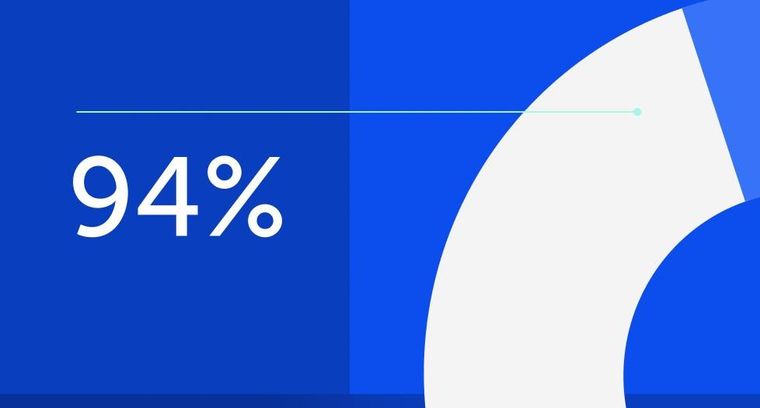
94% of researchers rate our articles as excellent or good
Learn more about the work of our research integrity team to safeguard the quality of each article we publish.
Find out more
REVIEW article
Front. Nutr., 04 August 2021
Sec. Nutrition and Microbes
Volume 8 - 2021 | https://doi.org/10.3389/fnut.2021.701511
This article is part of the Research TopicGut Microbial Response to Host Metabolic Phenotypes: Volume 1View all 24 articles
Ruminants are mostly herbivorous animals that employ rumen fermentation for the digestion of feed materials, including dairy cows. Ruminants consume plant fibre as their regular diet, but lack the machinery for their digestion. For this reason, ruminants maintain a symbiotic relation with microorganisms that are capable of producing enzymes to degrade plant polymers. Various species of microflora including bacteria, protozoa, fungi, archaea, and bacteriophages are hosted at distinct concentrations for accomplishing complete digestion. The ingested feed is digested at a defined stratum. The polysaccharic plant fibrils are degraded by cellulolytic bacteria, and the substrate formed is acted upon by other bacteria. This sequential degradative mechanism forms the base of complete digestion as well as harvesting energy from the ingested feed. The composition of microbiota readily gets tuned to the changes in the feed habits of the dairy cow. The overall energy production as well as digestion is decided by the intactness of the resident communal flora. Disturbances in the homogeneity gastrointestinal microflora has severe effects on the digestive system and various other organs. This disharmony in communal relationship also causes various metabolic disorders. The dominance of methanogens sometimes lead to bloating, and high sugar feed culminates in ruminal acidosis. Likewise, disruptive microfloral constitution also ignites reticuloperitonitis, ulcers, diarrhoea, etc. The role of symbiotic microflora in the occurrence and progress of a few important metabolic diseases are discussed in this review. Future studies in multiomics provides platform to determine the physiological and phenotypical upgradation of dairy cow for milk production.
Nearly 200 species of ruminants were identified till date, and among them, six were domesticated (1). Dairy cow was the most studied. Earlier studies provide insights into the knowledge of their digestive metabolism. Ruminants (mostly herbivores) employ foregut fermentation that allows them to digest cellulosic materials from plants. But during evolution, vertebrates lost the ability to produce enzymes that degrade cellulose and other complex polysaccharides (2). The ruminants rely upon a symbiotic relationship with microorganisms to digest such compounds. The microbiota produces enzymes to break the complex compounds into simpler molecules for easy absorption by the intestine. To carry out this, the host system has to provide an optimal environment and substrate for the survival of microflora. Thus, a commensal relationship is maintained where the host organism provides the substrates and maintains the environment required for the survival of the organism. In return, the microflora offers the nutrients required for the host organism (3).
The physiology and structure of the ruminant digestive system evolved billion years ago to ensure the effective digestion of cellulosic materials and various polysaccharides (4). The potency of the system lies in its design where the ingested feed material experiences a prolonged interaction with microflora (5). The ruminant stomach is a quadra compartmental digestive sac composed of the rumen, reticulum, omasum, and abomasum. Rumen internal environment is partitioned into different sacs by reticulo-ruminal fold in which the ingested food enters the rumen and then the reticulum (Figure 1). The rumen is lined with papillae, whereas the reticular epithelium forms a honeycomb structure. Feed consumed is directed toward the rumen through the reticulum (6). Reticulorumen (collective chamber of rumen and reticulum) stores the feed consumed for rumination and interaction with microflora. The feed is chewed to mix it with saliva and then swallowed. The ingested feed is then transferred to the anterior reticulorumen. Saliva is crucial for ingestion as well as rumination. It contains phosphate, potassium, and sodium bicarbonate in high concentrations to buffer the acids generated during fermentation. The reticulorumen appears to be a multifunctional fermentation sac with sizes varying from cattle (35–100 L) and sheep (3–5 L) (7). The physicochemical parameters of the rumen are described in Table 1 (9–13). The host organism maintains the environment of rumen through various mechanisms. The atmosphere in the reticulorumen is mostly anaerobic with carbon dioxide (65%), methane (27%), nitrogen (7%), and hydrogen (0.2%) (14, 15). Along with these, traces of O2, H2S, and CO are also present. This gas composition is due to the rigorous fermentation in the rumen by resident microflora. The ingested feed is regurgitated to facilitate proper fermentation through interaction with microflora, a process called rumination.
Rumination helps in increasing the surface area and decreasing the size of the feed particles, thereby promoting proper fermentation (16–18). In continuation, after the degradation of feed particles into smaller compounds, the feed is passed into the following chamber omasum. Omasum plays the role of a filter through which lesser size (<2 mm) particles can freely pass through (19). Then the digested fodder moves to abomasum, the true stomach. The abomasum has a distinct enzyme component lysozyme that attacks the cell walls of bacteria (20, 21). In abomasum, the digestion of bacterial proteins as well as digesta is done in a similar fashion as the other non-ruminants (17). Host genetics also play a crucial role in deciding the fate and constitution of rumen microflora, which in turn has an effect on fermentation and the products (22). The maintenance of the rumen environment is crucial for the host to digest the feed and survive (23). This process in turn effects the ability of the dairy cattle to produce milk. The constitution of microflora is very important for all the above reasons. Deviation of the constitution of microflora or intrusion of infective organisms through feed, environmental, and other factors leads to disturbances in the metabolism of the host. This leads to diseases, and the regulation of such process is mandatory. Present review throws light on the roles of gut microbes in the health and metabolic diseases of ruminants.
Ruminants feed on plants that are the sources of complex polysaccharides, viz., hemicellulose, cellulose, and lignin (24). However, due to the lack enzymatic system to degrade polysaccharides, they employ microflora that are capable of hydrolyzing these compounds in the gut for energy generation (25). These commensal microbes utilize the feed consumed by the host for survival, thereby establishing a healthy symbiotic relationship. The microbial population is habituated in the reticulorumen compartment. The reticulorumen environment is strictly anaerobic. It comprises dense and diverse microflora with eukarya (fungi and protozoa), archaea (methanogens), and bacteria at concentrations of 104, 106, and 1010, respectively (26). These bacterial populations seem to be very sensitive and can be influenced by little changes in the rumen environment. Fermentation by the rumen microflora is a complex process in which microorganisms act in coordination to generate simpler compounds that are easily metabolized by the host (27). The polysaccharides are metabolized into two simpler forms. The former one is the proteins required for bacterial cell wall synthesis and the later form is the volatile fatty acid (VFA), which are end products of fermentation (28, 29). VFA plays a crucial role in ruminant metabolism and acts as a source of host energy. They participate in vital pathways such as fatty acid synthesis and gluconeogenesis. Recent findings indicate that VFA holds ~70% of plant polysaccharides energy content (30, 31).
Primarily rumen is hosted by various microorganisms that assist the host organism in the digestion of complex polysaccharides of the dairy cow. The infants derive basal flora from the environment, feed consumed, partners, etc. The early gut microflora is developed from breast feeding (43%) and environment (28%), whereas non-breastfed lambs receive from vagina (46%) and air (31%) (32). The first floor of the rumen of neonates is colonized by enterococcus and streptococcus species which transform the gut environment to anaerobic (33). This helps in the recruitment of strict anaerobes in the gut to maintain the anaerobic ambience. Facultative anaerobes and aerobes are present in very less quantities, approximately 100-fold lesser in comparison with anaerobic organisms. The digestive capability of the dairy cow is directly proportional to the existing rumen microflora activity. Most of the organisms present in the rumen are non-culturable, whereas the culturable biota were studied in all aspects (34, 35).
The constitution of gut microbiota varies with the host, indicating a solid environmental-driven specificity of the host. The microbial composition of the feces in twins was more similar than in siblings (36). This implies the involvement of host genetics in deciding the individual gut microflora. Individuals also vary in fungal and archaeal compositions. The choice and development of gut microflora hence is a collaborative play of host genetics as well as environment. It is an ardent fact that the physiology of the individual has a strong relationship with gut microbial development. Apart from this, microflora differs from section to section in gut regions. Strict segregation of microflora between digestive and epithelium starts in the early stages of the life of a calf. The methanogenic composition also differs down the gastrointestinal tract. In neonatal calves the phylum Bacteroides is predominant, whereas in adult animals the phyla Prevotella and Bacteroidetes are abundant. Studies indicate that the microflora of 21-day-old calves has Prevotella (15.1%) and Bacteroidetes (15.8%), implying a starter-feed-driven rumen microbiome development during maturation. Methanogens and cellulolytic members were observed at 3–4 days of age, and this population is similar to that of matured mammals. Cellulolytic flora is present in 1-day individuals, indicating their importance in the ruminant system. Surprisingly, the rumen microflora of 14-day-old calves harbors more profuse yet ephemeral microorganisms in comparison with adult organisms. Metagenomic studies indicate that archaea (0.6–4%), eukarya (1.5%), and bacteria were present in ascending order of magnitude, with bacteria contributing 95% of the coding sequence.
Bacteria occupies the major portion of gut microflora, and their presence is crucial for the health of the dairy cow. They aid in the fermentation and degradation of plant polymers by the secretion of various enzymes (37, 38). Rumen contains about 1 × 103 bacteria/mL, and this consortium is complex in terms of functionality and taxon identification. The communal interaction of various bacteria enables the breakdown of the ingested fiber. The identification of these bacteria and their unique functionality has become the focal point of many studies. With the advancement of next generation sequencing technology, microbiological techniques, culture free approaches, and genetic engineering it has become easier to step forward in studying the role of commensal flora in host metabolism. Gene sequencing helped to identify and classify bacteria based on 16 s rRNA and physicochemical properties. The predominant microflora in the rumen are Proteobacteria, Bacteroidetes, and Firmicutes. In a study, Prevotella has also been identified with 42–60% of rRNA composition in two lactating cows. The coordinated metabolism of the microflora in which the metabolic product of one organism acts as a substrate for the other allows the sequential digestion of plant polymers (39, 40). The bacterial consortium is highly complex, and hence most of the bacteria are uncultured. The flora which are dominant and have a specific role in the metabolism are covered here so far.
The cell wall of a plant is comprised of a hemicellulose matrix with embedded cellulose fibers in it. The initial degradation of this matrix is carried out by a particular taxon of bacteria that secretes cellulolytic enzymes (24, 25). In general, bacteria contribute to most of the xylanase and endoglucanase activities in the rumen. These degrade the cellulose into smaller oligo/disaccharides which are then acted upon by other organisms. The first order cellulolytic bacteria includes Ruminococcus flavefaciens, Ruminococcus albus, and Fibrobacter succinogenes. Also, Butyrivibrio fibrisolvens is present in a lesser extent in comparison with the above said organisms. Apart from these, other uncultured bacteria can also act upon the substrate to degrade cellulose fibers. Some organisms like Cellulosilyticum ruminicola H1, from the rumen of Yak, also have the capability to produce lignocellulolytic enzymes. On the other hand, coculturing of some organisms implicated negative interaction and decreased enzyme efficiency (41). This inhibition is found to be an effect of the bacteriocins secreted as a part of the defense mechanism and competition for the substrate (42). For instance, R. flavefaciens and R. albus secrete bacteriocins in competition for cellulose (43, 44). Non-cellulolytic bacteria also secrete bacteriocins and are supposed to be tough competitors for different substrates in a rumen environment (45, 46).
The end products of cellulolytic bacterial interaction act as substrates for different microflora that start further degradation of such compounds. Other important polymers, such as starch, are hydrolyzed by Selenomonas ruminantium, Succinomonas amylolytica, Butyrivibrio fibrisolvens, Streptococcus bovis, Ruminobacter amylophilus, and Prevotella species, whereas pectin is degraded by Lachnospira multiparus and Succinovibro dextrinosolvens. Besides, the constitution of bacteria changes with the type of feed consumed by the host (5, 47, 48). Animal feeding differs in various places. High fiber feed is rich in cellulose whereas high grain feed is packed with starchy material. This influences the type of bacteria required to digest the material consumed and has a strong impact on microflora constitution in the gut environment (49, 50). Sugar and starch fermenters constitute most of the rumen bacteria. Maximum energy is extracted from the plant polysaccharides as the end products of bacterial fermentation serve as substrate to many other organisms. Megasphaera elsdenii acts upon lactate (end product of bacterial fermentation) and Veillonella alcalescens utilizes succinate, acetate, and hydrogen (51, 52).
Recent metagenomic studies on gut microflora of various mammalian species revealed that in ruminant and herbivore microflora the anabolic pathways for the synthesis of amino acids (AAs) are more prevalent in comparison to carnivores. This is because the diet of a carnivore would be rich in protein, and therefore the constitution of gut microbiota is chosen to be more proteolytic. In the point of herbivores, the diet is fiber rich, and carbohydrate is the core source of energy (53). Hence in the microbiota of rumen, the AA synthesis pathways are commonly seen. Indeed, a certain cellulolytic activity some organisms also exhibit potent proteolytic activity, such as B. fibrisolvens, P. ruminicola, S. ruminantium, and R. amylophilus. P. ruminicola exhibits deaminase and proteolytic activities and produces higher amounts of ammonia (NH3) in the rumen. This activity is considered to be crucial as the rumen environment has lesser protein and ammonia that act as nitrogen sources for AA and protein synthesis (54). Other classes of bacteria include sulfate-reducing bacteria that assist in the reduction of sulfate to H2S. In addition, it has to be noted that the rumen microbiota is fine-tuned depending upon the dietary changes to assist degradation and fermentation of various complex compounds. They also have communal relations with each other and with the host to ensure their survival as well as maximum energy production. They also play a role in supplying VFAs and proteins to the host organism. Disturbances in concentrations of microbiota sometimes have a heavy impact on the host system and may lead to diseases. Different types of bacteria are listed in Table 2.
Anaerobic methanogens make up most of the archaea constituting ~0.6–3.3% of the total rumen microbiota (65). Major archaea members of rumen microbiota are listed in Table 3. Metagenomic studies and 16 s rRNA sequencing analyses revealed the presence of archaea in the rumen environment. Studies revealed that about 3.6% of microbiota in rumen exhibited autofluorescence, a distinctive property exhibited by methanogenic bacteria (71). Methanogens, as the name indicates, generate methane (CH4) either by the reduction of CO2 or by the hydrolysis of acetate to CH4 and CO2. Most of the ruminal methane is produced via the reduction of CO2 rather than dissimilating acetate. The process of CO2 reduction requires electrons which come from various sources, including methylamine, methanol, formate, and hydrogen produced as metabolic intermediates (72, 73). Archaea are clustered under Euryarchaeota and are classified as Methanomicrobiales, Methanosarcinales, Methanococcales, Methanobacteriales, and Methanopyrales. Most of the ruminant methanogens fall under one of the three categories identified. They are ordered as Methnaomicrobiales < Methnaomicrobium and Methanobacteriales (14.9%) < Methanobrevibacter (61.6%). Apart from this, another set of uncultured ruminal archaea were categorized under rumen cluster C (RCC). A study on the ruminal archaea community of red deer, cattle, and sheep disclosed the fact that their composition is maintained throughout different species. They are more conserved when compared to the bacterial members. The dominant archaea species stood same in all the rumens. Species belonging to Methanobrevibacter is found to be dominant in rumen. About 26.5% of the total archaea is occupied by members of RCC (55, 66).
Methane production by various archaea is mediated by cytochrome in few methanogens, whereas alternative complexes mediate this process in some methanogens. The genus Methanosarcinales comprises of methanogens and has the capability to grow on a wide range of substrates. Hydrogen concentration in the environment plays a crucial role in the production of methane. Cytochrome-based methanogens have higher growth yields when compared with non-cytochromic methanogens. Non-cytochromic methanogens need lesser hydrogen concentration to produce methane whereas cytochromic methanogens need about 10-fold higher concentrations of hydrogen for the optimal growth. This is the reason for the presence of non-cytochromic methanogens in higher concentrations in the rumen. Hydrogen utilization by methanogens is crucial as it decreases the pressure, allowing the conversion of endergonic metabolic reactions to exergonic reactions. This makes bacterial fermentation energetically favorable (74). Hydrogen consumption by methanogens stands as a good example of the symbiotic relationship between methanogenic and cellulolytic bacteria, wherein the hydrogen produced by the latter is consumed by the former for its survival. Coculturing of rumen methanogens and ruminal fungus has a heavy influence on cellulolytic and fermentation activities. Hydrogen transfer among methanogens and other microflora in rumen is best described by coculturing methanogens with protozoa. Even though archaea and bacteria fall prey to protozoa, methanogens get habituated inside and help in the generation of energy by consuming the hydrogen produced during the metabolism (74–76). Hydrogen consumption by methanogens forms the root of symbiosis with other microbiota in the rumen for maximal energy production (77–79). The commensal interactions of methanogens with protozoa and other rumen microbiota facilitate the complete degradation of complex plant polymers. The methane production is directly related to the amount of fodder and hemicellulose degradation (80–82). About 19% of the total energy of the feed is lost during the production of methane gas by methanogens. The commensal interaction of methanogens with other microbiota in the rumen enhances energy production to a maximum extent. But the gas production has a hinderance effect on the overall energy harvested from the ingested feed.
Protozoa are unicellular organisms bound by pellicle or cuticle in the rumen. They are the simplest forms of eukaryotes found in the universe (Table 3). Most of the protozoa are parasitic as they feed on microorganisms, organic matter, and cell debris. Ciliates are more prevalent in ruminant gut in comparison with several flagellate species. Ciliates are subcategorized into Vestibuliferida and Entodiniomorphida with 25 genera. Protozoa in the rumen have specialized functions tuned to survive in a rumen environment (83, 84). Most of the protozoa are anaerobic, but very few species are supposed to sequester oxygen. Oxygen sequestration from the environment is advantageous to the host as it maintains the anaerobic ambience of the reticulorumen. This also helps in the survival of strict anaerobes and promotes the digestive degradation. Various complex carbohydrates viz., lignocellulose, starch, and sugar are consumed by protozoa for energy production. Around 50% of the total biomass in the rumen is composed of protozoa. Degradation of fats, proteins, and carbohydrates is facilitated by direct engulfing (85). The lignocellulosic digestion capacity by protozoa is presumed to be the result of lateral gene transfer from the bacteria they engulf (86). Protozoa prey on selective species of bacteria, and the reason for feeding on particular bacteria is not clearly understood (87–89). Ciliates play a crucial role in fermentation and plant fiber degradation. The products obtained as a result of protozoan fermentation are found similar to that of bacteria. In contrast to bacteria, protozoa divide at a much slower rate (15–24 h). To overcome the washing out of protozoa before division, they tend to reside in the lower layers of the rumen. Many methanogens reside on the protozoan surface for H2. Hydrogen gas is produced is used for the reduction of CO2 to methane. Methanogens residing on protozoa account for around 9–25% of total rumen methane (77, 90). Protozoa are capable of engulfing and store more starch at once, which decreases acid production by lowering pH (91).
Protozoa (holotrich) produces pectin esterase, invertase, amylase, and polygalactouronase to degrade plant sugars and fibers. Protozoa also produce cellulolytic and hemicellulolytic bacteria in lower quantities compared with that of entodiniomorphids. Ciliates in the rumen secrete proteolytic enzymes, resulting in the production of AAs and ammonia. The type of engulfed microbiota decides the nitrogen metabolism of the protozoa. Generation of nitrogenous compounds in turn influences the recycling of nitrogen. Rumen ciliates also influence ammonia as well as VFA production. The symbiosis of protozoa and rumen bacteria were investigated and showed that the presence of rumen protozoa effected the bacterial composition in rumen. Absence of protozoa has a positive effect on the growth of cellulolytic and hemicellulolytic bacteria. Lambs with no protozoan population showed increased growth of wool as much as 10% when compared to lambs with rumen protozoa. No proper effect of protozoa on methane production is observed. Variations in the composition of digested material in both omasum and abomasum are observed in defaunated and faunated animals. It is an ardent fact that protozoa influence many processes in the metabolism of host (92–94).
Rumen is a repository of anaerobic fungi with an explicit capacity of lignocellulose degradation. Fungi contribute to 20% of the overall microbiota in the rumen. They are deliberate members of plant fiber degradation. Fungi also exhibit proteolytic activity. In the fungal structure, polycentric or monocentric thallus is observed, and the zoospores are polyflagellate or uniflagellate. Asexual life cycle of anaerobic fungi is mostly observed (95). Most of the fungi are not present alone in the rumen of the animals but are vividly present along the digestive tract. Fungal species were also isolated from the feces and saliva of the dairy cow. Domestic animals host Chytridiomycetes for assisting their digestion. These organisms occupy about 8% of total ruminal microbiota in the animals fed on forage, which allows more retention in the rumen (45). But in the case of high grain diets, fungal population decreases. Enzymes secreted by fungal cultures degrade lignin, hemicellulose, starch, and cellulose (33). In addition, fungi are strict anaerobes, and hence carbohydrate fermentation is the sole source of energy production. Fungi are devoid of cytochromes and mitochondria that are coplayers of oxidative phosphorylation. Despite that, they contain Hygrogenosomes that facilitate the generation of energy. Hydrogenosomes are mitochondrial derivatives that occurred during evolution, and they are not only confined to fungal genera. Various anaerobic eukaryotes and trichomonads are also found to contain this organelle. Hydrogenosomes differ from conventional mitochondria by possessing pyruvate/ferredoxin reductase instead of dehydrogenase. They also provide room for ATP production and pyruvate conversion.
Commensal interplay of fungi and bacteria is a well-studied concept. In vitro studies were carried out to understand the degradative dynamics of fungi when cocultured with cellulolytic bacteria. Cellulose degradation capacity of the fungi increases manifold with Megasphera elsdenii, Selenomonas ruminantium, and Viellonella alcalescens. Xylan consumption is increased by coculturing Neocallimastix frontalis with cellulolytic bacteria like Selenomonas ruminantium, Prevotella ruminicola, and Succinivibrio dextrinosolvens (Table 3). On the other hand, coculturing with Streptococcus brevis or Lachnospira sp. has a negative effect on xylan degradation. R. flavefaciens, and R. albus coculturing with fungi have shown adverse effects on cellulolytic activity. These bacteria release a polypeptide into the broth that has detrimental effects on cellulolytic activity of the fungus. The fungal activity in the degradation of cellulosic materials is considered minimum than that of bacteria. This might be due to their larger doubling time, inhibition by bacteria, competition for substrates, and decreased retention. Nevertheless, they exhibit remarkable activity in the degradation of lignocellulosic material, as the rhizoids pervade the cell wall of plants and make it easily accessible by the rest of the rumen microbiota (96).
Bacteriophages are obligate parasites and play a crucial role in rumen microbiota. Bacteriophages infect bacteria and lyse them after their replication (Table 3). Through lysis, the overall bacterial population is maintained in the host digestive environment. Bacterial lysis releases bacterial proteins that act as precursors of AA synthesis (97). Bacteriophages are found to vary with the organism, i.e., they are specific for a particular organism. This may be used by the researchers to destroy a particular genus of microbes from the rumen environment. Very little information is known about the bacteriophages infecting protozoans, methanogens, and archaea. It was identified that siphophages are capable of infecting methanogenic bacteria. The knowledge about the enzymatic profile and genetic makeup of rumen phages is limited and yet to be explored to manipulate the rumen environment (98).
Disturbances in the homogeneity of gastrointestinal microflora have severe effects on the digestive system and various organs. This disharmony in the communal relationship also causes various metabolic disorders, including bloat, ruminal acidosis, hypoglycemia, diarrhea, ulcers in gastrointestinal (GI) tract, and retivuloperitonitis (Figure 1).
The rumen tympany, also called as bloat, is associated with a condition in which excess gas is accumulated in the rumen. This is observed in animals fed with higher quantities of grains or forages (99), which can be categorized into free gas and frothy bloat. Free-gas bloat is associated with pathological/physical problems hindering gas release from the stomach of the dairy cow. Esophagus obstructions (external particles cloths and fruit material, etc.), cysts, blisters, tumors, thoracic or cervical enlargement, reticular dysfunction, and hypocalcemia are major conditions affecting gas belching (100–102). Frothy bloat is the result of feed ingestion, which continuously produces froth that cannot be easily expelled from the stomach. Testing with a stomach tube helps in figuring the type of bloat. If the causative agents are physical obstructions, they have to be removed manually to ensure the gas expulsion. Frothy bloat contains both hydrophobic and hydrophilic properties. The foam is the result of partial digestion of polymeric compounds including, lipopolysaccharides, fatty acids, glycans, and glycolipids. Presence of these partially digested compounds increases rumen viscosity and hinders gas removal. Gaseous distension exerts pressure on the nearby organs causing edema, pain, organ failure, and death. Several practices that are employed to treat free bloat and frothy bloat include using a stomach tube to remove gas and partially digested feed, anti-foaming agent administration, and the placement of fistula or cannula (103).
Apart from physical factors, the microbiota in the rumen also contribute to the development of gas. Gas is generated as a result of methanogenic bacterial action upon various substrates. This methane, hydrogen, and CO2 gases produced in excess when left unattended by downstream flora results in the accumulation of gas in the stomach. The hydrogen gas produced as a part of methanogen metabolism also has to be addressed. It is a well-known fact that the rumen environment is highly anaerobic. But excess CO2 can cause subtle changes in the rumen. CO2 can be reduced by methanogens to generate methane and/or as such CO2 in excess can cause tympany. It is nevertheless necessary to attend to the excess production of these gases to maintain ruminal microbial harmony. Hence to maintain the environment, probiotics can be used to replenish the rumen flora. Treated and high fiber feed also helps in relieving the stress caused by methanogenic bacteria (104).
Ruminal acidosis is caused by the consumption of more fermentable carbohydrate-rich feed material than grainy feeds (105, 106). Molasses, sugar beets, potatoes, and cereal grains result in acidosis. Fermentation of such compounds result in higher amounts of lactic acid production and hence pH of rumen is drastically reduced (107, 108). Due to this, many gram-negative bacteria are destroyed releasing endotoxin into the rumen. All these results in low pH, accumulation of fluid, disturbance of microbiota, and partial digestion. Low pH and acid production have destructive effects on the inner epithelium of the stomach causing ulcers as well as mucosal inflammation. Drastic fall in pH also inhibits the cellulolytic bacteria but enhances propionate-producing bacteria in the rumen. Rumen microbiota alteration leads to improper metabolism which can cause liver dysfunction, lung-related diseases, and can also lead to death (109–111).
Hypoglycemia is a disorder observed when the rate of glucose uptake is very less in comparison to the rate of utilization (112, 113). Vitamin B12 plays a key role in the synthesis of glucose from propionate, and its deficiency is also related to the occurrence of hypoglycemia. In new-born calves and lambs in a cold environment, hypoglycemia leads to death. Gluconeogenesis does require NADH and ATP apart from substrates made available in the ruminant environment. For this reason, an organism depends primarily on dietary carbohydrates for glucose rather than synthesis. Deficiency in glucose supply caused hypoglycemia in all the animals. On the other hand, hypoglycemia is also seen in animals whose diet is rich in inhibitors of fatty acid beta oxidation in the kidney and liver. Required amounts of AAs, fatty acids, ambience, and vitamins have to be provided for treating hypoglycemia (114–116).
Most of the fed polysaccharides should be degraded to glucose for energy production. Disharmony in the activity of rumen microbiota contributes to impaired degradation of polysaccharides that in turn affects glucose turnover. Proper diet at regular intervals with the maintenance of a favorable environment and supplementing cellulolytic bacteria may also address this issue in less severe conditions (117, 118).
Ulcers in the dairy cow are more common in the duodenum and abomasum. They are often observed in cows and buffaloes than in sheep (119, 120). Ulcers are mostly associated with improper feed intake, over grazing stress, microbial infection, and malnutrition. These occur in concomitance with other diseases, viz., salmonellosis and blue tongue (Clostridium perfringens abomasitis). Over usage of non-steroidal antiinflammatory drugs can also cause ulcers. Perforating ulcers are generally more infectious and have adverse effects on the epithelium of gastrointestinal tract than non-perforating ulcers (121).
The disruption of the outer epithelium of gastrointestinal tract is caused by acid production and can be alleviated by the administration of probiotics containing lactic acid bacteria. Antihistamine with iron injection can also reduce the pain and bleeding in adult ruminants (122).
Reticuloperitonitis, also called as traumatic reticulitis or hardware disease, is mainly observed in cattle with unsystematic feeding (123, 124). Indiscriminate feeding habits of dairy cow leads to the disturbances in the harmony of rumen microbiota. Continuous feeding deters bacterial revival and causes improper digestion which may lead to bloat and ruminal acidosis. It is a noncontagious disease which if not properly observed causes devastating effects. Proper dietary consumption at regular intervals will enable bacterial resurgence and revival. Usage of probiotic syrups, administration of antibiotics, and digestive aids may help in the initial stages and rumenotomy is suggested during severity index (125).
Diarrhea is a severe problem prevalent in young calf. It is associated with various symptoms including disturbance of electrolyte balance, dehydration, and weakness. The reason for the disease varies with geographical location, type of feed, type of infection, and host metabolic issues. In most of the time, the disease occurrence is multifactorial. Pathogens namely, bacteria, virus, parasites, and protozoa can trigger infection. Infection by bacterial diarrhea includes Enterobacter sp. mycobacterium paratuberculosis, Clostridium perfringens, Salmonella sp. as well as Staphylococcus. Rotavirus and adenoviruses contribute to viral infections. Trichonema sp. and Strongylus sp. are major parasites infecting the gastrointestinal tract of the dairy cow. Nonetheless, Trichomonas sp., Entamoeba sp., and Giardia sp. contribute to protozoan infection. Infection of the ruminant flora by either of the above species causes disturbance in the homogeneity and functionality, culminating in disease. Malabsorption or hypersecretion of fluids into the gut usually results in the secretion of excessive fluid from the intestine. Severe outflux of fluids with salts leads to weakness. Things to be observed to treat diarrhea are suppressing the infection and adjusting physiological imbalance. This allows eradication of the causative agent helping in faster recovery. Usage of antibiotic drugs will also help in wiping out the existing infection and maintaining the functional role of microflora (126).
Gut microbiota plays a crucial role in ruminant digestion as well as energy production. Hence it is essential to study the genomic environment to predict the changes that cause genetic and metabolic disorders. But it is difficult to isolate and study the genome of a particular flora in the consortium. For this reason, the whole genome of the consortium is studied under the branch metagene “omics”. The complete genome of the gut microflora, termed as gut microbiome, is obtained by sequencing methodologies and omics approaches (127). The identity of the microbiome is determined by general sequencing protocols, whereas “omics” determine the actual functionality of the microbiome present in rumen. Omics approaches embrace metabolomics, metaproteomics, metatranscriptomics, and metagenomics. The relationship between host and microbiota is well-studied by omics approaches. For instance, metagenomics approaches revealed that Bacteroidetes is energetically less favorable to the host in comparison to Firmicutes. The action of Firmicutes increases nutrient availability to the host, which culminated in obesity.
Role of omics in the physiology and functionality of livestock is an area which is yet to be explored. Many omics-related approaches succeeded in finding the relation between microbiome composition and livestock production (128). These studies also helped in revealing taxonomical differences in the ruminal microenvironment of the organisms based on the dietary changes and environmental variations (22, 129). Recent studies on profiling microbiome of the rumen in a large sample set (>700) revealed a diet-dependent relationship between the host and microflora. The type of feed ingest decides the flora in the rumen (130). In depth analysis of the rumen microbiome using omics approaches helps in identifying markers that decide the variability in feed efficiency in cattle. Omics-based studies also help in assessing colonization patterns in the dairy cow.
In the last few decades, the role of GI microbiota in health and disease has become the focal point of many studies. Involvement of gut microbiota in digestion and various diseases in humans is well-studied. However, in the case of dairy cows, the underlying mechanisms of host–microbial interactions are yet to be uncovered. The interaction of rumen or gut microflora is purely symbiotic in which one organism benefits the others. The higher organisms lost the capacity to degrade plant cell wall and other materials during evolution to use it as a source of energy. Hence, ruminants employed microorganisms to digest plant materials and in turn provided them nutrients required for survival. Several types of microorganisms reside in the rumen and gut of the dairy cow. These organisms are from all the main groups such as bacteria, protozoa, archaea fungi, and bacteriophages. Composition of rumen microbiota varies with the geographical location and type of regular feed. However, the dominant strains in the rumen environment are always conserved. Surprisingly, the microbiota adapts to the feed intake and changes its constitution to meet the requirement of the host. Bacteria occupy a major part of the ruminal microflora. Microorganisms are adopted in such a way that most of the energy is extracted from the provided substrate. Collaborative action of various species of organisms helps in proper digestion and energy production. The end product of one organism acts as a substrate for the secondary organism. In this manner, the degradation of the plant fiber is carried out to harvest maximum energy from the ingest.
To understand the metabolic disease of dairy cow, many factors have to be taken into consideration. This should start with the type of feed, interval of feed, grazing area, and response of the ruminant system to various drugs. Rumen microflora are the crucial role players in the digestion as well as energy generation for the dairy cow. Hence, it is nevertheless necessary for a dairy cow to maintain the ambience in the GI tract to ensure the proper symbiotic relationship with the resident bacteria. Infection by pathogens can lead to disharmony in the commensalism of the bacteria that culminates in various diseases. Prior identification of the infection, proper care, and treatment are required to rescue the organism. Preventive measures like proper ingest, probiotic supplementation, and vaccination protect the organisms from infections, thereby increasing the productivity. In depth analysis of microbiome using omics approaches helps in attaining knowledge about gut microbial mechanisms and functional activities at various conditions. Also, the variations in the gut microbiome have a strong impact on the phenotypic definition and physiology of the host. Gut microbiota has an influence on the health and productivity of dairy cow. Future studies in multiomics provide a platform to determine the physiological and phenotypical upgradation of the dairy cow for milk production.
QX and QQ wrote and prepared the original draft. YG, JH, MH, and YD edited the manuscript. QX, KZ, and XL critically reviewed the manuscript. All authors reviewed and approved the final manuscript.
This work was supported by grants from the Fundamental Research Funds for the Central Universities (2662019QD021), the Innovation Team of Development and Research on Food Technology of Fuyang Local Agricultural Products (FXKCT02), the State Key Laboratory of Animal Nutrition (2004DA125184F1906), the Open Project Program of Key Laboratory of Feed Biotechnology, Key Laboratory of Molecular Animal Nutrition of Zhejiang University, and the National Natural Science Foundation of China (C31802087).
The authors declare that the research was conducted in the absence of any commercial or financial relationships that could be construed as a potential conflict of interest.
All claims expressed in this article are solely those of the authors and do not necessarily represent those of their affiliated organizations, or those of the publisher, the editors and the reviewers. Any product that may be evaluated in this article, or claim that may be made by its manufacturer, is not guaranteed or endorsed by the publisher.
1. Russell JB. “Rumen,” in Encyclopedia of Microbiology, ed. M. B. T.-E. of M. Third E. Schaechter Oxford: Elsevier p. 163–174. doi: 10.1016/B978-012373944-5.00061-4
2. Mackie RI. Mutualistic Fermentative Digestion in the Gastrointestinal Tract: Diversity and Evolution. Integr Comp Biol. (2002) 42:319–26. doi: 10.1093/icb/42.2.319
3. Choudhury P, Sirohi S, Puniya A, Chaudhary PP. “Harnessing the Diversity of Rumen Microbes using Molecular Approaches,” in Livestock Green House Gases: emission and options for mitigation. Satish Serial Publishing House p. 65–82.
4. Oltjen JW, Beckett JL. Role of ruminant livestock in sustainable agricultural systems. J Anim Sci. (1996) 74:1406. doi: 10.2527/1996.7461406x
5. Krause DO, Denman SE, Mackie RI, Morrison M, Rae AL, Attwood GT, et al. Opportunities to improve fiber degradation in the rumen: microbiology, ecology, and genomics. FEMS Microbiol Rev. (2003) 27:663–93. doi: 10.1016/S0168-6445(03)00072-X
6. Castillo-González A, Burrola-Barraza M, Domínguez-Viveros J, Chávez-Martínez A. Rumen microorganisms and fermentation. Arch Med Vet. (2014) 46:349–61. doi: 10.4067/S0301-732X2014000300003
7. Sahu NP, Kamra DN. Microbial eco-system of the gastro-intestinal tract of wild herbivorous animals. J Appl Anim Res. (2002) 21:207–30. doi: 10.1080/09712119.2002.9706370
8. Castro-Montoya JM, Makkar HPS, Becker K. Chemical composition of rumen microbial fraction and fermentation parameters as affected by tannins and saponins using an in vitro rumen fermentation system. Can J Anim Sci. (2011) 91:433–48. doi: 10.4141/cjas2010-028
9. Hillman ET, Lu H, Yao T, Nakatsu CH. Microbial ecology along the gastrointestinal tract. Microbes Environ. (2017) 32:300–13. doi: 10.1264/jsme2.ME17017
10. Firkins JL, Yu Z, Park T, Plank JE. Extending Burk Dehority's perspectives on the role of ciliate protozoa in the rumen. Front Microbiol. (2020) 11:123. doi: 10.3389/fmicb.2020.00123
11. Wahrmund JL, Ronchesel JR, Krehbiel CR, Goad CL, Trost SM, Richards CJ. Ruminal acidosis challenge impact on ruminal temperature in feedlot cattle1. J Anim Sci. (2012) 90:2794–801. doi: 10.2527/jas.2011-4407
12. Liu K, Zhang Y, Yu Z, Xu Q, Zheng N, Zhao S, et al. Ruminal microbiota–host interaction and its effect on nutrient metabolism. Anim Nutr. (2021) 7:49–55. doi: 10.1016/j.aninu.2020.12.001
13. Matthews C, Crispie F, Lewis E, Reid M, O'Toole PW, Cotter PD. The rumen microbiome: A crucial consideration when optimising milk and meat production and nitrogen utilisation efficiency. Gut Microbes. (2019) 10:115–32. doi: 10.1080/19490976.2018.1505176
14. Burns JC, ASAS. Centennial Paper: Utilization of pasture and forages by ruminants: A historical perspective1. J Anim Sci. (2008) 86:3647–63. doi: 10.2527/jas.2008-1240
15. Kingston-Smith AH, Marshall AH, Moorby JM. Breeding for genetic improvement of forage plants in relation to increasing animal production with reduced environmental footprint. Animal. (2013) 7:79–88. doi: 10.1017/S1751731112000961
16. McAllister TA, Bae HD, Jones GA, Cheng K-J. Microbial attachment and feed digestion in the rumen. J Anim Sci. (1994) 72:3004–18. doi: 10.2527/1994.72113004x
17. Merchen NR, Elizalde JC, Drackley JK. Current perspective on assessing site of digestion in ruminants. J Anim Sci. (1997) 75:2223–34. doi: 10.2527/1997.7582223x
18. Hoover WH, Miller TK. Rumen digestive physiology and microbial ecology. Vet Clin North Am Food Anim Pract. (1991) 7:311–25. doi: 10.1016/S0749-0720(15)30801-X
19. Weimer PJ. Why don't ruminal bacteria digest cellulose faster? J Dairy Sci. (1996) 79:1496–502. doi: 10.3168/jds.S0022-0302(96)76509-8
20. Jollès P, Schoentgen F, Jollès J, Dobson DE, Prager EM, Wilson AC. Stomach lysozymes of ruminants. II Amino acid sequence of cow lysozyme 2 and immunological comparisons with other lysozymes. J Biol Chem. (1984) 259:11617–25. doi: 10.1016/S0021-9258(18)90908-7
21. Jollès J, Jolles P, Bowman BH, Prager EM, Stewart C-B, Wilson AC. Episodic evolution in the stomach lysozymes of ruminants. J Mol Evol. (1989) 28:528–35. doi: 10.1007/BF02602933
22. Li F, Li C, Chen Y, Liu J, Zhang C, Irving B, et al. Host genetics influence the rumen microbiota and heritable rumen microbial features associate with feed efficiency in cattle. Microbiome. (2019) 7:92. doi: 10.1186/s40168-019-0699-1
23. Suttle N. “NUTRIENTS, DIGESTION AND ABSORPTION | Absorption of Minerals and Vitamins,” In: Encyclopedia of Dairy Sciences, ed. H. B. T.-E. of D. S. Roginski. Oxford: Elsevier p. 2127–2132. doi: 10.1016/B0-12-227235-8/00363-1
24. Flint HJ, Bayer EA. Plant Cell Wall Breakdown by Anaerobic Microorganisms from the Mammalian Digestive Tract. Ann N Y Acad Sci. (2008) 1125:280–8. doi: 10.1196/annals.1419.022
25. Flint HJ, Bayer EA, Rincon MT, Lamed R, White BA. Polysaccharide utilization by gut bacteria: potential for new insights from genomic analysis. Nat Rev Microbiol. (2008) 6:121–31. doi: 10.1038/nrmicro1817
26. Ozutsumi Y, Tajima K, Takenaka A, Itabashi H. The effect of protozoa on the composition of rumen bacteria in cattle using 16S rRNA gene clone libraries. Biosci Biotechnol Biochem. (2005) 69:499–506. doi: 10.1271/bbb.69.499
27. Mcallister TA, Rode LM, Major DJ, Cheng K-J, Buchanan-Smith JG. Effect of ruminal microbial colonization on cereal grain digestion. Can J Anim Sci. (1990) 70:571–9. doi: 10.4141/cjas90-069
28. Kay RNB. Digestion of protein in the intestines of adult ruminants. Proc Nutr Soc. (1969) 28:140–51. doi: 10.1079/PNS19690025
29. Strom E, Øskov ER. The nutritive value of rumen micro-organisms in ruminants. Br J Nutr. (1984) 52:613–20. doi: 10.1079/BJN19840128
30. Russell JB, Muck RE, Weimer PJ. Quantitative analysis of cellulose degradation and growth of cellulolytic bacteria in the rumen. FEMS Microbiol Ecol. (2009) 67:183–97. doi: 10.1111/j.1574-6941.2008.00633.x
31. Weimer PJ, Russell JB, Muck RE. Lessons from the cow: What the ruminant animal can teach us about consolidated bioprocessing of cellulosic biomass. Bioresour Technol. (2009) 100:5323–31. doi: 10.1016/j.biortech.2009.04.075
32. Bi Y, Cox MS, Zhang F, Suen G, Zhang N, Tu Y, et al. Feeding modes shape the acquisition and structure of the initial gut microbiota in newborn lambs. Environ Microbiol. (2019) 21:2333–46. doi: 10.1111/1462-2920.14614
33. Jami E, Israel A, Kotser A, Mizrahi I. Exploring the bovine rumen bacterial community from birth to adulthood. ISME J. (2013) 7:1069–79. doi: 10.1038/ismej.2013.2
34. Malmuthuge N, Li M, Goonewardene LA, Oba M, Guan LL. Effect of calf starter feeding on gut microbial diversity and expression of genes involved in host immune responses and tight junctions in dairy calves during weaning transition. J Dairy Sci. (2013) 96:3189–200. doi: 10.3168/jds.2012-6200
35. Fonty G, Gouet P, Jouany J-P, Senaud J. Establishment of the microflora and anaerobic fungi in the rumen of lambs. Microbiology. (1987) 133:1835–43. doi: 10.1099/00221287-133-7-1835
36. Dill-McFarland KA, Breaker JD, Suen G. Microbial succession in the gastrointestinal tract of dairy cows from 2 weeks to first lactation. Sci Rep. (2017) 7:40864. doi: 10.1038/srep40864
37. Li F, Wang Z, Dong C, Li F, Wang W, Yuan Z, et al. Rumen bacteria communities and performances of fattening lambs with a lower or greater subacute ruminal acidosis risk. Front Microbiol. (2017) 8:1–10. doi: 10.3389/fmicb.2017.02506
38. Zhou M, Chen Y, Guan LL. “Rumen Bacteria,” in Rumen Microbiology: From Evolution to Revolution (New Delhi: Springer India), 79–95. doi: 10.1007/978-81-322-2401-3_6
39. Brulc JM, Antonopoulos DA, Berg Miller ME, Wilson MK, Yannarell AC, Dinsdale EA, et al. Gene-centric metagenomics of the fiber-adherent bovine rumen microbiome reveals forage specific glycoside hydrolases. Proc Natl Acad Sci. (2009) 106:1948–53. doi: 10.1073/pnas.0806191105
40. Mizrahi I, Jami E. Review: The compositional variation of the rumen microbiome and its effect on host performance and methane emission. Animal. (2018) 12:s220–32. doi: 10.1017/S1751731118001957
41. Cai S, Li J, Hu FZ, Zhang K, Luo Y, Janto B, et al. Cellulosilyticum ruminicola, a newly described rumen bacterium that possesses redundant fibrolytic-protein-encoding genes and degrades lignocellulose with multiple carbohydrate- borne fibrolytic enzymes. Appl Environ Microbiol. (2010) 76:3818–24. doi: 10.1128/AEM.03124-09
42. Kalmokoff ML, Teather RM. Isolation and characterization of a bacteriocin (Butyrivibriocin AR10) from the ruminal anaerobe Butyrivibrio fibrisolvens AR10: evidence in support of the widespread occurrence of bacteriocin-like activity among ruminal isolates of B. fibrisolvens Appl Environ Microbiol. (1997) 63:394–402. doi: 10.1128/aem.63.2.394-402.1997
43. Saluzzi L, Smith A, Stewart CS. Analysis of bacterial phospholipid markers and plant monosaccharides during forage degradation by Ruminococcus flavefaciens and Fibrobacter succinogenes in co-culture. J Gen Microbiol. (1993) 139:2865–73. doi: 10.1099/00221287-139-11-2865
44. Odenyo AA, Mackie RI, Stahl DA, White BA. The use of 16S rRNA-targeted oligonucleotide probes to study competition between ruminal fibrolytic bacteria: development of probes for Ruminococcus species and evidence for bacteriocin production. Appl Environ Microbiol. (1994) 60:3688–96. doi: 10.1128/aem.60.10.3688-3696.1994
45. Rychlik JL, Russell JB. Bacteriocin-like activity of Butyrivibrio fibrisolvens JL5 and its effect on other ruminal bacteria and ammonia production. Appl Environ Microbiol. (2002) 68:1040–6. doi: 10.1128/AEM.68.3.1040-1046.2002
46. Chen J, Stevenson DM, Weimer PJ, Albusin B. A bacteriocin from the ruminal bacterium ruminococcus albus 7 that inhibits growth of ruminococcus flavefaciens. Appl Environ Microbiol. (2004) 70:3167–70. doi: 10.1128/AEM.70.5.3167-3170.2004
47. Erickson DL, Nsereko VL, Morgavi DP, Selinger LB, Rode LM, Beauchemin KA. Evidence of quorum sensing in the rumen ecosystem: detection of N-acyl homoserine lactone autoinducers in ruminal contents. Can J Microbiol. (2002) 48:374–8. doi: 10.1139/w02-022
48. Mitsumori M, Xu L, Kajikawa H, Kurihara M, Tajima K, Hai J, et al. Possible quorum sensing in the rumen microbial community: detection of quorum-sensing signal molecules from rumen bacteria. FEMS Microbiol Lett. (2003) 219:47–52. doi: 10.1016/S0378-1097(02)01192-8
49. Callaway TR, Dowd SE, Edrington TS, Anderson RC, Krueger N, Bauer N, et al. Evaluation of bacterial diversity in the rumen and feces of cattle fed different levels of dried distillers grains plus solubles using bacterial tag-encoded FLX amplicon pyrosequencing1. J Anim Sci. (2010) 88:3977–83. doi: 10.2527/jas.2010-2900
50. Fernando SC, Purvis HT, Najar FZ, Sukharnikov LO, Krehbiel CR, Nagaraja TG, et al. Rumen microbial population dynamics during adaptation to a high-grain diet. Appl Environ Microbiol. (2010) 76:7482–90. doi: 10.1128/AEM.00388-10
51. Johns A. Isolation of a bacterium, producing propionic acid, from the rumen of sheep. J Gen Microbiol. (1951) 5:317–25. doi: 10.1099/00221287-5-2-317
52. Elsden SR, Volcani BE, Gilchrist FMC, Lewis D. Properties of a fatty acid forming organism isolated from the rumen of sheep. J Bacteriol. (1956) 72:681–689. doi: 10.1128/jb.72.5.681-689.1956
53. Muegge BD, Kuczynski J, Knights D, Clemente JC, Gonzalez A, Fontana L, et al. Diet drives convergence in gut microbiome functions across mammalian phylogeny and within humans. Science (80-). (2011) 332:970–4. doi: 10.1126/science.1198719
54. Stevenson DM, Weimer PJ. Dominance of prevotella and low abundance of classical ruminal bacterial species in the bovine rumen revealed by relative quantification real-time PCR. Appl Microbiol Biotechnol. (2007) 75:165–74. doi: 10.1007/s00253-006-0802-y
55. Jeyanathan J, Kirs M, Ronimus RS, Hoskin SO, Janssen PH. Methanogen community structure in the rumens of farmed sheep, cattle and red deer fed different diets. FEMS Microbiol Ecol. (2011) 76:311–26. doi: 10.1111/j.1574-6941.2011.01056.x
56. Pfister P, Wasserfallen A, Stettler R, Leisinger T. Molecular analysis of Methanobacterium phage ΨM2. Mol Microbiol. (1998) 30:233–44. doi: 10.1046/j.1365-2958.1998.01073.x
57. Luo Y, Pfister P, Leisinger T, Wasserfallen A. The genome of archaeal prophage ΨM100 encodes the lytic enzyme responsible for autolysis ofmethanothermobacter wolfeii. J Bacteriol. (2001) 183:5788–92. doi: 10.1128/JB.183.19.5788-5792.2001
58. Kamra DN. Rumen microbial ecosystem. Curr Sci. (2005) 89:124–135. Available at: http://www.jstor.org/stable/24110438
59. Wright A-DG, Klieve AV. Does the complexity of the rumen microbial ecology preclude methane mitigation? Anim Feed Sci Technol. (2011) 166–167:248–253. doi: 10.1016/j.anifeedsci.2011.04.015
60. Kumar S, Choudhury PK, Carro MD, Griffith GW, Dagar SS, Puniya M, et al. New aspects and strategies for methane mitigation from ruminants. Appl Microbiol Biotechnol. (2014) 98:31–44. doi: 10.1007/s00253-013-5365-0
61. Fuentes MC, Calsamiglia S, Cardozo PW, Vlaeminck B. Effect of pH and level of concentrate in the diet on the production of biohydrogenation intermediates in a dual-flow continuous culture. J Dairy Sci. (2009) 92:4456–66. doi: 10.3168/jds.2008-1722
62. Duskova D, Marounek M. Fermentation of pectin and glucose, and activity of pectin-degrading enzymes in the rumen bacterium Lachnospira multiparus. Lett Appl Microbiol. (2001) 33:159–63. doi: 10.1046/j.1472-765x.2001.00970.x
63. Sales-Duval M, Lucas F, Blanchart G. Effects of exogenous ammonia or free amino acids on proteolytic activity and protein breakdown products in streptococcus bovis, prevotella albensis, and butyrivibrio fibrisolvens. Curr Microbiol. (2002) 44:435–43. doi: 10.1007/s00284-001-0013-9
64. Cotta MA, Hespell RB. Proteolytic activity of the ruminal bacterium Butyrivibrio fibrisolvens. Appl Environ Microbiol. (1986) 52:51–8. doi: 10.1128/aem.52.1.51-58.1986
65. Yanagita K, Kamagata Y, Kawaharasaki M, Suzuki T, Nakamura Y, Minato H. Phylogenetic Analysis of Methanogens in Sheep Rumen Ecosystem and Detection of Methanomicrobium mobile by Fluorescence In Situ Hybridization. Biosci Biotechnol Biochem. (2000) 64:1737–42. doi: 10.1271/bbb.64.1737
66. Hook SE, Wright A-DG, McBride BW. Methanogens: methane producers of the rumen and mitigation strategies. Archaea. (2010) 2010:1–11. doi: 10.1155/2010/945785
67. Coleman GS. The metabolism of free amino acids by washed suspensions of the rumen ciliate entodinium caudatum. J Gen Microbiol. (1967) 47:433–47. doi: 10.1099/00221287-47-3-433
68. Moniello G, Richardson AJ, Duncan SH, Stewart CS. Effects of coumarin and sparteine on attachment to cellulose and cellulolysis by Neocallimastix frontalis RE1. Appl Environ Microbiol. (1996) 62:4666–8. doi: 10.1128/aem.62.12.4666-4668.1996
69. Hodrová B, Kopečný J, Petr O. Interaction of the rumen fungus Orpinomyces joyonii with Megasphaera elsdenii and Eubacterium limosum. Lett Appl Microbiol. (1995) 21:34–7. doi: 10.1111/j.1472-765X.1995.tb01001.x
70. Dashtban M, Schraft H, Qin W. Fungal bioconversion of lignocellulosic residues; opportunities & perspectives. Int J Biol Sci. (2009) 5:578–95. doi: 10.7150/ijbs.5.578
71. Gorris LG, van der Drift C. Cofactor contents of methanogenic bacteria reviewed. Biofactors. (1994) 4:139–45. Available at: http://www.ncbi.nlm.nih.gov/pubmed/7916957
72. Thauer RK, Kaster A-K, Seedorf H, Buckel W, Hedderich R. Methanogenic archaea: ecologically relevant differences in energy conservation. Nat Rev Microbiol. (2008) 6:579–91. doi: 10.1038/nrmicro1931
73. Hungate RE, Smith W, Bauchop T, Yu I, Rabinowitz JC. Formate as an intermediate in the bovine rumen fermentation. J Bacteriol. (1970) 102:389–97. doi: 10.1128/jb.102.2.389-397.1970
74. Stams AJM, Plugge CM. Electron transfer in syntrophic communities of anaerobic bacteria and archaea. Nat Rev Microbiol. (2009) 7:568–77. doi: 10.1038/nrmicro2166
75. Latham MJ, Wolin MJ. Fermentation of cellulose by Ruminococcus flavefaciens in the presence and absence of Methanobacterium ruminantium. Appl Environ Microbiol. (1977) 34:297–301. doi: 10.1128/aem.34.3.297-301.1977
76. Pavlostathis SG, Miller TL, Wolin MJ. Kinetics of insoluble cellulose fermentation by continuous cultures of Ruminococcus albus. Appl Environ Microbiol. (1988) 54:2660–3. doi: 10.1128/aem.54.11.2660-2663.1988
77. Vogels GD, Hoppe WF, Stumm CK. Association of methanogenic bacteria with rumen ciliates. Appl Environ Microbiol. (1980) 40:608–12. doi: 10.1128/aem.40.3.608-612.1980
78. Krumholz LR, Forsberg CW, Veira DM. Association of methanogenic bacteria with rumen protozoa. Can J Microbiol. (1983) 29:676–80. doi: 10.1139/m83-110
79. Tóthová T, Piknová M, Kišidayová S, Javorský P, Pristaš P. Distinctive archaebacterial species associated with anaerobic rumen protozoan Entodinium caudatum. Folia Microbiol (Praha). (2008) 53:259–62. doi: 10.1007/s12223-008-0039-5
80. Joblin KN, Naylor GE, Williams AG. Effect of methanobrevibacter smithii on xylanolytic activity of anaerobic ruminal fungi. Appl Environ Microbiol. (1990) 56:2287–95. doi: 10.1128/aem.56.8.2287-2295.1990
81. Marvin-Sikkema FD, Richardson AJ, Stewart CS, Gottschal JC, Prins RA. Influence of hydrogen-consuming bacteria on cellulose degradation by anaerobic fungi. Appl Environ Microbiol. (1990) 56:3793–3797. doi: 10.1128/aem.56.12.3793-3797.1990
82. Teunissen MJ, Kets EPW, Op den Camp HJM, Huis in't Veld JHJ, Vogels GD. Effect of coculture of anaerobic fungi isolated from ruminants and non-ruminants with methanogenic bacteria on cellulolytic and xylanolytic enzyme activities. Arch Microbiol. (1992) 157:176–82. doi: 10.1007/BF00245287
84. Dehority BA. Effects of microbial synergism on fibre digestion in the rumen. Proc Nutr Soc. (1991) 50:149–59. doi: 10.1079/PNS19910026
85. Stewart CS, Duncan SH, Richardson AJ, Backwell C, Begbie R. The inhibition of fungal cellulolysis by cell-free preparations from ruminococci. FEMS Microbiol Lett. (1992) 97:83–7. doi: 10.1111/j.1574-6968.1992.tb05444.x
86. Ricard G, McEwan NR, Dutilh BE, Jouany J-P, Macheboeuf D, Mitsumori M, et al. Horizontal gene transfer from Bacteria to rumen Ciliates indicates adaptation to their anaerobic, carbohydrates-rich environment. BMC Genomics. (2006) 7:22. doi: 10.1186/1471-2164-7-22
87. Gutierrez J. Observations on Bacterial Feeding by the Rumen Ciliate Isotricha prostoma. J Protozool. (1958) 5:122–6. doi: 10.1111/j.1550-7408.1958.tb02538.x
88. Gutierrez J, Davis RE. Bacterial ingestion by the rumen ciliates entodinium and diplodinium. J Protozool. (1959) 6:222–6. doi: 10.1111/j.1550-7408.1959.tb04361.x
89. Mah RA. Factors influencing the in vitro culture of the rumen ciliate ophryoscolex purkynei stein. J Protozool. (1964) 11:546–52. doi: 10.1111/j.1550-7408.1964.tb01796.x
90. Newbold CJ, Lassalas B, Jouany JP. The importance of methanogens associated with ciliate protozoa in ruminal methane production in vitro. Lett Appl Microbiol. (1995) 21:230–4. doi: 10.1111/j.1472-765X.1995.tb01048.x
91. Ranilla MJ, Jouany J-P, Morgavi DP. Methane production and substrate degradation by rumen microbial communities containing single protozoal species in vitro. Lett Appl Microbiol. (2007) 45:675–80. doi: 10.1111/j.1472-765X.2007.02251.x
92. Sirohi SK, Choudhury PK, Dagar SS, Puniya AK, Singh D. Isolation, characterization and fibre degradation potential of anaerobic rumen fungi from cattle. Ann Microbiol. (2013) 63:1187–94. doi: 10.1007/s13213-012-0577-6
93. Sirohi SK, Singh N, Dagar SS, Puniya AK. Molecular tools for deciphering the microbial community structure and diversity in rumen ecosystem. Appl Microbiol Biotechnol. (2012) 95:1135–54. doi: 10.1007/s00253-012-4262-2
94. Sirohi SK, Chaudhary PP, Singh N, Singh D, Puniya AK. The 16S rRNA and mcrA gene based comparative diversity of methanogens in cattle fed on high fibre based diet. Gene. (2013) 523:161–6. doi: 10.1016/j.gene.2013.04.002
95. Dehority BA, Tirabasso PA. Antibiosis between Ruminal Bacteria and Ruminal Fungi. Appl Environ Microbiol. (2000) 66:2921–7. doi: 10.1128/AEM.66.7.2921-2927.2000
96. Bernalier A, Fonty G, Bonnemoy F, Gouet P. Inhibition of the cellulolytic activity of Neocallimastix frontalis by Ruminococcus flavefaciens. J Gen Microbiol. (1993) 139:873–80. doi: 10.1099/00221287-139-4-873
97. Klieve AV, Heck GL, Prance MA, Shu Q. Genetic homogeneity and phage susceptibility of ruminal strains of Streptococcus bovis isolated in Australia. Lett Appl Microbiol. (1999) 29:108–12. doi: 10.1046/j.1365-2672.1999.00596.x
98. McAllister TA, Newbold CJ. Redirecting rumen fermentation to reduce methanogenesis. Aust J Exp Agric. (2008) 48:7. doi: 10.1071/EA07218
99. Shenkoru T, Faciola A, Schultz B. Perryman B. Frothy Bloat (Primary Ruminal Tympany) Potential and Nutrient Content of Forage Kochia (Bassia prostrata L). J Arid Land Studies. (2015) 180:177–80.
100. Kaba T, Abera B, Kassa T. Esophageal groove dysfunction: a cause of ruminal bloat in newborn calves. BMC Vet Res. (2018) 14:276. doi: 10.1186/s12917-018-1573-2
101. Clarke RTJ, Reid CSW. Foamy Bloat of Cattle. A Review J Dairy Sci. (1974) 57:753–85. doi: 10.3168/jds.S0022-0302(74)84964-7
102. Priyanka M, Dey S. Ruminal impaction due to plastic materials - An increasing threat to ruminants and its impact on human health in developing countries. Vet World. (2018) 11:1307–15. doi: 10.14202/vetworld.2018.1307-1315
103. Rahman MM, Bhuiyan MMU, Islam MT, Shamsuddin M. Efficacy of simethicone for treatment of bloat in ruminants. Asian J Med Biol Res. (2017) 2:635–8. doi: 10.3329/ajmbr.v2i4.31008
104. Dougherty RW. Bloat in Ruminants. Nutr Rev. (2009) 16:300–1. doi: 10.1111/j.1753-4887.1958.tb00631.x
105. Hernández J, Benedito JL, Abuelo A, Castillo C. Ruminal acidosis in feedlot: from aetiology to prevention. Sci World J. (2014) 2014:702572. doi: 10.1155/2014/702572
106. Nagaraja TG, Titgemeyer EC. Ruminal acidosis in beef cattle: the current microbiological and nutritional outlook. J Dairy Sci. (2007) 90:E17–38. doi: 10.3168/jds.2006-478
107. Abdela N. Sub-acute Ruminal Acidosis (SARA) and its consequence in dairy cattle: a review of past and recent research at global prospective. Achiev Life Sci. (2016) 10:187–96. doi: 10.1016/j.als.2016.11.006
108. Jaramillo-López E, Itza-Ortiz MF, Peraza-Mercado G, Carrera-Chávez JM. Ruminal acidosis: strategies for its control. Austral J Vet Sci. (2017) 49:139–48. doi: 10.4067/S0719-81322017000300139
109. Krause KM, Oetzel GR. Inducing subacute ruminal acidosis in lactating dairy cows. J Dairy Sci. (2005) 88:3633–9. doi: 10.3168/jds.S0022-0302(05)73048-4
110. Plaizier JC, Krause DO, Gozho GN, McBride BW. Subacute ruminal acidosis in dairy cows: The physiological causes, incidence and consequences. Vet J. (2008) 176:21–31. doi: 10.1016/j.tvjl.2007.12.016
111. Kleen JL, Hooijer GA, Rehage J, Noordhuizen JPTM. Subacute ruminal acidosis (sara): a review. J Vet Med Ser A. (2003) 50:406–14. doi: 10.1046/j.1439-0442.2003.00569.x
112. Klein KA, Clark C, Allen AL. Hypoglycemia in sick and moribund farmed elk calves. Can Vet J = La Rev Vet Can. (2002) 43:778–781. Available at: https://pubmed.ncbi.nlm.nih.gov/12395760
113. Kronfeld DS. Hypoglycemia in ketotic cows. J Dairy Sci. (1971) 54:949–61. doi: 10.3168/jds.S0022-0302(71)85951-9
114. Trefz FM, Lorenz I, Lorch A, Constable PD. Clinical signs, profound acidemia, hypoglycemia, and hypernatremia are predictive of mortality in 1,400 critically ill neonatal calves with diarrhea. PLoS ONE. (2017) 12:1–27. doi: 10.1371/journal.pone.0182938
115. Qaid MM, Abdelrahman MM. Role of insulin and other related hormones in energy metabolism: A review. Cogent Food Agric. (2016) 2. doi: 10.1080/23311932.2016.1267691
116. Aschenbach JR, Kristensen NB, Donkin SS, Hammon HM, Penner GB. Gluconeogenesis in dairy cows: The secret of making sweet milk from sour dough. IUBMB Life. (2010) 62:869–77. doi: 10.1002/iub.400
117. Wu G. “Management of metabolic disorders (including metabolic diseases) in ruminant and nonruminant animals,” in Animal Agriculture (Elsevier), 471–491.
118. Yuan L, Hensley C, Mahsoub HM, Ramesh AK, Zhou P. Microbiota in viral infection and disease in humans and farm animals. 1st ed Elsevier Inc. (2020). doi: 10.1016/bs.pmbts.2020.04.005
119. Sanz-Fernandez MV, Daniel J-B, Seymour DJ, Kvidera SK, Bester Z, Doelman J, et al. Targeting the Hindgut to Improve Health and Performance in Cattle. Animals. (2020) 10:1817. doi: 10.3390/ani10101817
120. Peek SF, Mcguirk SM, Sweeney RW, Cummings KJ. “6 - Infectious Diseases of the Gastrointestinal Tract,” in, eds. S. F. Peek, T. J. B. T.-R. D. of D. C. Third E. Divers. Elsevier. p 249–356. doi: 10.1016/B978-0-323-39055-2.00006-1
121. Belknap EB, Navarre CB. Differentiation of gastrointestinal diseases in adult cattle. Vet Clin North Am Food Anim Pract. (2000) 16:59–86. doi: 10.1016/S0749-0720(15)30137-7
122. Fecteau M-E, Whitlock RH. “Abomasal Ulcers,” in Food Animal Practice, eds. D. E. Anderson, D. M. B. T.-F. A. P. Fifth E. Rings. Saint Louis: Elsevier. p. 29–34. doi: 10.1016/B978-141603591-6.10010-7
123. El-Ashker M, Salama M, El-Boshy M. Traumatic reticuloperitonitis in water buffalo (Bubalus bubalis): clinical findings and the associated inflammatory response. J Vet Med. (2013) 2013:808656. doi: 10.1155/2013/808656
124. Braun U, Gerspach C, Ohlerth S, Warislohner S, Nuss K. Aetiology, diagnosis, treatment and outcome of traumatic reticuloperitonitis in cattle. Vet J. (2020) 255:105424. doi: 10.1016/j.tvjl.2020.105424
125. Braun U, Warislohner S, Gerspach C, Ohlerth S, Nuss K. Treatment of 503 cattle with traumatic reticuloperitonitis. Acta Vet Scand. (2018) 60:55. doi: 10.1186/s13028-018-0410-8
126. Bhattacharjya R, Chatterjee A, Pandey T. Diarrhoeain ruminantsand its control. A Review. IOSR J Agric Vet Sci. (2018) 11:19–22.
127. Mulder IE, Schmidt B, Lewis M, Delday M, Stokes CR, Bailey M, et al. Restricting microbial exposure in early life negates the immune benefits associated with gut colonization in environments of high microbial diversity. PLoS ONE. (2011) 6:e28279. doi: 10.1371/journal.pone.0028279
128. Li RW, Connor EE, Li C, Baldwin VI RL, Sparks ME. Characterization of the rumen microbiota of pre-ruminant calves using metagenomic tools. Environ Microbiol. (2012) 14:129–39. doi: 10.1111/j.1462-2920.2011.02543.x
129. Li F, Hitch TCA, Chen Y, Creevey CJ, Guan LL. Comparative metagenomic and metatranscriptomic analyses reveal the breed effect on the rumen microbiome and its associations with feed efficiency in beef cattle 06 Biological Sciences 0604 Genetics 06 Biological Sciences 0605 Microbiology. Microbiome. (2019) 7:1–21. doi: 10.1186/s40168-019-0618-5
Keywords: gastrointestinal microflora, metabolic diseases, rumen, ruminants, dairy cow, omics
Citation: Xu Q, Qiao Q, Gao Y, Hou J, Hu M, Du Y, Zhao K and Li X (2021) Gut Microbiota and Their Role in Health and Metabolic Disease of Dairy Cow. Front. Nutr. 8:701511. doi: 10.3389/fnut.2021.701511
Received: 28 April 2021; Accepted: 28 June 2021;
Published: 04 August 2021.
Edited by:
Jie Yin, Hunan Agricultural University, ChinaReviewed by:
Hongbing Fan, University of Alberta, CanadaCopyright © 2021 Xu, Qiao, Gao, Hou, Hu, Du, Zhao and Li. This is an open-access article distributed under the terms of the Creative Commons Attribution License (CC BY). The use, distribution or reproduction in other forums is permitted, provided the original author(s) and the copyright owner(s) are credited and that the original publication in this journal is cited, in accordance with accepted academic practice. No use, distribution or reproduction is permitted which does not comply with these terms.
*Correspondence: Xiang Li, eHhpYW5nbGxpQG1haWwuaHphdS5lZHUuY24=
Disclaimer: All claims expressed in this article are solely those of the authors and do not necessarily represent those of their affiliated organizations, or those of the publisher, the editors and the reviewers. Any product that may be evaluated in this article or claim that may be made by its manufacturer is not guaranteed or endorsed by the publisher.
Research integrity at Frontiers
Learn more about the work of our research integrity team to safeguard the quality of each article we publish.