- Department of Environmental and Molecular Toxicology, Linus Pauling Institute, Oregon State University, Corvallis, OR, United States
Hydrolysis of glucobrassicin by plant or bacterial myrosinase produces multiple indoles predominantly indole-3-carbinol (I3C). I3C and its major in vivo product, 3,3'-diindolylmethane (DIM), are effective cancer chemopreventive agents in pre-clinical models and show promise in clinical trials. The pharmacokinetics/pharmacodynamics of DIM have been studied in both rodents and humans and urinary DIM is a proposed biomarker of dietary intake of cruciferous vegetables. Recent clinical studies at Oregon State University show surprisingly robust metabolism of DIM in vivo with mono- and di-hydroxylation followed by conjugation with sulfate or glucuronic acid. DIM has multiple mechanisms of action, the most well-characterized is modulation of aryl hydrocarbon receptor (AHR) signaling. In rainbow trout dose-dependent cancer chemoprevention by dietary I3C is achieved when given prior to or concurrent with aflatoxin B1, polycyclic aromatic hydrocarbons, nitrosamines or direct acting carcinogens such as N-methyl-N'-nitro-nitrosoguanidine. Feeding pregnant mice I3C inhibits transplacental carcinogenesis. In humans much of the focus has been on chemoprevention of breast and prostate cancer. Alteration of cytochrome P450-dependent estrogen metabolism is hypothesized to be an important driver of DIM-dependent breast cancer prevention. The few studies done to date comparing glucobrassicin-rich crucifers such as Brussels sprouts with I3C/DIM supplements have shown the greater impact of the latter is due to dose. Daily ingestion of kg quantities of Brussels sprouts is required to produce in vivo levels of DIM achievable by supplementation. In clinical trials these supplement doses have elicited few if any adverse effects. Sulforaphane from glucoraphanin can act synergistically with glucobrassicin-derived DIM and this may lead to opportunities for combinatorial approaches (supplement and food-based) in the clinic.
Introduction
Glucosinolates are found primarily in cruciferous vegetables (1–3). This review will focus primarily on the hydrolysis product of glucobrassicin, indole-3-carbinol (I3C), and the major in vivo I3C product, 3,3'-diindolylmethane (DIM). Glucobrassicin (Figure 1) is one of dozens of glucosinolates and is especially rich in the crucifer family. Brussels sprouts contain 1.6–2.7 mg/g dry weight glucobrassicin (4). Myrosinase (β-thioglucoside glucohydrolase, EC 3.2.3.1), present in plant cells (and in some gut bacteria), hydrolyzes glucobrassicin to the unstable indole-3-methylisothiocyanate which spontaneously decomposes yielding primarily indole-3-acetonitrile and I3C (Figure 1). On a molar basis the yield of I3C from glucobrassicin is about 20% corresponding to 0.11–0.18 mg/g dry weight for Brussels sprouts.
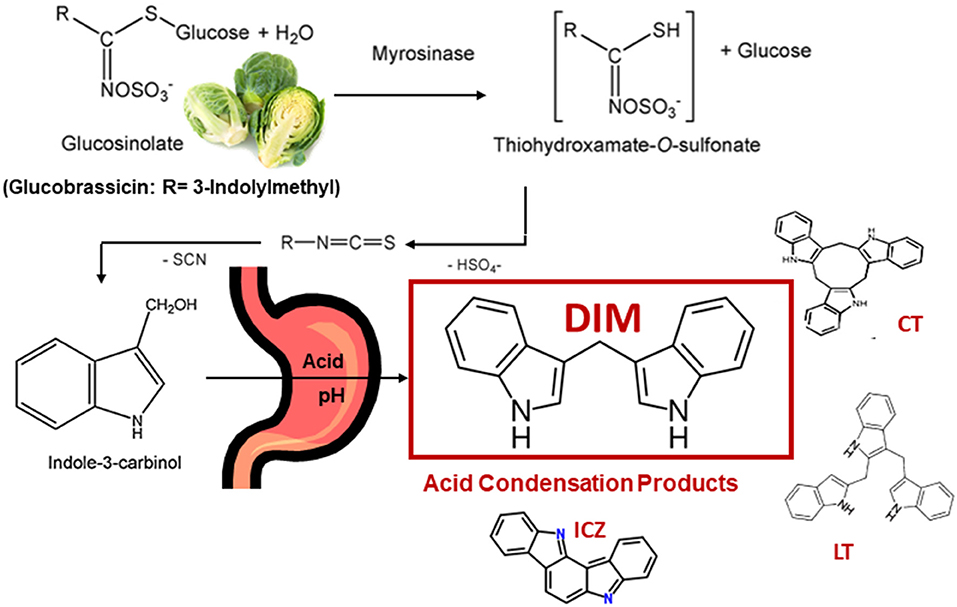
Figure 1. Cleavage of Glucobrassicin in Cruciferous Vegetable by Myrosinase, Formation of Indole-3-Carbinol (I3C) and Examples of Acid Condensation Products Produced in vivo. 3,3'-Diindolylmethane (DIM) is the Major Product. Cyclic CT) and Linear Trimers (LT) as well as Indolo[3,2-b]carbazole (ICZ) are Present in Trace Amounts.
Acid Condensation Products: Should I3C Be Considered a “Pronutraceutical”?
I3C is subject to decomposition especially under acidic conditions (5). In the stomach, I3C undergoes extensive condensation to yield a mixture of DIM as well as linear and cyclic trimers and tetramers (2, 3, 5, 6) (Figure 1). DIM predominates as the major product under acidic conditions both in vitro and in vivo (5, 6). The pharmacological activity of I3C is markedly reduced or eliminated if exposure bypasses the stomach strongly suggesting beneficial properties of I3C, such as cancer chemoprevention, are not due to I3C itself but rather component(s) of the acid condensation mixture (6). Unfortunately, with the exceptions of one linear trimer [LT, 2-(indol-3-ylmethyl)-3,3'-diindolylmethane], one cyclic trimer [CT, 5,6,11,12,17,18-hexahydrocyclononal (1,2-b:4,5-b':7,8-b”) triindole] and indolo[3,2-b]carbazole (ICZ), the pharmacological activities of individual acid condensation mixture products have not been described to date. The focus of the rest of this review will be on the efficacy and mechanism(s) of action for I3C and DIM in vitro and in vivo as cancer chemoprevention agents. A number of excellent reviews on I3C/DIM in cancer chemoprevention, as well as potential therapeutic agents for other disease, can be found in the literature and those cited here are not a complete list (7–18). A comprehensive set of abstracts on DIM research can be found at the DIM Information Resource Center (www.diindolylmethane.org/references.htm).
In vitro Studies
There have been numerous studies employing cancer cell lines of I3C and DIM potency and efficacy as well as mechanism(s) of action as anticarcinogens. Given that I3C spontaneously dimerizes to DIM even at neutral pH (19), there is the question of physiological relevance in studying I3C in cell-based systems and this is reinforced by the observation that I3C is rarely, if ever, detected in blood after oral ingestion. A reasonable presumption is that a portion, if not the majority, of the cellular effects reported following incubation with I3C, especially with long incubations at concentrations typically ≥100 μM, are due to DIM rather than I3C. The relevance of many in vitro studies of DIM (typically at concentrations of 10–100 μM) in cancer cells could also be questioned as plasma levels following ingestion of supplements have been reported to be ≤ 1 μM and recent evidence in humans show that a significant portion exists as DIM metabolites with unknown pharmacological activity (20).
I3C/DIM impacts cancer cells in numerous ways including inhibition of proliferation, stimulation of apoptosis and alteration of cell cycle control (9, 16–33). DIM is a weak agonist of the aryl hydrocarbon receptor (AHR) with Kd of 90 nM (34). ICZ, by comparison, is a strong agonist with a Kd of 0.19 nM (5). However, the concentrations of ICZ achievable in vivo are much lower than with DIM ( ≤ 1 μM). One cancer cell line that has been extensively studied with DIM is the MCF-7 human breast cancer cell line as, in addition to AHR, DIM impacts estrogen receptor (ER)-dependent signaling in these ER-regulated cells (35–45). Riby et al., demonstrated that the CT acid condensation product is an ER agonist (EC50 = 100 nM in MCF-7 cells (39). As AHR and ER exhibit “cross-talk” through a number of mechanisms, with a resultant impact on breast cancer cells (45–47), it is not surprising that human trials have examined chemoprevention of breast cancer (7, 8, 48). DIM is an antagonist of the androgen receptor which has also led to a focus on prostate as a target for I3C/DIM chemoprevention (45, 49). One target gene for DIM-dependent AHR activation is cytochrome P450 (CYP) 1B1 which is effective in estrogen hydroxylation at the 2- and 4-positions (50). The net result of DIM-dependent AHR modification of E2 metabolism is a decrease in the ratio of 16α/2-hydroxy-E2 and concomitant decrease in E2 levels (51). 16α-Hydroxy-E2 retains estrogenic activity and has been referred to as the “bad” E2 metabolite whereas 2-hydroxy-E2 has been termed the “good” E2 metabolite. The ratio of 16α/2-hydroxy-E2 and total E2 levels are often used as biomarkers for DIM in chemoprevention studies of estrogen-driven cancers such as breast (51, 52).
The large number of targets demonstrated for I3C and DIM in chemoprevention are likely due, in part, to epigenetic mechanisms in control of gene expression in addition to ligand binding to transcription factors such as AHR, ER, AR, Sp1, Nrf-2 (both directly and via AHR) and NFκB (33, 53, 54). Modulation of the expression and/or activities of components of epigenetics e.g., DNA methyl transferases (DNMTs), histone deacetylases (HDACs), and non-coding RNAs (miRNAs and lncRNAs) may be dependent or independent of I3C/DIM ligand transcription factor activation. Increased DNA methylation often silences tumor suppressor genes and amelioration of this silencing is thought to be an important mechanism in cancer chemoprevention. DIM was effective in down-regulation of Dnmt/DNMT in mouse and human prostate cancer cells (55, 56) and repressing DNMT in normal prostate (PrEC) as well as androgen-dependent (LnCAP) or androgen-independent (PC3) prostate cancer cells (56). DIM-dependent inhibition of DNMT tended to down-regulate expression of oncogenes while enhancing tumor suppressor genes (55). In mouse TRAMP C1 prostate cancer cells, DIM reduced expression of both mRNA and protein for Dnmt1, Dnmt3a and Dnmt3b (55).
DIM was found to selectively down-regulate protein levels of class I HDACs (HDAC1, HDAC2, HDAC3 and HDAC8) in human colon cancer and prostate cancer cell lines and the mechanism is enhanced proteosomal HDAC degradation (57, 58). Most HDAC inhibitors [e.g., suberoylanilide hydroxamic acid (SAHA) and trichostatin] are competitive enzyme activity inhibitors and this novel mechanism exhibited by DIM could be used in the clinic with HDAC enzyme inhibitors to more effectively reduce histone acetylation and/or lower the concentrations of HDAC inhibitors required. As with other HDAC inhibitors, DIM could enhance AHR-mediated gene transcription by a mechanism other than ligand binding. In both mouse colonocytes and human Caco-2 cells, HDAC inhibitors including butyrate, Panobinostat and Vorinostat (SAHA) synergistically enhanced ligand- (including indole) dependent AHR induction of CYP1A1 and other AHR target genes (59). HDAC and DNMT together contribute to the balance of permissive (H3K4me3) or repressive (H3K27me3) chromatin marks (60) and the bivalent nature of these marks is impacted in vitro by DIM.
I3C and DIM modulate expression of a number of miRNAs and lncRNAs (53, 54). The miRNAs regulated by DIM include let-7a-e, miRNA-15a, miRNA-16, miRNA17-5p, miRNA-19a, miRNA-20a, miR-21, miR-27b, miR-30e, miR-31, miR-34a, miRNA-92a, miRNA-106a, miR-124, miR-146, miRNA-181a, miRNA-181b, miRNA-210, miR-219, miRNA-221, miR-320, miR-490, miR-495 and miR-1192 (11, 53, 60–63). Not surprisingly, miRNAs known to be tumor suppressors tend to be up-regulated while oncomirs are down-regulated (64). A number of the target genes are important in regulation of the cell cycle and apoptosis. LncRNAs regulated by DIM include PCGEM1, HOTAIR and CCAT-L (65–67). This induction/repression of miRNAs and LncRNAs may be due, in part, to DIM-dependent binding to transcription factors such as AHR or AR. The majority of studies above were performed in cell culture and the importance of I3C/DIM regulation of non-coding RNA expression in chemoprevention or therapeutic intervention in human cancer is currently unknown.
In vivo Studies: Preclinical Models
There are excellent reviews on I3C/DIM as chemopreventive agents in preclinical models of cancer (9, 10, 12). In addition to liver, mammary and colon, dietary I3C reduces lung carcinogenesis by the tobacco-specific nitrosamine, 4-(methylnitrosamino)-1-(3-pyridyl)-1-butanone (NNK) and the PAH, benzo[a]pyrene (BaP) (68).
Cancer Prevention in Rainbow Trout
The rainbow trout cancer model was developed at Oregon State University through pioneering studies by Dr. Russell Sinnhuber and others in the late 1960s-early 1970s (69). Later the model was employed in chemoprevention studies in efforts led by Dr. George S. Bailey (70). There are numerous advantages with this animal model including a low spontaneous background incidence in liver (historically 0.1%), and a high sensitivity to known human carcinogens including aflatoxins, particularly aflatoxin B1 (AFB1, a known human liver carcinogen), polycyclic aromatic hydrocarbons (PAHs), nitrosamines and direct acting carcinogens such as N-methyl-N'-nitro-nitrosoguanidine (MNNG) (71, 72). Additionally, the low per diem costs for raising trout to 10–12 months of age compared to mice allowed for design of studies employing numbers of animals not achievable in rodent models. Examples include the ED001 studies with over 40,000 animals that established the dose-response relationship for dibenzo [def,p]chrysene (DBC) to 1 cancer in 5,000 animals and showed a significant deviation from the linear extrapolation model used by regulatory agencies (the calculated dietary dose of DBC resulting in 1 cancer in 106 was 1000-fold higher than the LED10 estimate) (73). Interestingly, a subsequent study with dietary AFB1 showed a linear dose-response to 1 cancer in 1000 animals although the slope was >1 (1.31) and, again, the dose producing a 1 in 106 cancer estimate was higher (17-fold) than would have been predicted by the LED10 (74).
The trout has been used extensively in dietary cancer chemoprevention and promotion studies. Again, the properties of the model could be exploited to address questions that would be difficult in rodent models. When fed prior to and during carcinogen exposure, I3C was an effective chemopreventive agent against a number of carcinogens (70–72). Surprisingly, long-term post-initiation feeding of I3C promoted hepatocarcinogenesis in this model (75–77). Chemoprevention was via the classic “blocking” mechanism resulting from I3C modulation of metabolism. In the case of AFB1, induction of trout hepatic CYP1A1 increased detoxication via induced hydroxylation at the 4 position to AFM1 and scavenging of the ultimate carcinogenic metabolite, AFB1-8,9-exo-epoxide (78, 79). I3C administration to rats showed markedly reduced hepatic AFB1-DNA adduction associated with induction of both CYP1A1 production of AFM1 and GSTa-associated conjugation of AFB1-8,9-exo-epoxide (80, 81). Subsequent studies documented that DIM promoted hepatocarcinogenesis in trout via functioning as a strong phytoestrogen (82). Utilizing a custom microarray the correlation (r = 0.87) between DIM and E2 in modulation of hepatic gene expression in trout was highly significant (p < 0.0001) (83).
Cancer Prevention in Rodents
Xenotransplant studies utilize immune-deficient mice, such as the nude or NOD mouse. These mice are implanted with a human cancer cell line and then administered I3C or DIM by diet to examine the inhibition of tumor growth over time (25, 84, 85). Advantages of this model include the use of human cancer cells and ability to easily measure the rate of growth of these surface (often implanted in the flank) tumors. An obvious disadvantage is the absorption, distribution, metabolism and excretion of DIM could exhibit species differences and the implanted tumor cells are growing in a milieu distinct from the actual human cancer. We examined human T-ALL cells in vitro for sensitivity to I3C or DIM inhibition of proliferation and viability (85). Concentrations of I3C required to inhibit proliferation or viability of human T-ALL cells (CCRF-CEM cells) were 8-fold higher than DIM (Table 1). Following implantation into SCID (NOD.CB17-Prkdcscid/SzJ) mice the efficacy of dietary DIM and I3C in inhibition of tumor growth was assessed. The tumor volume and doubling time of human CCRF-CEM T-ALL cell xenografts in these mice were both significantly impacted by 100 ppm dietary DIM. Dietary I3C (500 and 2,000 ppm) had a lower inhibitory effect on the growth of the T-ALL xenotransplants (Table 1) (85). The IC50 measurements of proliferation and viability were assessed following 24–48 h incubations during which time a significant amount of dimerization of I3C could have accorded.
As is the case with trout, long-term post-initiation administration of I3C to rats promoted hepatocarcinogenesis (86, 87). In contrast, in the infant mouse model, long-term feeding of I3C significantly (p < 0.0005) reduced liver tumors induced by diethylnitrosamine (88). A multi-organ, multi-carcinogen rat model found that prevention vs. promotion was carcinogen- and target tissue-dependent (89). In models of colon cancer, I3C and DIM are chemopreventive in an AHR-dependent fashion which also is responsive to microbial production of indole AHR ligands from dietary tryptophan metabolism (90, 91).
Our laboratory developed a transplacental mouse model of cancer chemoprevention. C57BL/6J (Ahrb/b) mice were bred with 129 (Ahrd/d) mice and dams dosed with the potent PAH, DBC (30-times more potent that BaP), 2–3 days prior to parturition. Offspring that were exposed in utero (and to some degree during lactation) developed an aggressive T-cell acute lymphoblastic leukemia (T-ALL) which began at 3 months of age (92–94). All of the surviving offspring exhibited multiple lung tumors at 10 months of age. The severity of the response was a function of both the maternal and offspring phenotype (i.e., Ahrb allele is “responsive” and Ahrd “non-responsive”) (92) and was absent in Cyp1b1 null offspring (93). Administration of phytochemicals known to be chemopreventive in adult models to the maternal diet (gestation day 9 to weaning), including I3C (95, 96), green tea (97) and chlorophyllin (98) provided protection for the offspring from both the young adult onset T-ALL mortality and the mid-life lung cancer. I3C supplementation (2,000 ppm) of the maternal diet (offspring fed control diet throughout lifetime) was the most efficacious and the response was independent of Ahr genotype (95, 96). Neither Brussels sprouts nor broccoli sprouts (10% in AIN93G diet) were protective when added to maternal diet and the same was observed for sulforaphane (96). The lack of response with the whole food is likely related to the small amount of I3C achievable in such diets (e.g., it would require consumption of an estimated 10–60 Kg daily of Brussels sprouts to achieve an I3C level of 1,000 ppm in the diet) (96). Administration of dietary I3C to pregnant rats up-regulated CYP1A1 and CYP1B1 expression in neonatal liver (99). In examination of chemoprevention of breast cancer in rats induced by 7,12-dimethylbenzanthracene (DMBA) or the direct-acting carcinogen, N-methylnitrosourea (MNU), I3C was effective whereas DIM was not (100). These results caution against attributing the chemoprevention of I3C in every model to DIM formation. Protection against DBC-dependent T-ALL in female, but not male, offspring born to mothers fed I3C during gestation required expression of ERβ (101). Such results would be consistent with I3C and DIM ER-dependent chemoprevention in breast (7, 8, 37–44).
Cancer Prevention in Clinical Trials
Epidemiological studies show an inverse correlation between cruciferous vegetable intake and some cancers (1, 12, 14, 15, 102). Both I3C and DIM have been studied in human clinical trials, primarily to test efficacy against breast and prostate cancer (Table 2) (8, 11, 48, 52, 53, 108–110). The focus on breast cancer in women derives from the demonstrated capacity for I3C or DIM to CYP1-dependent estrogen metabolism. 2-Hydroxyestrogen exhibits reduced pharmacological activity whereas 16α-hydroxyestrogen retains estrogenic activity. The ratio of 2-hydroxy/16α-hydroxy estrogen has become a biomarker for risk of estrogen-dependent cancer and cervical intraepithelial neoplasia (CIN). Clinical trials with both I3C and DIM result in an increase in this ratio and, in the case of CIN, demonstrably improved clinical outcomes. There is little evidence to suggest that supplementation for months represents a significant risk from women. One study (48) in Table 2 highlights a potential concern regarding DIM alteration in metabolism of co-administered pharmaceutics (tamoxifen) not surprising given that DIM inhibits, as well as induces, a number of CYPs. DIM inhibits human CYP3A4, responsible for metabolism of 60% of prescribed drugs, with an IC50 of 14.5 μM (Table 3). The concern regarding DIM and adverse drug responses is discussed further in the section titled Potential Risks of Long-Term I3C/DIM Supplementation: Inhibition of CYP Activity and Levels of Flavin-Containing Monooxygenase: A Potential ‘Drug-Drug' Interaction? Studies to date on DIM supplementation in treatment of prostate cancer progression also suggests the potential for some benefit in slowing progression. Following DIM supplementation in men scheduled for prostatectomy, not only were androgen receptor levels in prostate reduced but there was exclusion of the receptor from the nucleus (109) (Table 2). With one exception, the clinical trials in Table 2 examine the cancer therapeutic potential of DIM and not strictly chemoprevention. Double-blind, placebo-controlled studies in disease-free subjects are needed to better determine the potency and efficacy of DIM as a chemopreventive supplement. One important finding from these studies, primarily in humans with existing disease, is that supplementation with DIM at 200–400 mg/day is not likely to represent a risk (again, with the caveat that co-administration of some drugs could cause an adverse effect).
Pharmacokinetics of DIM in Rodents and Humans
The pharmacological mechanism(s) of action of DIM (and to a large extent I3C) has been attributed to DIM and the impact of the pharmacodynamics of I3C/DIM has largely been ignored. As discussed above, DIM is the major, if not sole, I3C product detected in vivo after oral administration and urinary levels of DIM have been proposed as a biomarker for glucobrassicin consumption (114, 115). Pharmacokinetics of I3C following administration to humans has been done by analysis of plasma and urinary DIM levels (103, 107). Incubations of DIM with liver microsomes from female rats fed diets containing β-naphthoflavone (BNF), phenobarbital (PB) or I3C, produced two unidentified mono-hydroxylated metabolites (112). Human MCF-7 breast cancer cells incubated with 1 μM [3H]-DIM for 24–72 h yielding three mono-hydroxylated CYP products, 3-[(1H-indole-3-yl) methyl]indolin-2-one (2-oxo-DIM, a tautomer with 2-hydroxy-DIM), bis (1H-indol-3-yl)methanol (3-methylenehydroxy-DIM) and 3-hydroxy-DIM along with two di-hydroxylated metabolites (3-methylenehydroxy-2-ox-DIM and 3-hydroxy-2-ox-DIM) and their sulfate conjugates (43). Co-incubation with isoform-selective CYP inhibitors suggested that CYP1A2 played the primary role in metabolism of DIM in MCF-7 cells. Surprisingly, in vivo pharmacokinetic studies in both mice, rats and humans have failed to report the formation of any phase 1 or phase 2 metabolite in plasma or urine (52, 103, 106, 108, 109, 116–119). In a recently published study with humans taking BioResponse DIM®, a formulation with clinically demonstrated enhanced absorption, we found extensive and rapid appearance of metabolites in both plasma (Figure 2) and urine (Figure 3) (20). The profile of these metabolites was very similar to what Staub et al., (43) observed in vitro with MCF-7 cells (43). Unlike their study, the major site of hydroxylation in our in vivo study was not the 2-position but rather the 3-methylene position of DIM. Both of these mono-hydroxylated DIM metabolites were rapidly conjugated and sulfation seem to predominant (as in MCF-7 cells) although glucuronides were also present (Figure 2) (20). No di-sulfate, di-glucuronide or sulfate-glucuronide conjugates were found in plasma or in urine. As with the in vitro study with MCF-7 cells, we found free and conjugated di-hydroxylated-DIM. We did not report finding 3-hydroxy-DIM either free or conjugated. Interestingly, 3-methylenehydroxy-DIM was not stable and spontaneously rearranged to a putative pyrano metabolite, 5a,6a,11b-tetrahydro-5-H-pyrano [,2,3-b:6,5-b'] diindole (pyrano-DIM). As has been observed in previous pharmacokinetic studies with I3C or DIM in humans (52, 103, 106, 108, 109, 116–119), there was significant inter-individual variability (Figure 2).
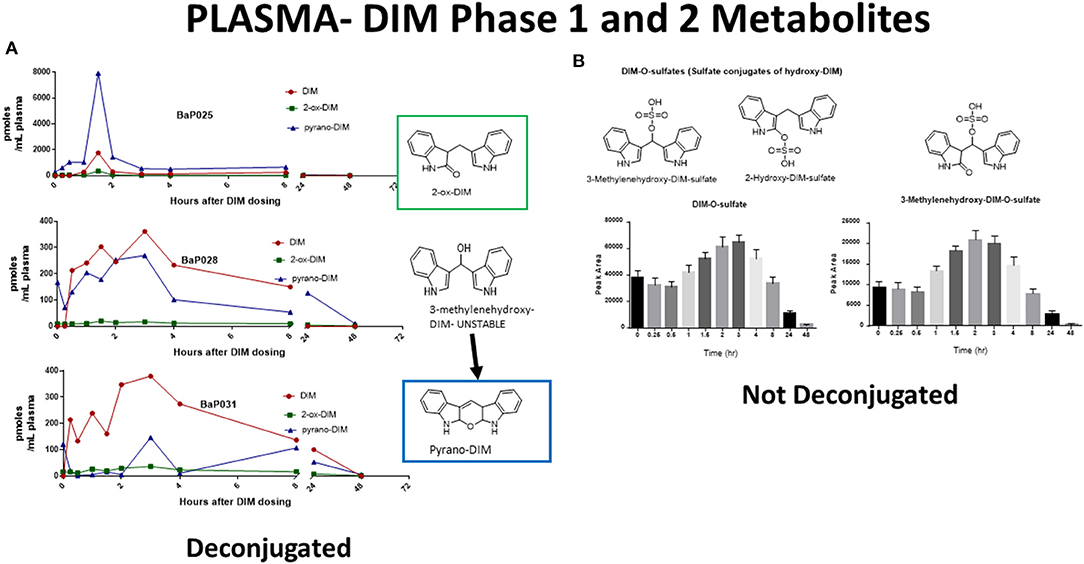
Figure 2. DIM Metabolites in Plasma Over 48 h. (A) Levels of DIM and mono-hydroxylated metabolites following deconjugation. Shown are individuals with extensive (BaP025), intermediate (BaP028) and slow (BaP031) metabolism. 3-Methylenehydroxy-DIM is unstable and is quantitated as pyrano-DIM. (B) Mean and S.D. of sulfate conjugates in plasma over 48 hr. Glucuronides were also present but are not shown. See Maier et al., (20) for complete details.
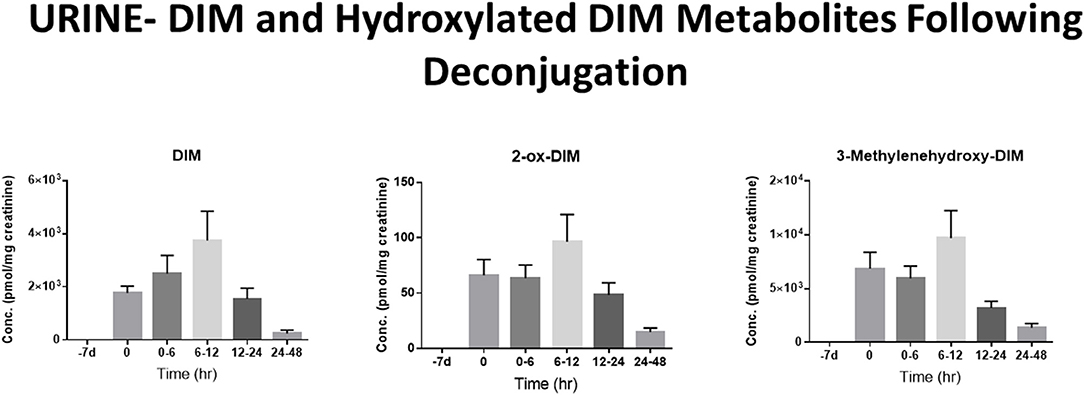
Figure 3. DIM Metabolites in Urine Over 48 h. Levels of DIM and mono-hydroxylated metabolites following deconjugation with β-glucuronidase and sulfatase. DIM and 2-ox-DIM were quantitated with standard curves and are reported in pmol/mg creatinine. 3-Methylenehydroxy-DIM is unstable and is quantitated as pyrano-DIM assuming instrument response similar to 2-ox-DIM. See Maier et al., (20) for complete details.
In MCF-7 cells the major mono-hydroxylated metabolite of DIM, 2-ox-DIM, failed to demonstrate any estrogenic activity. When we examined this same metabolite in an AHR reporter system we found it to be a more potent agonist than parent DIM and as potent or more so than known indole AHR agonists derived from microbial or host metabolism of tryptophan (i.e., indole-3-acetonitrile, kynurenine, indole, indole-3-aldehyde and indole-3-acetate) (20, 120). There is a possibility that metabolites of DIM may contribute to its beneficial chemopreventive properties. The extensive metabolism of DIM also highlights the potential for DIM-drug adverse interactions via competitive inhibition with CYPs, SULTs or UGTs. The high inter-individual variability in pharmacokinetics may reflect differences in genetic- and environmental-dependent levels of the CYPs, SULTs and UGT isoforms important in DIM metabolism.
Additional Health Benefits: I3C and DIM in Prevention or Therapy of Disease other than Cancer
I3C and DIM impact many cellular pathways [cell cycle, epithelial mesenchymal transition, cell proliferation, apoptosis, autophagy, oxidative stress, inflammation, metastasis (migration, adhesion and invasion), angiogenesis, multiple drug resistance, endoplasmic reticulum stress, and DNA repair]. (10, 16–18, 28, 29, 31, 32). Thus, it is not surprising to find proposed applications in the treatment or prevention of other toxicities, diseases or cancers (other than breast, ovarian, prostate, lung, liver and colon) including neurotoxicity, ionizing radiation, thyroid disease, endometriosis, Epstein-Barr viral Burkitt's lymphoma, papilloma viral-dependent cancers, cervical dysplasia/cancer and metabolic syndrome (107, 121–128). Descriptions of clinical trials for DIM and systemic lupus (129), childhood laryngeal papilloma (130), thyroid disease (131) and recurrent respiratory papillomatosis (132) can be found at www.ClinicalTrials.gov.
In recent years indoles, other than I3C and DIM, derived from microbial (e.g., indole, indole-3-acetate, indole-3-aldehyde, tryptamine) or host (e.g., kynurenine, kynurenic acid, xanthurenic) metabolism of dietary tryptophan were shown to be AHR ligands (120, 133). AHR activation by these indoles in the intestine protects against inflammatory-related chronic disease such as Crohn's disease, irritable bowel syndrome, metabolic syndrome and obesity (134–137). Absence of intestinal AHR activity results in dysbiosis of the gut microbiome and a compromised epithelial barrier with increased intestinal inflammation, enhanced “leakage” with susceptibility to pathogenic bacteria (e.g., Citrobacter rodentium) and colon cancer (138). Addition of dietary AHR ligands such as DIM ameliorate dysbiosis, inflammation, compromised barrier function and colon cancer from deprivation of bacteria-derived indoles (90, 91). Cruciferous vegetables or DIM intake alters the gut microbiome and the relationship is truly bidirectional (139, 140). I3C ameliorated murine colitis and DIM reversed dysbiosis in a murine model of colorectal cancer (91). These observations provide evidence for I3C/DIM promotion of intestinal health.
Potential Benefits of Combination With Whole Food and/or Other Phytochemicals: Example of Synergism With Sulforaphane
In a comprehensive review in this issue, Bouranis et al., detail the formation of sulforaphane (SFN) from the glucosinolate, glucoraphanin, also present in crucifers. As is the case with I3C and DIM, SFN has been demonstrated to be an effective cancer chemopreventive agent in preclinical models and is the focus of a number of clinical trials. I3C and DIM have been shown to have the potential to act synergistically with SFN in Nrf-2 signaling in a human cancer cell line (HepG2-C8) (141) and inhibition of cell proliferation in human colon cancer cells (40–16) (30). These observations open up new strategies for the design of human clinical trials and also cautions against reliance solely on a reductionist approach (a single phytochemical) when studying the human health benefit of foods.
Potential Risks of Long-term I3C/DIM Supplementation: Inhibition of CYP Activity and Levels of Flavin-containing Monooxygenase: A Potential “Drug-Drug” Interaction?
As discussed above I3C and DIM function as AHR agonists and induce CYPs in the 1 family as well as phase 2 conjugating enzymes [glutathione-S-transferases (GSTs), UDP-glucuronosyltransferases (UGTs) and sulfotransferase (SULTs)], a major contributor to the “blocking” mechanism of chemoprevention. I3C/DIM activation of Nrf-2-dependent signaling, either directly or via AHR upregulation of Nrf-2, induces a number of phase 2 enzymes that contribute to blocking by enhancing detoxication and excretion through conjugation. In addition to induction of CYPs, DIM has been demonstrated in vitro to be an effective inhibitor of the catalytic activity of a number of CYPs with Kis in the low μM range (Table 3) (111, 112). The contribution of DIM-dependent inhibition of carcinogen metabolism in cancer chemoprevention is not known. However, this observation could raise potential concerns about potential adverse drug interactions with long-term use.
CYPs are not the only phase 1 enzymes impacted by I3C/DIM. The flavin-containing monooxygenase, like CYP, is a superfamily of monooxygenases present in the endoplasmic reticulum of tissues such as liver, lung, intestine and kidney, and utilizes the reducing power of NADPH to insert one atom of O2 into a substrate and the other into formation of H2O (142). Each family is comprised of a single enzyme and in humans there are 5 expressed FMOs (FMO1, FMO2, FMO3, FMO4 and FMO5) (143). In mammals, with the exception of primates, FMO1 is the major FMO in liver and metabolizes a wide range of xenobiotics (143). Humans express FMO1 in liver prior to parturition after which time it is replaced with FMO3 (144). FMO1 in rat (but not guinea pig, mouse or rabbit) and FMO3 in humans are inhibited by dietary I3C/DIM (145–149). Human FMO3 is responsible for N-oxygenation of the noxious odorant trimethylamine (TMA) to the odorless trimethylamine-N-oxide (TMAO) (150). Genetic variants of human FMO3, resulting in reduced conversion of TMA to TMAO, are associated with the genetic disease trimethylaminuria and individuals with the disease exhibit severe body odor problems (as well as associated psycho-social issues) due to elevated levels of TMA in urine and sweat (150). Feeding Brussels sprouts to humans resulted in a marked in vivo increase in the ratio of TMA/TMAO and the mechanism was shown in vitro to be I3C, DIM and LT inhibition of FMO3 catalytic activity (149). Thus, trimethylaminuria patients could see worsening symptoms if ingesting significant amounts of Brussels sprouts or taking I3C or DIM supplements. On the contrary, evidence has accumulated that FMO3-dependent formation of TMAO is associated with increased risk of cardiovascular disease (151) in which case individuals at risk (which greatly outnumber trimethylaminuria patients) could benefit from diets high in crucifers or I3C/DIM supplements. This may be the rationale behind a clinical trial, “Targeting FMO-Mediated TMAO Formation in Kidney Disease (TMAO) Study (TMAO)” (NCT03152097) (152). Induction of CYPs, with concurrent down-regulation of FMO by dietary indoles, may lead to alterations in the profile of metabolites from drugs/xenobiotics that are substrates for both monooxygenases and could represent an adverse “drug-drug” interaction (Table 4) (145). N,N-dimethylaniline (DMA), (S)-nicotine (NIC) and tamoxifen (TAM) are all tertiary amines which tend to be N-dealkylated by CYPs whereas FMOs catalyze formation of the N-oxide. Feeding I3C or DIM to rats for 4 weeks produced a dose-dependent reduction in liver microsomal FMO1 protein (Table 4). The ratio of FMO/CYP-mediated metabolism of DMA was reduced in a dose-dependent fashion by dietary I3C from 1.1 to 0.22 and 0.07 at the low and high dose, respectively. DIM lowered the ratio of FMO/CYP DMA metabolism to 0.14 and 0.02 at the low and high dose, respectively. We and others had previously documented the role of FMO in metabolism of NIC (154, 155). Again, the FMO product is the N-oxide whereas, CYP N-demethylates or produces the Δ (1, 5)-iminium ion of NIC. The rate of CYP metabolism of NIC did not change with diet but N-oxygenation was markedly inhibited and no N-oxide could be detected with liver microsomes from rats fed 2,500 ppm I3C or 1,000 ppm DIM (Table 4). TAM (an ER antagonist) is used in the chemoprevention or treatment of ER-dependent breast cancer but use is limited by ovarian toxicity, primarily due to 4-hydroxylation (156). As with NIC, formation of TAM-N-oxide is regarded as detoxication and inhibition of the metabolism to the N-oxide is likely to enhance toxicity of both NIC and TAM (157).
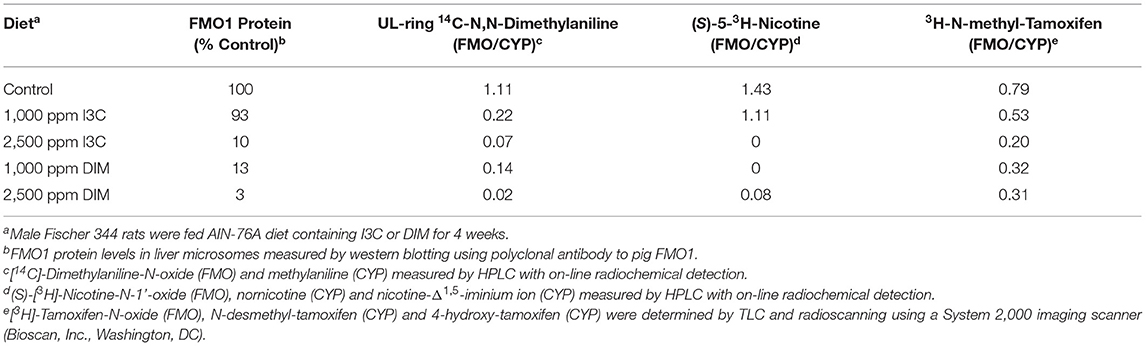
Table 4. Dietary I3C and DIM alteration of FMO1 in rat liver microsomes and FMO/CYP-mediated metabolism of N,N-dimethylaniline, nicotine and tamoxifen (145, 153).
Conclusions
I3C and DIM, the major indoles released upon hydrolysis and ingestion of glucobrassicin from Brussels sprouts and other cruciferous vegetables, have been studied extensively and their beneficial impact on cancer (and other diseases) is well-documented. The doses employed in preclinical and clinical models are not realistically achievable by ingestion of the whole food so supplementation is necessary to achieve the benefits attributed to I3C and DIM. Ingestion of I3C results in 20–40% conversion to DIM but dozens of other acid condensation products are also produced, the pharmacological properties of which are largely unknown, so at present supplementation with DIM would seem preferable. DIM is chemopreventive against cancer via a variety of mechanisms. AHR, ER and AR binding (with resultant agonism or antagonism) are associated with mechanisms ascribed to DIM. There are however unresolved questions related to the use of DIM in prevention or treatment of disease. DIM has been shown to act in both AHR-dependent up-regulation of drug/carcinogen metabolizing enzymes (e.g., CYPs, GSTs, UGTs, SULTs) and in vitro inhibition of catalytic activity (CYPs). What is the net effect in vivo? Does DIM supplementation pose any concerns for an adverse “drug-drug” type interaction and, if so, who would be at risk? Based on results from clinical trials, long-term supplementation with 300 mg daily would not produce adverse effects. One caveat is that these trials typically employed subjects with disease or with exclusion criteria that would prevent elucidation of adverse drug interactions. Another variable of potential concern is the bidirectional interaction between DIM and an individual's microbiome. The DIM-intestinal AHR-microbiome axis is an important component for future development of a “personalized nutraceutical” (concept similar to personalized medicine) approach to achieving optimal health.
It is recognized that other components in crucifers, such as sulforaphane derived from hydrolysis of glucoraphanin, contribute to the health benefits from crucifers and these vegetables should be an important component of the diet. Future research efforts should focus on the potential for inhibition, additivity or synergy from combinations of whole foods or other phytochemical nutraceuticals given with I3C/DIM in cancer chemoprevention.
Many of the mechanisms of action attributed to I3C and DIM have been elucidated from in vitro cell culture studies. Based on pharmacokinetic data with I3C/DIM following oral ingestion, and recent results from our laboratory on extensive “first-pass” metabolism in humans, the relevance of results obtained following prolonged incubation with any level of I3C or non-physiologically relevant concentrations of DIM (≥ 15 μM) is questionable.
Author Contributions
The author confirms being the sole contributor of this work and has approved it for publication.
Funding
This work was supported by NIH grant ESR01ES028600 and NIFA project W4122.
Conflict of Interest
The author declares that the research was conducted in the absence of any commercial or financial relationships that could be construed as a potential conflict of interest.
Publisher's Note
All claims expressed in this article are solely those of the authors and do not necessarily represent those of their affiliated organizations, or those of the publisher, the editors and the reviewers. Any product that may be evaluated in this article, or claim that may be made by its manufacturer, is not guaranteed or endorsed by the publisher.
References
1. Hayes JD, Kelleher MO, Eggleston IM. The cancer chemopreventive actions of phytochemicals derived from glucosinolates. Eur J Nutr. (2008) 47(Suppl. 2):73–88. doi: 10.1007/s00394-008-2009-8
2. Bradfied CA, Bjeldanes LF. High-performance liquid chromatographic analysis of anticarcinogenic indoles in Brassica oleracea. J Ag Fd Chem. (1987) 35:46–9. doi: 10.1021/jf00073a010
3. Bradfied CA, Bjeldanes LF. Dietary modification of xenobiotic metabolism: contribution of indolylic compounds present in Brassica oleracea. J Ag Fd Chem. (1987) 35:896–900. doi: 10.1021/jf00078a011
4. Kushad MM, Brown AF, Kurilich AC, Juvik JA, Klein BP, Wallig EH, et al. Variation of glucosinolates in vegetable crops of Brassica oleracea. J Ag Fd Chem. (1999) 47:1541–8. doi: 10.1021/jf980985s
5. Bjeldanes LF, Kim J-Y, Grose KR, Bartholomew JC, Bradfield CA. Aromatic hydrocarbon responsiveness-receptor agonists generated from indole-3-carbinol in vitro and in vivo: comparisons with 2,3,7,8-tetrachlorodibenzo-p-dioxin. Proc Natl Acad Sci USA. (1991) 88:9543–7. doi: 10.1073/pnas.88.21.9543
6. Bradfied CA, Bjeldanes LF. Structure-activity relationships of dietary indoles: a proposed mechanism of xenobiotic metabolism. J Toxicol Environ Hlth. (1987) 21:311–23. doi: 10.1080/15287398709531021
7. Thomson CA, Ho E, Strom MB. Chemopreventive properties of 3,3'-diindolylmethane in breast cancer: evidence from experimental and human studies. Nutr Rev. (2016) 74:432–43. doi: 10.1093/nutrit/nuw010
8. Bradlow HL. Review. Indole-3-carbinol as a chemoprotective agent in breast and prostate cancer. In Vivo. (2008) 22:441–5.
9. Maruthanila VL, Poornima J, Mirunalini S. Attenuation of carcinogenesis and the mechanism underlying by the influence of indole-3-carbinol and its metabolite 3,3'-diindolylmethane: a therapeutic marvel. Adv Pharmacol Sci. (2014) 2014:832161. doi: 10.1155/2014/832161
10. Weng J-R, Tsai C-H, Kulp SK, Chen CS. Indole-3-carbinol as a chemopreventive and anti-cancer agent. Cancer Lett. (2008) 262:153–63. doi: 10.1016/j.canlet.2008.01.033
11. Li Y, Sarkar FH. Role of BioResponse 3,3'-diindolylmethane in the treatment of human prostate cancer: clinical experience. Med Princ Pract. (2015) 25(Suppl. 2):11–7. doi: 10.1159/000439307
12. Fujioka N, Fritz V, Upadhyaya P, Kassie F, Hecht SS. Research on cruciferous vegetables, indole-3-carbinol, and cancer prevention: a tribute to Lee W. Wattenberg Molec Nutr Fd Res. (2016) 60:1228–38. doi: 10.1002/mnfr.201500889
13. Minich DM, Bland JS. A review of the clinical efficacy and safety of cruciferous vegetable phytochemicals. Nutr Rev. (2007) 65:259–67. doi: 10.1111/j.1753-4887.2007.tb00303.x
14. Ambrosone CB, Tang L. Cruciferous vegetable intake and cancer prevention: role of nutrigenetics. Cancer Prev Res. (2009) 2:298–300. doi: 10.1158/1940-6207.CAPR-09-0037
15. Higdon JV, Delage B, Williams DE, Dashwood RH. Cruciferous vegetables and human cancer risk: epidemiologic evidence and mechanistic basis. Pharmacol Res. (2007) 55:224–36. doi: 10.1016/j.phrs.2007.01.009
16. Rogan EG. The natural chemopreventive compound indole-3-carbinol: state of the science. In Vivo. (2006) 20:221–8.
17. Kim JK, Park SU. Current results on the biological and pharmacological activities of Indole-3-carbinol. EXCLI J. (2018) 17:181–5. doi: 10.17179/excli2017-1028
18. Banerjee S, Kong D, Wang Z, Bao B, Hillman GG, Sarkar FH. Attenuation of multi-targeted proliferation-linked signaling by 3,3'-diindolylmethane (DIM): from bench to clinic. Mutat Res. (2011) 728:47–66. doi: 10.1016/j.mrrev.2011.06.001
19. Bradlow HL, Zeligs MA. Diindolylmethane (DIM). Spontaneously forms from indole-3-carbinol (I3C) during cell culture experiments. In Vivo. (2010) 24:387–91.
20. Maier MLV, Siddens LK, Uesugi SL, Choi J, Leonard SW, Pennington JM, et al. 3,3'-Diindolylmethane (BioResponse DIM®) exhibits significant metabolism following oral dosing in humans. Drug Metabol Dispos. (2021) 49:694–705. doi: 10.1124/dmd.120.000346
21. Huang Z, Zuo L, Zhang Z, Liu J, Chen J, Dong J, et al. 3,3'-Diindolylmethane decreases VCAM-1 expression and alleviates experimental colitis via a BRCA1-dependent antioxidant pathway. Free Radic Biol Med. (2010) 50:228–36. doi: 10.1016/j.freeradbiomed.2010.10.703
22. Gao X, Liu J, Cho KB, Kedika S, Guo B. Chemopreventive agent 3,3'-diindolylmethane inhibits MDM2 in colorectal cancer cells. Int J Molec Sci. (2020) 21:4642. doi: 10.3390/ijms21134642
23. Megna BW, Carney PR, Nukaya M, Geiger P, Kennedy GD. Indole-3-carbinol induces tumor cell death: function follows form. J Surg Res. (2016) 204:47–54. doi: 10.1016/j.jss.2016.04.021
24. Leem S-H, Li XJ, Park MH, Park BH, Kim SM. Genome-wide transcriptome analysis reveals inactivation of Wnt/β-catenin by 3,3'-diindolylmethane inhibiting proliferation of colon cancer cells. Int J Oncol. (2015) 47:918–26. doi: 10.3892/ijo.2015.3089
25. Wu Y, Li RW, Huang H, Fletcher A, Yu L, Pham Q, et al. Inhibition of tumor growth by dietary indole-3-carbinol in a prostate cancer xenograft model may be associated with disrupted gut microbial interactions. Nutrients. (2019) 11:467. doi: 10.3390/nu11020467
26. Cho HJ, Seon MR, Lee YM, Kim J, Kim JK, Kim JH, et al. 3,3'-Diindolylmethane suppresses the inflammatory response to lipopolysaccharide in murine macrophages. J Nutr. (2008) 138:17–23. doi: 10.1093/jn/138.1.17
27. Machijima Y, Ishikawam C, Sawada S, Okudaira T, Uchihara JN, Tanaka Y N, et al. Anti-adult T-cell leukemia/lymphoma effects of indole-3-carbinol. Retrovirology. (2009) 6:7. doi: 10.1186/1742-4690-6-7
28. Rajoria S, Suriano R, Wilson YL, Schantz SP, Moscatello A, Geliebter RK, et al. 3,3'-Diindolylmethane inhibits migration and invasion of human cancer cells through combined suppression of ERK and AKT pathways. Oncol Rep. (2011) 25:491–7. doi: 10.3892/or.2010.1076
29. Li WX, Chen LP, Sun MY, Li JT, Liu W, Zhu HZ. 3'3-Diindolylmethane inhibits migration, invasion and metastasis of hepatocellular carcinoma by suppressing FAK signaling. Oncotarget. (2015) 6:23776–92. doi: 10.18632/oncotarget.4196
30. Pappa G, Strathmann J, Löwinger M, Bartsch H, Gerhäuser C. Quantitative combination effects between sulforaphane and 3,3'-diindolylmethane on proliferation of human colon cancer cells in vitro. Carcinogenesis. (2007) 28:1471–7. doi: 10.1093/carcin/bgm044
31. Kim SM. Cellular and molecular mechanisms of 3,3'-diindolylmethane in gastrointestinal cancer. Int J Molec Sci. (2016) 17:1155. doi: 10.3390/ijms17071155
32. Aggarwal BB, Ichikawa H. Molecular targets and anticancer potential of indole-3-carbinol and its derivatives. A review. Cell Cycle. (2005) 4:1201–15. doi: 10.4161/cc.4.9.1993
33. Kim YS, Milner J.A. Targets for indole-3-carbinol in cancer prevention. J Nutr Biochem. (2005) 16:65–73. doi: 10.1016/j.jnutbio.2004.10.007
34. Jellinck PH, Forkert PG, Riddick DS, Okey AB, Michnovicz HL, et al. Ah receptor binding properties of indole carbinols and induction of hepatic estradiol hydroxylation. Biochem Pharmacol. (1993) 45:1129–36. doi: 10.1016/0006-2952(93)90258-X
35. Auborn KJ, Fan S, Rosen EM, Goodwin L, Chandreskaren A, Williams DE, et al. Indole-3-carbinol is a negative regulator of estrogen. J Nutr. (2003) 13(Suppl. 3):1S−6S. doi: 10.1093/jn/133.7.2470S
36. Chen I, McDougal A, Wang F, Safe S. Aryl hydrocarbon receptor-mediated antiestrogenic and antitumorigenic activity of diindolylmethane. Carcinogenesis. (1998) 19:1631–9. doi: 10.1093/carcin/19.9.1631
37. Firestone GL, Bjeldanes LF. Indole-3-carbinol and 3-3'-diindolylmethane antiproliferative signaling pathways control cell-cycle gene transcription in human breast cancer cells by regulating promoter-Sp1 transcription factor interactions. J Nutr. (2003) 133(Suppl. 7):2448S−55S. doi: 10.1093/jn/133.7.2448S
38. Wang TTY, Milner MJ, Milner JA, Kim YS. Estrogen receptor alpha as a target for indole-3-carbinol. J Nutr Biochem. (2006) 17:659–64. doi: 10.1016/j.jnutbio.2005.10.012
39. Riby JE, Feng C, Chang Y.-C., Schaldach CM, Firestone LF, et al. The major cyclic trimeric product of indole-3-carbinol is a strong agonist of the estrogen receptor signaling pathway. Biochemistry. (2000) 39:910–8. doi: 10.1021/bi9919706
40. Lee J. 3,3'-Diindolylmethane inhibits TNF-α- and TGF-β-induced epithelial-mesenchymal transition in breast cancer cells. Nutr Cancer. (2019) 71:992–1006. doi: 10.1080/01635581.2019.1577979
41. Tin AS, Park AH, Sundar SN, Firestone G.L. Essential role of the cancer stem/progenitor cell marker nucleostemin for indole-3-carbinol anti-proliferative responsiveness in human breast cancer cells. BMC Biol. (2014) 12:72. doi: 10.1186/s12915-014-0072-6
42. Saati GE, Archer MC. Inhibition of fatty acid synthase and Sp1 expression by 3,3'-diindolylmethane in human breast cancer cells. Nutr Cancer. (2011) 63:790–94. doi: 10.1080/01635581.2011.570896
43. Staub RE, Onisko B, Bjeldanes LF. Fate of 3,3'-diindolylmethane in cultured MCF-7 human breast cancer cells. Chem Res Toxicol. (2006) 19:436–42. doi: 10.1021/tx050325z
44. Staub RE, Feng C, Onisko B, Bailey GS, Firestone GL, Bjeldanes L.F. Fate of indole-3-carbinol in cultured human breast tumor cells. Chem Res Toxicol. (2002) 15:101–9. doi: 10.1021/tx010056m
45. Firestone GL, Sundar SN. Minireview: modulation of hormone receptor signaling by dietary anticancer indoles. Molec Endocrinol. (2009) 23:1940–7. doi: 10.1210/me.2009-0149
46. Donovan MG, Selmin OI, Romagnolo DF. Aryl hydrocarbon receptor diet and breast cancer risk. Yale J Biol Med. (2018) 91:105–27.
47. Safe S, Wormke M. Inhibitory aryl hydrocarbon receptor-estrogen receptor alpha cross-talk and mechanisms of action. Chem Res Toxicol. (2003) 16:807–16. doi: 10.1021/tx034036r
48. Thomson CA, Chow HHS, Wertheim BC, Roe DJ, Stopeck A, Maskarinec G, et al. A randomized, placebo-controlled trial of diindolylmethane for breast cancer biomarker modulation in patients taking tamoxifen. Breast Cancer Res Treat. (2017) 165:97–107. doi: 10.1007/s10549-017-4292-7
49. Le HT, Schaldach CM, Firestone GL, Bjeldanes LF. Plant-derived 3,3'-diindolylmethane is a strong androgen antagonist in human prostate cancer cells. J Biol Chem. (2003) 278:21136–45. doi: 10.1074/jbc.M300588200
50. Hayes CL, Spink DC, Spink BC, Cao JQ, Walker NJ, Sutter TR. 17-Beta-estradiol hydroxylation catalyzed by human cytochrome P450 1B1. Proc Natl Acad Sci USA. (1996) 93:9776–81. doi: 10.1073/pnas.93.18.9776
51. Lord RS, Bongiovanni B, Bralley JA. Estrogen metabolism and the diet-cancer connection: rationale for assessing the ratio of urinary hydroxylated estrogen metabolites. Altern Med Rev. (2002) 7:112–29.
52. Dalessandri KM, Firestone GL, Fitch MD, Bradlow HL, Bjeldanes LF. Pilot study: effect of 3,3'-diindolylmethane supplements on urinary hormone metabolites in postmenopausal women with a history of early-stage breast cancer. Nutr Cancer. (2004) 50:161–7. doi: 10.1207/s15327914nc5002_5
53. Watson GW, Beaver L.M, Williams DE, Dashwood RH, Ho E. Phytochemicals from cruciferous vegetables, epigenetics, and prostate cancer prevention. AAPS J. (2013) 15:951–61. doi: 10.1208/s12248-013-9504-4
54. Kaur P, Shorey L, Ho E, Dashwood RH, Williams DE. The epigenome as a potential mediator of cancer and disease prevention in prenatal development. Nutr Rev. (2013) 71:441–57. doi: 10.1111/nure.12030
55. Wu T-U, Khor TO, Su Z-Y, Saw L-L, Shu L, Cheung KL, et al. Epigenetic modifications of Nrf2 by 3,3'-diindolylmethane in vitro in TRAMP C1 cell line and in vivo TRAMP prostate tumors. AAPS J. (2013) 15:864–74. doi: 10.1208/s12248-013-9493-3
56. Wong CP, Hsu A, Buchanan A, Palomera-Sanchez Z, Beaver LM, Houseman EA, et al. Effects of sulforaphane and 3,3'-diindolylmethane on genome-wide promoter methylation in normal prostate epithelial cells and prostate cancer cells. PLoS ONE. (2014) 9:e86787. doi: 10.1371/journal.pone.0086787
57. Yongming Li Y, Li X, Guo B. Chemopreventive agent 3,3'-diindolylmethane selectively induces proteasomal degradation of class I histone deacetylases. Cancer Res. (2010) 70:646–54. doi: 10.1158/0008-5472.CAN-09-1924
58. Beaver LM, Yu T-W, Sokolowski EI, Williams DE, Dashwood E, et al. 3,3'Diindolylmethane, but not indole-3-carbinol, inhibits histone deacetylase activity in prostate cancer cells. Toxicol Appl Pharmcol. (2012) 263:345–51. doi: 10.1016/j.taap.2012.07.007
59. Jin U-H, Cheng Y, Park H, Davidson LA, Callaway ES, et al. Short chain fatty acids enhance aryl hydrocarbon (Ah) responsiveness in mouse colonocytes and Caco-2 human colon cancer cells. Sci Rep. (2017) 7:10163. doi: 10.1038/s41598-017-10824-x
60. Palmoera-Sanchez Z, Watson GW, Wong CP, Beaver LM, Williams DE, Dashwood E, et al. The phytochemical 3,3'-diindolylmethane decreases expression of AR-controlled DNA damage repair genes through repressive chromatin modifications and is associated with DNA damage in prostate cancer cells. J Nutr Biochem. (2017) 47:113–19. doi: 10.1016/j.jnutbio.2017.05.005
61. Phuah NH, Nagoor NH. Regulation of microRNAs by natural agents: new strategies in cancer therapies. Biomed Res Int. (2014) 2014:804510. doi: 10.1155/2014/804510
62. Ye Y, Fang Y, Xu W, Wang Q, Zhou J, Lu R. 3,3'-Diindolylmethane induces anti-human gastric cancer cells by the miR-30e-ATG5 modulating autophagy. Biochem Pharmacol. (2016) 115:77–84. doi: 10.1016/j.bcp.2016.06.018
63. Nikulin SV, Alekseev BY, Sergeeva NS, Karalkin PA, Nezhurina EK, Kirsanova VA, et al. Breast cancer organoid model allowed to reveal potentially beneficial combinations of 3,3'-diindolylmethane and chemotherapy drugs. Biochimie. (2020) 179:217–27. doi: 10.1016/j.biochi.2020.10.007
64. El-Daly SM, Gamal-Eldeen AM, Gouhar SA, Abo-Elfadl MT, El-Saeed G. Modulatory effect of indoles on the expression of miRNAs regulating G1/S cell cycle phase in breast cancer cells. Appl Biochem Biotechnol. (2020) 192:1208–23. doi: 10.1007/s12010-020-03378-8
65. Ho TT, Huang J, Zhou N, Zhang Z, Koirala P, Zhou X, et al. Regulation of PCGEM1 by p54/nrb in prostate cancer. Sci Rep. (2016) 6:34529. doi: 10.1038/srep34529
66. Kalhori MR, Khodayari H, Khodayari S, Vesovic M, Jackson G, Farzaei A, et al. Regulation of long non-coding RNAs by plant secondary metabolites: a novel anticancer therapeutic approach. Cancers. (2021) 13:1274. doi: 10.3390/cancers13061274
67. Zinovieva OL, Grineva EN, Prokofjeva MM, Karpov DS, Krasnov GS, Prassolov VS, et al. Treatment with anti-cancer agents results in profound changes in lncRNA expression in colon cancer cells. Molec Biol. (2017) 51:841–8. doi: 10.1134/S0026893317050247
68. Kassie F, Anderson LB, Scherber R, Yu N, Lahti D, Upadhyaya SS, et al. Indole-3-carbinol inhibits 4-(methylnitrosamino)-1-(3-pyridyl)-1-butanone plus benzo(a)pyrene-induced lung tumorigenesis in A/J mice and modulates carcinogen-induced alterations in protein levels. Cancer Res. (2007) 67:6502–11. doi: 10.1158/0008-5472.CAN-06-4438
69. Sinnhuber RO, Hendricks JD, Wales JH, Putnam GB. Neoplasms in rainbow trout, a sensitive animal model for environmental carcinogenesis. Ann NY Acad Sci. (1977) 298:389–408. doi: 10.1111/j.1749-6632.1977.tb19280.x
70. Nixon JE, Hendricks JD, Pawlowski NE, Pereira CB, Sinnhuber RO, Bailey GS. Inhibition of aflatoxin B1 carcinogenesis in rainbow trout by flavone and indole compounds. Carcinogenesis. (1984) 5:615–9. doi: 10.1093/carcin/5.5.615
71. Williams DE. The rainbow trout liver cancer model: response to environmental chemicals and studies on promotion and chemoprevention. Comp Biochem Physiol C Toxicol Pharmacol. (2011) 155:121–7. doi: 10.1016/j.cbpc.2011.05.013
72. Bailey GS, Williams DE, Hendricks JD. Fish models for environmental carcinogenesis: the rainbow trout. Environ Hlth Perspect. (1996) 104(Suppl. 1):5–21. doi: 10.1289/ehp.96104s15
73. Bailey GS, Reddy AP, Pereira CB, Harttig U, Baird W, Spitsbergen JM, et al. Nonlinear cancer response at ultralow dose: a 40800-animal ED(001) tumor and biomarker study. Chem Res Toxicol. (2009) 22:1264–76. doi: 10.1021/tx9000754
74. Bailey G, Williams D, Orner G, Hendricks J, Pereira C. Review. Cancer risk at ultra-low dose: Lessons learned from. 40:000–animal cancer dose–response studies. Genes Environm. (2012) 34:157–64. doi: 10.1525/nr.2012.16.2.110
75. Bailey GS, Dashwood RH, Fong AT, Williams DE, Scanlan RA, Hendricks JD. Modulation of mycotoxin and nitrosamine carcinogenesis by indole 3 carbinol: quantitative analysis of inhibition versus promotion. In: O'Neill IK, Chen J, Bartsch H, editors. Relevance to Human Cancer of N nitroso Compounds, Tobacco Smoke and Mycotoxins. New York: Oxford Univ Press (1991). p. 275–80.
76. Dashwood RH, Fong AT, Williams DE, Hendricks JD, Bailey GS. Promotion of aflatoxin B1 carcinogenesis by the natural tumor modulator indole-3-carbinol: influence of dose, duration, and intermittent exposure on indole-3-carbinol promotional potency. Cancer Res. (1991) 51:2362–5.
77. Oganesian A, Hendricks JD, Pereira CB, Orner GA, Bailey GS, Williams D.E. Potency of dietary indole-3-carbinol as a promoter of aflatoxin B1-initiated hepatocarcinogenesis: results from a 9000 animal tumor study. Carcinogenesis. (1999) 20:453–8. doi: 10.1093/carcin/20.3.453
78. Fong AT, Swanson HI, Dashwood RH, Williams DE, Hendricks JD, Bailey GS. Mechanisms of anti-carcinogenesis by indole-3-carbinol. Studies of enzyme induction, electrophile-scavenging, and inhibition of aflatoxin B1 activation. Biochem Pharmacol. (1990) 39:19–26. doi: 10.1016/0006-2952(90)90643-Y
79. Takahashi N, Dashwood RH, Bjeldanes LF, Williams DE, Bailey GS. Mechanisms of indole-3-carbinol (I3C) anticarcinogenesis: inhibition of aflatoxin B1-DNA adduction and mutagenesis by I3C acid condensation products. Fd Chem Toxicol. (1995) 33:851–7. doi: 10.1016/0278-6915(95)00054-6
80. Stresser DM, Bailey GS, Williams DE. Indole-3-carbinol and beta-naphthoflavone induction of aflatoxin B1 metabolism and cytochromes P-450 associated with bioactivation and detoxication of aflatoxin B1 in the rat. Drug Metabol Dispos. (1994) 22:383–91.
81. Stresser DM, Williams DE, McLellan LI, Harris TM, Bailey GS. Indole-3-carbinol induces a rat liver glutathione transferase subunit (Yc2) with high activity toward aflatoxin B1 exo-epoxide. Association with reduced levels of hepatic aflatoxin-DNA adducts in vivo. Drug Metabol Dispos. (1994) 22:392–9.
82. Shilling AD, Carlson DB, Katchamart S, Williams DE. 3,3'-Diindolylmethane, a major condensation product of indole-3-carbinol, is a potent estrogen in the rainbow trout. Toxicol Appl Pharmacol. (2001) 170:191–200. doi: 10.1006/taap.2000.9100
83. Tilton SC, Hendricks JD, Orner GA, Pereira CB, Bailey GS, Williams DE. Gene expression analysis during tumor enhancement by the dietary phytochemical and supplement, 3,3'-diindolylmethane, in rainbow trout. Carcinogenesis. (2007) 28:1589–98. doi: 10.1093/carcin/bgm017
84. Kiselev VI, Drukh VM, Muyzhnek EL, Kuznetsov IN, Pchelintseva OI, Paltsev MA. Preclinical antitumor activity of the diindolylmethane formulation in xenograft mouse model of prostate cancer. Exp Oncol. (2014) 36:90–3.
85. Shorey LE, Hagman AM, Williams DE, Ho E, Dashwood RH, Benninghoff AD. 3,3'-Diindolylmethane induces G1 arrest and apoptosis in human acute T-cell lymphoblastic leukemia cells. PLoS ONE. (2012) 7:e34975. doi: 10.1371/journal.pone.0034975
86. Kim DJ, Han BS, Ahn B, Hasegawa R, Shirai T, Ito H, et al. Enhancement by indole-3-carbinol of liver and thyroid gland neoplastic development in a rat medium-term multiorgan carcinogenesis model. Carcinogenesis. (1997) 18:377–81. doi: 10.1093/carcin/18.2.377
87. Shimamoto K, Hayashi H, Taniai E, Morita R, Imaoka M, Ishii Y, et al. Antioxidant N-acetyl-L-cysteine (NAC) supplementation reduces reactive oxygen species (ROS)-mediated hepatocellular tumor promotion of indole-3-carbinol (I3C) in rats. J Toxicol Sci. (2011) 36:775–86. doi: 10.2131/jts.36.775
88. Oganesian A, Hendricks JD, Williams DE. Long term dietary indole-3-carbinol inhibits diethylnitrosamine-initiated hepatocarcinogenesis in the infant mouse model. Cancer Lett. (1997) 118:87–94. doi: 10.1016/S0304-3835(97)00235-8
89. Stoner G, Casto B, Ralston S, Roebuck B, Pereira C, Bailey G. Development of a multi-organ rat model for evaluating chemopreventive agents: efficacy of indole-3-carbinol. Carcinogenesis. (2002) 23:265–72. doi: 10.1093/carcin/23.2.265
90. Kawajiri K, Kobayashi Y, Ohtake F, Ikuta T, Matsushima Y, Mimura Y, et al. Aryl hydrocarbon receptor suppresses intestinal carcinogenesis in ApcMin/+ mice with natural ligands. Proc Natl Acad Sci USA. (2009) 106:13481–6. doi: 10.1073/pnas.0902132106
91. Kim YH, Kwon H-S, Kim DH, Shin EK, Kang H, Park JHY, et al. 3,3'-Diindolylmethane attenuates colonic inflammation and tumorigenesis in mice. Inflamm Bowel Dis. (2009) 15:1164–73. doi: 10.1002/ibd.20917
92. Yu Z, Loehr C, Fischer KA, Louderback M, Krueger SK, Dashwood RH, et al. In utero exposure of mice to dibenzo[a,l]pyrene produces lymphoma in the offspring: role of the aryl hydrocarbon receptor. Cancer Res. (2006) 66:755–62. doi: 10.1158/0008-5472.CAN-05-3390
93. Madeen EP, Löhr CV, You H, Siddens LK, Krueger SK, Dashwood RH, et al. Dibenzo[def,p]chrysene transplacental carcinogenesis in wild-type, Cyp1b1 knockout, and CYP1B1 humanized mice. Molec Carcinog. (2017) 56:163–71. doi: 10.1002/mc.22480
94. Shorey L, Castro DJ, Baird W, Siddens B, Löhr, Matzke M, et al. Transplacental carcinogenesis with dibenzo[def,p]chrysene (DBC): timing of maternal exposures determines target tissue response in offspring. Cancer Lett. (2012) 317:49–55. doi: 10.1016/j.canlet.2011.11.010
95. Yu Z, Mahadevan B, Löhr CV, Fischer KA, Louderback MA, Krueger SK, et al. Indole-3-carbinol in the maternal diet provides chemoprotection for the fetus against transplacental carcinogenesis by the polycyclic aromatic hydrocarbon, dibenzo[a,l]pyrene. Carcinogenesis. (2006) 27:2116–23. doi: 10.1093/carcin/bgl072
96. Shorey LE, Madeen EP, Atwell LL, Ho E, Löhr CV, Pereira CB, et al. Differential modulation of dibenzo[def,p]chrysene transplacental carcinogenesis: maternal diets rich in indole-3-carbinol versus sulforaphane. Toxicol Appl Pharmacol. (2013) 270:60–9. doi: 10.1016/j.taap.2013.02.016
97. Castro DJ, Yu Z, Löhr CV, Pereira CB, Giovanini J, Fischer KA, et al. Chemoprevention of dibenzo[a,l]pyrene transplacental carcinogenesis in mice born to mothers administered green tea: primary role of caffeine. Carcinogenesis. (2008) 29:1581–6. doi: 10.1093/carcin/bgm237
98. Castro DJ, Löhr C, Fischer K, Waters K, Webb-Robertson B-J, Dishwood RH, et al. Identifying efficacious approaches to chemoprevention with chlorophyllin, purified chlorophylls and freeze-dried spinach in a mouse model of transplacental carcinogenesis. Carcinogenesis. (2009) 30:315–20. doi: 10.1093/carcin/bgn280
99. Larsen-Su S, Williams DE. Transplacental exposure to indole-3-carbinol induces sex-specific expression of CYP1A1 and CYP1B1 in the liver of Fischer 344 neonatal rats. Toxicol Sci. (2001) 64:162–8. doi: 10.1093/toxsci/64.2.162
100. Lubet RA, Heckman BM, De Flora SL, Steele VE, Crowell JA, Juliana CJ, et al. Effects of 5,6-benzoflavone, indole-3-carbinol (I3C) and diindolylmethane (DIM) on chemically-induced mammary carcinogenesis: is DIM a substitute for I3C? Oncol Rep. (2011) 26:731–6. doi: 10.3892/or.2011.1316
101. Benninghoff AD, Williams D.E. The role of estrogen receptor beta in transplacental cancer prevention by indole-3-carbinol. Cancer Prev Res. (2013) 6:339–48. doi: 10.1158/1940-6207.CAPR-12-0311
102. Ki MK, Park JHY. Cruciferous vegetable intake and the risk of human cancer: epidemiological evidence. Proc Nutr Soc. (2009) 68:103–10. doi: 10.1017/S0029665108008884
103. Sepkovic DW, Bradlow HL, Bell M. Quantitative determination of 3,3'-diindolylmethane in urine of individuals receiving indole-3-carbinol. Nutr Cancer. (2001) 41:57–63. doi: 10.1080/01635581.2001.9680612
104. Bell MC, Crowley-Nowick P, Bradlow HL, Sepkovic DW, Schmidt-Grimminger D, Howell P, et al. Placebo-controlled trial of indole-3-carbinol in the treatment of CIN. Gynecol Oncol. (2000) 78:123–9. doi: 10.1006/gyno.2000.5847
105. Reed GA, Peterson KS, Smith HJ, Gray JC, Sullivan DK, Mayo MS, et al. A phase 1 study of indole-3-carbinol in women: tolerability and effects. Cancer Epidemiol Biomarkers Prev. (2005) 14:1953–60. doi: 10.1158/1055-9965.EPI-05-0121
106. Reed GA, Arneson DW, Putnam WC, Smith HJ, Gray JC, Sullivan DK, et al. Single-dose and multiple-dose administration of indole-3-carbinol to women: pharmacokinetics based on 3,3'-diindolylmethane. Cancer Epidemiol Biomarkers Prev. (2006) 15:2477–81. doi: 10.1158/1055-9965.EPI-06-0396
107. Ashrafian L, Sukhikh G, Kiselev V, Paltsev M, Drukh V, Kuznetsov I, et al. Double-blind randomized placebo-controlled multicenter clinical trial (phase IIa) on diindolylmethane's efficacy and safety in the treatment of CIN: implications for cervical cancer prevention. EPMA J. (2015) 6:25. doi: 10.1186/s13167-015-0048-9
108. Heath EI, Heilbrun LK, Li J, Vaishampayan U, Harper F, Pemberton FH, et al. A phase 1 dose-escalation study of oral BR-DIM (BioResponse 3,3'-diindolylmethane) in castrate-resistant, non-metastatic prostate cancer. Am J Transl Res. (2010) 2:402–11.
109. Hwang C, Sethi S, Heibrun LK, Gupta NS, Chitale DA, Sakr WA, et al. Anti-androgenic activity of absorption-enhanced 3,3'-diindolylmethane in prostatectomy patients. Am J Transl Res. (2016) 8:166–76.
110. Paltsev M, Kiselev V, Drukh V, Muyzhnek E, Kuznetsov I, Andrianova P, et al. First results of the double-blind randomized placebo-controlled multicenter clinical trial of DIM-based therapy designed as personalized approach to reverse prostatic intraepithelial neoplasia (PIN). EPMA J. (2016) 7:5. doi: 10.1186/s13167-016-0057-3
111. Stresser DM, Bjeldanes LF, Bailey GS, Williams DE. The anticarcinogen 3,3'-diindolylmethane is an inhibitor of cytochrome P-450. J Biochem Toxicol. (1995) 10:191–201. doi: 10.1002/jbt.2570100403
112. Parkin DR, Lu Y, Bliss RL, Malejka-Giganti D. Inhibitory effects of a dietary phytochemical 3,3'-diindolylmethane on the phenobarbital-induced hepatic CYP mRNA expression and CYP-catalyzed reactions in female rats. Fd Chem Toxicol. (2008) 46:2451–8. doi: 10.1016/j.fct.2008.03.029
113. Gross-Steinmeyer K., Stapleton PL, Tracy JH, Bammler TK, Strom SC, Buhler DL, et al. Modulation of aflatoxin B1-mediated genotoxicity in primary cultures of human hepatocytes by diindolylmethane, curcumin, and xanthohumols. Toxicol Sci. (2009) 112:303–10. doi: 10.1093/toxsci/kfp206
114. Fujioka N, Ainslie-Waldman CE, Upadhyaya P, Carmella SG, Fritz VA, Rohwer C, et al. Urinary 3,3'-diindolylmethane: a biomarker of glucobrassicin exposure and indole-3-carbinol uptake in humans. Cancer Epidemiol Biomarkers Prev. (2014) 23:282–87. doi: 10.1158/1055-9965.EPI-13-0645
115. Fujioka N, Ransom BW, Carmella SG, Upadhyaya P, Lindgren BR, Roper-Batker A, et al. Harnessing the power of cruciferous vegetables: developing a biomarker for brassica vegetable consumption using urinary 3,3'-diindolylmethane. Cancer Prev Res. (2016) 9:788–93. doi: 10.1158/1940-6207.CAPR-16-0136
116. Anderton MJ, Manson MM, Verschoyle R, Gescher A, Steward WP, Williams DE, et al. Physiological modeling of formulated and crystalline 3,3'-diindolylmethane pharmacokinetics following oral administration in mice. Drug Metabol Disp. (2004) 32:632–8. doi: 10.1124/dmd.32.6.632
117. Anderton MJ, Manson MM, Verschoyle RD, Gescher A, Lamb JH, Farmer PB, et al. Pharmacokinetics and tissue disposition of indole-3-carbinol and its acid condensation products after oral administration to mice. Clin Cancer Res. (2004) 10:5233–41. doi: 10.1158/1078-0432.CCR-04-0163
118. Paltsev M, Kiselev V, Muyzhnek E, Drukh V, Kuznetsov I, Pchelintseva O. Comparative preclinical pharmacokinetics study of 3,3'-diindolylmethane formulations: is personalized treatment and targeted chemoprevention in the horizon? EPMA J. (2013) 4:25. doi: 10.1186/1878-5085-4-25
119. Reed GA, Sunega JM, Sullivan DK, Gray JC, Mayo MS, Crowell A, et al. Single-dose pharmacokinetics and tolerability of absorption-enhanced 3,3'-diindolylmethane in healthy subjects. Cancer Epidemol Biomarkers Prev. (2008) 17:2619–624. doi: 10.1158/1055-9965.EPI-08-0520
120. Hubbard TD, Murray IA, Perdew GH. Indole and tryptophan metabolism: endogenous and dietary routes to Ah receptor activation. Drug Metabol Dispos. (2015) 43:1522–35. doi: 10.1124/dmd.115.064246
121. Rzemieniec J, Wnuk A, Lasoń W, Bilecki W, Kajta M. The neuroprotective action of 3,3'-diindolylmethane against ischemia involves an inhibition of apoptosis and autophagy that depends on HDAC and AhR/CYP1A1 but not ERα/CYP19A1 signaling. Apoptosis. (2019) 24:435–52. doi: 10.1007/s10495-019-01522-2
122. Esteve M. Mechanisms underlying biological effects of cruciferous glucosinolate-derived isothiocyanates/indoles: a focus on metabolic syndrome. Front Nutr. (2020) 7:111. doi: 10.3389/fnut.2020.00111
123. Rajoria S, Suriano R, Parmar PS, Wilson YL, Megwalu U, Moscatello A, et al. 3,3'-Diindolylmethane modulates estrogen metabolism in patients with thyroid proliferative disease: a pilot study. Thyroid. (2011) 21:299–304. doi: 10.1089/thy.2010.0245
124. Priore GD, Gudipudi DK, Montemarano N, Restivo AM, Malanowska-Stega J, Arslan AA. Oral diindolylmethane (DIM): pilot evaluation of a nonsurgical treatment for cervical dysplasia. Gynecol Oncol. (2010) 116:464–7. doi: 10.1016/j.ygyno.2009.10.060
125. Perez-Chacon G, de Los Rios C, Zapata JM. Indole-3-carbinol induces cMYC and IAP-family downmodulation and promotes apoptosis of Epstein-Barr virus (EBV)-positive but not of EBV-negative Burkitt's lymphoma cell lines. Pharmacol Res. (2014) 89:46–56. doi: 10.1016/j.phrs.2014.08.005
126. Sepkovic DW, Raucci L, Stein J, Carlisle AD, Auborn K, Ksieski HB, et al. 3,3'-Diindolylmethane increases serum interferon-γ levels in the K14-HPV16 transgenic mouse model for cervical cancer. In Vivo. (2012) 26:207–11.
127. Morales-Prieto DM, Herrmann J, Osterwald H, Kochhar PS, Schleussner E, Markert, et al. Comparison of dienogest effects upon 3,3'-diindolylmethane supplementation in models of endometriosis and clinical cases. Reprod Biol. (2018) 18:252–8. doi: 10.1016/j.repbio.2018.07.002
128. Fan S, Meng Q, Xu J, Jiao Y, Zhao L, Zhang X, et al. DIM (3,3'-diindolylmethane). Confers protection against ionizing radiation by a unique mechanism. Proc Natl Acad Sci USA. (2013) 110:18650–5. doi: 10.1073/pnas.1308206110
129. Available, online at: NCT02483624 at ClinicalTrials.gov (accessed June 15, 2021).
130. Available, online at: NCT00591305 at ClinicalTrials.gov (accessed June 15, 2021).
131. Available, online at: NCT01846364 at ClinicalTrials.gov (accessed June 15, 2021).
132. Available, online at: NCT03465280 at ClinicalTrials.gov (accessed June 15, 2021).
133. Hubbard TD, Murray IA, Bisson WH, Lahoti T, Gowda K, Amin SG, et al. Adaption of the human aryl hydrocarbon receptor to sense microbiota-derived indoles. Sci Rep. (2015) 5:12689. doi: 10.1038/srep12689
134. Rothhammer V, Quintana FJ. The aryl hydrocarbon receptor: an environmental sensor integrating immune responses in health and disease. Nature Rev Immunol. (2019) 19:184–97. doi: 10.1038/s41577-019-0125-8
135. Busbee PB, Rouse M, Nagarkatti M, Nagarkatti PS. Use of natural AhR ligands as potential therapeutic modalities against inflammatory disorders. Nutr Rev. (2013) 71:353–69. doi: 10.1111/nure.12024
136. Islam J, Sato S, Watanabe K, Watanabe T, Ardiansyah, Hirahara K, et al. Dietary tryptophan alleviates dextran sodium sulfate-induced colitis through aryl hydrocarbon receptor in mice. J Nutr Biochem. (2017) 42:43–50. doi: 10.1016/j.jnutbio.2016.12.019
137. Busbee PB, Menzel L, Alrafas HR, Dopkins N, Becker W, Miranda K, et al. Indole-3-carbinol prevents colitis and associated microbial dysbiosis in an IL-22-dependent manner. JCI Insight. (2020) 5:pii:127551. doi: 10.1172/jci.insight.127551
138. Julliard W, De Wolfe TJ, Fechner JH, Safdar N, Agni R, Mezrich J.D. Amelioration of clostridium difficile infection in mice by dietary supplementation with indole-3-carbinol. Ann Surg. (2017) 265:1183–91. doi: 10.1097/SLA.0000000000001830
139. Korecka A, Dona A, Lahiri S, Tett AJ, Al-Asmakh M, Braniste V, et al. Bidirectional communication between the aryl hydrocarbon receptor (AhR) and the microbiome tunes host metabolism. Nature Partner J Biofilms Microbiomes. (2016) 2:16014. doi: 10.1038/npjbiofilms.2016.14
140. Alrafas HRD, Busbee B, Nagarkatti P, Nagarkatti M. Effect of 3,3'-diindolylmethane on gut microbiome in colorectal cancer. J Immunol. (2018) 200 (1 Suppl):177.21.
141. Saw CL-L, Cintron M, Wu T-Y, Guo Y, Huang Y, Jeong W-S, et al. Pharmacodynamics of dietary phytochemical indoles I3C and DIM: Induction of Nrf-2-mediated Phase II drug metabolizing and antioxidant genes and synergism with isothiocyanates. Biopharm Drug Dispos. (2011) 32:289–300. doi: 10.1002/bdd.759
142. Cashman JR, Zhang J. Human flavin-containing monooxygenases. Annu Rev Pharmacol Toxicol. (2006) 46:65–100. doi: 10.1146/annurev.pharmtox.46.120604.141043
143. Krueger SK, Williams D.E. Mammalian flavin-containing monooxygenases: structure/function, genetic polymorphisms and role in drug metabolism. Pharmacol Therap. (2005) 106:357–87. doi: 10.1016/j.pharmthera.2005.01.001
144. Koukouritaki SB, Simpson P, Yeung CK, Rettie AE, Hines R.N. Human hepatic flavin-containing monooxygenases 1 (FMO1) and 3 (FMO3) developmental expression. Pediatr Res. (2002) 51:236–43. doi: 10.1203/00006450-200202000-00018
145. Katchamart S, Stresser DM, Dehal SS, Kupfer D, Williams D.E. Concurrent flavin-containing monooxygenase down-regulation and cytochrome P-450 induction by dietary indoles in rat: implications for drug-drug interaction. Drug Metabol Dispos. (2000) 28:9309–6.
146. Larsen-Su S, Williams D.E. Dietary indole-3-carbinol inhibits FMO activity and the expression of flavin-containing monooxygenase form 1 in rat liver and intestine. Drug Metabol Dispos. (1996) 24:927–31.
147. Wang T, Shankar K, Ronis MJ, Mehendale HM. Potentiation of thioacetamide liver injury in diabetic rats is due to induced CYP2E1. J Pharmacol Exp Ther. (2000) 294:473–9.
148. Katchamart S, Williams D.E. Indole-3-carbinol modulation of hepatic monooxygenases CYP1A1, CYP1A2 and FMO1 in guinea pig, mouse and rabbit. Comp Biochem Physiol C Toxicol Pharmacol. (2001) 129:377–84. doi: 10.1016/S1532-0456(01)00217-4
149. Cashman JR, Xiong Y, Lin J, Verhagen H, van Poppel G, van Bladeren PJ, et al. In vitro and in vivo inhibition of human flavin-containing monooxygenase form 3 (FMO3) in the presence of dietary indoles. Biochem Pharmacol. (1999) 58:1047–55. doi: 10.1016/S0006-2952(99)00166-5
150. Phillips IR, Shephard E.A. Flavin-containing monooxygenase 3 (FMO3): genetic variants and their consequences for drug metabolism and disease. Xenobiotica. (2020) 50:19–33. doi: 10.1080/00498254.2019.1643515
151. Roncal C, Martínez-Aguilar E, Orbe J, Ravassa S, Fernandez-Montero A, Saenz-Pipaon G, et al. Trimethylamine-N-oxide (TMAO) predicts cardiovascular mortality in peripheral artery disease. Sci Rep. (2019) 9:15580. doi: 10.1038/s41598-019-52082-z
152. Available, online at: https://clinicaltrials.gov/ct2/show/NCT03152097?term=3%2C3%27-diindolylmethane&draw=3&rank=11 (accessed June 17, 2021).
153. Krueger SK, Van Dyke JE, Hines RN, Williams DE. The role of flavin-containing monooxygenase (FMO) in the metabolism of tamoxifen and other tertiary amines. Drug Metabol Rev. (2006) 38:139–47. doi: 10.1080/03602530600569919
154. Williams DE, Shigenaga MK, Castagnoli N Jr. The role of cytochromes P-450 and flavin containing monooxygenase in the metabolism of (S) nicotine by rabbit lung. Drug Metabol Dispos. (1990) 18:418–428.
155. Cashman JR, Park SB, Berkman CE, Cashman LE. Role of hepatic flavin-containing monooxygenase 3 in drug and chemical metabolism in adult humans. Chem Biol Interact. (1995) 96:33–46. doi: 10.1016/0009-2797(94)03581-R
156. Vogel VG. Reducing the risk of breast cancer with tamoxifen in women at increased risk. J Clin Oncol. (2001) 19(Suppl. 18):87S−92S.
Keywords: glucobrassicin, indole-3-carbinol, cancer, chemoprevention, pharmacokinetics, 3, 3'-diindolylmethane
Citation: Williams DE (2021) Indoles Derived From Glucobrassicin: Cancer Chemoprevention by Indole-3-Carbinol and 3,3'-Diindolylmethane. Front. Nutr. 8:734334. doi: 10.3389/fnut.2021.734334
Received: 01 July 2021; Accepted: 27 August 2021;
Published: 01 October 2021.
Edited by:
Donato Angelino, University of Teramo, ItalyReviewed by:
Diego A. Moreno, CEBAS-CSIC Food Science and Technology Department (Spanish National Research Council), SpainYanling Wang, Georgia State University, United States
Copyright © 2021 Williams. This is an open-access article distributed under the terms of the Creative Commons Attribution License (CC BY). The use, distribution or reproduction in other forums is permitted, provided the original author(s) and the copyright owner(s) are credited and that the original publication in this journal is cited, in accordance with accepted academic practice. No use, distribution or reproduction is permitted which does not comply with these terms.
*Correspondence: David E. Williams, ZGF2aWQud2lsbGlhbXNAb3JlZ29uc3RhdGUuZWR1