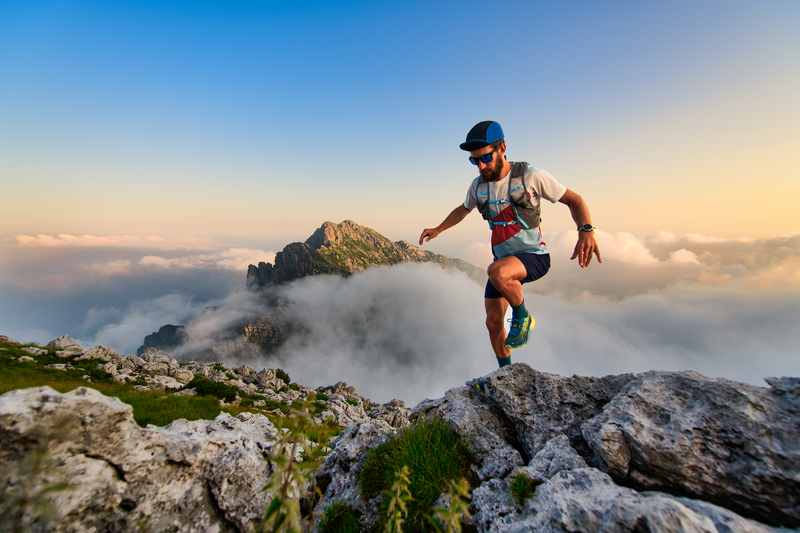
95% of researchers rate our articles as excellent or good
Learn more about the work of our research integrity team to safeguard the quality of each article we publish.
Find out more
REVIEW article
Front. Nutr. , 17 December 2021
Sec. Nutrition and Metabolism
Volume 8 - 2021 | https://doi.org/10.3389/fnut.2021.696966
This article is part of the Research Topic Precocious Gut Maturation: Environmental and Dietary Factors View all 8 articles
Differentiation of the digestive tube and formation of the gut unit as a whole, are regulated by environmental factors through epigenetic modifications which enhance cellular plasticity. The critical period of DNA imprinting lasts from conception until approximately the 1,000th day of human life. During pregnancy, besides agents that may directly promote epigenetic programming (e.g., folate, zinc, and choline supplementation), some factors (e.g., antibiotic use, dietary components) can affect the composition of the mother's microbiota, in turn affecting the fetal microbiome which interacts with the offspring's intestinal epithelial cells. According to available literature that confirms intrauterine microbial colonization, the impact of the microbiome and its metabolites on the genome seems to be key in fetal development, including functional gut maturation and the general health status of the offspring, as well as later on in life. Although the origin of the fetal microbiome is still not well-understood, the bacteria may originate from both the vagina, as the baby is born, as well as from the maternal oral cavity/gut, through the bloodstream. Moreover, the composition of the fetal gut microbiota varies depending on gestational age, which in turn possibly affects the regulation of the immune system at the barrier between mother and fetus, leading to differences in the ability of microorganisms to access and survive in the fetal environment. One of the most important local functions of the gut microbiota during the prenatal period is their exposure to foreign antigens which in turn contributes to immune system and tissue development, including fetal intestinal Innate Lymphoid Cells (ILCs). Additional factors that determine further infant microbiome development include whether the infant is born premature or at term, the method of delivery, maternal antibiotic use, and the composition of the mother's milk, among others. However, the latest findings highlight the fact that a more diverse infant gut microbiome at birth facilitates the proliferation of stem cells by microbial metabolites and accelerates infant development. This phenomenon confirms the unique role of microbiome. This review emphasizes the crucial perinatal and postnatal factors that may influence fetal and neonatal microbiota, and in turn gut maturation.
From the 3rd week of human embryogenesis, the digestive tube starts differentiating, leading to the formation of epithelial cells and eventually into the structural and functional gut unit (intestinal villi and crypts), along with the development of the enteric nervous system in the weeks to follow (1). The human gut is composed of the three germ layers—endoderm (which forms the epithelial lining of the lumen), mesoderm (which forms the smooth muscle layers), and ectoderm (which includes the most anterior and posterior luminal digestive structure and the enteric nervous system) (2). Besides for the well-known genetic and transcription factors which directly influence organogenesis, the early colonization of the gut with microbiota is pivotal in the development of a functional intestine and thus in the general health status of the infant, as well as that of the human in adult life. The gut microbiome provides us with both genetic and metabolic agents that we have not been required to evolve on our own, including the synthesis of various inaccessible nutrients (3). The human gut microbiome consists of a collective genome of microorganisms including bacteria, fungi, viruses, eukaryotes, and archaea and exceeds the size of all other microbial communities, with trillions of bacteria, often representing thousands of species, predominating (4). For about a century the fetus was believed to be sterile, with microbes only colonizing the newborn during birth. In the last few years however, it has been proven that the development of the structural and functional gut unit is a complex process which requires microbial input (5). Contact with the mothers' microbes and their metabolites during prenatal life may impact the infant's immune system and prepare the offspring for colonization during delivery and postnatal life (6). The mode of birth, contact between the infant and the mother's skin and breastfeeding are the next pivotal factors that determine the neonate's microbial diversity, as well as developmental and health consequences in the future (6–8). The critical period of DNA imprinting and early colonization last from conception until about the 1,000th day of human life. It should be emphasized that the gut microbiota is not only a local community of organisms, but should be considered as an active “organ” which provides the host with metabolic, trophic, and immunological benefits (9). Microbiota ensure protection against pathogens, stimulate innate, and adaptive (humoral and cellular) immune responses, regulate the development of enterocytes and take part in the synthesis of vitamins, short-chain fatty acids (SCFAs), and polyamines (9). Moreover, disturbances of the microbiome during early life are also associated with a higher incidence of chronic pediatric diseases, including obesity, allergic diseases, irritable bowel syndrome, or inflammatory bowel diseases (10).
In this review, we highlight the role of some perinatal and postnatal factors in modifying the composition of fetal and neonatal microbiota which in turn influences gut maturation processes. Moreover, we emphasize the role of the gut microbiota in the transformation of the “dead” food consumed by the host into “living integrated food,” with immunological and nutritional benefits for the host.
A research study performed in 2014, using next-generation sequencing, described a unique microbiome in the human placenta, which challenged the sterile womb paradigm (11). Results of studies that followed showed the existence of microbial communities in the fetal environment (placenta, cord blood, amniotic fluid, fetus, and meconium) and gave rise to the hypothesis of “in utero colonization” (12–15). The origin of this fetal microbiome is not well-known, however, the microbes may originate both from the vagina through ascendant colonization and from the maternal oral cavity/gut, through the bloodstream (5, 11, 16, 17).
The results of some previous studies performed, with an emphasis on sterile conditions, confirmed the presence of a viable mammalian fetal microbiota during in utero development (18, 19). Liu et al. showed that the distribution patterns of bacterial communities in the meconium of neonates is not dependent on the mode of delivery/birth, which in turn confirmed the exposure of the fetus to bacteria prior to birth (19). He et al. revealed that the microbiota in meconium shares more features with that of the amniotic fluid, compared to the maternal fecal and vaginal microbiota (20). Culture examination of meconium in 21 healthy, full-term neonates, born from healthy mothers, taken within 2 h of birth, and prior to feeding, revealed a diverse group of Gram-positive and Gram-negative bacteria with a dominance of Enterococcus and Staphylococcus (21). On the other hand, the microbiota from meconium collected from 14 preterm infants mainly consisted of Bacillus and other Firmicutes (22). Moreover, results from a study performed on human and mouse dyads demonstrate a dynamic, viable mammalian fetal microbiota during in utero development. Cultivatable bacteria were found in the fetal intestines only during mid-gestation, suggesting fetal exposure to viable and cultivatable bacteria during mid-gestation and subsequently to non-cultivatable bacteria during late gestation (18). These findings imply differences with regards to the ability of the microorganisms to access and persist in the fetal environment, caused by changes in immune regulation at the materno-fetal barrier during gestation, or the possibility of some of the microbes to exist in a viable, but non-cultivatable state (18, 23). On the other hand, several other previous studies have put forward arguments which contradict the possibility of infant microbial colonization in utero (24).
It has previously been shown that many prenatal factors, such as maternal diet, obesity, cigarette smoking and the use of antibiotics, may influence maternal microbiota composition, and in turn that of the neonate as well (25). Besides the “still controversial” hypothesis of “in utero colonization,” it should be emphasized that fetal development, including gut maturation, is definitely regulated by maternal microbial metabolites, transported to the fetus through the placenta [(24, 26); Figure 1]. Molecules from symbiotic microorganisms may initiate the microbial–host mutualism in utero, before acquisitioning microbial biomass in the offspring. The metabolites which take part in epigenetic DNA imprinting are synthesized endogenously by the maternal microbiota or originate from food compounds that are consumed by the mother (26). On the other hand, the presence of fetal microbiota, in the absence of any infection or inflammation, supports the hypothesis that microbes colonize the fetus before birth and also play a role in the physiological development of the fetus, including that of the gastrointestinal tract (5). It was previously shown in an animal model (Cesarean-derived germfree newborn piglets) colonized with adult swine feces that resident microbiota induced the expression of genes contributing to intestinal epithelial cell turnover, mucus biosynthesis and the priming of the immune system (29). Moreover, the early microbial colonization in humans, over and above the influence of other maternal factors like hormones and cytokines, is necessary to maintain a balance between maternal and fetal immunity (30). Fetal microbiota, or their molecular signatures, may form mucosal immunity and prepare the tissues for colonization following birth (18). This is especially significant in the context of beneficial bacteria immune regulation, such as Bifidobacteria and Lactobacilli present in the human placenta (31, 32). Microbiota colonization is associated with gut-associated lymphoid tissue (GALT) development (33). Toll-like receptors (TLRs) present on the surface of various cells (macrophages, mast cells, and dendritic cells) may recognize distinct bacteria driving the development of a potential inflammatory response (5). Intrauterine bacteria have been shown to promote the development of the fetal immune system, though the TLRs (34). Moreover, the production of SCFAs that may induce T-cell activation and modulate IL-10 release is another potential mechanism by which microbes influence the immune system (5, 35). The potential mechanisms of maternal microbiota influence on fetal immunity are presented in Figure 2.
Figure 1. Factors influencing the gut microbiota composition in the fetal period and infancy. Some factors like maternal diet, obesity, cigarette smoking, and the use of antibiotics influence the maternal microbiota composition and, consequently, fetal microbiome (25). The fetal microbiome probably originates both from the vagina through ascendant colonization and from the maternal oral cavity/gut, through the bloodstream (5, 11). The maternal microbiota metabolites transported to the fetus through the placenta regulate fetal development, including gut maturation and prepare the offspring for colonization during delivery and postnatal life (6, 26). The other important factors that influence the early microbiota composition and diversity are the way of delivery, contact between the infant and the mother's skin and feeding introduction (27, 28).
Figure 2. Potential mechanisms of maternal microbiota, vaginal delivery, breast feeding effects on the fetal/infant immunity. (A) The early microbial colonization in humans is necessary to maintain a balance between maternal and fetal immunity (30). Fetal microbiota, or their molecular signatures, may form mucosal immunity and prepare the tissues for colonization following birth (18). The maternal microbes and their metabolites have impact on immunity development by epigenetic modifications influencing Th1/Th2 balance, production of SCFAs that may induce T-cell activation and modulate IL-10 release, as well as regulation of TLRs (5, 33–35). (B,C) Vaginal delivery assures own mother's vaginal microbiota colonization. Breast feeding with all bioactive factors assures protective role against infections and maintains the mucosal epithelium and the development of the microbiome (36). Moreover, mother-derived active molecules (including immunoglobulins) assure gut homeostasis with regard to the newly colonizing microbiota by regulating the immune system in the GALT and shaping Innate Lymphoid Cell development in the intestine (36). Milk-derived antibodies from the mother's milk support the child with a passive immunity during the infancy (37). Short-chain fatty acids (SCFAs), Toll-like receptors (TLRs), and gut-associated lymphoid tissue (GALT).
After delivery a unique interaction or symbiotic relationship develops between the microbiome and host, which is necessary for survival. The bacterial community structure and host defenses influence the gut endothelial cells by modifying function, enhancing protection, and maintaining barrier function (38). The intestinal microbiome also participates in the synthesis of amino acids, vitamins and metabolites, which contribute to the general well-being of the host (26). Moreover, the gut microbiota also play a role with regards to the overall enzymatic function of the intestine, by ensuring the host with the necessary enzymes to digest complex carbohydrates (3). Taking into account that the human body is considered a holobiont, the genetic variation among hologenomes, including the combination of genetic content between the host and its associated microbiota, may result due to modifications in the host genome or the genomes of the constituent symbiotic microorganisms (39). These processes are essential especially for host health during the establishment of the infant gut microbiota, when trans-kingdom interactions between host and microbial cells are initiated (39).
The microbial composition of infants changes substantially in the days directly post-birth and is dependent on various factors including mode and time of delivery, maternal diet and infant diet (breast feeding vs. formula feeding), among others [(27, 36, 39, 40); Figure 1].
Mode of delivery is one of the crucial factors that influence the composition of the microbiota in newborns (27). Dominguez-Bello et al. showed that infants delivered by Cesarean section (CS) harbored bacterial communities similar to those found on the skin surface, dominated by Staphylococcus, Corynebacterium, and Propionibacterium spp.; while vaginally delivered newborns acquired bacterial communities resembling their own mother's vaginal microbiota, dominated by Lactobacillus, Prevotella, or Sneathia spp. (27). Moreover, Kim et al. showed delayed establishment of intestinal microbiota in newborns delivered by CS. In the group of vaginally delivered infants the overrepresentation of starch/sucrose, amino acid, and nucleotide metabolism in gut microbiota with depleted lipopolysaccharide biosynthesis was detected as compared to newborns delivered by CS (41). Taking into account that early gut colonization affects all stages of the human development, this phenomenon may lead to the disturbance in the processes of immune maturation, metabolic programming, and in turn gut maturation in newborns delivered by CS (41, 42). The intestinal microbiota and its metabolites influence the growth and differentiation of the epithelial cells of the intestinal lumen, promote digestion and metabolism of food, take part in vitamin and amino acids synthesis and ion absorption (43). The significant differences in microbiota composition of neonates born vaginally vs. those born via CS lead to the initiation of the Vaginal seeding (VS) procedure. During VS, maternal vaginal bacteria are artificially administered to babies following birth by CS. This procedure was initiated in attempt to mimic the exposure of the offspring to the vaginal microbiota, which would normally occur during a vaginal birth (44, 45). However, the safety of the above mentioned procedure is controversial due to exposure to both vaginal commensals and potential pathogens that may lead to severe infection (44).
In the case of premature birth, post-delivery maturation of the gut is crucial for survival and the general and future well-being of the infant (46). The intestinal microbiota of preterm infants is characterized by a different biodiversity of microorganisms compared to that of full-term infants (47, 48). Moreover, available data suggests that microbiota development is driven by host biology and associated with gestational age (49). Bacilli initially dominate, followed by Gammaproteobacteria, and finally by Clostridia by ~37 weeks post-menstrual age (50). Prematurity predisposes the infant to gut dysbiosis with a dominance of Gram-negative bacteria of the class Gammaproteobacteria and its constituent families Enterobacteriaceae, Vibrionaceae, Pseudomonadaceae, and decreased relative abundance of Firmicutes and Bacteroidetes (51–53). Alterations in microbiota composition puts infants at risk for the development of diseases such as necrotizing enterocolitis (NEC) and chronic lung disease (51–54). Clinical observations have confirmed a link between factors produced during chronic inflammation and growth failure (55). A distinctive pattern of host microbiota interaction in a humanized gnotobiotic mouse model of intestine development has previously been described (56). In the above-mentioned studies, the pregnant mice were colonized with fecal microbiota from preterm infants with low (MPI-L) or high (MPI-H) growth rates. Microbiome analysis showed a greater contribution of Bacteroidetes and Actinobacteria in MPI-H-colonized mice compared to MPI-L mice. Moreover, it was noted that the mice offspring showed the original preterm infants' growth phenotype—MPI-H offspring, exhibiting a higher growth rate and advanced maturation of the intestine (well-organized tight junctions, higher goblet and Paneth cell numbers, and higher expression of intestinal epithelial maturation marker gene, Lgr5), in comparison to MPI-L pups. The reduced development of the MPI-L pups related to the overexpression of pro-inflammatory markers such as monocyte chemoattractant protein-1(MCP-1), vascular cell adhesion molecule-1 (VCAM-1) and nuclear factor kappa-light-chain-enhancer of activated B cells (NF-κB), in the intestinal mucosa (56, 57). Dougherty et al. found that the distinct microbial communities acquired by full-term and pre-term neonates generate distinct metabolomic profiles in the developing small intestine (58). Full-term infant microbial communities were characterized by a lack of diversity and an overrepresentation of various Bacilli spp., relative to that of pre-term infants. Therefore, the pre-term neonate metabolome was characterized by an increase in bacterially transformed products, vitamins, and amino acid derivatives that induce stem cell proliferation (58).
Despite the adequate supply of amino acids by enteral and parenteral nutrition, the gut bacteria are responsible for polyamine production (59). Putrescine is a polyamine which serves as a precursor for cellular division and increased cell proliferation (60). In the case of premature infants, ornithine and putrescine levels were found to be related to the abundance of specific bacterial groups including Clostridia, Gammaproteobacteria, and Actinobacteria (58). Moreover, the pre-term intestinal metabolome is richer in deoxycholic acid (DCA) which promote stem cell proliferation during early life (58). However, the gut dysbiosis that is usually observed in premature infants may negatively influence innate immunity. NEC has been shown to be associated with increased intestinal expression of TLR4 and decreased expression of TLR9, which is modulated by bacterial lipopolysaccharide (61). However, stimulation of this pathway is dependent on microbiota composition, since some LPS isoforms have been reported to inhibit TLR4 signaling (62). The microbiota is shaped not only by gestational age, but also by the environment in the neonatal intensive care unit, nutrition, and common clinical practices in neonatal care (46).
The postnatal period is crucial for continuation of microbiota colonization, as well as the development of host–microbe interactions and immune homeostasis (63). During the intrauterine period the innate immunity is highly adapted to facilitate the fetal–postnatal transition to a rapidly increasing microbial biomass and the development of long-term host microbial mutualism (26). The most important modifier of the intestinal microbiome, regardless of developmental stage, is diet (36). Gut microbiota colonization, caused by the introduction of feeding, is a milestone in the development of adaptive immunity, the production of digestive enzymes and in the transformation of the consumed milk into “living integrated food” (chyme). Exogenous factors, including the diet, are essential in the colonization of the gut by bacteria during the early neonatal period, as well as in the establishment of a “life-long” microbiota composition (Figure 3). Fulde et al. reported that enhanced expression of the flagellin receptor, TLR5, by the murine neonatal epithelium, contributes to the selection of a beneficial gut microbiota that strongly influences the composition of the microbiota throughout life (63).
Figure 3. Influence of diet introduction and food composition on the infant microbiota diversity. The introduction of feeding is a milestone in the development of adaptive immunity, the production of digestive enzymes and the transformation of the consumed milk into “living integrated food” (chyme). The diet is essential in the colonization processes during the early neonatal period, as well as in the establishment of a “lifelong” microbiota composition. The microbiome of breastfed infants consists predominantly of Bifidobacterium (B. breve, B. longum, and B. bifidum) and Lactobacillus (64). The utilization of HMOs from human milk by Bifidobacteria is especially beneficial for the production catabolites (Acetate Lactate Formate 1,2-Propanediol) and AAA-derived Co-HMO metabolism products (65). Formula-fed infants have a more diverse microbiota, with increased abundance of Bacteroidetes, Firmicutes, Clostridium difficile, and Enterobacteriaceae and in turn BCAA and AAA catabolites (64, 65). Complementary feeding increases gut microbial diversity with dominance of Lachnospiraceae, Ruminococcaceae, Bacteroidaceae, and Prevotellaceae species and production of BCFAs and SCFAs (65, 66). Human milk oligosaccharide (HMO), aromatic amino acid (AAA), branched chain amino acid (BCAA), indolelactate (ILA), phenyllactate (PLA), 4-hydroxypheyllactate (4-OH-PLA), branched chain fatty acids (BCFAs), and short-chain fatty acids (SCFAs).
Human breast milk is recommended as the gold standard of infant nutrition. The first few portions of mothers own milk (MOM) provide the infant with colostrum, which is low in volume but rich in immune substances, which are essential for gut development (28). The MOM portions that follow supply the infant with more nutrients, along with immunomodulatory substances including antibodies, oligosaccharides, anti-microbial agents, epidermal growth factors, hormones, digestive enzymes, and microorganisms to colonize the neonate's intestines [(28); Figure 2]. Breast milk contains a diverse population of bacteria that are transferred to the infant's gut (8). The MOM microbiome is similar with regards to composition as the skin microbiome and is dominated by Staphylococcaceae and Streptococcaceae, with lesser amounts of Lactobacilliaceae, Corynebacteriaceae, and other organisms (36). The microbiome of breastfed human infants consists predominantly of Bifidobacterium (B. breve, B. longum, and B. bifidum) and Lactobacillus. Formula-fed infants, on the other hand, have a more diverse microbiome (64). The pasteurized donor human milk (DHM) recommended for preterm infants when MOM is lacking, also influences the infant's metabolic phenotype and microbiota. Although preterm infants fed DHM present with microbial profiles closer to those of neonates fed MOM, compared to those fed formula, some differences were reported. Preterm infants fed MOM show a significantly greater presence of Bifidobacteriaceae and a lower presence of Staphylococcaceae, Clostridiaceae, and Pasteurellaceae, compared to preterm infants fed DHM (67). Piñeiro-Ramos et al. showed significant differences in the galactose, starch and sucrose metabolism pathways when comparing MOM and DHM, as well as differences in the gut microbiota composition (68). Moreover, it was shown that the protective effect of DHM is not complete probably due to destruction of the enzymes and immune molecules during pasteurization process (69, 70). MOM is the primary source of secretory IgA (sIgA) for neonates. sIgA is secreted by the B cells of the mammary gland and is shaped by the intestinal microorganisms of the mother (36). The protection provided by maternal sIgA is important to ensure a stable relationship between the microbiota and the host immune system (64). sIgA has a local gut action and provides anti-bacterial effects by binding to bacteria and preventing them from invading the mucosal epithelium (36). Beside the anti-bacterial action, sIgA may also promote physiological gut colonization with Bacteroides and Firmicutes and limit the growth of inflammatory facultative anaerobes, such as Enterobacteriaceae. sIgA supports the stable colonization of Bacteroides by acting as a carbohydrate source and through the regulation of the commensal colonizing factor (ccf), which enhances coating by sIgA and increases epithelial adherence of those bacteria (36, 71). Considering that IgG transplacental transfer takes place in the third trimester of pregnancy, immunoglobulin A, M, and G delivery through the MOM is especially pivotal in the case of premature infants, until endogenous immunoglobulin production begins (64). The action of IgG in the gut is related to the prevention of the development of potential enteric infections. The cooperation of maternal IgG with IgA ensures intestinal homeostasis, such that in the absence of either IgA or IgG, antibodies of the other isotypes are sufficient to assure immune responses. Dual recognition of microbes by both IgA and IgG assures the cooperative nature of these antibodies (72). Moreover, mother-derived IgG and IgA assure gut homeostasis with regards to the newly colonizing microbiota by regulating the immune system in the GALT and shaping Innate Lymphoid Cell development in the intestine (36).
Human milk oligosaccharides (HMOs) are another key factor in promoting favorable gut colonization assuring the protective, metabolic, and trophic roles of microbiota. HMOs consist of five monosaccharide building blocks, glucose (Glc), galactose (Gal), N-acetylglucosamine (GlcNAc), fucose (Fuc), and the sialic acid N-acetyl-neuraminic acid (Neu5Ac). HMOs are involved in the composition of neonate microbiota by regulation of colonization (73). HMOs have been suggested to inhibit the growth of pathogenic bacteria including Streptococcus pneumoniae and Haemophilus influenzae by interfering with the adhesion of the bacteria to epithelial cells (74). On the other hand, some bacteria, including Bifidobacterium and Bacteroides, metabolize HMOs for energy production (73). The utilization of HMOs by Bifidobacteria is especially beneficial for the production of SCFAs (36). SCFAs are the primary end-products of the fermentation of non-digestible carbohydrates available to the gut microbiota. They represent the major flow of carbon from the diet, through the microbiome, to the host (75). SCFAs including acetate, butyrate, and propionate and to a lesser degree the branched-chain fatty acids, are the main products of nutrient breakdown by microbes (76). The SCFAs may be absorbed or used as an energy source by colonocytes to maintain the colonic epithelium, however, the metabolites have other systemic effects in the host through their action as signaling molecules and involvement as regulators of gene expression. SCFAs play an important role in regulating the integrity of the epithelial barrier through the stimulation of tight junction proteins, which are essential in decreasing intestinal permeability and the translocation of bacteria and/or their cell wall components, which trigger inflammatory responses (75). The inhibition of bacterial translocation protects against the activation of TLR4, by bacterial LPS, which may in turn mediate a pro-inflammatory cascade in immune cells (75). Bridgman et al., in a study performed on infants, confirmed that breastfeeding strongly influences the composition of fecal microbial metabolites in infancy. Compared to non-breastfed infants, those exclusively breastfed were four times more likely to have a higher proportion of acetate relative to other SCFAs in their gut (77).
Formula feeding is recommended when MOM is lacking. As mentioned above, formula-fed infants have a more diverse microbiota, with increased abundance of Bacteroidetes, Firmicutes, Clostridium difficile, and Enterobacteriaceae (64). The differences in early microbiota colonization in this group of infants when compared to breast fed neonates result from higher proteins content and lack of HMOs in the formula. The formula milk usually contains excess protein, only partially digested and absorbed by upper gastrointestinal tract. Incompletely digested protein, peptides, and amino acids are metabolized in the colon by gut microbes, including opportunistic pathogenic bacteria (e.g., Clostridium difficile, Escherichia coli), into various metabolites such as isovalerate, isobutyrate, phenylacetate, p-cresol, and tryptamine (65).
In recent years, the infant formulas have been enriched with bioactive ingredients (e.g., probiotics, prebiotics, polyunsaturated fatty acids, synthetically produced HMOs) to mimic the composition of the human milk. However, the beneficial effects of this supplementation on both, fecal microbiome, and metabolome are discussed (65, 78–80).
The full maturation of the adaptive immune system is essential predominantly at weaning, when the newborn host is exposed to new antigens (26). It was previously shown that the antibodies in the milk, shaped by the mother's microbiota, are transferred to the newborn mammals during feeding and influence the development of immune system memory. Moreover, the change in microbiota composition following weaning is also pivotal for immune development. At weaning (in humans during the introduction of milk formula or solid food) the intestinal microbiota induce a vigorous immune response (“weaning reaction”) that may lead led to pathological imprinting and increased susceptibility to colitis, allergic inflammation, and cancer later in life (26). Prevention of this phenomenon relates to the action of RORγt+ regulatory T cells, which required bacterial and dietary metabolites—SCFAs and retinoic acid (81). Moreover, the weaning response may be also inhibited by epidermal growth factor, transferred to the infant by maternal milk (82).
The development of the human gut, including gut microbiome, from infancy to childhood is driven by many factors including complementary feeding. The World Human Organization (WHO) recommends starting complementary feeding around the age of 6 months. Late introduction of foods other than MOM may lead to the disturbances in growth and development (83). On the other hand, it was shown, that early introduction of solid foods increased the risk of childhood obesity and immune-mediated conditions (84) that might be associated with higher gut microbiota diversity and altered gut microbiota composition (85). Complementary feeding increases production of branched chain fatty acids (BCFAs) and SCFAs by microbes with potential implication for growth and development (65). Compared with HMOs, the carbohydrates from the first foods based on cereals, fruit and vegetables are highly diverse, which promotes selection of microbes (Lachnospiraceae, Ruminococcaceae, Bacteroidaceae, and Prevotellaceae species) that can utilize these complex substrates for growth (66). Moreover, Homann et al. showed that higher fiber intake and high dietary diversity were associated with the greatest impact on the gut microbiome, with stability of the gut microbiota, as solids were introduced (86). The metabolites produced by the microbiota at the onset of solid food ingestion contribute to the maturation of the gut barrier at the suckling-to-weaning transition (87).
In this review, we highlight the influence of some agents on early human microbiota colonization and intestinal development. Although, according to available data, the in-utero colonization hypothesis is debatable, the effects of maternal microbiota metabolites on the fetus, that promote immune regulation for example, are well-documented. After delivery/birth, the unique interaction between the microbiome and host provides various benefits for the neonate including metabolic, trophic, and immunological functions. The metabolome of premature infants is characterized by increased bacterially transformed products, vitamins, and amino acid derivatives, that stimulate stem cell proliferation and gastrointestinal tract development. With regards to environmental factors, the mode of delivery/birth and breastfeeding are key factors which affect microbiota colonization and thus determine the overall health status of the organism, not only in infancy but also in adulthood.
AS-B and KP: conceptualization. AS-B: writing—original draft preparation. AS-B, KP, MP, and EC: writing—review and editing. MP: visualization. All authors have read and agreed to the published version of the manuscript.
This study was funded by the Ministry of Science and Higher Education in Poland—subsidy for conducting research activities at the Polish Mother's Memorial Hospital—Research Institute.
The authors declare that the research was conducted in the absence of any commercial or financial relationships that could be construed as a potential conflict of interest.
All claims expressed in this article are solely those of the authors and do not necessarily represent those of their affiliated organizations, or those of the publisher, the editors and the reviewers. Any product that may be evaluated in this article, or claim that may be made by its manufacturer, is not guaranteed or endorsed by the publisher.
1. Bhatia A, Shatanof RA, Bordoni B. Embryology, Gastrointestinal. Treasure Island, FL: StatPearls (2020).
2. De Santa Barbara P, van den Brink GR, Roberts DJ. Development and differentiation of the intestinal epithelium. Cell Mol Life Sci. (2020) 60:1322–32. doi: 10.1007/s00018-003-2289-3
3. Bäckhed F, Ley RE, Sonnenburg JL, Peterson DA, Gordon JI. Host-bacterial mutualism in the human intestine. Science. (2005) 307:1915–20. doi: 10.1126/science.1104816
4. Walker RW, Clemente JC, Peter I, Loos RJF. The prenatal gut microbiome: are we colonized with bacteria in utero? Pediatr Obes. (2017) 12(Suppl. 1):3–17. doi: 10.1111/ijpo.12217
5. D'Argenio V. The prenatal microbiome: a new player for human health. High Throughput. (2018) 7:38. doi: 10.3390/ht7040038
6. Rodríguez JM, Murphy K, Stanton C, Ross RP, Kober OI, Juge N, et al. The composition of the gut microbiota throughout life, with an emphasis on early life. Microb Ecol Health Dis. (2015) 26:26050. doi: 10.3402/mehd.v26.26050
7. Dogra S, Sakwinska O, Soh SE, Ngom-Bru C, Brück WM, Berger B, et al. Dynamics of infant gut microbiota are influenced by delivery mode and gestational duration and are associated with subsequent adiposity. mBio. (2015) 6:e02419–14. doi: 10.1128/mBio.02419-14
8. Pannaraj PS, Li F, Cerini C, Bender JM, Yang S, Rollie A, et al. Association between breast milk bacterial communities and establishment and development of the infant gut microbiome. JAMA Pediatr. (2017) 171:647–54. doi: 10.1001/jamapediatrics.2017.0378
9. Adamek K, Skonieczna-Zydecka K, Wegrzyn D, Łoniewska B. Prenatal and early childhood development of gut microbiota. Eur Rev Med Pharmacol Sci. (2019) 23:9667–80. doi: 10.26355/eurrev_201911_19461
10. Zhuang L, Chen H, Zhang S, Zhuang J, Li Q, Feng Z. Intestinal microbiota in early life and its implications on childhood health. Genomics Proteomics Bioinformatics. (2019) 17:13–25. doi: 10.1016/j.gpb.2018.10.002
11. Aagaard K, Ma J, Antony KM, Ganu R, Petrosino J, Versalovic J. The placenta harbors a unique microbiome. Sci Transl Med. (2014) 6:237ra26. doi: 10.1126/scitranslmed.3008599
12. Tang N, Luo ZC, Zhang L, Zheng T, Fan P, Tao Y, et al. The association between gestational diabetes and microbiota in placenta and cord blood. Front Endocrinol. (Lausanne). (2020) 11:550319. doi: 10.3389/fendo.2020.550319
13. Stinson L, Hallingstrom M, Barman M, Viklund F, Keelan J, Kacerovsky M, et al. Comparison of bacterial DNA profiles in midtrimester amniotic fluid samples from preterm and term deliveries. Front Microbiol. (2020) 11:415. doi: 10.3389/fmicb.2020.00415
14. Saavedra JM, Dattilo AM. Early development of intestinal microbiota: implications for future health. Gastroenterol Clin N Am. (2012) 41:717–31. doi: 10.1016/j.gtc.2012.08.001
15. Ardissone AN, de la Cruz DM, Davis-Richardson AG, Rechcigl KT, Li N, Drew JC, et al. Meconium microbiome analysis identifies bacteria correlated with premature birth. PLoS ONE. (2014) 9:e90784. doi: 10.1371/journal.pone.0090784
16. Jones HE, Harris KA, Azizia M, Bank L, Carpenter B, Hartley JC, et al. Differing prevalence and diversity of bacterial species in fetal membranes from very preterm and term labor. PLoS ONE. (2009) 4:e8205. doi: 10.1371/journal.pone.0008205
17. Jiménez E, Fernández L, Marín ML, Martín R, Odriozola JM, Nueno-Palop C, et al. Isolation of commensal bacteria from umbilical cord blood of healthy neonates born by cesarean section. Curr Microbiol. (2005) 51:270–4. doi: 10.1007/s00284-005-0020-3
18. Younge N, McCann JR, Ballard J, Plunkett C, Akhtar S, Araújo-Pérez F, et al. Fetal exposure to the maternal microbiota in humans and mice. JCI Insight. (2019) 4:e127806. doi: 10.1172/jci.insight.127806
19. Liu CJ, Liang X, Niu ZY, Jin Q, Zeng XQ, Wang WX, et al. Is the delivery mode a critical factor for the microbial communities in the meconium? EBioMedicine. (2019) 49:354–63. doi: 10.1016/j.ebiom.2019.10.045
20. He Q, Kwok LY, Xi X, Zhong Z, Ma T, Xu H, et al. The meconium microbiota shares more features with the amniotic fluid microbiota than the maternal fecal and vaginal microbiota. Gut Microbes. (2020) 12:1794266. doi: 10.1080/19490976.2020.1794266
21. Jiménez E, Marín ML, Martín R, Odriozola JM, Olivares M, Xaus J, et al. Is meconium from healthy newborns actually sterile? Res Microbiol. (2008) 159:187–93. doi: 10.1016/j.resmic.2007.12.007
22. Moles L, Gómez M, Heilig H, Bustos G, Fuentes S, de Vos W, et al. Bacterial diversity in meconium of preterm neonates and evolution of their fecal microbiota during the first month of life. PLoS ONE. (2013) 8:e66986. doi: 10.1371/journal.pone.0066986
23. Li L, Mendis N, Trigui H, Oliver JD, Faucher SP. The importance of the viable but non-culturable state in human bacterial pathogens. Front Microbiol. (2014) 5:258. doi: 10.3389/fmicb.2014.00258
24. Walter J, Hornef MW. A philosophical perspective on the prenatal in utero microbiome debate. Microbiome. (2021) 9:5. doi: 10.1186/s40168-020-00979-7
25. Vandenplas Y, Carnielli VP, Ksiazyk J, Luna MS, Migacheva N, Mosselmans JM, et al. Factors affecting early-life intestinal microbiota development. Nutrition. (2020) 78:110812. doi: 10.1016/j.nut.2020.110812
26. Ganal-Vonarburg SC, Hornef MW, Macpherson AJ. Microbial-host molecular exchange and its functional consequences in early mammalian life. Science. (2020) 368:604–7. doi: 10.1126/science.aba0478
27. Dominguez-Bello MG, Costello EK, Contreras M, Magris M, Hidalgo G, Fierer N, et al. Delivery mode shapes the acquisition and structure of the initial microbiota across multiple body habitats in newborns. Proc Natl Acad Sci USA. (2010) 107:11971–5. doi: 10.1073/pnas.1002601107
28. Ballard O, Morrow AL. Human milk composition: nutrients and bioactive factors. Pediatr Clin North Am. (2013) 60:49–74. doi: 10.1016/j.pcl.2012.10.002
29. Chowdhury SR, King DE, Willing BP, Band MR, Beever JE, Lane AB, et al. Transcriptome profiling of the small intestinal epithelium in germfree versus conventional piglets. BMC Genomics. (2007) 8:215. doi: 10.1186/1471-2164-8-215
30. Hsu P, Nanan R. Foetal immune programming: hormones, cytokines, microbes and regulatory T cells. J Reprod Immunol. (2014) 104-105:2–7. doi: 10.1016/j.jri.2014.02.005
31. Romano-Keeler J, Weitkamp JH. Maternal influences on fetal microbial colonization and immune development. Pediatr Res. (2015) 77:189–95. doi: 10.1038/pr.2014.163
32. Satokari R, Grönroos T, Laitinen K, Salminen S, Isolauri E. Bifidobacterium and Lactobacillus DNA in the human placenta. Lett Appl Microbiol. (2009) 48:8–12. doi: 10.1111/j.1472-765X.2008.02475.x
33. Kasarello K, Sajdel-Sulkowska EM. Developmental significance of early gut-associated lymphoid tissue (GALT) - microbiota interactions in health and disease: creating balance between tolerance and inflammation in children. Open J Pediatr Child Health. (2019) 4:040–6. doi: 10.17352/ojpch.000019
34. Mei C, Yang W, Wei X, Wu K, Huang D. The unique microbiome and innate immunity during pregnancy. Front Immunol. (2019) 10:2886. doi: 10.3389/fimmu.2019.02886
35. Smith PM, Howitt MR, Panikov N, Michaud M, Gallini CA, et al. The microbial metabolites, short-chain fatty acids, regulate colonic Treg cell homeostasis. Science. (2013) 341:569–73. doi: 10.1126/science.1241165
36. Gopalakrishna KP, Hand TW. Influence of maternal milk on the neonatal intestinal microbiome. Nutrients. (2020) 12:823. doi: 10.3390/nu12030823
37. Parigi SM, Eldh M, Larssen P, Gabrielsson S, Villablanca EJ. Breast milk and solid food shaping intestinal immunity. Front Immunol. (2015) 6:415. doi: 10.3389/fimmu.2015.00415
38. Berrington JE, Stewart CJ, Cummings SP, Embleton ND. The neonatal bowel microbiome in health and infection. Curr Opin Infect Dis. (2014) 27:236–43. doi: 10.1097/QCO.0000000000000061
39. Milani C, Duranti S, Bottacini F, Casey E, Turroni F, Mahony J, et al. The first microbial colonizers of the human gut: composition, activities, and health implications of the infant gut microbiota. Microbiol Mol Biol Rev. (2017) 81:e00036-17. doi: 10.1128/MMBR.00036-17
40. Gosalbes MJ, Llop S, Vallès Y, Moya A, Ballester F, Francino MP. Meconium microbiota types dominated by lactic acid or enteric bacteria are differentially associated with maternal eczema and respiratory problems in infants. Clin Exp Allergy. (2013) 43:198–211. doi: 10.1111/cea.12063
41. Kim G, Bae J, Kim MJ, Kwon H, Park G, Kim SJ, et al. Delayed establishment of gut microbiota in infants delivered by cesarean section. Front Microbiol. (2020) 11:2099. doi: 10.3389/fmicb.2020.02099
42. Lozupone CA, Stombaugh JI, Gordon JI, Jansson JK, Knight R. Diversity, stability and resilience of the human gut microbiota. Nature. (2012) 489:220–30. doi: 10.1038/nature11550
43. Shaterian N, Abdi F, Ghavidel N, Alidost F. Role of cesarean section in the development of neonatal gut microbiota: a systematic review. Open Med. (2021) 16:624–39. doi: 10.1515/med-2021-0270
44. Cunnington AJ, Sim K, Deierl A, Kroll JS, Brannigan E, Darby J. “Vaginal seeding" of infants born by caesarean section. BMJ. (2016) 352:i227. doi: 10.1136/bmj.i227
45. Butler ÉM, Reynolds AJ, Derraik JGB, Wilson BC, Cutfield WS, Grigg CP. The views of pregnant women in New Zealand on vaginal seeding: a mixed-methods study. BMC Pregnancy Childbirth. (2021) 21:49. doi: 10.1186/s12884-020-03500-y
46. Henderickx JGE, Zwittink RD, van Lingen RA, Knol J, Belzer C. The preterm gut microbiota: an inconspicuous challenge in nutritional neonatal care. Front Cell Infect Microbiol. (2019) 9:85. doi: 10.3389/fcimb.2019.00085
47. Rougé C, Goldenberg O, Ferraris L, Berger B, Rochat F, Legrand A, et al. Investigation of the intestinal microbiota in preterm infants using different methods. Anaerobe. (2010) 16:362–70. doi: 10.1016/j.anaerobe.2010.06.002
48. Hallab JC, Leach ST, Zhang L, Mitchell HM, Oei J, Lui K, et al. Molecular characterization of bacterial colonization in the preterm and term infant's intestine. Indian J Pediatr. (2013) 80:1–5. doi: 10.1007/s12098-012-0753-5
49. Grier A, Qiu X, Bandyopadhyay S, Holden-Wiltse J, Kessler HA, Gill AL, et al. Impact of prematurity and nutrition on the developing gut microbiome and preterm infant growth. Microbiome. (2017) 5:158. doi: 10.1186/s40168-017-0377-0
50. La Rosa PS, Warner BB, Zhou Y, Weinstock GM, Sodergren E, Hall-Moore CM, et al. Patterned progression of bacterial populations in the premature infant gut. Proc Natl Acad Sci USA. (2014) 111:12522–7. doi: 10.1073/pnas.1409497111
51. Warner BB, Deych E, Zhou Y, Hall-Moore C, Weinstock GM, Sodergren E, et al. Gut bacteria dysbiosis and necrotising enterocolitis in very low birthweight infants: a prospective case-control study. Lancet. (2016) 387:1928–36. doi: 10.1016/S0140-6736(16)00081-7
52. Pammi M, Cope J, Tarr PI, Warner BB, Morrow AL, Mai V, et al. Intestinal dysbiosis in preterm infants preceding necrotizing enterocolitis: a systematic review and meta-analysis. Microbiome. (2017) 5:31. doi: 10.1186/s40168-017-0248-8
53. Fundora JB, Guha P, Shores DR, Pammi M, Maheshwari A. Intestinal dysbiosis and necrotizing enterocolitis: assessment for causality using Bradford Hill criteria. Pediatr Res. (2020) 87:235–48. doi: 10.1038/s41390-019-0482-9
54. Speer CP. New insights into the pathogenesis of pulmonary inflammation in preterm infants. Biol Neonate. (2001) 79:205–9. doi: 10.1159/000047092
55. Lu L, Claud EC. Intrauterine inflammation, epigenetics, and microbiome influences on preterm infant health. Curr Pathobiol Rep. (2018) 6:15–21. doi: 10.1007/s40139-018-0159-9
56. Lu L, Yu Y, Guo Y, Wang Y, Chang EB, Claud EC. Transcriptional modulation of intestinal innate defense/inflammation genes by preterm infant microbiota in a humanized gnotobiotic mouse model. PLoS ONE. (2015) 10:e0124504. doi: 10.1371/journal.pone.0124504
57. Yu Y, Lu L, Sun J, Petrof EO, Claud EC. Preterm infant gut microbiota affects intestinal epithelial development in a humanized microbiome gnotobiotic mouse model. Am J Physiol Gastrointest Liver Physiol. (2016) 311:G521–32. doi: 10.1152/ajpgi.00022.2016
58. Dougherty MW, Kudin O, Mühlbauer M, Neu J, Gharaibeh RZ, Jobin C. Gut microbiota maturation during early human life induces enterocyte proliferation via microbial metabolites. BMC Microbiol. (2020) 20:205. doi: 10.1186/s12866-020-01892-7
59. Matsumoto M, Kibe R, Ooga T, Aiba Y, Kurihara S, Sawaki E, et al. Impact of intestinal microbiota on intestinal luminal metabolome. Sci Rep. (2012) 2:233. doi: 10.1038/srep00233
60. Liu L, Guo X, Rao JN, Zou T, Marasa BS, Chen J, et al. Polyamine-modulated c-Myc expression in normal intestinal epithelial cells regulates p21Cip1 transcription through a proximal promoter region. Biochem J. (2006) 398:257–67. doi: 10.1042/BJ20060217
61. Coats SR, Do CT, Karimi-Naser LM, Braham PH, Darveau RP. Antagonistic lipopolysaccharides block E. coli lipopolysaccharide function at human TLR4 via interaction with the human MD-2 lipopolysaccharide binding site. Cell Microbiol. (2007) 9:1191–202. doi: 10.1111/j.1462-5822.2006.00859.x
62. Gribar SC, Sodhi CP, Richardson WM, Anand RJ, Gittes GK, Branca MF, et al. Reciprocal expression and signaling of TLR4 and TLR9 in the pathogenesis and treatment of necrotizing enterocolitis. J Immunol. (2009) 182:636–46. doi: 10.4049/jimmunol.182.1.636
63. Fulde M, Sommer F, Chassaing B, van Vorst K, Dupont A, Hensel M, et al. Neonatal selection by Toll-like receptor 5 influences long-term gut microbiota composition. Nature. (2018) 560:489–93. doi: 10.1038/s41586-018-0395-5
64. Granger CL, Embleton ND, Palmer JM, Lamb CA, Berrington JE, Stewart CJ. Maternal breastmilk, infant gut microbiome and the impact on preterm infant health. Acta Paediatr. (2021) 110:450–7. doi: 10.1111/apa.15534
65. Laursen MF. Gut microbiota development: influence of diet from infancy to toddlerhood. Ann Nutr Metab. (2021) 30:1–14. doi: 10.1159/000517912
66. Kim CC, Parkar SG, Gopal PK. Developing infant gut microflora and complementary nutrition. J R Soc New Zeal. (2020) 50:384–96. doi: 10.1080/03036758.2020.1718716
67. Parra-Llorca A, Gormaz M, Alcántara C, Cernada M, Nuñez-Ramiro A, Vento M, et al. Preterm gut microbiome depending on feeding type: significance of donor human milk. Front Microbiol. (2018) 9:1376. doi: 10.3389/fmicb.2018.01376
68. Piñeiro-Ramos JD, Parra-Llorca A, Ten-Doménech I, Gormaz M, Ramón-Beltrán A, Cernada, et al. Effect of donor human milk on host-gut microbiota and metabolic interactions in preterm infants. Clin Nutr. (2020) 40:1296–309. doi: 10.1016/j.clnu.2020.08.013
69. Socha-Banasiak A, Pierzynowski SG, Szczurek P, Woliński J, Wesołowska A, Czkwianianc E, et al. Gut response to pasteurized donor human milk in a porcine model of the premature infant. J Biol Regul Homeost Agents. (2020) 34:2003–15. doi: 10.23812/20-272-A
70. Silano M, Milani GP, Fattore G, Agostoni C. Donor human milk and risk of surgical necrotizing enterocolitis: a meta-analysis. Clin Nutr. (2019) 38:1061–6. doi: 10.1016/j.clnu.2018.03.004
71. Lee SM, Donaldson GP, Mikulski Z, Boyajian S, Ley K, Mazmanian SK. Bacterial colonization factors control specificity and stability of the gut microbiota. Nature. (2013) 501:426–9. doi: 10.1038/nature12447
72. Koch MA, Reiner GL, Lugo KA, Kreuk LS, Stanbery AG, Ansaldo E, et al. Maternal IgG and IgA antibodies dampen mucosal T helper cell responses in early life. Cell. (2016) 165:827–41. doi: 10.1016/j.cell.2016.04.055
73. Bode L. Human milk oligosaccharides: every baby needs a sugar mama. Glycobiology. (2012) 22:1147–62. doi: 10.1093/glycob/cws074
74. Andersson B, Porras O, Hanson LA, Lagergård T, Svanborg-Edén C. Inhibition of attachment of Streptococcus pneumoniae and Haemophilus influenzae by human milk and receptor oligosaccharides. J Infect Dis. (1986) 153:232–7. doi: 10.1093/infdis/153.2.232
75. Morrison DJ, Preston T. Formation of short chain fatty acids by the gut microbiota and their impact on human metabolism. Gut Microbes. (2016) 7:189–200. doi: 10.1080/19490976.2015.1134082
76. Kumari M, Kozyrskyj AL. Gut microbial metabolism defines host metabolism: an emerging perspective in obesity and allergic inflammation. Obes Rev. (2017) 18:18–31. doi: 10.1111/obr.12484
77. Bridgman SL, Azad MB, Field CJ, Haqq AM, Becker AB, Mandhane PJ, et al. Fecal short-chain fatty acid variations by breastfeeding status in infants at 4 months: differences in relative versus absolute concentrations. Front Nutr. (2017) 4:11. doi: 10.3389/fnut.2017.00011
78. Phan M, Momin SR, Senn MK, Wood AC. Metabolomic insights into the effects of breast milk versus formula milk feeding in infants. Curr Nutr Rep. (2019) 8:295–306. doi: 10.1007/s13668-019-00284-2
79. Zhu B, Zheng S, Lin K, Xu X, Lv L, Zhao Z, et al. Effects of infant formula supplemented with prebiotics and OPO on infancy fecal microbiota: a pilot randomized clinical trial. Front Cell Infect Microbiol. (2021) 11:650407. doi: 10.3389/fcimb.2021.650407
80. Ma J, Li Z, Zhang W, Zhang C, Zhang Y, Mei H, et al. Comparison of gut microbiota in exclusively breast-fed and formula-fed babies: a study of 91 term infants. Sci Rep. (2020) 10:15792. doi: 10.1038/s41598-020-72635-x
81. Al Nabhani Z, Dulauroy S, Marques R, Cousu C, Al Bounny S, Déjardin F, et al. A weaning reaction to microbiota is required for resistance to immunopathologies in the adult. Immunity. (2019) 50:1276–88.e5. doi: 10.1016/j.immuni.2019.02.014
82. Good M, Sodhi CP, Egan CE, Afrazi A, Jia H, Yamaguchi Y, et al. Breast milk protects against the development of necrotizing enterocolitis through inhibition of Toll-like receptor 4 in the intestinal epithelium via activation of the epidermal growth factor receptor. Mucosal Immunol. (2015) 8:1166–79. doi: 10.1038/mi.2015.30
83. World Health Organization. Available online at: https://www.who.int/health-topics/complementary-feeding#tab=tab_1 (accessed April 2, 2021).
84. Agostoni C, Przyrembel H. The timing of introduction of complementary foods and later health. World Rev Nutr Diet. (2013) 108:63–70. doi: 10.1159/000351486
85. Differding MK, Benjamin-Neelon SE, Hoyo C, Østbye T, Mueller NT. Timing of complementary feeding is associated with gut microbiota diversity and composition and short chain fatty acid concentrations over the first year of life. BMC Microbiol. (2020) 20:56. doi: 10.1186/s12866-020-01723-9
86. Homann CM, Rossel CAJ, Dizzell S, Bervoets L, Simioni J, Li J, et al. Infants' first solid foods: impact on gut microbiota development in two intercontinental cohorts. Nutrients. (2021) 13:2639. doi: 10.3390/nu13082639
Keywords: microbiota, prenatal factors, gut maturation, early life, epigenetics
Citation: Socha-Banasiak A, Pawłowska M, Czkwianianc E and Pierzynowska K (2021) From Intrauterine to Extrauterine Life—The Role of Endogenous and Exogenous Factors in the Regulation of the Intestinal Microbiota Community and Gut Maturation in Early Life. Front. Nutr. 8:696966. doi: 10.3389/fnut.2021.696966
Received: 18 April 2021; Accepted: 29 November 2021;
Published: 17 December 2021.
Edited by:
Kirsi Laitinen, University of Turku, FinlandReviewed by:
Gonzalo Solís Sánchez, Central University Hospital of Asturias, SpainCopyright © 2021 Socha-Banasiak, Pawłowska, Czkwianianc and Pierzynowska. This is an open-access article distributed under the terms of the Creative Commons Attribution License (CC BY). The use, distribution or reproduction in other forums is permitted, provided the original author(s) and the copyright owner(s) are credited and that the original publication in this journal is cited, in accordance with accepted academic practice. No use, distribution or reproduction is permitted which does not comply with these terms.
*Correspondence: Anna Socha-Banasiak, c29jaGFiYW5hc2lha0BnbWFpbC5jb20=
Disclaimer: All claims expressed in this article are solely those of the authors and do not necessarily represent those of their affiliated organizations, or those of the publisher, the editors and the reviewers. Any product that may be evaluated in this article or claim that may be made by its manufacturer is not guaranteed or endorsed by the publisher.
Research integrity at Frontiers
Learn more about the work of our research integrity team to safeguard the quality of each article we publish.