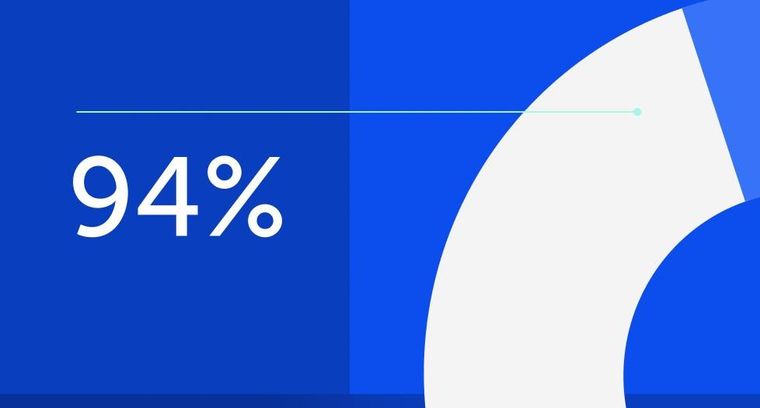
94% of researchers rate our articles as excellent or good
Learn more about the work of our research integrity team to safeguard the quality of each article we publish.
Find out more
MINI REVIEW article
Front. Nutr., 13 May 2021
Sec. Nutrition and Metabolism
Volume 8 - 2021 | https://doi.org/10.3389/fnut.2021.655833
This article is part of the Research TopicDietary Protein for Performance, Health and Disease ManagementView all 11 articles
Low protein diets are associated with increased lifespan and improved cardiometabolic health primarily in rodents, and likely improve human health. There is strong evidence that moderate to severe reduction in dietary protein content markedly influences caloric intake and energy expenditure, which is often followed by a decrease in body weight and adiposity in animal models. While the neuroendocrine signals that trigger hyperphagic responses to protein restriction are better understood, there is accumulating evidence that increased sympathetic flux to brown adipose tissue, fibroblast growth factor-21 and serotonergic signaling are important for the thermogenic effects of low protein diets. This mini-review specifically focuses on the effect of low protein diets with variable carbohydrate and lipid content on energy intake and expenditure, and the underlying mechanisms of actions by these diets. Understanding the mechanisms by which protein restriction influences energy balance may unveil novel approaches for treating metabolic disorders in humans and improve production efficiency in domestic animals.
Energy balance is a fundamental biological process that is dependent on a complex interplay of calories consumed as macronutrients (carbohydrate, fat, and protein), and energy expended and stored. A dysregulation of the mechanisms that sense and signal dietary nutrients in the gut may predispose to obesity and metabolic complications. Among the macronutrients, the intake of protein is tightly regulated and dietary protein restriction is purported to extend lifespan, improve energy balance and cardiometabolic health (1); however, the underlying mechanisms remain poorly understood. Here we review the potential mechanisms by which dietary protein restriction modulates energy homeostasis to alter energy intake and energy expenditure (EE).
The “protein leverage” hypothesis posits that protein intake is tightly regulated in several species including rodents, small animal pets, birds and humans (2–4). When isocaloric high protein diets are fed, in order to keep the amount of protein consumed constant and avoid protein excess, the total caloric intake and hence the intake of carbohydrates and fats is reduced. As a corollary, when low protein diets are fed, in order to avoid protein deficiency and to meet the protein requirements, the total food consumption is increased and hence the total caloric intake from carbohydrate and fat is also increased as a consequence (2, 3, 5). In humans, diets that are moderately deficient in protein were reported to increase food consumption in some (6–8), but not all (9, 10) studies. Notably, protein intake across 13 countries was found to be remarkably stable at ~16% of total calories (11), and even partial protein leverage caused by a reduction in protein intake was predicted to contribute to at least one-third of weight gain and the obesity epidemic (12). In contrast to other species, previous studies were unable to detect a hyperphagic response to mild [<25% lower crude protein (CP) than requirements] and moderate (25–50% lower CP than requirement) protein restriction in pigs. We and others showed that moderate protein restriction (12–14% CP) reduced feed intake in pigs (13–15), but slightly low protein diets (25% lower CP than requirement) did not change their energy intake (14, 16, 17). Reduced food intake in response to low protein diets (22% metabolizable energy) has been also reported in cats (18). However, severely low protein diets (50% lower CP than requirements) have been shown to increase the energy intake in pigs (19–21). A caveat is that most swine studies have primarily focused on improving production efficiency by supplementing essential amino acids to low protein diets, which adds complexity in interpreting the energy intake data. The resistance of species such as pigs to mount a hyperphagic response to mild to moderate dietary protein restriction, but showing an increased energy intake in response to severe protein restriction, is suggestive of differences in protein dilution threshold sensing by different species, which warrants further studies. Thus, gaining insights into the mechanisms of food intake regulation by low protein diets is important for developing effective prevention strategies for weight gain and improving feed efficiency.
The hepatic amino acid sensing and signaling mechanisms play an important role in detecting amino acid insufficiency to coordinate a systemic response to restore protein balance. A relative deficiency of dietary amino acids leads to accumulation of uncharged cytoplasmic tRNA that bind to general control non-derepressible (GCN2) which in turn phosphorylates eukaryotic translation initiation factorα (eIF2α) leading to activation of activating transcription factor 4 (ATF4) and CCAAT/Enhancer-binding protein homologous protein (CHOP) to inhibit protein synthesis and increase fibroblast growth factor-21 (FGF21) expression and secretion (22–25). Further, GCN2 through other intermediaries inactivates mTORC1 leading to dephosphorylation of 4E-binding protein (4EBP1) to inhibit protein synthesis (22, 26). Consistent with these studies that were mostly conducted in vitro and with amino acids, we (27) and others (28–30) showed that a similar pathway for sensing dietary protein deficiency also operates in the liver to upregulate hepatic FGF21 expression and secretion. Interestingly, we found that similar amino acid sensing pathways were also upregulated in the duodenum (31) suggesting that the intestine may detect protein deficiency prior to the liver, and/or that the intestinal sensing may serve to amplify the hepatic response to protein restriction. Independent of sensing by gut-associated tissues, amino acid deprivation causes a rapid anorexic response that is triggered by GCN2 signaling in the piriform cortex (32, 33).
Accumulating evidence indicates that moderate protein restriction in rodents (5–8% protein kcal) stimulates FGF21 secretion from the liver which acts on β-klotho receptors in the brain to promote hyperphagia (34–36) (Figure 1). We have also shown that such hyperphagic responses to protein dilution are a consequence of increased meal size in rodents (31). The hyperphagic responses to moderate protein dilution are also associated with reduction in circulating concentrations of leptin and IGF-1, increased plasma ghrelin, and upregulation of orexigenic neuropeptide Y transcripts in the rodent hypothalamus (27, 31, 37, 38). Although the role of anorexigenic lower gut peptides such as peptide YY and glucagon-like peptide-1 (GLP-1) in high-protein induced hypophagia is well-documented in rodents (39–41) and humans (42), we were unable to detect a graded insufficiency in circulating concentrations of these and other anorectic gut hormones (e.g., gastric inhibitory polypeptide and amylin) in rats (27, 31) that might explain the hyperphagia with protein dilution. It is unknown whether sensitivity to hypophagic effects of these satiety hormones is impaired with dietary protein restriction. However, birds fed with low protein diets were found to have a higher occurrence of GLP-1-immunoreactive cells in the ileum (43). Though the hyperphagic responses to protein dilution are consistently observed in a no-choice condition (27–31, 36), when protein restricted rodents are given a choice of macronutrients, they tend to prefer protein (8, 34, 44) with reduced preference for carbohydrates (45, 46). Similarly, in humans, the fMRI BOLD responses to food cues were greater in reward areas such as orbitofrontal cortex and striatum under low protein conditions (7). In contrast to mild protein restriction, severe protein restriction or depletion (<5% protein kcal) leads to profound hypophagia with a rapid reduction in both meal size and number in rats (31). Such aversive responses are due to rapid sensing of the amino acid imbalance by the anterior piriform cortex where the GCN2–eIF2α pathway is activated to repress protein synthesis, together with activation of glutamatergic outputs to the hypothalamus to cause anorexia (47). The relative importance of peripheral and central sensing and signaling mechanisms that regulate food intake in response to varying degrees of dietary protein restriction across species remains to be resolved.
Figure 1. Potential mechanisms by which protein dilution alters energy intake and energy expenditure. Protein restriction concurrently increases energy intake and energy expenditure. Amino acids insufficiency is sensed by liver, small intestine, and brain by the involvement of unchanged tRNA, non-derepressible (GCN2), eukaryotic translation initiation factorα (eIF2α) and activating transcription factor 4 (ATF4) which results in hyperphagia to restore protein balance. The low protein induced hyperphagia is possibly mediated by increased circulating concentrations of ghrelin and fibroblast growth factor-21 (FGF21), and neuropeptide Y (NPY) expression in the hypothalamus. Whether reduced secretion of anorexigenic gut peptides such as peptide YY (PYY) and glucagon-like peptide 1 (GLP-1) contribute to increased energy intake following protein dilution is not completely known. The increased energy expenditure in response to protein restriction is mediated by multiple mechanisms including increased sympathetic flux and upregulation of thermogenic markers in brown adipose tissue (BAT), and muscle thermogenesis. Whether local FGF21 secreted by skeletal muscle, small intestine and BAT, and 5-hydroxytryptamine (5HT) signaling in BAT play a role in low protein induced thermogenesis via autocrine, paracrine and endocrine pathways remains unclear. B-AR, beta adrenergic receptors; UCP1, uncoupling protein-1; PGC-1α, proliferator-activated receptor gamma coactivator 1-alpha; TPH1, tryptophan hydroxylase 1; SERT, serotonin transporter.
Protein or total amino acid restriction increases thermogenesis in rodents (27, 29, 31, 35, 36, 48–61). An increased EE was also reported in pigs fed with severely low protein diets (19–21). In one study, pigs maintained equal body weight when fed protein deficient diets with high or low energy content suggestive of enhanced EE in these groups (62). We also showed that moderate reduction of dietary protein results in an increased EE in early weeks of study in young pigs (13, 14). Similarly, protein restricted diets have also been shown to acutely increase EE in humans (63–65). Overall, increased EE and subsequently reduced food efficiency may contribute to reduced weight gain and lean mass during protein restriction (27, 36, 56, 58, 66).
Given the concurrent increase in EE and energy intake in response to protein restriction (27, 51, 56, 60), and as increased EE is generally considered a compensatory response to hyperphagia (67–69), one may question whether the enhanced EE with protein restriction is a consequence of increased energy intake. Leveraging effect of dietary protein content on energy intake has been reported previously (49) suggesting that low protein induced EE could be considered as a primary response to dietary protein content. Recently, we showed that protein restricted rats sustain an enhanced EE in the absence of hyperphagia, which is suggestive of an energy intake independent pathway for low protein induced thermogenesis (31). Likewise, others have shown that low protein induced EE occurs independent of hyperphagia (35) and that energy intake changes as a secondary response to compensate for the enhanced EE (70). Therefore, it appears that increased EE in response to protein restriction is partly related to enhanced basal metabolic rate component of total EE (53), although the contribution of basal metabolic rate and diet-induced thermogenesis was reported to be negligible (71). An increased spontaneous motor activity was reported in mice fed with low protein diets (71), which does not seem to be related with the overall activity level (69, 71). Further research is required to better understand the effect of low protein diets with variable dietary carbohydrate and fat contents on different components of total EE.
Due to high carbohydrate content of low protein diets (13, 14, 27, 48, 49, 51, 53–56, 60, 61) an increased EE in response to such diets could be the result of either high carbohydrate or low protein content. In an independent study, using obesity-prone rats we showed that low protein diets with fixed carbohydrate, but variable fat contents increased EE (31), similar to low protein-high carbohydrate diets (27). A greater EE response to protein dilution was also observed in humans regardless of dietary carbohydrate and fat content (65). Altogether, these studies suggest that enhanced EE is likely a primary response to protein restriction rather than carbohydrate or lipid content, or energy intake; however, further studies are required to assess the contribution of dietary carbohydrate and fat content to enhanced EE under protein restriction.
Despite intense efforts to gain insights into the hyperphagic responses driven by protein dilution, the underlying mechanisms of changes in EE received less scrutiny. The thermogenic effects of low protein diets have been associated with (i) increased sympathetic flux to brown adipose tissue (BAT) via β-adrenergic receptor (β-AR) signaling (50, 51, 55–57, 72, 73), as well as stimulation of thermogenesis in white adipose tissue (74) and muscle (27), (ii) FGF21 and mitochondrial uncoupling protein-1 (UCP1) mediated mechanisms (27, 29, 31, 36, 70, 75) and (iii) serotonergic signaling (27, 76) (Figure 1).
The BAT plays an important role in diet-induced thermogenesis (77–79). An increased sympathetic influx to BAT appears to be essential for the thermogenic effects of low protein diets (50–52, 55, 56). This involves release of noradrenaline from postganglionic sympathetic nerve terminals and subsequent interaction of noradrenaline with β-AR, in particular β3-AR in BAT (80–83). We and others showed that the transcripts of adrenergic signaling and thermogenic markers including β2 and β3-AR, peroxisome proliferator-activated receptor gamma coactivator 1-alpha and UCP1 were increased in BAT of rats fed with protein deficient diets (27, 57). We also showed that low protein induced EE is attenuated following administration of propranolol, a β1 and β2-AR antagonist (27, 31). This suggests that low protein induced EE is mediated by sympathetic signaling.
Fibroblast growth factor-21 is released in response to nutrient deficiency and stimulates browning of white adipose tissue to regulate adaptive thermogenesis (53, 74). Infusion of FGF21 increases EE and core body temperature in rodent models (84–86). Using FGF21 deficient rodent models, the effect of protein restricted diets on basal and cold-induced EE have been shown to be FGF21 dependent (35, 61, 87, 88). Although hepatic FGF21 seems to stimulate BAT thermogenesis via an endocrine pathway (29, 30, 36, 74, 89), the FGF21 expression and secretion in BAT is also increased by sympathetic stimulation (72, 90). We and others showed that plasma FGF21 concentrations and transcripts in liver, small intestine, skeletal muscle and BAT were increased when rodents are fed with low protein diets (27, 31, 36, 53, 60, 75, 91). Further, methionine and leucine restriction increases FGF21 expression particularly in liver and circulation (92–94). Dietary protein restriction also increases circulating FGF21 concentrations in humans (36, 61, 63–65). FGF21 appears to be produced in variety of organs including skeletal muscle in response to cellular stress triggered by various stimuli (95–99). Whether FGF21 derived from BAT, muscle and other organs reaches the circulation and contributes to low protein induced thermogenesis via endocrine pathway remains unclear, but it appears that FGF21 most likely mediates the low protein induced EE through endocrine, paracrine and autocrine signaling. Further, FGF21 signaling in glutamatergic neurons of the ventromedial hypothalamus appears to be essential for the increase in EE with dietary protein dilution (45). The roles of peripheral and central FGF21 sensing and signaling mechanisms in the thermogenic effects of dietary protein restriction remains to be further delineated.
The role of serotonergic neurons in regulation of thermogenesis and BAT activity has been previously documented (100, 101). In particular, central serotonin signaling is crucial for the activity of BAT and thermoregulation (102–104). Serotonergic signaling also appears to be associated with low protein induced EE. We showed that the mRNA abundance of tryptophan hydroxylase 1, an enzyme involved in biosynthesis of serotonin, and serotonin transporter is increased in the BAT of rats fed with low protein diets which might suggest a paracrine or autocrine control of low protein enhanced EE by local 5-hydroxytryptamine (5-HT or serotonin). The VO2 and EE were shown to be decreased by administration of a non-selective 5-HT receptor antagonist, metergoline (76) and 5-HT3 receptor antagonist, ondansetron (27) in rats fed low protein diets. This is suggestive of higher serotonergic tone in rats fed with protein restricted diets. Whether both central and peripheral serotonergic signaling are equally essential for the thermogenic effects of low protein diets remains to be studied.
Dietary protein restriction results in reduced concentration of most essential amino acids in the circulation, which play a role in metabolic adaptations to protein deficient diets. Among the amino acids studied, methionine (60, 105–108), tryptophan (41), and leucine (109) restriction have been shown to enhance EE. This is suggestive of the importance of amino acid profile and protein quality in regulation of thermogenesis. We showed that methionine restriction can partly recapitulate the total amino acid restriction-induced EE (48). This increase in EE in response to methionine restriction has been linked with greater secretion of hepatic FGF21 (92, 94, 110), upregulation of UCP1 in BAT (105, 107) and increased sympathetic signaling (48, 108). We and others using pharmacological (i.e., chemical sympathectomy and propranolol) and genetic (i.e., β3 receptor knockout mice) tools, showed that sympathetically driven enhanced EE in response to methionine restriction is mediated by β-AR (48, 108). Similarly, increased EE following tryptophan and leucine restrictions is mediated through sympathetic system and upregulation of UCP1 in BAT as well as FGF21 (41, 93, 111, 112). Whether deficiency of other essential amino acids play a role in the higher thermogenic effects of low protein diets, and the underlying pathways and organs involved, warrants further investigation.
Dietary protein restriction orchestrates a whole organismal physiological response to stimulate energy intake and EE. The low protein induced thermogenesis is largely driven by dietary protein content and less likely by the concurrent hyperphagia. Though the liver and brain appear to be the primary sites for sensing protein deficiency, the coordination of these tissues with intestinal sensing mechanisms to promote hyperphagia and thermogenesis remains poorly understood. Although liver driven FGF21 seems essential for stimulating EE in response to protein or amino acid restricted diets, the role of local FGF21 synthesized and released from BAT, skeletal muscle and gut in the thermogenic responses to low protein diets remains to be determined. Further, the roles of systemic and local amino acid concentrations, as well as sympathetic and serotonergic signaling in brain, adipose and muscle tissues to coordinate intake and expenditure responses to dietary protein restriction remains largely unexplored. A deeper understanding of the neuroendocrine mechanisms by which dietary protein dilution modulates energy balance may lead to the development of novel strategies for preventing and treating obesity and associated comorbidities in humans, as well as for improving production efficiency in domestic animals.
AP and PC wrote the article and agree to be accountable for the content of the work. All authors contributed to the article and approved the submitted version.
This work was supported by Animal Health and Production and Animal Products: Improved Nutritional Performance, Growth, and Lactation of Animals grant no. 2018-67016-27471/project accession no. 1014892, grant no. 2021-67016-33399/project accession no. 1024334 and Hatch project accession no. 1012889 from the USDA National Institute of Food and Agriculture (NIFA) to AP; and by operating grants from the Natural Sciences and Engineering Research Council of Canada (NSERC no. 355993) and funding from the Texas Tech University School of Veterinary Medicine to PC.
The authors declare that the research was conducted in the absence of any commercial or financial relationships that could be construed as a potential conflict of interest.
1. Kitada M, Ogura Y, Monno I, Koya D. The impact of dietary protein intake on longevity and metabolic health. EBioMedicine. (2019) 43:632–40. doi: 10.1016/j.ebiom.2019.04.005
2. Raubenheimer D, Machovsky-Capuska GE, Gosby AK, Simpson S. Nutritional ecology of obesity: from humans to companion animals. Br J Nutr. (2015) 113:S26–39. doi: 10.1017/S0007114514002323
3. Simpson SJ, Raubenheimer D. Obesity: the protein leverage hypothesis. Obes Rev. (2005) 6:133–42. doi: 10.1111/j.1467-789X.2005.00178.x
4. Buyse J, Decuypere E, Berghman L, Kuhn ER, Vandesande F. Effect of dietary protein content on episodic growth hormone secretion and on heat production of male broiler chickens. Br Poult Sci. (1992) 33:1101–9. doi: 10.1080/00071669208417552
5. Gosby AK, Conigrave AD, Raubenheimer D, Simpson SJ. Protein leverage and energy intake. Obes Rev. (2014) 15:183–91. doi: 10.1111/obr.12131
6. Gosby AK, Conigrave AD, Lau NS, Iglesias MA, Hall RM, Jebb SA, et al. Testing protein leverage in lean humans: a randomised controlled experimental study. PLoS ONE. (2011) 6:e25929. doi: 10.1371/journal.pone.0025929
7. Griffioen-Roose S, Smeets PA, vanden Heuvel E, Boesveldt S, Finlayson G, de Graaf C. Human protein status modulates brain reward responses to food cues. Am J Clin Nutr. (2014) 100:113–22. doi: 10.3945/ajcn.113.079392
8. Griffioen-Roose S, Mars M, Siebelink E, Finlayson G, Tome D, de GC. Protein status elicits compensatory changes in food intake and food preferences. Am J Clin Nutr. (2012) 95:32–8. doi: 10.3945/ajcn.111.020503
9. Martens EA, Tan SY, Dunlop MV, Mattes RD, Westerterp-Plantenga MS. Protein leverage effects of beef protein on energy intake in humans. Am J Clin Nutr. (2014) 99:1397–406. doi: 10.3945/ajcn.113.078774
10. Martens EA, Tan SY, Mattes RD, Westerterp-Plantenga MS. No protein intake compensation for insufficient indispensable amino acid intake with a low-protein diet for 12 days. Nutr Metab. (2014) 11:38. doi: 10.1186/1743-7075-11-38
11. Simpson SJ, Raubenheimer D. The power of protein. Am J Clin Nutr. (2020) 112:6–7. doi: 10.1093/ajcn/nqaa088
12. Hall KD. The potential role of protein leverage in the US obesity epidemic. Obesity. (2019) 27:1222–4. doi: 10.1002/oby.22520
13. Spring S, Premathilake H, Bradway C, Shili C, DeSilva U, Carter S, et al. Effect of very low-protein diets supplemented with branched-chain amino acids on energy balance, plasma metabolomics and fecal microbiome of pigs. Sci Rep. (2020) 10:15859. doi: 10.1038/s41598-020-72816-8
14. Spring S, Premathilake H, DeSilva U, Shili C, Carter S, Pezeshki A. Low protein-high carbohydrate diets alter energy balance, gut microbiota composition and blood metabolomics profile in young pigs. Sci Rep. (2020) 10:3318. doi: 10.1038/s41598-020-60150-y
15. Yin J, Ma J, Li Y, Ma X, Chen J, Zhang H, et al. Branched-chain amino acids, especially of leucine and valine, mediate the protein restricted response in a piglet model. Food Funct. (2020) 11:1304–11. doi: 10.1039/c9fo01757g
16. Le Bellego L, van Milgen J, Noblet J. Effect of high temperature and low-protein diets on the performance of growing-finishing pigs. J Anim Sci. (2002) 80:691–701. doi: 10.2527/2002.803691x
17. Kerr BJ, Southern LL, Bidner TD, Friesen KG, Easter RA. Influence of dietary protein level, amino acid supplementation, and dietary energy levels on growing-finishing pig performance and carcass composition. J Anim Sci. (2003) 81:3075–87. doi: 10.2527/2003.81123075x
18. Allaway D, de Alvaro CH, Hewson-Hughes A, Staunton R, Morris P, Alexander J. Impact of dietary macronutrient profile on feline body weight is not consistent with the protein leverage hypothesis. Br J Nutr. (2018) 120:1310–8. doi: 10.1017/S000711451800257X
19. Gurr MI, Mawson R, Rothwell NJ, Stock MJ. Effects of manipulating dietary protein and energy intake on energy balance and thermogenesis in the pig. J Nutr. (1980) 110:532–42. doi: 10.1093/jn/110.3.532
20. Fuller MF. Energy and nitrogen balances in young pigs maintained at constant weight with diets of differing protein content. J Nutr. (1983) 113:15–20. doi: 10.1093/jn/113.1.15
21. Miller DS, Payne PR. Weight maintenance and food intake. J Nutr. (1962) 78:255–62. doi: 10.1093/jn/78.3.255
22. Broer S, Broer A. Amino acid homeostasis and signalling in mammalian cells and organisms. Biochem J. (2017) 474:1935–63. doi: 10.1042/BCJ20160822
23. Wan XS, Lu XH, Xiao YC, Lin Y, Zhu H, Ding T, et al. ATF4- and CHOP-dependent induction of FGF21 through endoplasmic reticulum stress. Biomed Res Int. (2014) 2014:807874. doi: 10.1155/2014/807874
24. Salminen A, Kaarniranta K, Kauppinen A. Integrated stress response stimulates FGF21 expression: systemic enhancer of longevity. Cell Signal. (2017) 40:10–21. doi: 10.1016/j.cellsig.2017.08.009
25. De Sousa-Coelho AL, Marrero PF, Haro D. Activating transcription factor 4-dependent induction of FGF21 during amino acid deprivation. Biochem J. (2012) 443:165–71. doi: 10.1042/BJ20111748
26. Battu S, Minhas G, Mishra A, Khan N. Amino acid sensing via general control nonderepressible-2 kinase and immunological programming. Front Immunol. (2017) 8:1719. doi: 10.3389/fimmu.2017.01719
27. Pezeshki A, Zapata RC, Singh A, Yee NJ, Chelikani PK. Low protein diets produce divergent effects on energy balance. Sci Rep. (2016) 6:25145. doi: 10.1038/srep25145
28. Raimondi C. Neuropilin-1 enforces extracellular matrix signalling via ABL1 to promote angiogenesis. Biochem Soc Trans. (2014) 42:1429–34. doi: 10.1042/BST20140141
29. Laeger T, Albarado DC, Burke SJ, Trosclair L, Hedgepeth JW, Berthoud HR, et al. Metabolic responses to dietary protein restriction require an increase in FGF21 that is delayed by the absence of GCN2. Cell Rep. (2016) 16:707–16. doi: 10.1016/j.celrep.2016.06.044
30. Hill CM, Laeger T, Dehner M, Albarado DC, Clarke B, Wanders D, et al. FGF21 signals protein status to the brain and adaptively regulates food choice and metabolism. Cell Rep. (2019) 27:2934–47.e3. doi: 10.1016/j.celrep.2019.05.022
31. Zapata RC, Singh A, Pezeshki A, Avirineni BS, Patra S, Chelikani PK. Low-protein diets with fixed carbohydrate content promote hyperphagia and sympathetically mediated increase in energy expenditure. Mol Nutr Food Res. (2019) 63:e1900088. doi: 10.1002/mnfr.201900088
32. Hao S, Sharp JW, Ross-Inta CM, McDaniel BJ, Anthony TG, Wek RC, et al. Uncharged tRNA and sensing of amino acid deficiency in mammalian piriform cortex. Science. (2005) 307:1776–8. doi: 10.1126/science.1104882
33. Rudell JB, Rechs AJ, Kelman TJ, Ross-Inta CM, Hao S, Gietzen DW. The anterior piriform cortex is sufficient for detecting depletion of an indispensable amino acid, showing independent cortical sensory function. J Neurosci. (2011) 31:1583–90. doi: 10.1523/JNEUROSCI.4934-10.2011
34. Lee Y. Handwritten digit recognition using K nearest-neighbor, radial-basis function, and backpropagation neural networks. Neural Comput. (1991) 3:440–9. doi: 10.1162/neco.1991.3.3.440
35. Hill CM, Laeger T, Albarado DC, McDougal DH, Berthoud HR, Munzberg H, et al. Low protein-induced increases in FGF21 drive UCP1-dependent metabolic but not thermoregulatory endpoints. Sci Rep. (2017) 7:8209. doi: 10.1038/s41598-017-07498-w
36. Laeger T, Henagan TM, Albarado DC, Redman LM, Bray GA, Noland RC, et al. FGF21 is an endocrine signal of protein restriction. J Clin Invest. (2014) 124:3913–22. doi: 10.1172/JCI74915
37. White BD, Porter MH, Martin RJ. Effects of age on the feeding response to moderately low dietary protein in rats. Physiol Behav. (2000) 68:673–81. doi: 10.1016/s0031-9384(99)00229-2
38. White BD, Dean RG, Martin RJ. An association between low levels of dietary protein, elevated NPY gene expression in the basomedial hypothalamus and increased food intake. Nutr Neurosci. (1998) 1:173–82. doi: 10.1080/1028415X.1998.11747227
39. Reidelberger R, Haver A, Chelikani PK. Role of peptide YY(3-36) in the satiety produced by gastric delivery of macronutrients in rats. Am J Physiol Endocrinol Metab. (2013) 304:E944–50. doi: 10.1152/ajpendo.00075.2013
40. Mietlicki-Baase EG, Koch-Laskowski K, McGrath LE, Krawczyk J, Pham T, Lhamo R, et al. Daily supplementation of dietary protein improves the metabolic effects of GLP-1-based pharmacotherapy in lean and obese rats. Physiol Behav. (2017) 177:122–8. doi: 10.1016/j.physbeh.2017.04.017
41. Zapata RC, Singh A, Ajdari NM, Chelikani PK. Dietary tryptophan restriction dose-dependently modulates energy balance, gut hormones, and microbiota in obesity-prone rats. Obesity. (2018) 26:730–9. doi: 10.1002/oby.22136
42. Batterham RL, Heffron H, Kapoor S, Chivers JE, Chandarana K, Herzog H, et al. Critical role for peptide YY in protein-mediated satiation and body-weight regulation. Cell Metab. (2006) 4:223–33. doi: 10.1016/j.cmet.2006.08.001
43. Monir MM, Hiramatsu K, Matsumoto S, Nishimura K, Takemoto C, Shioji T, et al. Influences of protein ingestion on glucagon-like peptide (GLP)-1-immunoreactive endocrine cells in the chicken ileum. Anim Sci J. (2014) 85:581–7. doi: 10.1111/asj.12177
44. Bilheimer DW. Metabolic effects of portacaval shunt surgery and liver transplantation in familial hypercholesterolemia. Beitr Infusionsther. (1988) 23:61–73
45. Flippo KH, Jensen-Cody SO, Claflin KE, Potthoff MJ. FGF21 signaling in glutamatergic neurons is required for weight loss associated with dietary protein dilution. Sci Rep. (2020) 10:19521. doi: 10.1038/s41598-020-76593-2
46. Jensen-Cody SO, Flippo KH, Claflin KE, Yavuz Y, Sapouckey SA, Walters GC, et al. FGF21 signals to glutamatergic neurons in the ventromedial hypothalamus to suppress carbohydrate Intake. Cell Metab. (2020) 32:273–86.e6. doi: 10.1016/j.cmet.2020.06.008
47. Anthony TG, Gietzen DW. Detection of amino acid deprivation in the central nervous system. Curr Opin Clin Nutr Metab Care. (2013) 16:96–101. doi: 10.1097/MCO.0b013e32835b618b
48. Spring S, Singh A, Zapata RC, Chelikani PK, Pezeshki A. Methionine restriction partly recapitulates the sympathetically mediated enhanced energy expenditure induced by total amino acid restriction in rats. Nutrients. (2019) 11:707. doi: 10.3390/nu11030707
49. Hu S, Wang L, Yang D, Li L, Togo J, Wu Y, et al. Dietary fat, but not protein or carbohydrate, regulates energy intake and causes adiposity in mice. Cell Metab. (2018) 28:415–31.e4. doi: 10.1016/j.cmet.2018.06.010
50. Rothwell NJ, Stock MJ. Influence of carbohydrate and fat intake on diet-induced thermogenesis and brown fat activity in rats fed low protein diets. J Nutr. (1987) 117:1721–6. doi: 10.1093/jn/117.10.1721
51. Rothwell NJ, Stock MJ, Tyzbir RS. Mechanisms of thermogenesis induced by low protein diets. Metabolism. (1983) 32:257–61. doi: 10.1016/0026-0495(83)90190-7
52. Rothwell NJ, Stock MJ, Tyzbir RS. Energy balance and mitochondrial function in liver and brown fat of rats fed “cafeteria” diets of varying protein content. J Nutr. (1982) 112:1663–72. doi: 10.1093/jn/112.9.1663
53. Dommerholt MB, Blankestijn M, Vieira-Lara MA, van Dijk TH, Wolters H, Koster MH, et al. Short-term protein restriction at advanced age stimulates FGF21 signalling, energy expenditure and browning of white adipose tissue. FEBS J. (2020) 288:2257–77. doi: 10.1111/febs.15604
54. Pereira MP, Ferreira LAA, da Silva FHS, Christoffolete MA, Metsios GS, Chaves VE, et al. A low-protein, high-carbohydrate diet increases browning in perirenal adipose tissue but not in inguinal adipose tissue. Nutrition. (2017) 42:37–45. doi: 10.1016/j.nut.2017.05.007
55. Aparecida de Franca S, Pavani Dos Santos M, Nunes Queiroz da Costa RV, Froelich M, Buzelle SL, Chaves VE, et al. Low-protein, high-carbohydrate diet increases glucose uptake and fatty acid synthesis in brown adipose tissue of rats. Nutrition. (2014) 30:473–80. doi: 10.1016/j.nut.2013.10.004
56. Aparecida de Franca S, Dos Santos MP, Garofalo MA, Navegantes LC, Kettelhut Ido C, Lopes CF, et al. Low protein diet changes the energetic balance and sympathetic activity in brown adipose tissue of growing rats. Nutrition. (2009) 25:1186–92. doi: 10.1016/j.nut.2009.03.011
57. de Franca SA, dos Santos MP, Przygodda F, Garofalo MA, Kettelhut IC, Magalhaes DA, et al. A low-protein, high-carbohydrate diet stimulates thermogenesis in the brown adipose tissue of rats via ATF-2. Lipids. (2016) 51:303–10. doi: 10.1007/s11745-016-4119-z
58. Huang X, Hancock DP, Gosby AK, McMahon AC, Solon SM, Le Couteur DG, et al. Effects of dietary protein to carbohydrate balance on energy intake, fat storage, and heat production in mice. Obesity. (2013) 21:85–92. doi: 10.1002/oby.20007
59. Solon-Biet SM, Mitchell SJ, Coogan SC, Cogger VC, Gokarn R, McMahon AC, et al. Dietary protein to carbohydrate ratio and caloric restriction: comparing metabolic outcomes in mice. Cell Rep. (2015) 11:1529–34. doi: 10.1016/j.celrep.2015.05.007
60. Chaumontet C, Azzout-Marniche D, Blais A, Piedcoq J, Tome D, Gaudichon C, et al. Low-protein and methionine, high-starch diets increase energy intake and expenditure, increase FGF21, decrease IGF-1, and have little effect on adiposity in mice. Am J Physiol Regul Integr Comp Physiol. (2019) 316:R486–R501. doi: 10.1152/ajpregu.00316.2018
61. Maida A, Zota A, Sjoberg KA, Schumacher J, Sijmonsma TP, Pfenninger A, et al. A liver stress-endocrine nexus promotes metabolic integrity during dietary protein dilution. J Clin Invest. (2016) 126:3263–78. doi: 10.1172/JCI85946
62. Pond WG, Barnes RH, Bradfield RB, Kwong E, Krook L. Effect of dietary energy intake on protein deficiency symptoms and body composition of baby pigs fed equalized but suboptimal amounts of protein. J Nutr. (1965) 85:57–66. doi: 10.1093/jn/85.1.57
63. Schlogl M, Piaggi P, Pannacciuli N, Bonfiglio SM, Krakoff J, Thearle MS. Energy expenditure responses to fasting and overfeeding identify phenotypes associated with weight change. Diabetes. (2015) 64:3680–9. doi: 10.2337/db15-0382
64. Hollstein T, Ando T, Basolo A, Krakoff J, Votruba SB, Piaggi P. Metabolic response to fasting predicts weight gain during low-protein overfeeding in lean men: further evidence for spendthrift and thrifty metabolic phenotypes. Am J Clin Nutr. (2019) 110:593–604. doi: 10.1093/ajcn/nqz062
65. Vinales KL, Begaye B, Bogardus C, Walter M, Krakoff J, Piaggi P. FGF21 is a hormonal mediator of the human “thrifty” metabolic phenotype. Diabetes. (2019) 68:318–23. doi: 10.2337/db18-0696
66. Du F, Higginbotham DA, White BD. Food intake, energy balance and serum leptin concentrations in rats fed low-protein diets. J Nutr. (2000) 130:514–21. doi: 10.1093/jn/130.3.514
67. Danforth E Jr. Dietary-induced thermogenesis: control of energy expenditure. Life Sci. (1981) 28:1821–7. doi: 10.1016/0024-3205(81)90353-2
68. Himms-Hagen J. Thermogenesis in brown adipose tissue as an energy buffer. Implications for obesity. N Engl J Med. (1984) 311:1549–58. doi: 10.1056/NEJM198412133112407
69. Swick RW, Gribskov CL. The effect of dietary protein levels on diet-induced thermogenesis in the rat. J Nutr. (1983) 113:2289–94. doi: 10.1093/jn/113.11.2289
70. Hill CM, Berthoud HR, Munzberg H, Morrison CD. Homeostatic sensing of dietary protein restriction: a case for FGF21. Front Neuroendocrinol. (2018) 51:125–31. doi: 10.1016/j.yfrne.2018.06.002
71. Blais A, Chaumontet C, Azzout-Marniche D, Piedcoq J, Fromentin G, Gaudichon C, et al. Low-protein diet-induced hyperphagia and adiposity are modulated through interactions involving thermoregulation, motor activity, and protein quality in mice. Am J Physiol Endocrinol Metab. (2018) 314:E139–E51. doi: 10.1152/ajpendo.00318.2017
72. Chartoumpekis DV, Habeos IG, Ziros PG, Psyrogiannis AI, Kyriazopoulou VE, Papavassiliou AG. Brown adipose tissue responds to cold and adrenergic stimulation by induction of FGF21. Mol Med. (2011) 17:736–40. doi: 10.2119/molmed.2011.00075
73. Glick Z, Teague RJ, Bray GA. Brown adipose tissue: thermic response increased by a single low protein, high carbohydrate meal. Science. (1981) 213:1125–7. doi: 10.1126/science.7268419
74. Fisher FM, Kleiner S, Douris N, Fox EC, Mepani RJ, Verdeguer F, et al. FGF21 regulates PGC-1alpha and browning of white adipose tissues in adaptive thermogenesis. Genes Dev. (2012) 26:271–81. doi: 10.1101/gad.177857.111
75. Chalvon-Demersay T, Even PC, Tome D, Chaumontet C, Piedcoq J, Gaudichon C, et al. Low-protein diet induces, whereas high-protein diet reduces hepatic FGF21 production in mice, but glucose and not amino acids up-regulate FGF21 in cultured hepatocytes. J Nutr Biochem. (2016) 36:60–7. doi: 10.1016/j.jnutbio.2016.07.002
76. Rothwell NJ, Stock MJ. Effect of diet and fenfluramine on thermogenesis in the rat: possible involvement of serotonergic mechanisms. Int J Obes. (1987) 11:319–24.
77. Clark I, Brashear HR. Dietary induction of osteitis fibrosa cystica in the rat: roles of parathyroid hormone and calcitonin. Calcif Tissue Res. (1976) 21(Suppl):272–7.
78. Rothwell NJ, Stock MJ. A role for brown adipose tissue in diet-induced thermogenesis. Nature. (1979) 281:31–5. doi: 10.1038/281031a0
79. Rothwell NJ, Stock MJ. A role for brown adipose tissue in diet-induced thermogenesis. Obes Res. (1997) 5:650–6. doi: 10.1002/j.1550-8528.1997.tb00591.x
80. Hsieh AC, Carlson LD. Role of adrenaline and noradrenaline in chemical regulation of heat production. Am J Physiol. (1957) 190:243–6. doi: 10.1152/ajplegacy.1957.190.2.243
81. Zhao J, Unelius L, Bengtsson T, Cannon B, Nedergaard J. Coexisting beta-adrenoceptor subtypes: significance for thermogenic process in brown fat cells. Am J Physiol. (1994) 267(4 Pt 1):C969–79. doi: 10.1152/ajpcell.1994.267.4.C969
82. Thomas SA, Palmiter RD. Thermoregulatory and metabolic phenotypes of mice lacking noradrenaline and adrenaline. Nature. (1997) 387:94–7. doi: 10.1038/387094a0
83. Soderlund V, Larsson SA, Jacobsson H. Reduction of FDG uptake in brown adipose tissue in clinical patients by a single dose of propranolol. Eur J Nucl Med Mol Imaging. (2007) 34:1018–22. doi: 10.1007/s00259-006-0318-9
84. Coskun T, Bina HA, Schneider MA, Dunbar JD, Hu CC, Chen Y, et al. Fibroblast growth factor 21 corrects obesity in mice. Endocrinology. (2008) 149:6018–27. doi: 10.1210/en.2008-0816
85. Xu J, Lloyd DJ, Hale C, Stanislaus S, Chen M, Sivits G, et al. Fibroblast growth factor 21 reverses hepatic steatosis, increases energy expenditure, and improves insulin sensitivity in diet-induced obese mice. Diabetes. (2009) 58:250–9. doi: 10.2337/db08-0392
86. Sarruf DA, Thaler JP, Morton GJ, German J, Fischer JD, Ogimoto K, et al. Fibroblast growth factor 21 action in the brain increases energy expenditure and insulin sensitivity in obese rats. Diabetes. (2010) 59:1817–24. doi: 10.2337/db09-1878
87. Maida A, Zota A, Vegiopoulos A, Appak-Baskoy S, Augustin HG, Heikenwalder M, et al. Dietary protein dilution limits dyslipidemia in obesity through FGF21-driven fatty acid clearance. J Nutr Biochem. (2018) 57:189–96. doi: 10.1016/j.jnutbio.2018.03.027
88. Perez-Marti A, Garcia-Guasch M, Tresserra-Rimbau A, Carrilho-Do-Rosario A, Estruch R, Salas-Salvado J, et al. A low-protein diet induces body weight loss and browning of subcutaneous white adipose tissue through enhanced expression of hepatic fibroblast growth factor 21 (FGF21). Mol Nutr Food Res. (2017) 61:1600725. doi: 10.1002/mnfr.201600725
89. BonDurant LD, Ameka M, Naber MC, Markan KR, Idiga SO, Acevedo MR, et al. FGF21 regulates metabolism through adipose-dependent and -independent mechanisms. Cell Metab. (2017) 25:935–44.e4. doi: 10.1016/j.cmet.2017.03.005
90. Hondares E, Iglesias R, Giralt A, Gonzalez FJ, Giralt M, Mampel T, et al. Thermogenic activation induces FGF21 expression and release in brown adipose tissue. J Biol Chem. (2011) 286:12983–90. doi: 10.1074/jbc.M110.215889
91. Azzout-Marniche D, Chalvon-Demersay T, Pimentel G, Chaumontet C, Nadkarni NA, Piedcoq J, et al. Obesity-prone high-fat-fed rats reduce caloric intake and adiposity and gain more fat-free mass when allowed to self-select protein from carbohydrate:fat intake. Am J Physiol Regul Integr Comp Physiol. (2016) 310:R1169–76. doi: 10.1152/ajpregu.00391.2015
92. Lees EK, Krol E, Grant L, Shearer K, Wyse C, Moncur E, et al. Methionine restriction restores a younger metabolic phenotype in adult mice with alterations in fibroblast growth factor 21. Aging Cell. (2014) 13:817–27. doi: 10.1111/acel.12238
93. Wanders D, Stone KP, Dille K, Simon J, Pierse A, Gettys TW. Metabolic responses to dietary leucine restriction involve remodeling of adipose tissue and enhanced hepatic insulin signaling. Biofactors. (2015) 41:391–402. doi: 10.1002/biof.1240
94. Wanders D, Forney LA, Stone KP, Burk DH, Pierse A, Gettys TW. FGF21 mediates the thermogenic and insulin-sensitizing effects of dietary methionine restriction but not its effects on hepatic lipid metabolism. Diabetes. (2017) 66:858–67. doi: 10.2337/db16-1212
95. Tezze C, Romanello V, Sandri M. FGF21 as modulator of metabolism in health and disease. Front Physiol. (2019) 10:419. doi: 10.3389/fphys.2019.00419
96. Keipert S, Ost M, Johann K, Imber F, Jastroch M, van Schothorst EM, et al. Skeletal muscle mitochondrial uncoupling drives endocrine cross-talk through the induction of FGF21 as a myokine. Am J Physiol Endocrinol Metab. (2014) 306:E469–82. doi: 10.1152/ajpendo.00330.2013
97. Harris LA, Skinner JR, Shew TM, Pietka TA, Abumrad NA, Wolins NE. Perilipin 5-driven lipid droplet accumulation in skeletal muscle stimulates the expression of fibroblast growth factor 21. Diabetes. (2015) 64:2757–68. doi: 10.2337/db14-1035
98. Vandanmagsar B, Warfel JD, Wicks SE, Ghosh S, Salbaum JM, Burk D, et al. Impaired mitochondrial fat oxidation induces FGF21 in muscle. Cell Rep. (2016) 15:1686–99. doi: 10.1016/j.celrep.2016.04.057
99. Pereira RO, Tadinada SM, Zasadny FM, Oliveira KJ, Pires KMP, Olvera A, et al. OPA1 deficiency promotes secretion of FGF21 from muscle that prevents obesity and insulin resistance. EMBO J. (2017) 36:2126–45. doi: 10.15252/embj.201696179
100. Nakamura K, Morrison SF. Central efferent pathways for cold-defensive and febrile shivering. J Physiol. (2011) 589(Pt 14):3641–58. doi: 10.1113/jphysiol.2011.210047
101. Nakamura K, Matsumura K, Hubschle T, Nakamura Y, Hioki H, Fujiyama F, et al. Identification of sympathetic premotor neurons in medullary raphe regions mediating fever and other thermoregulatory functions. J Neurosci. (2004) 24:5370–80. doi: 10.1523/JNEUROSCI.1219-04.2004
102. McGlashon JM, Gorecki MC, Kozlowski AE, Thirnbeck CK, Markan KR, Leslie KL, et al. Central serotonergic neurons activate and recruit thermogenic brown and beige fat and regulate glucose and lipid homeostasis. Cell Metab. (2015) 21:692–705. doi: 10.1016/j.cmet.2015.04.008
103. Fuller NJ, Stirling DM, Dunnett S, Reynolds GP, Ashwell M. Decreased brown adipose tissue thermogenic activity following a reduction in brain serotonin by intraventricular p-chlorophenylalanine. Biosci Rep. (1987) 7:121–7. doi: 10.1007/BF01121875
104. Cerpa V, Gonzalez A, Richerson GB. Diphtheria toxin treatment of Pet-1-Cre floxed diphtheria toxin receptor mice disrupts thermoregulation without affecting respiratory chemoreception. Neuroscience. (2014) 279:65–76. doi: 10.1016/j.neuroscience.2014.08.018
105. Wanders D, Burk DH, Cortez CC, Van NT, Stone KP, Baker M, et al. UCP1 is an essential mediator of the effects of methionine restriction on energy balance but not insulin sensitivity. FASEB J. (2015) 29:2603–15. doi: 10.1096/fj.14-270348
106. Malloy VL, Krajcik RA, Bailey SJ, Hristopoulos G, Plummer JD, Orentreich N. Methionine restriction decreases visceral fat mass and preserves insulin action in aging male Fischer 344 rats independent of energy restriction. Aging Cell. (2006) 5:305–14. doi: 10.1111/j.1474-9726.2006.00220.x
107. Hasek BE, Stewart LK, Henagan TM, Boudreau A, Lenard NR, Black C, et al. Dietary methionine restriction enhances metabolic flexibility and increases uncoupled respiration in both fed and fasted states. Am J Physiol Regul Integr Comp Physiol. (2010) 299:R728–39. doi: 10.1152/ajpregu.00837.2009
108. Plaisance EP, Henagan TM, Echlin H, Boudreau A, Hill KL, Lenard NR, et al. Role of beta-adrenergic receptors in the hyperphagic and hypermetabolic responses to dietary methionine restriction. Am J Physiol Regul Integr Comp Physiol. (2010) 299:R740–50. doi: 10.1152/ajpregu.00838.2009
109. Lees EK, Banks R, Cook C, Hill S, Morrice N, Grant L, et al. Direct comparison of methionine restriction with leucine restriction on the metabolic health of C57BL/6J mice. Sci Rep. (2017) 7:9977. doi: 10.1038/s41598-017-10381-3
110. Forney LA, Wanders D, Stone KP, Pierse A, Gettys TW. Concentration-dependent linkage of dietary methionine restriction to the components of its metabolic phenotype. Obesity. (2017) 25:730–8. doi: 10.1002/oby.21806
111. Cheng Y, Zhang Q, Meng Q, Xia T, Huang Z, Wang C, et al. Leucine deprivation stimulates fat loss via increasing CRH expression in the hypothalamus and activating the sympathetic nervous system. Mol Endocrinol. (2011) 25:1624–35. doi: 10.1210/me.2011-0028
Keywords: low protein, food intake, energy expenditure, neuroendocrine, energy balance
Citation: Pezeshki A and Chelikani PK (2021) Low Protein Diets and Energy Balance: Mechanisms of Action on Energy Intake and Expenditure. Front. Nutr. 8:655833. doi: 10.3389/fnut.2021.655833
Received: 19 January 2021; Accepted: 20 April 2021;
Published: 13 May 2021.
Edited by:
Tyler A. Churchward-Venne, McGill University, CanadaReviewed by:
Tania Paulette Markovic, The University of Sydney, AustraliaCopyright © 2021 Pezeshki and Chelikani. This is an open-access article distributed under the terms of the Creative Commons Attribution License (CC BY). The use, distribution or reproduction in other forums is permitted, provided the original author(s) and the copyright owner(s) are credited and that the original publication in this journal is cited, in accordance with accepted academic practice. No use, distribution or reproduction is permitted which does not comply with these terms.
*Correspondence: Adel Pezeshki, YWRlbC5wZXplc2hraUBva3N0YXRlLmVkdQ==; Prasanth K. Chelikani, cGNoZWxpa2FAdHR1LmVkdQ==
Disclaimer: All claims expressed in this article are solely those of the authors and do not necessarily represent those of their affiliated organizations, or those of the publisher, the editors and the reviewers. Any product that may be evaluated in this article or claim that may be made by its manufacturer is not guaranteed or endorsed by the publisher.
Research integrity at Frontiers
Learn more about the work of our research integrity team to safeguard the quality of each article we publish.