- 1Interdisciplinary Program in Biotechnology, Graduate School, Chiang Mai University, Chiang Mai, Thailand
- 2Faculty of Agro-Industry, Chiang Mai University, Chiang Mai, Thailand
- 3Cluster of Agro Bio-Circular-Green Industry (Agro-BCG), Chiang Mai University, Chiang Mai, Thailand
- 4College of Maritime Studies and Management, Chiang Mai University, Samut Sakhon, Thailand
- 5Plant Bioactive Compound Laboratory (BAC), Department of Plant and Soil Sciences, Faculty of Agriculture, Chiang Mai University, Chiang Mai, Thailand
- 6Department of Marine Food Science and Technology, Gangneung-Wonju National University, Gangneung, South Korea
- 7Department of Food Science, College of Agriculture and Life Science, Cornell University, Ithaca, NY, United States
- 8Department of Preventive Medicine and Public Health, Food Science, Toxicology and Forensic Medicine, Faculty of Pharmacy, Universitat de València, Valencia, Spain
Viral infections may cause serious human diseases. For instance, the recent appearance of the novel virus, SARS-CoV-2, causing COVID-19, has spread globally and is a serious public health concern. The consumption of healthy, proper, functional, and nutrient-rich foods has an important role in enhancing an individual's immune system and preventing viral infections. Several polysaccharides from natural sources such as algae, bacteria, and fungi have been considered as generally recognized as safe (GRAS) by the US Food and Drug Administration. They are safe, low-toxicity, biodegradable, and have biological activities. In this review, the bioactive polysaccharides derived from various microorganisms, including bacteria, fungi, and algae were evaluated. Antiviral mechanisms of these polysaccharides were discussed. Finally, the potential use of microbial and algal polysaccharides as an antiviral and immune boosting strategy was addressed. The microbial polysaccharides exhibited several bioactivities, including antioxidant, anti-inflammatory, antimicrobial, antitumor, and immunomodulatory activities. Some microbes are able to produce sulfated polysaccharides, which are well-known to exert a board spectrum of biological activities, especially antiviral properties. Microbial polysaccharide can inhibit various viruses using different mechanisms. Furthermore, these microbial polysaccharides are also able to modulate immune responses to prevent and/or inhibit virus infections. There are many molecular factors influencing their bioactivities, e.g., functional groups, conformations, compositions, and molecular weight. At this stage of development, microbial polysaccharides will be used as adjuvants, nutrient supplements, and for drug delivery to prevent several virus infections, especially SARS-CoV-2 infection.
Introduction
Viruses are the most numerous living organisms on the earth and can be found in terrestrial and aquatic environments. They are infectious agents containing a genetic material within a protein coat—requiring an appropriate host cell where they can replicate (called infection) often resulting in diseases. Viruses can infect all types of organisms: prokaryotes (archaea and bacteria), eukaryotes (animals, algae, plants, and protozoa), and giant viruses namely virophages (1). Like other viruses, human viruses are able to replicate and mutate. A new virus was discovered in December 2019 and characterized as a pandemic by World Health Organization (WHO) on March 11, 2020 (2). This virus was characterized as the severe acute respiratory syndrome coronavirus 2 (SARS-CoV-2), which causes human infection called coronavirus disease 2019 (COVID-19). The disease has spread worldwide and caused over 200 million cases and 4 million deaths from its start until August 2021 (3).
Coronaviruses (CoV) are enveloped positive-sense single-stranded RNA (+ ssRNA) viruses with crown-like spikes on their spherical surface (4). Coronaviruses belong to the order Nidovirales, the suborder Coronavirineae, and the family Caronaviridae. The family was divided into the subfamilies of Orthocoronavirinae and Letovirinae by the International Committee on Taxonomy of Viruses (ICTV) in 2018 (5). The former sorts into 4 genera, including Alphacoronavirus, Betacoronavirus, Gammacoronavirus, and Deltacoronavirus, with α- and β-coronaviruses infect mammalian species, but γ-, and δ-coronaviruses infect avian species causing respiratory and enteric diseases both as acute and persistent infections (6, 7). The human coronavirus first emerged in patients with the common cold in the 1960s. Other human coronaviruses have emerged within the last two decades: SARS-CoV-1 (2003), Middle East respiratory syndrome (MERS-CoV, 2012), as well as SARS-CoV-2 (2019), which is the seventh human-infecting coronavirus identified (7).
SARS-CoV-2 belongs to the β-coronaviruses, which include SARS-CoV-1 and MERS-CoV. These viruses are highly pathogenic and have a high mortality rate (8). The RNA genome of SARS-CoV-2 is 25–32 kb and similar to SARS-CoV-1 (82% similarity) (4). The structural proteins of SARS-CoV-2 are the envelope (E), membrane (M), nucleocapsid (N), and spike (S) proteins (Figure 1). The spike of coronaviruses (S protein) is a glycoprotein associated with the pathogenesis because it is involved in virus adsorption and entry. Thus, the S protein is the virus' important virulence factor (5). The virus uses the S protein for cell binding and membrane fusion. The S protein binds to the angiotensin-converting enzyme 2 (ACE2), a host cell receptor, primed by the transmembrane protease serine protease 2 (TMPRSS2), with the interaction mediating the virus attachment and entry into a host cell (5, 6, 9). The ACE2 receptor is found in a range of human tissues and organs, including the small intestine, lungs, heart, testis, kidneys, blood vessels, muscle, adipose tissues, bladder, epithelia cells of the oral cavity, and the upper esophagus (10–12). Therefore, there are many targets for SARS-CoV-2 infection.
Four antiviral drugs were repurposed for COVID-19, including tocilizumab, remdesivir, favipiravir, and dexamethasone (13). Remdesivir, a drug approved by the US Food and Drug Administration (FDA) for COVID-19, showed a high efficacy as a COVID-19 treatment (14, 15). On the other hand, the WHO Solidarity Trial Consortium announced that remdesivir had little or no effect on hospitalized patients with COVID-19 (16). Various researchers have been evaluating and developing other antiviral agents, as well as vaccines, with high efficacy and low-toxicity (17). As of August 2021, people have been administered different Covid vaccines in many countries, but the pandemic still goes on. Nutrients in foods have an important role in stimulating human immunity and preventing viral infections. Several nutrients including polysaccharides, proteins, and lipids have been reported to have antiviral and immune-enhancing properties. In addition, micronutrients, such as vitamin A, C, D, and E, and few minerals, such as iron, selenium, and zinc have the potential to improve the immune system. Furthermore, natural extracts containing some non-nutrients, such as polyphenols, flavonoids, alkaloids, thiophenes, terpenoids, tannins, and lignins have also shown biological activities (18). For instance, Erodium glaucophyllum extracts containing phenolic compounds such as gallic acid, quercetin 3-O-glucuronide, (+)-gallocatechin, and (+)-catechin exhibited antibacterial and antiviral activities (19). During the COVID-19 pandemic, healthy, nutrient-rich, and functional foods may be more important because their consumption may prevent and modulate the immune system (20). In addition, the development of bioactive ingredients, functional, and nutrient-rich foods that can moderate consumers' overall health will be more interested (21).
Polysaccharides are polymeric carbohydrates, defined as composed of more than 10 monomers that are linked by glycosidic linkages. Polysaccharides are grouped into 2 classes: homopolysaccharides (contain one type of monomer) and heteropolysaccharide (contain more than one type of monomer) (22). Polysaccharides from each sources have different branched chains, composition of monosaccharides, molecular weight (MW), and structural conformations (23). Polysaccharides are the most abundant biological macromolecules in nature and can be obtained from every living organisms including animals (24), plants (25), and microorganisms (26). In living cells, polysaccharides are involved in structure, storage, adhesion, and cell recognition (27). Microorganisms including archaea, bacteria, fungi, and microalgae produced diverse polysaccharides with different structures and functions. Moreover, microorganisms synthesize polysaccharides and secrete them to the outside, these are called exopolysaccharides (EPS). Their functions include cell adhesion, migration of bacteria in groundwater, protection from predators and white blood cells, protection from undesired environments (extreme environments), intercellular signal transduction, and molecular recognition (28, 29). Microbial polysaccharides are composed of not only monosaccharides, but also proteins, lipids, metal ions, extracellular DNA (eDNA), and other organic and inorganic compounds (30). Furthermore, polysaccharides derived from microorganisms, especially marine microorganisms may include sulfate groups, and are called sulfated polysaccharides (31). Sulfated polysaccharides are negatively-charged biopolymers found in the cell wall of marine algae (green, brown, and red algae). Sulfate groups are linked to the sugar structure's backbone to stabilize the structure in extreme environments, especially high salinity (32). Sulfated polysaccharides can be founded not only in marine microalgae and macroalgae, but also in marine animals, and marine bacteria (31). Microbial polysaccharides and sulfated polysaccharides show various biological activities such as immunomodulatory, antioxidant, antimicrobial, anticancer, and anti-inflammatory activities (30). In particular, the antiviral activity of microbial polysaccharides has been studied, showing in several cases an inhibitory effect against various animal, human, and plant pathogenic viruses (33–35). Many studies have reported that natural and modified polysaccharides could inhibit various virus infections (36). Some microbial polysaccharides had antiviral activity against various viruses including Herpes simplex, influenza, Newcastle disease (NDV), Varicella zoster (VZV), human immunodeficiency viruses (HIV), and human adenoviruses (37–45). According to their biological activities, the bioactive polysaccharides can be applied as a bioactive ingredients to improve the immune system and reduce the damage caused by viruses (23). Among microbial polysaccharides, EPS produced by lactic acid bacteria (LAB) have been recognized as GRAS, which allows their use in food without the need for regulatory oversight in the USA (46). Although bioactive polysaccharides have been derived from plants, many researchers have investigated the characteristics, compositions, properties, biological activities of novel polysaccharides from various microorganisms (30). There are many advantages to using microbial polysaccharides compared to other polysaccharides. For example, the microbial polysaccharide production can be done using optimized conditions indoors. Microbes grow easily and fast with a high yield of polysaccharides. The recovery process of polysaccharides is simple. Moreover, microbial growth media are simple and non-toxic. If agricultural wastes are used as microbial growth media, the cost of the production is often decreased (32, 47). Microbial polysaccharides are biocompatible and biodegradable, and have no known toxic effects (23). As mentioned above, microbial polysaccharides show antioxidant, anti-inflammatory, antiviral, and immunomodulatory activities; therefore, microbial polysaccharides are attractive as antiviral agents or bioactive ingredients to treat viral infectious diseases, especially COVID-19. This review focuses on microbial polysaccharides with antiviral and immunomodulatory activities and their antiviral mechanisms, and provides the potential approach to use microbial polysaccharides as bioactive ingredients.
Microbial Polysaccharides With Antiviral Activity
Antiviral Polysaccharides From Algae
Algae are eukaryotic photosynthetic organisms, often microorganisms. Some algae are unicellular, but some of them are multicellular organisms lacking of specialized tissues. Both micro- and macroalgae are good sources of biomedical compounds, especially polysaccharides (48). Algal polysaccharides are nontoxic, edible, biocompatible, biodegradable, and easily available; therefore, these biopolymers have been applied in many fields such as the food, pharmaceutical, and biomedical industries (49). Algal polysaccharides have several pharmaceutical properties, including anticancer (50), antioxidant (51), antimicrobial (52), anti-inflammatory (53), and immunomodulatory activities (54). Moreover, several algae, especially marine algae, can produced sulfate polysaccharides, which have different beneficial biological activities (50, 55–58). Different algal polysaccharides possess a variety of structures, composition, and conformations, which influence their properties (55). A summary of algal polysaccharides with antiviral potential are shown in Table 1.
Most of the algal polysaccharides have the ability to decrease viral infections by blocking the attachment of virus particles to host cell surfaces. In this line, three polysaccharides extracted from Sargassum trichophyllum (a brown alga) were characterized as laminaran, alginate and fucoidan, observing that only fucoidan showed an antiviral effect against herpes simplex virus type 2 (HSV-2) (72). The 50% inhibitory concentration (IC50) of the fucoidan was 18 μg·mL−1 when it was added during the viral infection, being lower than adding after the viral infection. Therefore, this polysaccharide might inhibit HSV-2 at the virus adsorption and/or penetration step(s) (72). Sargassum henslowianum produced antiviral fucoidans against both HSV-1 and HSV-2 (84). For instance, the authors observed how two fractions of the fucoidans (SHAP-1 and SHAP-2) could inhibit HSV-1 with IC50 of 0.89 and 0.82 μg·mL−1, respectively. Both SHAP-1 and SHAP-2 showed higher antiviral activity against HSV-2 with IC50 of 0.48 μg·mL−1. These fucoidans interfered with the virions' attachment to host cells (84). Moreover, low MW fucoidan fractions (LF1 and LF2) from Laminaria japonica could inhibit I-type influenza virus, adenovirus and parainfluenza virus I in vitro. The IC50 for LF1 were 0.3, 0.6, and 0.3 mg·mL−1, respectively, whereas The IC50 for LF2 were 0.6, 1.2, and 0.6 mg·mL−1, respectively (66). Fucoidan from Cladosiphon okamuranus also showed higher antiviral activity against NDV with lower cytotoxicity than Ribavirin, an antiviral drug, preventing this polysaccharide the viral infection at early steps by blocking the F protein (85). In addition, Scytosiphon lomentaria, a brown seaweed, also produced fucoidans with antiviral activity, in particular, they had the ability to block HSV-1 and HSV-2 infections (86). Moreover, a fucoidan with high levels of sulfate groups also showed the highest antiviral activity against HSV-1 and HSV-2 (86).
Carrageenans are sulfated linear polysaccharides extracted from some red algae, such as Chondrus, Gigartina, Hypnea, and Eucheuma spp. (49). These polysaccharides showed an antiviral activity against several viruses. For instance, González et al. (87) reported a good inhibitory effect of carrageenan against some enveloped viruses, including HSV-1, HSV-2, Semliki Forest, vaccinia, and swine fever viruses, but they did not find any impact on vesicular stomatitis and measles viruses. Moreover, these authors also showed the antiviral activity of carrageenan against encephalomyocarditis virus (EMCV), a naked virus, but they did not observe significant effects on poliovirus or adenovirus. The carrageenan interfered with the viral protein synthesis. On the other hand, λ-carrageenan and moderately cyclized μ/ℓ-carrageenan extracted from a red seaweed (Gigartina skottsbergii) inhibited the viral attachment of HSV-1 and HSV-2 (88). Various types of carrageenans also have shown antiviral activities against hepatitis A virus (HAV). The 50% effective dose (ED50) for ι-carrageenan, λ-carrageenan, and κ-carrageenan against HAV were >400, >222, and >10 μg·mL−1, respectively (89). λ-Carrageenan from G. skottsbergii showed an inhibitory effect on both bovine herpesvirus type 1 (BoHV-1) and Suid herpesvirus type 1 (SuHV-1). The IC50 of this polysaccharide was 0.52 and 10.4 μg·mL−1, respectively (90).
The red micro algae, Porphyridium spp., produce antiviral polysaccharides against many types of viruses, including HSV-1, HSV-2, and VZV. The algae inhibited viral entry and/or blocked virus replication in host cells (38, 43). In this line, the sulfated polysaccharide SP-2a obtained from a brown alga, Sargassum patens, exhibited strong antiviral property against different strains of HSV-1. The EC50 of SP-2a against the standard, acyclovir (ACV)-sensitive and -resistant strains of HSV-1 were 5.5, 1.5, and 4.1 μg·mL−1, respectively. The SP-2a had a weak virucidal activity against the standard and ACV-sensitive strains of HSV-1, but not the ACV-resistant strain. During virus adsorption, the SP-2a showed ≥80% inhibition of adsorption against all strains of HSV-1 (71). p-KG03 is a sulfated exopolysaccharide with an average MW of 1.87 × 107 Da extracted from a dinoflagellate, Gyrodinium impudicum strain KG03. The p-KG03 could inhibit EMCV in HeLa cells with an EC50 of 26.9 μg·mL−1, and influenza A at the virus adsorption step, but was ineffective against influenza B, HSV-1, HSV-2, human immunodeficiency virus type 1 (HIV-1), HIV-2, Coxsackie B virus type 3 (Cox-B3), and vesicular stomatitis virus (VSV). In addition, the p-KG03 also showed antiviral activity against influenza A virus at the virus adsorption step, but did not inhibit all influenza B virus isolates. The EC50 for p-KG03 against different strains of influenza A virus (H1N1: PR8 and Tw; H3N2: Se) ranged from 0.19 to 0.48 μg·mL−1 (56, 60). Lee et al. (67) reported that Navicula directa, a diatom collected from deep-sea water in Toyama Bay (Japan), produced naviculan (a sulfated polysaccharide). The naviculan is a heteropolysaccharide consisting of fucose, xylose, galactose, mannose, rhamnose, and sulfate with an average MW of ~2.2 × 105 Da. It is a broad-spectrum antiviral against HSV-1, HSV-2, and influenza A virus with IC50 of 14, 7.4, and 170 μg·mL−1, respectively, at the virus adsorption phase. Moreover, it could also interfere with the cell-cell fusion of HIV gp160- and CD4-expressing HeLa cells. Therefore, it might prevent HIV infections.
Antiviral Polysaccharides From Bacteria
Bacteria (including cyanobacteria or blue-green algae) have the ability to synthesize polysaccharides for various purposes such as storage, cell protection, and adhesion. Polysaccharides accumulated in cells are called intracellular polysaccharides (ICP). While those outside of cell are called extracellular polysaccharides or EPS. The latter are secreted by cells or produced extracellularly using cell wall-anchored enzymes (28). Bacterial polysaccharides show biological (bioactive) activities, including anti-inflammatory, anticancer, antimicrobial, antioxidant, and immunomodulatory (91–97). They showed an inhibitory effect against various viruses, both DNA and RNA viruses. The inhibitory effect is usually associated with the viral adsorption and/or replication phases in host cells. For example, Arthrospira platensis (formerly Spirulina platensis) produced calcium spirulan, a sulfated polysaccharide, with antiviral activity against several enveloped viruses. The calcium spirulan composed of rhamnose, ribose, mannose, fructose, galactose, xylose, glucose, glucuronic acid, galacturonic acid, sulfate, and calcium. This polysaccharide showed antiviral activity against HSV-1, human cytomegalovirus (HCMV), measles, mumps, influenza A, and HIV-1 viruses by inhibiting virus penetration (74). Spirulan-like substances extracted from A. platensis showed strong antiviral activity against HCMV, HSV-1, human herpesvirus type 6 (HHV-6), and HIV-1. Their mechanisms depended on the type of virus. For example, in the case of herpesviruses, spirulan-like substances inhibited virus adsorption and/or penetration steps, while HIV-1 was inhibited after viral entry. For HCMV, the inhibition occurred at intracellular steps, especially the viral protein synthesis step (44). EPS from A. platensis also inhibited koi herpesviruses (KHV). Reichert et al. (75) reported that EPS from A. platensis between 18 and 36 μg·mL−1 suppressed KHV in vitro Furthermore, nostoflan, an acidic polysaccharide from Nostoc flagelliforme (a cyanobacterium), showed an interesting antiviral activity against enveloped viruses: HSV-1, HSV-2, HCMV, and influenza A viruses. The virus infections were blocked when nostoflan was added at the same time as viral infections. Therefore, nostoflan blocked the viruses at the virus adsorption stage. The IC50 values of nostoflan for HSV-1, HSV-2, HCMV, and influenza A viruses were 0.37, 2.9, 0.47, and 78 μg·mL−1, respectively (79). In addition, other authors also observed how an EPS derived from Bacillus licheniformis strain T14 can prevent HSV-2 infection at 300 and 400 μg·mL−1 in human peripheral blood mononuclear cells (PBMC) (76). EPS26a from Lactobacillus sp. could completely inhibit human adenovirus type 5 (HAdV-5) formation and release (41).
Bacterial polysaccharides also indirectly inhibited virus infections by modulation of the immune response. For instance, an EPS produced by Lactobacillus delbrueckii OLL1073R-1 activated the Toll-like receptor 3 (TLR3) and the expression of interferon (IFN)-α, IFN-β, MxA, and RNase L in porcine intestinal epithelial (PIE) cells, which was associated with the innate antiviral immune response (77). Mizuno et al. (98) reported that an EPS from Streptococcus thermophilus ST538 can activate TLR3, thus promoting the expression of IFN-β, interleukin 6 (IL-6), and C-X-C motif chemokine 10 (CXCL10). Antiviral bacterial polysaccharides are also shown in Table 1.
Antiviral Polysaccharides From Fungi
Fungi are unicellular-to-multicellular eukaryotic microorganisms. They can produce a plethora of biologically active compounds, especially secondary metabolites. Similar to algae and bacteria, fungal polysaccharides (primary metabolites) also showed antiviral activity. Fungal polysaccharides can be derived from culture broth, mycelial culture and/or fruiting bodies (99). Fungal polysaccharides, such as glucan, chitin, mannan, PSK or lentinan, showed antiviral potential against animal, human, and plant viruses (100–103). Fungal polysaccharides with antiviral activity are summarized in Table 1. For example, BAS-F, a polysaccharide from Fomes fomentarius, at 2 μg·mL−1 can prevent tobacco mosaic virus (TMV) infection on the leaf surfaces (34). Porodaedalea pini (formerly known as Phellinus pini) produced two antiviral polysaccharides (EP-AV1 and EP-AV2) against HSV-1 and coxsackie virus B3 (CVB3) in Vero and HeLa cells, respectively. The EP-AV2 with a lower MW (~100 kDa) showed more potent antiviral activity than EP-AV1 (~1,010 kDa) against CVB3. It was observed how EP-AV1 and EP-AV2 polysaccharides inhibited the plaque formation caused by CVB3 in HeLa cells by 32 and 84% at 1 mg·mL−1, respectively. These polysaccharides specifically inhibited HSV-1 more than CVB3 as indicated by their EC50 values. The EC50 values of EP-AV1 and EP-AV2 for HSV-1 were 0.20 and 0.21 μg·mL−1, respectively, whereas CVB3 were 1 and 0.576 mg·mL−1, respectively (81). Furthermore, a polysaccharide extracted from the mycelium and fruiting body of Lentides edodes was able to inhibit poliovirus type 1 (PV-1) and bovine herpes virus type 1 (BoHV-1) with IC50 values of 0.19 and 0.1 mg·mL−1, respectively (101). In another study, Grifola frondosa mycelia were evaluated as a source of antiviral polysaccharides, observing that it had the antiviral polysaccharide, GFP1. This polysaccharide was a heteropolysaccharide containing glucose and fucose with a MW of ~40.5 kDa. Zhao et al. (82) reported that GFP1 blocked enterovirus 71 (EV71) infection at the virus replication phase. The GFP1 suppressed the viral protein expression and viral RNA genome synthesis. Fungal polysaccharides also showed important antiviral properties against animal viruses. For example, a polysaccharide from L. edodes, called lentinan comprised of glucose, mannose, and galactose with MW of ~3.79 × 105 Da showed antiviral activity against infectious hematopoietic necrosis virus (IHNV) infecting rainbow trout (Oncorhynchus mykiss) and several species of salmon. The LNT-I acted both direct inactivation and inhibition of viral replication with 62.3, and 82.4% inhibition, respectively (83). Inonotus obliquus, chaga mushroom, also produced broad-spectrum antiviral polysaccharides against feline viruses. The polysaccharides suppressed infections of feline calicivirus (FCV), feline herpesvirus 1 (FHV-1), feline panleukopenia (FPV), feline coronavirus (FCoV), and feline influenza (FIV, H3N2, and H5N6) viruses. These polysaccharides had low toxicity and blocked virus entry by affecting the virions and/or the receptor(s) on host cell surfaces (35).
Therefore, polysaccharides from different species show various biological activities with different levels of action. Sulfated polysaccharides derived from marine microalgae and seaweeds showed many different bioactive properties and were effective against viruses at low concentrations, compared to other polysaccharides. In addition, bacteria and fungi are easily grow on simple media or agricultural wastes. The production can be done using controllable conditions and they produce high amounts of polysaccharides. Therefore, it would also benificial if the bioactivities and physicochemical properties of bacterial and fungal polysaccharides could be modified. The molecular modification of polysaccharides is an alternative approach to modulate their properties.
Antiviral Mechanisms of Microbial Polysaccharides
In viral replication, there are 6 major steps during the infection: (1) virus attachment, (2) penetration, (3) uncoating, (4) genome replication and protein synthesis, (5) viral assembly, and (6) release of new virions (104). Different microbial polysaccharides have different antiviral mechanisms depending on virus types. The polysaccharides mostly prevented the initial steps of the virus life cycle. They interacted with virus particles and/or receptors on host cells to interfere with virus adsorption and invasion. However, some microbial polysaccharides could inhibit viral replication and protein translation. While others showed immune-enhancing activity, especially antiviral immune responses, which prevent virus infections and reduce disease severity (31, 105).
Inactivating Virus Particles Directly
Microbial polysaccharides, especially sulfated polysaccharides, have a negative charge that can interact directly with the viral surfaces. The virucidal activity of microbial polysaccharides is caused by theses interactions (106). The complexes interfere with the viral infection process, reducing viral proliferation in host cells (Figure 2). For example, polysaccharides extracted from Auricularia auricular, a basidiomycete mushroom, can inhibit NDV in CEF cells. During the process of adding polysaccharides and virus simultaneously, the virus inhibitory rates were higher than pre- and post-addition of the polysaccharides. These polysaccharides might be combined with virus particles to block virus attachment to host cells (42). Inonotus obliquus polysaccharides also directly blocked feline virus virions (FCV, FHV-1, FPV, feline coronavirus FCoV, and FIV). These polysaccharides were mixed with the viruses for 1 h before adding to the cell lines, decreasing significantly the viral infectivity compared to untreated viruses (35).
Inhibiting Virus Adsorption and Penetration
Viruses bind to a host cell surface using electrostatic interactions. Some microbial polysaccharides mimic virus particles. Microbial polysaccharides, especially sulfated polysaccharides, are strongly anionic and bind to the positively charged host cell receptors blocking virus attachment, which prevents virus infection (Figure 3) (7). Additionally, some microbial polysaccharides are able to prevent the allosteric process of viral protein formation and/or virus internalization and uncoating steps (106).
Many microbial polysaccharides act at this step. For example, a polysaccharide SP-2a from S. patens showed ≥80% inhibition against all strains of HSV-1 when added during virus adsorption (71). Fucoidans from a brown alga, Padina tetrastromatica showed the highest percentage (>70%) inhibition against HSV-1 during the virus adsorption period (37). Human influenza virus H1-364 was blocked by sulfated polysaccharides from Gracilaria lemaneiformis, a red alga, at virus adsorption and replication on host cells. The sulfated polysaccharides inhibited against the virus at ≥60% during virus adsorption and replication, while these polysaccharides were not effective at the virus release step. The polysaccharides at 62.5 μg·mL−1 showed 83.5 and 83.0% inhibition against human influenza virus H1-364 at the virus adsorption and replication steps, respectively (59).
Inhibiting Viral Genome Replication and Protein Synthesis
Microbial polysaccharides, especially the low MW polysaccharides, show antiviral effects on infected host cells. They interfere directly with enzymes associated with the viral replication and inhibit other intracellular targets (107) as presented in Figure 4. Carrageenans are sulfated polysaccharide that are available from most of red seaweeds. These polysaccharides show a broad-spectrum antiviral activity. González et al. (87) reported that carrageenan inhibited HSV-1 at viral protein synthesis. When carrageenan was added 1 h after HSV-1 infection, viral proteins were not detected, whereas when carrageenan was added immediately, viral proteins were detected. Furthermore, polysaccharide GFP1 from G. frondosa, which was composed of glucose and fucose with a MW of 40.5 kDa, acted on viral replication and protein synthesis against EV71. The GFP1 was effective in inhibiting EV71 when it was added before or shortly after the viral inoculation. The viral RNA synthesis and VP1 protein were suppressed in a dose-dependent manner (82).
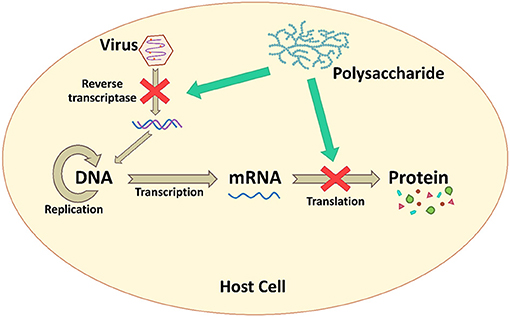
Figure 4. The inhibition mechanism by interfering with viral genome replication and protein synthesis.
Modulating Host Antiviral Immune Responses
During virus infection in animals, the body induces the immune responses to defend against viral infection. The responses regulate immune cells such as natural killer (NK) cells and macrophages, and increase the production of cytokines, i.e., the type I interferon system (IFN-α/β system) (36). The microbial polysaccharides interact with cell receptors on the macrophage and NK cell, and then activate the cells using the nuclear factor kappa B (NF-κB) and the mitogen-activated protein kinase (MAPK) signaling pathways. These proteins are inducible factors, which increases the gene expression of various cytokines, chemokines, enzymes, and other proteins involving both innate and adaptive immunity (27). The IFN secreted from activated immune cells triggers activation of other immune cells including NK cells, macrophages, and T-cell lymphocytes, which have important roles in the host immune system and antiviral responses. Meanwhile, microbial polysaccharides can activate NK cells that non-specifically kill virus-infected cells by secreting perforins and granzymes (Figure 5).
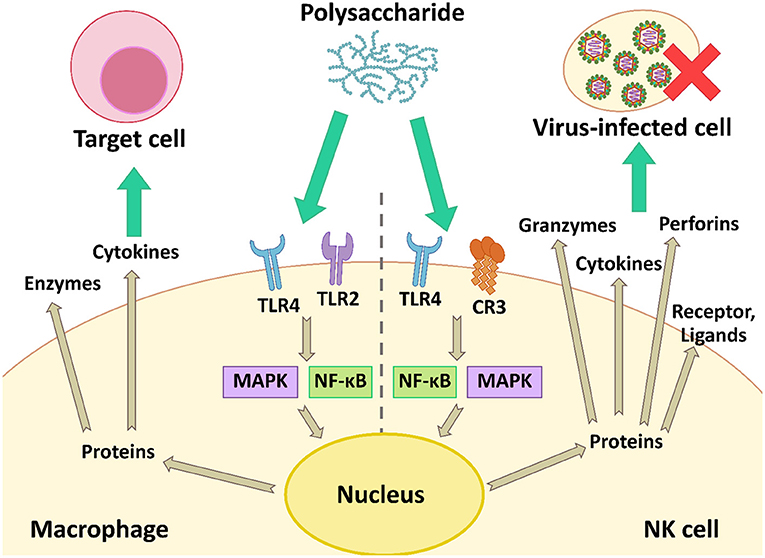
Figure 5. The modulation of the antiviral immune response by activation of macrophage and NK cell using the NF-κB and MAPK signaling pathways.
Several polysaccharides can enhance the antiviral immune responses, thus reducing the number of virus particles and the severity of diseases. For example, an EPS extracted from S. thermophilus ST538 was able to induce the expression of IFN-β, IL-6, and CXCL10 in response to TLR3 stimulation. These immune factors are associated with antiviral immune responses, which induce the recruitment and activation of immune cells to struggle pathogens (98). Moreover, L. delbrueckii OLL1073R-1 produced immunomodulatory EPS. These EPS activated TLR3 and induced the expression of IFN-α, IFN-β, MxA, and RNase L. The latter two factors are known as antiviral factors (77). Polysaccharides also showed immune-enhancing activity. Ren et al. (83) reported that LNT-I from L. edodes mycelia could modulate the immune response by up-regulating the expression of IFN-1 and IFN-γ to prevent IHNV infection. In addition, an acidic polysaccharide (APS) from Cordyceps militaris enhanced TNF-α, IFN-γ, and nitric oxide (NO) production, and induced the expression of several cytokines: IL-1β, IL-6, IL-10, and TNF-α. These cytokines have the potential to prevent influenza A virus infection (39). Cao et al. (64) also reported a polysaccharide from L. japonica which could increase IFN-α secretion in a dose-dependent manner. The IFN-α level was 144 pg·mL−1 when cells were treated with 1000 μg·mL−1 of polysaccharide.
Factors Influencing the Antiviral Activity
Polysaccharides derived from different sources showed several unique characteristics, properties, and bioactivities at different levels. Table 2 shows various microbial polysaccharides and/or polysaccharide fractions with different characteristics. Their MW, compositions, functional groups, and structural conformations including type of linkage and degree of branching associate with their biological properties, especially antiviral and immunomodulatory activities. Moreover, extraction and purification methods affect the compositions of polysaccharides; therefore, these factors also influence biological activities of the polysaccharides (110).
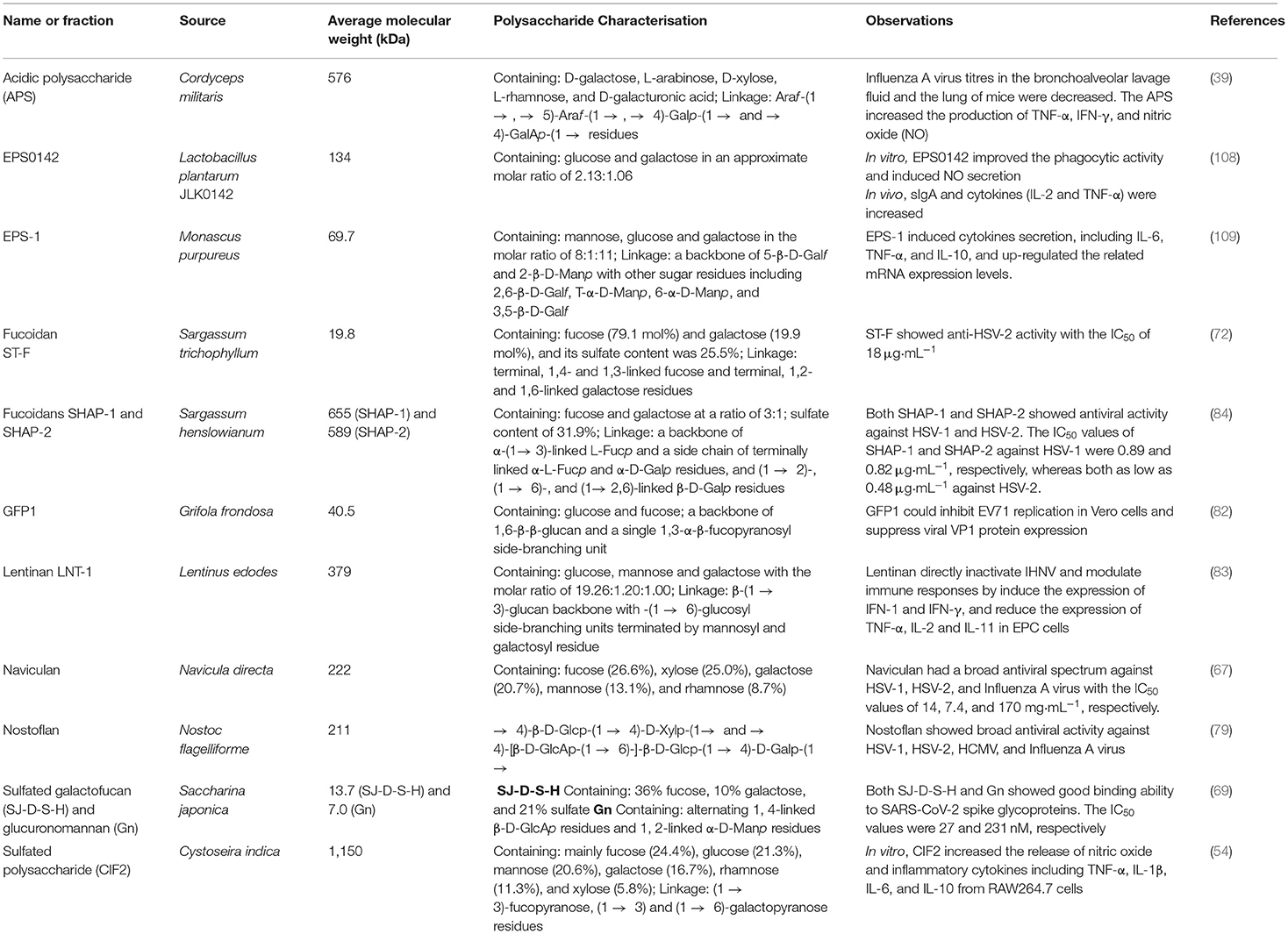
Table 2. Some microbial polysaccharides from various sources with different characteristics and bioactivities.
Sulfate Content
Several studies have reported that sulfated polysaccharides could exhibit several biological activities (antiviral, anticancer, antioxidant, and immunomodulatory activities), so the sulfate contents could be an important factor affecting antiviral and other bioactivities. Sulfation has been used for enhancing various biological activities of polysaccharides (111, 112). For example, a marine Pseudomonas sp. WAK-1 produces extracellular glycosaminoglycan (A1) and sulfated polysaccharide (A2) with antiviral activity. Matsuda et al. (80) modified the polysaccharides by over-sulfation using a dicyclohexyl-carbodiimide-mediated reaction. The over-sulfated polysaccharides were called A1S and A2S, respectively. These 4 compounds showed antiviral activity against influenza A virus with EC50 values of >100, >100, 11.0, and 2.9 μg·mL−1, respectively. From the results, over-sulfated polysaccharides (A1S and A2S) showed higher antiviral activity against influenza A virus than the natural polysaccharides (A1 and A2). Moreover, a xylogalactofucan (sulfated polysaccharide) from a brown alga Sphacelaria indica also exhibited antiviral activity against HSV-1. The sulfate contents of the polysaccharide affected the antiviral property. The sulfate content of the purified polysaccharide was 4% (w/w). Bandyopadhyay et al. (113) chemically modified the xylogalactofucan produced derivatives with up to 7% (w/w). The IC50 values of natural and artificially over-sulfated polysaccharides were 1.3 and 1.5 μg·mL−1, respectively, while a desulfated derivative of the polysaccharide had no effect on HSV-1. Furthermore, Ponce et al. (86) also reported that the level of sulfated esters in sulfated galactofucans extracted from a brown alga Scytosiphon lomentaria was an important factor influencing the antiviral activity. The whole extract (A) of S. lomentaria was fractionated to yield fractions A0, A5, A10, A20, A30, and A40, with different components, MW, and monosaccharide composition. A0 was soluble and the fraction A5 was an uronofucoidan. A10–A40 were galactofucans and showed antiviral activity against HSV-1 and HSV-2 with IC50 values in the range 0.76–10.0 μg·mL−1. Among the 4 galactofucan fractions, A30 (pure galactofucan) contained the highest sulfate content (29.5% SO3Na) and the lowest uronate content (1.8%). A30 showed the strongest antiviral activity against HSV-1 and HSV-2 with IC50 values of 0.76 and 1.3 μg·mL−1, respectively. Therefore, the low content of uronic acids and the high content of sulfate was associated with the antiviral activity of these polysaccharides. In conclusion, sulfate content is an important factor influencing biological activities. Adding sulfate groups into polysaccharide structures led to enhance bioactivities, whereas desulfation decreased their bioactivities.
Molecular Weight
The MW of polysaccharides also influenced their biological properties. Polysaccharides with low MW could easily pass through target cells to act inside the cells. Moreover, the low MW polysaccharides might bind better to cell receptors to inactivate or activate the target cells (114). Some polysaccharides with lower MW showed high biological activities, but some polysaccharides with higher MW were better. For example, Surayot et al. (26) reported the effect of MW of an EPS from Weisella confusa on immunomodulatory activity. The EPS with low MW (≤70 × 103 Da) could stimulate RAW264.7 cells to induce NO and production of various cytokines such as TNF-α, IL-1β, IL-6, and IL-10, but the native EPS (MW of ~506 × 106 Da) had no immunomodulatory activity. In addition, Ponce et al. (86) reported that the galactofucan fraction A30 had the lowest MW (~8.5 kDa) and the low MW might be another factor influencing the antiherpes activity against HSV-1 and HSV-2. On the contrary, high molecular weight carrageenans from different rea algae (Chondrus armatus, Kappaphycus alvarezii, and Tichocarpus crinitus) had effective antiviral activity (115). The different carrageenans with molecular weight of 250, 390, and 400 kDa, respectively show antiviral activity with 88, 85, and 77%, respectively. While low molecular weight (LMW) derivatives (1.2–3.5 kDa) were obtained from different depolymerization methods. The LMW derivatives showed low antiviral properties (28–54%) compared to the native polysaccharides. Therefore, the antiviral activity of these polysaccharides depended on their molecular weight (115).
Enhancement of Bioactivities
To enhance the biological activities, natural microbial polysaccharides need molecular modification of their structure, size, and functional groups to optimize activity (114). The MW can be reduced using external energy and/or specific enzymes to break glucoside chains. Using ultrasonic disruption and microwave exposure to reduce the MW are “physical modification,” whereas the enzymatic degradation is “biological modification” (114). For instance, Surayot et al. (26) hydrolyzed an EPS from W. confusa TISTR 1498 using HCl and heating in hot water or in a microwave oven. The low MW products could induce production of cytokines from RAW264.7 macrophage cells. Bioactivities were also enhanced by changing the functional substituents of polysaccharides, which is “chemical modification,” such as alkylation, sulfation, sulfonation, phosphorylation, carboxymethylation, and selenization (114).
The Potential Uses of Microbial Polysaccharides to Prevent Viral Diseases
Microbial polysaccharides showed various bioactivities, while almost always having any significant side-effects, yet are biodegradable, biocompatible, and cost-effective. Microbial and algal polysaccharides may be applied as drug resistance solutions. These polysaccharides can combine with other antiviral drugs for preventing drug-resistance strains (110). In addition to the prevention of viral infections, these polysaccharides also prevent recurrence of latent viruses. For example, calcium spirulan (Ca-SP) derived from Spirulina platensis was developed as microalgal cream, which effectively prevented the recurrence of HSV-1 (116). Therefore, the bioactive polysaccharides may be used to prevent viral diseases and reduce the risks of diseases, especially COVID-19.
SARS-CoV-2 has an S-protein on its envelope and the protein has an important role with binding to a host cell receptor (ACE2) (8). Heparin, heparan sulfates, and other sulfated polysaccharides can bind tightly to the S-protein in vitro (117). The binding inhibits viral infection. Other microbial polysaccharides showed immunomodulatory properties that stimulated the immune system to prevent SARS-CoV-2 infection. Several microbes can produce sulfated polysaccharides. Beneficial sulfated polysaccharides might be produced from natural microbial polysaccharides (114). Type I IFN, including IFN-α, -β, -ε, -κ, -ω, -δ, -ζ, and -τ, are essential cytokines for antiviral immune responses. Type I IFN can induce antiviral responses within infected and neighboring cells that block the spread of virus particles. They activate both innate and adaptive immune responses that promote NK cell functions and antibody production (118, 119). Hadjadj et al. (120) reported that most severe COVID-19 patients had a low type I IFN response (No IFN-β and low IFN-α production and activity). Many microbial and algal polysaccharides induced type I IFN production in vivo. Thus, these polysaccharides might be applied to modulate the immune system in both patients and healthy people. In severe COVID-19 cases, aggressive inflammatory responses were found and the inflammation caused tissue damage in many organs (121). Some microbial polysaccharides showed anti-inflammatory activity. These polysaccharides inhibited the production of pro-inflammatory cytokines including TNF-α, IL-1β, IL-6, and IL-8 (122–124).
ACE2 receptors are expressed by several tissues and organs as described above, especially the respiratory and gastrointestinal tracts. Microbial polysaccharides with antiviral activity can be used as a nasal spray, metered dose inhaler, or delivered orally to prevent the binding of SARS-CoV-2 (117). Several natural polysaccharides have been designed as nanomaterials for drug delivery systems, such as antiviral agent. These nanomaterials may be not only used to treat the virus, but also to modulate the immune responses (7).
Algal, bacterial, and fungal polysaccharides and sulfated polysaccharides showed pharmaceutical properties due to their biological activities as mentioned above. These polysaccharides could be used as bioactive supplements in foods and could enrich nutritional quality. Indeed, some of these polysaccharides have been granted as GRAS status by the US FDA, so they can consume to enhance immune response and reduce the severity of viral diseases, especially COVID-19 (117). Some microbial polysaccharides have prebiotic properties, which enhance the proliferation of beneficial intestinal microflora, especially Bifidobacterium spp. (125). In addition, some algal polysaccharides (alginate and laminaran) could be fermented by gut microbiota and promoted the growth of Bacteroides, Bifidobacterium, and Lactobacillus species (126). When microbial polysaccharides were consumed, they could enhance the host's immune response and modulate the microbial community (microflora). The microbes degrade the polysaccharides into short-chain fatty acids (SCFA) such as acetic, propionic, and butyric acids. SCFA show benefit for the maintenance intestinal cells and modulating of the immune system (27). Microbial polysaccharides have the potential to be bioactive ingredients that can be added into foods or food products to enhance the nutritional quality of foods by modulating consumers' immune response. Therefore, the biological activities of foods supplemented with these polysaccharides should be investigated. The intake of foods with bioactive polysaccharides in patients and healthy people to prevent viruses and/or reduce the adverse symptoms needs further study.
Conclusion
Microorganisms produce various types of polysaccharides with unique characteristics and can be produced on a large scale with controllable conditions. Several microbial/algal polysaccharides show bioactivities, especially antiviral and immunomodulatory activities. They have strong antiviral effects by interfering with the life cycle of viruses and/or modulating host immune responses, which may benefit patients infected with COVID-19. Polysaccharides and sulfated polysaccharides from different microorganism and algae species have different characteristics and levels of bioactivities. Their constituents, structural conformations, MW, and functional groups significantly influence their bioactivities. To enhance their activities, physical, chemical, or biological modifications might be beneficial. The microbial polysaccharides have potential uses as adjuvants for antiviral vaccines and micro- and/or nano-particles for drug delivery systems. Some sulfated polysaccharides obtained from microbes and algae have been approved as GRAS, which may be used as bioactive ingredients adding in food products to prevent viruses. Many microbial polysaccharides are safe, biocompatible, biodegradable, and easily available. Therefore, the intake of proper dosage of the right polysaccharides may modulate physiological functions to prevent viral diseases and decrease their damage. They may be an alternative therapy to treat COVID-19 patients. In the future, the development of polysaccharides as functional food products should be explored. For foods supplemented with bioactive polysaccharides, more pharmaceutical investigations and clinical evidence are required to analyze their antiviral and immune-enhancing effects. The mechanisms that occur in the food products against viral infections should also be further investigated.
Author Contributions
PS, TC, CT, and SY contributed to conception and supervised the project. WC, NL, KJ, and SS contributed in doing literature searches and wrote the manuscript draft. YP, PR, SW, JR, FB, and PS equally revised and approved the manuscript. All authors have read and approved the final draft manuscript.
Funding
The authors gratefully acknowledge the financial support from the National Research Council of Thailand (NRCT) through the Royal Golden Jubilee Ph.D. Program, Thailand (grant no. PHD/0185/2560) to WC and PS. Additionally, this work was also partially financially supported along with in-kind support by the Biotechnology Program of the Graduate School of Chiang Mai University; the Cluster of Agro Bio-Circular-Green Industry (Agro BCG) Faculty of Agro-Industry; and Chiang Mai University.
Conflict of Interest
The authors declare that the research was conducted in the absence of any commercial or financial relationships that could be construed as a potential conflict of interest.
Publisher's Note
All claims expressed in this article are solely those of the authors and do not necessarily represent those of their affiliated organizations, or those of the publisher, the editors and the reviewers. Any product that may be evaluated in this article, or claim that may be made by its manufacturer, is not guaranteed or endorsed by the publisher.
Abbreviations
ACE2, Angiotensin-converting enzyme 2; ACV, Acyclovir; APS, Acidic polysaccharide; BoHV-1, Bovine herpesvirus type 1; BoHV-1, Bovine herpes virus type 1; CoV, Coronaviruses; COVID-19, Coronavirus disease 2019; Cox-B3, Coxsackie B virus type 3; CVB3, Coxsackie virus B3; CXCL10, C-X-C motif chemokine 10; ED50, 50% effective dose; EMCV, Encephalomyocarditis virus; EPS, Exopolysaccharides; EV71, Enterovirus 71; FCoV, Feline coronavirus; FCV, Feline calicivirus; FDA, US Food and Drug Administration; FHV-1, Feline herpesvirus 1; FIV, Feline influenza; FPV, Feline panleukopenia; HAdV-5, Human adenovirus type 5; HAV, Hepatitis A virus; HCMV, Human cytomegalovirus; HHV-6, Human herpesvirus type 6; HIV, Human immunodeficiency virus; HSV, Herpes simplex virus; IC50, 50% inhibitory concentration; ICP, Intracellular polysaccharides; ICTV, International Committee on Taxonomy of Viruses; IFN, Interferon; IHNV, Hematopoietic necrosis virus; IL, Interleukin; KHV, Koi herpesviruses; LAB, Lactic acid bacteria; MAPK, Mitogen-activated protein kinase; MERS-CoV, Middle East respiratory syndrome; MW, Molecular weight; NDV, Newcastle disease virus; NF-κB, Nuclear factor kappa B; NK, Natural killer cells; NO, Nitric oxide; PBMC, Peripheral blood mononuclear cells; PV-1, Poliovirus type 1; SARS-CoV-2, Severe acute respiratory syndrome coronavirus 2; SCFA, Short-chain fatty acids; SuHV-1, Suid herpesvirus type 1; TLR3, Toll-like receptor 3; TMPRSS2, Transmembrane protease serine protease 2; TMV, Tobacco mosaic virus; VSV, Vesicular stomatitis virus; VZV, Varicella zoster virus; WHO, World Health Organization.
References
1. Dimmock NJ, Easton AJ, Leppard KN. Introduction to Modern Virology. Chichester: John Wiley & Sons (2016). 544 p.
2. World Health Organization. Rolling Updates on Coronavirus Disease (COVID-19) (2021). Available online at: https://www.who.int/emergencies/diseases/novel-coronavirus-2019/events-as-they-happen (accessed May 3, 2021).
3. World Health Organization. WHO Coronavirus (COVID-19) Dashboard (2021). Available online at: https://covid19.who.int/ (accessed August 9, 2021).
4. Mohan SV, Hemalatha M, Kopperi H, Ranjith I, Kumar AK. SARS-CoV-2 in environmental perspective: occurrence, persistence, surveillance, inactivation and challenges. Chem Eng J. (2021) 405:126893. doi: 10.1016/j.cej.2020.126893
5. Chen B, Tian EK, He B, Tian L, Han R, Wang S, et al. Overview of lethal human coronaviruses. Signal Transduct Target Ther. (2020) 5:89. doi: 10.1038/s41392-020-0190-2
6. V'Kovski P, Kratzel A, Steiner S, Stalder H, Thiel V. Coronavirus biology and replication: implications for SARS-CoV-2. Nat Rev Microbiol. (2020) 19:155–70. doi: 10.1038/s41579-020-00468-6
7. Chen X, Han W, Wang G, Zhao X. Application prospect of polysaccharides in the development of anti-novel coronavirus drugs and vaccines. Int J Biol Macromol. (2020) 164:331–43. doi: 10.1016/j.ijbiomac.2020.07.106
8. Cevik M, Kuppalli K, Kindrachuk J, Peiris M. Virology, transmission, and pathogenesis of SARS-CoV-2. BMJ. (2020) 371:m3862. doi: 10.1136/bmj.m3862
9. Di Nardo M, van Leeuwen G, Loreti A, Barbieri MA, Guner Y, Locatelli F, et al. A literature review of 2019 novel coronavirus (SARS-CoV2) infection in neonates and children. Pediatr Res. (2020) 89:1101–8. doi: 10.1038/s41390-020-1065-5
10. Jia HP, Look DC, Shi L, Hickey M, Pewe L, Netland J, et al. ACE2 receptor expression and severe acute respiratory syndrome coronavirus infection depend on differentiation of human airway epithelia. J Virol. (2005) 79:14614–21. doi: 10.1128/JVI.79.23.14614-14621.2005
11. Li MY, Li L, Zhang Y, Wang XS. Expression of the SARS-CoV-2 cell receptor gene ACE2 in a wide variety of human tissues. Infect Dis Poverty. (2020) 9:45. doi: 10.1186/s40249-020-00662-x
12. Xu H, Zhong L, Deng J, Peng J, Dan H, Zeng X, et al. High expression of ACE2 receptor of 2019-nCoV on the epithelial cells of oral mucosa. Int J Oral Sci. (2020) 12:8. doi: 10.1038/s41368-020-0074-x
13. Kelleni MT. Tocilizumab, remdesivir, favipiravir, and dexamethasone repurposed for COVID-19: a comprehensive clinical and pharmacovigilant reassessment. SN Compr Clin Med. (2021) 3:919–23. doi: 10.1007/s42399-021-00824-4
14. Beigel JH, Tomashek KM, Dodd LE, Mehta AK, Zingman BS, Kalil AC, et al. Remdesivir for the treatment of covid-19 - final report. N Engl J Med. (2020) 383:1813–26. doi: 10.1056/NEJMoa2007764
15. Centers for Disease Control and Prevention. Treatments Your Healthcare Provider Might Recommend if You Are Sick (2020). Available online at: https://www.cdc.gov/coronavirus/2019-ncov/your-health/treatments-for-severe-illness.html (accessed December 24, 2020).
16. WHO Solidarity Trial Consortium. Repurposed antiviral drugs for COVID-19—interim WHO solidarity trial results. N Engl J Med (2021) 384:497–511. doi: 10.1056/NEJMoa2023184
17. Centers for Disease Control and Prevention. Different COVID-19 Vaccines (2020). Available online at: https://www.cdc.gov/coronavirus/2019-ncov/vaccines/different-vaccines.html (accessed December 24, 2020).
18. Thirumdas R, Kothakota A, Pandiselvam R, Bahrami A, Barba FJ. Role of food nutrients and supplementation in fighting against viral infections and boosting immunity: a review. Trends Food Sci Technol. (2021) 110:66–77. doi: 10.1016/j.tifs.2021.01.069
19. Abdelkebir R, Alcántara C, Falcó I, Sánchez G, Garcia-Perez JV, Neffati M, et al. Effect of ultrasound technology combined with binary mixtures of ethanol and water on antibacterial and antiviral activities of Erodium glaucophyllum extracts. Innov Food Sci Emerg Technol. (2019) 52:189–96. doi: 10.1016/j.ifset.2018.12.009
20. Galanakis CM, Aldawoud TMS, Rizou M, Rowan NJ, Ibrahim SA. Food ingredients and active compounds against the coronavirus disease (COVID-19) pandemic: a comprehensive review. Foods. (2020) 9:1701. doi: 10.3390/foods9111701
21. Galanakis CM, Rizou M, Aldawoud TMS, Ucak I, Rowan NJ. Innovations and technology disruptions in the food sector within the COVID-19 pandemic and post-lockdown era. Trends Food Sci Technol. (2021) 110:193–200. doi: 10.1016/j.tifs.2021.02.002
22. El Khadem HS. Carbohydrates. In: Meyers RA, editor. Encyclopedia of Physical Science and Technology, 3rd Edn. Amsterdam, Netherlands: Elsevier B.V (2003). p. 369–416.
23. Barbosa JR, de Carvalho RN Junior. Polysaccharides obtained from natural edible sources and their role in modulating the immune system: biologically active potential that can be exploited against COVID-19. Trends Food Sci Technol. (2021) 108:223–35. doi: 10.1016/j.tifs.2020.12.026
24. Shi Y, Xiong Q, Wang X, Li X, Yu C, Wu J, et al. Characterization of a novel purified polysaccharide from the flesh of Cipangopaludina chinensis. Carbohydr Polym. (2016) 136:875–83. doi: 10.1016/j.carbpol.2015.09.062
25. Surin S, Surayot U, Seesuriyachan P, You SG, Phimolsiripol Y. Antioxidant and immunomodulatory activities of sulphated polysaccharides from purple glutinous rice bran (Oryza sativa L.). Int J Food Sci. (2018) 53:994–1004. doi: 10.1111/ijfs.13674
26. Surayot U, Wang J, Seesuriyachan P, Kuntiya A, Tabarsa M, Lee Y, et al. Exopolysaccharides from lactic acid bacteria: structural analysis, molecular weight effect on immunomodulation. Int J Biol Macromol. (2014) 68:233–40. doi: 10.1016/j.ijbiomac.2014.05.005
27. Chaisuwan W, Jantanasakulwong K, Wangtueai S, Phimolsiripol Y, Chaiyaso T, Techapun C, et al. Microbial exopolysaccharides for immune enhancement: fermentation, modifications and bioactivities. Food Biosci. (2020) 35:100564. doi: 10.1016/j.fbio.2020.100564
28. Nwodo UU, Green E, Okoh AI. Bacterial exopolysaccharides: functionality and prospects. Int J Mol Sci. (2012) 13:14002–15. doi: 10.3390/ijms131114002
29. Wang J, Salem DR, Sani RK. Extremophilic exopolysaccharides: a review and new perspectives on engineering strategies and applications. Carbohydr Polym. (2019) 205:8–26. doi: 10.1016/j.carbpol.2018.10.011
30. Rana S, Upadhyay LSB. Microbial exopolysaccharides: synthesis pathways, types and their commercial applications. Int J Biol Macromol. (2020) 157:577–83. doi: 10.1016/j.ijbiomac.2020.04.084
31. Andrew M, Jayaraman G. Marine sulfated polysaccharides as potential antiviral drug candidates to treat Corona Virus disease (COVID-19). Carbohydr Res. (2021) 505:108326. doi: 10.1016/j.carres.2021.108326
32. Muthukumar J, Chidambaram R, Sukumaran S. Sulfated polysaccharides and its commercial applications in food industries—A review. J Food Sci Technol. (2021) 58:2453–66. doi: 10.1007/s13197-020-04837-0
33. Ipper NS, Cho S, Lee SH, Cho JM, Hur JH, Lim CK. Antiviral activity of the exopolysaccharide produced by Serratia sp. strain Gsm01 against Cucumber mosaic virus. J Microbiol Biotechnol. (2008) 18:67–73.
34. Aoki M, Tan M, Fukushima A, Hieda T, Kubo S, Takabayashi M, et al. Antiviral substances with systemic effects produced by Basidiomycetes such as Fomes fomentarius. Biosci Biotechnol Biochem. (1993) 57:278–82. doi: 10.1271/bbb.57.278
35. Tian J, Hu X, Liu D, Wu H, Qu L. Identification of Inonotus obliquus polysaccharide with broad-spectrum antiviral activity against multi-feline viruses. Int J Biol Macromol. (2017) 95:160–7. doi: 10.1016/j.ijbiomac.2016.11.054
36. Chen L, Huang G. The antiviral activity of polysaccharides and their derivatives. Int J Biol Macromol. (2018) 115:77–82. doi: 10.1016/j.ijbiomac.2018.04.056
37. Karmakar P, Pujol CA, Damonte EB, Ghosh T, Ray B. Polysaccharides from Padina tetrastromatica: structural features, chemical modification and antiviral activity. Carbohydr Polym. (2010) 80:513–20. doi: 10.1016/j.carbpol.2009.12.014
38. Huleihel M, Ishanu V, Tal J, Arad SM. Antiviral effect of red microalgal polysaccharides on Herpes simplex and Varicella zoster viruses. J Appl Phycol. (2001) 13:127–34. doi: 10.1023/A:1011178225912
39. Ohta Y, Lee JB, Hayashi K, Fujita A, Park DK, Hayashi T. In vivo anti-influenza virus activity of an immunomodulatory acidic polysaccharide isolated from Cordyceps militaris grown on germinated soybeans. J Agric Food Chem. (2007) 55:10194–9. doi: 10.1021/jf0721287
40. Komatsu T, Kido N, Sugiyama T, Yokochi T. Antiviral activity of acidic polysaccharides from Coccomyxa gloeobotrydiformi, a green alga, against an in vitro human influenza A virus infection. Immunopharmacol Immunotoxicol. (2013) 35:1–7. doi: 10.3109/08923973.2012.710636
41. Biliavska L, Pankivska Y, Povnitsa O, Zagorodnya S. Antiviral activity of exopolysaccharides produced by lactic acid bacteria of the genera Pediococcus, Leuconostoc and Lactobacillus against human adenovirus type 5. Medicina. (2019) 55:519. doi: 10.3390/medicina55090519
42. Nguyen TL, Chen J, Hu Y, Wang D, Fan Y, Wang J, et al. In vitro antiviral activity of sulfated Auricularia auricula polysaccharides. Carbohydr Polym. (2012) 90:1254–8. doi: 10.1016/j.carbpol.2012.06.060
43. Abu-Galiyun E, Huleihel M, Levy-Ontman O. Antiviral bioactivity of renewable polysaccharides against Varicella Zoster. Cell Cycle. (2019) 18:3540–9. doi: 10.1080/15384101.2019.1691363
44. Rechter S, König T, Auerochs S, Thulke S, Walter H, Dörnenburg H, et al. Antiviral activity of Arthrospira-derived spirulan-like substances. Antivir Res. (2006) 72:197–206. doi: 10.1016/j.antiviral.2006.06.004
45. Stepanenko LS, Maksimov OB, Fedoreev SA, Miller GG. Polysaccharides of lichens and their sulfated derivatives: antiviral activity. Chem Nat Compd. (1998) 34:337–8. doi: 10.1007/BF02282419
46. Angelin J, Kavitha M. Exopolysaccharides from probiotic bacteria and their health potential. Int J Biol Macromol. (2020) 162:853–65. doi: 10.1016/j.ijbiomac.2020.06.190
47. Delbarre-Ladrat C SC, Lebellenger L, Zykwinska A, Colliec-Jouault S. Exopolysaccharides produced by marine bacteria and their applications as glycosaminoglycan-like molecules. Front Chem. (2014) 2:85. doi: 10.3389/fchem.2014.00085
48. Rosales-Mendoza S, García-Silva I, González-Ortega O, Sandoval-Vargas JM, Malla A, Vimolmangkang S. The potential of algal biotechnology to produce antiviral compounds and biopharmaceuticals. Molecules. (2020) 25:4049. doi: 10.3390/molecules25184049
49. Ahmadi A, Moghadamtousi SZ, Abubakar S, Zandi K. Antiviral potential of algae polysaccharides isolated from marine sources: a review. Biomed Res Int. (2015) 2015:825203. doi: 10.1155/2015/825203
50. Alboofetileh M, Rezaei M, Tabarsa M, You S. Bioactivities of Nizamuddinia zanardinii sulfated polysaccharides extracted by enzyme, ultrasound and enzyme-ultrasound methods. J Food Sci Technol. (2019) 56:1212–20. doi: 10.1007/s13197-019-03584-1
51. Gong G, Dang T, Deng Y, Han J, Zou Z, Jing S, et al. Physicochemical properties and biological activities of polysaccharides from Lycium barbarum prepared by fractional precipitation. Int J Biol Macromol. (2018) 109:611–8. doi: 10.1016/j.ijbiomac.2017.12.017
52. Vishwakarma J, Vavilala SL. Evaluating the antibacterial and antibiofilm potential of sulphated polysaccharides extracted from green algae Chlamydomonas reinhardtii. J Appl Microbiol. (2019) 127:1004–17. doi: 10.1111/jam.14364
53. Xu Y, Xu J, Ge K, Tian Q, Zhao P, Guo Y. Anti-inflammatory effect of low molecular weight fucoidan from Saccharina japonica on atherosclerosis in apoE-knockout mice. Int J Biol Macromol. (2018) 118:365–74. doi: 10.1016/j.ijbiomac.2018.06.054
54. Bahramzadeh S, Tabarsa M, You S, Li C, Bita S. Purification, structural analysis and mechanism of murine macrophage cell activation by sulfated polysaccharides from Cystoseira indica. Carbohydr Polym. (2019) 205:261–70. doi: 10.1016/j.carbpol.2018.10.022
55. Sanjeewa KKA, Kang N, Ahn G, Jee Y, Kim YT, Jeon YJ. Bioactive potentials of sulfated polysaccharides isolated from brown seaweed Sargassum spp in related to human health applications: a review. Food Hydrocoll. (2018) 81:200–8. doi: 10.1016/j.foodhyd.2018.02.040
56. Yim JH, Kim SJ, Ahn SH, Lee CK, Rhie KT, Lee HK. Antiviral effects of sulfated exopolysaccharide from the marine microalga Gyrodinium impudicum strain KG03. Mar Biotechnol. (2004) 6:17–25. doi: 10.1007/s10126-003-0002-z
57. Cho M, Yang C, Kim SM, You S. Molecular characterization and biological activities of watersoluble sulfated polysaccharides from Enteromorpha prolifera. Food Sci Biotechnol. (2010) 19:525–33. doi: 10.1007/s10068-010-0073-3
58. Cao RA, Lee Y, You S. Water soluble sulfated-fucans with immune-enhancing properties from Ecklonia cava. Int J Biol Macromol. (2014) 67:303–11. doi: 10.1016/j.ijbiomac.2014.03.019
59. Chen MZ, Xie HG, Yang LW, Liao ZH, Yu J. In vitro anti-influenza virus activities of sulfated polysaccharide fractions from Gracilaria lemaneiformis. Virol Sin. (2010) 25:341–51. doi: 10.1007/s12250-010-3137-x
60. Kim M, Yim JH, Kim SY, Kim HS, Lee WG, Kim SJ, et al. In vitro inhibition of influenza a virus infection by marine microalga-derived sulfated polysaccharide p-KG03. Antivir Res. (2012) 93:253–9. doi: 10.1016/j.antiviral.2011.12.006
61. Santoyo S, Plaza M, Jaime L, Ibañez E, Reglero G, Señorans J. Pressurized liquids as an alternative green process to extract antiviral agents from the edible seaweed Himanthalia elongata. J Appl Phycol. (2010) 23:909–17. doi: 10.1007/s10811-010-9611-x
62. Wang H, Ooi VEC, Ang PO. Anti-herpesviral property and mode of action of a polysaccharide from brown seaweed (Hydroclathrus clathratus). World J Microbiol Biotechnol. (2010) 26:1703–13. doi: 10.1007/s11274-010-0348-0
63. Wang H, Ooi EV, Ang PO Jr. Antiviral polysaccharides isolated from Hong Kong brown seaweed Hydroclathrus clathratus. Sci China C Life Sci. (2007) 50:611–8. doi: 10.1007/s11427-007-0086-1
64. Cao YG, Hao Y, Li ZH, Liu ST, Wang LX. Antiviral activity of polysaccharide extract from Laminaria japonica against respiratory syncytial virus. Biomed Pharmacother. (2016) 84:1705–10. doi: 10.1016/j.biopha.2016.10.082
65. Yue Y, Li Z, Li P, Song N, Li B, Lin W, et al. Antiviral activity of a polysaccharide from Laminaria japonica against enterovirus 71. Biomed Pharmacother. (2017) 96:256–62. doi: 10.1016/j.biopha.2017.09.117
66. Sun T, Zhang X, Miao Y, Zhou Y, Shi J, Yan M, et al. Studies on antiviral and immuno-regulation activity of low molecular weight fucoidan from Laminaria japonica. J Ocean Univ China. (2018) 17:705–11. doi: 10.1007/s11802-018-3794-1
67. Lee JB, Hayashi K, Hirata M, Kuroda E, Suzuki E, Kubo Y, et al. Antiviral sulfated polysaccharide from Navicula directa, a diatom collected from deep-sea water in Toyama Bay. Biol Pharm Bull. (2006) 29:2135–9. doi: 10.1248/bpb.29.2135
68. Talyshinsky MM, Souprun YY, Huleihel MM. Anti-viral activity of red microalgal polysaccharides against retroviruses. Cancer Cell Int. (2002) 2:8. doi: 10.1186/1475-2867-2-8
69. Jin W, Zhang W, Mitra D, McCandless MG, Sharma P, Tandon R, et al. The structure-activity relationship of the interactions of SARS-CoV-2 spike glycoproteins with glucuronomannan and sulfated galactofucan from Saccharina japonica. Int J Biol Macromol. (2020) 163:1649–58. doi: 10.1016/j.ijbiomac.2020.09.184
70. Sun Y, Chen X, Zhang L, Liu H, Liu S, Yu H, et al. The antiviral property of Sargassum fusiforme polysaccharide for avian leukosis virus subgroup J in vitro and in vivo. Int J Biol Macromol. (2019) 138:70–8. doi: 10.1016/j.ijbiomac.2019.07.073
71. Zhu W, Chiu LC, Ooi VE, Chan PK, Ang PO Jr. Antiviral property and mechanisms of a sulphated polysaccharide from the brown alga Sargassum patens against Herpes simplex virus type 1. Phytomedicine. (2006) 13:695–701. doi: 10.1016/j.phymed.2005.11.003
72. Lee J-B, Takeshita A, Hayashi K, Hayashi T. Structures and antiviral activities of polysaccharides from Sargassum trichophyllum. Carbohydr Polym. (2011) 86:995–9. doi: 10.1016/j.carbpol.2011.05.059
73. Chiu YH, Chan YL, Li TL, Wu CJ. Inhibition of Japanese encephalitis virus infection by the sulfated polysaccharide extracts from Ulva lactuca. Mar Biotechnol. (2012) 14:468–78. doi: 10.1007/s10126-011-9428-x
74. Hayashi T, Hayashi K. Calcium spirulan, an inhibitor of enveloped virus replication, from a blue-green alga Spirulina platensis. J Nat Prod. (1996) 59:83–7. doi: 10.1021/np960017o
75. Reichert M, Bergmann SM, Hwang J, Buchholz R, Lindenberger C. Antiviral activity of exopolysaccharides from Arthrospira platensis against koi herpesvirus. J Fish Dis. (2017) 40:1441–50. doi: 10.1111/jfd.12618
76. Gugliandolo C, Spano A, Lentini V, Arena A, Maugeri TL. Antiviral and immunomodulatory effects of a novel bacterial exopolysaccharide of shallow marine vent origin. J Appl Microbiol. (2014) 116:1028–34. doi: 10.1111/jam.12422
77. Kanmani P, Albarracin L, Kobayashi H, Iida H, Komatsu R, Kober AKMH, et al. Exopolysaccharides from Lactobacillus delbrueckii OLL1073R-1 modulate innate antiviral immune response in porcine intestinal epithelial cells. Mol Immunol. (2018) 98:253–65. doi: 10.1016/j.molimm.2017.07.009
78. Yang Y, Song H, Wang L, Dong W, Yang Z, Yuan P, et al. Antiviral effects of a probiotic metabolic products against transmissible gastroenteritis Coronavirus. J Probiotics Health. (2017) 5:3. doi: 10.4172/2329-8901.1000184
79. Kanekiyo K, Lee JB, Hayashi K, Takenaka H, Hayakawa Y, Endo S, et al. Isolation of an antiviral polysaccharide, nostoflan, from a terrestrial cyanobacterium, Nostoc flagelliforme. J Nat Prod. (2005) 68:1037–41. doi: 10.1021/np050056c
80. Matsuda M, Shigeta S, Okutani K. Antiviral activities of marine Pseudomonas polysaccharides and their oversulfated derivatives. Mar Biotechnol. (1999) 1:68–73. doi: 10.1007/PL00011753
81. Lee SM, Kim SM, Lee YH, Kim WJ, Park JK, Park YI, et al. Macromolecules isolated from Phellinus pini fruiting body: chemical characterization and antiviral activity. Macromol Res. (2010) 18:602–9. doi: 10.1007/s13233-010-0615-9
82. Zhao C, Gao L, Wang C, Liu B, Jin Y, Xing Z. Structural characterization and antiviral activity of a novel heteropolysaccharide isolated from Grifola frondosa against enterovirus 71. Carbohydr Polym. (2016) 144:382–9. doi: 10.1016/j.carbpol.2015.12.005
83. Ren G, Xu L, Lu T, Yin J. Structural characterization and antiviral activity of lentinan from Lentinus edodes mycelia against infectious hematopoietic necrosis virus. Int J Biol Macromol. (2018) 115:1202–10. doi: 10.1016/j.ijbiomac.2018.04.132
84. Sun QL, Li Y, Ni LQ, Li YX, Cui YS, Jiang SL, et al. Structural characterization and antiviral activity of two fucoidans from the brown algae Sargassum henslowianum. Carbohydr Polym. (2020) 229:115487. doi: 10.1016/j.carbpol.2019.115487
85. Elizondo-Gonzalez R, Cruz-Suarez LE, Ricque-Marie D, Mendoza-Gamboa E, Rodriguez-Padilla C, Trejo-Avila LM. In vitro characterization of the antiviral activity of fucoidan from Cladosiphon okamuranus against Newcastle Disease Virus. Virol J. (2012) 9:307. doi: 10.1186/1743-422X-9-307
86. Ponce NMA, Flores ML, Pujol CA, Becerra MB, Navarro DA, Cordoba O, et al. Fucoidans from the phaeophyta Scytosiphon lomentaria: chemical analysis and antiviral activity of the galactofucan component. Carbohydr Res. (2019) 478:18–24. doi: 10.1016/j.carres.2019.04.004
87. González ME, Alarcón B, Carrasco L. Polysaccharides as antiviral agents: antiviral activity of carrageenan. Antimicrob Agents Chemother. (1987) 31:1388–93. doi: 10.1128/AAC.31.9.1388
88. Carlucci MJ, Pujol CA, Ciancia M, Noseda MD, Matulewicz MC, Damonte EB, et al. Antiherpetic and anticoagulant properties of carrageenans from the red seaweed Gigartina skottsbergii and their cyclized derivatives: correlation between structure and biological activity. Int J Biol Macromol. (1997) 20:97–105. doi: 10.1016/S0141-8130(96)01145-2
89. Girond S, Crance JM, Van Cuyck-Gandre H, Renaudet J, Deloince R. Antiviral activity of carrageenan on hepatitis a virus replication in cell culture. Res Virol. (1991) 142:261–70. doi: 10.1016/0923-2516(91)90011-Q
90. Diogo JV, Novo SG, Gonzalez MJ, Ciancia M, Bratanich AC. Antiviral activity of lambda-carrageenan prepared from red seaweed (Gigartina skottsbergii) against BoHV-1 and SuHV-1. Res Vet Sci. (2015) 98:142–4. doi: 10.1016/j.rvsc.2014.11.010
91. El-Newary SA, Ibrahim AY, Asker MS, Mahmoud MG, El Awady ME. Production, characterization and biological activities of acidic exopolysaccharide from marine Bacillus amyloliquefaciens 3MS 2017. Asian Pac J Trop Med. (2017) 10:652–62. doi: 10.1016/j.apjtm.2017.07.005
92. Farag MMS, Moghannem SAM, Shehabeldine AM, Azab MS. Antitumor effect of exopolysaccharide produced by Bacillus mycoides. Microb Pathog. (2020) 140:103947. doi: 10.1016/j.micpath.2019.103947
93. Kumar CG, Mongolla P, Pombala S. Lasiosan, a new exopolysaccharide from Lasiodiplodia sp. strain B2 (MTCC 6000): Structural characterization and biological evaluation. Process Biochem. (2018) 72:162–9. doi: 10.1016/j.procbio.2018.06.014
94. Lobo RE, Gómez MI, Font de Valdez G, Torino MI. Physicochemical and antioxidant properties of a gastroprotective exopolysaccharide produced by Streptococcus thermophilus CRL1190. Food Hydrocoll. (2019) 96:625–33. doi: 10.1016/j.foodhyd.2019.05.036
95. Sahana TG, Rekha PD. A bioactive exopolysaccharide from marine bacteria Alteromonas sp. PRIM-28 and its role in cell proliferation and wound healing in vitro. Int J Biol Macromol. (2019) 131:10–8. doi: 10.1016/j.ijbiomac.2019.03.048
96. Nehal F, Sahnoun M, Smaoui S, Jaouadi B, Bejar S, Mohammed S. Characterization, high production and antimicrobial activity of exopolysaccharides from Lactococcus lactis F-mou. Microb Pathog. (2019) 132:10–9. doi: 10.1016/j.micpath.2019.04.018
97. Domingos-Lopes MFP, Nagy A, Stanton C, Ross PR, Gelencsér E, Silva CCG. Immunomodulatory activity of exopolysaccharide producing Leuconostoc citreum strain isolated from Pico cheese. J Funct Foods. (2017) 33:235–43. doi: 10.1016/j.jff.2017.03.054
98. Mizuno H, Tomotsune K, Islam MA, Funabashi R, Albarracin L, Ikeda-Ohtsubo W, et al. Exopolysaccharides from Streptococcus thermophilus ST538 modulate the antiviral innate immune response in porcine intestinal epithaliocytes. Front Microbiol. (2020) 11:894. doi: 10.3389/fmicb.2020.00894
99. He X, Fang J, Guo Q, Wang M, Li Y, Meng Y, et al. Advances in antiviral polysaccharides derived from edible and medicinal plants and mushrooms. Carbohydr Polym. (2020) 229:115548. doi: 10.1016/j.carbpol.2019.115548
100. Cardozo FT, Camelini CM, Mascarello A, Rossi MJ, Nunes RJ, Barardi CR, et al. Antiherpetic activity of a sulfated polysaccharide from Agaricus brasiliensis mycelia. Antivir Res. (2011) 92:108–14. doi: 10.1016/j.antiviral.2011.07.009
101. Rincão VP, Yamamoto KA, Ricardo NM, Soares SA, Meirelles LD, Nozawa C, et al. Polysaccharide and extracts from Lentinula edodes: Structural features and antiviral activity. Virol J. (2012) 9:37. doi: 10.1186/1743-422X-9-37
102. Tochikura TS, Nakashima H, Hirose K, Yamamoto N. A biological response modifier, PSK, inhibits human immunodeficiency virus infection in vitro. Biochem Biophys Res Commun. (1987) 2:726–33. doi: 10.1016/0006-291X(87)90936-3
103. Tochikura TS, Nakashima H, Ohashi Y, Yamamoto N. Inhibition (in vitro) of replication and of the cytopathic effect of human immunodeficiency virus by an extract of the culture medium of Lentinus edodes mycelia. Med Microbiol Immunol. (1988) 177:235–44. doi: 10.1007/BF00189409
104. Ryu WS. Virus life cycle. In: Ryu WS, editor. Molecular Virology of Human Pathogenic Viruses. Cambridge, MA: Academic Press (2017). p. 31–45.
105. Seo DJ, Changsun C. Antiviral bioactive compounds of mushrooms and their antiviral mechanisms: a review. Viruses. (2021) 13:350. doi: 10.3390/v13020350
106. Wang W, Wang SX, Guan HS. The antiviral activities and mechanisms of marine polysaccharides: an overview. Mar Drugs. (2012) 10:2795–816. doi: 10.3390/md10122795
107. Shi Q, Wang A, Lu Z, Qin C, Hu J, Yin J. Overview on the antiviral activities and mechanisms of marine polysaccharides from seaweeds. Carbohydr Res. (2017) 453–454:1–9. doi: 10.1016/j.carres.2017.10.020
108. Wang J, Wu T, Fang X, Min W, Yang Z. Characterization and immunomodulatory activity of an exopolysaccharide produced by Lactobacillus plantarum JLK0142 isolated from fermented dairy tofu. Int J Biol Macromol. (2018) 115:985–93. doi: 10.1016/j.ijbiomac.2018.04.099
109. Wang N, Jia G, Wang C, Chen M, Xie F, Nepovinnykh NV, et al. Structural characterisation and immunomodulatory activity of exopolysaccharides from liquid fermentation of Monascus purpureus (Hong Qu). Food Hydrocoll. (2020) 103:105636. doi: 10.1016/j.foodhyd.2019.105636
110. Liu ZH, Niu FJ, Xie YX, Xie SM, Liu YN, Yang YY, et al. A review: natural polysaccharides from medicinal plants and microorganisms and their anti-herpetic mechanism. Biomed Pharmacother. (2020) 129:110469. doi: 10.1016/j.biopha.2020.110469
111. Liu C, Chen H, Chen K, Gao Y, Gao S, Liu X, et al. Sulfated modification can enhance antiviral activities of Achyranthes bidentata polysaccharide against porcine reproductive and respiratory syndrome virus (PRRSV) in vitro. Int J Biol Macromol. (2013) 52:21–4. doi: 10.1016/j.ijbiomac.2012.09.020
112. Sinha S, Astani A, Ghosh T, Schnitzler P, Ray B. Polysaccharides from Sargassum tenerrimum: structural features, chemical modification and anti-viral activity. Phytochemistry. (2010) 71:235–42. doi: 10.1016/j.phytochem.2009.10.014
113. Bandyopadhyay SS, Navid MH, Ghosh T, Schnitzler P, Ray B. Structural features and in vitro antiviral activities of sulfated polysaccharides from Sphacelaria indica. Phytochemistry. (2011) 72:276–83. doi: 10.1016/j.phytochem.2010.11.006
114. Li S, Xiong Q, Lai X, Li X, Wan M, Zhang J, et al. Molecular modification of polysaccharides and resulting bioactivities. Compr Rev Food Sci Food Saf. (2016) 15:237–50. doi: 10.1111/1541-4337.12161
115. Kalitnik AA, Byankina Barabanova AO, Nagorskaya VP, Reunov AV, Glazunov VP, Solov'eva TF, et al. Low molecular weight derivatives of different carrageenan types and their antiviral activity. J Appl Phycol. (2012) 25:65–72. doi: 10.1007/s10811-012-9839-8
116. Mader J, Gallo A, Schommartz T, Handke W, Nagel CH, Günther P, et al. Calcium spirulan derived from Spirulina platensis inhibits herpes simplex virus 1 attachment to human keratinocytes and protects against herpes labialis. J Allergy Clin Immunol. (2016) 137:197–203. doi: 10.1016/j.jaci.2015.07.027
117. Kwon PS, Oh H, Kwon SJ, Jin W, Zhang F, Fraser K, et al. Sulfated polysaccharides effectively inhibit SARS-CoV-2 in vitro. Cell Discov. (2020) 6:50. doi: 10.1038/s41421-020-00192-8
118. Lee AJ, Ashkar AA. The dual nature of type I and type II interferons. Front Immunol. (2018) 9:2061. doi: 10.3389/fimmu.2018.02061
119. Ivashkiv LB, Donlin LT. Regulation of type I interferon responses. Nat Rev Immunol. (2014) 14:36–49. doi: 10.1038/nri3581
120. Hadjadj J, Yatim N, Barnabei L, Corneau A, Boussier J, Smith N, et al. Impaired type I interferon activity and inflammatory responses in severe COVID-19 patients. Science. (2020) 369:718–24. doi: 10.1126/science.abc6027
121. Hu B, Guo H, Zhou P, Shi ZL. Characteristics of SARS-CoV-2 and COVID-19. Nat Rev Microbiol. (2021) 19:141–54. doi: 10.1038/s41579-020-00459-7
122. Cheng J-J, Chao C-H, Chang P-C, Lu M-K. Studies on anti-inflammatory activity of sulfated polysaccharides from cultivated fungi Antrodia cinnamomea. Food Hydrocoll. (2016) 53:37–45. doi: 10.1016/j.foodhyd.2014.09.035
123. Wu G-J, Shiu S-M, Hsieh M-C, Tsai G-J. Anti-inflammatory activity of a sulfated polysaccharide from the brown alga Sargassum cristaefolium. Food Hydrocoll. (2016) 53:16–23. doi: 10.1016/j.foodhyd.2015.01.019
124. Dinić M, Pecikoza U, Djokić J, Stepanović-Petrović R, Milenković M, Stevanović M, et al. Exopolysaccharide produced by probiotic strain Lactobacillus paraplantarum BGCG11 reduces inflammatory hyperalgesia in rats. Front Pharmacol. (2018) 9:1. doi: 10.3389/fphar.2018.00001
125. Kansandee W, Moonmangmee D, Moonmangmee S, Itsaranuwat P. Characterization and Bifidobacterium sp. growth stimulation of exopolysaccharide produced by Enterococcus faecalis EJRM152 isolated from human breast milk. Carbohydr Polym. (2019) 206:102–9. doi: 10.1016/j.carbpol.2018.10.117
Keywords: sulfated polysaccharides, immunomodulation, SARS-CoV-2, COVID-19, antiviral activity
Citation: Chaisuwan W, Phimolsiripol Y, Chaiyaso T, Techapun C, Leksawasdi N, Jantanasakulwong K, Rachtanapun P, Wangtueai S, Sommano SR, You S, Regenstein JM, Barba FJ and Seesuriyachan P (2021) The Antiviral Activity of Bacterial, Fungal, and Algal Polysaccharides as Bioactive Ingredients: Potential Uses for Enhancing Immune Systems and Preventing Viruses. Front. Nutr. 8:772033. doi: 10.3389/fnut.2021.772033
Received: 07 September 2021; Accepted: 15 October 2021;
Published: 05 November 2021.
Edited by:
Ding-Tao Wu, Chengdu University, ChinaReviewed by:
Kit Leong Cheong, Shantou University, ChinaBin Du, Hebei Normal University of Science and Technology, China
Copyright © 2021 Chaisuwan, Phimolsiripol, Chaiyaso, Techapun, Leksawasdi, Jantanasakulwong, Rachtanapun, Wangtueai, Sommano, You, Regenstein, Barba and Seesuriyachan. This is an open-access article distributed under the terms of the Creative Commons Attribution License (CC BY). The use, distribution or reproduction in other forums is permitted, provided the original author(s) and the copyright owner(s) are credited and that the original publication in this journal is cited, in accordance with accepted academic practice. No use, distribution or reproduction is permitted which does not comply with these terms.
*Correspondence: Phisit Seesuriyachan, cGhpc2l0LnNAY211LmFjLnRo