- 1Center of Drug Metabolism and Pharmacokinetics, China Pharmaceutical University, Nanjing, China
- 2Nutrilite Health Institute, Shanghai, China
Vitamin B consists of a group of water-soluble micronutrients that are mainly derived from the daily diet. They serve as cofactors, mediating multiple metabolic pathways in humans. As an integrated part of human health, gut microbiota could produce, consume, and even compete for vitamin B with the host. The interplay between gut microbiota and the host might be a crucial factor affecting the absorbing processes of vitamin B. On the other hand, vitamin B supplementation or deficiency might impact the growth of specific bacteria, resulting in changes in the composition and function of gut microbiota. Together, the interplay between vitamin B and gut microbiota might systemically contribute to human health. In this review, we summarized the interactions between vitamin B and gut microbiota and tried to reveal the underlying mechanism so that we can have a better understanding of its role in human health.
Introduction
Vitamin B is a group of water-soluble essential nutrients that serve as precursors of essential cofactors in numerous metabolic pathways. Vitamin B deficiency would cause a series of diseases including cognitive dysfunction, neuropathy, cardiovascular disease (CVD), and osteoporosis (1–7). Humans cannot synthesize these vitamins de novo, so vitamin B is mainly supplied through diet and, to a lesser extent, synthesized by gut microbiota. With the assistance of specific transporters, dietary vitamin B is primarily absorbed in the small intestine while the vitamin B synthesized by gut microbiota is absorbed in the colon, where microbes are densely populated.
As the most dominant member of the gut microbiota, gut bacteria could interfere with the utilization of vitamin B either directly or indirectly (8). Gut bacteria that are capable of synthesizing and supplying an excessive amount of vitamin B for the host, as well as other intestinal microbes are defined as vitamin B-producers. On the other hand, bacteria that can’t produce vitamin B but require vitamin B to maintain normal physiological functions are called vitamin B-consumers. The balance between vitamin B-producers and -consumers determines the role of gut microbiota, whether as suppliers or competitors, to the host. Gut microbiota could indirectly affect vitamin B utilization by interfering with the nutrient absorption process, which is composed of the release of vitamin B from food and its transport across the intestinal epithelial layer (9). Several physiological factors jointly determine the absorption process of vitamin B: digestive enzymes, gut motility, acidity of gastrointestinal tract, the transporters, and the bound proteins. These factors are largely affected by disease progress of inflammatory bowel diseases (IBD), which could be triggered by invasion of the adherent pathogenic microbiota (10–12). In contrast, probiotics could prevent or alleviate IBD, resulting in a normalized physiological feature of the gut (13, 14). Hence, gut microbiota’s bidirectional effects on gut might influence the absorption process of vitamin B.
Moreover, vitamin B supplementation could change the profiles of gut microbiota, including the diversity, abundance, and function. Considering the crucial role of gut microbiota on human health, disruptions of gut microbiota are associated with multiple disease progressions, such as neurological disorders, CVD, obesity, metabolic diseases, and non-alcoholic liver disease (15–22). Since many bacterial functions originate from their metabolites, a variation in the production of metabolites caused by vitamin B could modulate host health. For now, short-chain fatty acids (SCFAs) are the most well-studied small-molecule metabolites of gut microbiota linking vitamin B nutrition to the maintenance of the host’s intestinal homeostasis and benefits of extra-intestinal organs (23). Other metabolites, however, due to limited published knowledge concerning vitamin B’s influences on them, would not be discussed in the current review.
The potential interaction between vitamin B and gut microbiota has raised the interest to study the relationship between vitamin B and gut microbiota. Recently, excellent review papers have been published to reveal the role of vitamin B on gut microbiota, how gut microbiota affect the absorption of vitamin B remains unclear (24, 25). To establish the relationship between vitamin B and gut microbiota, it is required to understand not only the role of gut microbiota on vitamin B absorption, but also the influence of gut microbiota on vitamin B absorption. The purpose of this review is to summarize the role gut microbiota on vitamin B absorption and the indirectly beneficial role of vitamin B on human health via gut microbiota.
Vitamin B1
Vitamin B1 and human health
Vitamin B1, also known as thiamine monochloride, thiamine chloride, and aneurine, is a thermal unstable water-soluble essential vitamin. Thiamine is essential for all organisms to metabolize carbohydrates and branched-chain amino acids through glycolysis and the tricarboxylic acid cycle (26). Dietary vitamin B1 occurs to be thiamin pyrophosphate (TPP), which is catalyzed by thiamine pyrophosphate kinase in the presence of ATP to produce thiamine. Vitamin B1 performs as a cofactor of transketolase in the pentose phosphate pathway and pyruvate dehydrogenase and α-ketoglutarate dehydrogenase in the mitochondria (27). The World Health Organization/Food and Agriculture Organization recommends a daily intake of 1.1–1.2 mg of vitamin B1 for adults (28). Vitamin B1 deficiency can induce drowsiness, beriberi, polyneuritis, and Wernicke–Korsakoff syndrome (WKS). Thiamine administration reduces the progression of neurological deficits caused by WKS (29). Nutritional intervention for the treatment of hepatic encephalopathy includes adequate, but not excessive, vitamin B1 supplementation (30).
Dietary vitamin B1 release and absorption
Dietary vitamin B1 is primarily absorbed in the small intestine, while those vitamin B1 produced by intestinal microbes are mainly absorbed in the large intestine (Figure 1; 27). Human beings are not able to store free thiamine, and only small amounts of phosphorylated thiamine exist in cells. Therefore, a continuous supply of dietary vitamin B1 is required. Most dietary thiamine is present in phosphorylated forms, which are subsequently hydrolyzed by intestinal alkaline phosphatase to free thiamine. Studies have suggested that the proximal small intestine is the primary site for the absorption of free thiamine (31). Thiamine absorption relies on both unsaturated passive diffusion and saturated active transport (31, 32). In the case of an oral dose below 5 mg, free thiamine is mainly absorbed by the intestinal epithelium through thiamine transporters (THTR-1 and THTR-2, encoded by genes SLC19A2 and SLC19A3) (33). Similarly, microbiota-produced vitamin B1 is absorbed either identically with to the dietary origin or directly by the colon through the TPP transporter (encoding gene SLC44A4) (34).
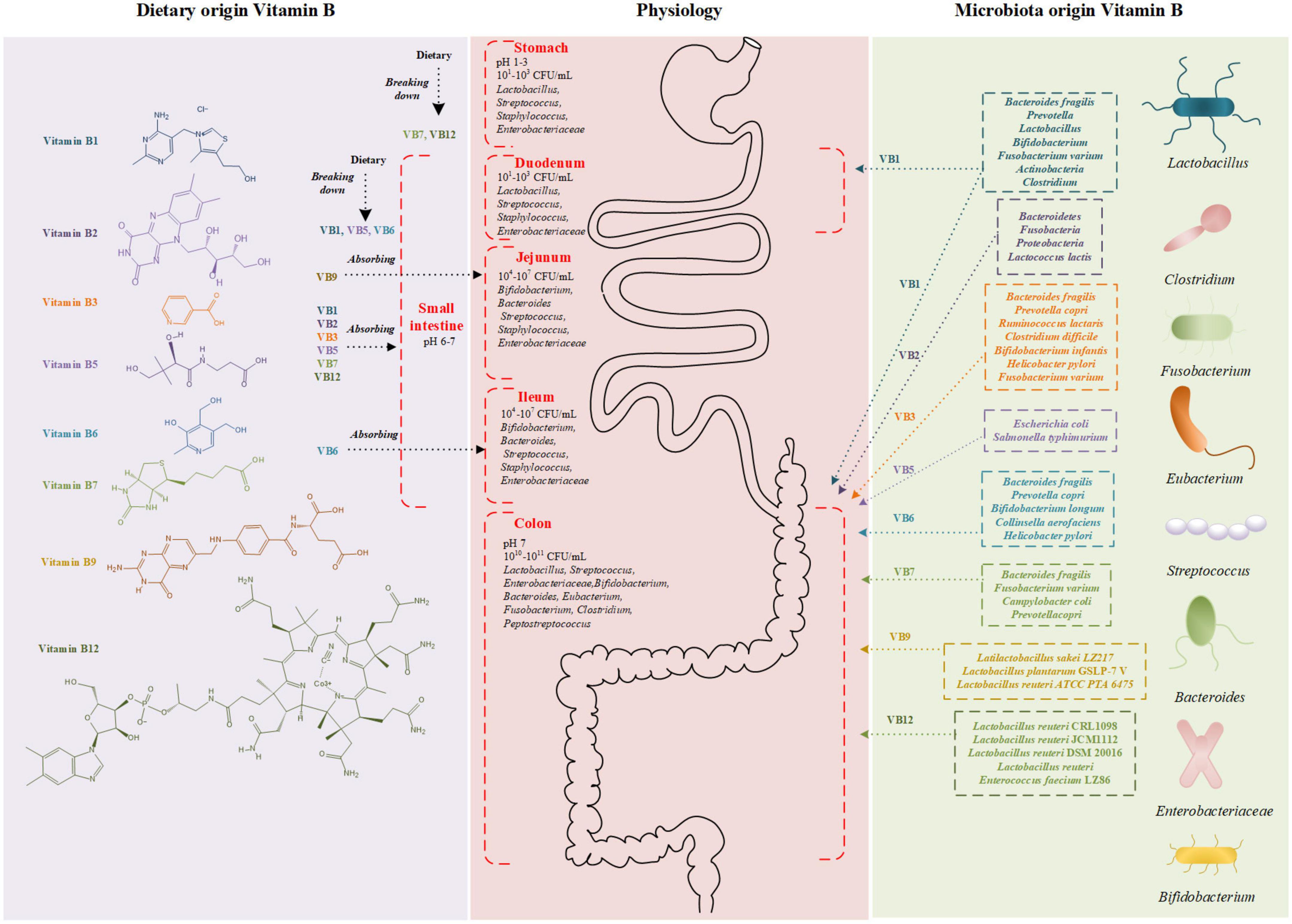
Figure 1. Schematic diagram of vitamin B structure and absorption sites. Vitamin B derived from diet (left) and microbiota (right) are absorbed in gastrointestinal tract (middle). The different colors represent bacteria producing different vitamin B. CFU, colony forming unit.
Influence of gut microbiota on vitamin B1 utilization
Bacteroidetes and Fusobacteria are the most common two bacterial phyla that are able to synthesize TPP (35). Some intestinal microbiota can produce vitamin B1, such as Bacteroides fragilis, Prevotella, Fusobacterium varium, Actinobacteria, and Clostridium (35–39). About half number of enzymes presented in these microbiotas take part in the de novo synthesis of thiamine (40). However, there also remains gut microbiota in which vitamin B1 synthesis pathway is absent and external source is required for their growth, such as Ruminococcaceae (41). For these bacteria, competing with the host for vitamin B1 is a potential approach for survival, especially in the small intestine.
The gut microbiota may have impact on gastrointestinal functions, therefore affecting the absorption of vitamin B1. Clostridium botulinum isolated from the feces of botulism infant can produce thiaminase I to degrade vitamin B1 (42). According to in vitro study on Caco-2 cells, thiamin uptake was significantly reduced by Enterotoxigenic Escherichia coli infection, which reduces the expression levels of THTR-1 and THTR-2 (43, 44). Transcellular H+/OH– gradient has been proved to be the driver of THTR-1 and THTR-2 dependent thiamine transport (33). In addition, vitamin B1 is easy to be oxidized and lose activity in neutral and especially alkaline environment. Lactic acid bacteria can produce acids such as Bifidobacterium, Lactobacillus, Enterococcus, and Streptococcus in the intestinal tract (45, 46). The growth of these microbiota is then deduced to improve the absorption of thiamine via their regulation of intestinal pH. In the study of Ibrahim Elmadfa et al., the probiotic yogurt microbiota including Lactobacillus casei GG could reduce vitamin B1 bioavailability in humans (47). In contrast, yogurt containing Lactobacillus. exhibited an oppositive effect on plasma vitamin B1 levels in healthy adult humans, and this observation might be attributable to the complex interactions among vitamin B1, gut microbiota, and host (48). Thus, further studies are required to reveal these complex interactions.
Indirect role of vitamin B1 on human health as mediated by gut microbiota
Vitamin B1 is essential to the growth of microorganisms, therefore changing the form of gut microbiota. In the absence of thiamine, the population of thiamine acquisition mutant strain was rapidly decreased in vitro (40). An initial population of 90% had fallen to 0.1% by day 5. Another study found that the Eubacterium rectale A1-86, Roseburia intestinalis M50/1 were unable to grow in the absence of thiamine even though they have the genes encoding thiamin synthesis (39). By contrast, the growth level was improved when supplied with thiamine. With the increase of exogenous thiamine, the population of thiamine acquisition mutant was increased in vitro (40). The optimal growth was achieved in human intestinal thiamine-nutrient-deficient bacteria, such as Subdoligranulum variabile DSM 15176, E. rectale A1-86, R. intestinalis M50/1, and Roseburia inulinivorans A2-194, when thiamine concentrations were at or above 5 ng/mL (39). The relative abundance is correlated with some intestinal bacteria that require peripheral intake of vitamin B1. A positive correlation was reported between vitamin B1 and the relative abundance of Ruminococcaceae (Firmicutes phylum), which lacks the vitamin B1 synthesis pathway and requires a supply of vitamin B1 from the host diet (41). Supplying thiamine to cows’ diet could increase the abundance of cellulolytic microbiota such as Bacteroides, Ruminococcus 1, Pyramidobacter, Succinivibrio, and Ruminobacter, and enhance ruminal acetic acid production. Their increase enhanced the fiber degradation and ruminal acetate production, thus improving ruminal function (49).
Vitamin B1 has an important influence on SCFAs production by gut microbiota. Faecalibacterium, the most abundant of the rumen cocci family, expresses pyruvate iron oxidoreductase to convert pyruvate to acetyl coenzyme in the butyrate production pathway. During this process, vitamin B1 is required as a coenzyme, emphasizing its importance in butyrate production (41).
Vitamin B2
Vitamin B2 and human health
As the precursor of flavin mononucleotide (FMN) and flavin adenine dinucleotide (FAD), vitamin B2 (Riboflavin) plays a crucial part in multiple biological redox reactions, energy metabolism, antioxidant, and anti-inflammatory, synthesis, and activation of vitamin B6 and vitamin B9 (50–52). Considering the protective role of vitamin B2 in various medical conditions, vitamin B2 deficiency is related to multi-system dysfunction. Lack of vitamin B2 can alter cellular metabolic homeostasis, which leads to night blindness, cataracts, anemia, migraines, and dermatological symptoms (53, 54). Early diagnosis coupled with riboflavin supplementation can heal up ariboflavinosis in an early stage. In addition, a previous study showed that vitamin B2 intake was inversely associated with colorectal cancer and its adequate supply can effectively lower blood pressure in hypertensive patients identified with the MTHFR 677TT genotype (55, 56).
Dietary vitamin B2 release and absorption
Dairy products, green vegetables, fruits, eggs, and meat are excellent sources of vitamin B2 (57). The absorption of vitamin B2 depends on the riboflavin hydrolyzed from FMN and FAD by alkaline phosphatases and FMN/FAD pyrophosphatases (Figure 1; 58, 59). Enterocytes actively involves in the absorption of vitamin B2 through a carrier-mediated process, contributing up to 30 mg of riboflavin at each meal (57). Following cellular uptake, riboflavin may generate FMN by ATP-dependent phosphorylation, and most FMN is further converted to FAD. Vitamin B2 can be absorbed through the basolateral membrane of the enterocytes in the form of free riboflavin or FMN, and then released into the portal blood toward the liver (60).
Influence of gut microbiota on vitamin B2 utilization
De novo synthesis pathway of vitamin B2 was found in nearly all genomes of Bacteroidetes, Fusobacteria, and Proteobacteria. It is estimated that 65% of bacterial genomes (a total number of 256 genomes), can produce vitamin B2 according to a systematic genome assessment of B-vitamin biosynthesis (35). Interestingly, Clostridium acetobutylicum, Eremothecium ashbyii, Ashbya gossypii, Bacillus subtilis, and many other strains have been industrialized to produce vitamin B2 (61). Vitamin B2 produced by gut microbiota is mainly adsorbed in the colon, serving as an additional source of daily vitamin B2 uptake other than dietary supply (59). Over-expression of riboflavin biosynthesis genes in Lactococcus lactis could switch its role from a consumer into a producer of vitamin B2. Compared with the native strain, administration of L. lactis modified to produce riboflavin in riboflavin-deficiency rats could improve their riboflavin status, suggesting its importance on maintaining the state of vitamin B2 (62).
Gut microbiota may change the physiological or pathological conditions of the gastrointestinal tract, and then influence the absorption of vitamin B2. The decreased gastric emptying rate has been reported to improve the bioavailability of vitamin B2 (63). Lactobacillus reuteri and Lactobacillus gasseri OLL2716were able to slow down the gastric emptying rate. The phenomenon indicated that these species of bacterial have a potential influence on vitamin B2 absorption (64, 65). On the other hand, several bacteria could intervene the gastric disease progression. For example, a lower plasma level of vitamin B2 was observed in gastric cancer patients with the presence of Helicobater pylori than in patients without infections (66). Meanwhile, lactic acid bacteria, as complementary treatments for intestinal inflammation, have been proved to be effective in the suppression or prevention of Helicobater pylori infection (67). These results indicate that gut microbiota may affect the absorption of vitamin B2 by the way of changing rate of gastric emptying (48).
Indirect role of vitamin B2 on human health as mediated by gut microbiota
The growth of anaerobic bacteria Blautia coccoides, R. intestinalis and Enterococcus faecalis was stimulated by vitamin B2 in vitro (68). Replenishment of vitamin B2 has been reported to alter the composition and β diversity of gut microbiota in mice (69). Colon-targeted vitamin B2 supplementation for 3 weeks improved the α diversity of gut microbiota in healthy volunteers but not in Crohn’s disease patients, suggesting that effects of vitamin B2 on gut microbiota are different between groups of people with different physiology situation microbiota (70–72). In clinical interventions, vitamin B2 supplementation for 2 weeks increased the relative abundance of F. prausnitzii in healthy individuals (73). Moreover, treatment of vitamin B2 and vitamin C significantly reduced the number of Proteobacteria, and showed a trend to increase the number of Firmicutes and decrease the number of Bacteroidetes (70). Another study also reported that the relative abundance of Streptococcus was negatively correlated with dietary intake of vitamin B2 (74). Several groups of Streptococcus are thought to be correlated with strep infection, such as Streptococcus pyogenes and Streptococcus pneumoniae. Consequently, sufficient daily intake of vitamin B2 might protect the host from strep infections. In volunteers with migraine, daily supplementation of vitamin B2 increased the abundance of anaerobic bacteria Roseburia, and F. prausnitzii, while decreased the abundance of E. coli (75).
Vitamin B2 forms part of the electron transfer flavoprotein complex of butyryl-CoA dehydrogenase. Consequently, butyrate production is directly affected by vitamin B2 (39). Concentration of SCFAs produced by human gut isolates rely upon vitamin B2 in in vitro condition (39). Furthermore, cecal SCFAs content of vitamin B2 depletion-repletion mice model was remarkably increased by the repletion of vitamin B2 (69). Faecalibacterium prausnitzii is an effective producer of SCFA, especially butyrate, and a 2-week vitamin B2 supplementation in healthy individuals could increase the abundance of Facealibacterium. prausnitzii, which is an effective producer of SCFAs, especially butyrate (39, 75, 76). A randomized trial showed that butyrate production was significantly increased in groups treated with 50 and 100 mg/d of vitamin B2 (73).
Vitamin B3
Vitamin B3 and human health
Nicotinamide (NAM) and nicotinic acid (NA) are collectively referred to as vitamin B3 (77). The functional cofactors derived from vitamin B3 are nicotinamide adenine dinucleotide and nicotinamide adenine dinucleotide phosphate, providing reducing equivalents for cellular biochemistry and energy metabolism (78, 79). Vitamin B3 is considered as a potent antioxidant capable of protecting the brain’s cellular membranes and is deemed to be effective in neurodegenerative diseases (80). Aging-related nicotinamide adenine dinucleotide deficiency in the retina is considered as a possible reason for glaucoma in the elderly, and oral administration of vitamin B3 could alleviate the symptoms (81). The most typical disease caused by vitamin B3 deficiency is pellagra, which is characterized by inflammation of mucous membranes, skin lesions, diarrhea, and dementia. Besides, NA is widely used to treat lipid disorders (82).
Dietary vitamin B3 release and absorption
Vitamin B3 can be obtained from both endogenous and exogenous sources. The endogenous source is produced from tryptophan, and the exogenous source is dietary NA and NAM (Figure 1) (83). NAM mainly exists in animal products, while NA mainly exists in plant-based foods such as beans. With human-derived intestinal epithelial Caco-2 cells and purified isolated brush-border membrane vesicles, a study on the mechanism of NA uptake in humans suggested the existence of an acidic pH-dependent and carrier-mediated mechanism in the physiological range of niacin concentrations (84). Based on the environmental acidity, NA is absorbed in the stomach and the upper part of small intestine by proton co-transporters and an anion antiporter (84–86). Besides, it has also been reported that vitamin B3 could be absorbed by passive diffusion at higher concentrations (87).
Influence of gut microbiota on vitamin B3 utilization
Several gut microbial species possess vitamin B3 biosynthesis pathways and can synthesize vitamin B3 from tryptophan (83). Bacteria with vitamin B3 biosynthesis pathway include B. fragilis, Prevotella copri, Ruminocccus lactaris, Clostridium difficile, Bifidobacterium infantis, H. pylori, and F. varium (35, 88). Nabokina et al. reported that NA uptake by Caco-2 cells increased when the extracellular pH reduced from 8.0 to 5.0 (84). Considering that the absorption of vitamin B3 is pH-dependent, we can deduce that lactic acid bacteria, such as Bifidobacterium, Lactobacillus, Enterococcus, and Streptococcus, can change the intestinal acidity and influence the absorption of NA.
Indirect role of vitamin B3 on human health as mediated by gut microbiota
For now, the role of vitamin B3 on gut microbiota remains unclear. Fangmann et al. found a reduced α diversity and a decreased abundance of Bacteroidetes in the microbiome of obese subjects under a low dietary NA intake (89). For this reason, delayed-release microcapsules targeting the ileocolonic region were developed to deliver vitamin B3 to the microbiome, avoiding the adverse side effects of NA. Such vitamin B3 containing microcapsules significantly increased the abundance of Bacteroidetes in human gut (89). Another study has also shown that NA plays an essential role in maintaining normal intestinal homeostasis, reducing intestinal inflammation, and regulating the production level of intestinal antimicrobial peptides (90). The supplementation of NA was also reported to increase the production of acetate while reducing the ratios of propionate/acetate and butyrate/acetate in the colonic contents of piglets, suggesting a beneficial effect of NA on SCFAs production (91).
Vitamin B5
Vitamin B5 and human health
Vitamin B5, also known as pantothenic acid, is available in a variety of plants and animal products and unprocessed grains (92). Food-derived vitamin B5 is converted to pantethine and then to Acetyl CoA and acyl carrier protein (93). These two compounds are critical in burning fats and carbohydrates in energy metabolism. Acetyl CoA is also required to produce adrenal hormones including Cortisol (steroid hormone). Hence, vitamin B5 deficiency is associated with impaired adrenal cortical function (94). Patients with rheumatoid arthritis were observed with lower blood pantothenic acid and the severity of arthritis is negatively correlated to the level of vitamin B5 (95). The most common symptoms associated with vitamin B5 deficiency were headache, fatigue, and a sensation of weakness. Health volunteers fed a semisynthetic, pantothenic acid-free diet for 9 weeks developed subclinical signs of fatigue ad listlessness without clinical symptoms (96). Low serum vitamin B5 level also associates with an increased incidence of hypertension (97).
Dietary vitamin B5 release and absorption
The intestinal tract is exposed to two sources of vitamin B5: diet- and bacteria-origin (98). Dietary vitamin B5 exists mainly in the form of coenzyme A, which is hydrolyzed to pantetheine by alkaline phosphatase and then quickly converted into the absorbable forms of pantothenic acid by pantetheinase in the intestinal lumen (Figure 1; 99). At low luminal concentrations, free pantothenic acid is actively transported via the sodium-dependent multivitamin transporter (SMVT, SLC5A6) (100–103). At higher concentrations, passive diffusion of vitamin B5 occurs, and no significant difference in the transport rate in different segments of the intestine. It is indicated that absorption of the bacterially synthesized vitamin B5 in the large intestine also involves the SMVT system, though direct evidence is lacking (100, 103, 104).
Influence of gut microbiota on vitamin B5 utilization
Vitamin B5-producing bacteria and vitamin B5-consuming bacteria coexist in the intestinal tract (25). Vitamin B5-producing bacteria include E. coli and Salmonella typhimurium. Escherichia coli can utilize aspartate and intermediate metabolites of valine biosynthesis as substrate (24, 105–107). Salmonella typhimurium produces pantothenate from alpha-ketoisovalerate using acetohydroxy acid synthase isozyme I and dihydroxy acid dehydratase enzymes (108). Other bacteria, such as Lactobacillus helveticus, Streptococcus, and E. faecalis, members of the vitamin B5-non-producing Firmicutes phylum, require vitamin B5 for their growth in vitro, which might compete vitamin B5 with the host (109, 110).
For now, the regulatory effect of gut microbiota on vitamin B5 absorption is still unclear. However, extensive evidence supports that utilization of bacterially synthesized vitamin B5 depends on the gut microbiota, especially in vitamin B5 deficiency. Chemicals containing sulfonamide functional group, including succinylsulfathiazole and sulfathiazole, can change the composition and function of the gut microbiota (111, 112). It was reported that mice consuming the vitamin B5-deficient diet and receiving succinylsulfathiazole exhibit signs of pantothenic acid deficiency (111). A similar phenomenon has also been observed in rats (112). Moreover, the addition of succinylsulfathiazole to a vitamin B5-normal diet deteriorated the deficiency of pantothenic acid, resulting in signs of pantothenic acid deficiency characterized by achromotrichia and porphyrin-caked whiskers appearing in 3 weeks.
Indirect role of vitamin B5 on human health as mediated by gut microbiota
At present, little is known about the role of dietary vitamin B5 supplementation on gut microbiota. Enhanced vitamin B5 intake appears to increase the relative abundance of Prevotella and Actinobacteria and to decrease the abundance of Bacteroides in lactating women (113). Non-linear effects of vitamin B5 on the diversity and abundance of intestinal microbiota were observed. In fish, a diet supplemented with 26.0 mg/kg of vitamin B5 increased the diversity and abundance of intestinal microbiota compared with other levels of vitamin B5 supplementation (114). The maximum portion of Proteobacteria, Firmicutes, and Mycoplasma appeared in the 20.0 mg/kg, 26.0 mg/kg, and the no-vitamin B5-supplement group, respectively. The minimum portion of Proteobacteria, Firmicutes, and Tenericutes appeared in the 37.0, 20.0, and 26.0 mg/kg groups. Among the investigated bacterial species, Mycoplasma is suggested to be correlated with infectious disease pathogenesis (115, 116). It can be inferred from the experimental results that vitamin B5-supplement could inhibit the number of Mycoplasma thus increasing the anti-infection abilities. An in vitro study of L. helveticus demonstrated that a vitamin B5-deficient medium greatly inhibited the synthesis of fatty acid and protein, and this observation is likely to be explained by the downregulated expression of genes associated with fatty acid synthesis and biotin metabolism (117). Together, these results indicate that deficiency of vitamin B5 might change the growth profile and the biological function of intestinal microbiota.
Vitamin B6
Vitamin B6 and human health
Vitamin B6 is a group of compounds with a defined structure, including pyridoxine (PN), pyridoxal, and pyridoxamine, and all of them have 5-position phosphoryl derivatives (118). All these compounds cannot be synthesized by the human body and are mainly provided by diet (119). Animal products mostly contain pyridoxal 5’-phosphate (PLP) and pyridoxamine 5’-phosphate, while pyridoxine 5’-phosphate (PNP) is the dominant form in plant products (119–122). The recommended daily intake amount is 1.3∼1.7 mg for adults (123). Vitamin B6 is a cofactor in many metabolic reactions that include amino acid metabolism, biosynthesis, and degradation of sphingolipid and carbohydrate metabolism (120, 124, 125). Moreover, PLP is the primary active form among all forms of these above derivatives. It acts as a cofactor for many enzymes, such as tryptophan synthase (126), O-acetylserine sulfhydrylase (127), 5-Aminolevulinate synthase (128), serine hydroxymethyltransferase (129), and branched-chain amino acid aminotransferase (130). Vitamin B6 deficiency may cause neuromuscular irritability, peripheral neuropathy, dermatitis, stomatitis, cheilosis, depression of the immune system, and sideroblastic anemia (131). Moreover, vitamin B6 has also shown its importance in maintaining a regular function of cognition and keeping the elderly away from CVD (132, 133). Additionally, supplementation of vitamin B6 could protect against reactive oxygen free radicals (132, 134, 135).
Dietary vitamin B6 release and absorption
The phosphorylated form of vitamin B6 in the diet is hydrolyzed by pyridoxal phosphatase and then absorbed in the intestinal lumen (Figure 1; 136). Many studies demonstrate that, under normal circumstances, the concentration of vitamin B6 in food is not saturable and vitamin B6 enters intestinal cells by passive diffusion (131). However, a study with Caco-2 cells has recently challenged this concept. It gives clear evidence for a specialized, carrier-mediated system for the uptake of PN (120). Another study finds that the unique carrier-mediated mechanism for PN uptake functional exists in mammalian colonocytes (124). And these results indicate that the uptake of vitamin B6 could be affected by several extracellular and intracellular factors (124). As an instance, Said et al. demonstrated a pH-dependent and amiloride-sensitive system involving PN uptake in intestinal epithelial cells (120).
Influence of gut microbiota on vitamin B6 utilization
For humans, there are two primary sources of vitamin B6, one is from the diet, and the other is produced by normal gut microbiota (124). For example, B. fragilis and P. copri (Bacteroidetes), Bifidobacterium longum and Collinsella aerofaciens (Actinobacteria) and H. pylori (Proteobacteria), and these species of bacteria can produce vitamin B6 (35). Furthermore, many studies have shown that there are two biosynthetic pathways, the deoxyxylulose 5-phosphate (DPX)-dependent pathway for PLP and the DPX-independent pathways for PNP (137), and different gut microflora decide on different pathways to choose (35).
Under normal circumstances, dietary and bacterial sources can offer enough vitamin B6 for the human body to absorb, and deficiency of vitamin B6 is a rare phenomenon (114). However, some researchers reported that drugs, alcohol, and smoking, deoxyxylulose 5-phosphate (DPX) (138). Furthermore, these factors above could change gastrointestinal motility, which might bring about a narrowed absorption window and a reduced bioavailability for vitamin B6 (139, 140). Moreover, such intestinal environmental factors could change gut microbiota composition (141–144). Ferrer et al. found that gut microbiota in the lean gut seems to get more involved with vitamin B6 biosynthesis and offer more vitamin B6 for absorption (145, 146). At the same time, the hydrolysis of vitamin B6 is a significant procedure for absorbing vitamin B6 and is closely related to the pH of the intestinal lumen. Since some species of lactic acid bacteria, including Bifidobacterium, Lactobacillus, Enterococcus, and Streptococcus (45–48), can produce acid to lower the pH, we may infer that variation in the proportion of these specific bacterial can influence vitamin B6. Meanwhile, alkaline phosphatase is the essential enzyme during this process. It is also able to affect the growth of gut microbiota (147). The intervention between alkaline phosphatase and gut microbiota is complicated, and it is essential to figure out its influence on vitamin B6 absorption. Unfortunately, there is not much investigation about it.
Indirect role of vitamin B6 on human health as mediated by gut microbiota
In the intestine, vitamin B6 serves as essential nutrients for the gut microbiota (25). Some species of microbiota lack the ability to biosynthesize vitamin B6, such as most genera within the Firmicutes phylum (Veillonella, Ruminococcus, Faecalibacterium, and Lactobacillus spp.) (35). Hence, they acquire exogenous vitamin B6 from the intestinal tract to maintain their life activities. The proportion of food compositing vitamin B6 can influence the profile of the gut microbiota. For example, a larger amount of vitamin B6 absorbed from food was associated with greater richness and evenness of the gut microbiota (124). Mayengbam et al. investigate the composition of gut microbiota and their metabolites in a rat model with vitamin B6 deficiency. They reported that arginine biosynthesis was impaired in this rat model, and the vitamin B6 metabolism was affected. Arginine not only performs as a substrate for protein synthesis but also as a precursor for a variety of molecules linked to cell function, such as nitric oxide. Insufficient vitamin B6 might interferes the host’s de novo protein synthesis and related cell functions. Vitamin B6 produced by microbiota is not enough for gut microbiota, and the composition of gut microbiota is changed for this reason (148).
Vitamin B7
Vitamin B7 and human health
Vitamin B7, also known as biotin, acts as a cofactor for multiple carboxylases that are associated with fatty acid, glucose, and amino acid metabolism (149). Vitamin B7 is exclusively synthesized by plants and microbiota such as bacteria and yeast, so vitamin B7 synthesized by microbiota in human large intestine make an important contribution to daily supplements for human in addition to food. The adequate daily requirement of vitamin B7 is 35 μg for infants and 150–300 μg for adults. And it is relatively non-toxic even at doses of greater than 60 mg/day for several months (149). Vitamin B7 participates in normal immune function, maintaining the integrity of the intestinal mucosa or homeostasis. It also plays an important role in maintaining skin health and anti-inflammation via inhibiting NF-κB activation (101, 150). Therefore, severe vitamin B7 deficiency results in skin abnormalities, neurological disturbances, and growth retardation. Symptoms of vitamin B7 deficiency contain inflammation, loss of appetite, glossitis, dandruff dermatitis, and hair removal. Hence, therapeutical vitamin B7 supplementation can improve hair loss and prevent not only seborrheic hair loss, but also juvenile gray hair in the pathological case of biotin deficiency (151). Although neuroleptic effect of vitamin B7 has not been proven, it does demonstrate a beneficial effect on treatment of depression and insomnia (139).
Dietary vitamin B7 release and absorption
Humans are exposed to two sources of vitamin B7, including dietary sources and bacterial source in the large intestine (9). Dietary vitamin B7 exists in either free form or protein-bound form. Ingested protein-bound forms of vitamin B7 are firstly broken down by gastrointestinal proteases and peptidases to biocytin (biotinyl-L-lysine) and biotin-oligopeptides (Figure 1). These products are further processed in the intestinal lumen to release free biotin before absorption. Free biotin from the gastrointestinal tract is rapidly absorbed. Absorption of free biotin by the proximal intestine is mediated by SMVT, which also transports vitamin B5 and antioxidant lipoates (136, 142). In the intestine, SMVT is exclusively expressed on the apical membrane of polarized intestinal absorptive cells and thus SMVT system is the only biotin uptake system in the mammalian gut (104).
Influence of gut microbiota on vitamin B7 utilization
It has long been recognized that the normal microbiota of large intestine can synthesize large amounts of biotin. Vitamin B7-producing microbiota includes B. fragilis, F. varium, and Campylobacter coli (24). Meanwhile, vitamin B7-consuming bacteria must gain vitamin B7 from the environment to maintain microbial functions and these bacteria lack the vitamin B7 biosynthetic pathways. For instance, Lactobacillus possesses genes involved in obtaining biotin from environments (145).
Human body lacks the capacity to produce vitamin B7, for which vitamin B7 is mainly supplied by the jejuna and to a lesser extent, from the distal gut. However, absence of gut microbiota may negatively affect circulating vitamin B7 levels. In rodent model, enhanced vitamin B7 transport was observed in decreased intestinal pH (152). Since lactic acid bacteria such as Bifidobacterium, Lactobacillus, Enterococcus, and Streptococcus can produce lactic acid and lower the local acidity in the intestinal lumen (45, 46), it is suggested that supplementation of lactic acid bacteria might increase the absorption of vitamin B7. Rat intestinal infection of Salmonella enterica serotype Salmonella typhi results in a significant reduction in intestinal vitamin B7 intake (150). Obese mice induced by a high-fat diet demonstrate a changed gut microbiota profile, resulting in fewer microbes expressing genes for vitamin B7 synthesis (153). As a result, a reduction in vitamin B7 synthesis and lowered plasma vitamin B7 levels in obese mice were observed, indicating the significant importance of intestinal microbiota in maintaining vitamin B7 levels in obesity (153).
Indirect role of vitamin B7 on human health as mediated by gut microbiota
The composition of gut microbiota may be influenced by vitamin B7. Vitamin B7-consuming bacteria with free biotin transporter, including Prevotella, Bifidobacteria, Ruminococcus, and Lactobacillus, require vitamin B7 to maintain their microbiological functions (154). Hence, vitamin B7 deficiency might interfere with the abundance of the above bacteria. For instance, deprivation of vitamin B7 has been reported to cause intestinal dysregulation and overgrowth of Lactobacillus murine (155).
Vitamin B9
Vitamin B9 and human health
Vitamin B9 (folate) is a micronutrient for the synthesis and functional regulation of many biomacromolecules in humans (156, 157). In fortified foods, supplements, and pharmaceuticals, vitamin B9 occurs in the synthetic form of folic acid (158). As a critical cofactor in one-carbon metabolism, vitamin B9 could transfer carbon units in methylation reaction, DNA and RNA biosynthesis, and amino acid metabolism (159). Megaloblastic anemia is one of the most common symptoms of vitamin B9 deficiency. The main reason for this disease is the inhibition of the maturation of erythropoietic precursors (159, 160). Lack of vitamin B9 also correlates with neural tube defects (161). Furthermore, vitamin B9 inadequacy is associated with the pathogenesis of several chronic diseases including CVD, cancers (colorectal, prostate, and breast cancer) (162–164), and Alzheimer’s disease (160, 165, 166).
Dietary vitamin B9 release and absorption
Natural folate and folic acid have similar absorption processes (158). In food, vitamin B9 usually occurs as folate polyglutamate, which is hydrolyzed to the monoglutamate form by glutamate carboxypeptidase II before the absorption in the brush border of the proximal part of the jejunum (Figure 1; 167). Monoglutamate folate can be transported by the proton-coupled folate transporter (PCFT) across the apical membrane of enterocytes (168, 169). After vitamin B9 is metabolized to 5-methyl-tetrahydrofolate in the enterocytes by dihydrofolate reductase, it can be transported by multidrug resistance-associated protein (MRP) into the portal vein. Vitamin B9 could experience enterohepatic circulation, which means it can be discharged into the bile and then reabsorbed in the intestine. Bacteria synthesized folate might be absorbed in the colon because the colon has abundant PCFTs for vitamin B9 absorption (170, 171). Moreover, the enzymes that favor the absorption of vitamin B9, such as folate hydrolase, γ-glutamyl hydrolase, and folate hydrolase 2, are also highly expressed in the colon (172, 173).
Influence of gut microbiota on vitamin B9 utilization
The gut microbiota also plays a valuable role in producing and consuming vitamin B9 (172, 174). According to an evaluation of human gastrointestinal bacterial genomes, 13.3% of the bacteria possess the ability of vitamin B9 de novo synthesis, and 39% could produce vitamin B9 with extra para-aminobenzoic acid provided from other bacteria or food (172). It is also reported that 26% of Actinobacteria, 71% of Proteobacteria, 79% of Fusobacteria, and 15% of Firmicutes in the human gut microbes have the potential to de novo synthesize vitamin B9 (35). A systematic genome evaluation of the vitamin B family suggested human intestinal microorganism is capable of producing 37% of daily required vitamin B9 in non-pregnant adults (35). Folate-producing strains have been extensively screened to fortify vitamin B9 content (175–177). Liu et al., have isolated Latilactobcillus sakei LZ217, a good producer of vitamin B9, from raw milk (176). Zhang et al. screened high vitamin B9-producing strains from 12 lactic acid bacteria and then obtained its variant, Lactobacillus plantarum GSLP-7 V after stressing with drugs (177). Based on a vitamin B9 deficient rat model induced by a vitamin B9-free diet, they further proved GSLP-7 V and its fermented yogurt could restore serum vitamin B9 and homocysteine (Hcy) to normal levels. A case that is closer to clinical application is L. reuteri ATCC PTA 6475. It has been proved to be safe for humans and could produce vitamin B9 with additional para-aminobenzoic acid (172). Collectively, the microbiome has beneficial potential in treating vitamin B9 deficiency. Another study pointed out that 86% of the 512 investigated bacterial reference genomes required vitamin B9 or its intermediates from food or other microbiota (172).
Indirect role of vitamin B9 on human health as mediated by gut microbiota
A vitamin B9-supplement diet slightly increased gut bacterial community richness according to the abundance-based coverage estimator, compared to a vitamin B9-deficient diet in the high-fat-diet-induced obesity mouse model. The relative abundance of Actinobacteria was significantly increased, while it is the opposite for Clostridia (178). However, Wang et al. proved additional folic acid didn’t make significant differences in the indices of diversity in the cecum, but increased relative abundance of Lactobacillus salivarius, L. reuteri, and Lactobacillus mucosae (179). Vitamin B9 deficiency can influence bacterial diversity. Compared to a micronutrient-sufficient diet in gnotobiotic mice, the vitamin B9 deficiency diet increased β diversity after 21-day treatment. But a 14-day full diet treatment did not change this trend (180). Another study based on human gut microbiota assessed fecal microbiota composition. The fecal microbiota community has lower α and β diversity when healthy volunteers with less vitamin B9 diet. And the fecal microbiota community has a higher potential to produce vitamin B9 in vitro experiments (181). It’s suggested that vitamin B9 deficiency probably decreases the richness of human gut microbiota.
Vitamin B9 also can influence the amount of SCFAs in the gastrointestinal tract. In the study of Wang et al., there were more acetic acid and valeric acid in the cecum and colon of weaned piglets fed with vitamin B9 supplementation (179). Liu et al. proved the vitamin B9-produced probiotics, L. sakei LZ217, could increase SCFAs content, especially for propionic acid and butyric acid in the fecal slurry cultures (176).
Vitamin B12
Vitamin B12 and human health
Vitamin B12 (cobalamin) is a member of the corrinoids that is required by methionine synthase and methylmalonyl-CoA mutase (182, 183). Methionine synthase is pivotal in catalyzing the conversion of Hcy to methionine. The subsequently adenosylated of methionine would generate S-adenosylmethionine to supply methyl groups for biological methylation modifications of proteins and nucleic acid. Methylmalonyl-CoA mutase is involved in mitochondrial metabolism. A daily intake of 4 μg is adequate to maintain the normal biological functions of vitamin B12 (184), which could be satisfied by dietary supplementation with 5–30 μg (185). Vitamin B12 deficiency is correlated with several pathological progressions due to its important role in methylation and catabolism. Without sufficient vitamin B12 to convert total homocysteine (tHcy) to methionine, circulating level of accumulated tHcy might increase the risk of CVD (186, 187). Moreover, vitamin B12 deficiency is responsible for cognitive impairment and neurological disorders, which might result from the accumulation of tHcy and methylmalonic acid (188–190). In addition, deficiency of vitamin B12 has also been reported to perform a positive association with osteoporosis (191), macular degeneration (192, 193), and frailty (194).
Dietary vitamin B12 release and absorption
For human, the major source of vitamin B12 is animal products while intestinal microbiota synthesis might also contribute to a minor fraction (195). Vitamin B12 could be absorbed by both passive diffusion and receptor mediated endocytosis in the intestine (Figure 1). Passive diffusion is negligible in physiological doses (100–1000 μg) supplied by food or supplementation (196). The absorption of vitamin B12 by receptor mediated endocytosis is a multistep process (197–199). In the upper gastrointestinal tract, vitamin B12 is released from the protein carriers with the assistance of gastric acid and pepsin and then binds to haptocorrin under the acidic condition. After the degradation of haptocorrin by pancreatic proteases, the released vitamin B12 binds to intrinsic factors in duodenum. The generated vitamin B12-intrinsic factor complex is then endocytosed by mucosal cells in the distal ileum with the help of receptor cubilin, transmembrane protein amnionless, and megalin/LRP2. After entering the mucosal cells, vitamin B12-intrinsic factor complex is dissociated from cubilin in the early endosomal compartment. In the lysosome, intrinsic factor is degraded and released vitamin B12 enters the cytoplasm via LMBD1. The exit of free vitamin B12 from enterocyte might depend on MRP1. With enterohepatic circulation, the secreted vitamin B12 in duodenum would bind to intrinsic factor and then reabsorbed into the circulation.
Influence of gut microbiota on vitamin B12 utilization
Intestinal microbiotas are either producers or consumers of vitamin B12. Moreover, the intestinal absorption of vitamin B12 could be in turn influenced by intestinal microbiota (35). Several bacteria have been reported to be vitamin B12 producers, such as L. reuteri, and Enterococcus faecium (200, 201). It is supposed that vitamin B12-producing bacteria supplementation could improve vitamin B12 utilization in the gastrointestinal tract. Such an assumption has been proved in mice fed with vitamin B12 deficient diets. The supplementation of L. reuteri CRL1098, a vitamin B12-producing strain, prevented the signs of vitamin B12 deficiency, suggesting the therapeutical effect of intestinal bacteria in vitamin B12 deficiency (202). However, these beneficial effects might be limited if the bacteria are colonized in the colon (203), where lack of necessary transporters. In order to develop a probiotic treatment for vitamin B12 deficiency, the position of bacterial colonization should be considered. Around 80% of microbiota in the gastrointestinal tract are considered as consumers of vitamin B12 (204). Hence, the overgrowth of bacteria might compete with the exogenous vitamin B12 with their host and then reduce the bioavailability (205). In small intestinal bacterial overgrowth, consumption of vitamin B12 by the increased anaerobesis was considered a major reason for vitamin B12 deficient symptoms (206). Reducing the abundance of vitamin B12-consuming bacteria is of benefit to vitamin B12 deficiency. For instance, daily probiotic treatment of Lactobacillus performed a beneficial effect on both bacterial overgrowth and vitamin B12 absorption, suggesting the probiotic treatment might improve the vitamin B12 deficiency via inhibiting the vitamin B12-consuming bacteria overgrowth (207, 208).
Besides production or consumption of vitamin B12, intestinal microbiota might indirectly change the bioavailability of vitamin B12 via exerting influences on the absorption-related physiological factors. Gastrointestinal diseases associated with reduced acid secretion or enzyme content might interfere with the release of vitamin B12 from food (209) or the translation of vitamin B12 to intrinsic factors (199). Reduced vitamin B12 absorption is also observed in IBD, which is characterized by abnormal gut permeability (198, 199). As a probiotic, Lacidofil treatment significantly improved the gastric acid secretion in H. pylori-infected mongolian gerbils, which conduced to the release of vitamin B12 from food (210). Some gut bacteria have also presented the remission effect on IBD, which might improve the absorption of vitamin B12 via normalizing the gut permeability (211). Besides, excessive competition between gut microbiota and the host might interfere with the bioavailability of vitamin B12. For instance, Bacteroides thetaiotaomicron expresses an essential surface-exposed lipoprotein for vitamin B12 transport named BtuG (212). The higher binding affinity of BtuG could remove vitamin B12 from intrinsic factors and reduce vitamin B12 absorption.
Indirect role of vitamin B12 on human health as mediated by gut microbiota
Vitamin B12 serves as a critical cofactor of diverse enzymes in human gut microbes for nucleotide synthesis, amino acid metabolism, carbon and nitrogen metabolism, and secondary metabolite synthesis (203, 213). The biosynthesis of vitamin B12 involves about 30 enzyme-mediated steps and only a small fraction of bacteria could produce this vitamin (214). Most gut bacteria utilize vitamin B12 that escapes the absorption in the ileum and reaches the large intestine (215). The competition of vitamin B12 in gut microbiota might influence their growth, colonization, and metabolic processes (5, 204, 216).
In vitro study of colonic model suggested that vitamin B12 supplementation may increase α diversity, but the results depend on the form and dose of cobalamin administered (217). In another in vitro study, α diversity is reduced after methylcobalamin supplementation but not in cyanocobalamin treatment group (218). In mice, no significant difference in α diversity had been observed after vitamin B12 treatment, even under different doses (182, 219). A study suggested that cyanocobalamin supplementation had an increase in the α diversity and exerted a significant difference in β diversity at the genus level (220). However, some studies were unable to support this conclusion (182, 221). In humans, vitamin B12 intake could promote the increase of α diversity in adults but not in infants or children (72, 113, 222, 223). However, the association between vitamin B12 intake and β diversity was only observed in infants at 6 months of age and in older veterans rather than in other observed groups, including infants at the age of 4 or 5 months, lactating women, and children aged between 2 and 9 years (72, 113, 222, 223).
In the colonic model, cobalamin supplementation would increase the relative abundance of Firmicutes and Bacteroidetes and is opposite to Proteobacteria and Pseudomonas (217). After 7 days of methylcobalamin supplementation, an increased proportion of Acinetobacter and declined a fraction of Bacteroides, Enterobacteriaceae, and Ruminococcaceae had been observed in another colonic model-based study (218). In the studies of murine, the associations between vitamin B12 supplementation and the relative abundance of bacteria have been also reported (182, 219, 220). The supplementation of vitamin B12 has elevated the fraction of Firmicutes and reduced the proportion of Bacteroidetes. Compared to methylcobalamin, cyanocobalamin treatment resulted in higher levels of Bacteroidetes and Proteobacteria and lower levels of Firmicutes in mice. In humans, vitamin B12 intake might increase the proportion of Proteobacteria (113) and Verrucomicrobia (72) and reduce the abundance of Bacteroidetes (224). However, some clinical studies also suggested that vitamin B12 intake had no influence on bacterial abundance (222, 223, 225–227). These controversial results might be due to the different study designs and participants.
In vitro study suggested that the addition of cobalamins increases the generation of SCFAs, especially butyrate and propionic acid (218). Another in vitro study indicated that low-dose cyanocobalamin-enriched spinach could increase the generation of butyrate and acetate (228). In mice, a reduction of SCFAs has been observed in dietary vitamin B12 restriction (229). However, the effect of oral vitamin B12 on cecal SCFA was absent in mice with dextran sodium sulfate induced colitis (220).
Conclusion and prospects
In this review, we summarized current knowledge about the interaction between gut microbiota and vitamin B nutrition (Figure 2) to infer the consequence of probiotics supplementation, which might be helpful to optimize the treatment of probiotics. Vitamin B perform as essential micronutrients for human. The absorption process of multiple dietary vitamin B requires the assistance of many transporters, which generally occurs in the small intestine (Table 1). At the same time, gut microbiota not only act as producers and/or consumers to modify the supplementation of vitamin B in the gut (Table 2) but also affect the absorption of vitamin B by altering the physiological or pathological factors of the gastrointestinal tract (Table 3). As cofactors of multiple enzymes, supplement with vitamin B can change the diversity, abundance, and functions of gut microbiota (Table 4). Understanding the interactions between vitamin B and gut microbiota can help us prevent vitamin B deficiency and, more importantly, allow us to recognize beneficial potential of probiotics on human healthy.
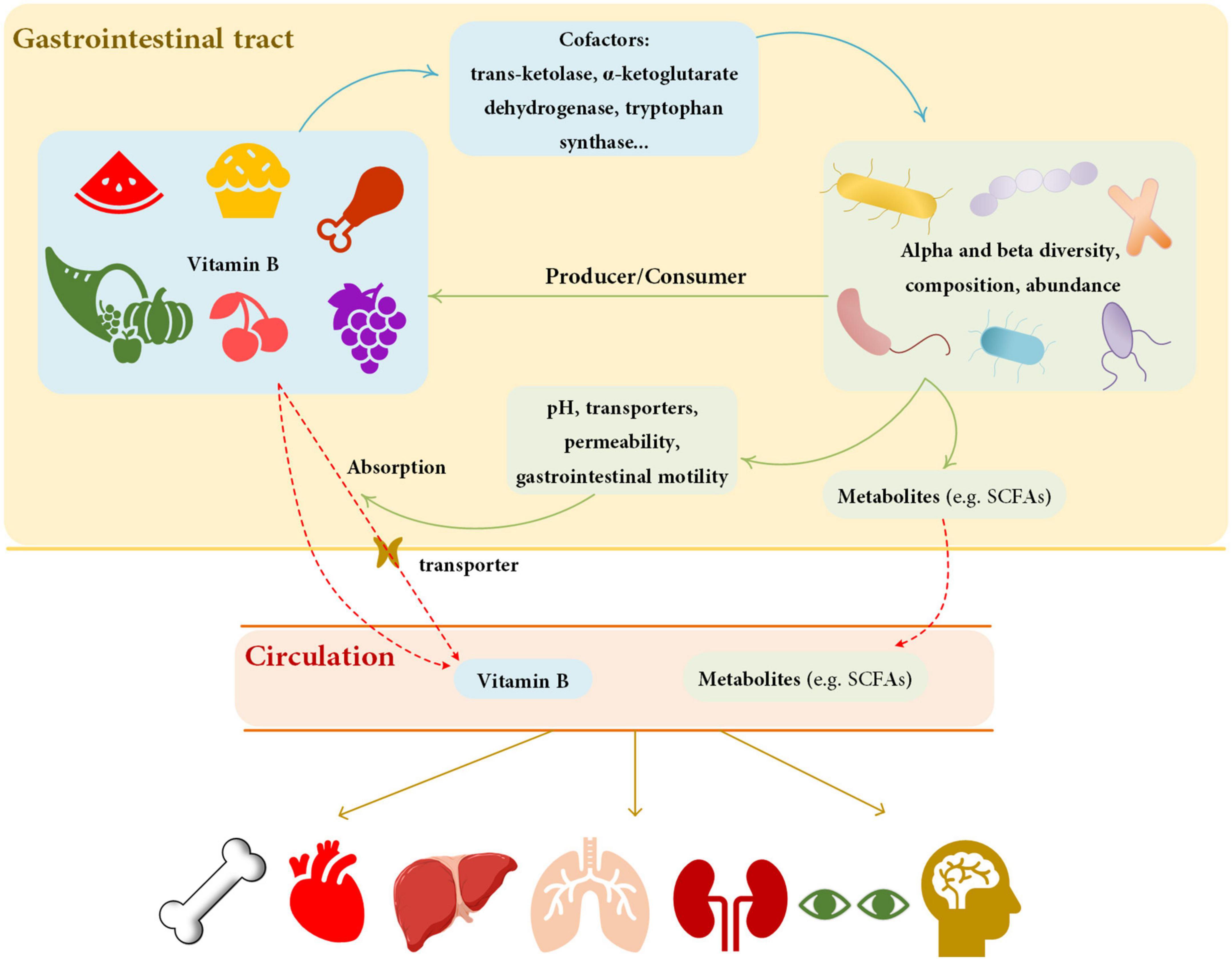
Figure 2. Summary of the relationship between vitamin B and gut microbiota. Vitamin B can affect the diversity, composition, and abundance of gut microbiota by influencing cofactors. Gut microbiota affects vitamin B by producing or consuming and by modifying the physiological properties of the gastrointestinal tract. Solid lines represent effect, dashed lines represent transportation. The blue lines show the effect of vitamin B on gut microbiota, and the green lines show the effect of vitamin B on gut microbiota. Red lines represent the process of substance transfers into circulation. Yellow lines represent the effects of circulation substances on organs. The metabolites of gut microbiota and dietary vitamin B can affect human health. SCFAs, short chain fatty acids.
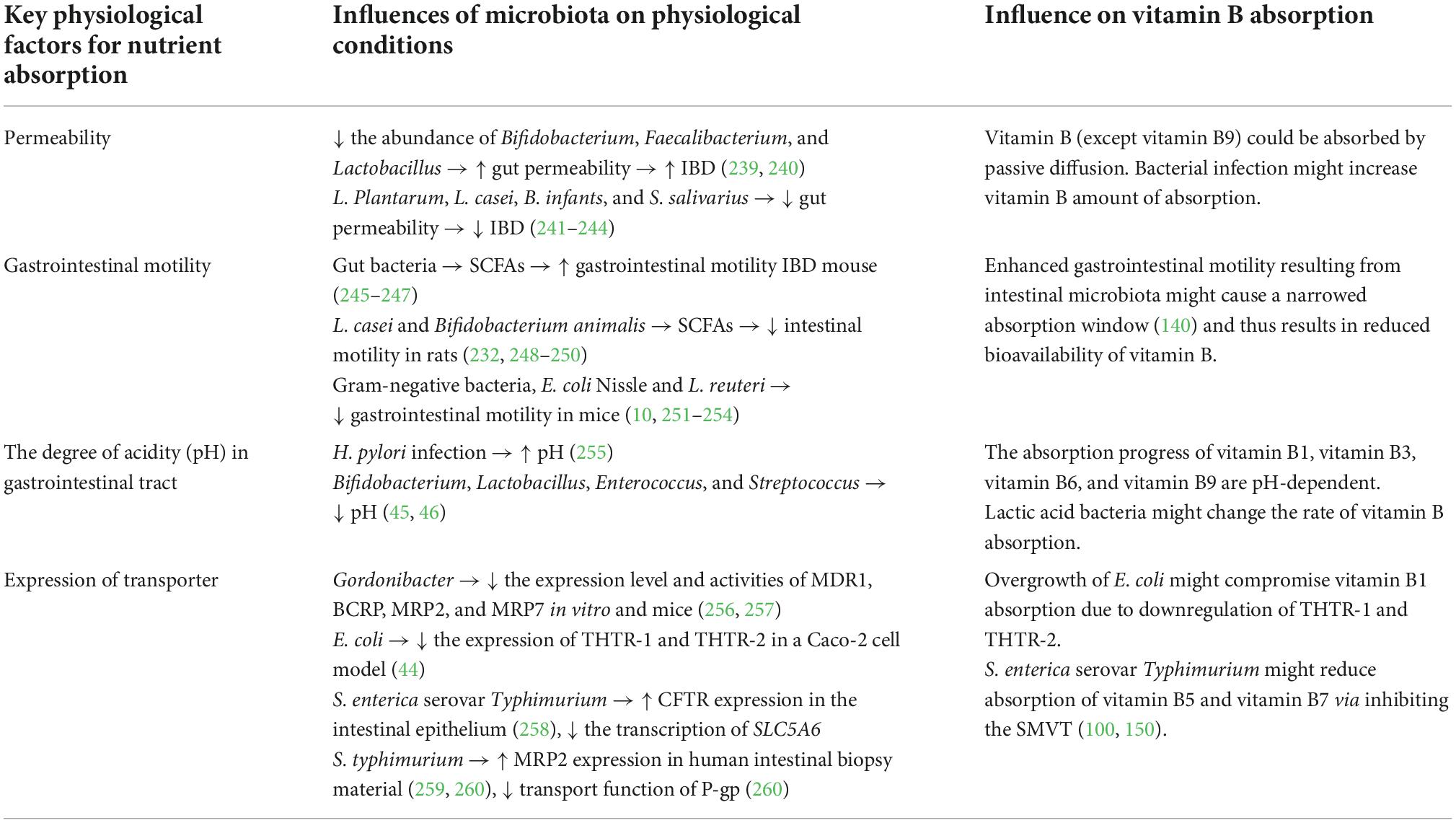
Table 3. Gut microbiota affect the absorption of vitamin B via modifying the physiological properties of gastrointestinal tract.
In addition to supplements, the probiotic product has recently been approved by FDA as the therapeutical approach for disease treatment, which has significantly extended the application of probiotics. Considering their ability for vitamin B production and intestinal function modification, probiotics might be a potential therapeutical approach for vitamin B deficiency. Although conventional vitamin B supplements have been applied, they are insufficient to treat vitamin B deficiency patients of malabsorption. In this population, improved intestinal absorption rather than vitamin B supplementation would be a more efficient approach to improve vitamin B nutrition. The combination of probiotics regulating intestinal function and vitamin B producers/supplements could further increase the absorption of vitamin B in the gastrointestinal tract. Despite the beneficial effect on human health, risks are remaining in probiotics treatment. The overgrowth of Lactobacillus murinus after vancomycin treatment might deplete vitamin B7 available, suggesting the potential risk of nutrition competition after probiotics treatment. For the purpose of reducing the risk of nutrition competition, vitamin B should be supplied along with probiotics. Another consideration of vitamin B supplementations in probiotics treatment is the beneficial effect of vitamin B on the growth and function of gut microbiota. But types and dosages of vitamin B should be optimized as supplements of probiotics treatment. Overall, probiotics treatment could be a potential treatment for vitamin B deficiency, and vitamin B supplement might either reduce the risk of probiotics-induced vitamin B deficiency or improve the efficacy of vitamin B. Nonetheless, these assumptions are still requiring further scientific and clinical studies to optimize the combination of vitamin B and probiotics treatment.
Author contributions
HH and JK designed the manuscript. ZW, LS, JWZ, SL, YXZ, RW, YCZ, JHZ, ZZ, JD, and LC performed the literature review. ZW, JHZ, and ZZ drafted the manuscript. ZW, HH, KH, and JK revised the manuscript. All authors contributed to the article and approved the submitted version.
Conflict of interest
The authors declare that the research was conducted in the absence of any commercial or financial relationships that could be construed as a potential conflict of interest.
Publisher’s note
All claims expressed in this article are solely those of the authors and do not necessarily represent those of their affiliated organizations, or those of the publisher, the editors and the reviewers. Any product that may be evaluated in this article, or claim that may be made by its manufacturer, is not guaranteed or endorsed by the publisher.
References
1. Fenech M. Vitamins associated with brain aging, mild cognitive impairment, and alzheimer disease: biomarkers, epidemiological and experimental evidence, plausible mechanisms, and knowledge gaps. Adv Nutr. (2017) 8:958–70. doi: 10.3945/an.117.015610
2. Calderon-Ospina C, Nava-Mesa M. B vitamins in the nervous system: current knowledge of the biochemical modes of action and synergies of thiamine, pyridoxine, and cobalamin. CNS Neurosci Ther. (2020) 26:5–13. doi: 10.1111/cns.13207
3. Selhub J, Troen A, Rosenberg I. B vitamins and the aging brain. Nutr Rev. (2010) 68:S112–8. doi: 10.1111/j.1753-4887.2010.00346.x
4. Bjorklund G, Peana M, Dadar M, Lozynska I, Chirumbolo S, Lysiuk R, et al. The role of B vitamins in stroke prevention. Crit Rev Food Sci Nutr. (2022) 62:5462–75. doi: 10.1080/10408398.2021.1885341
5. Roth W, Mohamadzadeh M. Vitamin B12 and gut-brain homeostasis in the pathophysiology of ischemic stroke. EBioMedicine. (2021) 73:103676. doi: 10.1016/j.ebiom.2021.103676
6. Morris M. The role of B vitamins in preventing and treating cognitive impairment and decline. Adv Nutr. (2012) 3:801–12. doi: 10.3945/an.112.002535
7. Clarke M, Ward M, Strain J, Hoey L, Dickey W, McNulty H. B-vitamins and bone in health and disease: the current evidence. Proc Nutr Soc. (2014) 73:330–9. doi: 10.1017/S0029665114000044
8. LeBlanc J, Milani C, de Giori G, Sesma F, van Sinderen D, Ventura M. Bacteria as vitamin suppliers to their host: a gut microbiota perspective. Curr Opin Biotechnol. (2013) 24:160–8. doi: 10.1016/j.copbio.2012.08.005
9. Said H, Nexo E. Gastrointestinal handling of water-soluble vitamins. Compr Physiol. (2018) 8:1291–311. doi: 10.1002/cphy.c170054
10. Dimidi E, Christodoulides S, Scott S, Whelan K. Mechanisms of action of probiotics and the gastrointestinal microbiota on gut motility and constipation. Adv Nutr. (2017) 8:484–94. doi: 10.3945/an.116.014407
11. Chotikatum S, Naim H, El-Najjar N. Inflammation induced er stress affects absorptive intestinal epithelial cells function and integrity. Int Immunopharmacol. (2018) 55:336–44. doi: 10.1016/j.intimp.2017.12.016
12. Mirsepasi-Lauridsen H, Vallance B, Krogfelt K, Petersen A. Escherichia coli pathobionts associated with inflammatory bowel disease. Clin Microbiol Rev. (2019) 32:18. doi: 10.1128/CMR.00060-18
13. Yoshimatsu Y, Mikami Y, Kanai T. Bacteriotherapy for inflammatory bowel disease. Inflamm Regen. (2021) 41:3. doi: 10.1186/s41232-020-00153-4
14. Valdes A, Walter J, Segal E, Spector T. Role of the gut microbiota in nutrition and health. BMJ. (2018) 361:k2179. doi: 10.1136/bmj.k2179
15. Fan Y, Pedersen O. Gut Microbiota in Human Metabolic Health and Disease. Nat Rev Microbiol. (2021) 19:55–71. doi: 10.1038/s41579-020-0433-9
16. Lynch S, Pedersen O. The human intestinal microbiome in health and disease. N Engl J Med. (2016) 375:2369–79. doi: 10.1056/NEJMra1600266
17. Tang W, Backhed F, Landmesser U, Hazen S. Intestinal microbiota in cardiovascular health and disease: JACC state-of-the-art review. J Am Coll Cardiol. (2019) 73:2089–105. doi: 10.1016/j.jacc.2019.03.024
18. Agus A, Clement K, Sokol H. Gut microbiota-derived metabolites as central regulators in metabolic disorders. Gut. (2021) 70:1174–82. doi: 10.1136/gutjnl-2020-323071
19. Cox T, Lundgren P, Nath K, Thaiss C. Metabolic control by the microbiome. Genome Med. (2022) 14:80. doi: 10.1186/s13073-022-01092-0
20. Lu Y, Yuan X, Wang M, He Z, Li H, Wang J, et al. Gut microbiota influence immunotherapy responses: mechanisms and therapeutic strategies. J Hematol Oncol. (2022) 15:47. doi: 10.1186/s13045-022-01273-9
21. Connell E, Le Gall G, Pontifex M, Sami S, Cryan J, Clarke G, et al. Microbial-derived metabolites as a risk factor of age-related cognitive decline and dementia. Mol Neurodegener. (2022) 17:43. doi: 10.1186/s13024-022-00548-6
22. Xu X, Poulsen K, Wu L, Liu S, Miyata T, Song Q, et al. Targeted therapeutics and novel signaling pathways in non-alcohol-associated fatty liver/steatohepatitis (Nafl/Nash). Signal Trans Target Ther. (2022) 7:287. doi: 10.1038/s41392-022-01119-3
23. Martin-Gallausiaux C, Marinelli L, Blottiere H, Larraufie P, Lapaque N. Scfa: mechanisms and functional importance in the gut. Proc Nutr Soc. (2021) 80:37–49. doi: 10.1017/S0029665120006916
24. Hossain K, Amarasena S, Mayengbam S. B vitamins and their roles in gut health. Microorganisms. (2022) 10:1168. doi: 10.3390/microorganisms10061168
25. Uebanso T, Shimohata T, Mawatari K, Takahashi A. Functional roles of B-vitamins in the gut and gut microbiome. Mol Nutr Food Res. (2020) 64:e2000426. doi: 10.1002/mnfr.202000426
26. Lonsdale D. A review of the biochemistry, metabolism and clinical benefits of thiamin(E) and its derivatives. Evid Based Complement Alternat Med. (2006) 3:49–59. doi: 10.1093/ecam/nek009
27. Peterson C, Rodionov D, Osterman A, Peterson S. B vitamins and their role in immune regulation and cancer. Nutrients. (2020) 12:380. doi: 10.3390/nu12113380
28. World Health Organization. Vitamin and mineral requirements in human nutrition. 2nd ed. Geneva: World Health Organization (2005).
29. Sechi G, Serra A. Wernicke’s encephalopathy: new clinical settings and recent advances in diagnosis and management. Lancet Neurol. (2007) 6:442–55. doi: 10.1016/S1474-442270104-7
30. Butterworth R. Hepatic encephalopathy in alcoholic cirrhosis. Handb Clin Neurol. (2014) 125:589–602. doi: 10.1016/B978-0-444-62619-6.00034-3
31. Rindi G, Laforenza U. Thiamine intestinal transport and related issues: recent aspects. Proc Soc Exp Biol Med. (2000) 224:246–55. doi: 10.1046/j.1525-1373.2000.22428.x
32. Smithline H, Donnino M, Greenblatt D. Pharmacokinetics of high-dose oral thiamine hydrochloride in healthy subjects. BMC Clin Pharmacol. (2012) 12:4. doi: 10.1186/1472-6904-12-4
33. Ganapathy V, Smith S, Prasad P. Slc19: the folate/thiamine transporter family. Pflugers Arch. (2004) 447:641–6. doi: 10.1007/s00424-003-1068-1
34. Nabokina S, Inoue K, Subramanian V, Valle J, Yuasa H, Said H. Molecular identification and functional characterization of the human colonic thiamine pyrophosphate transporter. J Biol Chem. (2014) 289:4405–16. doi: 10.1074/jbc.M113.528257
35. Magnusdottir S, Ravcheev D, de Crecy-Lagard V, Thiele I. Systematic genome assessment of B-vitamin biosynthesis suggests co-operation among gut microbes. Front Genet. (2015) 6:148. doi: 10.3389/fgene.2015.00148
36. Zhang G, Dai J, Lu Z, Dunaway-Mariano D. The phosphonopyruvate decarboxylase from Bacteroides fragilis. J Biol Chem. (2003) 278:41302–8. doi: 10.1074/jbc.M305976200
37. Shi Z, Qiu Y, Wang J, Fang Y, Zhang Y, Chen H, et al. Dysbiosis of gut microbiota in patients with neuromyelitis optica spectrum disorders: a cross sectional study. J Neuroimmunol. (2020) 339:577126. doi: 10.1016/j.jneuroim.2019.577126
38. Kuboniwa M, Hendrickson E, Xia Q, Wang T, Xie H, Hackett M, et al. Proteomics of porphyromonas gingivalis within a model oral microbial community. BMC Microbiol. (2009) 9:98. doi: 10.1186/1471-2180-9-98
39. Soto-Martin E, Warnke I, Farquharson F, Christodoulou M, Horgan G, Derrien M, et al. Vitamin biosynthesis by human gut butyrate-producing bacteria and cross-feeding in synthetic microbial communities. mBio. (2020) 11:20. doi: 10.1128/mBio.00886-20
40. Costliow Z, Degnan P. Thiamine acquisition strategies impact metabolism and competition in the gut microbe Bacteroides thetaiotaomicron. mSystems. (2017) 2:17. doi: 10.1128/mSystems.00116-17
41. Park J, Hosomi K, Kawashima H, Chen Y, Mohsen A, Ohno H, et al. Dietary vitamin B1 intake influences gut microbial community and the consequent production of short-chain fatty acids. Nutrients. (2022) 14:2078. doi: 10.3390/nu14102078
42. Ringe H, Schuelke M, Weber S, Dorner B, Kirchner S, Dorner M. Infant botulism: is there an association with thiamine deficiency? Pediatrics. (2014) 134:e1436–40. doi: 10.1542/peds.2013-3378
43. Ghosal A, Chatterjee N, Chou T, Said H. Enterotoxigenic Escherichia coli infection and intestinal thiamin uptake: studies with intestinal epithelial Caco-2 monolayers. Am J Physiol Cell Physiol. (2013) 305:C1185–91. doi: 10.1152/ajpcell.00276.2013
44. Ashokkumar B, Kumar J, Hecht G, Said H. Enteropathogenic Escherichia coli inhibits intestinal vitamin B1 (thiamin) uptake: studies with human-derived intestinal epithelial Caco-2 cells. Am J Physiol Gastrointest Liver Physiol. (2009) 297:G825–33. doi: 10.1152/ajpgi.00250.2009
45. Allaart J, van Asten A, Vernooij J, Grone A. Effect of Lactobacillus fermentum on beta2 toxin production by clostridium perfringens. Appl Environ Microbiol. (2011) 77:4406–11. doi: 10.1128/AEM.03002-10
46. Allaart J, van Asten A, Grone A. Predisposing factors and prevention of clostridium perfringens-associated enteritis. Comput Immunol Microbiol Infect Dis. (2013) 36:449–64. doi: 10.1016/j.cimid.2013.05.001
47. Elmadfa I, Heinzle C, Majchrzak D, Foissy H. Influence of a probiotic yoghurt on the status of vitamins B, B and B in the healthy adult human. Ann Nutr Metab. (2001) 45:13–8. doi: 10.1159/000046700
48. Fabian E, Majchrzak D, Dieminger B, Meyer E, Elmadfa I. Influence of probiotic and conventional yoghurt on the status of vitamins B1, B2 and B6 in young healthy women. Ann Nutr Metab. (2008) 52:29–36. doi: 10.1159/000114408
49. Pan X, Xue F, Nan X, Tang Z, Wang K, Beckers Y, et al. Illumina sequencing approach to characterize thiamine metabolism related bacteria and the impacts of thiamine supplementation on ruminal microbiota in dairy cows fed high-grain diets. Front Microbiol. (2017) 8:1818. doi: 10.3389/fmicb.2017.01818
50. LeBlanc J, Laino J, del Valle M, Vannini V, van Sinderen D, Taranto M, et al. B-group vitamin production by lactic acid bacteria–current knowledge and potential applications. J Appl Microbiol. (2011) 111:1297–309. doi: 10.1111/j.1365-2672.2011.05157.x
51. Ghosal A, Said H. Mechanism and regulation of vitamin B2 (riboflavin) uptake by mouse and human pancreatic beta-cells/islets: physiological and molecular aspects. Am J Physiol Gastrointest Liver Physiol. (2012) 303:G1052–8. doi: 10.1152/ajpgi.00314.2012
52. Thakur K, Tomar S, Singh A, Mandal S, Arora S. Riboflavin and health: a review of recent human research. Crit Rev Food Sci Nutr. (2017) 57:3650–60. doi: 10.1080/10408398.2016.1145104
53. Sinha T, Naash M, Al-Ubaidi M. Flavins act as a critical liaison between metabolic homeostasis and oxidative stress in the retina. Front Cell Dev Biol. (2020) 8:861. doi: 10.3389/fcell.2020.00861
54. Suwannasom N, Kao I, Pruss A, Georgieva R, Baumler H. Riboflavin: the health benefits of a forgotten natural vitamin. Int J Mol Sci. (2020) 21:950. doi: 10.3390/ijms21030950
55. Ben S, Du M, Ma G, Qu J, Zhu L, Chu H, et al. Vitamin B2 intake reduces the risk for colorectal cancer: a dose-response analysis. Eur J Nutr. (2019) 58:1591–602. doi: 10.1007/s00394-018-1702-5
56. McNulty H, Strain J, Hughes C, Ward M. Riboflavin, mthfr genotype and blood pressure: a personalized approach to prevention and treatment of hypertension. Mol Aspects Med. (2017) 53:2–9. doi: 10.1016/j.mam.2016.10.002
57. Powers H. Riboflavin (vitamin B-2) and health. Am J Clin Nutr. (2003) 77:1352–60. doi: 10.1093/ajcn/77.6.1352
58. Akiyama T, Selhub J, Rosenberg I. Fmn phosphatase and fad pyrophosphatase in rat intestinal brush borders: role in intestinal absorption of dietary riboflavin. J Nutr. (1982) 112:263–8. doi: 10.1093/jn/112.2.263
59. Balasubramaniam S, Christodoulou J, Rahman S. Disorders of riboflavin metabolism. J Inherit Metab Dis. (2019) 42:608–19. doi: 10.1002/jimd.12058
60. Barile M, Giancaspero T, Leone P, Galluccio M, Indiveri C. Riboflavin transport and metabolism in humans. J Inherit Metab Dis. (2016) 39:545–57. doi: 10.1007/s10545-016-9950-0
61. Revuelta J, Ledesma-Amaro R, Lozano-Martinez P, Diaz-Fernandez D, Buey R, Jimenez A. Bioproduction of riboflavin: a bright yellow history. J Ind Microbiol Biotechnol. (2017) 44:659–65. doi: 10.1007/s10295-016-1842-7
62. LeBlanc J, Burgess C, Sesma F, de Giori G, van Sinderen D. Lactococcus lactis is capable of improving the riboflavin status in deficient rats. Br J Nutr. (2005) 94:262–7. doi: 10.1079/bjn20051473
63. Houston J, Levy G. Effect of carbonated beverages and of an antiemetic containing carbohydrate and phosphoric acid on riboflavin bioavailability and salicylamide biotransformation in humans. J Pharm Sci. (1975) 64:1504–7. doi: 10.1002/jps.2600640917
64. Indrio F, Riezzo G, Raimondi F, Bisceglia M, Filannino A, Cavallo L, et al. Lactobacillus reuteri accelerates gastric emptying and improves regurgitation in infants. Eur J Clin Invest. (2011) 41:417–22. doi: 10.1111/j.1365-2362.2010.02425.x
65. Ohtsu T, Haruma K, Ide Y, Takagi A. The effect of continuous intake of lactobacillus gasseri oll2716 on mild to moderate delayed gastric emptying: a randomized controlled study. Nutrients. (2021) 13:1852. doi: 10.3390/nu13061852
66. Matnuri M, Zheng C, Sidik D, Bai G, Abdukerim M, Abdukadier A, et al. Correlation analysis of riboflavin, rft2 and helicobater pylori in gastric carcinoma. Int J Clin Exp Pathol. (2015) 8:13339–45.
67. Ren C, Faas M, de Vos P. Disease managing capacities and mechanisms of host effects of lactic acid bacteria. Crit Rev Food Sci Nutr. (2021) 61:1365–93. doi: 10.1080/10408398.2020.1758625
68. da Silva Ferreira A, Wardill H, Havinga R, Tissing W, Harmsen H. Prophylactic treatment with vitamins C and B2 for methotrexate-induced gastrointestinal mucositis. Biomolecules. (2020) 11:10034. doi: 10.3390/biom11010034
69. Zhu Y, Thakur K, Feng J, Zhang J, Hu F, Cespedes-Acuna C, et al. Riboflavin bioenriched soymilk alleviates oxidative stress mediated liver injury, intestinal inflammation, and gut microbiota modification in B2 depletion-repletion mice. J Agric Food Chem. (2022) 70:3818–31. doi: 10.1021/acs.jafc.2c00117
70. Pham V, Fehlbaum S, Seifert N, Richard N, Bruins M, Sybesma W, et al. Effects of colon-targeted vitamins on the composition and metabolic activity of the human gut microbiome- a pilot study. Gut Microbes. (2021) 13:1–20. doi: 10.1080/19490976.2021.1875774
71. von Martels J, Bourgonje A, Klaassen M, Alkhalifah H, Sadaghian Sadabad M, Vich Vila A, et al. Riboflavin supplementation in patients with crohn’s disease [the rise-up study]. J Crohns Colitis. (2020) 14:595–607. doi: 10.1093/ecco-jcc/jjz208
72. Gurwara S, Ajami N, Jang A, Hessel F, Chen L, Plew S, et al. Dietary nutrients involved in one-carbon metabolism and colonic mucosa-associated gut microbiome in individuals with an endoscopically normal colon. Nutrients. (2019) 11:613. doi: 10.3390/nu11030613
73. Liu L, Sadaghian Sadabad M, Gabarrini G, Lisotto P, von Martels J, Wardill H, et al. Riboflavin supplementation promotes butyrate production in the absence of gross compositional changes in the gut microbiota. Antioxid Redox Signal. (2022) 2022:33. doi: 10.1089/ars.2022.0033
74. Maitiniyazi G, Cao X, Chen Y, Zhang R, Liu Y, Li Z, et al. Impact of gut microbiota on the association between diet and depressive symptoms in breast cancer. Nutrients. (2022) 14:1186. doi: 10.3390/nu14061186
75. Steinert R, Sadaghian Sadabad M, Harmsen H, Weber P. The prebiotic concept and human health: a changing landscape with riboflavin as a novel prebiotic candidate? Eur J Clin Nutr. (2016) 70:1348–53. doi: 10.1038/ejcn.2016.119
76. Duncan S, Louis P, Flint H. Lactate-utilizing bacteria, isolated from human feces, that produce butyrate as a major fermentation product. Appl Environ Microbiol. (2004) 70:5810–7. doi: 10.1128/AEM.70.10.5810-5817.2004
77. Jacobson M, Jacobson E. Vitamin B3 in health and disease: toward the second century of discovery. Methods Mol Biol. (2018) 1813:3–8. doi: 10.1007/978-1-4939-8588-3_1
78. Makarov M, Trammell S, Migaud M. The chemistry of the vitamin B3 metabolome. Biochem Soc Trans. (2019) 47:131–47. doi: 10.1042/BST20180420
79. Kirkland J, Meyer-Ficca M. Niacin. Adv Food Nutr Res. (2018) 83:83–149. doi: 10.1016/bs.afnr.2017.11.003
80. Kamat J, Devasagayam T. Nicotinamide (vitamin B3) as an effective antioxidant against oxidative damage in rat brain mitochondria. Redox Rep. (1999) 4:179–84. doi: 10.1179/135100099101534882
81. Williams P, Harder J, Foxworth N, Cochran K, Philip V, Porciatti V, et al. Vitamin B3 modulates mitochondrial vulnerability and prevents glaucoma in aged mice. Science. (2017) 355:756–60. doi: 10.1126/science.aal0092
82. Figge H, Figge J, Souney P, Mutnick A, Sacks F. Nicotinic acid: a review of its clinical use in the treatment of lipid disorders. Pharmacotherapy. (1988) 8:287–94. doi: 10.1002/j.1875-9114.1988.tb04085.x
83. Yoshii K, Hosomi K, Sawane K, Kunisawa J. Metabolism of dietary and microbial vitamin B family in the regulation of host immunity. Front Nutr. (2019) 6:48. doi: 10.3389/fnut.2019.00048
84. Nabokina S, Kashyap M, Said H. Mechanism and regulation of human intestinal niacin uptake. Am J Physiol Cell Physiol. (2005) 289:C97–103. doi: 10.1152/ajpcell.00009.2005
85. Bechgaard H, Jespersen S. Gi absorption of niacin in humans. J Pharm Sci. (1977) 66:871–2. doi: 10.1002/jps.2600660635
86. Takanaga H, Maeda H, Yabuuchi H, Tamai I, Higashida H, Tsuji A. Nicotinic acid transport mediated by ph-dependent anion antiporter and proton cotransporter in rabbit intestinal brush-border membrane. J Pharm Pharmacol. (1996) 48:1073–7. doi: 10.1111/j.2042-7158.1996.tb05902.x
87. Sadoogh-Abasian F, Evered D. Absorption of nicotinic acid and nicotinamide from rat small intestine in vitro. Biochim Biophys Acta. (1980) 598:385–91. doi: 10.1016/0005-273690016-4
88. Deguchi Y, Morishita T, Mutai M. Comparative studies on synthesis of water-soluble vitamins among human species of bifidobacteria. Agric Biol Chem. (1985) 49:13–9.
89. Fangmann D, Theismann E, Turk K, Schulte D, Relling I, Hartmann K, et al. Targeted microbiome intervention by microencapsulated delayed-release niacin beneficially affects insulin sensitivity in humans. Diabetes Care. (2018) 41:398–405. doi: 10.2337/dc17-1967
90. Hashimoto T, Perlot T, Rehman A, Trichereau J, Ishiguro H, Paolino M, et al. Ace2 links amino acid malnutrition to microbial ecology and intestinal inflammation. Nature. (2012) 487:477–81. doi: 10.1038/nature11228
91. Feng J, Wang L, Chen Y, Xiong Y, Wu Q, Jiang Z, et al. Effects of niacin on intestinal immunity, microbial community and intestinal barrier in weaned piglets during starvation. Int Immunopharmacol. (2021) 95:107584. doi: 10.1016/j.intimp.2021.107584
93. Walsh J, Wyse B, Hansen R. Pantothenic acid content of 75 processed and cooked foods. J Am Diet Assoc. (1981) 78:140–4.
94. Bean W, Hodges R, Daum K. Pantothenic acid deficiency induced in human subjects. J Clin Invest. (1955) 34:1073–84. doi: 10.1172/JCI103156
95. Barton-Wright E, Elliott W. The pantothenic acid metabolism of rheumatoid arthritis. Lancet. (1963) 2:862–3. doi: 10.1016/s0140-673692748-x
96. Fry P, Fox H, Tao H. Metabolic response to a pantothenic acid deficient diet in humans. J Nutr Sci Vitaminol. (1976) 22:339–46. doi: 10.3177/jnsv.22.339
97. Koyanagi T, Hareyama S, Kikuchi R, Kimura T. Effect of diet on the pantothenic acid content in serum and on the incidence of hypertension among villagers. Tohoku J Exp Med. (1966) 88:93–7. doi: 10.1620/tjem.88.93
98. Cummings J. The large intestine. its role in mammalian nutrition and homeostasis. Quart Rev Biol. (1981) 22:896–901.
99. Shibata K, Gross C, Henderson L. Hydrolysis and absorption of pantothenate and its coenzymes in the rat small intestine. J Nutr. (1983) 113:2107–15. doi: 10.1093/jn/113.10.2107
100. Said H, Ortiz A, McCloud E, Dyer D, Moyer M, Rubin S. Biotin uptake by human colonic epithelial ncm460 cells: a carrier-mediated process shared with pantothenic acid. Am J Physiol. (1998) 275:C1365–71. doi: 10.1152/ajpcell.1998.275.5.C1365
101. Said H. Cellular uptake of biotin: mechanisms and regulation. J Nutr. (1999) 129:490S–3S. doi: 10.1093/jn/129.2.490S
102. Chatterjee N, Kumar C, Ortiz A, Rubin S, Said H. Molecular mechanism of the intestinal biotin transport process. Am J Physiol. (1999) 277:C605–13. doi: 10.1152/ajpcell.1999.277.4.C605
103. Prasad P, Wang H, Huang W, Fei Y, Leibach F, Devoe L, et al. Molecular and functional characterization of the intestinal na+-dependent multivitamin transporter. Arch Biochem Biophys. (1999) 366:95–106. doi: 10.1006/abbi.1999.1213
104. Ghosal A, Lambrecht N, Subramanya S, Kapadia R, Said H. Conditional knockout of the slc5a6 gene in mouse intestine impairs biotin absorption. Am J Physiol Gastrointest Liver Physiol. (2013) 304:G64–71. doi: 10.1152/ajpgi.00379.2012
105. Begley T, Kinsland C, Strauss E. The biosynthesis of coenzyme a in bacteria. Vitam Horm. (2001) 61:157–71. doi: 10.1016/s0083-672961005-7
106. Tahiliani A, Beinlich C. Pantothenic acid in health and disease. Vitam Horm. (1991) 46:165–228. doi: 10.1016/s0083-672960684-6
107. Cronan J, Gennis R, Maloy S. Escherichia coli and Salmonella typhimurium. Cell Mol Biol. (1987) 1987:31–55.
108. Lee-Peng F, Hermodson M, Kohlhaw G. Transaminase B from Escherichia coli: quaternary structure, amino-terminal sequence, substrate specificity, and absence of a separate valine-alpha-ketoglutarate activity. J Bacteriol. (1979) 139:339–45. doi: 10.1128/jb.139.2.339-345.1979
109. Khoshayand F, Goodarzi S, Shahverdi A, Khoshayand M. Optimization of culture conditions for fermentation of soymilk using Lactobacillus casei by response surface methodology. Probiot Antim Proteins. (2011) 3:159–67. doi: 10.1007/s12602-011-9079-2
110. Khan H, Flint S, Yu P. Development of a chemically defined medium for the production of enterolysin a from enterococcus faecalis B9510. J Appl Microbiol. (2013) 114:1092–102. doi: 10.1111/jam.12115
111. Stein E, Diamond J. Do dietary levels of pantothenic acid regulate its intestinal uptake in mice? J Nutr. (1989) 119:1973–83. doi: 10.1093/jn/119.12.1973
112. Wright L, Welch A. The role of “folic acid” and biotin in the utilization of pantothenic acid by the rat. Science. (1943) 97:426–7. doi: 10.1126/science.97.2523.426
113. Carrothers J, York M, Brooker S, Lackey K, Williams J, Shafii B, et al. Fecal microbial community structure is stable over time and related to variation in macronutrient and micronutrient intakes in lactating women. J Nutr. (2015) 145:2379–88. doi: 10.3945/jn.115.211110
114. Xun P, Lin H, Zhou C, Huang Z, Yu W, Yang Y, et al. Effects of dietary pantothenic acid supplement on hepatic antioxidative abilities and intestinal microflora in juvenile golden pompano (Trachinotus ovatus). Israeli J Aquac Bamidgeh. (2019) 71:1–8.
115. Chaudhry R, Ghosh A, Chandolia A. Pathogenesis of mycoplasma pneumoniae: an update. Indian J Med Microbiol. (2016) 34:7–16. doi: 10.4103/0255-0857.174112
116. Burki S, Spergser J, Bodmer M, Pilo P. A dominant lineage of mycoplasma bovis is associated with an increased number of severe mastitis cases in cattle. Vet Microbiol. (2016) 196:63–6. doi: 10.1016/j.vetmic.2016.10.016
117. Yao C, Chou J, Wang T, Zhao H, Zhang B. Pantothenic acid, vitamin C, and biotin play important roles in the growth of lactobacillus helveticus. Front Microbiol. (2018) 9:1194. doi: 10.3389/fmicb.2018.01194
118. Coburn S. Modeling vitamin B6 metabolism. Adv Food Nutr Res. (1996) 40:107–32. doi: 10.1016/s1043-452660023-6
119. Albersen M, Bosma M, Knoers N, de Ruiter B, Diekman E, de Ruijter J, et al. The intestine plays a substantial role in human vitamin B6 metabolism: a Caco-2 cell model. PLoS One. (2013) 8:e54113. doi: 10.1371/journal.pone.0054113
120. Said H, Ortiz A, Ma TYA. Carrier-mediated mechanism for pyridoxine uptake by human intestinal epithelial Caco-2 cells: regulation by a pka-mediated pathway. Am J Physiol Cell Physiol. (2003) 285:C1219–25. doi: 10.1152/ajpcell.00204.2003
121. Ueland P, McCann A, Midttun O, Ulvik A. Inflammation, vitamin B6 and related pathways. Mol Aspects Med. (2017) 53:10–27. doi: 10.1016/j.mam.2016.08.001
122. Coburn S. Vitamin B-6 metabolism and interactions with tnap. Subcell Biochem. (2015) 76:207–38. doi: 10.1007/978-94-017-7197-9_11
123. World Health Organization, Food and Agriculture Organization of the United Nations. Vitamin and Mineral Requirements in Human Nutrition. Geneva: World Health Organization (2004).
124. Said Z, Subramanian V, Vaziri N, Said H. Pyridoxine uptake by colonocytes: a specific and regulated carrier-mediated process. Am J Physiol Cell Physiol. (2008) 294:C1192–7. doi: 10.1152/ajpcell.00015.2008
125. Merrill A Jr., Henderson J. Diseases associated with defects in vitamin B6 metabolism or utilization. Annu Rev Nutr. (1987) 7:137–56. doi: 10.1146/annurev.nu.07.070187.001033
126. Miles E. Tryptophan synthase: a multienzyme complex with an intramolecular tunnel. Chem Res. (2001) 1:140–51. doi: 10.1002/tcr.4
127. Tai C, Cook P. Pyridoxal 5’-phosphate-dependent alpha, beta-elimination reactions: mechanism of o-acetylserine sulfhydrylase. Acces Chem Res. (2001) 34:49–59. doi: 10.1021/ar990169l
128. Ferreira G, Gong J. 5-aminolevulinate synthase and the first step of heme biosynthesis. J Bioenerg Biomembr. (1995) 27:151–9. doi: 10.1007/BF02110030
129. Rao N, Talwar R, Savithri H. Molecular organization, catalytic mechanism and function of serine hydroxymethyltransferase–a potential target for cancer chemotherapy. Int J Biochem Cell Biol. (2000) 32:405–16. doi: 10.1016/s1357-272500126-0
130. Hutson S. Structure and function of branched chain aminotransferases. Prog Nucleic Acid Res Mol Biol. (2001) 70:175–206. doi: 10.1016/s0079-660370017-7
131. Hoyumpa A. Mechanisms of vitamin deficiencies in alcoholism. Alcohol Clin Exp Res. (1986) 10:573–81. doi: 10.1111/j.1530-0277.1986.tb05147.x
132. Kannan K, Jain S. Effect of vitamin B6 on oxygen radicals, mitochondrial membrane potential, and lipid peroxidation in h2o2-treated u937 monocytes. Free Radic Biol Med. (2004) 36:423–8. doi: 10.1016/j.freeradbiomed.2003.09.012
133. Bryan J, Calvaresi E, Hughes D. Short-term folate, vitamin B-12 or vitamin B-6 supplementation slightly affects memory performance but not mood in women of various ages. J Nutr. (2002) 132:1345–56. doi: 10.1093/jn/132.6.1345
134. Bilski P, Li M, Ehrenshaft M, Daub M, Chignell C. Vitamin B6 (pyridoxine) and its derivatives are efficient singlet oxygen quenchers and potential fungal antioxidants. Photochem Photobiol. (2000) 71:129–34. doi: 10.1562/0031-865520000712.0.co;2
135. Ehrenshaft M, Bilski P, Li M, Chignell C, Daub M. A highly conserved sequence is a novel gene involved in de novo vitamin b6 biosynthesis. Proc Natl Acad Sci U.S.A. (1999) 96:9374–8. doi: 10.1073/pnas.96.16.9374
136. Said H. Recent advances in carrier-mediated intestinal absorption of water-soluble vitamins. Ann Rev Physiol. (2004) 66:419–46. doi: 10.1146/annurev.physiol.66.032102.144611
137. Fitzpatrick T, Amrhein N, Kappes B, Macheroux P, Tews I, Raschle T. Two independent routes of de novo vitamin B6 biosynthesis: not that different after all. Biochem J. (2007) 407:1–13. doi: 10.1042/BJ20070765
138. Ulvik A, Ebbing M, Hustad S, Midttun O, Nygard O, Vollset S, et al. Long- and short-term effects of tobacco smoking on circulating concentrations of B vitamins. Clin Chem. (2010) 56:755–63. doi: 10.1373/clinchem.2009.137513
139. Reininghaus E, Platzer M, Kohlhammer-Dohr A, Hamm C, Morkl S, Bengesser S, et al. Provit: supplementary probiotic treatment and vitamin b7 in depression-a randomized controlled trial. Nutrients. (2020) 12:422. doi: 10.3390/nu12113422
140. Davis S. Formulation strategies for absorption windows. Drug Discov Today. (2005) 10:249–57. doi: 10.1016/S1359-644603351-3
141. Said H, Ortiz A, Subramanian V, Neufeld E, Moyer M, Dudeja P. Mechanism of thiamine uptake by human colonocytes: studies with cultured colonic epithelial cell line ncm460. Am J Physiol Gastrointest Liver Physiol. (2001) 281:G144–50. doi: 10.1152/ajpgi.2001.281.1.G144
142. Said H, Ortiz A, Kumar C, Chatterjee N, Dudeja P, Rubin S. Transport of thiamine in human intestine: mechanism and regulation in intestinal epithelial cell model Caco-2. Am J Physiol. (1999) 277:C645–51. doi: 10.1152/ajpcell.1999.277.4.C645
143. Rothschild D, Weissbrod O, Barkan E, Kurilshikov A, Korem T, Zeevi D, et al. Environment dominates over host genetics in shaping human gut microbiota. Nature. (2018) 555:210–5. doi: 10.1038/nature25973
144. Bajaj J, Idilman R, Mabudian L, Hood M, Fagan A, Turan D, et al. Diet affects gut microbiota and modulates hospitalization risk differentially in an international cirrhosis cohort. Hepatology. (2018) 68:234–47. doi: 10.1002/hep.29791
145. Zhang H, Wang Q, Fisher D, Cai M, Chakravartty V, Ye H, et al. Deciphering a unique biotin scavenging pathway with redundant genes in the probiotic bacterium Lactococcus lactis. Sci Rep. (2016) 6:25680. doi: 10.1038/srep25680
146. Ferrer M, Ruiz A, Lanza F, Haange S, Oberbach A, Till H, et al. Microbiota from the distal guts of lean and obese adolescents exhibit partial functional redundancy besides clear differences in community structure. Environ Microbiol. (2013) 15:211–26. doi: 10.1111/j.1462-2920.2012.02845.x
147. Malo M, Moaven O, Muhammad N, Biswas B, Alam S, Economopoulos K, et al. Intestinal Alkaline phosphatase promotes gut bacterial growth by reducing the concentration of luminal nucleotide triphosphates. Am J Physiol Gastrointest Liver Physiol. (2014) 306:G826–38. doi: 10.1152/ajpgi.00357.2013
148. Mayengbam S, Chleilat F, Reimer R. Dietary vitamin B6 deficiency impairs gut microbiota and host and microbial metabolites in rats. Biomedicines. (2020) 8:10469. doi: 10.3390/biomedicines8110469
149. Said H. Biotin: biochemical, physiological and clinical aspects. Subcell Biochem. (2012) 56:1–19. doi: 10.1007/978-94-007-2199-9_1
150. Ghosal A, Jellbauer S, Kapadia R, Raffatellu M, Said H. Salmonella infection inhibits intestinal biotin transport: cellular and molecular mechanisms. Am J Physiol Gastrointest Liver Physiol. (2015) 309:G123–31. doi: 10.1152/ajpgi.00112.2015
151. Patel D, Swink S, Castelo-Soccio LA. Review of the use of biotin for hair loss. Skin Appendage Disord. (2017) 3:166–9. doi: 10.1159/000462981
152. Said H, Redha R, Nylander W. Biotin transport in the human intestine: site of maximum transport and effect of Ph. Gastroenterology. (1988) 95:1312–7. doi: 10.1016/0016-508590366-6
153. Belda E, Voland L, Tremaroli V, Falony G, Adriouch S, Assmann K, et al. Impairment of gut microbial biotin metabolism and host biotin status in severe obesity: effect of biotin and prebiotic supplementation on improved metabolism. Gut. (2022) 2021:325753. doi: 10.1136/gutjnl-2021-325753
154. Fisher D, Fernandez R, Adams N, Maurelli A. Uptake of biotin by chlamydia spp. through the use of a bacterial transporter (bioy) and a host-cell transporter (smvt). PLoS One. (2012) 7:e46052. doi: 10.1371/journal.pone.0046052
155. Hayashi A, Mikami Y, Miyamoto K, Kamada N, Sato T, Mizuno S, et al. Intestinal dysbiosis and biotin deprivation induce alopecia through overgrowth of lactobacillus murinus in mice. Cell Rep. (2017) 20:1513–24. doi: 10.1016/j.celrep.2017.07.057
156. Naderi N, House J. Recent developments in folate nutrition. Adv Food Nutr Res. (2018) 83:195–213. doi: 10.1016/bs.afnr.2017.12.006
157. Dary O. Nutritional interpretation of folic acid interventions. Nutr Rev. (2009) 67:235–44. doi: 10.1111/j.1753-4887.2009.00193.x
158. Pietrzik K, Bailey L, Shane B. Folic acid and L-5-methyltetrahydrofolate: comparison of clinical pharmacokinetics and pharmacodynamics. Clin Pharmacokinet. (2010) 49:535–48. doi: 10.2165/11532990-000000000-00000
159. McNulty H, Scott J. Intake and status of folate and related B-vitamins: considerations and challenges in achieving optimal status. Br J Nutr. (2008) 99:S48–54. doi: 10.1017/S0007114508006855
161. Wallingford J, Niswander L, Shaw G, Finnell R. The continuing challenge of understanding, preventing, and treating neural tube defects. Science. (2013) 339:1222002. doi: 10.1126/science.1222002
162. Troen A. Folate and vitamin B12: function and importance in cognitive development. Nestle Nutr Inst Workshop Ser. (2012) 70:161–71. doi: 10.1159/000337684
163. Ericson U, Sonestedt E, Gullberg B, Olsson H, Wirfalt E. High folate intake is associated with lower breast cancer incidence in postmenopausal women in the malmo diet and cancer cohort. Am J Clin Nutr. (2007) 86:434–43. doi: 10.1093/ajcn/86.2.434
164. Cole B, Baron J, Sandler R, Haile R, Ahnen D, Bresalier R, et al. Folic acid for the prevention of colorectal adenomas: a randomized clinical trial. JAMA. (2007) 297:2351–9. doi: 10.1001/jama.297.21.2351
165. Graham I, Daly L, Refsum H, Robinson K, Brattstrom L, Ueland P, et al. Plasma homocysteine as a risk factor for vascular disease. The european concerted action project. JAMA. (1997) 277:1775–81. doi: 10.1001/jama.1997.03540460039030
166. House J, Braun K, Ballance D, O’Connor C, Guenter W. The enrichment of eggs with folic acid through supplementation of the laying hen diet. Poult Sci. (2002) 81:1332–7. doi: 10.1093/ps/81.9.1332
167. Chandler C, Wang T, Halsted C. Pteroylpolyglutamate hydrolase from human jejunal brush borders. purification and characterization. J Biol Chem. (1986) 261:928–33.
168. Visentin M, Diop-Bove N, Zhao R, Goldman I. The intestinal absorption of folates. Annu Rev Physiol. (2014) 76:251–74. doi: 10.1146/annurev-physiol-020911-153251
169. Zhao R, Diop-Bove N, Visentin M, Goldman I. Mechanisms of membrane transport of folates into cells and across epithelia. Annu Rev Nutr. (2011) 31:177–201. doi: 10.1146/annurev-nutr-072610-145133
170. Qiu A, Jansen M, Sakaris A, Min S, Chattopadhyay S, Tsai E, et al. Identification of an intestinal folate transporter and the molecular basis for hereditary folate malabsorption. Cell. (2006) 127:917–28. doi: 10.1016/j.cell.2006.09.041
171. Lakoff A, Fazili Z, Aufreiter S, Pfeiffer C, Connolly B, Gregory J III, et al. Folate is absorbed across the human colon: evidence by using enteric-coated caplets containing 13c-labeled [6s]-5-formyltetrahydrofolate. Am J Clin Nutr. (2014) 100:1278–86. doi: 10.3945/ajcn.114.091785
172. Engevik M, Morra C, Roth D, Engevik K, Spinler J, Devaraj S, et al. Microbial metabolic capacity for intestinal folate production and modulation of host folate receptors. Front Microbiol. (2019) 10:2305. doi: 10.3389/fmicb.2019.02305
173. Chave K, Ryan T, Chmura S, Galivan J. Identification of single nucleotide polymorphisms in the human gamma-glutamyl hydrolase gene and characterization of promoter polymorphisms. Gene. (2003) 319:167–75. doi: 10.1016/s0378-111900807-2
174. Hill M. Intestinal flora and endogenous vitamin synthesis. Eur J Cancer Prev. (1997) 6:S43–5. doi: 10.1097/00008469-199703001-00009
175. Pompei A, Cordisco L, Amaretti A, Zanoni S, Matteuzzi D, Rossi M. Folate production by bifidobacteria as a potential probiotic property. Appl Environ Microbiol. (2007) 73:179–85. doi: 10.1128/AEM.01763-06
176. Liu M, Chen Q, Sun Y, Zeng L, Wu H, Gu Q, et al. Probiotic potential of a folate-producing strain latilactobacillus sakei lz217 and its modulation effects on human gut microbiota. Foods. (2022) 11:234. doi: 10.3390/foods11020234
177. Zhang J, Cai D, Yang M, Hao Y, Zhu Y, Chen Z, et al. Screening of folate-producing lactic acid bacteria and modulatory effects of folate-biofortified yogurt on gut dysbacteriosis of folate-deficient rats. Food Funct. (2020) 11:6308–18. doi: 10.1039/d0fo00480d
178. Chen S, Yang M, Wang R, Fan X, Tang T, Li P, et al. Suppression of high-fat-diet-induced obesity in mice by dietary folic acid supplementation is linked to changes in gut microbiota. Eur J Nutr. (2022) 61:2015–31. doi: 10.1007/s00394-021-02769-9
179. Wang L, Zou L, Li J, Yang H, Yin Y. Effect of dietary folate level on organ weight, digesta ph, short-chain fatty acid concentration, and intestinal microbiota of weaned piglets. J Anim Sci. (2021) 99:15. doi: 10.1093/jas/skab015
180. Hibberd M, Wu M, Rodionov D, Li X, Cheng J, Griffin N, et al. The effects of micronutrient deficiencies on bacterial species from the human gut microbiota. Sci Transl Med. (2017) 9:390. doi: 10.1126/scitranslmed.aal4069
181. Malinowska A, Schmidt M, Kok D, Chmurzynska A. Ex vivo folate production by fecal bacteria does not predict human blood folate status: associations between dietary patterns, gut microbiota, and folate metabolism. Food Res Int. (2022) 156:111290. doi: 10.1016/j.foodres.2022.111290
182. Lurz E, Horne R, Maattanen P, Wu R, Botts S, Li B, et al. Vitamin B12 deficiency alters the gut microbiota in a murine model of colitis. Front Nutr. (2020) 7:83. doi: 10.3389/fnut.2020.00083
183. Watanabe F, Bito T. Vitamin B12 sources and microbial interaction. Exp Biol Med. (2018) 243:148–58. doi: 10.1177/1535370217746612
184. Obeid R, Heil S, Verhoeven M, van den Heuvel E, de Groot L, Eussen S. Vitamin B12 intake from animal foods, biomarkers, and health aspects. Front Nutr. (2019) 6:93. doi: 10.3389/fnut.2019.00093
185. Vincenti A, Bertuzzo L, Limitone A, D’Antona G, Cena H. Perspective: practical approach to preventing subclinical B12 deficiency in elderly population. Nutrients. (2021) 13:913. doi: 10.3390/nu13061913
186. Wald D, Law M, Morris J. Homocysteine and cardiovascular disease: evidence on causality from a meta-analysis. BMJ. (2002) 325:1202. doi: 10.1136/bmj.325.7374.1202
187. Holmes M, Newcombe P, Hubacek J, Sofat R, Ricketts S, Cooper J, et al. Effect Modification by Population Dietary Folate on the Association between Mthfr Genotype, Homocysteine, and Stroke Risk: A Meta-Analysis of Genetic Studies and Randomised Trials. Lancet. (2011) 378:584–94. doi: 10.1016/S0140-673660872-6
188. Boumenna T, Scott T, Lee J, Palacios N, Tucker K. Folate, vitamin B-12, and cognitive function in the boston puerto rican health study. Am J Clin Nutr. (2020) 2020:293. doi: 10.1093/ajcn/nqaa293
189. Bailey R, Jun S, Murphy L, Green R, Gahche J, Dwyer J, et al. High folic acid or folate combined with low vitamin B-12 status: potential but inconsistent association with cognitive function in a nationally representative cross-sectional sample of us older adults participating in the nhanes. Am J Clin Nutr. (2020) 112:1547–57. doi: 10.1093/ajcn/nqaa239
190. Stein J, Geisel J, Obeid R. Association between neuropathy and B-vitamins: a systematic review and meta-analysis. Eur J Neurol. (2021) 28:2054–64. doi: 10.1111/ene.14786
191. Herrmann M, Peter Schmidt J, Umanskaya N, Wagner A, Taban-Shomal O, Widmann T, et al. The role of hyperhomocysteinemia as well as folate, vitamin b and b deficiencies in osteoporosis: a systematic review. Clin Chem Lab Med. (2007) 45:1621–32. doi: 10.1515/CCLM.2007.362
192. Nowak M, Swietochowska E, Wielkoszynski T, Marek B, Kos-Kudla B, Szapska B, et al. Homocysteine, vitamin B12, and folic acid in age-related macular degeneration. Eur J Ophthalmol. (2005) 15:764–7.
193. Rochtchina E, Wang J, Flood V, Mitchell P. Elevated serum homocysteine, low serum vitamin B12, folate, and age-related macular degeneration: the blue mountains eye study. Am J Ophthalmol. (2007) 143:344–6. doi: 10.1016/j.ajo.2006.08.032
194. Matteini A, Walston J, Fallin M, Bandeen-Roche K, Kao W, Semba R, et al. Markers of B-vitamin deficiency and frailty in older women. J Nutr Health Aging. (2008) 12:303–8. doi: 10.1007/BF02982659
195. Stabler S, Allen R. Vitamin B12 deficiency as a worldwide problem. Annu Rev Nutr. (2004) 24:299–326. doi: 10.1146/annurev.nutr.24.012003.132440
196. Gueant J, Gueant-Rodriguez R, Alpers D. Vitamin B12 absorption and malabsorption. Vitam Horm. (2022) 119:241–74. doi: 10.1016/bs.vh.2022.01.016
197. Kozyraki R, Cases O. Vitamin B12 absorption: mammalian physiology and acquired and inherited disorders. Biochimie. (2013) 95:1002–7. doi: 10.1016/j.biochi.2012.11.004
198. Green R, Allen L, Bjorke-Monsen A, Brito A, Gueant J, Miller J, et al. Vitamin B12 deficiency. Nat Rev Dis Primers. (2017) 3:17040. doi: 10.1038/nrdp.2017.40
199. Nielsen M, Rasmussen M, Andersen C, Nexo E, Moestrup S. Vitamin B12 transport from food to the body’s cells–a sophisticated, multistep pathway. Nat Rev Gastroenterol Hepatol. (2012) 9:345–54. doi: 10.1038/nrgastro.2012.76
200. Li P, Gu Q, Wang Y, Yu Y, Yang L, Chen J. Novel vitamin B12-producing enterococcus spp. and preliminary in vitro evaluation of probiotic potentials. Appl Microbiol Biotechnol. (2017) 101:6155–64. doi: 10.1007/s00253-017-8373-7
201. Gu Q, Zhang C, Song D, Li P, Zhu X. Enhancing vitamin B12 content in soy-yogurt by lactobacillus reuteri. Int J Food Microbiol. (2015) 206:56–9. doi: 10.1016/j.ijfoodmicro.2015.04.033
202. Molina V, Medici M, Taranto M. Font de valdez g. lactobacillus reuteri crl 1098 prevents side effects produced by a nutritional vitamin B deficiency. J Appl Microbiol. (2009) 106:467–73. doi: 10.1111/j.1365-2672.2008.04014.x
203. Degnan P, Taga M, Goodman A. Vitamin B12 as a modulator of gut microbial ecology. Cell Metab. (2014) 20:769–78. doi: 10.1016/j.cmet.2014.10.002
204. Degnan P, Barry N, Mok K, Taga M, Goodman A. Human gut microbes use multiple transporters to distinguish vitamin B analogs and compete in the gut. Cell Host Microbe. (2014) 15:47–57. doi: 10.1016/j.chom.2013.12.007
205. Murphy M, Sourial N, Burman J, Doyle D, Tabaqchali S, Mollin D. Megaloblastic anaemia due to vitamin B12 deficiency caused by small intestinal bacterial overgrowth: possible role of vitamin B12 analogues. Br J Haematol. (1986) 62:7–12. doi: 10.1111/j.1365-2141.1986.tb02894.x
206. Quigley E, Murray J, Pimentel M. Aga clinical practice update on small intestinal bacterial overgrowth: expert review. Gastroenterology. (2020) 159:1526–32. doi: 10.1053/j.gastro.2020.06.090
207. Woodard G, Encarnacion B, Downey J, Peraza J, Chong K, Hernandez-Boussard T, et al. Probiotics improve outcomes after roux-en-y gastric bypass surgery: a prospective randomized trial. J Gastrointest Surg. (2009) 13:1198–204. doi: 10.1007/s11605-009-0891-x
208. Barkhidarian B, Roldos L, Iskandar M, Saedisomeolia A, Kubow S. Probiotic supplementation and micronutrient status in healthy subjects: a systematic review of clinical trials. Nutrients. (2021) 13:3001. doi: 10.3390/nu13093001
209. Lam J, Schneider J, Zhao W, Corley D. Proton pump inhibitor and histamine 2 receptor antagonist use and vitamin B12 deficiency. JAMA. (2013) 310:2435–42. doi: 10.1001/jama.2013.280490
210. Brzozowski T, Konturek P, Mierzwa M, Drozdowicz D, Bielanski W, Kwiecien S, et al. Effect of probiotics and triple eradication therapy on the cyclooxygenase (cox)-2 expression, apoptosis, and functional gastric mucosal impairment in Helicobacter pylori-infected mongolian gerbils. Helicobacter. (2006) 11:10–20. doi: 10.1111/j.0083-8703.2006.00373.x
211. Sanders M, Merenstein D, Reid G, Gibson G, Rastall R. Probiotics and prebiotics in intestinal health and disease: from biology to the clinic. Nat Rev Gastroenterol Hepatol. (2019) 16:605–16. doi: 10.1038/s41575-019-0173-3
212. Wexler A, Schofield W, Degnan P, Folta-Stogniew E, Barry N, Goodman A. Human gut Bacteroides capture vitamin B12 via cell surface-exposed lipoproteins. Elife. (2018) 7:37138. doi: 10.7554/eLife.37138
213. Sokolovskaya O, Shelton A, Taga M. Sharing vitamins: cobamides unveil microbial interactions. Science. (2020) 369:165. doi: 10.1126/science.aba0165
214. Kang Z, Zhang J, Zhou J, Qi Q, Du G, Chen J. Recent advances in microbial production of delta-aminolevulinic acid and vitamin B12. Biotechnol Adv. (2012) 30:1533–42. doi: 10.1016/j.biotechadv.2012.04.003
215. Guetterman H, Huey S, Knight R, Fox A, Mehta S, Finkelstein J. Vitamin B-12 and the gastrointestinal microbiome: a systematic review. Adv Nutr. (2021) 2021:123. doi: 10.1093/advances/nmab123
216. Goodman A, McNulty N, Zhao Y, Leip D, Mitra R, Lozupone C, et al. Identifying genetic determinants needed to establish a human gut symbiont in its habitat. Cell Host Microbe. (2009) 6:279–89. doi: 10.1016/j.chom.2009.08.003
217. Wang H, Shou Y, Zhu X, Xu Y, Shi L, Xiang S, et al. Stability of vitamin B12 with the protection of whey proteins and their effects on the gut microbiome. Food Chem. (2019) 276:298–306. doi: 10.1016/j.foodchem.2018.10.033
218. Xu Y, Xiang S, Ye K, Zheng Y, Feng X, Zhu X, et al. Cobalamin (vitamin B12) induced a shift in microbial composition and metabolic activity in an in vitro colon simulation. Front Microbiol. (2018) 9:2780. doi: 10.3389/fmicb.2018.02780
219. Zhu X, Xiang S, Feng X, Wang H, Tian S, Xu Y, et al. Impact of cyanocobalamin and methylcobalamin on inflammatory bowel disease and the intestinal microbiota composition. J Agric Food Chem. (2019) 67:916–26. doi: 10.1021/acs.jafc.8b05730
220. Kelly C, Alexeev E, Farb L, Vickery T, Zheng L, Eric L, et al. Oral vitamin B12 supplement is delivered to the distal gut, altering the corrinoid profile and selectively depleting Bacteroides in C57bl/6 mice. Gut Microbes. (2019) 10:654–62. doi: 10.1080/19490976.2019.1597667
221. Park S, Kang S, Sol Kim D. Folate and vitamin B-12 deficiencies additively impaire memory function and disturb the gut microbiota in amyloid-beta infused rats. Int J Vitam Nutr Res. (2022) 92:169–81. doi: 10.1024/0300-9831/a000624
222. Herman D, Rhoades N, Mercado J, Argueta P, Lopez U, Flores G. Dietary habits of 2- to 9-year-old american children are associated with gut microbiome composition. J Acad Nutr Diet. (2020) 120:517–34. doi: 10.1016/j.jand.2019.07.024
223. Boran P, Baris H, Kepenekli E, Erzik C, Soysal A, Dinh D. The impact of vitamin B12 deficiency on infant gut microbiota. Eur J Pediatr. (2020) 179:385–93. doi: 10.1007/s00431-019-03517-2
224. Shah N, Chakradeo P, Engen P, Keshavarzian A, Mutlu E. Dietary patterns and mucosa associated colonic bacterial microbiota and stool microbiota in healthy subjects. Gastroenterology. (2017) 152:S1. doi: 10.1016/S0016-508530384-0
225. Seura T, Yoshino Y, Fukuwatari T. The relationship between habitual dietary intake and gut microbiota in young japanese women. J Nutr Sci Vitaminol. (2017) 63:396–404. doi: 10.3177/jnsv.63.396
226. Babakobi M, Reshef L, Gihaz S, Belgorodsky B, Fishman A, Bujanover Y, et al. Effect of maternal diet and milk lipid composition on the infant gut and maternal milk microbiomes. Nutrients. (2020) 12:2539. doi: 10.3390/nu12092539
227. Mandal S, Godfrey K, McDonald D, Treuren W, Bjornholt J, Midtvedt T, et al. Fat and vitamin intakes during pregnancy have stronger relations with a pro-inflammatory maternal microbiota than does carbohydrate intake. Microbiome. (2016) 4:55. doi: 10.1186/s40168-016-0200-3
228. Zheng Y, Xiang S, Zhang H, Ye K, Zhang Y, Ge Y, et al. Vitamin B12 enriched in spinach and its effects on gut microbiota. J Agric Food Chem. (2021) 69:2204–12. doi: 10.1021/acs.jafc.0c07597
229. Ge Y, Zadeh M, Mohamadzadeh M. Vitamin B12 coordinates ileal epithelial cell and microbiota functions to resist Salmonella infection in mice. J Exp Med. (2022) 219:57. doi: 10.1084/jem.20220057
230. Sklan D, Trostler N. Site and extent of thiamin absorption in the rat. J Nutr. (1977) 107:353–6. doi: 10.1093/jn/107.3.353
231. Mosegaard S, Dipace G, Bross P, Carlsen J, Gregersen N, Olsen R. Riboflavin deficiency-implications for general human health and inborn errors of metabolism. Int J Mol Sci. (2020) 21:3847. doi: 10.3390/ijms21113847
232. Middleton H. Uptake of pyridoxine hydrochloride by the rat jejunal mucosa in vitro. J Nutr. (1977) 107:126–31. doi: 10.1093/jn/107.1.126
233. Turner J. Pyridoxal phosphate breakdown by an alkaline-phosphatase preparation. Biochem J. (1961) 80:663–8. doi: 10.1042/bj0800663
234. Wilson R, Davis R. Clinical chemistry of vitamin B6. Adv Clin Chem. (1983) 23:1–68. doi: 10.1016/s0065-242360397-2
235. Ragaller V, Lebzien P, Bigalke W, Sudekum K, Huther L, Flachowsky G. Effects of a pantothenic acid supplementation to different rations on ruminal fermentation, nutrient flow at the duodenum, and on blood and milk variables of dairy cows. J Anim Physiol Anim Nutr. (2011) 95:730–43. doi: 10.1111/j.1439-0396.2010.01103.x
236. Taranto M, Vera J, Hugenholtz J, De Valdez G, Sesma F. Lactobacillus reuteri Crl1098 produces cobalamin. J Bacteriol. (2003) 185:5643–7. doi: 10.1128/JB.185.18.5643-5647.2003
237. Santos F, Wegkamp A, de Vos W, Smid E, Hugenholtz J. High-level folate production in fermented foods by the B12 producer Lactobacillus reuteri JCM1112. Appl Environ Microbiol. (2008) 74:3291–4. doi: 10.1128/AEM.02719-07
238. Sriramulu D, Liang M, Hernandez-Romero D, Raux-Deery E, Lunsdorf H, Parsons J, et al. Lactobacillus reuteri Dsm 20016 produces cobalamin-dependent diol dehydratase in metabolosomes and metabolizes 1,2-propanediol by disproportionation. J Bacteriol. (2008) 190:4559–67. doi: 10.1128/JB.01535-07
239. Michielan A, D’Inca R. Intestinal permeability in inflammatory bowel disease: pathogenesis, clinical evaluation, and therapy of leaky gut. Mediators Inflamm. (2015) 2015:628157. doi: 10.1155/2015/628157
240. Gong D, Gong X, Wang L, Yu X, Dong Q. Involvement of reduced microbial diversity in inflammatory bowel disease. Gastroenterol Res Pract. (2016) 2016:6951091. doi: 10.1155/2016/6951091
241. Ananthakrishnan A. Epidemiology and risk factors for ibd. Nat Rev Gastroenterol Hepatol. (2015) 12:205–17. doi: 10.1038/nrgastro.2015.34
242. Chen Y, Zhang L, Hong G, Huang C, Qian W, Bai T, et al. Probiotic mixtures with aerobic constituent promoted the recovery of multi-barriers in dss-induced chronic colitis. Life Sci. (2020) 240:117089. doi: 10.1016/j.lfs.2019.117089
243. Mardini H, Grigorian A. Probiotic mix Vsl#3 is effective adjunctive therapy for mild to moderately active ulcerative colitis: a meta-analysis. Inflamm Bowel Dis. (2014) 20:1562–7. doi: 10.1097/MIB.0000000000000084
244. Selvamani S, Mehta V, Ali El Enshasy H, Thevarajoo S, El Adawi H, Zeini I, et al. Efficacy of probiotics-based interventions as therapy for inflammatory bowel disease: a recent update. Saudi J Biol Sci. (2022) 29:3546–67. doi: 10.1016/j.sjbs.2022.02.044
245. Xiao Y, Kashyap P. Microbially derived polyunsaturated fatty acid as a modulator of gastrointestinal motility. J Clin Invest. (2022) 132:1572. doi: 10.1172/JCI161572
246. Bhattarai Y, Williams B, Battaglioli E, Whitaker W, Till L, Grover M, et al. Gut microbiota-produced tryptamine activates an epithelial g-protein-coupled receptor to increase colonic secretion. Cell Host Microbe. (2018) 23:775–85e5. doi: 10.1016/j.chom.2018.05.004
247. Yano J, Yu K, Donaldson G, Shastri G, Ann P, Ma L, et al. Indigenous bacteria from the gut microbiota regulate host serotonin biosynthesis. Cell. (2015) 161:264–76. doi: 10.1016/j.cell.2015.02.047
248. Squires P, Rumsey R, Edwards C, Read N. Effect of short-chain fatty acids on contractile activity and fluid flow in rat colon in vitro. Am J Physiol. (1992) 262:G813–7. doi: 10.1152/ajpgi.1992.262.5.G813
249. Matsumoto K, Takada T, Shimizu K, Moriyama K, Kawakami K, Hirano K, et al. Effects of a probiotic fermented milk beverage containing lactobacillus casei strain shirota on defecation frequency, intestinal microbiota, and the intestinal environment of healthy individuals with soft stools. J Biosci Bioeng. (2010) 110:547–52. doi: 10.1016/j.jbiosc.2010.05.016
250. Veiga P, Pons N, Agrawal A, Oozeer R, Guyonnet D, Brazeilles R, et al. Changes of the human gut microbiome induced by a fermented milk product. Sci Rep. (2014) 4:6328. doi: 10.1038/srep06328
251. Anitha M, Vijay-Kumar M, Sitaraman S, Gewirtz A, Srinivasan S. Gut microbial products regulate murine gastrointestinal motility via toll-like receptor 4 signaling. Gastroenterology. (2012) 143:1006–16e4. doi: 10.1053/j.gastro.2012.06.034
252. Singh P, Grabauskas G, Zhou S, Gao J, Zhang Y, Owyang C. High fodmap diet causes barrier loss via lipopolysaccharide-mediated mast cell activation. JCI Insight. (2021) 6:146529. doi: 10.1172/jci.insight.146529
253. Bar F, Von Koschitzky H, Roblick U, Bruch H, Schulze L, Sonnenborn U, et al. Cell-free supernatants of Escherichia coli Nissle 1917 modulate human colonic motility: evidence from an in vitro organ bath study. Neurogastroenterol Motil. (2009) 21:559–66. doi: 10.1111/j.1365-2982.2008.01258.x
254. Kunze W, Mao Y, Wang B, Huizinga J, Ma X, Forsythe P, et al. Lactobacillus reuteri enhances excitability of colonic ah neurons by inhibiting calcium-dependent potassium channel opening. J Cell Mol Med. (2009) 13:2261–70. doi: 10.1111/j.1582-4934.2009.00686.x
255. Bugaytsova J, Bjornham O, Chernov Y, Gideonsson P, Henriksson S, Mendez M, et al. Helicobacter pylori adapts to chronic infection and gastric disease via ph-responsive baba-mediated adherence. Cell Host Microbe. (2017) 21:376–89. doi: 10.1016/j.chom.2017.02.013
256. Ghosh S, Singh R, Vanwinkle Z, Guo H, Vemula P, Goel A, et al. Microbial metabolite restricts 5-fluorouracil-resistant colonic tumor progression by sensitizing drug transporters via regulation of foxo3-foxm1 axis. Theranostics. (2022) 12:5574–95. doi: 10.7150/thno.70754
257. Selma M, Beltran D, Garcia-Villalba R, Espin J, Tomas-Barberan F. Description of urolithin production capacity from ellagic acid of two human intestinal gordonibacter species. Food Funct. (2014) 5:1779–84. doi: 10.1039/c4fo00092g
258. Lyczak J, Pier G. Salmonella enterica serovar typhi modulates cell surface expression of its receptor, the cystic fibrosis transmembrane conductance regulator, on the intestinal epithelium. Infect Immun. (2002) 70:6416–23. doi: 10.1128/IAI.70.11.6416-6423.2002
259. Siccardi D, Mumy K, Wall D, Bien J, McCormick B. Salmonella enterica serovar typhimurium modulates p-glycoprotein in the intestinal epithelium. Am J Physiol Gastrointest Liver Physiol. (2008) 294:G1392–400. doi: 10.1152/ajpgi.00599.2007
260. Pazos M, Siccardi D, Mumy K, Bien J, Louie S, Shi H, et al. Multidrug resistance-associated transporter 2 regulates mucosal inflammation by facilitating the synthesis of hepoxilin A3. J Immunol. (2008) 181:8044–52. doi: 10.4049/jimmunol.181.11.8044
261. Kles K, Chang E. Short-chain fatty acids impact on intestinal adaptation, inflammation, carcinoma, and failure. Gastroenterology. (2006) 130:S100–5. doi: 10.1053/j.gastro.2005.11.048
Keywords: vitamin B, gut microbiota, interaction, absorption, human health
Citation: Wan Z, Zheng J, Zhu Z, Sang L, Zhu J, Luo S, Zhao Y, Wang R, Zhang Y, Hao K, Chen L, Du J, Kan J and He H (2022) Intermediate role of gut microbiota in vitamin B nutrition and its influences on human health. Front. Nutr. 9:1031502. doi: 10.3389/fnut.2022.1031502
Received: 30 August 2022; Accepted: 28 November 2022;
Published: 13 December 2022.
Edited by:
Mercedes G. López, Instituto Politécnico Nacional de México (CINVESTAV), MexicoReviewed by:
Diana Ir, University of Colorado Anschutz Medical Campus, United StatesRuihua Dong, Fudan University, China
Copyright © 2022 Wan, Zheng, Zhu, Sang, Zhu, Luo, Zhao, Wang, Zhang, Hao, Chen, Du, Kan and He. This is an open-access article distributed under the terms of the Creative Commons Attribution License (CC BY). The use, distribution or reproduction in other forums is permitted, provided the original author(s) and the copyright owner(s) are credited and that the original publication in this journal is cited, in accordance with accepted academic practice. No use, distribution or reproduction is permitted which does not comply with these terms.
*Correspondence: Juntao Kan, anVub3Qua2FuQGFtd2F5LmNvbQ==; Hua He, aHVhaGVfY3B1cGtAY3B1LmVkdS5jbg==
†These authors have contributed equally to this work and share first authorship