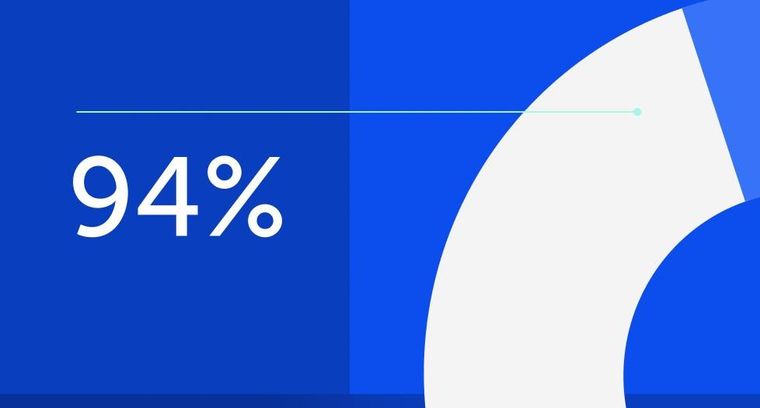
94% of researchers rate our articles as excellent or good
Learn more about the work of our research integrity team to safeguard the quality of each article we publish.
Find out more
REVIEW article
Front. Nutr., 10 August 2021
Sec. Nutrition and Metabolism
Volume 8 - 2021 | https://doi.org/10.3389/fnut.2021.709435
This article is part of the Research TopicPersonalized Nutrition with Polyphenols – which, for whom, when and how?View all 6 articles
The healing of any injury requires a dynamic balance of initiation and resolution of inflammation. This hypothesis-generating review presents an overview of the various nutrients that can act as signaling agents to modify the metabolic responses essential for the optimal healing of injury-induced inflammation. In this hypothesis-generating review, we describe a defined nutritional program consisting of an integrated interaction of a calorie-restricted anti-inflammatory diet coupled with adequate levels of omega-3 fatty acids and sufficient levels of dietary polyphenols that can be used in clinical trials to treat conditions associated with insulin resistance. Each dietary intervention works in an orchestrated systems-based approach to reduce, resolve, and repair the tissue damage caused by any inflammation-inducing injury. The orchestration of these specific nutrients and their signaling metabolites to facilitate healing is termed the Resolution Response. The final stage of the Resolution Response is the activation of intracellular 5' adenosine monophosphate-activated protein kinase (AMPK), which is necessary to repair tissue damaged by the initial injury-induced inflammation. The dietary optimization of the Resolution Response can be personalized to the individual by using standard blood markers. Once each of those markers is in their appropriate ranges, activation of intracellular AMPK will be facilitated. Finally, we outline how the resulting activation of AMPK will affect a diverse number of other intercellular signaling systems leading to an extended healthspan.
There are two distinct phases to the body's response to any injury: the initiation of inflammation and its resolution. Although the molecular biology of the initiation of inflammation is well-understood, the detailed knowledge of the molecular biology of the resolution of inflammation remains an emerging field (1). Successful healing of any inflammation-induced injury requires the coordinated reduction of the initial acute inflammation, followed by resolution of any residual inflammation, and finally repairing the damaged tissue leading to a return to homeostasis. We term this complex process that leads to healing as the Resolution Response (2).
If the Resolution Response is not sufficiently robust to address the initial acute inflammation induced by an injury, there will be a build-up of chronic low-level unresolved inflammation. Although this type of resulting unresolved inflammation is below the perception of pain, it is also a major driving factor for developing a wide variety of age-related chronic diseases, including diabetes, cardiovascular disease, cancer, auto-immune and neurological conditions (2).
The Resolution Response is an evolutionarily conserved mechanism to protect the organism from unresolved injury-induced inflammation. Although microbial invasions will generate an inflammatory response, there are a far greater number of other potential causes of injury-induced inflammation, as shown in Table 1.
While there are diverse types of injuries that can induce an initial inflammatory response, the individual components of the Resolution Response that control the healing of the damage are governed by ancient and highly conserved mechanisms under robust dietary control.
The Resolution Response's molecular components that can be directly affected by the diet can be divided into two separate broad classes of signaling agents. One class of signaling agents is hormones consisting of eicosanoids and specialized pro-resolving mediators or SPMs. Eicosanoids would include prostaglandins and leukotrienes, whereas SPMs would include resolvins, protectins, and maresins. The other class of signaling agents is gene modulators. These gene modulators include NF-κB, the gene transcription factor central to the initiation of inflammation, and 5'-adenosine monophosphate-activated protein kinase (AMPK), the master switch of metabolism. AMPK reduces not only NF-κB activity but also is essential to repair damaged tissue.
A graphical depiction of these signaling agents is shown in Figure 1.
Figure 1. Illustration of the balancing of the signaling agents involved in the Resolution Response. AMPK, 5' adenosine monophosphate-activated protein kinase; NF-κB, Nuclear factor kappa-B; SPMs, Specialized pro-resolving meditators.
These signaling agents of the Resolution Response represent on-demand responses and must be continually balanced to maintain homeostasis. We use the image of a gyroscope to reflect this need for dynamic balance to maintain dynamic flexibility to switch from inflammation to resolution. Over activation or excessive inhibition of one particular signaling system in Figure 1 may have unintended consequences for the other linked systems. As described later, the ranges of specific blood markers can be altered by the diet to maintain these cellular signaling systems in equilibrium.
Furthermore, acute inflammation is only activated by injury, and it is the onset of inflammation that triggers the start of resolution. The initial acute inflammatory response is protective as it alerts the immune system to respond to the injury. However, if the initial inflammatory response is unresolved, this leads to chronic low-level inflammation. This unresolved inflammation results in tissue damage that transforms the otherwise protective initial inflammatory response associated with many chronic disease conditions. To successfully heal from any injury-induced inflammation, one must increase those diet-controlled nutrients or their metabolites to activate AMPK and enhance SPM formation to address unresolved inflammation. Simultaneously, one also has to decrease the intake of those diet-controlled nutrients that can promote excessive NF-κB activity and increased eicosanoid formation. Thus, rather than concentrating on any single dietary component of the Resolution Response, one must focus on the broader vision of maintaining all of the diet-controlled factors of the Resolution Response within appropriate operating ranges. Thus, the Resolution Response can be best understood from a dynamic systems-based biology viewpoint consisting of complex, interrelated systems necessary for successful healing.
It should be pointed out that the role of vitamins and minerals in the immune response is relatively minor (3, 4). The Resolution Response is dependent on how specific dietary factors are important in the generation of either signaling hormones (eicosanoids and SPMs) or the control of intracellular factors (NF-κB and AMPK) are far more critical in controlling the immune response.
The three distinct phases of the Resolution Response can be summarized as (a) reducing injury-induced inflammation, (b) resolving residual inflammation, and (c) repairing the tissue damage caused by injury-induced inflammation, as shown in Figure 2.
Figure 2. A graphic illustration of the sequential events for a successful Resolution Response to injury-induced inflammation. AMPK, 5' adenosine monophosphate-activated protein kinase; SPMs, Specialized pro-resolving meditators.
It should be noted that both acute inflammation and the Resolution Response are quiescence systems that are only activated by injuries that induce inflammation.
Acute inflammation is critical for proper immunological function. However, an equally robust Resolution Response must counter acute inflammation to prevent the build-up of unresolved inflammation that can lead to either fibrosis or cellular senescence, as shown in Figure 3.
Figure 3. A graphic illustration of the consequences of a blocked Resolution Response preventing healing of an injury-induced inflammation.
A highly effective way to reduce existing inflammation is following a highly defined anti-inflammatory diet. The problem is how to describe such a diet.
The most important consideration for any anti-inflammatory diet is calorie restriction. Any reduction of excess calorie intake will lead to a decrease in systemic oxidative stress. Calorie restriction has been the most successful therapeutic intervention to improve healthspan (defined as longevity minus years of disability) in virtually every species studied (5). Significant metabolic benefits have been achieved by calorie restriction in healthy overweight and normal-weight individuals who participated in the various CALERIE (Comprehensive Assessment of the Long-Term Effects of Reducing Intake of Energy) studies (6, 7).
Successful lifetime calorie restriction depends on the ability of such a diet to increase satiety. From this perspective, consuming adequate protein levels at each meal may represent a necessary first step. This concept is known as protein leveraging (8, 9). Potential protein leveraging mechanisms may include increasing glucagon levels to stabilize blood glucose levels in the blood (10) and the increased release of satiety hormones such as PYY and GLP-1 from the gut (11). In addition, the intake of fermentable fiber in a calorie-restricted diet is also essential for generating short-chain fatty acids (SCFA) that further enhance the signaling intensity of PYY and GLP-1 generated by the protein intake at a meal (12).
An anti-inflammatory diet should also substantially reduce the omega-6 fatty acid arachidonic acid (AA) levels in the plasma membrane. AA is the primary building block of eicosanoids. The vast majority of eicosanoids derived from AA are pro-inflammatory hormones that can significantly intensify any initial inflammatory response, making it more challenging to resolve the initial acute inflammation. However, a specific AA level in the plasma membrane is necessary to generate the eicosanoids required to create an acute inflammatory response. This process begins with the activation of phospholipase A2 that releases AA from the plasma membrane phospholipids. This free AA is immediately metabolized into eicosanoids. However, excess AA levels in the plasma membrane will cause increased amplification of the initial inflammatory response. Although reducing the dietary intake of AA is one possible way to achieve this goal, it should be emphasized that much of the AA in the body (and especially in the plasma membrane) comes from the metabolism of the linoleic acid into AA (13). The metabolic conversion of linoleic acid to AA is accelerated by elevated insulin levels generated either by a consistently high glycemic load of the diet or by existing insulin resistance. In either case, elevated insulin levels will activate the rate-limiting enzymes (delta-6-desaturase and delta-5-desaturase), leading to increased AA formation from dietary intake of linoleic acid (14).
On the other hand, the hormone glucagon, induced by the diet's protein content, will inhibit the same desaturase enzymes (14, 15). Thus, the balance of the protein-to-glycemic load of an anti-inflammatory diet is essential in reducing excess formation of AA that can result in excessive eicosanoid-driven inflammation (13). Thus, a primary requirement of an anti-inflammatory diet is to be low in linoleic acid, which will further decrease AA levels by the above-mentioned metabolic pathways.
The development of insulin resistance can also increase AA formation. Insulin resistance appears to be strongly influenced by cytokine levels (especially TNFα) induced by activation of NF-κB (16–18).
NF-κB is activated by high levels of saturated fatty acids (primarily palmitic acid) in the blood that can interact with the toll-like receptors TLR-2 and TLR-4. Activation of NF-κB results in the increased production of cytokines (19, 20). Thus, an additional dietary requirement for an anti-inflammatory diet is a low intake of saturated fats, especially palmitic acid.
Increased insulin resistance eventually leads to elevated blood glucose levels that cause beta-cell function in the pancreas to begin to fail (21). The combination of high blood glucose levels and oxidative stress generated by excess calorie intake can increase Advanced Glycosylated End products or AGE (22). These glycosylated proteins can interact with specific receptors (RAGE) on the cell surface, providing another diet-related pathway to increase cytokine production by activating NF-κB (23).
Just as it is necessary to reduce the dietary intake of omega-6 and saturated fatty acids, it is also essential to increase the omega-3 fatty acid intake, especially of the long-chain omega-3 fatty acids eicosapentaenoic acid (EPA) and docosahexaenoic acid (DHA). EPA is a feedback inhibitor of the delta-5-desaturase enzyme that is the rate-limiting step in the production of AA (24). Thus, the higher the levels of EPA in the diet, the less AA is generated.
Eicosanoids can be generated from EPA, but not DHA. The eicosanoids generated from the EPA are ~100–1,000 times lower in their inflammatory intensity than the same eicosanoids derived from AA (25). Thus, the eicosanoids derived from EPA are not strictly anti-inflammatory hormones, but they are far weaker pro-inflammatory hormones than those derived from AA. The net result is a reduction in the intensity of the inflammatory response.
Another reason for increasing the omega-3 levels in an anti-inflammatory diet is the reduction of inflammasome activation. Inflammasomes are intracellular structures formed in response to microbe-derived pathogen-associated molecular patterns (PAMPs) or danger-associated molecular patterns (DAMPs) sensed within the cell (26). Once activated, inflammasomes will cause the generation of pro-inflammatory cytokines such as IL-1β and IL-18 (27). The NLRP3 inflammasome is the most investigated of the various inflammasomes (28). Omega-3 fatty acids such as EPA and DHA can inhibit the activation of inflammasomes (29). However, it appears that DHA may be more effective than EPA in this regard (30).
Finally, an anti-inflammatory diet should reduce the inflammatory effects of metabolic endotoxemia by strengthening the mucosal barrier in the gut (31). Improvement in the integrity of the gut's tight junctions can result from the increased levels of the bacterium Akkermansia muciniphila. The population of this bacterium in the gut can be enhanced with an increased intake of fermentable fiber, omega-3 fatty acids, and polyphenols (32, 33).
The dietary foundation for reducing diet-induced inflammation would consist of the following nutritional composition: calorie restriction with adequate protein coupled with a moderate level of low glycemic-load carbohydrates to reduce excess glucose intake. Furthermore, the diet should be low in total fat (especially omega-6 and saturated fatty acids), yet with sufficient levels of fermentable fiber, omega-3 fatty acids, and polyphenols.
Reducing the inflammation caused by an injury is only the first step toward the ultimate healing of any tissue damage. The second obligatory step of the Resolution Response is resolving any residual inflammation (34). Unlike the variety of dietary interventions that help reduce inflammation, increasing resolution of any residual inflammation is purely a function of omega-3 fatty acids in the diet that are the building blocks to produce levels of adequate Specialized Pro-Resolving Mediators (SPMs). SPMs are hormones that control the resolution of residual inflammation. SPMs represent a diverse superfamily of hormones consisting of three primary subfamilies: resolvins, maresins, and protectins. These SPMs are biosynthesized from EPA, DHA, and docosapentaenoic acid (DPA) (35, 36).
SPMs are critical for several distinct stages of resolution, including (a) stopping neutrophil swarming to the injury site, (b) causing the transition of pro-inflammatory macrophages (M1) to pro-resolution macrophages (M2) to remove cell debris from the injury site, and (c) increasing efferocytosis to remove apoptotic cells (37). In addition, the production of SPMs may also be a critical factor in preventing the priming of the inflammasome (38, 39).
The levels of omega-3 fatty acids required to achieve these goals can be estimated by the ratio of leukotrienes to SPMs (40, 41). Unfortunately, such a determination requires highly sophisticated instrumentation. However, leukotrienes are derived from AA, and many SPMs (such as the E-series resolvins) are derived from EPA. Consequently, the ratio of AA/EPA in the blood can serve as an upstream surrogate marker to determine whether therapeutic levels of omega-3 fatty acids are present to generate sufficient levels of SPMs to complete the resolution phase of the Resolution Response.
The final and most complex phase of the Resolution Response is activating the master switch of metabolism, AMPK (2, 42). AMPK is a highly conserved energy sensor controlled by the balance of AMP and ATP levels in a cell. As the AMP/ATP ratio increases as occurs with calorie restriction, AMPK is activated, which sets in motion the phosphorylation of a broad cascade of gene transcription factors that switch metabolism from anabolic to catabolic to restore ATP levels (43–46).
Figure 4 indicates just a few of the many metabolic effects that take place once AMPK is activated.
Figure 4. Metabolic effects of AMPK activation. Green arrows on the “spokes” indicate activation, red lines with a bar at the end indicate inhibition. ACC 1 and ACC2, -Acetyl-CoA carboxylase 1 and 2; AMPK, 5' adenosine monophosphate-activated protein kinase; Glut, Glucose transporter protein; GS, Glycogen synthetase; mTOR, mammalian target of rapamycin; NF-κB, Nuclear factor kappa-B; NOS, Nitrogen oxide synthetase; PGC-1α, Peroxisome proliferator-activated receptor gamma coactivator 1-alpha; SREBP-1c, Sterol regulatory element-binding protein 1c.
While all these actions will significantly affect metabolism, perhaps the most critical benefit of AMPK activation is inhibiting NF-κB activity (47–49). The inhibition of NF-κB leads to a substantial reduction of both excess eicosanoid and cytokine levels. The activation of AMPK thus reduces inflammation induced by NF-κB activation. This metabolic control of NF-κB by AMPK is critical for the successful repair of damaged tissue.
This repair process starts with increased autophagy to supply the molecular building blocks for tissue repair and increased mitophagy to replace damaged mitochondria to provide the energy required for tissue repair (50). These processes are controlled by AMPK via activation of ULK-1, which is the first step to increase mitophagy (50).
A potential link between increased SPM formation and increased AMPK activity appears to be mediated by receptors for various SPMs. One well-characterized receptor is FPR2/ALX, a receptor for lipoxin A4 and the anti-inflammatory/pro-resolution protein annexin (51). FPR2/ALX is also a receptor for the Resolvin D1 (RvD1) derived from DHA (52). Thus, it is very likely that RvD1 and other SPMs, signaling through similar receptors, may also be instrumental for increased AMPK activation (53–55).
However, the most potent dietary effector of AMPK may be polyphenols. Polyphenols activate AMPK indirectly by binding to various sirtuins (SIRT) which are deacetylating enzymes dependent on NAD+ (56, 57). One of the multiple targets for SIRT is liver kinase B1 (LKB1). Once LKB1 is deacetylated, it activates AMPK, which inhibits NF-κB (58, 59). In addition, AMPK activates the rate-limiting enzyme (nicotinamide phosphoribosyltransferase or NAMPT) of the salvage pathway that regenerates NAD+, needed for the deacetylating activity of various SIRT proteins. This crosstalk between SIRT and AMPK creates a positive feedback loop for AMPK activation (60–64). This is shown in Figure 5.
Figure 5. Crosstalk between SIRT and AMPK. Any decrease in the cell's energy state measured by an increased AMP/ATP ratio will activate AMPK. This activation of AMPK leads to increasing NAMPT activity that produces NAD+ required for SIRT deacetylation activity. SIRT then deacetylates LKB1, which activates AMPK. AMPK, 5' adenosine monophosphate-activated protein kinase; LKB1, Liver kinase B1; NAMPT, Nicotinamide phosphoribosyltransferase; SIRT, Sirtuins.
The only problem with dietary polyphenols as AMPK activators is their limited water-solubility. However, specific subclasses of polyphenols such as anthocyanins (particularly delphinidins) have high water-solubility making it possible to obtain adequate blood levels to increase AMPK activity (65–69).
AMPK activity is under robust dietary control activated by calorie restriction, SPMs, and polyphenols. On the other hand, AMPK activity is inhibited by excess calorie intake and elevated blood glucose levels (70, 71). Thus, one can obtain the maximum dietary activation of AMPK activity by following a calorie-restricted diet with a low glycemic index with adequate omega-3 fatty acids and water-soluble polyphenols such as anthocyanins, especially bioavailable delphinidins (72, 73).
Optimizing the Resolution Response requires viewing dietary nutrients as signaling agents, as shown in Table 2.
Adequate protein intake plays a critical role in the long-term adherence of calorie restriction to activate AMPK. Sufficient protein levels at every meal are necessary to control satiety via protein leveraging (9, 74). Protein leveraging is based upon the hypothesis that the protein levels at each meal determine the level of appetite suppression of that meal. Without adequate appetite suppression between meals, long-term success for calorie restriction is highly unlikely. There are two potential mechanisms of protein leveraging. The first mechanism is to have adequate dietary protein at any meal to release sufficient levels of hormones such as PYY and GLP-1 from the small intestine that goes directly to the brain via the vagal nerve to reach the hypothalamus to reduce hunger (75, 76). Thus, the less protein consumed in a meal, the more likely additional calories will be needed to be consumed at that meal to cause sufficient appetite suppression by alternative pathways. The second mechanism is the increase in glucagon levels in the blood stimulated by dietary protein (10). Glucagon will release stored glycogen from the liver to maintain stable blood glucose levels, thereby reducing hunger. This outcome of improved hunger control is also suggested by a recent study demonstrating that the postprandial glycemic dip at 2–3 h predicted future appetite and energy intake (77).
Clinical data suggest that weight regain after controlled purposeful weight loss is reduced with a higher protein percentage in the diet. It appears that ~25% of the total calories as protein at each meal may be a threshold for this effect (10, 78). For a 400-calorie meal, this would mean consuming ~25 g of protein. However, the daily dietary protein required is based on the individual's lean body mass and physical activity (13). For the average female, this will be ~75 g of protein per day. For the average male, the protein level will be about 100 g of protein per day.
It should be noted that these protein intake levels for an anti-inflammatory diet are typical for the US population (79). Although these recommended daily protein levels are slightly higher than the generally recommended minimum daily protein intake, they are necessary because calorie restriction for an anti-inflammatory diet can reduce lean body mass (80). Furthermore, the total protein should be spread evenly throughout the day for improved hormonal control (13, 81).
Another nuance of protein leveraging is the timing of protein consumption during a meal. Eating protein before consuming carbohydrates generates more significant glycemic control in type 2 diabetic subjects than consuming carbohydrates first before protein (82, 83). One potential reason may be that protein requires a longer transit time in the small intestine to reach the L-cells that secrete PYY and GLP-1. These gut hormones stimulated by protein go directly via the vagal nerve to the brain's appetite center in the hypothalamus to generate satiety. This timing factor suggests that the blood levels of amino acids needed to stimulate glucagon will rise more slowly than glucose levels (especially high-glycemic load carbohydrates rich in glucose) required to stimulate insulin release. Thus, consuming protein first in a meal should generate a more favorable postprandial glucagon-to insulin balance after the meal. Clinical data suggests an improved balance of glucagon-to-insulin leads to a substantial reduction in consumed calories under ad libitum conditions after consuming two consecutive balanced meals compared to isocaloric meals with a lower protein-to-carbohydrate ratio (10).
Since excess dietary glucose is an inhibitor of AMPK, the glucose content in a meal and its entry rate into the bloodstream will be essential for optimizing the Resolution Response. The glycemic index measures the rate of entry of glucose in the blood of a defined amount of particular carbohydrate-containing food. However, a more relevant metabolic parameter is the glycemic load. The glycemic load considers how rapidly the total carbohydrate of a meal raises blood glucose levels (84). Meals consisting of a high glycemic load will inhibit AMPK activity. The highest glycemic load comes from having grains and starches as the primary carbohydrates in a meal. Therefore, for minimum inhibition of AMPK, the carbohydrate content of a meal should consist primarily of carbohydrates with a low glycemic impact. These carbohydrates would mainly consist of primarily non-starchy vegetables and limited amounts of fruits (mostly berries). Epidemiology studies have indicated that individuals consuming 10 servings per day of non-starchy vegetables and fruits have lower mortality and morbidity than those consuming fewer servings of these low glycemic impact carbohydrates (85). This epidemiological observation would be consistent with decreased inhibition of AMPK activity.
Total fat content should remain low for maintaining calorie restriction. However, one must also consider the composition of that total fat intake. The balance of omega-6 to omega-3 fatty acids should be no >2:1. A lower omega-6 to omega-3 fatty acid ratio will ensure a better balance of their bioactive biosynthetic products (eicosanoids coming from omega-6 fatty acids and SPMs from omega-3 fatty acids) help improve the resolution of any injury-induced inflammation. The omega-6 to omega-3 fatty acid balance in the United States was ~10:1 in 1999 (86, 87). The levels of saturated fats (principally palmitic acid) should also remain low because of their potential generation of inflammation in the hypothalamus (88). Increased hypothalamic inflammation can also potentially attenuate satiety signals from the gut and the blood (89). As discussed earlier, high levels of palmitic acid can interact with the TLR-2 and TLR-4 receptors to activate NF-κB to generate cytokines (19, 20). Thus, the bulk of the limited fat content for an anti-inflammatory diet should come from monounsaturated fatty acids.
The macronutrient balance of the diet at every meal can also further control hormonal responses. Ideally, the level of low-glycemic-load carbohydrates should be approximately one-third more than the protein content in a meal (13). Such a protein-to-carbohydrate balance is very similar to that estimated for a Paleolithic diet (90). Furthermore, this balance of protein-to-carbohydrate should be consistent for each meal. It is known from clinical experiments that the protein-to-carbohydrate ratio profoundly affects the blood's resulting insulin-to-glucagon balance under isocaloric conditions (10). This hormonal balance is essential since the delta-6 and delta-5 desaturase enzymes that convert the omega-6 fatty acid linoleic acid into arachidonic acid (AA) are activated by insulin (14). In contrast, glucagon inhibits these same enzymes, decreasing the potential excess formation of AA, thereby reducing possible excess eicosanoid formation (14, 15).
Finally, to maintain appropriate calorie restriction, the total fat content of the diet should be <50 g per day. Thus, the macronutrient composition of an anti-inflammatory diet should be ~1 g of fat for every 2 g of protein and every 3 g of carbohydrate at every meal. This total macronutrient balance is also consistent with estimates of Paleolithic diets (90). Placebo-controlled trials using calorie-restricted diets have demonstrated that the macronutrient balance described above appears to reduce inflammation (91–93) significantly. These differences in the reduction of inflammation based on the protein-to-carbohydrate ratio may be related to the change in the balance of insulin and glucagon observed in different protein-to-carbohydrate ratios seen in cross-over diet studies (10). Changes in the insulin-glucagon balance can affect the activity of various desaturases are critical for preventing excess arachidonic acid production (14). These initial results suggest that the protein-to-carbohydrate balance of a calorie-restricted diet may significantly improve its anti-inflammatory effects.
Gut bacteria require the dietary intake of fermentable fiber to generate short-chain fatty acids (SCFA). SCFA act as signaling agents to maintain the gut barrier's integrity by decreasing inflammation in the gut wall (94, 95).
Improving the integrity of the gut wall will reduce metabolic endotoxemia as a significant source of gut-induced inflammation (96). SCFA produced in the gut can also significantly affect neurological function via the vagus nerve (97). Finally, SCFA have a significant role in maintaining satiety by enhancing the secretion of PYY and GLP-1 from the gut (98, 99).
Polyphenols constitute a large group of more than 8,000 compounds that can be potentially metabolized into less complex phenolic compounds that can also act as signaling agents (100). It is challenging to measure polyphenols in the blood. However, the metabolites of those polyphenols that are absorbed can be found in the urine. Studies have suggested that the increased levels of polyphenols in the urine are associated with reduced frailty and mortality compared to the estimated dietary intake of polyphenols from food diaries (101, 102). Furthermore, only specific polyphenol structures appear maximally active as allosteric agents to increase the sirtuins' deacetylating activity, as described earlier. The polyphenols with the most significant potential to cause an allosteric activation of sirtuins appear to require an intact flavonoid structure and a net positive charge to enhance their water solubility (57). The subgroup of polyphenols that has both structural characteristics is anthocyanins. Berries are a rich source of anthocyanins. It should be noted that increased anthocyanin intake is associated with decreased incidence of cardiovascular disease, although the effect appears to be stronger in women than men (103, 104).
Compared to the above-mentioned dietary factors, the role of vitamins and minerals will have a relatively minor effect on inflammation and resolution and the immune response (3, 4). Furthermore, it has been calculated that a calorie-restricted anti-inflammatory diet described earlier is rich in non-starchy vegetables and limited amounts of fruits and would supply adequate levels of vitamins and minerals (105).
The ultimate approach to healing is to optimize the Resolution Response. Success requires reducing, resolving, and repairing inflammatory damage caused by any injury. This goal can be achieved by reducing the stresses on lipid, glycemic, and inflammatory responses. These various metabolic responses are under significant dietary control. Therefore, the more the stress levels of each of these three metabolic responses are maintained within appropriate ranges, the greater the degree to which the Resolution Response becomes optimized.
Successful dietary management of the Resolution Response can be determined by the extent to which each of the clinical markers of metabolic stress is maintained within their desired ranges. Such blood markers must be highly validated, easily obtained, and provide clear guidelines for personalizing the individual's diet.
The three clinical markers that meet these criteria are the following:
(a) Reducing lipid stress: A primary factor is causing lipid stress is insulin resistance. Therefore, the TG/HDL ratio (measured in mg/dL) should be <1 for controlling insulin resistance (106–113).
(b) Reducing inflammatory stress: Inflammatory stress is caused by an imbalance in the production of eicosanoids and SPMs. The AA/EPA ratio should be maintained between 1.5 and 3 to maintain an appropriate ratio of precursors for a balanced formation of both eicosanoids and SPMs. A significant reduction of various cytokines is observed when this range of the AA/EPA ratio is achieved by appropriate supplementation with omega-3 fatty acids (114–116). The average AA/EPA ratio in the US is >20 (117, 118), indicating the existence of an unfavorable balance of eicosanoids to SPMs.
(c) Reducing glycemic stress: The HbA1c level should be maintained between 4.9 and 5.1 percent, indicating the lack of glucose inhibition of AMPK activity (70).
The recommended appropriate ranges for these markers are lower than the typical average levels for healthy individuals, but they are still within normal ranges. Therefore, only when all three of these three clinical markers are in their appropriate ranges can the Resolution Response be considered to be optimized for an individual.
Furthermore, each of the markers can be modulated by specific dietary interventions. For example, the level of insulin resistance can be significantly reduced by following an anti-inflammatory diet. In particular, the dietary intake of omega-3 fatty acids strongly influences the AA/EPA ratio. Finally, the dietary intake of polyphenols to activate AMPK will strongly affect the HbA1c levels. It should be noted that AMPK can also be activated by increased SPM synthesis and calorie restriction that is the foundation for an anti-inflammatory diet. Thus, there is significant crosstalk of the various dietary components as discussed above.
In principle, an appropriate anti-inflammatory diet should be sufficient to optimize the Resolution Response. However, the diet will often require supplementation with omega-3 fatty acids and polyphenols to reach the target ranges of the clinical markers described above. This need for potential supplementation is because the levels of omega-3 fatty acids in the blood required to resolve the residual inflammation caused by the injury sufficiently are often beyond the intake provided by the best anti-inflammatory diet. This need for supplementation is also the case for the amounts of polyphenols needed to activate AMPK to repair damaged tissue.
In particular, supplementation with EPA and DHA concentrates may be required to increase the biosynthesis of the SPMs needed to resolve residual inflammation and reduce inflammasome formation (29, 38, 39, 119–121). The best sources of polyphenols to enhance AMPK activity will come from polyphenol concentrates of the anthocyanin family. These polyphenols can enhance the allosteric activation of sirtuins to indirectly activate AMPK (55) and inhibit inflammasome formation (122, 123). Such polyphenol concentrates should also be devoid of glucose that would otherwise impede the activation of AMPK.
The recommended dosage of the above supplements can be obtained from the selected clinical trials. It has been demonstrated that a dose of 2.5 g of EPA and DHA per day for 8 weeks can significantly reduce cytokines levels in elderly obese individuals compared to a placebo group (115). The REDUCE-IT study used 3.9 g of EPA over an average of 4.9-years demonstrated a significantly reduced number of CHD events in subjects with elevated triglycerides and already taking statins compared to the placebo group (124). In patients with coronary artery disease, a daily dose of 3.4 g of EPA and DHA per day for year generated SPMs in the active group, but not the placebo group (125). Thus, it would be recommended that an initial starting supplemental dose of omega-3 fatty acids would be between 2.5 and 4 g per day. The precise level of omega-3 supplementation can be determined from the level required to reduce the AA/EPA ratio in the blood to between 1.5 and 3.
The levels of polyphenols, and in particular anthocyanins, are estimated to be at least −150 to 500 mg per day. An open study using 180 mg of anthocyanins per day demonstrated a statistically significant decrease in the HgA1c levels in prediabetics within 4 weeks that continued to decrease at 12 weeks (126). A placebo-controlled study using 450 mg per day of anthocyanin supplementation produced a statistically significant decrease in oxidative stress after 30 days of supplementation in the smokers in the active group compared to the control group of smokers as measured by decreased isoprostane levels. After anthocyanin supplementation was stopped, the level of isoprostanes in the active group returned to their original levels after 40 days (127).
Reaching the target HbA1c ranges determines the amount of optimal level of polyphenol supplementation that may be required. Since individuals are not genetically identical, the levels of potential supplementation are personalized by the blood markers already described.
While any one of the three dietary interventions is helpful for partial activation of AMPK, we contend that the combination of all three nutritional interventions will be needed to have a significant and sustained effect on AMPK activation for meaningful therapeutic results.
This need for multiple dietary interventions for maximum activation of AMPK is shown in Figure 6.
The concept of insulin resistance has been used for more than 80 years (128). Its relationship to a larger group of chronic conditions began to be more recognized by the work of Gerald Reaven (129). However, it still is not clear exactly what causes insulin resistance (130, 131). However, it is known that insulin resistance is also associated with chronic low-level inflammation (132–135). Furthermore, the clinical marker of insulin resistance is hyperinsulinemia. Exactly how hyperinsulinemia causes the wide variety of metabolic disturbances associated with insulin resistance is still open to question (130, 131).
An alternative hypothesis can be formulated that a deficiency of AMPK activity may be the central factor in developing metabolic disturbances within the cell that are associated with insulin resistance. For example, it is known that insulin resistance is strongly associated with reduced AMPK activity (44, 136–138). Consequently, metabolic disorders such as obesity, metabolic syndrome, type 2 diabetes, and non-alcoholic fatty liver disease (NAFLD) strongly associated with insulin resistance have been directly linked to decreased AMPK activity (43, 139, 140). Furthermore, other chronic conditions related to increased insulin resistance include hypertension (141), cardiovascular disease (142), polycystic ovary syndrome (143), chronic kidney disease (144), various types of cancer (145), depression (146), and neurodegenerative diseases such as Alzheimer's and Parkinson's (147). These diverse chronic conditions are also considered pro-inflammatory conditions related to unresolved inflammation caused by blocked Resolution Response leading to deficiency of AMPK activity in target cells. Therefore, maintaining a constant dietary optimization of the Resolution Response may offer a fundamental central dietary approach for potentially managing those many chronic conditions associated with insulin resistance, as shown in Figure 7.
Figure 7. Potential linkage of the Resolution Response to insulin resistance and related chronic disease conditions.
From this perspective, one could potentially redefine insulin resistance as a deficiency of AMPK activation caused by a blocked Resolution Response. It should be noted that the most successful group of drugs for treating insulin resistance have been thiazolidinediones (TZDs).
One hypothesized mode of action of this class of drugs is the activation of AMPK (148–150). Therefore, we feel that optimization of the Resolution Response by the appropriate dietary interventions to increase the activity of AMPK within the cell may potentially have a significant therapeutic impact on each of the chronic conditions associated with insulin resistance without the past toxicity problems associated with the use of TZDs (151).
Systems-based biology takes into account the interconnected signaling pathways within the cell that are required to maintain homeostasis. Because of these complex relationships, pharmaceutical intervention in one pathway may adversely affect other intracellular pathways. Our working hypothesis is that dietary optimization of the Resolution Response will result in the activation of AMPK that can more effectively coordinate these pathways. Although direct measurement of AMPK activity in any tissue is complex because it requires a biopsy, one can use the clinical blood markers described earlier that define optimization of the Resolution Response as surrogate markers for the maintenance of intracellular AMPK activity. How AMPK activity is intimately connected to many of these diverse intracellular signaling pathways is shown in Figure 8.
Figure 8. The potential effect of dietary optimization of the Resolution Response on various regulatory proteins and gene transcription factors. AMPK, 5' adenosine monophosphate-activated protein kinase; FOXO, Forkhead box transcription factors class O; mTOR, mammalian target of rapamycin; NF-κB, Nuclear factor kappa-B; PI3K/AKT, Phosphatidylinositol 3-kinase/AKT (protein kinase B).
As shown in Figure 8, there is significant cross-signaling between these various metabolic systems within the cell and potential inhibition or activation of one system by another. In some cases, there is mutual activation, such as between AMPK and SIRT or between PI3K/AKT, mTOR, and NF-κB. In other cases, there can be reciprocal inhibition between systems, such as between PI3K/AKT and AMPK. Finally, there can also be unidirectional inhibition or activation between various signaling pathways.
AMPK may represent the molecular link between these diverse signaling systems and the diet. This control is possible since AMPK is an evolutionarily conserved energy sensor that controls metabolism. In essence, AMPK becomes the checkpoint for metabolic control that links diet to these various intracellular signaling systems. In particular, activation of AMPK will inhibit the activity of NF-κB, thereby reducing the generation of pro-inflammatory mediators such as cytokines and eicosanoids (48), but also inhibit other intracellular systems such as mTOR and the PI3K/AKT that can activate NF-κB (152). In addition, the repair of damaged tissue also requires increased AMPK activity for both the repair of damaged tissue and the prevention of fibrosis (153, 154).
However, one can only routinely monitor the blood, not the interior of the cell. This is why constant monitoring of the blood markers that define the Resolution Response provides an easily obtained insight into AMPK activity. Thus, continuous dietary optimization of the Resolution Response results in the dietary control intracellular AMPK activity. In doing so, it may be possible to maintain these other internal cellular signaling pathways within their optimal operating parameters. The successful result leads to improved metabolic efficacy generating a potentially extended healthspan.
On the other hand, any reduction in AMPK activity leads to the over-expression of pro-inflammatory signaling systems shown in Figure 8. One of the linked systems that would increase with a decrease in AMPK activity would include NF-κB. Increased NF-κB activity is related to increased inflammatory activity associated with cardiovascular disease (155) and cancer (156). Likewise, reduced activity of AMPK would lead to potentially excessive activity of mTOR and the PI3K/AKT signaling pathways associated with cancer (157, 158).
How the activity of AMPK acts as the central hub linking various other cellular signaling systems at the molecular level is described in more detail in the following summaries.
One of the primary benefits of activating AMPK is the inhibition of NF-κB, resulting in the reduction of cytokine and eicosanoid formation. The lowering of inflammation is achieved through several different routes orchestrated by AMPK (48). One pathway is inhibiting NF-κB by the direct activation of AMPK (159). Another route is through the activation of SIRT1 by increasing NAD+ levels (63). Finally, AMPK activates the rate-limiting enzyme in the NAD+ salvage pathway that provides the necessary NAD+ to enable SIRT1 to deacetylate the Rel/p65 component of NF-κB to prevent its binding to the cell's DNA that is required to express inflammatory mediators (160).
Additional AMPK-mediated pathways that inhibit NF-κB activity include the activation of PGC-1α (161) and the phosphorylation of FOXO (49).
Activation of AMPK is the primary inhibitor of mTOR. At the molecular level, mTOR inhibition is due to phosphorylation of the raptor component of mTORC1 and TSC2 (162). In addition, the association of increased SIRT1 activity with the inhibition of mTOR (163) can be induced by the AMPK's activation of the rate-eliminating enzyme of the NAD+ salvage pathway (161). On the other hand, any increase in AKT activity will up-regulate mTOR, which activates NF-κB (164).
The PI3K/AKT pathway is activated by insulin and results in cellular growth activation (165). If the PI3K/AKT pathway is too active, this will inhibit the activity of AMPK (166, 167). On the other hand, any increase in AMPK activation will inhibit AKT activity (166, 167).
The inhibition of AMPK and FOXO activity by AKT can be reduced by optimization of the Resolution Response. One way in which PI3K activity can be reduced by lowering blood insulin levels following an anti-inflammatory diet. The reduction of PI3K activity leads to decreased activation of AKT. Long-term studies using the previously described anti-inflammatory diet have demonstrated success in the long-term management of type 2 diabetes (168, 169).
The FOXO family of gene transcription factors consisting of FOXO1, FOXO3, FOXO4, and FOXO6. The FOXO family is vital in controlling cellular senescence, stem cell maintenance, and lifespan in animal models (170). FOXO upregulation can be achieved either by phosphorylation via AMPK or deacetylation by SIRT (171, 172). In addition to the direct effect of AMPK activation on FOXO, any increase in AMPK activity will increase the activity of the rate-limiting enzyme (NAMPT) in the synthesis of NAD+, thereby activating SIRT, which also increases FOXO activity (173).
An indirect route to activate FOXO is via the AMPK-induced inhibition of AKT (171). On the other hand, any up-regulation of AKT by a deficit in AMPK activity will reduce FOXO activity (174–176). This central role of AMPK in FOXO activation may explain why activation of AMPK has been hypothesized to control the aging process (177).
Another inflammatory pathway that can be modulated by AMPK is JAK-STAT, which mediates cytokine signaling (178).
Considering the complexity of these interactions with cellular signaling mechanisms in the cell, optimizing the Resolution Response may have a far greater potential to bring a cell back to homeostasis than any potential drug therapy proposed for healthspan extension (179). If so, then the continuous optimization of the Resolution Response by the diet may play an essential role in extending the human healthspan.
Our working hypothesis is that gaining control of the complex systems biology that occurs inside a cell will require consistent maintenance of AMPK within defined operating parameters to respond to constantly changing metabolic needs. Furthermore, we feel no single dietary intervention will provide that necessary control of AMPK to be used as a therapeutic intervention to treat the various chronic conditions associated with insulin resistance. While there is suggestive evidence that each dietary component of the Resolution Response (an anti-inflammatory calorie-restricted diet, adequate intake of omega-3 fatty acids and polyphenols) can have some health benefits in humans, we believe that their synergistic interactions will result in far more significant clinical benefits potentially mediated by their synergistic interactions on AMPK activity.
One major limitation of our hypothesis is the difficulty of measuring AMPK activity in humans as it requires a tissue biopsy. On the other hand, we feel the dietary optimization of the Resolution Response using defined blood markers can be titrated to their appropriate ranges results allows the development of the necessary clinical trials combining all three dietary interventions being applied simultaneously can be undertaken today.
Another limitation is the potential genetic variations between individuals. However, this can be overcome by titration of the dietary components of the Resolution Response to reach the appropriate target ranges in the blood. In essence, one would be undertaking an “AMPK” clamp to determine if the clinical condition being studied responds with equal, if not superior, clinical results to this type of dietary therapy compared to standard drug therapy.
To answer the question of the widespread clinical utility of our hypothesis requires doing clinical trials. Since one is using dietary interventions, clinical trials to optimize the Resolution Response bypass the need for animal models. As demonstrated by the CALERIE studies, calorie restriction can be maintained for an extended period of time (6, 7). Using omega-3 fatty acids and polyphenols at levels that have Generally Regarded as Safe (GRAS) status set the upper limits for their supplementation to a calorie-restricted anti-inflammatory diet with a defined protein-carbohydrate ratio. The most likely chronic conditions that would be applicable to such immediate clinical trials are those associated with insulin resistance with defined clinical endpoints such as metabolic syndrome, type 2 diabetes, and non-alcoholic fatty liver disease. If successful, then the same dietary technology should be applicable to other chronic diseases associated with insulin resistance.
We hypothesize that the ability to heal from any injury-induced inflammation depends significantly on the dietary control of the body's internal Resolution Response. The final result of the optimization of the Resolution Response is the activation of AMPK. This hypothesis is based on the ability of AMPK to modulate internal cellular signaling through systems-based biology. While there is no single specific nutrient to optimize the body's internal capacity to heal from injury-induced inflammation, an appropriate combination of dietary interventions can alter signaling pathways that can lead to the molecular goal of increasing AMPK activity. This concept of requiring a defined combination of multiple dietary interventions to achieve the appropriate activation of AMPK is no different from using various combinations of discrete chemotherapeutic drugs to treat cancer. However, unlike numerous combination drug therapies used for cancer treatment, each dietary intervention described earlier can be easily modulated using the clinical markers that define the boundaries that optimize the Resolution Response for dietary guidance. Thus, the use of blood markers becomes the foundation for precision nutrition.
In conclusion, we believe understanding the complex interaction of highly defined dietary interventions that result in the optimization of the Resolution Response can provide a new appreciation of a new comprehensive nutritional strategy to treat many chronic conditions, especially those associated with insulin resistance. Furthermore, the dietary approach we have outlined can be optimized on an individual basis using validated blood markers to orchestrate a wide variety of internal cellular signaling systems. By using such blood markers to titrate each dietary component of the Resolution Response to their appropriate ranges moves precision nutrition into the realm of personalized medicine. Reaching and maintaining the appropriate ranges of those clinical markers that define the optimization of the Resolution Response may potentially be translated into an increased healthspan using diet as if it were a drug that controls the complex systems-based biology of healing.
BS and AS wrote the paper. All authors contributed to the article and approved the submitted version.
This study was supported by the Inflammation Research Foundation, Peabody, MA, USA.
BS and AS are employed by Zone Labs, Inc., a medical foods company.
All claims expressed in this article are solely those of the authors and do not necessarily represent those of their affiliated organizations, or those of the publisher, the editors and the reviewers. Any product that may be evaluated in this article, or claim that may be made by its manufacturer, is not guaranteed or endorsed by the publisher.
1. Serhan CN. Pro-resolving lipid mediators are leads for resolution physiology. Nature. (2014) 510:92–101. doi: 10.1038/nature13479
2. Sears B, Perry M, Saha AK. Dietary technologies to optimize healing from injury-induced inflammation. Antiinflamm Antiallergy Agents Med Chem. (2021) 20:123–31. doi: 10.2174/1871523019666200512114210
3. McMillan DC, Maguire D, Talwar D. Relationship between nutritional status and the systemic inflammatory response: micronutrients. Proc Nutr Soc. (2019) 78:56–67. doi: 10.1017/S0029665118002501
4. Fantacone ML, Lowry MB, Uesugi SL, Michels AJ, Choi J, Leonard SW, et al. The effect of a multivitamin and mineral supplement on immune function in healthy older adults: a double-blind, randomized, controlled trial. Nutrients. (2020) 2020:122447. doi: 10.3390/nu12082447
5. Most J, Tosti V, Redman LM, Fontana L. Calorie restriction in humans: an update. Age Res Rev. (2017) 39:36–45. doi: 10.1016/j.arr.2016.08.005
6. Martin CK, Das SK, Lindblad L, Racette SB, McCrory MA, Weiss EP. Effect of calorie restriction on the free-living physical activity levels of non-obese humans: results of three randomized trials. J Appl Physiol. (2011) 110:956–63. doi: 10.1152/japplphysiol.00846.2009
7. Das SK, Roberts SB, Bhapkar MV, Villareal DT, Fontana L, Martin CK. CALERIE-2 Study Group Body-composition changes in the Comprehensive Assessment of Long-term Effects of Reducing Intake of Energy (CALERIE)-2 study: a 2-y randomized controlled trial of calorie restriction in nonobese humans. Am J Clin Nutr. (2017) 105:913–27. doi: 10.3945/ajcn.116.137232
8. Simpson SJ, Raubenheimer D. Obesity: the protein leverage hypothesis. Obesity Rev. (2005) 6:133–42. doi: 10.1111/j.1467-789X.2005.00178.x
9. Gosby AK, Conigrave AD, Raubenheimer D, Simpson SJ. Protein leverage and energy intake. Obesity Rev. (2014) 15:183–91. doi: 10.1111/obr.12131
10. Ludwig DS, Majzoub JA, Al-Zahrani A, Dallal GE, Blanco I, Roberts SB. High glycemic index foods, overeating, and obesity. Pediatrics. (1999) 103:E26. doi: 10.1542/peds.103.3.e26
11. Klaauw vAA, Keogh JM, Henning E. High protein intake stimulates postprandial GLP1 and PYY release. Obesity. (2013) 21:1602–07. doi: 10.1002/oby.20154
12. Chambers ES, Morrison DJ, Frost G. Control of appetite and energy intake by SCFA: what are the potential underlying mechanisms? Proc Nutr Soc. (2015) 74:328–36. doi: 10.1017/S0029665114001657
14. Brenner RR. Hormonal modulation of delta-6 and delta-5 desaturases: case of diabetes. Prosta Leukortiene Essen Fatty Acids. (2003) 68:151–62. doi: 10.1016/S0952-3278(02)00265-X
15. Christophersen BO, Hagve TA, Norseth A. Studies on the regulation of arachidonic acid synthesis in isolated rat liver cells. Biochim Biophys Acta. (1982) 712:305–14. doi: 10.1016/0005-2760(82)90348-4
16. Hotamisligil GS, Shargill NS, Spiegelman BM. Adipose expression of tumor necrosis factor-alpha: direct role in obesity-linked insulin resistance. Science. (1993) 259:87–91. doi: 10.1126/science.7678183
17. Borst SE. The role of TNF-alpha in insulin resistance. Endocrin. (2004) 23:177–82. doi: 10.1385/ENDO:23:2-3:177
18. Akash MSH, Rehman K, Liaqat A. Tumor necrosis factor-alpha: role in development of insulin resistance and pathogenesis of type 2 diabetes mellitus. J Cell Biochem. (2018) 119:105–10. doi: 10.1002/jcb.26174
19. Hwang DH, Kim JA, Lee JY. Mechanisms for the activation of Toll-like receptor 2/4 by saturated fatty acids and inhibition by docosahexaenoic acid. Eur J Pharma. (2016) 785:24–35. doi: 10.1016/j.ejphar.2016.04.024
20. Schaeffler A, Gross P, Buettner R, Bollheimer C, Buechler C, Neumeier M, et al. Fatty acid-induced induction of Toll-like receptor-4/nuclear factor-kappaB pathway in adipocytes links nutritional signaling with innate immunity. Immunology. (2009) 126:233–45. doi: 10.1111/j.1365-2567.2008.02892.x
21. Taylor R, Al-Mrabeh A, Sattar N. Understanding the mechanisms of reversal of type 2 diabetes. Lancet Diab Endocrin. (2019) 9:726–36. doi: 10.1016/S2213-8587(19)30076-2
22. Moldogazieva NT, Mokhosoev IM, Melnikova TI, Porozov YB, Terentiev AA. Oxidative stress and advanced lipo-oxidation and glycation end products (ALEs and AGEs) in aging and age-related diseases. Oxid Med Cell Longev. (2019) 2019:3085756. doi: 10.1155/2019/3085756
23. Ott C, Jacobs K, Haucke E, Navarrete Santos A, Grune T, Simm A. Role of advanced glycation end products in cellular signaling. Redox Biol. (2014) 2:411–29. doi: 10.1016/j.redox.2013.12.016
24. Dias VC, Parsons HG. Modulation in delta 9, delta 6, and delta 5 fatty acid desaturase activity in the human intestinal CaCo-2 cell line. J Lipid Res. (1995) 36:552–63. doi: 10.1016/S0022-2275(20)39889-8
25. Calder PC. Omega-3 fatty acids and inflammatory processes: from molecules to man. Biochem Soc Trans. (2017) 45:1105–15. doi: 10.1042/BST20160474
26. Guo H, Callaway JB, Ting J P-Y. Inflammasomes: mechanisms of action, role in disease, and therapeutics. Nat Med. (2015) 21:677–87. doi: 10.1038/nm.3893
27. Man SM, Kanneganti TD. Regulation of inflammasome activation. Immun Rev. (2015) 625:6–21. doi: 10.1111/imr.12296
28. Sutterwala FS, Haasken S, Cassel SL. Mechanism of NLRP3 inflammasome activation. Ann NY Acad Sci. (2014) 1319:82–95. doi: 10.1111/nyas.12458
29. Yan Y, Jiang W, Spinetti T. Omega-3 fatty acids prevent inflammation and metabolic disorder through inhibition of NLRP3 inflammasome activation. Immunity. (2013) 38:1154–63. doi: 10.1016/j.immuni.2013.05.015
30. Lee KR, Midgette Y, Shah R. Fish oil derived omega 3 fatty acids suppress adipose NLRP3 inflammasome signaling in human obesity. J Endocrin Soc. (2019) 3:504–15. doi: 10.1210/js.2018-00220
31. Cani PD, Amar J, Iglesias MA, Poggi M, Knauf C, Bastelica D, et al. Metabolic endotoxemia initiates obesity and insulin resistance. Diabetes. (2007) 56:1761–72. doi: 10.2337/db06-1491
32. Roopchand DE, Carmody RN, Kuhn P, Moskal K, Rojas-Silva P, Turnbaugh PJ, et al. Dietary polyphenols promote growth of the gut bacterium Akkermansia muciniphila and attenuate high-fat diet-induced metabolic syndrome. Diabetes. (2015) 64:2847–58. doi: 10.2337/db14-1916
33. Kaliannan K, Wang B, Li XY, Kim KJ, Kang JX. A host-microbiome interaction mediates the opposing effects of omega-6 and omega-3 fatty acids on metabolic endotoxemia. Sci Report. (2015) 5:11276. doi: 10.1038/srep11276
34. Loftus RM, Finlay DK. Immunometabolism: cellular metabolism turns immune regulator. J Biol Chem. (2016) 291:1–10. doi: 10.1074/jbc.R115.693903
35. Serhan CN, Hong S, Gronert K, Colgan SP, Devchand PR, Mirick G, et al. Resolvins: a family of bioactive products of omega-3 fatty acid transformation circuits initiated by aspirin treatment that counter proinflammation signals. J Exp Med. (2002) 196:1025–37.
36. Serhan CN, Levy BD. Resolvins in inflammation: emergence of the pro-resolving superfamily of mediators. J Clin Invest. (2018) 128:2657–69. doi: 10.1172/JCI97943
37. Dalli J, Serhan CN. Pro-resolving mediators in regulating and conferring macrophage function. Front Immun. (2017) 8:1400. doi: 10.3389/fimmu.2017.01400
38. Yin Y, Chen F, Wang W, Wang H, Zhang X. Resolvin D1 inhibits inflammatory response in STZ-induced diabetic retinopathy rats: possible involvement of NLRP3 inflammasome and NF-kappaB signaling pathway. Mol Vision. (2017) 23:242–50.
39. Lopategi A, Flores-Costa R, Rius B, Lopez-Vicario C, Alcaraz-Quiles J, Titos E, et al. Specialized pro-resolving lipid mediators inhibit the priming and activation of the macrophage NLRP3 inflammasome. J Leuk Biol. (2019) 105:25–36. doi: 10.1002/JLB.3HI0517-206RR
40. Fredman G. Hellmann, J, Proto JD, Kuriakose G, Colas, RA, Dorweiler B, et al. An imbalance between specialized pro-resolving lipid mediators and pro-inflammatory leukotrienes promotes instability of atherosclerotic plaques. Nat Commun. (2016) 7:12859. doi: 10.1038/ncomms12859
41. Rymut N, Heinz J, Sadhu S, Hosseini Z, Riley CO, Marinello M. Resolvin D1 promotes efferocytosis in aging by limiting senescent cell-induced MerTK cleavage. Fed Am Soc Exp Biol J. (2020) 34:597–609. doi: 10.1096/fj.201902126R
43. Jeon SM. Regulation and function of AMPK in physiology and diseases. Exp Mol Med. (2016) 48:e245. doi: 10.1038/emm.2016.81
44. Day EA, Ford RJ, Steinberg GR. AMPK as a therapeutic target for treating metabolic diseases. Trend Endocrin Metabol. (2017) 28:545–60. doi: 10.1016/j.tem.2017.05.004
45. Hardie DG. Keeping the home fires burning: AMP-activated protein kinase. J Royal Soc Interface. (2018) 138:20170774. doi: 10.1098/rsif.2017.0774
46. Herzig S, Shaw RJ. AMPK: Guardian of metabolism and mitochondrial homeostasis. Nat Rev Mol Cel Biol. (2018) 19:121–35. doi: 10.1038/nrm.2017.95
47. Chen J, Zhu Y, Zhang Y, Peng X, Zhou X, Li F. Delphinidin induced protective autophagy via mTOR pathway suppression and AMPK pathway activation in HER-2 positive breast cancer cells. BMC Cancer. (2018) 18:342. doi: 10.1186/s12885-018-4231-y
48. Salminen A, Hyttinen JMT, Kaarniranta K. AMP-activated protein kinase inhibits NF-κB signaling and inflammation: impact on healthspan and lifespan. J Mol Med. (2011) 89:667–76. doi: 10.1007/s00109-011-0748-0
49. DAcquisto FD May MJ, Ghosh S. Inhibition of nuclear factor kappa B (NF-κB): an emerging theme in anti-inflammatory therapies. Mol Interv. (2002) 2:22–35. doi: 10.1124/mi.2.1.22
50. Li Y, Chen Y. AMPK and autophagy. Advan Exp Med Biol. (2019) 1206:85–108. doi: 10.1007/978-981-15-0602-4_4
51. McArthur S, Juban G, Gobbetti T, Desgeorges T, Theret M, Gondin J, et al. Annexin A1 drives macrophage skewing to accelerate muscle regeneration through AMPK activation. J Clin Invest. (2020) 130:1156–67. doi: 10.1172/JCI124635
52. Norling LV, Dalli J, Flower RJ, Serhan CN, Perretti M. Resolvin D1 limits polymorphonuclear leukocyte recruitment to inflammatory loci: receptor-dependent actions. Arterioscler Thromb Vas Biol. (2012) 32:1970–78. doi: 10.1161/ATVBAHA.112.249508
53. Park J, Langmead CJ, Riddy DM. New advances in targeting the resolution of inflammation: implications for specialized pro-resolving mediator GPCR drug discovery. ACS Pharm Transl Sci. (2020) 3:88–106. doi: 10.1021/acsptsci.9b00075
54. Hellmann J, Tang Y, Kosuri M, Bhatnagar A, Spite M. Resolvin D1 decreases adipose tissue macrophage accumulation and improves insulin sensitivity in obese-diabetic mice. Fed Am Soc Exp Biol J. (2011) 25:2399–407. doi: 10.1096/fj.10-178657
55. Hosseini Z, Marinello M, Decker C, Sansbury BE, Sadhu S, Gerlach BD, et al. Resolvin D1 enhances necroptotic cell clearance through promoting macrophage fatty acid oxidation and oxidative phosphorylation. Arterioscler Thromb Vasc Biol. (2021) 41:1062–75. doi: 10.1161/ATVBAHA.120.315758
56. Chung S, Yao H, Caito S, Hwang JW, Arunachalam G, Rahman I. Regulation of SIRT1 in cellular functions: role of polyphenols. Arch Biochem Biophys. (2010) 501:79–90. doi: 10.1016/j.abb.2010.05.003
57. Rahnasto-Rilla M, Tyni J. Huovinen, M, Jarho E, Kulikowicz T, Ravichandran SA, et al. Natural polyphenols as sirtuin 6 modulators. Sci Rep. (2018) 8:4163. doi: 10.1038/s41598-018-22388-5
58. Shackelford DB, Shaw RJ. The LKB1-AMPK pathway: metabolism and growth control in tumour suppression. Nat Rev Cancer. (2009) 9:63–75. doi: 10.1038/nrc2676
59. Alexander A, Walker CL. The role of LKB1 and AMPK in cellular responses to stress and damage. FEBS Lett. (2011) 585:952–57. doi: 10.1016/j.febslet.2011.03.010
60. Liu TF, Brown CM, El Gazzar M, McPhail L, Millet P, Rao A. Fueling the flame: bioenergy couples metabolism and inflammation. J Leuk Biol. (2012) 92:499–507. doi: 10.1189/jlb.0212078
61. Canto C, Gerhart-Hines Z, Feige J N, Lagouge M, Noriega L, Milne JC, et al. AMPK regulates energy expenditure by modulating NAD+ metabolism and SIRT1 activity. Nature. (2009) 458:1056–60. doi: 10.1038/nature07813
62. Ruderman NB, Xu XJ, Nelson L, Cacicedo JM, Saha AK, Lan F, et al. AMPK and SIRT1: a long-standing partnership? Am J Physiol Endocrinol Metab. (2010) 298:E751–60. doi: 10.1152/ajpendo.00745.2009
63. Brandauer J, Vienberg SG, Andersen MA, Ringholm S, Risis S, Larsen PS. et al. AMP-activated protein kinase regulates nicotinamide phosphoribosyl transferase expression in skeletal muscle. J Physiol. (2011) 591:5207–20. doi: 10.1113/jphysiol.2013.259515
64. Price NL, Gomes AP, Ling AJ, Duarte FV, Martin-Montalvo A, North BJ. SIRT1 is required for AMPK activation and the beneficial effects of resveratrol on mitochondrial function. Cell Met. (2012) 15:675–90. doi: 10.1016/j.cmet.2012.04.003
65. Schon C, Wacker R, Micka A, Steudle J, Lang S, Bonnlander B. Bioavailability study of maqui berry extract in healthy subjects. Nutrienst. (2018) 10:1720. doi: 10.3390/nu10111720
66. Lizuka Y, Ozeki A, Tani T, Tsuda T. Blackcurrant extract ameliorates hyperglycemia in type 2 diabetic mice in association with increased basal secretion of glucagon-like peptide-1 and activation of AMP-activated protein kinase. J Nutr Sci Vita. (2018) 64:258–64. doi: 10.3177/jnsv.64.258
67. Park M, Sharma A, Lee HJ. Anti-adipogenic effects of delphinidin-3-O-beta-glucoside in 3T3-L1 preadipocytes and primary white adipocytes. Molecule. (2019) 24:1848. doi: 10.3390/molecules24101848
68. Lai D, Huang M, Zhao L, Tian Y, Li Y, Liu D, et al. Delphinidin-induced autophagy protects pancreatic beta cells against apoptosis resulting from high-glucose stress via AMPK signaling pathway. Acta Biochim Biophys Sinica. (2019) 51:1242–49. doi: 10.1093/abbs/gmz126
69. Lee YK, Lee WS, Kim GS, Park OJ. Anthocyanins are novel AMPKa1 stimulators that suppress tumor growth by inhibiting mTOR phosphorylation. Onc Rep. (2010) 24:1471–7. doi: 10.3892/or_00001007
70. Lin SC, Hardie DG. AMPK: Sensing glucose as well as cellular energy status. Cell Met. (2018) 27:299–313. doi: 10.1016/j.cmet.2017.10.009
71. Coughlan KA, Valentine RJ, Ruderman NB, Saha AK. AMPK activation: a therapeutic target for type 2 diabetes? Diabetes Metab Syndr Obesity. (2014) 7:241–53. doi: 10.2147/DMSO.S43731
72. Goszcz K, Sherine I, Deakin J, Duthie GG, Stewart D, Megson IL. Bioavailable concentrations of delphinidin and its metabolite, gallic acid, induce antioxidant protection associated with increased intracellular glutathione in cultured endothelial cells. Oxidative Med Cell Long. (2017) 2017:1–17. doi: 10.1155/2017/9260701
73. Lappi J, Raninen K, Väkeväinen K, Kårlund A, Törrönen R, Kolehmainen M. Blackcurrant (Ribes nigrum) lowers sugar-induced postprandial glycaemia independently and in a product with fermented quinoa: a randomized crossover trial. Brit J Nutr. (2020) 9:1–10. doi: 10.1017/S0007114520004468
74. Raubenheimer D, Simpson SJ. Protein leverage: theoretical foundations and ten points of clarification. Obesity. (2019) 27:1225–38. doi: 10.1002/oby.22531
75. Batterham RL, Heffron H, Kapoor S, Chivers JE, Chandarana K, Herzog H, et al. Critical role for peptide YY in protein-mediated satiation and body-weight regulation. Cell Metabol. (2006) 3:223–33. doi: 10.1016/j.cmet.2006.08.001
76. Westerterp-Plantenga MS. Protein intake and energy balance. Regul Peptides. (2008) 149:67–9. doi: 10.1016/j.regpep.2007.08.026
77. Wyatt P, Berry SE, Finlayson G, O'Driscoll R, Hadjigeorgiou G, Drew DA, et al. Postprandial glycaemic dips predict appetite and energy intake in healthy individuals. Nat Metab. (2021) 3:523–9. doi: 10.1038/s42255-021-00383-x
78. Hochstenbach-Waelen A, Veldhorst MA, Nieuwenhuizen AG, Westerterp-Plantenga MS, Westerterp KR. Comparison of 2 diets with either 25% or 10% of energy as casein on energy expenditure, substrate balance, and appetite profile. Am J Clin Nutr. (2009) 89:831–38. doi: 10.3945/ajcn.2008.26917
79. Fulgoni VL. Current protein intake in America: analysis of the National Health and Nutrition Examination Survey, 2003-2004. Am J Clin Nutr. (2008) 87: 1554S−7S. doi: 10.1093/ajcn/87.5.1554S
80. Amamou T, Normandin E, Pouliot J, Dionne IJ, Brochu M, Riesco E. Effect of a high-protein energy-restricted diet combined with resistance training on metabolic profile in older individuals with metabolic impairments. J Nutr Health Aging. (2017) 21:67–74. doi: 10.1007/s12603-016-0760-8
82. Shukla P, Andono J, Touhamy SH, Casper A, Lliescu RG, Mauer E, et al. Carbohydrate-last meal pattern lowers postprandial glucose and insulin excursions in type 2 diabetes. BMJ Open Diab Res Care. (2017) 5:e000440. doi: 10.1136/bmjdrc-2017-000440
83. Shukla AP, Dickison M, Coughlin N, Karan A, Mauer E, Truong W. The impact of food order on postprandial glycaemic excursions in prediabetes. Diab Obesity Metabol. (2019) 21:377–81. doi: 10.1111/dom.13503
84. Ludwig DS. The glycemic index: physiological mechanisms relating to obesity, diabetes, and cardiovascular disease. J Am Med Assoc. (2002) 287:2414–23. doi: 10.1001/jama.287.18.2414
85. Aune D, Giovannucci E, Boffetta P, Fadnes LT, Keum N, Norat T, et al. Fruit and vegetable intake and the risk of cardiovascular disease, total cancer and all-cause mortality-a systematic review and dose-response meta-analysis of prospective studies. Int J Epidem. (2017) 46:1029–56. doi: 10.1093/ije/dyw319
86. Blasbalg TL, Hibbeln JR, Ramsden CE, Majchrzak SF, Rawlings RR. Changes in consumption of omega-3 and omega-6 fatty acids in the United States during the 20th century. Am J Clin Nutr. (2011) 93:950–62. doi: 10.3945/ajcn.110.006643
87. Guyenet SJ, Carlson SE. Increase in adipose tissue linoleic acid of US adults in the last half century. Adv Nutri. (2015) 6:660–64. doi: 10.3945/an.115.009944
88. Tse EK, Salehi Clemenzi MN, Belsham DD. Role of the saturated fatty acid palmitate in the interconnected hypothalamic control of energy homeostasis and biological rhythms. Am J Physiol Endocrin Metab. (2018) 315:E133–40. doi: 10.1152/ajpendo.00433.2017
89. Cheng L, Yu Y, Szabo A, Wu Y, Wang H, Camer D. Palmitic acid induces central leptin resistance and impairs hepatic glucose and lipid metabolism in male mice. J Nutr Biochem. (2015) 26:541–48. doi: 10.1016/j.jnutbio.2014.12.011
90. Kuipers RS, Luxwolda MF, Janneke Dijck-Brouwer DA, Boyd Eaton S, Crawford MA, Cordain L, et al. Estimated macronutrient and fatty acid intakes from an East African Paleolithic diet. Br J Nutr. (2010) 104:1666–87. doi: 10.1017/S0007114510002679
91. Johnston CS, Tjonn SL, Swan PD, White A, Hutchins H, Sears B. Ketogenic low-carbohydrate diets have no metabolic advantage over non-ketogenic low-carbohydrate diets. Am J Clin Nutr. (2006) 83:1055–61. doi: 10.1093/ajcn/83.5.1055
92. Pittas AG, Roberts SB, Das SK, Gilhooly CH, Saltzman E, Golden J, et al. The effects of the dietary glycemic load on type 2 diabetes risk factors during weight loss. Obesity. (2006) 14:2200–9. doi: 10.1038/oby.2006.258
93. Pereira MA, Swain J, Goldfine AB, Rifai N, Ludwig DS. Effects of a low-glycemic load diet on resting energy expenditure and heart disease risk factors during weight loss. J Am Med Assoc. (2004) 292:2482–90. doi: 10.1001/jama.292.20.2482
94. Parada Venegas M, De la Fuente MK, Landskron G, González MJ, Quera R, Dijkstra G. Short chain mediated fatty acids (SCFAs) mediated gut epithelial and immune regulation and its relevance for inflammatory bowel diseases. Front Immun. (2019) 10:277. doi: 10.3389/fimmu.2019.00277
95. Sitolo GC, Mitarai A, Adesina PA, Yamamoto Y, Suzuki T. Fermentable fibers up-regulate suppressor of cytokine signaling1 in the colon of mice and intestinal Caco-2 cells through butyrate production. Biosci Biotech Biochem. (2020) 84:233–46. doi: 10.1080/09168451.2020.1798212
96. Cani PD, Bibiloni R, Knauf C, Waget A, Neyrinck AM, Delzenne NM, et al. Changes in gut microbiota control metabolic endotoxemia-induced inflammation in high-fat diet–induced obesity and in mice. Diabetes. (2008) 57:1470–81. doi: 10.2337/db07-1403
97. Silva YP, Bernardi A, Frozza RL. The role of short-chain fatty acids from gut microbiota in gut-brain communication. Front Endocrin. (2020) 1:25. doi: 10.3389/fendo.2020.00025
98. Kaji I, Karaki S, Kuwahara A. Short-chain fatty acid receptor and its contribution to glucagon-like peptide-1 release. Digestion. (2014) 89:31–6. doi: 10.1159/000356211
99. Larraufie P, Martin-Gallausiaux C, Lapaque N, Dore J, Gribble FM, Reimann F, et al. SCFAs strongly stimulate PYY production in human enteroendocrine cells. Sci Rep. (2018) 8:74. doi: 10.1038/s41598-017-18259-0
100. Luca SV, Macovei Bujor A, Miron A, Skalicka-Wozniak W, Aprotosoaie AC. Bioactivity of dietary polyphenols: the role of metabolites. Cri Rev Food Sci Nutr. (2020) 604:626–59. doi: 10.1080/10408398.2018.1546669
101. Zamora-Ros R, Rabassa M, Cherubini A. High concentrations of a urinary biomarker of polyphenol intake are associated with decreased mortality in older adults. J Nutr. (2013) 143:1445–50. doi: 10.3945/jn.113.177121
102. Urpi-Sarda M, Andres-Lacueva C, Rabassa M. The relationship between urinary total polyphenols and the frailty phenotype in a community-dwelling older population: the InCHIANTI Study. J Geront Bio Sci Med Sci. (2015) 70:1141–47. doi: 10.1093/gerona/glv026
103. Cassidy A, Mukamal KJ, Liu L, Franz M, Eliassen AH, Rimm EB. High anthocyanin intake is associated with a reduced risk of myocardial infarction in young and middle-aged women. Circulation. (2013) 127:188–96. doi: 10.1161/CIRCULATIONAHA.112.122408
104. Cassidy A, Bertoia M, Chiuve S, Flint A, Forman J, Rimm EB. Habitual intake of anthocyanins and flavanones and risk of cardiovascular disease in men. Am J Cln Nutr. (2016) 104:587–94. doi: 10.3945/ajcn.116.133132
105. Gardner CD, Kim S, Bersamin A, Dopler-Nelson M, Otten J, Oelrich B, et al. Micronutrient quality of weight-loss diets that focus on macronutrients: results from the A TO Z study. Am J Clin Nutr. (2010) 92:304–12. doi: 10.3945/ajcn.2010.29468
106. McLaughlin T, Allison G, Abbasi F, Lamendola C, Reaven GM. Prevalence of insulin resistance and associated cardiovascular disease risk factors among normal weight, overweight, and obese individuals. Metabolism. (2004) 53:495–99. doi: 10.1016/j.metabol.2003.10.032
107. Gonzalez-Chavez A, Simental-Mendia LE, Elizondo-Argueta S. Elevated triglycerides/HDL-cholesterol ratio associated with insulin resistance. Cir Cir. (2011) 79:126–31.
108. Pantoja-Torres B, Toro-Huamanchumo CJ, Urrunaga-Pastor D, Guarnizo-Poma M, Lazaro-Alcantara H, Paico-Palacios S, et al. high triglyceride to HDL-cholesterol ratio is associated with insulin resistance in normal-weight healthy adults. Diab Met Syndr. (2019) 13:382–88. doi: 10.1016/j.dsx.2018.10.006
109. Murguia-Romero M, Jimenez-Flores JR, Sigrist-Flores SC, Espinoza-Camacho MA, Jiménez-Morales M, Pina E, et al. Plasma triglyceride/HDL-cholesterol ratio, insulin resistance, and cardiometabolic risk in young adults. J Lipid Res. (2013) 54:2795–99. doi: 10.1194/jlr.M040584
110. Fan X, Liu EY, Hoffman VP, Potts AJ, Sharma B, Henderson DC. Triglyceride/high-density lipoprotein cholesterol ratio: a surrogate to predict insulin resistance and low-density lipoprotein cholesterol particle size in nondiabetic patients with schizophrenia. J Clin Psych. (2011) 72:806–12. doi: 10.4088/JCP.09m05107yel
111. Quispe R, Martin SS, Jones SR. Triglycerides to high-density lipoprotein-cholesterol ratio, glycemic control and cardiovascular risk in obese patients with type 2 diabetes. Cur Opin Endocrin Diab Obesity. (2016) 23:150–56. doi: 10.1097/MED.0000000000000241
112. Salazar MR, Carbajal HA, Espeche WG, Aizpurúa M, Marillet AG, Leiva Sisnieguez CE. Use of the triglyceride/high-density lipoprotein cholesterol ratio to identify cardiometabolic risk: impact of obesity? J Invest Med. (2017) 65:323–27. doi: 10.1136/jim-2016-000248
113. Lind L, Ingelsson E, Arnlov J, Sundström J, Zethelius B, Reaven GM. Can the plasma concentration ratio of triglyceride/high-density lipoprotein cholesterol identify individuals at high risk of cardiovascular disease during 40-year follow-up? Met Synd Related Dis. (2018) 16:433–39. doi: 10.1089/met.2018.0058
114. Endres S, Ghorbani R, Kelley VE, Georgilis K, Lonnemann G, van der Meer JW, et al. The effect of dietary supplementation with n-3 polyunsaturated fatty acids on the synthesis of interleukin-1 and tumor necrosis factor by mononuclear cells. New Eng J Med. (1989) 320:265–71. doi: 10.1056/NEJM198902023200501
115. Tan A, Sullenbarger B, Prakash R, McDaniel JC. Supplementation with eicosapentaenoic acid and docosahexaenoic acid reduces high levels of circulating pro-inflammatory cytokines in aging adults: a randomized, controlled study. Prostaglandins Leukot Essent Fatty Acids. (2018) 132:23–9. doi: 10.1016/j.plefa.2018.03.010
116. Madison AA, Belury MA, Andridge R, Renna ME, Shrout MR, Malarkey WB, et al. Omega-3 supplementation and stress reactivity of cellular aging biomarkers. Mol Psychiatry. (2021) 2021:2. doi.org/10.1038/s41380-021-01077-2
117. Harris WS, Pottala JV, Lacey SM, Vasan RG, Larson MG, Robins SJ. Clinical correlates and heritability of erythrocyte eicosapentaenoic and docosahexaenoic acid content in the Framingham Heart Study. Atherosclerosis. (2012) 225:425–31. doi: 10.1016/j.atherosclerosis.2012.05.030
118. Harris WS, Pottala JV, Varvel SA, Borowski JJ, Ward JN, McConnell JP. Erythrocyte omega-3 fatty acids increase and linoleic acid decreases with age: observations from 160,000 patients. Pros Leuko Essen Fatty Acid. (2013) 88:257–63. doi: 10.1016/j.plefa.2012.12.004
119. Lin C, Chao H, Li Z, Xu X, Liu Y, Bao Z, et al. Omega-3 fatty acids regulate NLRP3 inflammasome activation and prevent behavior deficits after traumatic brain injury. Exp Neur. (2017) 290:115–22. doi: 10.1016/j.expneurol.2017.01.005
120. Martínez-Micaelo N, González-Abuín N, Pinent M, Ardévol A, Blay M. Dietary fatty acid composition is sensed by the NLRP3 inflammasome: Omega-3 fatty acid (DHA) prevents NLRP3 activation in human macrophages. Food Func. (2016) 7:3480–87. doi: 10.1039/C6FO00477F
121. Williams-Bey Y, Boularan C, Vural A. Omega-3 free fatty acids suppress macrophage inflammasome activation by inhibiting NF-κB activation and enhancing autophagy. PLoS ONE. (2014) 9:e97957. doi: 10.1371/journal.pone.0097957
122. Zhu MJ, Kang Y, Xue Y. Red raspberries suppress NLRP3 inflammasome and attenuate metabolic abnormalities in diet-induced obese mice. J Nutr Biochem. (2018) 53:96–103. doi: 10.1016/j.jnutbio.2017.10.012
123. Fan R, You M, Toney AM, Kim J, Giraud D, Xian Y, et al. Red raspberry polyphenols attenuate high-fat diet-driven activation of NLRP3 inflammasome and its paracrine suppression of adipogenesis via histone modifications. Mol Nutr Food Res. (2020) 64:e1900995. doi: 10.1002/mnfr.201900995
124. Bhatt DL, Steg PG, Miller M, Brinton EA, Jacobson TA, Ketchum SB, et al. REDUCE-IT Investigators. Cardiovascular risk reduction with Icosapent Ethyl for hypertriglyceridemia. N Engl J Med. (2019) 380:11–22. doi: 10.1056/NEJMoa1812792
125. Elajami TK, Colas RA, Dalli J, Chiang N, Serhan CN, Welty FK. Specialized pro-resolving lipid mediators in patients with coronary artery disease and their potential for clot remodeling. FASEB J. (2016) 30:2792–801. doi: 10.1096/fj.201500155R
126. Alvarado J, Schoenlau F, Leschot A, Salgad AM, Vigil Portales P. Delphinol standardized maqui berry extract significantly lowers blood glucose and improves blood lipid profile in prediabetic individuals in three-month clinical trial. Panminerva Med. (2016) 58(3Suppl.1):1–6. doi: 10.1155/2016/9070537
127. Davinelli S, Bertoglio JC, Zarrelli A, Pina R, Scapagnini G. A randomized clinical trial evaluating the efficacy of an anthocyanin-maqui berry extract (Delphinol®) on oxidative stress biomarkers. J Am Coll Nutr. (2015) 34(Suppl.1):28–33. doi: 10.1080/07315724.2015.1080108
128. Himsworth HP. Diabetes mellitus: its differentiation into insulin-sensitive and insulin-insensitive types. Lancet. (1936) 227:127–30. doi: 10.1016/S0140-6736(01)36134-2
129. Reaven GM. Banting lecture 1988. Role of insulin resistance in human disease. Diabetes. (1988) 37:1595–607. doi: 10.2337/diabetes.37.12.1595
130. Yaribeygi H, Farrokhi FR, Butler AE, Sahebkar A. Insulin resistance: review of the underlying molecular mechanisms. J Cell Physiol. (2019) 234:8152–61. doi: 10.1002/jcp.27603
131. Petersen MC, Shulman GI. Mechanisms of insulin action and insulin resistance. Physiol Rev. (2018) 98:2133–223. doi: 10.1152/physrev.00063.2017
132. Hotamisligil GS. Inflammation and metabolic disorders. Nature. (2006) 444:860–7. doi: 10.1038/nature05485
133. Chen L, Chen R, Wang H, Liang F. Mechanisms linking inflammation to insulin resistance. Int J Endocrinol. (2015) 2015:508409. doi: 10.1155/2015/508409
134. Hotamisligil GS. Foundations of immunometabolism and implications for metabolic health and disease. Immun. (2017) 47:406–20. doi: 10.1016/j.immuni.2017.08.009
135. Lee YS, Olefsky J. Chronic tissue inflammation and metabolic disease. Genes Dev. (2021) 35:307–28. doi: 10.1101/gad.346312.120
136. Saha AK, Xu XJ, Balon TW, Brandon A, Kraegen EW, Ruderman NB. Insulin resistance due to nutrient excess: is it a consequence of AMPK downregulation? Cell Cycle. (2011) 10:3447–51. doi: 10.4161/cc.10.20.17886
137. Ruderman NB, Carling D, Prentki M, Cacicedo JM. AMPK insulin resistance, and the metabolic syndrome. J Clin Invest. (2013) 123:2764–72. doi: 10.1172/JCI67227
138. Lyons CL, Roche HM. Nutritional modulation of AMPK-Impact upon metabolic-inflammation. Int J Mol Sci. (2018) 19:3092. doi: 10.3390/ijms19103092
139. Smith BK, Marcinko K, Desjardins EM, Lally JS, Ford RJ, Steinberg GR. Treatment of non-alcoholic fatty liver disease: role of AMPK. Am J Physiol Endocrinol Metab. (2016) 311:E730–40. doi: 10.1152/ajpendo.00225.2016
140. Desjardins EM, Steinberg GR. Emerging role of AMPK in brown and beige adipose tissue (BAT): implications for obesity, insulin resistance, and type 2 diabetes. Curr Diab Rep. (2018) 18:80. doi: 10.1007/s11892-018-1049-6
141. Wang F, Han L, Hu D. Fasting insulin, insulin resistance and risk of hypertension in the general population: a meta-analysis. Clin Chim Acta. (2017) 464:57–63. doi: 10.1016/j.cca.2016.11.009
142. Laakso M. Kuusisto Insulin resistance and hyperglycaemia in cardiovascular disease development. J Nat Rev Endocrinol. (2014) 10:293–302. doi: 10.1038/nrendo.2014.29
143. Diamanti-Kandarakis E, Dunaif A. Insulin resistance and the polycystic ovary syndrome revisited: an update on mechanisms and implications. Endocr Re. (2012) 33:981–1030. doi: 10.1210/er.2011-1034
144. Spoto B, Pisano A, Zoccali C. Insulin resistance in chronic kidney disease: a systematic review. Am J Physiol Renal Physiol. (2016) 311:F1087–108. doi: 10.1152/ajprenal.00340.2016
145. Arcidiacono B, Iiritano S, Nocera A, Possidente K, Nevolo MT, Ventura V, et al. Insulin resistance and cancer risk: an overview of the pathogenetic mechanisms. Exp Diabetes Res. (2012) 2012:789174. doi: 10.1155/2012/789174
146. Leonard BE, Wegener G. Inflammation, insulin resistance and neuroprogression in depression. Acta Neuropsychiatr. (2020) 32:1–9. doi: 10.1017/neu.2019.17
147. Hölscher C. Brain insulin resistance: role in neurodegenerative disease and potential for targeting. Expert Opin Investig Drugs. (2020) 29:333–48. doi: 10.1080/13543784.2020.1738383
148. Nawrocki AR, Rajala MW, Tomas E, Pajvani UB, Saha AK, Trumbauer ME, et al. Mice lacking adiponectin show decreased hepatic insulin sensitivity and reduced responsiveness to peroxisome proliferator-activated receptor gamma agonists. J Biol Chem. (2006) 281:2654–60. doi: 10.1074/jbc.M505311200
149. Ye JM, Dzamko N, Hoy AJ, Iglesias MA, Kemp B, Kraegen E. Rosiglitazone treatment enhances acute AMP-activated protein kinase-mediated muscle and adipose tissue glucose uptake in high-fat-fed rats. Diabetes. (2006) 55:2797–804. doi: 10.2337/db05-1315
150. Zhang W, Wu R, Zhang F, Xu Y, Liu B, Yang Y, et al. Thiazolidinediones improve hepatic fibrosis in rats with non-alcoholic steatohepatitis by activating the adenosine monophosphate-activated protein kinase signalling pathway. Clin Exp Pharmacol Physiol. (2012) 39:1026–33. doi: 10.1111/1440-1681.12020
151. Lebovitz HE. Thiazolidinediones: the forgotten diabetes medications. Curr Diab Rep. (2019) 19:151. doi: 10.1007/s11892-019-1270-y
152. Ghoneum A, Abdulfattah AY, Said N. Targeting the PI3K/AKT/mTOR/NFkappaB axis in ovarian cancer. J Cell Immunol. (2020) 2:68–73. doi: 10.33696/immunology.1.022
153. Jian MY, Alexeyev MF, Wolkowicz PE, Zmijewski JW, Creighton JR. Metformin-stimulated AMPK-alpha1 promotes microvascular repair in acute lung injury. Am J Physiol Lung Cell Mol Physiol. (2013) 305:L844–855. doi: 10.1152/ajplung.00173.2013
154. Liang Z, Li T, Jiang S, Xu J, Di W, Yang Z, et al. A novel target for treating hepatic fibrosis. Oncotarget. (2017) 8:62780–92. doi: 10.18632/oncotarget.19376
155. Kaptoge S, Seshasai SR, Gao P, Freitag DF, Butterworth AS, Borglykke A. Inflammatory cytokines and risk of coronary heart disease: new prospective study and updated meta-analysis. Eur Heart J. (2014) 35:578–89. doi: 10.1093/eurheartj/eht367
156. Hoesel B, Schmid JA. The complexity of NF-kappaB signaling in inflammation and cancer. Mol Cancer. (2013) 12:86. doi: 10.1186/1476-4598-12-86
157. Mossmann D, Park S, Hall MN. mTOR signalling and cellular metabolism are mutual determinants in cancer. Nat Rev Cancer. (2018) 18:744–57. doi: 10.1038/s41568-018-0074-8
158. Noorolyai S, Shajari N, Baghbani E, Sadreddini S, Baradaran B. The relation between PI3K/AKT signalling pathway and cancer. Gene. (2019) 698:120–28. doi: 10.1016/j.gene.2019.02.076
159. Xiang HC, Lin LX, Hu XF, Zhu H, Li HP, Zhang RY, et al. activation attenuates inflammatory pain through inhibiting NF-kappaB activation and IL-1beta expression. J Neuroinflammation. (2019) 16:34. doi: 10.1186/s12974-019-1411-x
160. Kauppinen A, Suuronen T, Ojala J, Kaarniranta K, Salminen A. Antagonistic crosstalk between NF-kappaB and SIRT1 in the regulation of inflammation and metabolic disorders. Cell Signal. (2013) 25:1939–48. doi: 10.1016/j.cellsig.2013.06.007
161. Jäger S, Handschin C, St-Pierre J, Spiegelman BM. AMP-activated protein kinase (AMPK) action in skeletal muscle via direct phosphorylation of PGC-1alpha. Proc Natl Acad Sci USA. (2007) 104:12017–22. doi: 10.1073/pnas.0705070104
162. Shaw RJ. LBI and AMPK control of mTOR signalling and growth. Acta Physiol. (2009) 196:65–80. doi: 10.1111/j.1748-1716.2009.01972.x
163. Ghosh HS, McBurney M, Robbins PD. SIRT1 negatively regulates the mammalian target of rapamycin. PLoS ONE. (2010) 5:e9199. doi: 10.1371/journal.pone.0009199
164. Dan HC, Cooper MJ, Cogswell PC, Duncan JA, Ting JP-Y, Baldwin SAS. Akt-dependent regulation of NF-κBis controlled by mTOR and Raptor in association with Iκκ. Genes Dev. (2008) 22:1490–500. doi: 10.1101/gad.1662308
165. Hopkins BD, Goncalves MD, Cantley LC. Insulin-PI3K signalling: an evolutionarily insulated metabolic driver of cancer. Nat Rev Endocrinol. (2020) 16:276–83. doi: 10.1038/s41574-020-0329-9
166. Schultze SM, Hemmings BA, Niessen M, Tschopp O. PI3K/AKT, MAPK and AMPK signalling: protein kinases in glucose homeostasis. Expert Rev Mol Med. (2012) 14:e1. doi: 10.1017/S1462399411002109
167. Zhao Y, Hu X, Liu Y, Dong S, Wen Z, He W, et al. ROS signaling under metabolic stress: crosstalk between AMPK and AKT pathway. Mol Cancer. (2017) 16:79. doi: 10.1186/s12943-017-0648-1
168. Hamdy O, Carver C. The Why WAIT program: improving clinical outcomes through weight management in type 2 diabetes. Curr Diab Res. (2008) 8:413–20. doi: 10.1007/s11892-008-0071-5
169. Hamdy O, Mottalib A, Morsi A, El-Sayed N, Goebel-Fabbri A, Arathuzik G, et al. Long-term effect of intensive lifestyle intervention on cardiovascular risk factors in patients with diabetes in real-world clinical practice: a 5-year longitudinal study. BMJ Open Diabetes Res Care. (2017) 5:e000259. doi: 10.1136/bmjdrc-2016-000259
170. Jiramongkol Y, Lam EW-F. FOXO transcription factor family in cancer and metastasis. Cancer Metastasis Rev. (2020) 39:681–709. doi: 10.1007/s10555-020-09883-w
171. Zhang X, Tang N, Hadden TJ, Rishi AK. Akt, FoxO and regulation of apoptosis. Biochim Biophys Acta. (2011) 1813:1978–86. doi: 10.1016/j.bbamcr.2011.03.010
172. Wang F, Nguyen M, Qin FX, Tong Q. SIRT2 deacetylates FOXO3a in response to oxidative stress and caloric restriction. Aging Cell. (2007) 6:505–14. doi: 10.1111/j.1474-9726.2007.00304.x
173. Mouchiroud L, Houtkooper RH, Moullan N, Katsyuba E, Ryu D, Canto C, et al. The NAD(+)/Sirtuin pathway modulates longevity through activation of mitochondrial UPR and FOXO signaling. Cell. (2013) 154:430–41. doi: 10.1016/j.cell.2013.06.016
174. Hay N. Interplay between FOXO, TOR, and Akt. Biochim Biophys Acta. (2011) 1813:1965–70. doi: 10.1016/j.bbamcr.2011.03.013
175. Tzivion G, Dobson M, Ramakrishnan G. FoxO transcription factors; Regulation by AKT and 14-3-3 proteins. Biochim Biophys Acta. (2011) 1813:1938–45. doi: 10.1016/j.bbamcr.2011.06.002
176. Janku F, Yap TA, Meric-Berstam F. Targeting the PI3K pathway in cancer. Nat Rev Clin Oncol. (2018) 15:273–91. doi: 10.1038/nrclinonc.2018.28
177. Salminen A, Kaarniranta K. AMP-activated protein kinase (AMPK) controls the aging process via an integrated signaling network. Ageing Res Rev. (2012) 11:230–41. doi: 10.1016/j.arr.2011.12.005
178. Rutherford C, Speirs C, Williams JJ, Ewart MA, Mancini SJ, Hawley SA, et al. Phosphorylation of Janus kinase 1 (JAK1) by AMP-activated protein kinase (AMPK) links energy sensing to anti-inflammatory signaling. Sci Signal. (2016) 9:ra109. doi: 10.1126/scisignal.aaf8566
179. Longo VD, Antebi A, Bartke A, Barzilai N, Brown-Borg HM, Caruso C, et al. Interventions to slow aging in humans: are we ready? Aging Cell. (2015) 14:497–510. doi: 10.1111/acel.12338
AA, Arachidonic acid; ACC 1 and ACC2, Acetyl-CoA carboxylase 1 and 2; AGE, Advanced glycosylated end products; AMPK, 5' adenosine monophosphate-activated protein kinase; APC, antigen-presenting cell; COX-2, Cyclooxygenase-2; DAMP, Danger-associated molecular patterns; DHA, Docosahexaenoic acid; DPA, Docosapentaenoic acid; ECM, Extracellular matrix; EPA, Eicosapentaenoic acid; FOXO, Forkhead box transcription factors class O; GLP-1, Glucagon-like peptide; Glut, Glucose transporter protein; GS, Glycogen synthetase; HbA1c, Hemoglobin A1c; JAK, Janus kinase; LKB1, Liver kinase B1; mTOR, Mammalian target of rapamycin; NAMPT, Nicotinamide phosphoribosyltransferase; NF-κB, Nuclear factor kappa-B; NOS, Nitrogen oxide synthetase; PYY, Peptide YY; PGC-1α, Peroxisome proliferator-activated receptor gamma coactivator 1-alpha; RAGE, Receptor for advanced glycation end products; RvD1, Resolvin D1; SASP, Senescence associated secretory phenotype; SCFA, Short-chain fatty acids; SIRT, Sirtuins (silent information regulator family of proteins); SPMs, Specialized pro-resolving meditators; SREBP-1c, Sterol regulatory element-binding protein-1c; STAT, Signal transducer and activator of transcription; TNFα, Tumor necrosis factor-alpha; TLR-2, Toll-like receptor-2; TLR-4, Toll-like receptor-4; TSC2, Tuberous sclerosis complex 2; ULK-1, Unc-51-like kinase 1.
Keywords: 5' adenosine monophosphate-activated protein kinase, SPMs, calorie-restriction, anti-inflammatory diet, omega-3 fatty acids, resolution response, polyphenols, inflammation
Citation: Sears B and Saha AK (2021) Dietary Control of Inflammation and Resolution. Front. Nutr. 8:709435. doi: 10.3389/fnut.2021.709435
Received: 13 May 2021; Accepted: 13 July 2021;
Published: 10 August 2021.
Edited by:
Mireille Sthijns, Maastricht University, NetherlandsReviewed by:
Sabine Daemen, Washington University in St. Louis, United StatesCopyright © 2021 Sears and Saha. This is an open-access article distributed under the terms of the Creative Commons Attribution License (CC BY). The use, distribution or reproduction in other forums is permitted, provided the original author(s) and the copyright owner(s) are credited and that the original publication in this journal is cited, in accordance with accepted academic practice. No use, distribution or reproduction is permitted which does not comply with these terms.
*Correspondence: Barry Sears, YnNlYXJzQGRyc2VhcnMuY29t
Disclaimer: All claims expressed in this article are solely those of the authors and do not necessarily represent those of their affiliated organizations, or those of the publisher, the editors and the reviewers. Any product that may be evaluated in this article or claim that may be made by its manufacturer is not guaranteed or endorsed by the publisher.
Research integrity at Frontiers
Learn more about the work of our research integrity team to safeguard the quality of each article we publish.