- College of Food and Chemical Engineering, Shaoyang University, Shaoyang, China
Compared with small-molecule synthetic drugs, bioactive peptides have desirable advantages in efficiency, selectivity, safety, tolerance, and side effects, which are accepted by attracting extensive attention from researchers in food, medicine, and other fields. However, unacceptable barriers, including mucus barrier, digestive enzyme barrier, and epithelial barrier, cause the weakening or the loss of bioavailability and biostability of bioactive peptides. The nanocarrier system for bioactive peptide delivery needs to be further probed. We provide a comprehensive update on the application of versatile delivery systems for embedding bioactive peptides, including liposomes, polymer nanoparticles, polysaccharides, hydrogels, and self-emulsifying delivery systems, and further clarify their structural characterization, advantages, and disadvantages as delivery systems. It aims to provide a reference for the maximum utilization of bioactive peptides. It is expected to be an effective strategy for improving the bioavailability and biostability of bioactive peptides.
Introduction
Recently, researchers have focused on bioactive peptides from various origins, including animal (1), plant (2, 3), marine organisms (4, 5), bean products (6), milk (7), and fermented products (8). They are generally composed of 2–50 amino acid residues in different combinations and arrangements forming linear or cyclic structures (9). In addition, bioactive peptides have a variety of human body metabolism and physiological regulation functions, which are easy to digest and absorb, and have the effects of promoting immunity, hormone regulation, antibacterial, antiviral, decreasing blood pressure, and reducing blood lipid (9–11). They are widely used in food, medicine, and other fields. Particularly, it is the most popular research topic and functional factor with great development prospects in the current international food industry.
Bioactive peptides play an important role in cancer treatment, new drug screening, vaccine development, and nutritional drug delivery and are considered desired substitutes for drugs. Compared with macromolecular proteins, bioactive peptides have the advantages of high activity, tissue permeability, and weak immunogenicity under the same unit mass conditions. Compared with small molecular substances, bioactive peptides have stronger potency, selectivity, specificity, and lower cytotoxicity and drug interactions (12). Oral administration is a direct and effective way of bioactive peptide administration. However, after oral administration, bioactive peptides may be degraded by gastric acidic secretion, digestive enzymes, intestinal brush border membrane, and intestinal epithelial cell endopeptidase in the gastrointestinal tract (13). During oral administration, it is possible that bioactive peptides are hydrolyzed by 40 kinds of enzymes at least, causing subsequent loss of bioactivity before reaching systemic circulation. It was reported that most food protein-derived bioactive peptides containing more than two–three amino acid residues do not withstand simulated gastrointestinal enzymatic digestion (14). Although dipeptides and tripeptides can be fully absorbed through the synergistic transport mechanism of peptide transporters (PepT1 and PepT2), and the brush border membrane Na+/ H+ exchange transport system, or enter cells through endocytosis and cell bypass (15) as shown in Figure 1, it is still degraded into free amino acids by peptidases in blood and lose bioactivity (16). We studied the gastrointestinal digestion stability of bioactive peptides and found that the peptide composed of amino acid residues such as Phe, Tyr, Val, Leu, Arg, and Lys were not tolerant to gastrointestinal fluid digestion (5, 17, 18). It was reported that after intravenous injection of angiotensin-converting enzyme (ACE) inhibitory peptide Ile-Val-Tyr in the spontaneously hypertensive rat (SHR), it can be degraded into dipeptide Val-Tyr by the aminopeptidase existing in the blood. After intravenous injection, Ile-Val-Tyr had a better blood pressure reduction effect and could maintain for 15 min. Val-Tyr alone had an acute hypotension effect, but the blood pressure returned to normal after 5 min of injection (19). Therefore, the bioavailability of bioactive peptides depends directly on their amino acid sequence. When entering the body to perform an anti-hypertensive effect, it is likely to be degraded and weakened or lost activity.
The undesirable bioavailability of bioactive peptides has prompted researchers to explore efficient methods for complete delivery. Encapsulation of bioactive peptides in nanoparticles is an effective way to prevent their degradation and enhance their bioactivity (20). Many nanocarrier systems have been developed, including lipid particles, polysaccharide particles, biopolymer nanoparticles, and colloidal particles, each of which has its own advantages and limitations (21). There are various administration ways including subcutaneous injection, intravenous injection, and oral administration. In consideration of bioactive peptides derived from food resources, in general, used as functional foods and nutritional supplements, oral administration is preferential (22). Therefore, this study reviewed oral delivery systems of bioactive peptides. Furthermore, the structural characterization and application status of these delivery systems were summarized, and their advantages and disadvantages were analyzed, in order to provide a reference for the application of bioactive peptides.
Liposome carrier applied in bioactive peptide delivery
Structural characterization of the liposome delivery system
Liposome, as an efficient organic carrier, is a kind of closed vesicle similar to a biofilm structure formed by the inclusion of phospholipid and cholesterol. When the liposome is dispersed in the aqueous phase, the hydrophobic groups will spontaneously aggregate together under the hydrophobic interaction, and the hydrophilic groups will also aggregate with each other. When the system is stable, the closed ring multilayer structure of “head to head, tail to tail” will be formed (Figure 2A). The interactions between peptide and liposome depend on the hydrophobicity of the peptide, and the structure of the interfacial head-group region of the lipid bilayers of liposome was also important (23). Phospholipid-forming liposome is eliminated slowly in the blood, so drugs loaded on liposome remain in the blood circulation system for a long time. In addition, liposomes can dissolve in the cell membrane of the target organs or tissue to fulfill intracellular transportation of drugs and, thus, improve the bioavailability and stability of drugs. Liposomes contain polar, nonpolar, and amphiphilic regions in the same colloidal particles, so they are particularly suitable for the encapsulation of active peptides (24). The liposome has an aqueous solution core surrounded by a hydrophobic membrane in the form of a lipid bilayer. Hydrophilic solutes dissolved in the nucleus are not easily associated with the bilayer by hydrophobic chemicals. Therefore, liposomes can load hydrophobic and/or hydrophilic molecules. Moreover, amphiphilic peptides locate on the interface between the shell and core of the liposome structure, which would interact with the hydrophobic and hydrophilic amino acid residues, respectively. The liposome is similar to cell membranes and is favorable for the delivery of bioactive compounds, which can otherwise be degraded by the physiological environment (25).
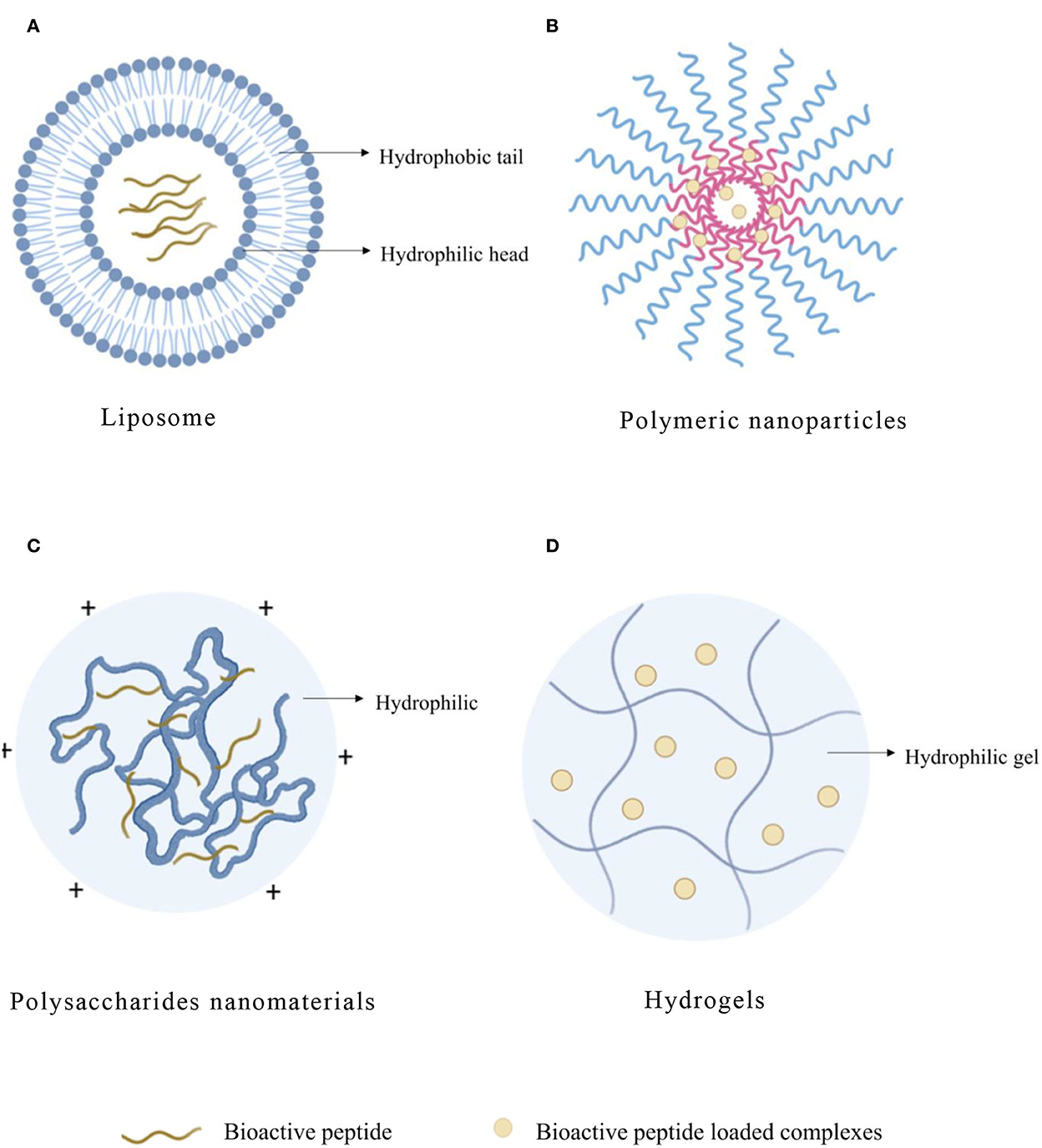
Figure 2. Typical structure of bioactive peptide carriers. (A) Liposome. (B) Polymeric nanoparticles. (C) Polysaccharides nanomaterials. (D) Hydrogels.
Practical application of the liposome delivery system
The liposome delivery system has been used to package, protect, and deliver bioactive peptides for the prevention or treatment of diseases in the pharmaceutical industry (26). KRX29 is a small cyclic peptide with potential pathological effects in the treatment of heart failure, which is an effective and selective G protein-coupled receptor kinase 2 (GRK2) activity inhibitor. Although KRX29 presents a small molecular weight (eight amino acids) and can be performed in oral and intravenous administration, it has certain disadvantages including low absorption, unstable physiological environment, and short half-life. It needs to be wrapped in suitable carriers to overcome these limitations. It was found that the bioavailability and stability of drugs could be improved by wrapping into the nano-sized liposomes by thin film hydration and then by ultrasonic-assisted size reduction process (27). In addition, the encapsulated Arg-Leu-Ser-Phe-Asn-Pro (RLSFNP), a hexapeptide milk-derived angiotensin-converting enzyme (ACE) inhibitory peptide, into liposomes with remarkable sustained release and storage capacity can be directly absorbed by Caco-2 cells (28). It has also been found that muramyl peptides encapsulated in liposomes can kill macrophages more effectively than unencapsulated ones (29). Rezaei et al. found that the mutant N-terminal peptide with a zinc binding ring replaced by a disulfide bond ring (ES-SS peptide) has anti-angiogenesis and antitumor properties compared with natural peptides. In order to improve the stability and plasma half-life of ES-SS peptide, ES-SS peptide nanoliposomes with different phosphatidylcholine (PC) ratios were synthesized. ES-SS peptide was gradually released by liposomes. Compared with free peptides, liposome peptide preparation has a higher cell survival rate (30). Heuts et al. (31) studied the possibility of carrying synthetic long peptide (SLP) antigen on liposomes based on 1,2-dioleoyl-3-trimethylammonium propane (DOTAP) and further verified the potential of DOTAP liposomes as a vaccine delivery platform for therapeutic cancer vaccines. Another study also found that P5HER-2/neu derived peptide (P5) in DOTAP-cholesterol drug liposomes injected with CpG-ODN significantly enhanced the response of cytotoxic T cells and highly inhibited the progression of tumors (32). The major problems limiting the widespread use of liposomes are stability, encapsulation efficiency, and lysosomal degradation. Chemical instability may be caused by the hydrolysis of ester bonds and/or oxidation of unsaturated acyl chains of lipids. Liposomal drug formulation could only be affirmative if the encapsulation efficiency is such that therapeutic doses could be delivered in a reasonable amount of lipid, since lipids in high doses may be toxic and also cause non-linear (saturable) pharmacokinetics. Liposomes may deliver the drug to the cells selectively, but the pharmacological activity depends on the ability of the intact drug against degradation to diffuse into the cytoplasm from the endosomes in sufficient amounts (33, 34).
Micelles are unilamellar vesicles made up of amphiphilic polymeric molecules with a hydrophobic core hidden inside the structure and a hydrophilic shell directed outwards with a size range of 10–100 nm (35). Drugs are loaded into micelles either through chemical covalent bonding or through physical encapsulation. Micelles have many advantages as drug delivery carriers, including ease of preparation, stability under physiological conditions, prolonged shelf life, extended circulation time in blood and biological fluids, increased bioavailability, and enhanced pharmacokinetics and biodistribution properties (36, 37). The peptide amphiphile micelles are small spherical particles able to stably associate with cells, making them attractive for the targeted delivery via the vasculature, and induce significant peptide-based toxicity in human lymphoma cells (38). Peptide amphiphile micelles (PAMs) are attractive vehicles for the delivery of a variety of therapeutic and prophylactic peptides (39). Furthermore, one cleavage-inducing target of interest could be glutathione, the primary antioxidant in the body, which is present in low concentrations in the blood, but much higher concentrations within cells (40–42). In the food industry, liposomal delivery of proteins, enzymes, vitamins, antioxidants, and seasonings has been studied (43). It was reported that encapsulation of ghrelin (a bioactive polypeptide) into liposomes can improve its chemical stability and increase the retention time of this appetite-suppressing hormone in the blood (44). Liposomes are also used to encapsulate bioactive peptides extracted from hydrolyzed fish protein (45). Liposome encapsulation has been proven to be an appropriate method to reduce the bitterness of bioactive peptides (46). Montero et al. wrapped the shrimp polypeptide component (ST1) with biological activity, including ABTS free radical scavenging ability, angiotensin-converting enzyme activity, and dipeptidyl polypeptidase-IV inhibitory activity, into partially purified soybean phospholipid nanoliposome (L-ST1). The soybean-derived phospholipid-forming liposomes can be bound to the edible membrane of sodium caseinate without significantly losing the integrity of the vesicle structure, thereby increasing the water solubility of the liposome delivery system. Furthermore, the increase in membrane humidity and the presence of liposomes are beneficial to the adhesion of the membrane, especially when they are loaded with polypeptides (47). It has shown that the inclusion of bacteriocins in liposomes can control food spoilage and pathogens and increase the effective safety and shelf life of some foods, as antimicrobial agents can coexist in the aqueous and lipid phases of liposomes (48). The large-scale production of liposome carry is a distinct advantage compared with other carrier systems. Liposomes adapted from the pharmaceutical industry have certain shortcomings in functional food applications. Particularly, the thermal instability of liposome-encapsulated food-derived peptides beyond the phase transition temperature of the phospholipid can limit their incorporation in thermally processed food (49).
The characteristics for applications of liposomes are shown in Table 1. Compared with other embedding techniques, the dominant advantage of the liposome delivery system is that they endow the substances with water-soluble and stability in high water activity (50). Liposomes can also protect the drugs against degradation and integrally release them into target organs or tissues that need to be treated so that the drug concentration in these target organs or tissues is increased, and the therapeutic effect of drugs can be improved. In addition, a drug-loaded liposome delivery system presents resistance to metabolism and degradation by intracellular enzymes, less adverse reaction, and benefits on biocompatibility, targeting ability, and controlled release of the drug. Liposomes have been shown to be stable in embedding materials free from a range of environmental and chemical changes, including enzyme and chemical modifications, as well as buffers against extreme pH and temperature (51). The phospholipids making up liposomes can be commonly found in nature, such as in soybean and egg yolks. However, these are difficult to use in clinical settings due to stability and contamination risks (52).
Solid lipid nanoparticles applied in bioactive peptide delivery
Structural characterization of solid lipid nanoparticle
Solid lipid nanoparticle (SLN) is made of natural, semi-synthetic, or synthetic lipids, which contain triglycerides, fatty acids, phospholipids, and steroids, and is widely considered a safe and biodegradable carrier (53). SLNS is a promising technology for the targeted delivery of anticancer drugs (54). The main disadvantage of SLNS is that the encapsulation efficiency is affected by the polymorph structure of solid lipids. If SLN is composed of high-purity single lipids, a perfect lattice may be formed during storage, which may reduce the solubility of drugs in the lipid matrix and eventually lead to the expulsion of encapsulated drugs. When SLN forms, lipids crystallize in disordered α and β crystal structures. During storage, lipids are gradually arranged in a more stable and orderly manner, resulting in β′ and β crystalline forms from which encapsulated drugs can be discharged (55). Drug-loaded SLN combines the best characteristics of polymer nanocarrier and liposome delivery system. It maximizes drug targeting and creates uniform particle distribution, thus providing low toxicity and good histocompatibility, but it is easy to be degraded. Therefore, the utilization efficiency is poor, and it cannot guarantee that the embedded SLN can reach the target organ and tissue and then release it (Table 2).
Practical application of solid lipid nanoparticle
Dumont et al. found that SLN is a promising system to overcome the inherent barriers of stability and bioavailability of the peptide by oral administration. It has been proved that SLN can encapsulate peptides after lipidation without peptide denaturation (56). Christophersen et al. (57) studied the degradation mechanisms of peptide-loaded SLNs containing different types of lipid excipients and found that the release rate became faster after lysozyme was added. However, the hydrophobicity of SLN limits the encapsulation of hydrophilic peptides. Su et al. (58) found that SLN could effectively maintain the stability of bioactive peptides in gastrointestinal digestion. In addition, the macromolecular peptide could be successfully wrapped in SLN and nanocrystallized, thereby improving their physical and chemical stability. In particular, the encapsulation in SLN protects the secondary hydrolysis of bioactive peptides in simulated gastrointestinal fluid, which helps maintain their dipeptidyl peptidase IV (DPP4) inhibitory function. It has encapsulated water-soluble peptides in biodegradable and biocompatible SLN, but the encapsulated peptides are easily released in simulated gastrointestinal fluid in the presence of proteases (59). SLN is a potential carrier system for transporting protein-type antigen vaccines while avoiding the use of organic solvents (60). Kadari et al. (61) found that angiopep-2-modified SLN was an excellently targeted drug delivery system for glioma therapy. SLN could be used to transport antioxidant glutathione (GSH) to immunocompetent fish cells, and the antioxidant activity of GSH in vitro is stable in the transport process (62).
Solid lipid nanoparticle-engineered nanostructured lipid carrier is formulated. Insulin and insulin analog—glargine insulin—are entrapped in the lipid matrix through hydrophobic ion pairing. Studies in vitro and in vivo were carried out by employing fluorescently labeled peptides. The superiority of glargine insulin-loaded nanostructured lipid carrier demonstrated significantly higher permeation (till 30% dose/ml) compared to the free peptide (63). Xu et al. (64) developed functional nanocarriers for efficient oral administration of biological macromolecules, and the developed SLN was proved to increase serum insulin concentration and produced good hypoglycemic reaction. In addition, the prepared insulin-loaded cationic solid lipid nanoparticle (CSLN) by water-in-oil emulsion technology can protect the encapsulated insulin from the degradation of pepsin and trypsin and improve its biostability (65). Hydrophobic ion pairing of leuprolide and insulin, chosen as model peptides, allowed the encapsulation of these molecules in SLN produced through the coacervation technique, nanoparticles in vitro can be used as a polypeptide sustained release system, maintaining the integrity of the peptide (66). SLN prepared by thermo-high pressure homogenization method has the advantages of good physical stability, protection of mixed unstable active substances, and controlled release, which is very suitable as a carrier for transporting active components to the skin. Furthermore, SLN technology allows polypeptides to enter the skin to play a protective role (67).
Since the basic template of SLNs is a solid lipid, lipophilic drugs have more affinity toward SLNs than hydrophilic ones. For the preparation of SLNs with lipophilic drugs, oil-in-water (O/W) emulsions are preferred. For the encapsulation of hydrophilic drugs, multiple emulsion systems such as water/oil/water (W/O/W) emulsion with or without the use of organic solvents are reported (68). Furthermore, water-in-oil high internal phase emulsions (W/O HIPEs) could be successfully used to encapsulate bitter peptides, which reduced their bitterness and improved their gastric stability (69). Double emulsion of the water-in-oil-in-water (W1/O/W2) type is one of the carrier systems used for encapsulation, protection, and delivery of both hydrophilic and hydrophobic active components (70). Recently, double emulsions have been studied in the delivery of hydrophilic bioactive compounds in the food and pharmaceutical industries (71, 72). Among the different bioactive peptide concentrations (1%−20%), 5% of bioactive peptides was determined to be the amount to encapsulate in the W1 phase in the double emulsion that would ensure emulsion droplet stability (72).
The self-emulsifying carrier applied in bioactive peptide delivery
Structural characterization of self-emulsifying drug delivery system
Self-emulsifying drug delivery system (SEDDS) is a lipid carrier, which is spontaneously formed by the isotropic mixture of oil, surfactant, and cosurfactant through mixing with water (73). SEDDS has high biocompatibility, which can protect polypeptides from degradation and increase membrane permeability. It has been widely used to improve the oral bioavailability of insoluble drugs, which can help overcome the obstacles encountered in the oral administration of hydrophilic macromolecules, namely, intestinal protease degradation, poor mucus permeability, and low cellular uptake (74). It has been proved that pepsin, trypsin, chymotrypsin, elastase, and other hydrolases are not soluble in oily SEDDS droplets. Therefore, due to the hydrophilicity of peptidases and proteolytic enzymes, they cannot enter the lipid droplets of SEDDS containing bioactive peptides, which can protect them against enzymatic degradation (75, 76). Compared with other nanocarriers, SEDDS preparation is simpler and more cost-effective. At present, the self-emulsifying method has been successfully applied to the transportation of anticancer drugs, antiviral drugs, antibacterial drugs, immunosuppressants, and natural products such as antioxidants and alkaloids (Table 3).
Practical application of self-emulsifying drug delivery system
Self-emulsifying drug delivery system is discovered for the oral administration of hydrophilic drugs such as peptides, proteins, polysaccharides, and pDNA (77). Due to hydrophobic ion pairing (HIP) with oppositely charged lipophilic auxiliary agents, the resulting complexes can be incorporated into the lipophilic phase of SEDDS. Depending on the solubility of the complex in the pre-concentrate SEDDS, drug release can be adjusted on purpose by choosing more or less lipophilic auxiliary agents in appropriate quantities for the HIP. Within the oily droplets formed in the gastrointestinal tract, drugs are protected from degradation by proteases and nucleases and thiol-disulfide exchange reactions with dietary proteins. The oily droplets can be made mucoadhesive or highly mucus permeating depending on their target site. Furthermore, it can be tuned by adjusting their zeta potential or decorating them with cell-penetrating peptides (74).
Sustained release of bioactive peptides from SEDDS seems essential to avoid their degradation in the gastrointestinal tract. SEDDS has been shown to penetrate effectively into the intestinal mucus gel layer, making peptides or protein drugs closely in contact with the absorption membrane (73). Compared with other drug delivery systems, SEDDS is the most effective mucus permeation system. In vitro studies showed that the penetration of exenatide containing SEDDS was 2.7 times higher than that of drugs without SEDDS. In vivo evaluation experiment showed that compared with the control group, the area under plasma concentration curves (AUC) of blood glucose in the SEDDS group was decreased by 20.6% (78). Zupančič et al. (79) found that SEDDS showed mucus permeability and had protective effects on the degradation of trypsin, α-chymotrypsin, elastase, simulated gastric juice, and simulated intestinal juice. Mahmood et al. added hydrophilic macromolecular drugs into SEDDS, which could protect them from the enzyme and thiol barriers in the gastrointestinal tract. In addition, SEDDS can penetrate the mucus gel barrier in a relatively effective way to promote the transport of the combined hydrophilic macromolecular drugs to the underlying epithelial cells (74).
Polymeric nanoparticles applied in bioactive peptide delivery
Structural characterization of polymeric nanoparticle
Polymeric nanoparticle (NP) refers to a solid particle with a size range from 10 to 1,000 nm, which can encapsulate drugs in the polymer matrix to prevent them from being degraded by enzymes (Figure 2B). Compared with liposome and SLN, NP also showed higher stability in biological fluids (Table 4). In addition, it is a promising delivery system for proteins and peptides because of the versatility, sustained release, protection of encapsulated proteins, and peptides from enzymatic degradation and biocompatibility of nanoparticles (80). Polymers have great functionalization ability and can be used to couple targeted ligands to improve biological distribution (81). NP can also compensate for the disadvantages of peptide treatment by increasing bioavailability, exhibiting controlled and sustained release, and enhancing cellular uptake (82). In addition, compared with other particles, NPs can effectively transfer across the epithelial surface (83). The polymer matrix can protect peptides from degradation and provide a desirable controlled release of peptides (84).
Polylactic-glycolic acid copolymer (PLGA) is a very important synthetic polymer material for preparing polymeric nanoparticles, which can achieve safe and effective delivery of drugs without causing tissue damage (85, 86). It has been confirmed that PLGA-based nanoparticles (PLGANPs) have a smaller particle size, better environmental adaptability, and biocompatibility than hydrogels, alginates, and chitosan (CS) polymer-coated particles (87). In addition, the adhesion and cell uptake of PLGANPs can be significantly improved by functionalizing positively charged polymers or proteins on the surface of particles (88–90).
Rastogi et al. used a modified s/o/w method to prepare insulin-loaded polycaprolactone-polymer poly (ethylene glycol)-polycaprolactone (PCL-PEG-PCL) nanoparticles in order to obtain higher drug loading. Insulin was hydrophobically modified by sodium deoxycholate. After pairing with sodium deoxycholate, the lipophilicity of insulin was increased by five times, and the encapsulation efficiency was increased by 10%−50% (91). In addition, polypeptide encapsulated with nanoparticles based on polymer poly (ethylene glycol)-block-polycaprolactone (PEG-b-PCL) presented the cytotoxicity induced by polyglutamine (80). Furthermore, Kelly et al. (92) screened and developed porous silicon nanoparticle (PSNP) composites for micRNA inhibition of intracellular delivery of peptide nucleic acid (PNA).
Practical application of polymeric nanoparticle
A recent study has shown that polyethylene glycol (PEG) embedding can be used as a protective method or a ligand competition for antimicrobial peptide BP100 transposition (93). It is suggested the feasibility of applying antimicrobial peptides carried on PEG-capped NPs for possible treatments of lung diseases. The anticancer peptide NuBCP-9 was encapsulated in NPs to enhance efficacy and avoid drug resistance, which was enhanced by increasing the PEG chain length in the block copolymers (94). Hoshino et al. confirmed that NPs accelerated the clearance of toxic peptides, which is a 26 amino acid cytolytic peptide isolated from bee venom, and finally accumulated in liver macrophages. PEG hydrophilic polymer particles also help to improve the biocompatibility of synthetic NPs and enhance their stability in plasma, revealing the potential and possible limitations of using synthetic ones as a plastic antidote (95). Cellulose nanocrystal (CNC) is a natural, non-toxic, and neutral material with a rod-like or spherical construction. It has been applied in various fields such as pharmaceuticals, food industry, paper industry, composites, and polymer composites (96). Cellulose-based composite macrogels made by cellulose fiber/cellulose nanofiber (CCNM) are used as an intestine delivery vehicle for probiotics (97).
Polysaccharide nanomaterial applied in bioactive peptide delivery
Structural characterization of polysaccharides nanomaterial
Polymer-based nanocarriers can be divided into natural polymers and synthetic polymers. Chitosan (CS) is a kind of natural polysaccharide isolated from the excrement of crustaceans and fungi (98). Most polysaccharides contain hydrophilic groups such as hydroxyl, carboxyl, and amino, which can form noncovalent bonds with intestinal mucus and promote the absorption of bioactive peptides (Figure 2C). As bioactive peptides contain amphipathic substances (that harbor both negatively and positively charged molecules) similar to proteins, they can also electrostatically interact with ionic polysaccharides with positive or negative charge to form associative complexes (99). Due to their effective biological activity, non-toxic, hydrophilic, good biocompatibility and biodegradability, and extensive diversity in structure and properties (100), polysaccharide-based nanomaterials are widely used in intracellular drug delivery as one of the most practical materials in nanomedicine field (Table 5).
Practical application of polysaccharides nanomaterial
The CS-based polysaccharide nanomaterial is prepared by the interaction between positively charged CS and negatively charged chlorinated polyethylene (PEC) through charges. It is considered to be a mild drug delivery method because no organic solvent or ultrasonic treatment is required (101). Yu et al. (102) developed a new type of CS-protamine nanoparticles for the anticancer effect of nuclear-targeted delivery of 5-fluorouracil (5-FU). Compared with free 5-FU, 5-FU-CS-protamine nanoparticles had the strongest cytotoxicity on tumor A549 cells and HeLa cells. In addition, an increase in apoptosis rate was also observed. It is worth noting that 5-FU-CS-protamine NPS can significantly reduce the tumor volume of BALB/c HeLa cell xenograft mice. Gao et al. (103) studied the combined delivery of irinotecan (IRN) and 5-FU by chitosan-hyaluronic acid (CS-HA) hybrid nanoparticles for the targeted treatment of gastric cancer. IRN/5-FU-CS-HA-NPs enhanced the killing effect on human gastric cancer cells in vitro, enhanced the antitumor activity in vivo of tumor-bearing mice, and had good antitumor activity. On the other hand, under extreme conditions, such as high temperature, the polysaccharide wall is susceptible to reacting with the peptide to form complex products (e.g., Maillard reaction products), which can be potentially toxic and also deplete the bioactive peptides. In order to circumvent this challenge, the reactive functional groups of polysaccharides have been modified such as carboxymethylation to produce relatively inert carriers. The molecular structure of polysaccharides contributes to their stability as carriers during the production and processing of encapsulated products (104, 105). Nasri et.al encapsulate goby fish protein hydrolysate (GPH), endowed with antioxidant activity, through an ionic gelation process using blue crab chitosan and tripolyphosphate anions and to evaluate the structural, thermal, and antioxidant properties of the elaborated microparticles. The increase in loaded GPH concentration led to an increase in encapsulation efficiency and a reduction in particle size. Furthermore, thermogravimetric analysis (TGA) profiles revealed the enhanced thermal stability of encapsulated biopeptides compared to the free ones (106).
Chitosan is a cationic polymer that allows electrostatic interaction with negatively charged sialic acid residues on the mucosal surface (107). Based on this principle, PLGA and FA-modified CS (FA-CS) composite nanocarriers were prepared by the electrostatic self-assembly method. The system can protect insulin against digestive enzymes and reduce the toxicity to HT-29 cells. In vivo experiments further showed that the system had an obvious hypoglycemic effect on diabetic mice, and the prepared composite nanocarriers were expected to become drug carriers for oral insulin and other biological macromolecules (108). Fish-purified antioxidant peptide (AOP)-loaded chitosan nanoparticles (CSNPs) were synthesized for Fourier transform infra-red (FT-IR) analysis, which demonstrated that the electrostatic interactions between biomolecule and polymer were considered as the driving force for peptide-loaded NPs formation (109). Similarly, sulfhydryl-modified low-molecular-weight chitosan was anchored to the lysine residue of insulin. These protein/chitosan conjugates have also proved to protect proteins against proteolytic enzymes, resulting in increased oral bioavailability of protein (110). PLGA covered with glycol-chitosan was used to encapsulate an HIV fusion inhibitor peptide (E2). Furthermore, the assessments ex vivo and in vivo were carried out to examine the ability of NPs to reach the vaginal epithelium. The penetration rate was measured in a swine model using confocal microscopy. E2-loaded NPs have interesting physicochemical and morphological features that provide them with mobility across the mucus, to reach the vaginal epithelium and release E2 (111). The W9-peptide, a tumor necrosis factor (TNF), was used to study the feasibility of cholesterol-bearing pullulan (CHP) nanogel as the drug delivery system. It found CHP nanogel could form a complex with the W9-peptide and prevents its aggregation in vitro (112). It suggested that the CHP nanogel worked as a suitable carrier for the W9 peptide. Santos et al. found that antimicrobial peptide (AMP) nisin has a wide range of action against pathogens and can treat diabetic foot ulcers (DFU) in patients with diabetes. However, AMP will be degraded or inactivated before reaching the target at the treatment concentration. Natural polysaccharide guar gum gel as the delivery system loading AMP can be used to treat systemic bacterial skin infections, especially those promoted by pathogens so that the sensitivity of bacteria to current antibiotics is reduced (113).
Hydrogel delivery system
Structural characterization of hydrogel delivery system
Hydrogel has three-dimensional networks of water swelling made of polymers, proteins, small molecules, or colloids (Figure 2D), and the obtained hydrogels show ultra-stable properties in highly acidic or alkaline aqueous solutions (114). The network of a hydrogel is established via covalent bonds or noncovalent interactions. Noncovalent interactions mainly include physical entanglements, hydrogen bonds, hydrophobic interactions, supramolecular interactions, electrostatic interactions, and coordination interactions (115). Hydrogel has adjustable physical properties. It prevents the degradation of unstable drugs as an effective drug delivery system. The versatility and diversity of hydrogels extend their applications to targeted drug delivery, wound dressings, contact lenses, and tissue engineering (Table 6). They contain 90% water and are highly porous to accommodate drugs for delivery and promote the controlled release (116).
Practical application of hydrogel delivery system
They developed a novel melittin-RADA28 (MR) hydrogel, composed of RADA28 and melittin, in order to promote the membrane permeability of tumor cells with the membrane-disrupting ability of melittin. It demonstrated that the formed melittin-RADA28-Lys-Leu-Ala-Lys-Leu-Ala-Lys-Lys-Leu-Ala-Lys-Leu-Ala-Lys (KLA) peptide (MRP) hydrogel has a nanofiber structure, sustained release, and attenuated hemolysis effects. Compared with free KLA, the MRP hydrogel markedly increased the cellular accumulation of KLA, produced the highest ratio of depolarized mitochondrial membrane, and decreased cell viability in vitro (117). The antibacterial effect of different concentrations of RADA16 on Staphylococcus aureus, Candida albicans, and Escherichia coli were compared. It was found that 0.25% of peptide-loaded RADA16 hydrogel could play a good antibacterial activity in a short time (118). The stable structure formed by RADA16 hydrogel provided an important carrier platform for the continuous release of antimicrobial peptides, which not only inhibited the reproduction of S. aureus, but also promoted the proliferation of bone marrow mesenchymal stem cells to a certain extent, and enhanced their osteogenic ability (119).
Dextran nanogels can be exploited as carriers for sustained/controlled release of bioactive molecules. Molinos et al. prepared a new bidimensional composite hydrogel, made of oxidized dextrin incorporating dextrin nanogel (oDex nanogel). It provides continuous delivery of protein and prevents problems of cyclic variations in the protein concentration in the blood with time. In addition, it offers maximum pharmacological efficiency at a minimum drug dose, reducing administration frequency and improving patient compliance with the therapy (120). Hirakura et al. reported a novel injectable hybrid hyaluronan (HA) hydrogel system of physically encapsulated CHP nanogels for sustained delivery of protein. Furthermore, a new hybrid hydrogel composed of CHP nanogels presented chaperone-like protection for fragile protein drugs and chemically cross-linked HA as the biocompatible controlled release matrix. CHP/protein complex nanogels were released from the hybrid hydrogels in a sustained manner in vitro and in vivo (121). The main challenge is to design a hydrogel system capable of providing drug release for several months and complete clearance at the end of drug release or in a relatively short period of time to avoid matrix accumulation (122).
Concluding remarks and future perspectives
The application of nanotechnology in biomedicine and functional food to improve human health is indispensable. The properties of nanocarriers with different materials can meet the different needs of bioactive peptide delivery. It shows great advantages in solving targeting, stability, loading, release rate, biodegradability, and biocompatibility of bioactive peptides. Certain problems to be solved urgently in the nanotechnology of bioactive peptides are as follows: (1) Due to the unique structure, it is difficult to separate and purify peptide drugs, and the effects of various modification methods and solvents may change the molecular structure of bioactive peptides, resulting in the loss of biological functions. (2) Although many nanocarriers have been shown to be low-toxic or non-toxic in vitro, it is still unclear whether they will affect organisms or even humans, and the preclinical acute and chronic toxicity research must be paid enough attention by researchers. (3) It is worth pondering whether bioactive peptides can effectively cross multiple biological barriers in vivo due to their complex forms of polymorphism and conformation. (4) After most bioactive peptides enter cells, they need to undergo intracellular transport and intracellular release to exert physiological and pharmacological effects depending on delivery efficiency. (5) It is another important problem that how nanocarriers adapt to individual differences. In summary, in the future, efforts should be made to further verify the physiological conditions such as pH value, protease level, mucus, epithelial barrier, and intestinal transport pathway, which can guide the formulation optimization and design of new delivery systems to improve stability and bioavailability of bioactive peptides after oral administration.
Author contributions
XZ composed the outline and drafted the manuscript. QW reviewed and approved the submitted version. XL, YZ, QZ, and YY read and approved the final version. All authors had full access to data and revised and approved the manuscript for publication.
Funding
We gratefully acknowledge the financial support from the National Natural Scientific Foundation of China (Grant Number 32202573) and the Natural Science Foundation of Hunan Province (Grant Number 2022JJ50028).
Conflict of interest
The authors declare that the research was conducted in the absence of any commercial or financial relationships that could be construed as a potential conflict of interest.
Publisher's note
All claims expressed in this article are solely those of the authors and do not necessarily represent those of their affiliated organizations, or those of the publisher, the editors and the reviewers. Any product that may be evaluated in this article, or claim that may be made by its manufacturer, is not guaranteed or endorsed by the publisher.
References
1. Bechaux J, Gatellier P, Le Page JF, Drillet Y, Sante-Lhoutellier V. A comprehensive review of bioactive peptides obtained from animal byproducts and their applications. Food Funct. (2019) 10:6244–66. doi: 10.1039/C9FO01546A
2. Zheng X, Li DS, Ding K. Purification and identification of angiotensin I-converting enzyme inhibitory peptides from fermented walnut residues. Int J Food Prop. (2017) 20:S3326–33. doi: 10.1080/10942912.2016.1258574
3. Wu Q, Li Y, Peng K, Wang XL, Ding Z, Liu L, et al. Isolation and characterization of three antihypertension peptides from the mycelia of Ganoderma lucidum (Agaricomycetes). J Agr Food Chem. (2019) 67:8149–59. doi: 10.1021/acs.jafc.9b02276
4. Kang HK, Lee HH, Seo CH, Park Y. Antimicrobial and immunomodulatory properties and applications of marine-derived proteins and peptides. Mar Drugs. (2019) 17:350. doi: 10.3390/md17060350
5. Wu Q, Cai QF, Tao ZP, Sun LC, Shen JD, Zhang LJ, et al. Purification and characterization of a novel angiotensin I-converting enzyme inhibitory peptide derived from abalone (Haliotis discus hannai Ino) gonads. Eur Food Res Technol. (2015) 240:137–45. doi: 10.1007/s00217-014-2315-8
6. Tamam B, Syah D, Suhartono MT, Kusuma WA, Tachibana S, Lioe HN, et al. Proteomic study of bioactive peptides from tempe. J Biosci Bioeng. (2019) 128:241–8. doi: 10.1016/j.jbiosc.2019.01.019
7. Shivanna SK, Nataraj BH. Revisiting therapeutic and toxicological fingerprints of milk-derived bioactive peptides: an overview. Food Biosci. (2020) 38:100771. doi: 10.1016/j.fbio.2020.100771
8. Wong FC, Xiao J, Wang S, Ee KY, Chai TT. Advances on the antioxidant peptides from edible plant sources. Trends Food Sci Technol. (2020) 99:44–57. doi: 10.1016/j.tifs.2020.02.012
9. Karami Z, Akbari-Adergani B. Bioactive food derived peptides: a review on correlation between structure of bioactive peptides and their functional properties. J Food Sci Tech. (2019) 56:535–47. doi: 10.1007/s13197-018-3549-4
10. Kapila R, Kapila S, Vij R. Efficacy of milk-derived bioactive peptides on health by cellular and animal models. In: Ronald Ross W, Robert JC, Victor R, editors. Preedy Nutrients in Dairy and their Implications on Health and Disease. Amsterdam: Elsevier (2017), p. 303–11. doi: 10.1016/B978-0-12-809762-5.00023-1
11. Acevedo-Juárez S, Guajardo-Flores D, Heredia-Olea E, Antunes-Ricardo M. Bioactive peptides from nuts: a review. Int J Food Sci Tech. (2022) 57:2226–34. doi: 10.1111/ijfs.15543
12. Waseem M, Kumar S, Kumar A. Bioactive Peptides. Hauppauge, NY: Nova Science Publisher Inc. (2018).
13. Martínez-Maqueda D, Miralles B, Recio I, Hernández-Ledesma B. Antihypertensive peptides from food proteins: a review. Food Funct. (2012) 3:350–61. doi: 10.1039/c2fo10192k
14. Peng S, Song H, Chen Y, Li S, Guan X. Oral Delivery of food-derived bioactive peptides: challenges and strategies. Food Rev Int. (2022) 4:1–29. doi: 10.1080/87559129.2022.2062772
15. Gallego M, Grootaert C, Mora L, Aristoy MC, Van Camp J, Toldrá F, et al. Transepithelial transport of dry-cured ham peptides with ACE inhibitory activity through a Caco-2 cell monolayer. J Funct Foods. (2016) 21:388–95. doi: 10.1016/j.jff.2015.11.046
16. Moskowitz DW. Is somatic angiotensin I-converting enzyme a mechanosensor. Diabetes Technol Ther. (2002) 4:841–58. doi: 10.1089/152091502321118847
17. Wu Q, Cai QF, Yoshida A, Sun LC, Liu YX, Liu GM, et al. Purification and characterization of two novel angiotensin I-converting enzyme inhibitory peptides derived from R-phycoerythrin of red algae (Bangia fusco-purpurea). Eur Food Res Technol. (2017) 243:779–89. doi: 10.1007/s00217-016-2792-z
18. Wu Q, Fu XP, Sun LC, Zhang Q, Liu GM, Cao MJ, et al. Effects of physicochemical factors and in vitro gastrointestinal digestion on antioxidant activity of R-phycoerythrin from red algae Bangia fusco-purpurea. Int J Food Sci Tech. (2015) 50:1445–51. doi: 10.1111/ijfs.12775
19. Iroyukifujita H, Eiichiyokoyama K, Yoshikawa M. Classification and antihypertensive activity of angiotensin I-converting enzyme inhibitory peptides derived from food proteins. J Food Sci. (2000) 65:564–9. doi: 10.1111/j.1365-2621.2000.tb16049.x
20. Chen SY, Xu XX, Li X, Yi NB, Li SZ, Xiang XC, et al. Recent advances in the intracellular delivery of macromolecule therapeutics. Biomater Sci. (2022) 4:1–29. doi: 10.1039/D2BM01348G
21. Hosseini SF, Ramezanzade L, McClements DJ. Recent advances in nanoencapsulation of hydrophobic marine bioactives: bioavailability, safety, and sensory attributes of nano-fortified functional foods. Trends Food Sci Tech. (2021) 109:322–39. doi: 10.1016/j.tifs.2021.01.045
22. Vigani B, Rossi S, Sandri G, Bonferoni MC, Caramella CM, Ferrari F, et al. Recent advances in the development of in situ gelling drug delivery systems for non-parenteral administration routes. Pharmaceutics. (2020) 12:859. doi: 10.3390/pharmaceutics12090859
23. Alavi, Mehran, Hamidi, Mehrdad. Passive and active targeting in cancer therapy by liposomes and lipid nanoparticles. Drug Metab Pers Ther. (2019) 34:20180032. doi: 10.1515/dmpt-2018-0032
24. Sakai-Kato K, Yoshida K, Takechi-Haraya Y, Izutsu KI. Physicochemical characterization of liposomes that mimic the lipid composition of exosomes for effective intracellular trafficking. Langmuir. (2020) 36:12735–44. doi: 10.1021/acs.langmuir.0c02491
25. Bartelds R, Nematollahi MH, Pols T, Stuart MC, Pardakhty A, Asadikaram G, et al. Niosomes, an alternative for liposomal delivery. PLoS ONE. (2018) 13:e0194179. doi: 10.1371/journal.pone.0194179
26. Sonju JJ, Dahal A, Singh SS, Jois SD. Peptide-functionalized liposomes as therapeutic and diagnostic tools for cancer treatment. J Control Release. (2021) 329:624–44. doi: 10.1016/j.jconrel.2020.09.055
27. Bochicchio S, Sala M, Spensiero A, Scala MC, Gomez-Monterrey IM, Lamberti G, et al. On the design of tailored liposomes for KRX29 peptide delivery. New J Chem. (2017) 41:11280–90. doi: 10.1039/C7NJ03115G
28. Zhang T, Su M, Jiang X, Xue Y, Zhang J, Zeng X, et al. Transepithelial transport route and liposome encapsulation of milk-derived ACE-inhibitory peptide Arg-Leu-Ser-Phe-Asn-Pro. J Agr Food Chem. (2019) 67:5544–51. doi: 10.1021/acs.jafc.9b00397
29. Nayar R, Schroit AJ, Fidler IJ. Liposome encapsulation of muramyl peptides for activation of macrophage cytotoxic properties. Methods Enzymol. (1986) 132:594–603. doi: 10.1016/S0076-6879(86)32044-5
30. Rezaei N, Mehrnejad F, Vaezi Z, Sedghi M, Asghari SM, Naderi-Manesh H, et al. Encapsulation of an endostatin peptide in liposomes: stability, release, and cytotoxicity study. Colloid Surface B. (2020) 185:110552. doi: 10.1016/j.colsurfb.2019.110552
31. Heuts J, Varypataki EM, van der Maaden K, Romeijn S, Drijfhout JW, van Scheltinga AT, et al. Cationic liposomes: a flexible vaccine delivery system for physicochemically diverse antigenic peptides. Pharm Res-Dordr. (2018) 35:1–9. doi: 10.1007/s11095-018-2490-6
32. Mansourian M, Badiee A, Jalali SA, Shariat S, Yazdani M, Amin M, et al. Effective induction of anti-tumor immunity using p5 HER-2/neu derived peptide encapsulated in fusogenic DOTAP cationic liposomes co-administrated with CpG-ODN. Immunol Lett. (2014) 162:87–93. doi: 10.1016/j.imlet.2014.07.008
33. Wang J, Gong J, Wei Z. Strategies for liposome drug delivery systems to improve tumor treatment efficacy. AAPS Pharm Sci Tech. (2022) 23:1–14. doi: 10.1208/s12249-021-02179-4
34. Guimarães D, Cavaco-Paulo A, Nogueira E. Design of liposomes as drug delivery system for therapeutic applications. Int J Pharm. (2021) 601:120571. doi: 10.1016/j.ijpharm.2021.120571
35. Amin MCIM, Butt AM, Amjad MW, Kesharwani P. Polymeric micelles for drug targeting and delivery. In: Mishra V, Kesharwani P, Mohd Amin MCM, Iyer A, editors. Nanotechnology-based Approaches for Targeting and Delivery of Drugs and Genes. Amsterdam: Elsevier Inc. (2017), p. 167–202. doi: 10.1016/B978-0-12-809717-5.00006-3
36. Lu Y, Zhang E, Yang J, Cao Z. Strategies to improve micelle stability for drug delivery. Nano Res. (2018) 11:4985–98. doi: 10.1007/s12274-018-2152-3
37. Hanafy NAN, El-Kemary M, Leporatti S. Micelles structure development as a strategy to improve smart cancer therapy. Cancers. (2018) 10:238. doi: 10.3390/cancers10070238
38. Smith JD, Cardwell LN, Porciani D, Nolla A, Cornelison BT, Schulte MC, et al. Therapeutic peptide delivery via aptamer-displaying, disulfide-linked peptide amphiphile micelles. Mol Syst Des Eng. (2020) 5:269–83. doi: 10.1039/C9ME00092E
39. Smith JD, Cardwell LN, Porciani D, Nguyen JA, Zhang R, Gallazzi F, et al. Aptamer-displaying peptide amphiphile micelles as a cell-targeted delivery vehicle of peptide cargoes. Phys Biol. (2018) 15:065006. doi: 10.1088/1478-3975/aadb68
40. Ju P, Hu J, Li F, Cao Y, Li L, Shi D, et al. A biodegradable polyphosphoester-functionalized poly (disulfide) nanocarrier for reduction-triggered intracellular drug delivery. J Mater Chem B. (2018) 6:7263–73. doi: 10.1039/C8TB01566J
41. Cheng YJ, Zhang AQ, Hu JJ, He F, Zeng X, Zhang XZ, et al. Multifunctional peptide-amphiphile end-capped mesoporous silica nanoparticles for tumor targeting drug delivery. ACS Appl Mater Inter. (2017) 9:2093–103. doi: 10.1021/acsami.6b12647
42. Wu D, Li Y, Shen J, Tong Z, Hu Q, et al. Supramolecular chemotherapeutic drug constructed from pillararene-based supramolecular amphiphile. Chem Commun. (2018) 54:8198–201. doi: 10.1039/C8CC04334E
43. Mohammadi A, Jafari SM, Mahoonak AS, Ghorbani M. Liposomal/nanoliposomal encapsulation of food-relevant enzymes and their application in the food industry. Food Bioprocess Tech. (2021) 14:23–38. doi: 10.1007/s11947-020-02513-x
44. Moeller EH, Holst B, Nielsen LH, Pedersen PS, Ostergaard J. Stability, liposome interaction, and in vivo pharmacology of ghrelin in liposomal suspensions. Int J Pharmaceut. (2010) 390:13–8. doi: 10.1016/j.ijpharm.2009.05.067
45. Ramezanzade L, Hosseini SF, Akbari-Adergani B, Yaghmur A. Cross-linked chitosan-coated liposomes for encapsulation of fish-derived peptide. Lwt. (2021) 150:112057. doi: 10.1016/j.lwt.2021.112057
46. Hosseini SF, Soofi M, Rezaei M. Enhanced physicochemical stability of ω-3 PUFAs concentrates-loaded nanoliposomes decorated by chitosan/gelatin blend coatings. Food Chem. (2021) 345:128865. doi: 10.1016/j.foodchem.2020.128865
47. Montero P, Mosquera M, Marín-Peñalver D, Alemán A, Martínez-Álvarez Ó, Gómez-Guillén MC, et al. Changes in structural integrity of sodium caseinate films by the addition of nanoliposomes encapsulating an active shrimp peptide fraction. J Food Eng. (2019) 244:47–54. doi: 10.1016/j.jfoodeng.2018.09.024
48. da Silva Malheiros P, Daroit DJ, Brandelli A. Food applications of liposome-encapsulated antimicrobial peptides. Trends Food Sci Tech. (2010) 21:284–92. doi: 10.1016/j.tifs.2010.03.003
49. Mohan A, Rajendran SR, He QS, Bazinet L, Udenigwe CC. Encapsulation of food protein hydrolysates and peptides: a review. RSC Adv. (2015) 5:79270–8. doi: 10.1039/C5RA13419F
50. Maja L, Željko K, Mateja P. Sustainable technologies for liposome preparation. J Supercrit Fluid. (2020) 165:104984. doi: 10.1016/j.supflu.2020.104984
51. Antimisiaris SG, Marazioti A, Kannavou M, Natsaridis E, Gkartziou F, Kogkos G, et al. Overcoming barriers by local drug delivery with liposomes. Adv Drug Deliver Rev. (2021) 174:53–86. doi: 10.1016/j.addr.2021.01.019
52. Kondratowicz A, Weiss M, Juzwa W, Majchrzycki Ł, Lewandowicz G. Characteristics of liposomes derived from egg yolk. Open Chem. (2019) 17:763–78. doi: 10.1515/chem-2019-0070
53. Wang T, Xue J, Hu Q, Zhou M, Luo Y. Preparation of lipid nanoparticles with high loading capacity and exceptional gastrointestinal stability for potential oral delivery applications. J Colloid Interf Sci. (2017) 507:119–30. doi: 10.1016/j.jcis.2017.07.090
54. Akbari J, Saeedi M, Ahmadi F, Hashemi SMH, Babaei A, et al. Solid lipid nanoparticles and nanostructured lipid carriers: a review of the methods of manufacture and routes of administration. Pharm Dev Technol. (2022) 27:525–44. doi: 10.1080/10837450.2022.2084554
55. Westesen K, Bunjes H, Koch MHJ. Physicochemical charac- terization of lipid nanoparticles and evaluation of their drug loading capacity and sustained release potential. J Control Release. (1997) 48:223–36. doi: 10.1016/S0168-3659(97)00046-1
56. Dumont C, Bourgeois S, Fessi H, Jannin V. Lipid-based nanosuspensions for oral delivery of peptides, a critical review. Int J Pharmaceut. (2018) 541:117–35. doi: 10.1016/j.ijpharm.2018.02.038
57. Christophersen PC, Birch D, Saarinen J, Isomäki A, Nielsen HM, Yang M, et al. Investigation of protein distribution in solid lipid particles and its impact on protein release using coherent anti-Stokes Raman scattering microscopy. J Control Release. (2015) 197:111–20. doi: 10.1016/j.jconrel.2014.10.023
58. Su L, Zhou F, Yu M, Ge R, He J, Zhang B, et al. Solid lipid nanoparticles enhance the resistance of oat-derived peptides that inhibit dipeptidyl peptidase IV in simulated gastrointestinal fluids. J Funct Foods. (2020) 65:103773. doi: 10.1016/j.jff.2019.103773
59. Dumont C, Bourgeois S, Fessi H, Dugas PY, Jannin V. In-vitro evaluation of solid lipid nanoparticles: ability to encapsulate, release and ensure effective protection of peptides in the gastrointestinal tract. Int J Pharmaceuti. (2019) 565:409–18. doi: 10.1016/j.ijpharm.2019.05.037
60. Francis JE, Skakic I, Smooker PM. Design and preparation of solid lipid nanoparticle (SLN)-mediated DNA vaccines. In: Sunil T, editors. Vaccine Design. New York, NY: Humana (2022), p. 355–66. doi: 10.1007/978-1-0716-1892-9_18
61. Kadari A, Pooja D, Gora RH, Gudem S, Kolapalli VRM, Kulhari H, et al. Design of multifunctional peptide collaborated and docetaxel loaded lipid nanoparticles for antiglioma therapy. Eur J Pharm Biopharm. (2018) 132:168–79. doi: 10.1016/j.ejpb.2018.09.012
62. Trapani A, Tripodo G, Mandracchia D, Cioffi N, Ditaranto N, Cerezuela R, et al. Glutathione loaded solid lipid nanoparticles: preparation and in vitro evaluation as delivery systems of the antioxidant peptide to immunocompetent fish cells. J Cellular Biotechnol. (2016) 2:1–14. doi: 10.3233/JCB-15022
63. Muntoni E, Marini E, Ahmadi N, Milla P, Ghè C, Bargoni A, et al. Lipid nanoparticles as vehicles for oral delivery of insulin and insulin analogs: preliminary ex vivo and in vivo studies. Acta Diabetol. (2019) 56:1283–92. doi: 10.1007/s00592-019-01403-9
64. Xu Y, Zheng Y, Wu L, Zhu X, Zhang Z, Huang Y, et al. Novel solid lipid nanoparticle with endosomal escape function for oral delivery of insulin. ACS Appl Mater Inter. (2018) 10:9315–24. doi: 10.1021/acsami.8b00507
65. Hecq J, Amighi K, Goole J. Development and evaluation of insulin-loaded cationic solid lipid nanoparticles for oral delivery. J Drug Deliv Sci Tech. (2016) 36:192–200. doi: 10.1016/j.jddst.2016.10.012
66. Gallarate M, Battaglia L, Peira E, Trotta M. Peptide-loaded solid lipid nanoparticles prepared through coacervation technique. Int J Chem Eng. (2011) 2011:1–6. doi: 10.1155/2011/132435
67. Souto EB, Baldim I, Oliveira WP, Rao R, Yadav N, Gama FM, et al. SLN and NLC for topical, dermal, and transdermal drug delivery. Expert Opin Drug Deliv. (2020) 17:357–77. doi: 10.1080/17425247.2020.1727883
68. Sakellari GI, Zafeiri I, Batchelor H, Spyropoulos F. Formulation design, production and characterisation of solid lipid nanoparticles (SLN) and nanostructured lipid carriers (NLC) for the encapsulation of a model hydrophobic active. Food Hydrocoll Health. (2021) 1:100024. doi: 10.1016/j.fhfh.2021.100024
69. Gao Y, Wu X, McClements DJ, Cheng C, Xie Y, Liang R, et al. Encapsulation of bitter peptides in water-in-oil high internal phase emulsions reduces their bitterness and improves gastrointestinal stability. Food Chem. (2022) 386:132787. doi: 10.1016/j.foodchem.2022.132787
70. Jo Y-J, Karbstein HP, van der Schaaf US. Collagen peptide-loaded W 1 /O single emulsions and W 1 /O/W 2 double emulsions: influence of collagen peptide and salt concentration, dispersed phase fraction and type of hydrophilic emulsifier on droplet stability and encapsulation efficiency. Food Funct. (2019) 10:3312–23. doi: 10.1039/C8FO02467G
71. Gharehbeglou P, Jafari SM, Homayouni A, Hamishekar H, Mirzaei H. Fabrication of double W1/O/W2 nano-emulsions loaded with oleuropein in the internal phase (W1) and evaluation of their release rate. Food Hydrocoll. (2019) 89:44–55. doi: 10.1016/j.foodhyd.2018.10.020
72. Choi MJ, Choi D, Lee J, Jo YJ. Encapsulation of a bioactive peptide in a formulation of W1/O/W2-type double emulsions: formation and stability. Food Struct. (2020) 25:100145. doi: 10.1016/j.foostr.2020.100145
73. Joyce P, Dening TJ, Meola TR, Schultz HB, Holm R, Thomas N, et al. Solidification to improve the biopharmaceutical performance of SEDDS: opportunities and challenges. Adv Drug Deliver Rev. (2019) 142:102–17. doi: 10.1016/j.addr.2018.11.006
74. Mahmood A, Bernkop-Schnürch A. SEDDS: a game changing approach for the oral administration of hydrophilic macromolecular drugs. Adv Drug Deliver Rev. (2018) 142:91–101. doi: 10.1016/j.addr.2018.07.001
75. Hetényi G, Griesser J, Moser M, Demarne F, Jannin V, Bernkop-Schnürch A, et al. Comparison of the protective effect of self-emulsifying peptide drug delivery systems towards intestinal proteases and glutathione. Int J Pharmaceut. (2017) 523:357–65. doi: 10.1016/j.ijpharm.2017.03.027
76. Griesser J, Hetényi G, Kadas H, Demarne F, Jannin V, Bernkop-Schnürch A, et al. Self-emulsifying peptide drug delivery systems: how to make them highly mucus permeating. Int J Pharmaceut. (2018) 538:159–66. doi: 10.1016/j.ijpharm.2018.01.018
77. AboulFotouh K, Allam AA, El-Badry M, El-Sayed AM. Role of self-emulsifying drug delivery systems in optimizing the oral delivery of hydrophilic macromolecules and reducing interindividual variability. Colloids Surface B. (2018) 167:82–92. doi: 10.1016/j.colsurfb.2018.03.034
78. Menzel C, Holzeisen T, Laffleur F, Zaichik S, Abdulkarim M, Gumbleton M, et al. In vivo evaluation of an oral self-emulsifying drug delivery system (sedds) for exenatide. J Control Release Soc. (2018) 277:165. doi: 10.1016/j.jconrel.2018.03.018
79. Zupančič O, Rohrer J, Thanh Lam H, Grießinger JA, Bernkop-Schnürch A. Development and in vitro characterization of self-emulsifying drug delivery system (SEDDS) for oral opioid peptide delivery. Drug Dev Ind Pharm. (2017) 43:1694–702. doi: 10.1080/03639045.2017.1338722
80. Kim MR, Feng T, Zhang Q, Chan HYE, Chau Y. Co-encapsulation and co-delivery of peptide drugs via polymeric nanoparticles. Polymers. (2019) 11:288. doi: 10.3390/polym11020288
81. Castro KCD, Costa JM, Campos MGN. Drug-loaded polymeric nanoparticles: a review. Int J Polym Mater. (2022) 71:1–13. doi: 10.1080/00914037.2020.1798436
82. Evans BC, Fletcher RB, Kilchrist KV, Dailing EA, Mukalel AJ, Colazo JM, et al. An anionic, endosome-escaping polymer to potentiate intracellular delivery of cationic peptides, biomacromolecules, and nanoparticles. Nat Commun. (2019) 10:1–19. doi: 10.1038/s41467-019-12906-y
83. Lee C, Choi JS, Kim I, Oh KT, Lee ES, Park ES, et al. Long-acting inhalable chitosan-coated poly (lactic-co-glycolic acid) nanoparticles containing hydrophobically modified exendin-4 for treating type 2 diabetes. Int J Nanomed. (2013) 8:2975. doi: 10.2147/IJN.S48197
84. Baghaee PT, Divsalar A, Chamani J, Donya A. Human serum albumin-malathion complex study in the presence of silver nanoparticles at different sizes by multi spectroscopic techniques. J Biomol Struct Dyn. (2019) 37:2254–64. doi: 10.1080/07391102.2018.1491416
85. Allahyari M, Mohit E. Peptide/protein vaccine delivery system based on PLGA particles. Hum Vaccines Immunother. (2016) 12:806–28. doi: 10.1080/21645515.2015.1102804
86. Castro PM, Baptista P, Madureira AR, Sarmento B, Pintado ME. Combination of PLGA nanoparticles with mucoadhesive guar-gum films for buccal delivery of antihypertensive peptide. Int J Pharmaceut. (2018) 547:593–601. doi: 10.1016/j.ijpharm.2018.05.051
87. Menon JU, Ravikumar P, Pise A, Gyawali D, Hsia CC, Nguyen KT, et al. Polymeric nanoparticles for pulmonary protein and DNA delivery. Acta Biomater. (2014) 10:2643–52. doi: 10.1016/j.actbio.2014.01.033
88. Zhang X, Sun M, Zheng A, Cao D, Bi Y, Sun J, et al. Preparation and characterization of insulin-loaded bioadhesive PLGA nanoparticles for oral administration. Eur J Pharm Sci. (2012) 45:632–8. doi: 10.1016/j.ejps.2012.01.002
89. Dyawanapelly S, Koli U, Dharamdasani V, Jain R, Dandekar P. Improved mucoadhesion and cell uptake of chitosan and chitosan oligosaccharide surface-modified polymer nanoparticles for mucosal delivery of proteins. Drug Deliv Transl Res. (2016) 6:365–79. doi: 10.1007/s13346-016-0295-x
90. Sheng J, Han L, Qin J, Ru G, Li R, Wu L, et al. N-trimethyl chitosan chloride-coated PLGA nanoparticles overcoming multiple barriers to oral insulin absorption. ACS Appl Mater Inter. (2015) 7:15430–41. doi: 10.1021/acsami.5b03555
91. Rastogi R, Anand S, Koul V. Evaluation of pharmacological efficacy of ‘insulin–surfoplex'encapsulated polymer vesicles. Int J Pharmaceut. (2009) 373:107–15. doi: 10.1016/j.ijpharm.2009.01.022
92. Kelly III IB, Fletcher RB, McBride JR, Weiss SM, Duvall CL. Tuning composition of polymer and porous silicon composite nanoparticles for early endosome escape of anti-microRNA peptide nucleic acids. ACS Appl Mater Inter. (2020) 12:39602–11. doi: 10.1021/acsami.0c05827
93. Souza FR, Fornasier F, Carvalho AS, Silva BM, Lima MC, Pimentel AS, et al. Polymer-coated gold nanoparticles and polymeric nanoparticles as nanocarrier of the BP100 antimicrobial peptide through a lung surfactant model. J Mol Liq. (2020) 314:113661. doi: 10.1016/j.molliq.2020.113661
94. Kumar M, Gupta D, Singh G, Sharma S, Bhat M, Prashant CK, et al. Novel polymeric nanoparticles for intracellular delivery of peptide cargos: antitumor efficacy of the BCL-2 conversion peptide NuBCP-9. Delivery of NuBCP in polymeric nanoparticles. Cancer Res. (2014) 74:3271–81. doi: 10.1158/0008-5472.CAN-13-2015
95. Hoshino Y, Koide H, Furuya K, Haberaecker III WW, Lee SH, et al. The rational design of a synthetic polymer nanoparticle that neutralizes a toxic peptide in vivo. Proc Natl Acad Sci. (2012) 109:33–8. doi: 10.1073/pnas.1112828109
96. Maheri H, Hashemzadeh F, Shakibapour N, Kamelniya E, Malaekeh-Nikouei B, Mokaberi P, et al. Glucokinase activity enhancement by cellulose nanocrystals isolated from jujube seed: a novel perspective for type II diabetes mellitus treatment (in vitro). J Mol Struct. (2022) 1269:133803. doi: 10.1016/j.molstruc.2022.133803
97. Luan Q, Zhou W, Zhang H, Bao Y, Zheng M, Shi J, et al. Cellulose-based composite macrogels from cellulose fiber and cellulose nanofiber as intestine delivery vehicles for probiotics. J Agr Food Chem. (2018) 66:339–45. doi: 10.1021/acs.jafc.7b04754
98. Silva SS, Mano JF, Reis RL. Ionic liquids in the processing and chemical modification of chitin and chitosan for biomedical applications. Green Chem. (2017) 19:1208–20. doi: 10.1039/C6GC02827F
99. Choi D, Min S-G, Jo Y-J. Functionality of porcine skin hydrolysates produced by hydrothermal processing for liposomal delivery system. J Food Biochem. (2018) 42:e12464. doi: 10.1111/jfbc.12464
100. Liu H, Wang C, Li C, Qin Y, Wang Z, Yang F, et al. A functional chitosan-based hydrogel as a wound dressing and drug delivery system in the treatment of wound healing. RSC Adv. (2018) 8:7533–49. doi: 10.1039/C7RA13510F
101. Genedy HH, Delair T, Montembault A. Chitosan based microRNA nanocarriers. Pharmaceuticals. (2022) 15:1036. doi: 10.3390/ph15091036
102. Yu X, Hou J, Shi Y, Su C, Zhao L. Preparation and characterization of novel chitosan-protamine nanoparticles for nucleus-targeted anticancer drug delivery. Int J Nanom. (2016) 11:6035. doi: 10.2147/IJN.S117066
103. Gao Z, Li Z, Yan J, Wang P. Irinotecan and 5-fluorouracil-co-loaded, hyaluronic acid-modified layer-by-layer nanoparticles for targeted gastric carcinoma therapy. Drug Des Dev Ther. (2017) 11:2595. doi: 10.2147/DDDT.S140797
104. Liu W, Ye A, Liu W, Liu C, Han J, Singh H, et al. Behaviour of liposomes loaded with bovine serum albumin during in vitro digestion. Food Chem. (2015) 175:16–24. doi: 10.1016/j.foodchem.2014.11.108
105. O'Neill GJ, Egan T, Jacquier JC, O'Sullivan M, O'Riordan ED. Kinetics of immobilisation and release of tryptophan, riboflavin and peptides from whey protein microbeads. Food Chem. (2015) 180:150–5. doi: 10.1016/j.foodchem.2015.01.131
106. Nasri R, Hamdi M, Touir S, Li S, Karra-Chaabouni M, Nasri M, et al. Development of delivery system based on marine chitosan: encapsulationand release kinetic study of antioxidant peptides from chitosan microparticle. Int J Biol Macromol. (2021) 167:1445–51. doi: 10.1016/j.ijbiomac.2020.11.098
107. Song X, Chen Y, Zhao G, Sun H, Che H, Leng X, et al. Effect of molecular weight of chitosan and its oligosaccharides on antitumor activities of chitosan-selenium nanoparticles. Carbohyd polym. (2020) 231:115689. doi: 10.1016/j.carbpol.2019.115689
108. Xu B, Jiang G, Yu W, Liu D, Liu Y, Kong X, et al. Preparation of poly (lactic-co-glycolic acid) and chitosan composite nanocarriers via electrostatic self assembly for oral delivery of insulin. Mat Sci Eng. (2017) 78:420–8. doi: 10.1016/j.msec.2017.04.113
109. Hosseini SF, Soleimani MR, Nikkhah M. Chitosan/sodium tripolyphosphate nanoparticles as efficient vehicles for antioxidant peptidic fraction from common kilka. Int J Biol Macromol. (2018) 111:730–7. doi: 10.1016/j.ijbiomac.2018.01.023
110. Safdar R, Thanabalan M. Developments in insulin delivery and potential of chitosan for controlled release application: a review. J Drug Deliv Sci Technol. (2022) 77:103873. doi: 10.1016/j.jddst.2022.103873
111. Ariza-Sáenz M, Espina M, Bolaños N, Calpena AC, Gomara MJ, Haro I, et al. Penetration of polymeric nanoparticles loaded with an HIV-1 inhibitor peptide derived from GB virus C in a vaginal mucosa model. Eur J Pharm Biopharm. (2017) 120:98–106. doi: 10.1016/j.ejpb.2017.08.008
112. Alles N, Soysa NS, Hussain MA, Tomomatsu N, Saito H, Baron R, et al. Polysaccharide nanogel delivery of a TNF-α and RANKL antagonist peptide allows systemic prevention of bone loss. Eur J Pharm Sci. (2009) 37:83–8. doi: 10.1016/j.ejps.2009.01.002
113. Santos R, Gomes D, Macedo H, Barros D, Tibério C, Veiga AS, et al. Guar gum as a new antimicrobial peptide delivery system against diabetic foot ulcers Staphylococcus aureus isolates. J Med Microbiol. (2016) 65:1092–9. doi: 10.1099/jmm.0.000329
114. Narayanaswamy R, Torchilin VP. Hydrogels and their applications in targeted drug delivery. Molecules. (2019) 24:603. doi: 10.3390/molecules24030603
115. Ou Y, Tian M. Advances in multifunctional chitosan-based self-healing hydrogels for biomedical application. J Mater Chem B. (2021).
116. Yang Z, McClements DJ, Li C, Sang S, Chen L, Long J, et al. Targeted delivery of hydrogels in human gastrointestinal tract: a review. Food Hydrocoll. (2022) 134:108013. doi: 10.1016/j.foodhyd.2022.108013
117. Feng JP, Zhu R, Jiang F, Xie J, Gao C, Li M, et al. Melittin-encapsulating peptide hydrogels for enhanced delivery of impermeable anticancer peptides. Biomater Sci. (2020) 8:4559–69. doi: 10.1039/C9BM02080B
118. Li HL, Xu L, He XD, Li JX. Exploration on antimicrobial activity of self-assembled peptide (RADA16-I). World Latest Med Inf . (2017) 66:19–21. doi: 10.19613/j.cnki.1671-3141.2017.66.008
119. Yang G, Huang T, Wang Y, Wang H, Li Y, Yu K, et al. Sustained release of antimicrobial peptide from self-assembling hydrogel enhanced osteogenesis. J Biomater Sci Polym Ed. (2018) 29:1812–24. doi: 10.1080/09205063.2018.1504191
120. Molinos M, Carvalho V, Silva DM, Gama FM. Development of a hybrid dextrin hydrogel encapsulating dextrin nanogel as protein delivery system. Biomacromolecules. (2012) 13:517–27. doi: 10.1021/bm2015834
121. Hirakura T, Yasugi K, Nemoto T, Sato M, Shimoboji T, Aso Y, et al. Hybrid hyaluronan hydrogel encapsulating nanogel as a protein nanocarrier: new system for sustained delivery of protein with a chaperone-like function. J Control Release. (2010) 142:483–9. doi: 10.1016/j.jconrel.2009.11.023
122. Chang D, Park K, Famili A. Hydrogels for sustained delivery of biologics to the back of the eye. Drug Discov Today. (2019) 24:1470–82. doi: 10.1016/j.drudis.2019.05.037
123. Kang JY, Eggert M, Mouli S, Aljuffali I, Fu X, Nie B, et al. Pharmacokinetics, antitumor and cardioprotective effects of liposome-encapsulated phenylaminoethyl selenide in human prostate cancer rodent models. Pharm Res. (2015) 32:852–62. doi: 10.1007/s11095-014-1501-5
124. Huang WC, Deng B, Lin C, Carter KA, Geng J, Razi A, et al. A malaria vaccine adjuvant based on recombinant antigen binding to liposomes. Nat Nanotechnol. (2018) 13:1174–81. doi: 10.1038/s41565-018-0271-3
125. Nsairat H, Khater D, Sayed U, Odeh F, Al Bawab A, Alshaer W, et al. Liposomes: structure, composition, types, and clinical applications. Heliyon. (2022) 8:e09394. doi: 10.1016/j.heliyon.2022.e09394
126. Mirchandani Y, Patravale VB, Brijesh S. Solid lipid nanoparticles for hydrophilic drugs. J Control Release. (2021) 335:457–64. doi: 10.1016/j.jconrel.2021.05.032
127. Nahum V, Domb AJ. Solid lipid microspheres decorated nanoparticles as drug carriers. Int J Pharm. (2022) 621:121797. doi: 10.1016/j.ijpharm.2022.121797
128. Salawi A. Self-emulsifying drug delivery systems: a novel approach to deliver drugs. Drug Deliv. (2022) 29:1811–23. doi: 10.1080/10717544.2022.2083724
129. Haddadzadegan S, Dorkoosh F, Bernkop-Schnurch A. Oral delivery of therapeutic peptides and proteins: technology landscape of lipid-based nanocarriers. Adv Drug Deliv Rev. (2022) 182:114097. doi: 10.1016/j.addr.2021.114097
130. Tran H. ElSayed ME. Progress and limitations of oral peptide delivery as a potentially transformative therapy. Expert Opin Drug Deliv. (2022) 19:163–78. doi: 10.1080/17425247.2022.2051476
131. Qiao R. Functional polymeric nanoparticles for drug delivery. Curr Pharm Design. (2022) 28:339–339. doi: 10.2174/138161282805220111142951
132. Dizaj SM, Salatin S, Khezri K, Lee JY, Lotfipour F. Targeting multidrug resistance with antimicrobial peptide-decorated nanoparticles and polymers. Front Microbiol. (2022) 13:831655. doi: 10.3389/fmicb.2022.831655
133. de Lima PHC, Tavares AA, de Lima Silva SM, de Moura MR, Aouada FA, Grillo R. Recent advances on nanohybrid systems constituting clay-chitosan with organic molecules-a review. Appl Clay Sci. (2022) 226:106548. doi: 10.1016/j.clay.2022.106548
134. Kumar S, Dhiman R, Prudencio CR, da Costa AC, Vibhuti A, Leal E, et al. Chitosan: applications in drug delivery system. Mini Rev Med Chem. (2022) 22. doi: 10.2174/1389557522666220609102010 [Online ahead of print].
135. Yu F, Zhu Y, Shang X, Yuan H, Hu F. Rationally-designed chitosan-based polymeric nanomaterials according to intrinsic characteristics for cancer therapy and theranostics: a review. Curr Med Chem. (2022) 30. doi: 10.2174/0929867329666220620164429 [Online ahead of print].
136. Yang D. Recent advances in hydrogels. Chem Mater. (2022) 34:1987–9. doi: 10.1021/acs.chemmater.2c00188
137. Huang S, Hong X, Zhao M, Liu N, Liu H, Zhao J, et al. Nanocomposite hydrogels for biomedical applications. Bioeng Transl Med. (2022) 7:e10315. doi: 10.1002/btm2.10315
138. Abune L, Wang Y. Affinity hydrogels for protein delivery. Trends Pharmacol Sci. (2021) 42:300–12. doi: 10.1016/j.tips.2021.01.005
139. Limasale YDP, Atallah P, Werner C, Freudenberg U, Zimmermann R. Tuning the local availability of VEGF within glycosaminoglycan-based hydrogels to modulate vascular endothelial cell morphogenesis. Adv Funct Mater. (2020) 30:2000068. doi: 10.1002/adfm.202000068
Keywords: bioactive peptide, delivery system, nanoparticle, peptide drug, bioavailability
Citation: Zhang X, Li X, Zhao Y, Zheng Q, Wu Q and Yu Y (2022) Nanocarrier system: An emerging strategy for bioactive peptide delivery. Front. Nutr. 9:1050647. doi: 10.3389/fnut.2022.1050647
Received: 22 September 2022; Accepted: 08 November 2022;
Published: 05 December 2022.
Edited by:
Mingqian Tan, Dalian Polytechnic University, ChinaReviewed by:
Jamshidkhan Chamani, Islamic Azad University of Mashhad, IranSutapa Biswas Majee, NSHM Knowledge Campus, India
Eleonore Fröhlich, Medical University of Graz, Austria
Copyright © 2022 Zhang, Li, Zhao, Zheng, Wu and Yu. This is an open-access article distributed under the terms of the Creative Commons Attribution License (CC BY). The use, distribution or reproduction in other forums is permitted, provided the original author(s) and the copyright owner(s) are credited and that the original publication in this journal is cited, in accordance with accepted academic practice. No use, distribution or reproduction is permitted which does not comply with these terms.
*Correspondence: Qiang Wu, cWlhbmd3dXNtaWxlJiN4MDAwNDA7MTYzLmNvbQ==; Yougui Yu, eXVmbHkyMjUmI3gwMDA0MDsxNjMuY29t