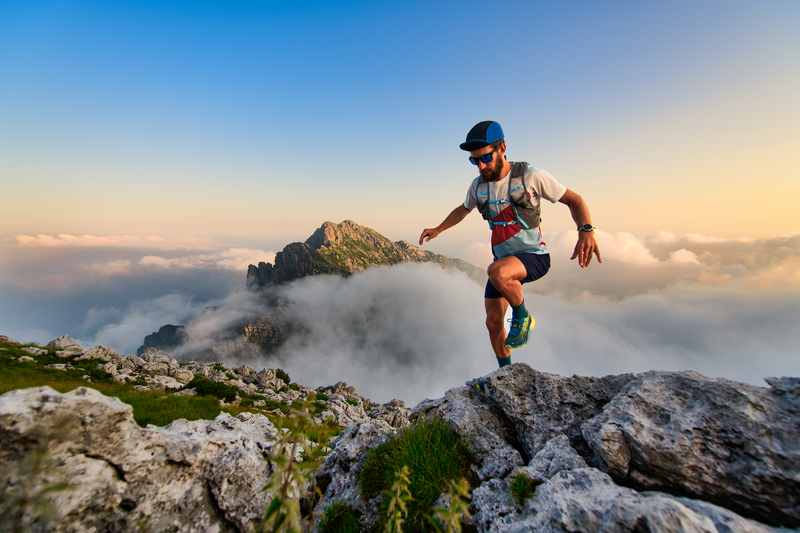
95% of researchers rate our articles as excellent or good
Learn more about the work of our research integrity team to safeguard the quality of each article we publish.
Find out more
ORIGINAL RESEARCH article
Front. Nutr. , 25 November 2021
Sec. Nutrition and Microbes
Volume 8 - 2021 | https://doi.org/10.3389/fnut.2021.737059
This article is part of the Research Topic The Interaction between Digestive Tract Microbes and Hosts in Poultry View all 25 articles
Monochromatic light is widely used in industry, medical treatment, and animal husbandry. Green-blue light has been found to stimulate the proliferation of satellite cells and the results of studies on the effects of blue light on poultry vary widely. It would be worthwhile to study the effect of blue light on poultry growth and how exposure to blue light affects metabolism and the intestinal microbiota. In this study, we irradiated Cherry Valley ducks with 460 nm wavelength light (blue light) for 3 weeks to explore the effects of blue light in comparison to those of white light (combined wavelength light) on animal growth and development. Our results showed that, under exposure to blue light, the body weight and average daily feed intake of ducks were decreased, but the leg muscle and relative length of the intestine were increased. Exposure to blue light chiefly enhanced the anti-inflammatory and antioxidant capacities of the animal and decreased lipid levels in serum and liver. Metabolomic analysis revealed that blue light heightened cysteine and methionine metabolism, and increased serum taurine and primary bile acid levels, as well as up-regulating the metabolites L-carnitine and glutamine. Treatment with blue light significantly increased the beta diversity of intestinal microbiota and the relative abundances of bile acid hydrolase-producing bacteria, especially Alistipes. These changes promote the synthesis of secondary bile acids to further enhance lipid metabolism in the host, thereby reducing cholesterol accumulation in ducks. These results should help us better understand the effects of exposure to blue light on metabolite levels and the intestinal microbiota, and suggest that it may be possible to use colored light to control the development of livestock and poultry.
Illumination plays an important role in the regulation of circadian rhythm and metabolic homeostasis. Monochromatic light is widely used in animal husbandry to influence embryo development and poultry production (1). Compared with mammals, birds have a unique visual adjustment mechanism, superior visual function, and a photosensitive range that has been ascribed to ciliary muscles and four types of retinal cone cells (2–4). However, it is unknown whether avian species have an intrinsic metabolic mechanism and microbial structure under stimulation with monochromatic light. Blue light is colored light with a wavelength ranging between 400 and 500 nm (5). Due to its fruitful broad-spectrum antibacterial effect (6–8), LED blue-light irradiation has been used for the treatment of skin diseases and wound sterilization (9–11). Various studies have revealed that blue light also plays a role in regulating physiological functions in vivo, e.g., it improves cognitive performance (12), promotes cell proliferation and differentiation (13, 14), and even inhibits tumor cell growth and metastasis (15, 16).
Investigations of the effects of blue light on poultry have not provided definitive conclusions. Several studies have indicated that blue light and blue-green light promote growth performance and improve the fatty acid composition in Cherry Valley ducks, and green-blue light stimulated the proliferation of chicken satellite cells (17, 18). In some cases, blue light clearly reduced the body weight of ducks at ages 1–35 days (19). However, whether and how exposure to blue light impacts the metabolic processes of poultry is unclear.
In this study, we hypothesized that blue light may reduce growth performance and lipid deposition in ducks, and impact the composition of the intestinal microbiota. Our results revealed that treatment with blue light improved bile acid synthesis in the intestine and suppressed cholesterol accumulation. Collectively, the results of this study suggest that exposure to blue light could intensify lipid metabolism and may be exploited for the regulation of growth and development.
Cherry Valley ducks (1-day-old, all male) were purchased from Foshan Guiliu Poultry Co. Ltd. (Foshan, China). A total of 120 ducklings were randomly divided into two groups, with 6 replicates for each treatment and 10 birds for each replicate; the breeding density was set to 6 per/m2. Birds were placed in environmentally controlled light-proof rooms separated from each other and exposed to white light (380–780 nm) and blue light (460 nm), respectively. Twenty-four hours of lighting were provided from light-emitting diode (LED) bulbs at 10 Lux for 21 days (Figure 1A). The photosynthetic photon flux density (PPFD) of the white light was 0.2 μmoL/s/m2 and that of blue light was 0.036 μmoL/s/m2 (Nuodake, Wuxi, China); LED lamp spacing 0.8 m, light-source calibration twice a week.
Figure 1. Exposure to blue light reduces body weight but increases the muscle percentage in ducklings. (A) Scheme of the experiments. (B) Effect of exposure to blue light on growth performance of ducks from days 1–21 (n = 6, mean with SEM). (C) Effect of exposure to blue light on the absolute weight and percentage of breast muscle. (D) Effect of exposure to blue light on the absolute weight and the percentage of leg muscle. (E) The effect of blue light irradiation on the relative length and weight of duck jejunum. (F) The effect of blue light irradiation on the relative length and weight of duck ileum. Data in (B–F) were analyzed using the unpaired t-test, *P < 0.05, **P < 0.01, ***P < 0.001.
The body weight, feed intake, and ratio of feed to gain of the animals were regularly recorded during the treatment period. The room temperature was maintained at 32–34°C for the first 3 days, and then reduced from 2 to 3°C per week to a final temperature of 26°C. The experimental period lasted for 3 weeks. Diets were formulated to meet the China Nutrient requirements for meat-type duck (2012) (Table 1).
After 3 weeks of rearing, one bird of average weight from each group was selected for slaughter. Animals were sacrificed by cervical dislocation, and 10 ml of blood was collected from the jugular vein; these procedures followed the agricultural industry standard of the People's Republic of China (NY/T 3741-2020). Plasma was prepared by centrifuging the blood at 3,000 r/min for 10 min and then stored at −20°C. The middle part of liver samples was collected for molecular biological analysis after washing with phosphate-buffered saline (PBS, pH = 7.2–7.4). Samples of the duodenum, jejunum, and ileum were snap-frozen in liquid nitrogen and stored at −80°C for mRNA analysis for RT-PCR.
Total cholesterol (TC) and activities of catalase (CAT), total superoxide dismutase (T-SOD), total antioxidant capability (T-AOC), and glutathione peroxidase (GSH-Px) in plasma and liver contents were assayed by colorimetric methods using commercial kits (Jiancheng, Nanjing, China). Insulin-like growth factor-1 (IGF-1), adiponectin, and leptin were determined using ELISA kits according to the manufacturer's instructions (Jiancheng, Nanjing, China).
In this study, UHPLC-MRM-MS/MS was used for high-throughput metabolomics detection and analysis on duck serum samples in the white- and blue-light groups. Chromatographic conditions and mass spectrometry were performed under the conditions described at www.researchsquare.com.
DNA extraction, PCR amplification, MiSeq sequencing, and processing of sequencing data were carried out as described at pubs.rsc.org.
ELISA kits (Nanjing Jiancheng Institute of Biotechnology, Nanjing, Nanjing) were used to measure the levels of cytokines including IL-2, IL-6, TNF-α, and IFN-γ in the liver, according to the manufacturer's instructions.
Total RNA was extracted from frozen tissue samples using a Magen HI Pure Universal RNA Mini Kit (Magen, Guangzhou, China). The first-strand cDNA was synthesized using oligonucleotide 20 and Superscript II reverse transcriptase (EZB, Guangzhou, China). Real-time PCR was performed on an ABI 7500 (Applied, Bio-Systems, Foster City, CA) using a SYBR Green Quantitative PCR Kit (TaKaRa, Japan). The mRNA expression of each gene was calculated by the 2−ΔΔCt method. β-actin was used as an internal standard to normalize the transcription level of target genes. Use the following primers for transcription analysis of related genes: ACC: 5′-TAG CCA TGC AGC CAC TTT GA-3′, 3′-ACC TTT GTA CGA GCT GCA CA-5′, FAS: 5′-GGA AGT GCC CCA GTT GAA GA-3′, 3′-GCT GCA ATG CCC CAT GAT G-5′, ChREBP: 5′-ACA TCA TCT TGC GGC AGT GA-3′, 3′-TGT GAT ACG CCG GCT TTC TAT-5′, FABP: 5′-ATG AGA CCA CAG CAG ATG ACA-3′, 3′-TTT GCC ATC CCA CTT CTG CA-5′, LPL: 5′-AAG CTC TGC GTC TGA TTG CT-3′, 3′-TGC TGG GCT TTT CTT CGT AGA-5′, SREBP-1: 5′- CAT CCA TCA ACG ACA AGA TCG T-3′, 3′-CTC AGG ATC GCC GAC TTG TT-5′, β-Actin (5′- GCT ATG TCG CCC TGG ATT T-3′, 3′-GGA TGC CAC AGG ACT CCA TAC-5′) was used as an internal control to normalize the transcription level of target genes.
Data are shown as mean ± SEM and SAS 9.2 (SAS Institute, Inc., Cary, NC) was used for statistical analysis. Significant differences were evaluated by an unpaired Student's t-test or the Mann-Whitney U test on both sides of non-normally distributed samples. Differences were considered to be significant at P < 0.05; *P < 0.05; **P < 0.01; ***P < 0.001; and ****P < 0.0001.
The body weight and feed intake of ducklings were monitored during the experiment to explore the effect of exposure to blue light (Figure 1A). We found that exposure to blue light significantly decreased the final weight (P = 0.001) and Average Daily Gain (ADG, P = 0.001) compared to those in the white-light group (Figure 1B). Interestingly, although the weight of ducklings in the blue-light group decreased, the absolute weight of their breast muscle and leg muscle tended to increase, and the ratio of breast muscle to leg muscle significantly increased (P < 0.05; Figures 1C,D). In addition, the relative length and weight of the jejunum of the ducklings in the blue-light group were significantly higher than those in the white-light group (P < 0.05), and the relative length and weight of the ileum also tended to be higher. These results demonstrate that exposure to blue light affects the growth and development of ducks (Figures 1E,F and Supplementary Figure S1).
Exposure to blue light significantly increased serum CAT (P < 0.05) and T-AOC levels (P < 0.001), and significantly increased GSH-Px and T-SOD levels in the liver (P < 0.001; Figures 2A,B). We also measured the levels of inflammatory cytokines in serum, and found that exposure to blue light significantly reduced the levels of pro-inflammatory cytokines such as IL-2 (P < 0.05) and IL-6 (P < 0.001) compared to those in the white-light group (Figure 2C). In general, these results indicate that exposure to blue light could improve antioxidant and anti-inflammatory capacities in ducks.
Figure 2. Exposure to blue light improves the antioxidant and anti-inflammatory capacities in ducks. (A) Effect of exposure to 460 nm LED blue light on the serum antioxidant indicators CAT, GSH-Px, T-SOD, and T-AOC in ducks (n = 6, mean with SEM). (B) Effect of exposure to blue light on the liver antioxidant indicators CAT, GSH-Px, T-SOD, and T-AOC in ducks (n = 6, mean with SEM). (C) Effect of exposure to blue light on serum cytokine levels in ducks, including IL-2 IL-6, TNF-α, and IFN-γ (n = 6, mean with SEM). Data in (A–C) were analyzed using the unpaired t-test, *P < 0.05, **P < 0.01, ***P < 0.001.
The increase in muscle weight and the decrease in body weight occurred at the same time, along with the improvements in antioxidant and anti-inflammatory capacities. These changes suggest that blue light may have an ameliorative effect on lipid metabolism. Therefore, we determined the levels of hormones in serum, the expression of genes related to lipid metabolism in the liver, and the content of cholesterol in the liver and serum.
Serum IGF-1 and leptin levels were down-regulated in the blue-light group (P < 0.01), while adiponectin and melatonin were up-regulated (P < 0.01; Figure 3A); exposure to blue light reduced the ratio of leptin to adiponectin (L/A) to 25.47% of that in the white-light group (P < 0.05; Figure 3B). The L/A ratio is a critical index of insulin resistance and obesity, and its elevation is often accompanied by metabolic diseases (20). Blue light also significantly reduced the expression levels of genes involved in fat synthesis, including ACC, FABP, and LPL, in the liver (P < 0.05; Figure 3C). Furthermore, irradiation with blue light also significantly reduced the accumulation of cholesterol in duck liver and serum (P < 0.05; Figure 3D): the cholesterol content in the liver was reduced by 47.1%, and that in the serum was reduced by 17.8%. These results collectively suggest that exposure to blue light can improve the efficiency of energy conversion and lipid metabolism, especially cholesterol metabolism.
Figure 3. Exposure to blue light reduces the accumulation of cholesterol in the liver and serum. (A) Effect of blue light on serum hormone levels of IGF-1, leptin, adiponectin, and melatonin in ducks (n = 6, mean with SEM). (B) Effect of blue light on serum ratio of leptin to adiponectin in ducks (n = 6, mean with SEM). (C) Relative mRNA expression of FAS, ACC, FABP, ChREBP, SREBP, and LPL in the liver of blue light-exposed ducks (n = 6, mean with SEM). (D) Effect of blue light on the liver and serum total cholesterol levels in ducks (n = 6, mean with SEM). Data in (A–D) were analyzed using the unpaired t-test, *P < 0.05, **P < 0.01.
To further explore the underlying mechanism by which irradiation with blue light regulates lipid metabolism, we performed a non-target metabolomics analysis of serum in the two groups. In this study, we extracted a total of 1,684 and 1,933 metabolic features in the POS (Positive) mode and NEG (Negative) mode, respectively. According to the OPLS-DA analysis, there was a vast metabolic difference between the white-light and blue-light groups (Figure 4A). The results of 200 permutation tests demonstrated that the developed models do not overfit (Supplementary Figure 2A). We calculated the Euclidean distance matrix of the quantitative values of the different metabolites, calculated the aggregated differential metabolites using the full-link method, and displayed the different metabolites using a heat map (Supplementary Figure 2). A total of 105 metabolite biomarkers were significantly different between the two groups, and exposure to blue light up-regulated 102 of them, which was also consistent with volcano plots (Figure 4B). Based on the KEGG database, MetaboAnalyst (4.0) was used to analyze the most relevant metabolic pathways that were changed by exposure to blue light, and we focused on the metabolic pathways for methionine, cysteine and lysine (Figure 4C).
Figure 4. Exposure to blue light improves glutathione and taurine levels in ducks by promoting methionine and cysteine metabolism. (A) OPLS-DA score plots of metabolomics (n = 6). (B) Volcano plot representation of the differences in metabolites (n = 6). (C) Metabolic pathway bubble chart (n = 6). (D) Heatmap of LC-MS data showing metabolite changes after LED treatment with 460 nm illumination (n = 6). (E) Correlation analysis of the serum L-carnitine level and glutamine level with breast muscle percentage and leg muscle percentage. Increases in metabolite levels are shown in red. Data in d were analyzed using the unpaired t-test, *P < 0.05, **P < 0.01. Data in (E) were analyzed using the computer non-parametric Spearman correlation ***P < 0.001.
As an essential precursor of cellular methylation, methionine is an indispensable substance in various physiological and biochemical processes (21). According to the pathway analysis, most of the metabolic biomarkers in this pathway were up-regulated (Figure 4D), which suggested that cysteine and methionine metabolism were positively associated with exposure to blue light in ducks. Compared to the white-light group, levels of methionine, homocysteine, cysteine, serine, γ-glutamyl cysteine, and glutathione were elevated (P < 0.05), with fold-changes of 1.96, 1.21, 1.38, 1.60, 2.06, and 2.02, respectively, in the blue-light group. Notably, compared to that in the white-light group, the taurine content in the blue-light group was also increased (P = 0.057). Under exposure to blue light, the metabolic pathways of lysine were also upregulated (Figure 4C). As a downstream metabolite of methionine and lysine, the L-carnitine level was significantly up-regulated [P < 0.01; (22)]. The serum glutamine content also tended to increase (P = 0.076; Figure 4D). Since both of these metabolites are associated with fat metabolism and muscle production, we analyzed the correlation between them and the muscle percentage. L-Carnitine and glutamine levels were positively correlated with the breast muscle rate and leg muscle percentages (Figure 4E).
In summary, exposure to blue light can significantly promote the metabolism of cysteine and methionine in ducks, leading to an increase in the content of the final metabolites: glutathione and taurine.
Glutathione and taurine are strongly correlated with antioxidant function (23, 24). This would explain the enhanced anti-oxidation capacity in the blue-light group mentioned above. Here, we examine the relationship between taurine and cholesterol metabolism (25). Taurine can improve the activity of cholesterol 7α-hydroxylase (CYP7A1), which converts cholesterol into primary bile acids (26) (Figure 5A).
Figure 5. Exposure to blue light promotes bile acid synthesis. (A) Effects of blue light on synthesis and secretion of primary and secondary bile acids in ducks. (B) Effects of blue light on primary bile acid levels of cholic acid, chenodeoxycholic acid, and taurocholic acid in ducks (n = 6, mean with SEM). (C) Effects of blue light on secondary bile acid levels of deoxycholic acid, lithocholic acid, and taurodeoxycholic acid in ducks (n = 6, mean with SEM). Data in (B,C) were analyzed using the unpaired t-test, *P < 0.05.
Exposure to blue light significantly increased primary bile acid levels (e.g., cholic acid, chenodeoxycholate, and taurocholate). In particular, the taurocholate level in the blue-light group was almost four times higher than that in the white-light group (P < 0.05; Figure 5B). Notably, compared to the white-light group, secondary bile acid levels (e.g., deoxycholic acid, lithocholic acid, and taurodeoxycholic acid) were elevated in the blue-light group, with fold-changes of 1.928, 2.173, and 2.335, respectively (Figure 5C).
Secondary bile acids are synthesized and metabolized in the intestine and are dominated by the intestinal microbiota. As mentioned above, the content of secondary bile acids in the blue-light group tended to be up-regulated, so the influence of blue light on intestinal microbes should also be considered. To illustrate the interaction between the intestinal microbiota and bile acids, we analyzed the cecum microbiota by 16S rRNA gene sequencing.
According to a PCA analysis, the cecal microbiota was significantly different between the two groups (Figure 6A). The β diversity of the microbiota in the blue-light group was significantly higher than that in the white-light group (Figure 6B). Next, we performed an LDA effect size (LEfSe) analysis to identify changes in the relative abundance of the intestinal microbiota at the phylum, order, and genus levels (Figure 6C; Supplementary Figure 3). Notably, exposure to blue light significantly up-regulated the relative abundance of Alistipes (P < 0.0001), Parabacteroides Clostridiales (P < 0.01), and Lachnospiraceae (P < 0.05) in the cecum, and the relative abundance of Clostridiales also increased (P = 0.067; Figure 6D). Interestingly, the changes in the above bacteria are all related to the up-regulation of bile acid conversion and metabolism, and have all been found to have alleviating effects on obesity (27). Subsequently, we performed a correlation analysis on the relative abundance of intestinal microbiota with the bile acid content and cholesterol levels. The relative abundance of these bacteria is positively correlated with the content of bile acids, and negatively correlated with the level of cholesterol (Figure 6E).
Figure 6. Exposure to blue light alters the intestinal microbiota. (A) Principal component analysis of microbial communities in the cecum from the white-light and blue-light groups (n = 6). (B) Beta diversity of microbial communities from the white-light and blue-light groups (n = 6). (C) Relative abundance of bacteria at the phylum level. OTUs with an occurrence lower than 1% are not shown. Relative abundance of the top 10 orders in each group (n = 6); Relative abundance of the top 10 genus in each group (n = 6). (D) Effect of blue light on the relative abundance of Alistpes, Clostridiales, Parabacteroides, and Lachnospiraceae (n = 6, mean with SEM). (E) Correlation analysis between microbiota and metabolites. Each row represents a different microorganism, and each column represents a different metabolite. Red indicates a positive correlation; blue indicates a negative correlation. Data in d were analyzed using the unpaired t-test, *P < 0.05, **P < 0.01, ****P < 0.0001. Data in (E) were analyzed using the computer non-parametric Spearman correlation, *P < 0.05, **P < 0.01.
These results indicate that blue light effectively shapes the intestinal microbiota of ducks and regulates the synthesis of bile acids through this process, ultimately reducing the accumulation of cholesterol.
Blue light refers to monochromatic light in a wavelength between 400 and 500 nm (5). Typically, short-wavelength blue light (400–450 nm) is considered to be toxic, while blue light at 450–480 nm is beneficial (13). Thus, in this study, we selected blue light with a wavelength of 460 nm to irradiate ducklings to explore its influence on the growth and development of animals compared with composite-wavelength light (380–780 nm). Our study is the first to offer experimental evidence that to blue light reduces cholesterol accumulation by altering taurine metabolism, shaping the intestinal microbiota, and promoting bile acid synthesis. Importantly, our findings demonstrate that exposure to blue light may have the potential to prevent obesity and alleviate inflammation, and may provide a new method for studying the influence of artificial light of different wavelengths as well as a foundation for research on the effects of monochromatic light in other scenarios.
Irradiation with blue light significantly reduced the weight of ducklings, but increased the weights of the breast and leg muscles, as well as their ratio. Blue light has been reported to promote broiler growth by stimulating testosterone secretion and muscle fiber growth (28). Other studies have reported that both blue light alone and a combination of blue and green light improve growth performance, bone mineral density, and fatty acid composition in ducks (17). A study on Pekin ducks revealed that blue light significantly reduced body weight and increased anxiety levels (19). These inconclusive results suggest that the effect of blue light on growth performance of animals may depend on their age, illumination intensity, light wavelength and other conditions. Further studies will be needed to clarify these points.
In this work, we found that exposure to blue light significantly reduced the IGF-1 level in serum. The elevation of IGF-1 is usually considered to be a sign of obesity and insulin resistance (29). Similarly, green light also regulates the muscle satellite cell proliferation mediated by the IGF-1 pathway (30). On the other hand, exposure to blue light significantly reduces the serum leptin and L/A levels, which suggests the optimization of lipid generation and a reduced risk of obesity (20). Significantly, the serum melatonin level is increased under exposure to blue light, possibly because blue light directly regulates hormone production through receptors in the retina and acts on the hypothalamus. The contribution of melatonin to lipid mechanism has been reported in experiments in high-fat diet mice and melatonin could up-regulate the relative abundance of Alistipes in the gut and thereby alleviate obesity in mice (31).
To accurately explain the role of blue light in promoting lipid metabolism and weight loss, we performed a serum metabolomics analysis in the white- and blue-light groups. Our results showed that exposure to blue light could significantly upregulate the metabolism of methionine and cysteine, thus increasing the levels of two end products: glutathione and taurine (32). Broadly, glutathione and taurine are closely related to antioxidant and lipid metabolism processes. As a powerful antioxidant, glutathione directly removes hydrogen peroxide from the organism, thereby alleviate the obesity process by decreasing lipid peroxidation (33). Therefore, glutathione depletion is usually considered to be an early warning sign of lipid peroxidation (34, 35). Simultaneously, as an isogeny product of glutathione, taurine is closely related to its synthesis. Moreover, taurine could promote SOD1 enzyme activity and inhibit mitochondrial complexes I and III to reduce ROS production (36, 37). Consequently, the increase in the antioxidant capacity of the liver and serum under irradiation by blue light is probably due to the increase in taurine and glutathione levels. In other words, the blue light-mediated up-regulation of methionine and cysteine metabolism may further promote antioxidant capacity in the duck.
The increase in the L-carnitine level is the result of the up-regulation of lysine and methionine metabolism (38). L-Carnitine is synthesized from the substrate 6-N-trimethyl-lysine; lysine residues in some proteins undergo N-methylation using S-adenosylmethionine as a methyl donor, forming 6-N-trimethyl-lysine residues (39). L-Carnitine is closely related to muscle production and fat metabolism. It is also well-known as a fat-burning agent, but it is rarely used in animal husbandry (40). It has been reported in the literature that the addition of L-carnitine to the summer diet improves the growth performance of ducks (41). Notably, L-carnitine significantly improved nutrient digestibility coefficients in ducks. Under blue light the serum glutamine level also tends to be up-regulated. Recent studies have reported that glutamine activated an mTOR signaling pathway and stimulated the proliferation of muscle satellite cells (42). Meanwhile, glutamine could also improve the oxidative damage in broiler thin muscle (43, 44). A correlation analysis of these two metabolites in serum and muscle development showed that blue light increased the contents of L-carnitine and L-glutamine by up-regulating the metabolism of methionine and cysteine, thereby promoting muscle development in ducks.
This study also demonstrated that blue light had an inhibitory effect on inflammatory cytokines, which is mostly reflected in the promotion of wound healing in vitro. Indeed, Lehrl et al. (12) reported that exposure to blue light attenuated the cognitive impairment caused by neuroinflammation. In this study, blue light also promoted anti-inflammatory capacity inside the body. We speculate that blue light may inhibit inflammatory cytokines through multiple pathways: i.e., via hormonal regulation (e.g., leptin, adiponectin, and melatonin) or the up-regulation of antioxidant levels (45–47).
In addition to the aforementioned antioxidant and cytoprotective effects, recent studies have shown that the use of taurine supplements can effectively reduce obesity (48). With the promotion of autophagy in adipocytes (49), taurine can inhibit the production of white adipose tissue (50, 51). More importantly, Guan and Miao (52) reported that taurine supplementation reduced total cholesterol and triglyceride levels in serum, thereby lowering blood pressure and improving blood lipids. Our research found that exposure to blue light can also significantly reduce serum and liver cholesterol levels, which may be related to changes in the taurine content. This result also suggests that blue-light regulation of cholesterol metabolism may play a pivotal role in achieving body weight loss.
After cholesterol is metabolized, bile acids are produced in the liver. As a downstream metabolite of cholesterol, bile acid can be classified as primary bile acid or secondary bile acid (53). The production, metabolism, and circulation of bile acids is a complex process. In the liver, cholesterol is converted into primary bile acids before it passes into the intestine. In the intestine, bacteria that produce bile acid hydrolase (BSH) convert primary bile acids into secondary bile acids. The interaction between intestinal flora and bile acids is homeostatic and synergetic. The intestinal microbiota affects the production and enterohepatic circulation of bile acids, while bile acids could also affect the gut microbe composition (54). Bile acid plays a key role in nutrient absorption and distribution, metabolism regulation, and homeostasis (55). Bile acid chelator supplement has even been used for the treatment of obesity (56).
Therefore, after noting the down-regulated cholesterol levels following exposure to blue light, we subsequently measured bile acid levels in serum. Interestingly, both primary and secondary bile acid levels tended to be up-regulated. The higher content of primary bile acids is predictable because taurine plays an indispensable role in converting cholesterol to bile acids. Taurine activates the expression of the CYP7A1 gene to promote cholesterol conversion and could be used as a precursor binding to cholesterol to form bile salts (57, 58). In addition to promoting lipid digestion, bile acids are also specific receptors for farnesol X (FXR). After FXR is activated, it can reduce the accumulation of cholesterol and triglycerides and relieve obesity (59–61). Our results indicated that exposure to blue light might control body weight by regulating cholesterol and bile acid homeostasis.
Despite these findings, secondary bile acid levels also tended to increase after blue-light irradiation. Generally, gut microbes play an integral role in converting primary bile acids to secondary bile acids (62). The results of 16S rRNA sequencing also showed that exposure to blue light significantly increased the abundance of Clostridiales, Alistipes, Parabacteroides, and Lachnospiraceae in the duck cecal microbiota. Clostridium scindens and Extibacter muris have been reported to metabolize primary bile acids to deoxycholic acid in mice (63). Moreover, as BSH-produced bacteria, Clostridiales play an essential role in converting and refluxing bile acids (64). In this experiment, exposure to blue light significantly increased the relative abundance of Clostridiales, and the content of deoxycholic acid was increased 1.92-fold. The relative abundance of Alistipes was also strongly up-regulated and that in the blue-light group reached 5.4-fold that in the white-light group, which may be due to the unique resistance of Alistipes to bile acids (65). The up-regulation of Alistipes may also be related to the alleviation of metabolic disorders and the reduction of cholesterol content. Various studies have explored the efficacy of Alistipes against obesity. For instance, Alistipes could reduce lipid metabolism disorder induced by a high-fat diet in mice by producing acetic acid (31). The up-regulation of probiotics such as Alistipes also stimulates CYP7A and promotes cholesterol conversion to bile acids (66). In addition, studies have shown that a high abundance of Alistipes can increase the intestinal absorption of cholesterol and reduce the synthesis of cholesterol in the liver (67). Thus, Alistipes seems to be able to directly use cholesterol to promote its own proliferation and further reduce the accumulation of cholesterol in the liver and serum. Furthermore, Parabacteroides has been reported to improve succinate and secondary bile acid levels and play critical roles in modulating host metabolism (68). In our study, the relative abundance of Parabacteroides in the blue-light group also increased significantly. In addition, Lachnospiraceae, as a kind of butyrate-producing probiotic, promotes the growth and development of the intestines (69). Irradiation with blue light increases the relative length of the intestine, which is probably the result of the increase in the relative abundance of short-chain fatty acid-producing bacteria, and the increase in the β diversity of the gut microbes in the blue-light group also increases the stability of the gut microecology (16, 70, 71).
More importantly, previous studies have found that the relative abundance of Lachnospiraceae in the intestines of obese mice is significantly down-regulated (72). These results suggested that blue light could shape the intestinal microbiota and regulate the changes in the relative abundance of BSH-related bacteria (e.g., Clostridiales, Alistipes, Parabacteroides, and Lachnospiraceae). The alteration of microbiota and metabolites significantly contributes to the variation of bile acid and lipid metabolism, resulting in a reduction in cholesterol accumulation and the loss of weight in ducks. However, more evidence is needed.
We concluded that blue light could regulate cysteine and methionine metabolism and shape the intestinal microbiota based on our experimental results. Our findings also opened up a new direction of conjecture (regarding the brain-gut axis) for exploring the mechanism by which blue light regulates lipid metabolism.
The present study applied non-targeted metabolomics and an intestinal microbiota diversity analysis to investigate the effects of exposure to blue light on duck metabolism. Blue light triggered cysteine and methionine metabolism, and improved lipid metabolism. By increasing bile acid production and bile acid hydrolase-producing bacteria, exposure to blue light reduced the accumulation of cholesterol in the host. These results highlighted the change in metabolic function and the intestinal microbiota in blue light exposure-induced weight loss. This study also provides a new experimental approach for the application of blue light in animal husbandry.
The datasets presented in this study can be found in online repositories. The names of the repository/repositories and accession number(s) can be found below: NCBI; PRJNA744139.
The animal study was reviewed and approved by Animal Care and Use of South China Agricultural University (No. 20110107-1, Guangzhou, China).
WW, LY, and JC designed the study. JC, YL, YF, and DX acquired the data and performed experiments. YZhu, HY, YF, and JQ helped conduct animal experiments. DX, XJ, GM, SW, and YZhao advised on data analysis. WW and DX wrote the manuscript. All authors read and approved the manuscript.
This study was sponsored by the National Nature Science Foundation of China (32072751), the National Key Research Program (2016YFD0500509-07), Natural Science Foundation of Guangdong Province (2019B1515210012), the China Agriculture Research System (CARS-42-15), and Modern Agricultural Industrial Technology System Innovation Team of Guangdong Province (2020KJ137).
XJ is employed by Moon (Guangzhou) Biotech Co. Ltd. SW and YF are employed by Guangdong Haida Group Co. Ltd. YZhao is employed by Gold Coin Feedmill (Dong Guan) Co. Ltd. JQ is employed by Cofco Feed (Foshan) Co. Ltd.
The remaining authors declare that the research was conducted in the absence of any commercial or financial relationships that could be construed as a potential conflict of interest.
All claims expressed in this article are solely those of the authors and do not necessarily represent those of their affiliated organizations, or those of the publisher, the editors and the reviewers. Any product that may be evaluated in this article, or claim that may be made by its manufacturer, is not guaranteed or endorsed by the publisher.
The Supplementary Material for this article can be found online at: https://www.frontiersin.org/articles/10.3389/fnut.2021.737059/full#supplementary-material
1. Raccoursier M, Thaxton YV, Christensen K, Aldridge DJ, Scanes CG. Light intensity preferences of broiler chickens: implications for welfare. Animal. (2019) 13:2857–63. doi: 10.1017/S175173111900123X
2. Olsson P, Lind O, Kelber A. Bird colour vision: behavioural thresholds reveal receptor noise. J Exp Biol. (2015) 218:184–93. doi: 10.1242/jeb.111187
3. Musser JM, Arendt D. Loss and gain of cone types in vertebrate ciliary photoreceptor evolution. Dev Biol. (2017) 431:26–35. doi: 10.1016/j.ydbio.2017.08.038
4. Scholtyßek C, Kelber A. Farbensehen der Tiere: Von farbenblinden Seehunden und tetrachromatischen Vögeln [Color vision in animals: From color blind seals to tetrachromatic vision in birds]. Ophthalmologe. (2017) 114:978–85. doi: 10.1007/s00347-017-0543-6
5. Coats JG, Maktabi B, Abou-Dahech MS, Baki G. Blue light protection, part II-Ingredients and performance testing methods. J Cosmet Dermatol. (2021) 20:718–23. doi: 10.1111/jocd.13854
6. Keshishyan ES, Zaporozhtseva ZV, Zenina OM, Zrodnikov VS. Photodynamic inactivation of bacteria in vitro under the effect of blue light. Bull Exp Biol Med. (2015) 158:475–7. doi: 10.1007/s10517-015-2788-x
7. Perlova T, Gruebele M, Chemla YR. Blue light is a universal signal for escherichia coli chemoreceptors. J Bacteriol. (2019) 201:e00762–18. doi: 10.1128/JB.00762-18
8. Darmani H, Am Smadi E, Mb Bataineh S. Blue light emitting diodes cripple Helicobacter pylori by targeting its virulence factors. Minerva Gastroenterol Dietol. (2019) 65:187–92. doi: 10.23736/S1121-421X.19.02593-5
9. Adamskaya N, Dungel P, Mittermayr R, Hartinger J, Feichtinger G, Wassermann K, et al. Light therapy by blue LED improves wound healing in an excision model in rats. Injury. (2011) 42:917–21. doi: 10.1016/j.injury.2010.03.023
10. Barbaric J, Abbott R, Posadzki P, Car M, Gunn LH, Layton AM, et al. Light therapies for acne. Cochrane Database Syst Rev. (2016) 9:CD007917. doi: 10.1002/14651858.CD007917.pub2
11. Campiche R, Curpen SJ, Lutchmanen-Kolanthan V, Gougeon S, Cherel M, Laurent G, et al. Pigmentation effects of blue light irradiation on skin and how to protect against them. Int J Cosmet Sci. (2020) 42:399–406. doi: 10.1111/ics.12637
12. Lehrl S, Gerstmeyer K, Jacob JH, Frieling H, Henkel AW, Meyrer R, et al. Blue light improves cognitive performance. J Neural Transm (Vienna). (2007) 114:457–60. doi: 10.1007/s00702-006-0621-4
13. Liebmann J, Born M, Kolb-Bachofen V. Blue-light irradiation regulates proliferation and differentiation in human skin cells. J Invest Dermatol. (2010) 130:259–69. doi: 10.1038/jid.2009.194
14. Jo HL, Jung Y, Suh BF, Cho E, Kim K, Kim E. Clinical evaluation method for blue light (456 nm) protection of skin. J Cosmet Dermatol. (2020) 19:2438–43. doi: 10.1111/jocd.13508
15. Ohara M, Kawashima Y, Kitajima S, Mitsuoka C, Watanabe H. Blue light inhibits the growth of skin tumors in the v-Ha-ras transgenic mouse. Cancer Sci. (2003) 94:205–9. doi: 10.1111/j.1349-7006.2003.tb01420.x
16. Zhang S, Zhong G, Shao D, Wang Q, Hu Y, Wu T, et al. supplementation with Bacillus subtilis promotes growth performance of broilers by altering the dominant microbial community. Poult Sci. (2021) 100:100935. doi: 10.1016/j.psj.2020.12.032
17. Hassan MR, Sultana S, Ryu KS. Effect of various monochromatic LED light colors on performance, blood properties, bone mineral density, and meat fatty acid composition of ducks. J Poult Sci. (2017) 54:66–72. doi: 10.2141/jpsa.0160012
18. Liu W, Wang Z, Chen Y. Effects of monochromatic light on developmental changes in satellite cell population of pectoral muscle in broilers during early post hatch period. Anat Rec. (2010) 293:1315–24. doi: 10.1002/ar.21174
19. Campbell CL, Colton S, Haas R, Rice M, Porter A, Schenk A, et al. Effects of different wavelengths of light on the biology, behavior, and production of grow-out Pekin ducks. Poult Sci. (2015) 94:1751–7. doi: 10.3382/ps/pev166
20. López-Jaramillo P, Gómez-Arbeláez D, López-López J, López-López C, Martínez-Ortega J, Gómez-Rodríguez A, et al. The role of leptin/adiponectin ratio in metabolic syndrome and diabetes. Horm Mol Biol Clin Investig. (2014) 18:37–45. doi: 10.1515/hmbci-2013-0053
21. Martínez Y, Li X, Liu L, Bin P, Yan W, Más D, et al. The role of methionine on metabolism, oxidative stress, and diseases. Amino Acids. (2017) 49:2091–8. doi: 10.1007/s00726-017-2494-2
22. Pekala J, Patkowska-Sokoĺa B, Bodkowski R, Jamroz D, Nowakowski P, Lochyński S, et al. L-carnitine–metabolic functions and meaning in humans life. Curr Drug Metab. (2011) 12:667–78. doi: 10.2174/138920011796504536
23. Seidel U, Huebbe P, Rimbach G. Taurine: a regulator of cellular redox homeostasis and skeletal muscle function. Mol Nutr Food Res. (2019) 63:e1800569. doi: 10.1002/mnfr.201800569
24. Wen C, Li F, Zhang L, Duan L, Guo Q, Wang W, et al. Taurine is involved in energy metabolism in muscles, adipose tissue, and the liver. Mol Nutr Food Res. (2019) 63:e1800536. doi: 10.1002/mnfr.201800536
25. Yokogoshi H, Oda H. Dietary taurine enhances cholesterol degradation and reduces serum and liver cholesterol concentrations in rats fed a high-cholesterol diet. Amino Acids. (2002) 23:433–9. doi: 10.1007/s00726-002-0211-1
26. Lam NV, Chen W, Suruga K, Nishimura N, Goda T, Yokogoshi H. Enhancing effect of taurine on CYP7A1 mRNA expression in Hep G2 cells. Amino Acids. (2006) 30:43–8. doi: 10.1007/s00726-005-0244-3
27. Zeng Q, Li D, He Y, Li Y, Yang Z, Zhao X, et al. Discrepant gut microbiota markers for the classification of obesity-related metabolic abnormalities. Sci Rep. (2019) 9:13424. doi: 10.1038/s41598-019-49462-w
28. Cao J, Liu WW. Green and blue monochromatic lights promote growth and development of broilers via stimulating testosterone secretion and myofiber growth. J Appl Poultry Res. (2008) 17:211–8. doi: 10.3382/japr.2007-00043
29. Doyle SL, Donohoe CL, Finn SP, Howard JM, Lithander FE, Reynolds JV, et al. IGF-1 and its receptor in esophageal cancer: association with adenocarcinoma and visceral obesity. Am J Gastroenterol. (2012) 107:196–204. doi: 10.1038/ajg.2011.417
30. Wang Y, Bai X, Wang Z, Cao J, Dong Y, Chen YV. Wavelengths affected myofiber development and satellite cell proliferation of chick embryos via the IGF-1 signaling pathway. Photochem Photobiol. (2017) 93:1492–501. doi: 10.1111/php.12806
31. Yin J, Li Y, Han H, Chen S, Gao J, Liu G, et al. Melatonin reprogramming of gut microbiota improves lipid dysmetabolism in high-fat diet-fed mice. J Pineal Res. (2018) 65:e12524. doi: 10.1111/jpi.12524
32. Bin P, Huang R, Zhou X. Oxidation resistance of the sulfur amino acids: methionine and cysteine. Biomed Res Int. (2017) 2017:9584932. doi: 10.1155/2017/9584932
33. Calabrese G, Morgan B, Riemer J. Mitochondrial glutathione: regulation and functions. Antioxid Redox Signal. (2017) 27:1162–77. doi: 10.1089/ars.2017.7121
34. Pompella A, Maellaro E, Benedetti A, Casini AF. Glutathione depletion, lipid peroxidation, and the antecedents of ferroptosis: what about cellular calcium? Free Radic Biol Med. (2019) 143:221–2. doi: 10.1016/j.freeradbiomed.2019.08.004
35. Bertrand RL. Iron accumulation, glutathione depletion, and lipid peroxidation must occur simultaneously during ferroptosis and are mutually amplifying events. Med Hypotheses. (2017) 101:69–74. doi: 10.1016/j.mehy.2017.02.017
36. Higuchi M, Celino FT, Shimizu-Yamaguchi S, Miura C, Miura T. Taurine plays an important role in the protection of spermatogonia from oxidative stress. Amino Acids. (2012) 43:2359–69. doi: 10.1007/s00726-012-1316-9
37. Wang Q, Fan W, Cai Y, Wu Q, Mo L, Huang Z, et al. Protective effects of taurine in traumatic brain injury via mitochondria and cerebral blood flow. Amino Acids. (2016) 48:2169–77. doi: 10.1007/s00726-016-2244-x
38. Krajcovicová-Kudlácková M, Simoncic R, Béderová A, Babinská K, Béder I. Correlation of carnitine levels to methionine and lysine intake. Physiol Res. (2000) 49:399–402.
39. Adeva-Andany MM, Calvo-Castro I, Fernández-Fernández C, Donapetry-García C, Pedre-Piñeiro AM. Significance of l-carnitine for human health. IUBMB Life. (2017) 69:578–94. doi: 10.1002/iub.1646
40. Jeukendrup AE, Randell R. Fat burners: nutrition supplements that increase fat metabolism. Obes Rev. (2011) 12:841–51. doi: 10.1111/j.1467-789X.2011.00908.x
41. Rizk YS, Fahim HN, Beshara MM, Mahrose KM, Awad AL. Response of duck breeders to dietary L-Carnitine supplementation during summer season. An Acad Bras Cienc. (2019) 91:e20180907. doi: 10.1590/0001-3765201920180907
42. Shang M, Cappellesso F, Amorim R, Serneels J, Virga F, Eelen G, et al. Macrophage-derived glutamine boosts satellite cells and muscle regeneration. Nature. (2020) 587:626–31. doi: 10.1038/s41586-020-2857-9
43. Li P, Kim SW, Nakagawa K, Zhou H, Wu G. Dietary supplementation with L-glutamine and AminoGutTM enhances protein synthesis in chicken skeletal muscle. FASEB J. (2010) 24(S1):740.21.
44. Hu Hong, 2020: Hu H, Dai S, Li J, Wen A, Bai X. Glutamine improves heat stress-induced oxidative damage in the broiler thigh muscle by activating the nuclear factor erythroid 2-related 2/Kelch-like ECH-associated protein 1 signaling pathway. Poult Sci. (2020) 99:1454–61. doi: 10.1016/j.psj.2019.11.001
45. Maachi M, Piéroni L, Bruckert E, Jardel C, Fellahi S, Hainque B, et al. Systemic low-grade inflammation is related to both circulating and adipose tissue TNFalpha, leptin and IL-6 levels in obese women. Int J Obes Relat Metab Disord. (2004) 28:993–7. doi: 10.1038/sj.ijo.0802718
46. Carrascal L, Nunez-Abades P, Ayala A, Cano M. Role of melatonin in the inflammatory process and its therapeutic potential. Curr Pharm Des. (2018) 24:1563–88. doi: 10.2174/1381612824666180426112832
47. Nicolas S, Chabry J, Guyon A, Zarif H, Heurteaux C, Petit-Paitel A. L'adiponectine - Un anti-inflammatoire et anti-dépresseur endogène? [Adiponectin: an endogenous molecule with anti-inflammatory and antidepressant properties?]. Med Sci (Paris). (2018) 34:417–23. doi: 10.1051/medsci/20183405014
48. Murakami S. The physiological and pathophysiological roles of taurine in adipose tissue in relation to obesity. Life Sci. (2017) 186:80–6. doi: 10.1016/j.lfs.2017.08.008
49. Kaneko H, Kobayashi M, Mizunoe Y, Yoshida M, Yasukawa H, Hoshino S. Taurine is an amino acid with the ability to activate autophagy in adipocytes. Amino Acids. (2018) 50:527–35. doi: 10.1007/s00726-018-2550-6
50. Guo YY, Li BY, Peng WA, Guo L, Tang QQ. Taurine-mediated browning of white adipose tissue is involved in its anti-obesity effect in mice. J Biol Chem. (2019) 294:15014–24. doi: 10.1074/jbc.RA119.009936
51. Kim KS, Jang MJ, Fang S, Yoon SG, Kim IY, Seong JK, et al. Anti-obesity effect of taurine through inhibition of adipogenesis in white fat tissue but not in brown fat tissue in a high-fat diet-induced obese mouse model. Amino Acids. (2019) 51:245–54. doi: 10.1007/s00726-018-2659-7
52. Guan L, Miao P. The effects of taurine supplementation on obesity, blood pressure and lipid profile: a meta-analysis of randomized controlled trials. Eur J Pharmacol. (2020) 885:173533. doi: 10.1016/j.ejphar.2020.173533
53. Luo J, Yang H, Song BL. Mechanisms and regulation of cholesterol homeostasis. Nat Rev Mol Cell Biol. (2020) 21:225–45. doi: 10.1038/s41580-019-0190-7
54. Staley C, Weingarden AR, Khoruts A, Sadowsky MJ. Interaction of gut microbiota with bile acid metabolism and its influence on disease states. Appl Microbiol Biotechnol. (2017) 101:47–64. doi: 10.1007/s00253-016-8006-6
55. Chávez-Talavera O, Tailleux A, Lefebvre P, Staels B. Bile acid control of metabolism and inflammation in obesity, type 2 diabetes, dyslipidemia, and nonalcoholic fatty liver disease. Gastroenterology. (2017) 152:1679–94.e3. doi: 10.1053/j.gastro.2017.01.055
56. Chiang JY. Bile acid metabolism and signaling. Compr Physiol. (2013) 3:1191–212. doi: 10.1002/cphy.c120023
57. Di Ciaula A, Garruti G, Lunardi Baccetto R, Molina-Molina E, Bonfrate L, Wang DQ, et al. Bile acid physiology. Ann Hepatol. (2017) 16(Suppl. 1):s4–14. doi: 10.5604/01.3001.0010.5493
58. Guo J, Gao Y, Cao X, Zhang J, Chen W. Cholesterol-lowing effect of taurine in HepG2 cell. Lipids Health Dis. (2017) 16:56. doi: 10.1186/s12944-017-0444-3
59. Mueller M, Thorell A, Claudel T, Jha P, Koefeler H, Lackner C, et al. Ursodeoxycholic acid exerts farnesoid X receptor-antagonistic effects on bile acid and lipid metabolism in morbid obesity. J Hepatol. (2015) 62:1398–404. doi: 10.1016/j.jhep.2014.12.034
60. Chiang JYL, Ferrell JM. Bile acid metabolism in liver pathobiology. Gene Expr. (2018) 18:71–87. doi: 10.3727/105221618X15156018385515
61. Li and Chiang 2011: Li T Chiang JY. Bile acid signaling in liver metabolism and diseases. J Lipids. (2012) 2012:754067. doi: 10.1155/2012/754067
62. Ramírez-Pérez O, Cruz-Ramón V, Chinchilla-López P, Méndez-Sánchez N. The role of the gut microbiota in bile acid metabolism. Ann Hepatol. (2017) 16(Suppl. 1):S21–6. doi: 10.5604/01.3001.0010.5672
63. Streidl T, Karkossa I, Segura Muñoz RR, Eberl C, Zaufel A, Plagge J, et al. The gut bacterium Extibacter muris produces secondary bile acids and influences liver physiology in gnotobiotic mice. Gut Microbes. (2021) 13:1–21. doi: 10.1080/19490976.2020.1854008
64. Marion S, Studer N, Desharnais L, Menin L, Escrig S, Meibom A, et al. Bernier-Latmani R. In vitro and in vivo characterization of Clostridium scindens bile acid transformations Gut Microbes. (2019) 10:481–503. doi: 10.1080/19490976.2018.1549420
65. Parker BJ, Wearsch PA, Veloo ACM, Rodriguez-Palacios A. The genus alistipes: gut bacteria with emerging implications to inflammation cancer and mental health. Front Immunol. (2020) 11:906. doi: 10.3389/fimmu.2020.00906
66. Huang S, Pang D, Li X, You L, Zhao Z, Cheung PC, et al. A sulfated polysaccharide from Gracilaria Lemaneiformis regulates cholesterol and bile acid metabolism in high-fat diet mice. Food Funct. (2019) 10:3224–36. doi: 10.1039/C9FO00263D
67. Le Roy T, Lécuyer E, Chassaing B, Rhimi M, Lhomme M, Boudebbouze S, et al. The intestinal microbiota regulates host cholesterol homeostasis. BMC Biol. (2019) 17:94. doi: 10.1186/s12915-019-0715-8
68. Wang K, Liao M, Zhou N, Bao L, Ma K, Zheng Z, et al. Parabacteroides distasonis alleviates obesity and metabolic dysfunctions via production of succinate and secondary bile acids. Cell Rep. (2019) 26:222–35.e5. doi: 10.1016/j.celrep.2018.12.028
69. Scott KP, Martin JC, Duncan SH, Flint HJ. Prebiotic stimulation of human colonic butyrate-producing bacteria and bifidobacteria, in vitro. FEMS Microbiol Ecol. (2014) 87:30–40. doi: 10.1111/1574-6941.12186
70. Barcenilla A, Pryde SE, Martin JC, Duncan SH, Stewart CS, Henderson C, et al. Phylogenetic relationships of butyrate-producing bacteria from the human gut. Appl Environ Microbiol. (2000) 66:1654–61. doi: 10.1128/AEM.66.4.1654-1661.2000
71. Hu Y, Wang L, Shao D, Wang Q, Wu Y, Han Y, et al. Selectived and reshaped early dominant microbial community in the cecum with similar proportions and better homogenization and species diversity due to organic acids as AGP alternatives mediate their effects on broilers growth. Front Microbiol. (2020) 10:2948. doi: 10.3389/fmicb.2019.02948
72. Zeng H, Larson KJ, Cheng WH, Bukowski MR, Safratowich BD, Liu Z, et al. Advanced liver steatosis accompanies an increase in hepatic inflammation, colonic, secondary bile acids and Lactobacillaceae/Lachnospiraceae bacteria in C57BL/6 mice fed a high-fat diet. J Nutr Biochem. (2020) 78:108336. doi: 10.1016/j.jnutbio.2019.108336
Keywords: blue light, methionine metabolism, intestinal microbiota, cholesterol, bile acid
Citation: Xia D, Yang L, Cui J, Li Y, Jiang X, Meca G, Wang S, Feng Y, Zhao Y, Qin J, Zhu Y, Ye H and Wang W (2021) Combined Analysis of the Effects of Exposure to Blue Light in Ducks Reveals a Reduction in Cholesterol Accumulation Through Changes in Methionine Metabolism and the Intestinal Microbiota. Front. Nutr. 8:737059. doi: 10.3389/fnut.2021.737059
Received: 06 July 2021; Accepted: 18 October 2021;
Published: 25 November 2021.
Edited by:
Shourong Shi, Poultry Institute, Chinese Academy of Agricultural Sciences (CAAS), ChinaReviewed by:
Chengbo Yang, University of Manitoba, CanadaCopyright © 2021 Xia, Yang, Cui, Li, Jiang, Meca, Wang, Feng, Zhao, Qin, Zhu, Ye and Wang. This is an open-access article distributed under the terms of the Creative Commons Attribution License (CC BY). The use, distribution or reproduction in other forums is permitted, provided the original author(s) and the copyright owner(s) are credited and that the original publication in this journal is cited, in accordance with accepted academic practice. No use, distribution or reproduction is permitted which does not comply with these terms.
*Correspondence: Wence Wang, d2FuZ3dlbmNlQHNjYXUuZWR1LmNu
†These authors share first authorship
Disclaimer: All claims expressed in this article are solely those of the authors and do not necessarily represent those of their affiliated organizations, or those of the publisher, the editors and the reviewers. Any product that may be evaluated in this article or claim that may be made by its manufacturer is not guaranteed or endorsed by the publisher.
Research integrity at Frontiers
Learn more about the work of our research integrity team to safeguard the quality of each article we publish.