275:361–8.
62. Subba Rao MVSST, Muralikrishna G. Evaluation of the antioxidant properties of free and bound phenolic acids from native and malted finger millet (Ragi, Eleusine coracana Indaf-15). J Agric Food Chem. (2002) 50:889–92. doi: 10.1021/jf011210d
63. Munir KM, Chandrasekaran S, Gao F, Quon MJ. Mechanisms for food polyphenols to ameliorate insulin resistance and endothelial dysfunction: therapeutic implications for diabetes and its cardiovascular complications. Am J Physiol Endocrinol Metab. (2013) 305:E679–86. doi: 10.1152/ajpendo.00377.2013
64. Shahwan M, Alhumaydhi F, Ashraf GM, Hasan PM, Shamsi A. Role of polyphenols in combating type 2 diabetes and insulin resistance. Int J Biol Macromol. (2022) 206:567–79.
65. Matacchione G, Gurãu F, Baldoni S, Prattichizzo F, Silvestrini A, Giuliani A, et al. Pleiotropic effects of polyphenols on glucose and lipid metabolism: focus on clinical trials. Ageing Res Rev. (2020) 61:101074. doi: 10.1016/j.arr.2020.101074
66. Bona KS, Bonfanti G, Bitencourt PE, Silva TP, Borges RM, Boligon A, et al. Protective effect of gallic acid and Syzygium cumini extract against oxidative stress-induced cellular injury in human lymphocytes. Drug Chem Toxicol. (2016) 39:256–63. doi: 10.3109/01480545.2015.1084631
67. Haute GV, Caberlon E, Squizani E, de Mesquita F, Pedrazza L, Martha B, et al. Gallic acid reduces the effect of LPS on apoptosis and inhibits the formation of neutrophil extracellular traps. Toxicol In Vitro. (2015) 30(1 Pt B):309–17. doi: 10.1016/j.tiv.2015.10.005
68. Yang K, Zhang L, Liao P, Xiao Z, Zhang F, Sindaye D, et al. Impact of gallic acid on gut health: focus on the gut microbiome, immune response, and mechanisms of action. Front Immunol. (2020) 11:580208. doi: 10.3389/fimmu.2020.580208
69. Nagao T, Meguro S, Hase T, Otsuka K, Komikado M, Tokimitsu I, et al. A catechin-rich beverage improves obesity and blood glucose control in patients with type 2 diabetes. Obesity. (2009) 17:310–7. doi: 10.1038/oby.2008.505
70. Cho SY, Park PJ, Shin HJ, Kim Y, Shin DW, Shin ES, et al. (-)-Catechin suppresses expression of Kruppel-like factor 7 and increases expression and secretion of adiponectin protein in 3T3-L1 cells. Am J Physiol Endocrinol Metab. (2007) 292:E1166–72. doi: 10.1152/ajpendo.00436.2006
71. Prabhakar PK, Prasad R, Ali S, Doble M. Synergistic interaction of ferulic acid with commercial hypoglycemic drugs in streptozotocin induced diabetic rats. Phytomedicine. (2013) 20:488–94. doi: 10.1016/j.phymed.2012.12.004
72. Mena P, Calani L, Bruni R, Del Rio D. Bioactivation of high-molecular-weight polyphenols by the gut microbiome. In: Tuohy K, Del Rio D editors. Diet-Microbe Interactions in the Gut. Amsterdam: Elsevier (2015). p. 73–101.
73. Mitharwal S, Kumar S, Chauhan K. Nutritional, polyphenolic composition and in vitro digestibility of finger millet (Eleusine coracana L.) with its potential food applications: a review. Food Biosci. (2021) 44:101382.
74. Sharma B, Gujral HS. Influence of nutritional and antinutritional components on dough rheology and in vitro protein & starch digestibility of minor millets. Food Chem. (2019) 299:125115. doi: 10.1016/j.foodchem.2019.125115
75. Sharma B, Gujral HS, Solah V. Effect of incorporating finger millet in wheat flour on mixolab behavior, chapatti quality and starch digestibility. Food Chem. (2017) 231:156–64. doi: 10.1016/j.foodchem.2017.03.118
76. Chandrasekara A, Shahidi F. Bioaccessibility and antioxidant potential of millet grain phenolics as affected by simulated in vitro digestion and microbial fermentation. J Funct Foods. (2012) 4:226–37.
77. Everard A, Lazarevic V, Derrien M, Girard M, Muccioli GG, Neyrinck AM, et al. Responses of gut microbiota and glucose and lipid metabolism to prebiotics in genetic obese and diet-induced leptin-resistant mice. Diabetes. (2011) 60:2775–86. doi: 10.2337/db11-0227
78. Song Y, Wu M, Tao G, Lu M, Lin J, Huang J. Feruloylated oligosaccharides and ferulic acid alter gut microbiome to alleviate diabetic syndrome. Food Res Int. (2020) 137:109410. doi: 10.1016/j.foodres.2020.109410
79. Zhang B, Sun W, Yu N, Sun J, Yu X, Li X, et al. Anti-diabetic effect of baicalein is associated with the modulation of gut microbiota in streptozotocin and high-fat-diet induced diabetic rats. J Funct Foods. (2018) 46:256–67.
80. Wei Y, Chang L, Liu G, Wang X, Yang Y, Hashimoto K. Long-lasting beneficial effects of maternal intake of sulforaphane glucosinolate on gut microbiota in adult offspring. J Nutr Biochem. (2022) 109:109098. doi: 10.1016/j.jnutbio.2022.109098
81. Xu X, Dai M, Lao F, Chen F, Hu X, Liu Y, et al. Effect of glucoraphanin from broccoli seeds on lipid levels and gut microbiota in high-fat diet-fed mice. J Funct Foods. (2020) 68:103858.
82. Napolitano M, Covasa M. Microbiota transplant in the treatment of obesity and diabetes: current and future perspectives. Front Microbiol. (2020) 11:590370. doi: 10.3389/fmicb.2020.590370
83. Wang H, Lu Y, Yan Y, Tian S, Zheng D, Leng D, et al. Promising treatment for type 2 diabetes: fecal microbiota transplantation reverses insulin resistance and impaired islets. Front Cell Infect Microbiol. (2020) 9:455. doi: 10.3389/fcimb.2019.00455
84. Asharani VT, Jayadeep A, Malleshi NG. Natural antioxidants in edible flours of selected small millets. Int J Food Propert. (2010) 13:41–50.
85. Maharajan T, Antony Ceasar S, Ajeesh Krishna T, Ignacimuthu S. Finger millet [Eleusine coracana (L.) Gaertn]: an orphan crop with a potential to alleviate the calcium deficiency in the semi-arid tropics of Asia and Africa. Front Sustain Food Syst. (2021) 5:684447. doi: 10.3389/fsufs.2021.684447
86. Koliada A, Syzenko G, Moseiko V, Budovska L, Puchkov K, Perederiy V, et al. Association between body mass index and Firmicutes/Bacteroidetes ratio in an adult Ukrainian population. BMC Microbiol. (2017) 17:120. doi: 10.1186/s12866-017-1027-1
87. Palmas V, Pisanu S, Madau V, Casula E, Deledda A, Cusano R, et al. Gut microbiota markers associated with obesity and overweight in Italian adults. Sci Rep. (2021) 11:5532. doi: 10.1038/s41598-021-84928-w
89. Divya M, Karthikeyan S, Ravi C, Govindarajan M, Alharbi NS, Kadaikunnan S, et al. Isolation of β-glucan from Eleusine coracana and its antibiofilm, antidiabetic, antioxidant, and biocompatible activities. Microbial Pathog. (2020) 140:103955. doi: 10.1016/j.micpath.2019.103955
90. Jayachandran M, Chen J, Chung SS, Xu B. A critical review on the impacts of β-glucans on gut microbiota and human health. J Nutr Biochem. (2018) 61:101–10.
91. Chandrasekara A, Shahidi F. Determination of antioxidant activity in free and hydrolyzed fractions of millet grains and characterization of their phenolic profiles by HPLC-DAD-ESI-MSn. J Funct Foods. (2011) 3:144–58.
92. Molino S, Lerma-Aguilera A, Jiménez-Hernández N, Gosalbes MJ, Rufián-Henares JÁ, Francino MP. Enrichment of food with tannin extracts promotes healthy changes in the human gut microbiota. Front Microbiol. (2021) 12:625782. doi: 10.3389/fmicb.2021.625782
93. Wang J, Xu W, Wang R, Cheng R, Tang Z, Zhang M. The outer membrane protein Amuc_1100 of Akkermansia muciniphila promotes intestinal 5-HT biosynthesis and extracellular availability through TLR2 signalling. Food Funct. (2021) 12:3597–610. doi: 10.1039/d1fo00115a
94. Xu J, Ge J, He X, Sheng Y, Zheng S, Zhang C, et al. Caffeic acid reduces body weight by regulating gut microbiota in diet-induced-obese mice. J Funct Foods. (2020) 74:104061.
95. Udayappan S, Manneras-Holm L, Chaplin-Scott A, Belzer C, Herrema H, Dallinga-Thie G, et al. Oral treatment with Eubacterium hallii improves insulin sensitivity in db/db mice. NPJ Biofilms Microbiomes. (2016) 2:16009. doi: 10.1038/npjbiofilms.2016.9
96. Kikuchi K, Ben Othman M, Sakamoto K. Sterilized bifidobacteria suppressed fat accumulation and blood glucose level. Biochem Biophys Res Commun. (2018) 501:1041–7. doi: 10.1016/j.bbrc.2018.05.105
97. Youn HS, Kim J, Lee JS, Yoon YY, Choi SJ, Lee JY, et al. Lactobacillus plantarum reduces low-grade inflammation and glucose levels in a mouse model of chronic stress and diabetes. Infect Immun. (2021) 89:e615–20. doi: 10.1128/IAI.00615-20
98. Okazaki Y, Katayama T. Phytic acid actions on hepatic lipids and gut microbiota in rats fed a diet high in sucrose is influenced by dietary fat level. Nutr Res. (2020) 74:45–51. doi: 10.1016/j.nutres.2019.11.010
99. Spranger J, Kroke A, Möhlig M, Hoffmann K, Bergmann MM, Ristow M, et al. Inflammatory cytokines and the risk to develop type 2 diabetes: results of the prospective population-based European prospective investigation into cancer and nutrition (EPIC)-potsdam study. Diabetes. (2003) 52:812–7. doi: 10.2337/diabetes.52.3.812
100. Kanmani S, Kwon M, Shin M, Kim MK. Association of C-reactive protein with risk of developing type 2 diabetes mellitus, and role of obesity and hypertension: a large population-based Korean cohort study. Sci Rep. (2019) 9:4573. doi: 10.1038/s41598-019-40987-8
101. Molehin OR, Adefegha SA, Adeyanju AA. Role of oxidative stress in the pathophysiology of type 2 diabetes and cardiovascular diseases. In: Maurya P, Dua K editors. Role of Oxidative Stress in Pathophysiology of Diseases. Singapore: Springer (2020). p. 277–97.
102. Yan SF, Ramasamy R, Schmidt AM. Mechanisms of disease: advanced glycation end-products and their receptor in inflammation and diabetes complications. Nat Clin Pract Endocrinol Metab. (2008) 4:285–93. doi: 10.1038/ncpendmet0786
103. Esteban-Torres M, Santamaría L, Cabrera-Rubio R, Plaza-Vinuesa L, Crispie F, Rivas B d, et al. A diverse range of human gut bacteria have the potential to metabolize the dietary component gallic acid. Appl Environ Microbiol. (2018) 84:e1558–1518. doi: 10.1128/AEM.01558-18
104. Zhang Z, Wu X, Cao S, Wang L, Wang D, Yang H, et al. Caffeic acid ameliorates colitis in association with increased Akkermansia population in the gut microbiota of mice. Oncotarget. (2016) 7:31790. doi: 10.18632/oncotarget.9306
105. Roopchand DE, Carmody RN, Kuhn P, Moskal K, Rojas-Silva P, Turnbaugh PJ, et al. Dietary polyphenols promote growth of the gut bacterium Akkermansia muciniphila and attenuate high-fat diet–induced metabolic syndrome. Diabetes. (2015) 64:2847–58. doi: 10.2337/db14-1916
106. Cani PD, Neyrinck AM, Fava F, Knauf C, Burcelin RG, Tuohy KM, et al. Selective increases of bifidobacteria in gut microflora improve high-fat-diet-induced diabetes in mice through a mechanism associated with endotoxaemia. Diabetologia. (2007) 50:2374–83. doi: 10.1007/s00125-007-0791-0
107. Lenoir M, Martín R, Torres-Maravilla E, Chadi S, González-Dávila P, Sokol H, et al. Butyrate mediates anti-inflammatory effects of Faecalibacterium prausnitzii in intestinal epithelial cells through Dact3. Gut Microbes. (2020) 12:1826748. doi: 10.1080/19490976.2020.1826748
108. Carlsson AH, Yakymenko O, Olivier I, Håkansson F, Postma E, Keita AV, et al. Faecalibacterium prausnitzii supernatant improves intestinal barrier function in mice DSS colitis. Scand J Gastroenterol. (2013) 48:1136–44. doi: 10.3109/00365521.2013.828773
109. Herder C, Schneitler S, Rathmann W, Haastert B, Schneitler H, Winkler H, et al. Low-grade inflammation, obesity, and insulin resistance in adolescents. J Clin Endocrinol Metab. (2007) 92:4569–74.
110. Akiyama H, Fujii K, Yamasaki O, Oono T, Iwatsuki K. Antibacterial action of several tannins against Staphylococcus aureus. J Antimicrob Chemother. (2001) 48:487–91. doi: 10.1093/jac/48.4.487
111. Larcombe S, Jiang J, Hutton ML, Abud HE, Peleg AY, Lyras D. A mouse model of Staphylococcus aureus small intestinal infection. J Med Microbiol. (2020) 69:290–7. doi: 10.1099/jmm.0.001163
112. Mergani A, Wanes D, Schecker N, Branitzki-Heinemann K, Naim HY, Köckritz-Blickwede M v. Staphylococcus aureus infection influences the function of intestinal cells by altering the lipid raft-dependent sorting of sucrase–isomaltase. Front Cell Dev Biol. (2021) 9:699970. doi: 10.3389/fcell.2021.699970
113. Kao PHN, Kline KA. Dr. Jekyll and Mr. Hide: how Enterococcus faecalis subverts the host immune response to cause infection. J Mol Biol. (2019) 431:2932–45. doi: 10.1016/j.jmb.2019.05.030
114. Iida N, Mizukoshi E, Yamashita T, Yutani M, Seishima J, Wang Z, et al. Chronic liver disease enables gut Enterococcus faecalis colonization to promote liver carcinogenesis. Nat Cancer. (2021) 2:1039–54. doi: 10.1038/s43018-021-00251-3
115. Lamba K, Nelson JA, Kimura AC, Poe A, Collins J, Kao AS, et al. Shiga Toxin 1–producing Shigella sonnei infections, California, United States, 2014–2015. Emerg Infect Dis. (2016) 22:679.
116. Brunner K, Samassa F, Sansonetti PJ, Phalipon A. Shigella-mediated immunosuppression in the human gut: subversion extends from innate to adaptive immune responses. Hum Vaccine Immunother. (2019) 15:1317–25. doi: 10.1080/21645515.2019.1594132
117. Pradhan S, Karve SS, Weiss AA, Hawkins J, Poling HM, Helmrath MA, et al. Tissue responses to Shiga toxin in human intestinal organoids. Cell Mol Gastroenterol Hepatol. (2020) 10:171–90.
118. Wenzler E, Kamboj K, Balada-Llasat J-M. Severe sepsis secondary to persistent Lysinibacillus sphaericus, Lysinibacillus fusiformis and Paenibacillus amylolyticus bacteremia. Int J Infect Dis. (2015) 35:93–5. doi: 10.1016/j.ijid.2015.04.016
119. Hossack DJN. Proteus vulgaris urinary tract infections in rats; treatment with nitrofuran derivatives. Br J Pharmacol Chemother. (1962) 19:306–12.
120. Jóźwiak J, Komar A, Jankowska E, Martirosian G. Determination of the cytotoxic effect of Clostridium histolyticum culture supernatant on HeLa cells in the presence of protease inhibitors. FEMS Immunol Med Microbiol. (2005) 45:137–42. doi: 10.1016/j.femsim.2005.03.005
121. Jóźwiak J, Grzela T, Jankowska-Steifer E, Komar A, Moskalewski S, Martirosian G. Lethal factor of Clostridium histolyticum kills cells by apoptosis. FEMS Immunol Med Microbiol. (2007) 49:296–303. doi: 10.1111/j.1574-695X.2006.00204.x
122. Nithiyanantham S, Kalaiselvi P, Mahomoodally MF, Zengin G, Abirami A, Srinivasan G. Nutritional and functional roles of millets—a review. J Food Biochem. (2019) 43:e12859. doi: 10.1111/jfbc.12859
123. Khare P, Maurya R, Bhatia R, Mangal P, Singh J, Podili K, et al. Polyphenol rich extracts of finger millet and kodo millet ameliorate high fat diet-induced metabolic alterations. Food Funct. (2020) 11:9833–47. doi: 10.1039/d0fo01643h
124. Manisseri C, Gudipati M. Prebiotic activity of purified xylobiose obtained from Ragi (Eleusine coracana, Indaf-15) Bran. Indian J Microbiol. (2012) 52:251–7. doi: 10.1007/s12088-011-0176-4
125. Ashwanandhini G, Reshma R, Preetha R. Synbiotic microencapsulation of Enterococcus faecium Rp1: a potential probiotic isolated from ragi porridge with antiproliferative property against colon carcinoma cell line. J Food Sci Technol. (2022) 59:3888–94. doi: 10.1007/s13197-022-05415-2
126. Chaudhary JK, Mudgal S. Effect of incorporation of finger millet (Eleusine coracana) on the antimicrobial, ACE inhibitory, antioxidant and antidiabetic potential of a milk-millet composite probiotic fermented product. Indian J Dairy Sci. (2020) 73:222–30.
127. Sato S, Mukai Y, Tokuoka Y, Mikame K, Funaoka M, Fujita S. Effect of lignin-derived lignophenols on hepatic lipid metabolism in rats fed a high-fat diet. Environ Toxicol Pharmacol. (2012) 34:228–34. doi: 10.1016/j.etap.2012.04.005
128. Fischer F, Romero R, Hellhund A, Linne U, Bertrams W, Pinkenburg O, et al. Dietary cellulose induces anti-inflammatory immunity and transcriptional programs via maturation of the intestinal microbiota. Gut Microbes. (2020) 12:1829962. doi: 10.1080/19490976.2020.1829962
129. Kim Y, Hwang SW, Kim S, Lee Y, Kim T, Lee S, et al. Dietary cellulose prevents gut inflammation by modulating lipid metabolism and gut microbiota. Gut Microbes. (2020) 11:944–61.
Keywords: bio accessibility, gut microbes, obesity, diabetes, insulin resistance, inflammation, endotoxemia, finger millet
Citation: Singh V, Lee G, Son H, Amani S, Baunthiyal M and Shin J-H (2022) Anti-diabetic prospects of dietary bio-actives of millets and the significance of the gut microbiota: A case of finger millet. Front. Nutr. 9:1056445. doi: 10.3389/fnut.2022.1056445
Received: 28 September 2022; Accepted: 05 December 2022;
Published: 22 December 2022.
Edited by:
Afam I. O. Jideani, University of Venda, South AfricaReviewed by:
Ramona Suharoschi, University of Agricultural Sciences and Veterinary Medicine of Cluj-Napoca, RomaniaCopyright © 2022 Singh, Lee, Son, Amani, Baunthiyal and Shin. This is an open-access article distributed under the terms of the Creative Commons Attribution License (CC BY). The use, distribution or reproduction in other forums is permitted, provided the original author(s) and the copyright owner(s) are credited and that the original publication in this journal is cited, in accordance with accepted academic practice. No use, distribution or reproduction is permitted which does not comply with these terms.
*Correspondence: Mamta Baunthiyal, ✉ mamtabaunthiyal@yahoo.co.in; Jae-Ho Shin, ✉ jhshin@knu.ac.kr
†These authors have contributed equally to this work
‡ORCID: Vineet Singh, orcid.org/0000-0003-2458-3282; Jae-Ho Shin, orcid.org/0000-0001-6450-9787
Disclaimer: All claims expressed in this article are solely those of the authors and do not necessarily represent those of their affiliated organizations, or those of the publisher, the editors and the reviewers. Any product that may be evaluated in this article or claim that may be made by its manufacturer is not guaranteed or endorsed by the publisher.
Research integrity at Frontiers
Learn more about the work of our research integrity team to safeguard the quality of each article we publish.