- 1Department of Molecular Biology and Genetics, Çanakkale Onsekiz Mart University, Çanakkale, Turkey
- 2Department of Chemistry, Çanakkale Onsekiz Mart University, Çanakkale, Turkey
- 3Department of Biomedical Engineering, Karabuk University, Karabük, Turkey
- 4Department of Nutrition, University of Nevada, Reno, NV, United States
- 5Department of Food Science and Technology, University of Nebraska Lincoln, Lincoln, NE, United States
Human milk is the optimal source of infant nutrition. Among many other health benefits, human milk can stimulate the development of a Bifidobacterium-rich microbiome through human milk oligosaccharides (HMOs). In recent years, the development of novel formulas has placed particular focus on incorporating some of the beneficial functional properties of human milk. These include adding specific glycans aimed to selectively stimulate the growth of Bifidobacterium. However, the bifidogenicity of human milk remains unparalleled. Dietary N-glycans are carbohydrate structures conjugated to a wide variety of glycoproteins. These glycans have a remarkable structural similarity to HMOs and, when released, show a strong bifidogenic effect. This review discusses the biocatalytic potential of the endo-β-N-acetylglucosaminidase enzyme (EndoBI-1) from Bifidobacterium longum subspecies infantis (B. infantis), in releasing N-glycans inherently present in infant formula as means to increase the bifidogenicity of infant formula. Finally, the potential implications for protein deglycosylation with EndoBI-1 in the development of value added, next-generation formulas are discussed from a technical perspective.
Introduction
Human milk is the optimal source of infant nutrition. It provides all the energy, nutrients, and bioactive compounds required for the growth and development of the infant. Human milk feeding is associated with numerous benefits, including a reduced risk of gastrointestinal and respiratory infections and improved immune development (1). Given the known benefits of human milk, there is a great interest in improving infant formulas to resemble the compositional profile of human milk (2) and reduce the relative deficits associated with infant formula consumption. Thus, a better understanding of human milk components and their biological functions is paramount to the improvement of infant formulas (3, 4).
One of the most significant differences between human milk-fed and formula-fed infants is the composition of the gut microbiome (4–6). Breastfed infants have a less diverse yet more stable microbiome, and certain species of infant-adapted bifidobacteria can reach up to 90% of total fecal microbiome (7–9). On the other hand, the microbiome of the formula-fed infants is more variable (8, 10). To mitigate these differences between infant formula and human milk, most formulations add prebiotics such as galacto-oligosaccharides (GOS) and fructo-oligosaccharides (FOS) (11, 12) and/or probiotics. Probiotics added to formula are currently limited to Lactobacillus rhamnosus GG and Bifidobacterium lactis (13). Human milk contains complex carbohydrates known as human milk oligosaccharides (HMOs). HMOs are not digested in the small intestine and reach the colon intact where they are fermented by specialized species of bifidobacteria (14). However, most prebiotic compounds added to formula are not selective for the growth of bifidobacterial (15). Thus, the difference in oligosaccharide content in human milk and infant formula is likely to explain, at least in part, the compositional differences in the microbiome of formula-fed and human milk-fed infants.
Recently, synthetic HMOs such as 2′-fucosyllactose (2′FL) and lacto-N-neotetraose (LNnT) have been added to infant formula with the intent to increase the bifidogenic effect of infant formula (16–19). However, HMO fortification of infant formulas has remained low when compared to the global average concentration of HMOs in human milk. On the other hand, little attention has thus far been given to N-glycans, which are naturally found as glycoconjugates in both human and bovine milk proteins and bear striking structural and compositional similarity to HMOs. Owing to both their compositional and structural similarities to HMOs, N-glycans derived from milk glycoproteins have been shown to be selectively bifidogenic. In this review, we describe human milk as a complex biofluid. We then describe the types, compositions, and indications for most infant formulas available in the market. Finally, we propose the use of specialized enzymes known to be active in the gut microbiome of breastfed infants colonized with Bifidobacterium in order to improve the bioavailability of N-glycans in infant formula and we discuss potential applications for the design on next-generation infant formulas to improve the suitability of infant formulas for Bifidobacterium.
Macronutrients in Human Milk
The composition of human milk is dynamic, and it has evolved to provide optimal infant nutrition. Human milk contains macronutrients including proteins, lipids, carbohydrates, and micronutrients such as vitamins and minerals. It also contains non-nutritional bioactive components, growth factors, hormones, immunological factors, noncoding RNAs, and microorganisms (20). The macronutrient composition of human milk ranges from 9 to 12 g/L protein, 32 to 36 g/L lipids, 67 to 78 g/L lactose, and 5 to 15 g/L HMOs (3, 21, 22) (Table 1).
Proteins in human milk comprise two major classes, caseins, and whey (28). The main casein proteins are α-, β-, and κ-casein, and whey proteins are α-lactalbumin, lactoferrin, immunoglobulins (Igs), serum albumin, and lysozyme (29, 30). Non-protein nitrogen-containing compounds including urea, uric acid, creatine, creatinine, amino acids, and nucleotides represent ~25% of human milk nitrogen (31).
Fat is the largest source of energy in human milk, contributing to 40–55% of the total energy provided by human milk. Triacylglycerols contribute ~98% of human milk fat. More than 200 fatty acids are present in human milk with different concentrations (32). Palmitic and oleic acids are the most abundant fat types in human milk (33). The content of fatty acids, particularly the long-chain polyunsaturated fatty acids (LCPUFAs), is mostly affected by maternal diet.
Lactose is the main nutritional carbohydrate in human milk comprising 67–78 g/L and supplies approximately half of the energy obtained in by the infant. The other significant carbohydrate fractions of human milk are HMOs. However, contrary to that of lactose, the concentration of HMOs varies depending on the stage of lactation and maternal genetic factors, ranging from 5 to 15 g/L (34).
Human Milk Oligosaccharides (HMOs)
HMOs are non-nutritive, functional, and complex carbohydrates in human milk. The composition of HMOs in human milk is influenced by maternal genetic and lactation stage (35). Nearly 200 distinct oligosaccharides have been described to date (36). The basic core structure of HMOs includes disaccharide lactose at the reducing end, which is elongated with N-acetyllactosamine units, by the action of specific glycosyltransferases in the mammary gland. HMOs are composed of both neutral and anionic species with five monosaccharides as building blocks. These building blocks are D-glucose (Glc), D-galactose (Gal), N-acetylglucosamine (GlcNAc), L-fucose (Fuc), and N-acetylneuraminic (or sialic acid; NeuAc). The length of the HMO chains varies from three to fifteen carbohydrate units, and HMO structures can be either linear or branched forms (37, 38). There are three main HMO categories: neutral N-containing (non-fucosylated) (42–55%), neutral (fucosylated) (35–50%), and acidic (sialylated) (12–14%) (39).
2′-3-Fucosyllactose (FL) or 3′-6′-sialyllactose (SL) is formed when the lactose core is conjugated with Fuc or NeuAc. The lactose core is coupled to repeats of lacto-N-biose (Galβ1-3GlcNAc; LNB), and these chains are known as type 1 chains. The most abundant HMO is lacto-N-tetraose (LNT) as type 1 (40). When an N-acetyllactosamine unit (LacNAc; Galβ1-4GlcNAc) is conjugated to the lactose core, the type 2 chain is formed. Lacto-N-neotetraose (LNnT) is a type 2 chain in HMOs. Type 1 chains in HMOs are more abundant than those of type 2. Type 1 and 2 chain HMOs could be further elongated with fucosyl and sialyl residues in α-linkages to form hexoses, octaoses, and larger HMOs and together represent ~70% of all human milk oligosaccharides (34, 41) (Table 1). These alterations increase the number and complexity of HMO structures (38, 42).
Functions of HMOs
HMOs are hypothesized to have many important roles in infant innate defense, metabolic health, and neural development (43–45). Clinical and in vitro studies suggest that HMOs may block pathogen adhesion by serving soluble ligand analogs (43, 46, 47). As HMOs have structural features that mimic epithelial surface carbohydrates, they are thought to also serve as decoy receptors for pathogens (46, 48–50). HMOs are also thought to promote several intracellular processes like differentiation and apoptosis of intestinal epithelial cells (51). They can also have direct bactericidal or bacteriostatic effects. For instance, some HMOs can directly inhibit the in vitro growth of Streptococcus agalactiae, a known invasive bacterial pathogen in newborns (27, 52); other HMOs have been demonstrated to reduce pathogen adherence to colonic cells in vitro (53). Specific components present in HMOs (e.g., sialic acid) are also critical for the development of neurons and brain development, as well as neuronal transmission, cognitive ability and synaptogenesis (45, 54, 55).
One of the most well-characterized functions of HMOs is to serve as a prebiotic source and shape the microbial community of the infant gastrointestinal tract (56). HMOs reach the colon undigested where they are utilized by specialized gut microbes (57) that possess the necessary molecular machinery for transport and metabolization of these complex structures. Specific species of infant-adapted bifidobacteria [Bifidobacterium longum subsp. infantis (B. infantis), Bifidobacterium bifidum (B. bifidum), Bifidobacterium breve (B. breve), and Bifidobacterium longum subsp. longum (B. longum)] have the capability to degrade and utilize oligosaccharides and thus often become the most dominant species in the breastfed infant gut (58–61). Short-chain fatty acids (SCFA) (acetate, propionate, and butyrate) are produced as a result of fermentation of HMO in the colon. These molecules create an acidic environment (low pH) which favors the growth of strains of bifidobacteria while concomitantly creating an unfavorable environment for the growth of pH-sensitive pathogens (7, 41, 62).
Human Milk Glycoproteins and their Functions
Glycosylation is a diverse and common type of posttranslational modification that involves the attachment of a saccharide chain to a protein structure (63, 64). Approximately 70% of human milk proteins are found in glycosylated forms including lactoferrin, lysozyme, bile salt-stimulated lipase (BSSL), secretory IgA (SIgA), casein, and α-lactalbumin (65, 66). Several preclinical and clinical studies suggest that human milk glycoproteins have key roles in infant development. For instance, osteopontin is involved in regulating mineral deposition and osteoclasts activity in the bones (67); insulin-like growth factors participate in the processes related to the development of the intestinal mucosa (68); bile salt-stimulated lipase aids milk fat digestion (69); lactoferrin facilitates iron uptake in the small intestine (70); and β-casein-based phosphopeptides facilitate calcium absorption (71, 72).
Human milk glycoproteins may also have roles in protecting infants against pathogen infection (73–75). Lactoferrin has been reported to have bacteriostatic and bactericidal effects (76, 77). Lysozyme cleaves glycosidic linkage in the peptidoglycan structure of bacterial cell walls, providing innate protection against microbial infections (78). Interestingly, the level of lysozyme susceptibility varies between different bifidobacteria strains (79, 80). Some bifidobacteria strains of human infant origin are more resistant to lysozyme relative to animal and dairy-derived strains (81). This may suggest that lysozyme in human milk acts as a selection factor for coevolved bifidobacteria in the infant gut, such as B. infantis (80, 82, 83). Another predominant human milk protein is SIgA. SIgA acts as a protective defense against pathogens in the infant gut (74, 84). Other human milk glycoproteins, including BSSL and lactadherin, also have protective effects on the infant's health (74). Notably, BSSL has been associated with inhibition of Norwalk virus, a common cause of gastroenteritis, in vitro (85).
The glycan structures found on these glycoproteins are strikingly similar to HMOs, in both their monosaccharide composition and linkage types (86) (Figure 1). N-glycans also form complex structures which increase their specificity. This may explain why N-glycans isolated from human and bovine milk are bifidogenic (90), although not equally across bifidobacterial species (91). Specifically, N-glycans released from bovine milk glycoproteins selectively stimulates the growth of infant-adapted B. infantis whereas B. animalis, associated with an animal origin, is not capable of utilizing these structures (91). Further, a recent in vivo study showed that 19 unique N-glycan structures that are attached to lactoferrin and immunoglobulins stimulate the growth of B. infantis (92). Similar to HMOs, N-glycans are fermented into SCFAs, mainly lactate, acetate, and also butyrate and propionate (93). The colonic epithelium and microbial ecosystem can be affected from these end products by absorbing SCFAs and lowering the pH of the ecosystem (93). These metabolites primarily lactate and acetate lower the intestinal pH providing resistance to microbial colonization (7, 62, 94). Importantly, fermentation of N-glycans into acidic end-products, such as acetate and lactate, disfavors the growth of bacteria that degrade gastrointestinal mucin, and contributes to a considerable reduction in potentially pathogenic bacteria (7, 94–96). This is because most pathogenic bacteria preferentially grow near neutral pH (pH: 6.0–7.0) or grow under acidic conditions inefficiently (97). Therefore, the establishment of the gut microbiome by limiting pathogenic bacterial composition maximizes nutrition for other microbes and reduces inflammation, virulence factors, and antibiotic-resistant genomes (ARGs) in the gut environment. Thanks to the results of the fermentation and these metabolites, colonization of probiotic bacterial level in the gut microbiome, especially Bifidobacterium and genes conferring utilization of N-glycans, significantly increases. Thus, the development of the gut microbiome by providing colonization resistance to intestinal pathogens is critical for the development of the infant gut microbiome (94, 98).
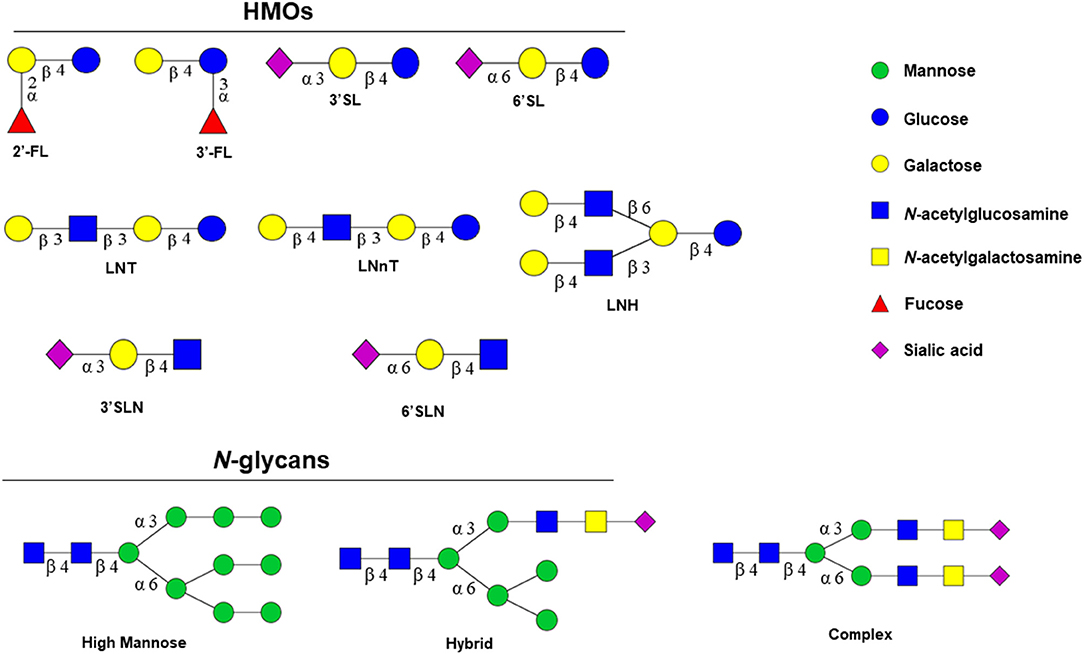
Figure 1. Structural similarities between HMOs and N-glycans. Structurally, N-glycans are bound to the amide group of asparagine (Asn) residue of the proteins via N-acetylglucosamine (HexNAc) in a specific amino acid sequence Asn-X-Ser/Thr or Asn-X-Cys (cysteine) (where X could be any amino acid except proline) (87, 88). N-glycans consist of a single core that has two N-acetylglucosamine (GlcNAc) followed by three mannoses. Further glycosylation determines the types of N-glycans that are classified into three main classes: high mannose (HM), hybrid (HY), and complex type (CT) based on composition (89); HM glycans typically contain unsubstituted terminal mannose sugars and 5–9 mannose residues attached to the chitobiose (GlcNAc2) core.
Infant Formula and N-Glycans
Infant formulas are intended as an effective breast milk substitute and are formulated to mimic nutritional composition, including macro- and micronutrients as well as bioactive components, of human milk (99). Most infant formulas are manufactured from bovine milk. The nutritional composition of all infant formulas must follow the global standards as recommended by the European Society for Pediatric Gastroenterology, Hepatology, and Nutrition's (ESPGHAN) international expert group that was commissioned by The Codex Alimentarius Commission in November 2004 (100, 101).
There are several types of infant formulas (102, 103). Some have specific clinical indications for use, including special formulas for preterm infants, protein hydrolysate or elemental formulas for infants that have cow's milk and soy protein allergies, or formulas for other specific nutritional requirements. Other types of formula include indications such as lactose-free formulas for lactose-intolerant infants, soy formulas for galactosemia, and sensitive formulas that contain partially hydrolyzed or reduced lactose content (Table 2).
The development of infant formulas has advanced significantly over the past 50 years. Nonetheless, an “ideal” microbiome where Bifidobacterium species predominate cannot yet be obtained with infant formula feeding. Previously, we reported that N-glycans, which are released from cow's milk proteins, have prebiotic activity supporting the growth of B. infantis (90, 91). Thus, releasing N-glycans from proteins being added to infant formulas may be an innovative and effective strategy to harness the activity of naturally active enzymes in the microbiome of breastfed infants to enhance the bifidogenicity of infant formulas.
Release of N-Glycans from Glycoproteins
N-glycans can be released by chemical and enzymatic methods (105). However, enzymatic release is considered a preferred method as it eliminates the possibility of chemical or residual contamination. Moreover, due to the highly specific nature of the enzymes, the enzymatic release of N-glycans represents a more targeted and efficient approach for releasing and increasing the bioavailability of these bifidogenic structures. There are two known enzymes that can release N-glycans: N-acetylglucosaminidases and endo-β-N-acetylglucosaminidases (ENGases).
ENGases belong to EC number 3.2.1.X which corresponds to the glycosylase-type hydrolyses cleaving O- and S-glycosyl compounds. ENGases are further classified according to their glycoside hydrolase (GH) family membership. These enzymes are classified into two groups, GH families 18 and 85, based on their amino acid sequence (106) within the Carbohydrate-Active enZymes (CAZy) Database (http://www.cazy.org) (107). Family GH18 is unusual in having glycoside hydrolases that are both catalytically active chitinases and ENGases and also subfamilies of non-hydrolytic proteins that function as carbohydrate-binding modules/ “lectins” or as xylanase inhibitors whereas family GH85 solely contains ENGases.
Although all of the ENGases carry out the same hydrolytic reaction, they have different tolerances as to the precise structure of the N-glycans that they can hydrolyze. The ENGases are all retaining glycosidases that hydrolyze substrates via a two-step mechanism involving general acid/base catalysis. The main difference between GH18 and GH85 ENGases is the active-site amino acids either being two carboxylic acid residues (Glu and Asp) or one carboxylic acid and one amino group (Glu and Asn), respectively. Regardless of whether the active site contains one or two carboxylic acids, the hydrolytic mechanism catalyzed by the ENGases involves neighboring group participation of the 2-acetamide of the second GlcNAc residue (108).
ENGase enzymes cleave N-N′-diacetyl chitobiose moieties found in the N-glycan core of high mannose (HM), complex (CT), and hybrid (HY) N-glycans (Figure 1) and the released N-glycans that stimulate the growth of B. infantis (109) (Figure 2). EndoBI-1 from B. infantis (ATCC 15697) is a product of the Blon_2468 gene. Other B. infantis strains known to produce EndoBI-1 are JCM 7007, JCM 7009, JCM 7011, JCM 11346, ATCC 15702, and ATCC 17930 (110). The enzyme is classified as a GH20 member in the National Center for Biotechnology Information Genetic Sequence Database (NCBI-GenBank: ACJ53522.1) and EMBL European Bioinformatics Institute (EBI) European Nucleotide Archive (ENA CP001095.1) (111) and as a GH18 member in The Universal Protein Resource Knowledgebase (UniProtKB: B7GPC7) (110). The other ENGase, EndoBI-2 from Bifidobacterium longum subsp. longum 157F (deposited as B. longum subsp. infantis 157F), is a product of the BLIF_1310 gene (112, 113). The enzyme is classified as a GH18 member in NCBI-GenBank (BAJ71450.1). To date, only EndoBI-1 has been shown to be active in the gut of healthy breastfed infants colonized by B. infantis EVC001 (92), but both are likely to be expressed in vivo. Interestingly, EndoBI-1 and EndoBI-2 have different distributions among strains of Bifidobacterium found in infants compared to adults, which may further suggest the importance of these enzymes in healthy gut microbiome formation in both adults and infants (114).
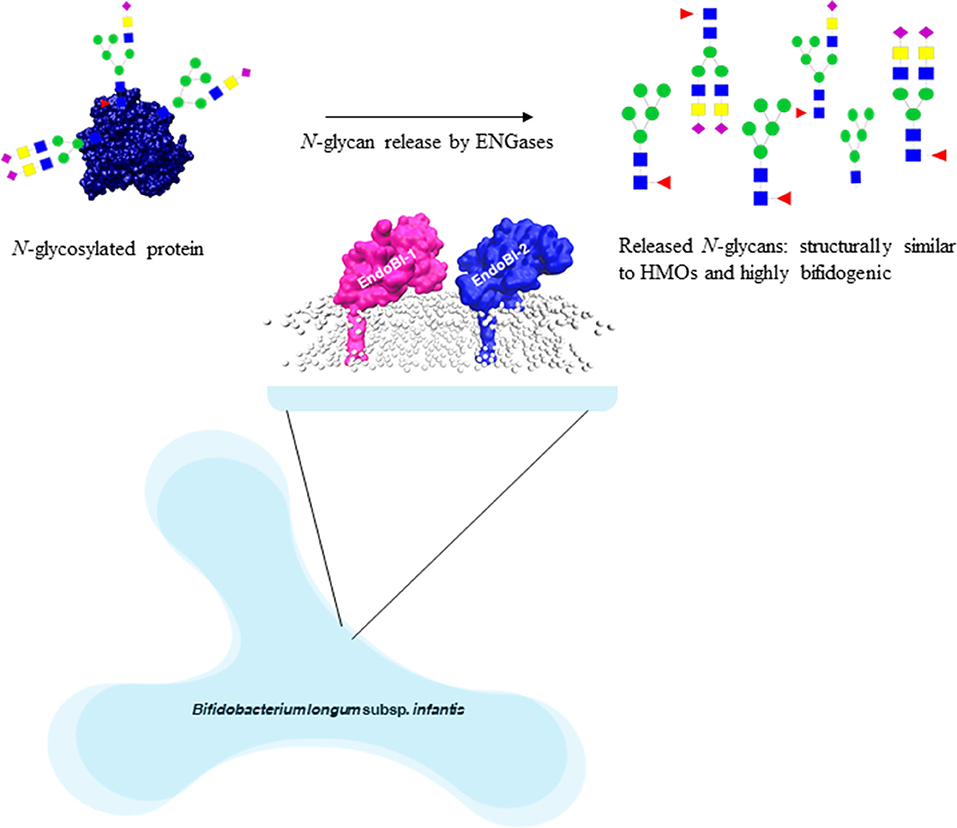
Figure 2. Formation of highly bifidogenic N-glycans by B. infantis ENGases from glycoproteins (91, 92).
EndoBI-1 and EndoBI-2 are unique among other ENGase members. EndoBI-1 and EndoBI-2 cleave N-glycans without perturbing the native glycan structure (115). The enzymes are considered fucose tolerant (110), meaning their activity is not affected by a fucosylated N-glycan core and therefore has a wider substrate specificity than similar enzymes (116). Both enzymes are active toward all major types of N-glycans found in glycosylated proteins (110). These unique enzymes are heat resistant, which enables broad applications even for industrial operations up to 95°C (110, 117), in contrast to the currently commercially available N-acetylglucosaminidases such as PNGase F of Flavobacterium meningosepticum which is heat labile (116). Further, both enzymes are considered safe for use in the food and pharmaceutical industries, especially when considering the sources of similar ENGase enzymes which are used by potential pathogens to evade the host immune system; such as Endo-COM from Cordyceps militaris (118), EndoS and EndoS2 from Streptococcus pyogenes (119, 120), EndoF3 from Elizabethkingia meningoseptica (121, 122), EndoH from Streptomyces plicatus (123, 124), EndoD from Streptococcus pneumoniae (3GDB.pdb), and EndoT from Hypocrea jecorina (125). Thus, making EndoBI-1 and EndoBI-2 the only two enzymes currently considered safe for food applications. Importantly, EndoBI-1 and EndoBI-2 could be easily cloned and/or mass produced with known microbiologic procedures and industrial techniques (110).
Challenges in the Study and Characterization of N-Glycans
One of the primary challenges facing the translation of technologies surrounding N-glycan release is the precise and accurate quantification and characterization of N-glycans. Structural analyses of oligosaccharides and glycoconjugates by high-throughput approaches are crucial for predicting their functions. A number of chromatographic techniques have been employed for the analysis of oligosaccharides (126). One of the most common is porous graphitized carbon chromatography–mass spectrometry (PGC-MS) (127). This method can distinguish the isomers of oligosaccharides and N- and O-glycans of glycoconjugates with different linkage positions. This ability of PGC-MS makes the method more powerful than previous techniques. To achieve the structural identifications of HMOs faster and with more precision, a library was recently presented for both native and sialylated oligosaccharides, including retention times, accurate masses, and tandem mass spectra of HMOs (38, 42). In addition, relative and absolute quantification of HMOs was performed using the PGC-MS approach (128). Thus, the alterations of HMO profiles could be monitored throughout certain periods such as lactation. For example, a specific method was recently demonstrated for the absolute quantification of neutral and acidic HMOs (129). PGC-MS can also be used for the characterization of glycoconjugates of human milk. In one study, N-glycans released from human milk whey glycoproteins were analyzed and compared with bovine milk N-glycans using the PGC-MS technique (130). On the other hand, a method including solid-phase permethylation step was presented for the analysis of HMOs and glycans derived from human and bovine milk whey glycoproteins by reverse-phase liquid chromatography mass spectrometry (RPLC-MS) (131). Matrix-assisted laser desorption/ionization time-of-flight mass spectrometry (MALDI-MS) has also been commonly used for the characterization of HMOs and glycoconjugates (132, 133). This approach makes the analysis very fast when compared with chromatographic and electrophoretic techniques. In this approach, typically neutral HMOs and N-/O-glycans can be quantified using MALDI-MS because of unstable sialic acid residues found in HMOs and glycoconjugates. However, sialic acids can be derivatized by certain methods to make them more stable during the MALDI-MS analysis (134).
Potential Application of ENGases in Next-Generation Formulas and Challenges
Although the composition of human milk is unparalleled in terms of suitability for infant nutrition, there are a number of logistical, practical, and medical reasons that necessitate the use for infant formulas with barriers to breastfeeding and racial inequities and/or socioeconomic barriers being prominent (135–138). The use of microbial enzymes is a staple of the industrial progress in the 21st century (139). The development of infant formula has not been the exception. For example, various next-generation infant formulas have been developed to manage cow milk protein allergy. Infant formulas with reduced allergenicity generally have partially or extensively hydrolyzed proteins, or amino acid-based formulations. Allergenicity is decreased by converting proteins to smaller peptides for modifying conformation or structure epitopes recognized by the immune system while maintaining caloric and protein and content, or by replacing intact proteins or peptides with amino acid formulations (140).
The process of producing partially or extensively hydrolyzed proteins involves complex proteolytic processing steps to reduce the size of bovine milk proteins. Protein glycosylation provides a stabilizing effect to proteins, making the native protein state more resistant to degradation (141, 142). Glycosylated proteins are more resistant to proteases compared to their aglycosylated (never glycosylated) or deglycosylated (enzymatically removed) counterparts (143–145). In fact, the rate of proteolysis and the amount of intact peptide (epitopes) available to reach up the intestinal tract are influenced by the presence of structural glycans (146). As bovine milk protein processing represents a major hurdle for the production of partially and extensively hydrolyzed proteins in infant formulas, the introduction of ENGases to this process has the potential to increase the efficiency and extent of protein hydrolysis required for infant formulas.
Deglycosylation may also have implication for bioactive proteins and the released N-glycans. For instance, lactoferrin, an important bioactive protein added to formula, is heavily glycosylated. Modifying glycosylation patterns is likely to change bioactive sites and catalytic activities (147). Further, the released N-glycans from glycoproteins can be recovered from protein production streams and used as an added source of highly specific prebiotics for the infant gut microbiome. These N-glycans are then converted into metabolites with energy value for the infant (e.g., acetate and lactate) when competent Bifidobacterium are present, as well as to enhance the colonization of specialized bifidobacteria, such as B. infantis, which provide essential ecosystem services to the infant gut (Figure 3) (94).
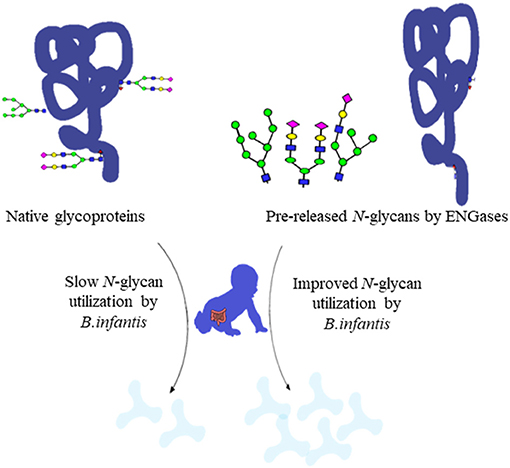
Figure 3. Utilization rate of native glycoproteins and pre-released glycans by ENGases (91).
The ENGase enzymes may be either used in the production step to release high N-glycan concentrations in the infant formulations or included as components of designed formulas to release N-glycans in situ in the gut. Theoretically both native ENGases produced by baby and infant safe organisms such as EndoBI enzymes of B. infantis and ENGases produced by recombinant organisms could be used for these applications. However, in practice regulations in most of the globe and especially in EU do not allow the use of GMO in baby and infant products.
Although baby food and infant formula prepared with ENGases produced by recombinant organisms used in the production step could be considered as products derived with GMOs, public and private standards for baby and infant products are too strict to use these products yet for both US and EU markets. Therefore, in the immediate future instead of ENGases produced by recombinant organisms, ENGases produced by baby and infant safe organisms such as EndoBI enzymes of B. infantis are more realistic. Although the regulators in the European Union can change the complete regulatory system from a process-based system to a strictly product-based system, such as in Canada in the future, these changes are unlikely to affect baby and infant products.
Conclusion
The use of ENGase enzymes in the production of infant formula has great potential to increase the nutritional values of formula by releasing additional carbohydrates as sources of energy and substrates from N-glycans, a so far underexploited and underappreciated source. Due to their structural similarity to the HMOs, the release of N-glycans is likely to be a more successful approach to increase the potential for infant formula to promote colonization of the infant gut by infant-adapted Bifidobacterium, leveraging ingredients already present in these formulations and a growing understanding of the microbial enzymes active in the infant gut ecosystem. Finally, deglycosylation of proteins also has the potential to create value-added formulations as well as to have implications on a manufacturing scale.
Author Contributions
SK organized the general content of the paper. HD was responsible for general editing and organizing the authors as well as the contribution for two sections. MK, AS, and HK contributed one section of the paper. AA and SF were responsible for one section of the paper. All authors contributed to the article and approved the submitted version.
Conflict of Interest
The authors declare that the research was conducted in the absence of any commercial or financial relationships that could be construed as a potential conflict of interest.
Acknowledgments
The authors thank RMD for critical reading of the manuscript and feedback. SK and SF have received funding from Evolve Biosystems, Inc., a company focused on the infant gut microbiome. SK has received funding from Uluova Süt Ticaret A.Ş (Uluova Milk Trading Co.), a company focused on the production of colostrum and lactoferrin.
References
1. Oftedal OT. The evolution of milk secretion and its ancient origins. Animal. (2012) 6:355–68. doi: 10.1017/S1751731111001935
2. Abayomi J. Infant formula - evaluating the safety of new ingredients. J Hum Nutr Diet. (2005) 18:226. doi: 10.1111/j.1365-277X.2005.00613.x
3. Ballard O, Morrow AL. Human milk composition. Nutrients and bioactive factors. Pediatr Clin North Am. (2013) 60:49–74. doi: 10.1016/j.pcl.2012.10.002
4. Martin C, Ling P-R, Blackburn G. Review of infant feeding: key features of breast milk and infant formula. Nutrients. (2016) 8:279. doi: 10.3390/nu8050279
5. Hascoët J-M, Hubert C, Rochat F, Legagneur H, Gaga S, Emady-Azar S, et al. Effect of formula composition on the development of infant gut microbiota. J Pediatr Gastroenterol Nutr. (2011) 52:756–62. doi: 10.1097/MPG.0b013e3182105850
6. Heird WC. Infant nutrition. In: Erdman JW, Macdonald IA, Zeisel SH, editors. Present Knowledge in Nutrition. New York, NY: John Wiley & Sons, Inc. (2012). p. 624–36.
7. Frese SA, Hutton AA, Contreras LN, Shaw CA, Palumbo MC, Casaburi G, et al. Persistence of supplemented Bifidobacterium longum subsp. infantis EVC001 in breastfed infants. mSphere. (2017) 2:e00501–17 doi: 10.1128/mSphere.00501-17
8. Ma J, Li Z, Zhang W, Zhang C, Zhang Y, Mei H, et al. Comparison of gut microbiota in exclusively breast-fed and formula-fed babies: a study of 91 term infants. Sci Rep. (2020) 10:15792. doi: 10.1038/s41598-020-72635-x
9. Wang M, Li M, Wu S, Lebrilla CB, Chapkin RS, Ivanov I, et al. Fecal microbiota composition of breast-fed infants is correlated with human milk oligosaccharides consumed. J Pediatr Gastroenterol Nutr. (2015) 60:825–33. doi: 10.1097/MPG.0000000000000752
10. Fan W, Huo G, Li X, Yang L, Duan C, Wang T, et al. Diversity of the intestinal microbiota in different patterns of feeding infants by Illumina high-throughput sequencing. World J Microbiol Biotechnol. (2013) 29:2365–72. doi: 10.1007/s11274-013-1404-3
11. Boehm G, Lidestri M, Casetta P, Jelinek J, Negretti F, Stahl B, et al. Supplementation of a bovine milk formula with an oligosaccharide mixture increases counts of faecal bifidobacteria in preterm infants. Arch Dis Child Fetal Neonatal Ed. (2002) 86:178F−81. doi: 10.1136/fn.86.3.F178
12. Vandenplas Y, Analitis A, Tziouvara C, Kountzoglou A, Drakou A, Tsouvalas M, et al. Safety of a new synbiotic starter formula. Pediatr Gastroenterol Hepatol Nutr. (2017) 20:167. doi: 10.5223/pghn.2017.20.3.167
13. Alles MS, Scholtens PAMJ, Bindels JG. Current trends in the composition of infant milk formulas. Curr Paediatr. (2004) 14:51–63. doi: 10.1016/j.cupe.2003.09.007
14. Walsh C, Lane JA, van Sinderen D, Hickey RM. Human milk oligosaccharides: Shaping the infant gut microbiota and supporting health. J Funct Foods. (2020) 72:104074. doi: 10.1016/j.jff.2020.104074
15. Vandenplas Y, Zakharova I, Dmitrieva Y. Oligosaccharides in infant formula: more evidence to validate the role of prebiotics. Br J Nutr. (2015) 113:1339–344. doi: 10.1017/S0007114515000823
16. Reverri EJ, Devitt AA, Kajzer JA, Baggs GE, Borschel MW. Review of the clinical experiences of feeding infants formula containing the human milk oligosaccharide 2′-fucosyllactose. Nutrients. (2018) 10:1346. doi: 10.3390/nu10101346
17. Marriage BJ, Buck RH, Goehring KC, Oliver JS, Williams JA. Infants fed a lower calorie formula with 2′ FL show growth and 2′ FL uptake like breast-fed infants. J Pediatr Gastroenterol Nutr. (2015) 61:649–58. doi: 10.1097/MPG.0000000000000889
18. Puccio G, Alliet P, Cajozzo C, Janssens E, Corsello G, Sprenger N, et al. Effects of infant formula with human milk oligosaccharides on growth and morbidity: a randomized multicenter trial. J Pediatr Gastroenterol Nutr. (2017) 64:624–31. doi: 10.1097/MPG.0000000000001520
19. Rodriguez-Herrera A, Mulder K, Bouritius H, Rubio R, Muñoz A, Agosti M, et al. Gastrointestinal tolerance, growth and safety of a partly fermented formula with specific prebiotics in healthy infants: a double-blind, randomized, controlled trial. Nutrients. (2019) 11:1530. doi: 10.3390/nu11071530
20. Hennet T, Borsig L. Breastfed at Tiffany's. Trends Biochem Sci. (2016) 41:508–18. doi: 10.1016/j.tibs.2016.02.008
21. Thomson P, Medina DA, Garrido D. Human milk oligosaccharides and infant gut bifidobacteria: molecular strategies for their utilization. Food Microbiol. (2018) 75:37–46. doi: 10.1016/j.fm.2017.09.001
22. Bode L. The functional biology of human milk oligosaccharides. Early Hum Dev. (2015) 91:619–22. doi: 10.1016/j.earlhumdev.2015.09.001
23. Holsinger VH. Handbook of Milk Composition. In: Robert G. Jensen, editor. Journal of Food Composition and Analysis. San Diego, CA: Academic Press (1996). 284 p.
24. Ahmed L, Islam S, Khan N, Nahid S. Vitamin C content in human milk (colostrum, transitional and mature) and serum of a sample of bangladeshi mothers. Malays J Nutr. (2004) 10:1–4.
25. Dorea JG. Calcium and phosphorus in human milk. Nutr Res. (1999) 19:709–39. doi: 10.1016/S0271-5317(99)00035-4
26. Dórea JG. Magnesium in human milk. J Am Coll Nutr. (2000) 19:210–9. doi: 10.1080/07315724.2000.10718919
27. Lin AE, Autran CA, Szyszka A, Escajadillo T, Huang M, Godula K, et al. Human milk oligosaccharides inhibit growth of group B Streptococcus. J Biol Chem. (2017) 292:11243–9. doi: 10.1074/jbc.M117.789974
28. Mosca F, Giannì ML. Human milk: composition and health benefits. La Pediatr Med Chir. (2017) 39:155. doi: 10.4081/pmc.2017.155
29. Lönnerdal B. Human milk proteins: key components for the biological activity of human milk. Adv Exp Med Biol. (2004) 554:11–25. doi: 10.1007/978-1-4757-4242-8_4
30. Andreas NJ, Kampmann B, Mehring Le-Doare K. Human breast milk: a review on its composition and bioactivity. Early Hum Dev. (2015) 91:629–35. doi: 10.1016/j.earlhumdev.2015.08.013
31. Bauer J, Gerss J. Longitudinal analysis of macronutrients and minerals in human milk produced by mothers of preterm infants. Clin Nutr. (2011) 30:215–20. doi: 10.1016/j.clnu.2010.08.003
32. Grote V, Verduci E, Scaglioni S, Vecchi F, Contarini G, Giovannini M, et al. Breast milk composition and infant nutrient intakes during the first 12 months of life. Eur J Clin Nutr. (2016) 70:250–6. doi: 10.1038/ejcn.2015.162
33. Saarela T, Kokkonen J, Koivisto M. Macronutrient and energy contents of human milk fractions during the first six months of lactation. Acta Paediatr Int J Paediatr. (2005) 94:1176–81. doi: 10.1080/08035250510036499
34. McGuire MK, Meehan CL, McGuire MA, Williams JE, Foster J, Sellen DW, et al. What's normal? Oligosaccharide concentrations and profiles in milk produced by healthy women vary geographically. Am J Clin Nutr. (2017) 105:1086–100. doi: 10.3945/ajcn.116.139980
35. Ninonuevo MR, Park Y, Yin H, Zhang J, Ward RE, Clowers BH, et al. A strategy for annotating the human milk glycome. J Agric Food Chem. (2006) 54:7471–80. doi: 10.1021/jf0615810
36. Triantis V, Bode L, van Neerven RJJ. Immunological effects of human milk oligosaccharides. Front Pediatr. (2018) 6:190. doi: 10.3389/fped.2018.00190
37. Kunz C, Rudloff S, Baier W, Klein N, Strobel S. Oligosaccharides in human milk: structural, functional, and metabolic aspects. Annu Rev Nutr. (2000) 20:699–722. doi: 10.1146/annurev.nutr.20.1.699
38. Wu S, Tao N, German JB, Grimm R, Lebrilla CB. Development of an annotated library of neutral human milk oligosaccharides. J Proteome Res. (2010) 9:4138–51. doi: 10.1021/pr100362f
39. Smilowitz JT, Lebrilla CB, Mills DA, German JB, Freeman SL. Breast milk oligosaccharides: structure-function relationships in the neonate. Annu Rev Nutr. (2014) 34:143–69. doi: 10.1146/annurev-nutr-071813-105721
40. Urashima T, Asakuma S, Leo F, Fukuda K, Messer M, Oftedal OT. The predominance of type i oligosaccharides is a feature specific to human breast milk. Adv Nutr. (2012) 3:473S−82S. doi: 10.3945/an.111.001412
41. Duar RM, Casaburi G, Mitchell RD, Scofield LNC, Ortega Ramirez CA, Barile D, et al. Comparative genome analysis of Bifidobacterium longum subsp. infantis strains reveals variation in human milk oligosaccharide utilization genes among commercial probiotics. Nutrients. (2020) 12:3247. doi: 10.3390/nu12113247
42. Wu S, Grimm R, German JB, Lebrilla CB. Annotation and structural analysis of sialylated human milk oligosaccharides. J Proteome Res. (2011) 10:856–68. doi: 10.1021/pr101006u
43. Wiciński M, Sawicka E, Gebalski J, Kubiak K, Malinowski B. Human milk oligosaccharides: health benefits, potential applications in infant formulas, and pharmacology. Nutrients. (2020) 12:266. doi: 10.3390/nu12010266
44. Bode L. Human milk oligosaccharides: every baby needs a sugar mama. Glycobiology. (2012) 22:1147–62. doi: 10.1093/glycob/cws074
45. Bienenstock J, Buck RH, Linke H, Forsythe P, Stanisz AM, Kunze WA. Fucosylated but not sialylated milk oligosaccharides diminish colon motor contractions. PLoS One. (2013) 8:e76236. doi: 10.1371/journal.pone.0076236
46. Morrow AL, Ruiz-Palacios GM, Altaye M, Jiang X, Lourdes Guerrero M, Meinzen-Derr JK, et al. Human milk oligosaccharides are associated with protection against diarrhea in breast-fed infants. J Pediatr. (2004) 145:297–303. doi: 10.1016/j.jpeds.2004.04.054
47. Newburg DS, Ruiz-Palacios GM, Morrow AL. Human milk glycans protect infants against enteric pathogens. Annu Rev Nutr. (2005) 23:37–58. doi: 10.1146/annurev.nutr.25.050304.092553
48. Ruiz-Palacios GM, Cervantes LE, Ramos P, Chavez-Munguia B, Newburg DS. Campylobacter jejuni binds intestinal H(O) antigen (Fucα1, 2Galβ1, 4GlcNAc), and fucosyloligosaccharides of human milk inhibit its binding and infection. J Biol Chem. (2003) 278:14112–20. doi: 10.1074/jbc.M207744200
49. Bode L. Human milk oligosaccharides: prebiotics and beyond. Nutr Rev. (2009) 67:S183–S91. doi: 10.1111/j.1753-4887.2009.00239.x
50. Leach JL, Garber SA, Marcon AA, Prieto PA. In vitro and in vivo effects of soluble, monovalent globotriose on bacterial attachment and colonization. Antimicrob Agents Chemother. (2005) 49:3842–6. doi: 10.1128/AAC.49.9.3842-3846.2005
51. Kuntz S, Rudloff S, Kunz C. Oligosaccharides from human milk influence growth-related characteristics of intestinally transformed and non-transformed intestinal cells. Br J Nutr. (2008) 99:462–71. doi: 10.1017/S0007114507824068
52. Ackerman DL, Doster RS, Weitkamp JH, Aronoff DM, Gaddy JA, Townsend SD. Human milk oligosaccharides exhibit antimicrobial and antibiofilm properties against group b Streptococcus. ACS Infect Dis. (2017) 3:595–605. doi: 10.1021/acsinfecdis.7b00064
53. Coppa GV, Zampini L, Galeazzi T, Facinelli B, Ferrante L, Capretti R, et al. Human milk oligosaccharides inhibit the adhesion to Caco-2 cells of diarrheal pathogens: Escherichia coli, Vibrio cholerae, and Salmonella fyris. Pediatr Res. (2006) 59:377–82. doi: 10.1203/01.pdr.0000200805.45593.17
54. Jacobi SK, Yatsunenko T, Li D, Dasgupta S, Yu RK, Berg BM, et al. Dietary isomers of sialyllactose increase ganglioside sialic acid concentrations in the corpus callosum and cerebellum and modulate the colonic microbiota of formula-fed piglets. J Nutr. (2016) 146:200–8. doi: 10.3945/jn.115.220152
55. Vázquez E, Barranco A, Ramírez M, Gruart A, Delgado-García JM, Martínez-Lara E, et al. Effects of a human milk oligosaccharide, 2′-fucosyllactose, on hippocampal long-term potentiation and learning capabilities in rodents. J Nutr Biochem. (2015) 26:455–65. doi: 10.1016/j.jnutbio.2014.11.016
56. Zivkovic AM, German JB, Lebrilla CB, Mills DA. Human milk glycobiome and its impact on the infant gastrointestinal microbiota. Proc Natl Acad Sci USA. (2011) 108:4653–8. doi: 10.1073/pnas.1000083107
57. Thongaram T, Hoeflinger JL, Chow J, Miller MJ. Human milk oligosaccharide consumption by probiotic and human-associated bifidobacteria and lactobacilli. J Dairy Sci. (2017) 100:7825–33. doi: 10.3168/jds.2017-12753
58. Marcobal A, Barboza M, Froehlich JW, Block DE, German JB, Lebrilla CB, et al. Consumption of human milk oligosaccharides by gut-related microbes. J Agric Food Chem. (2010) 58:5334–40. doi: 10.1021/jf9044205
59. Gotoh A, Katoh T, Sakanaka M, Ling Y, Yamada C, Asakuma S, et al. Sharing of human milk oligosaccharides degradants within bifidobacterial communities in faecal cultures supplemented with Bifidobacterium bifidum. Sci Rep. (2018) 8:13958. doi: 10.1038/s41598-018-32080-3
60. Asakuma S, Hatakeyama E, Urashima T, Yoshida E, Katayama T, Yamamoto K, et al. Physiology of consumption of human milk oligosaccharides by infant gut-associated bifidobacteria. J Biol Chem. (2011) 286:34583–92. doi: 10.1074/jbc.M111.248138
61. LoCascio RG, Niñonuevo MR, Kronewitter SR, Freeman SL, German JB, Lebrilla CB, et al. A versatile and scalable strategy for glycoprofiling bifidobacterial consumption of human milk oligosaccharides. Microb Biotechnol. (2009) 2:333–42. doi: 10.1111/j.1751-7915.2008.00072.x
62. Henrick BM, Hutton AA, Palumbo MC, Casaburi G, Mitchell RD, Underwood MA, et al. Elevated fecal pH indicates a profound change in the breastfed infant gut microbiome due to reduction of bifidobacterium over the past century. mSphere. (2018) 3:e00041-18 doi: 10.1128/mSphere.00041-18
63. Dallas DC, Martin WF, Strum JS, Zivkovic AM, Smilowitz JT, Underwood MA, et al. N-linked glycan profiling of mature human milk by high-performance microfluidic chip liquid chromatography time-of-flight tandem mass spectrometry. J Agric Food Chem. (2011) 59:4255–63. doi: 10.1021/jf104681p
64. Helenius A. Intracellular functions of N-linked glycans. Science. (2001) 291:2364–9. doi: 10.1126/science.291.5512.2364
65. Kirmiz N, Robinson RC, Shah IM, Barile D, Mills DA. Milk glycans and their interaction with the infant-gut microbiota. Annu Rev Food Sci Technol. (2018) 9:429–50. doi: 10.1146/annurev-food-030216-030207
66. Newburg DS. Glycobiology of human milk. Biochem. (2013) 78:771–85. doi: 10.1134/S0006297913070092
67. Foster BL, Ao M, Salmon CR, Chavez MB, Kolli TN, Tran AB, et al. Osteopontin regulates dentin and alveolar bone development and mineralization. Bone. (2018) 107:196–207. doi: 10.1016/j.bone.2017.12.004
68. Hoeflich A, Meyer Z. Functional analysis of the IGF-system in milk. Best Pract Res Clin Endocrinol Metab. (2017) 31:409–18. doi: 10.1016/j.beem.2017.10.002
69. Lindquist S, Hernell O. Lipid digestion and absorption in early life: an update. Curr Opin Clin Nutr Metab Care. (2010) 13:314–20. doi: 10.1097/MCO.0b013e328337bbf0
70. Davidson LA, Litov RE, Lönnerdal BO. Iron retention from lactoferrin-supplemented formulas in infant rhesus monkeys. Pediatr Res. (1990) 27:176–80. doi: 10.1203/00006450-199002000-00018
71. Hansen C, Werner E, Erbes HJ, Larrat V, Kaltwasser JP. Intestinal calcium absorption from different calcium preparations: influence of anion and solubility. Osteoporos Int. (1996) 6:386–93. doi: 10.1007/BF01623012
72. Hicks PD, Hawthorne KM, Berseth CL, Marunycz JD, Heubi JE, Abrams SA. Total calcium absorption is similar from infant formulas with and without prebiotics and exceeds that in human milk-fed infants. BMC Pediatr. (2012) 12:118. doi: 10.1186/1471-2431-12-118
73. Sherman MP, Bennett SH, Hwang FFY, Yu C. Neonatal small bowel epithelia: Enhancing anti-bacterial defense with lactoferrin and Lactobacillus GG. BioMetals. (2004) 17:285–9. doi: 10.1023/B:BIOM.0000027706.51112.62
74. Liu B, Newburg DS. Human Milk Glycoproteins Protect infants against human pathogens. Breastfeed Med. (2013) 8:354–62. doi: 10.1089/bfm.2013.0016
75. Gomez HF, Ochoa TJ, Carlin LG, Cleary TG. Human lactoferrin impairs virulence of Shigella flexneri. J Infect Dis. (2003) 187:87–95. doi: 10.1086/345875
76. Lönnerdal B. Bioactive proteins in breast milk. J Paediatr Child Health. (2013) 49:1–7. doi: 10.1111/jpc.12104
77. Karav S, German J, Rouquié C, Le Parc A, Barile D. Studying lactoferrin N-glycosylation. Int J Mol Sci. (2017) 18:870. doi: 10.3390/ijms18040870
78. Lönnerdal B. Nutritional and physiologic significance of human milk proteins. Am J Clin Nutr. (2003) 77:1537S−43S. doi: 10.1093/ajcn/77.6.1537S
79. Gagnon M. In vitro inhibition of Escherichia coli O157:H7 by bifidobacterial strains of human origin. Int J Food Microbiol. (2004) 92:69–78. doi: 10.1016/j.ijfoodmicro.2003.07.010
80. Rada V, Splichal I, Rockova S, Grmanova M, Vlkova E. Susceptibility of bifidobacteria to lysozyme as a possible selection criterion for probiotic bifidobacterial strains. Biotechnol Lett. (2010) 32:451–5. doi: 10.1007/s10529-009-0170-7
81. Minami J, Odamaki T, Hashikura N, Abe F, Xiao JZ. Lysozyme in breast milk is a selection factor for bifidobacterial colonisation in the infant intestine. Benef Microbes. (2016) 7:53–60. doi: 10.3920/BM2015.0041
82. Rockova S, Nevoral J, Rada V, Marsik P, Sklenar J, Hinkova A, et al. Factors affecting the growth of bifidobacteria in human milk. Int Dairy J. (2011) 21:504–8. doi: 10.1016/j.idairyj.2011.02.005
83. Ročková Š, Rada V, Havlík J, Švejstil R, Vlková E, Bunešová V, Janda K, et al. Growth of bifidobacteria in mammalian milk. Czech J Anim Sci. (2013) 58:99–105. doi: 10.17221/6666-CJAS
84. Arnold JN, Wormald MR, Sim RB, Rudd PM, Dwek RA. The impact of glycosylation on the biological function and structure of human immunoglobulins. Annu Rev Immunol. (2007) 25:21–50. doi: 10.1146/annurev.immunol.25.022106.141702
85. Ruvoën-Clouet N, Mas E, Marionneau S, Guillon P, Lombardo D, Le Pendu J. Bile-salt-stimulated lipase and mucins from milk of 'secretor' mothers inhibit the binding of Norwalk virus capsids to their carbohydrate ligands. Biochem J. (2006) 393:627–34. doi: 10.1042/BJ20050898
86. Mussatto SI, Mancilha IM. Non-digestible oligosaccharides: a review. Carbohydr Polym. (2007) 68:587–97. doi: 10.1016/j.carbpol.2006.12.011
87. Chu CS, Niñonuevo MR, Clowers BH, Perkins PD, An HJ, Yin H, et al. Profile of native N-linked glycan structures from human serum using high performance liquid chromatography on a microfluidic chip and time-of-flight mass spectrometry. Proteomics. (2009) 9:1939–51. doi: 10.1002/pmic.200800249
88. Lafite P, Daniellou R. Rare and unusual glycosylation of peptides and proteins. Nat Prod Rep. (2012) 29:729. doi: 10.1039/c2np20030a
89. Varki A, Lowe JB. Biological roles of glycans. In Varki A, Cummings RD, Esko JD, Freeze HH, Hart GW, Etzler ME, et al., editors. Essential of Glycobiology. Cold Spring Harbor, NY: Cold Spring Harbor Laboratory Press (2009).
90. Wang WL, Wang W, Du YM, Wu H, Yu XB, Ye KP, et al. Comparison of anti-pathogenic activities of the human and bovine milk N-glycome: fucosylation is a key factor. Food Chem. (2017) 235:167–74. doi: 10.1016/j.foodchem.2017.05.026
91. Karav S, Le Parc A, Leite Nobrega de Moura Bell JM, Frese SA, Kirmiz N, Block DE, et al. Oligosaccharides released from milk glycoproteins are selective growth substrates for infant-associated bifidobacteria. Appl Environ Microbiol. (2016) 82:3622–30. doi: 10.1128/AEM.00547-16
92. Karav S, Casaburi G, Arslan A, Kaplan M, Sucu B, Frese S. N-glycans from human milk glycoproteins are selectively released by an infant gut symbiont in vivo. J Funct Foods. (2019) 61:103485. doi: 10.1016/j.jff.2019.103485
93. Koropatkin NM, Cameron EA, Martens EC. How glycan metabolism shapes the human gut microbiota. Nat Rev Microbiol. (2012) 10:323–35. doi: 10.1038/nrmicro2746
94. Duar RM, Henrick BM, Casaburi G, Frese SA. Integrating the ecosystem services framework to define dysbiosis of the breastfed infant gut: the role of B. infantis and Human Milk Oligosaccharides. Front Nutr. (2020) 7:33 doi: 10.3389/fnut.2020.00033
95. Casaburi G, Frese SA. Colonization of breastfed infants by Bifidobacterium longum subsp. infantis EVC001 reduces virulence gene abundance. Hum Microbiome J. (2018) 9:7. doi: 10.1016/j.humic.2018.05.001
96. Karav S, Casaburi G, Frese SA. Reduced colonic mucin degradation in breastfed infants colonized by Bifidobacterium longum subsp. infantis EVC001. FEBS Open Biol. (2018) 8:1649–57. doi: 10.1002/2211-5463.12516
97. Stiles ME. Biopreservation by lactic acid bacteria. Antonie Van Leeuwenhoek. (1996) 70:331–45. doi: 10.1007/BF00395940
98. Olin A, Henckel E, Chen Y, Lakshmikanth T, Pou C, Mikes J, et al. Stereotypic immune system development in newborn children. Cell. (2018) 174:1277–92.e14. doi: 10.1016/j.cell.2018.06.045
99. Lönnerdal B. Preclinical assessment of infant formula. Ann Nutr Metab. (2012) 60:196–9. doi: 10.1159/000338209
100. Koletzko B, Baker S, Cleghorn G, Neto UF, Gopalan S, Hernell O, et al. Global standard for the composition of infant formula: Recommendations of an ESPGHAN coordinated international expert group. J Pediatr Gastroenterol Nutr. (2005) 41:584–99. doi: 10.1097/01.mpg.0000187817.38836.42
101. Koletzko B, Shamir R. Infant formula. Curr Opin Clin Nutr Metab Care. (2016) 19:205–7. doi: 10.1097/MCO.0000000000000277
102. Joeckel RJ, Phillips SK. Overview of infant and pediatric formulas. Nutr Clin Pract. (2009) 24:356–62. doi: 10.1177/0884533609335309
103. Teitelbaum JE, Lagmay JP. Familiarity of pediatricians with different commercially available neonatal and infant formulas. Clin Pediatr (Phila). (2007) 46:418–23. doi: 10.1177/0009922806298702
104. Newberry RE. The Infant Formula Act of 1980. J Assoc Off Anal Chem. (1982) 65:1472–3. doi: 10.1093/jaoac/65.6.1472
105. Stanley P, Taniguchi N, Aebi M. N-glycans. In: Varki A, Cummings RD, Esko JD, Stanley P, Hart GW, Aebi M, et al., editors. Essentials of Glycobiology. Cold Spring Harbor, NY: Cold Spring Harbor Press (2015–2017).
106. Murakami S, Takaoka Y, Ashida H, Yamamoto K, Narimatsu H, Chiba Y. Identification and characterization of endo-β-N-acetylglucosaminidase from methylotrophic yeast Ogataea minuta. Glycobiology. (2013) 23:736–44. doi: 10.1093/glycob/cwt012
107. Lombard V, Golaconda Ramulu H, Drula E, Coutinho PM, Henrissat B. The carbohydrate-active enzymes database (CAZy) in 2013. Nucleic Acids Res. (2014) 42:D490–D5. doi: 10.1093/nar/gkt1178
108. Fairbanks AJ. The ENGases: Versatile biocatalysts for the production of homogeneous: N-linked glycopeptides and glycoproteins. Chem Soc Rev. (2017) 46:5128–46. doi: 10.1039/C6CS00897F
109. Karav S, Bell JMLNDM, Parc A Le, Liu Y, Mills DA, Block DE, et al. Characterizing the release of bioactive N-glycans from dairy products by a novel endo-β-N-acetylglucosaminidase. Biotechnol Prog. (2015) 31:1331–9. doi: 10.1002/btpr.2135
110. Garrido D, Nwosu C, Ruiz-Moyano S, Aldredge D, German JB, Lebrilla CB, et al. Endo-β-N-acetylglucosaminidases from infant gut-associated bifidobacteria release complex N-glycans from human milk glycoproteins. Mol Cell Proteomics. (2012) 11:775–85. doi: 10.1074/mcp.M112.018119
111. Sela DA, Chapman J, Adeuya A, Kim JH, Chen F, Whitehead TR, et al. The genome sequence of Bifidobacterium longum subsp. infantis reveals adaptations for milk utilization within the infant microbiome. Proc Natl Acad Sci USA. (2008) 105:18964–9. doi: 10.1073/pnas.0809584105
112. Fukuda S, Toh H, Hase K, Oshima K, Nakanishi Y, Yoshimura K, et al. Bifidobacteria can protect from enteropathogenic infection through production of acetate. Nature. (2011) 469:543–9. doi: 10.1038/nature09646
113. Blanco G, Ruiz L, Tamés H, Ruas-Madiedo P, Fdez-Riverola F, Sánchez B, et al. Revisiting the metabolic capabilities of bifidobacterium longum susbp. longum and Bifidobacterium longum subsp. infantis from a glycoside hydrolase perspective. Microorganisms. (2020) 8:723. doi: 10.3390/microorganisms8050723
114. Sahutoglu AS, Duman H, Frese SA, Karav S. Structural insights of two novel N-acetyl-glucosaminidase enzymes through in silico methods. Turkish J Chem. (2020) 44:1703–12. doi: 10.3906/kim-2006-19
115. Karav S, Parc A Le, Moura Bell JMLN de, Rouquié C, Mills DA, Barile D, et al. Kinetic characterization of a novel endo-β-N-acetylglucosaminidase on concentrated bovine colostrum whey to release bioactive glycans. Enzyme Microb Technol. (2015) 77:46–53. doi: 10.1016/j.enzmictec.2015.05.007
116. Parc A Le, Karav S, Bell JMLNDM, Frese SA, Liu Y, Mills DA, et al. A novel endo-β-N-acetylglucosaminidase releases specific N-glycans depending on different reaction conditions. Biotechnol Prog. (2015) 31:1323–30. doi: 10.1002/btpr.2133
117. Sjögren J, Collin M. Bacterial glycosidases in pathogenesis and glycoengineering. Future Microbiol. (2014) 9:1039–51. doi: 10.2217/fmb.14.71
118. Seki H, Huang Y, Arakawa T, Yamada C, Kinoshita T, Iwamoto S, et al. Structural basis for the specific cleavage of core-fucosylated N-glycans by endo-N-acetylglucosaminidase from the fungus Cordyceps militaris. J Biol Chem. (2019) 294:17143–54. doi: 10.1074/jbc.RA119.010842
119. Trastoy B, Lomino JV, Pierce BG, Carter LG, Günther S, Giddens JP, et al. Crystal structure of Streptococcus pyogenes EndoS, an immunomodulatory endoglycosidase specific for human IgG antibodies. Proc Natl Acad Sci USA. (2014) 111:6714–9. doi: 10.1073/pnas.1322908111
120. Klontz EH, Trastoy B, Derecrcre D, Fields JK, Li C, Orwenyo J, et al. Molecular basis of broad spectrum N-glycan specificity and processing of therapeutic IgG monoclonal antibodies by endoglycosidase S2. ACS Cent Sci. (2019) 5:524–38. doi: 10.1021/acscentsci.8b00917
121. Waddling CA, Plummer TH, Tarentino AL, Van Roey P. Structural basis for the substrate specificity of endo-β-N-acetylglucosaminidase F3. Biochemistry. (2000) 39:7878–85. doi: 10.1021/bi0001731
122. Van Roey P, Rao V, Plummer TH, Tarentino AL. Crystal structure of endo-β-N-acetylglucosaminidase F1, an α/β-Barrel enzyme adapted for a complex substrate. Biochemistry. (1994) 33:13989–96. doi: 10.1021/bi00251a005
123. Rao V, Roey P, Van, Cui T, Guan C. Mutations of endo-β-N-acetylglucosaminidase H active site residues Asp 130 and Glu 132: Activities and conformations. Protein Sci. (2008) 8:2338–46. doi: 10.1110/ps.8.11.2338
124. Rao V, Guan C, Roey PV. Crystal structure of endo-β-N-acetylglucosaminidase H at 1.9 å resolution: active-site geometry and substrate recognition. Structure. (1995) 3:449–57. doi: 10.1016/S0969-2126(01)00178-2
125. Stals I, Karkehabadi S, Kim S, Ward M, Van Landschoot A, Devreese B, et al. High resolution crystal structure of the endo-N-Acetyl-β-D-glucosaminidase responsible for the deglycosylation of hypocrea jecorina cellulases. PLoS One. (2012) 7:e40854. doi: 10.1371/journal.pone.0040854
126. Yan J, Ding J, Liang X. Chromatographic methods for the analysis of oligosaccharides in human milk. Anal Methods. (2017) 9:1071–7. doi: 10.1039/C6AY02982E
127. Ruhaak LR, Deelder AM, Wuhrer M. Oligosaccharide analysis by graphitized carbon liquid chromatography-mass spectrometry. Anal Bioanal Chem. (2009) 394:163–74. doi: 10.1007/s00216-009-2664-5
128. Hong Q, Ruhaak LR, Totten SM, Smilowitz JT, German JB, Lebrilla CB. Label-free absolute quantitation of oligosaccharides using multiple reaction monitoring. Anal Chem. (2014) 86:2640–7. doi: 10.1021/ac404006z
129. Tonon KM, Miranda A, Abrão ACFV, de Morais MB, Morais TB. Validation and application of a method for the simultaneous absolute quantification of 16 neutral and acidic human milk oligosaccharides by graphitized carbon liquid chromatography-electrospray ionization-mass spectrometry. Food Chem. (2019) 274:691–7. doi: 10.1016/j.foodchem.2018.09.036
130. Nwosu CC, Aldredge DL, Lee H, Lerno LA, Zivkovic AM, German JB, et al. Comparison of the human and bovine milk N-Glycome via high-performance microfluidic chip liquid chromatography and tandem mass spectrometry. J Proteome Res. (2012) 11:2912–324. doi: 10.1021/pr300008u
131. Dong X, Zhou S, Mechref Y. LC-MS/MS analysis of permethylated free oligosaccharides and N-glycans derived from human, bovine, and goat milk samples. Electrophoresis. (2016) 37:1532–48. doi: 10.1002/elps.201500561
132. Elwakiel M, Bakx EJ, Szeto IM, Li Y, Hettinga KA, Schols HA. Serum protein N-glycans in colostrum and mature milk of Chinese mothers. J Agric Food Chem. (2020) 68:6873–83. doi: 10.1021/acs.jafc.0c02161
133. Gao X, Lu Y, Wei M, Yang M, Zheng C, Wang C, et al. Matrix-assisted laser desorption/ionization time-of-flight mass spectrometry analysis of human milk neutral and sialylated free oligosaccharides using Girard's reagent P on-target derivatization. J Agric Food Chem. (2019) 67:8958–66. doi: 10.1021/acs.jafc.9b02635
134. Zhang Y, Wang B, Jin W, Wen Y, Nan L, Yang M, et al. Sensitive and robust MALDI-TOF-MS glycomics analysis enabled by Girard's reagent T on-target derivatization (GTOD) of reducing glycans. Anal Chim Acta. (2019) 1048:105–14. doi: 10.1016/j.aca.2018.10.015
135. Bentley ME, Dee DL, Jensen JL. Breastfeeding among low income, African-American women: power, beliefs and decision making. J Nutr. (2003) 133:305S−9S. doi: 10.1093/jn/133.1.305S
136. Hedberg IC. Barriers to breastfeeding in the WIC population. MCN Am J Matern Nurs. (2013) 38:244–9. doi: 10.1097/NMC.0b013e3182836ca2
137. Earle S. Factors affecting the initiation of breastfeeding: implications for breastfeeding promotion. Health Promot Int. (2002) 17:205–14. doi: 10.1093/heapro/17.3.205
138. Thulier D. Breastfeeding in America: a history of influencing factors. J Hum Lact. (2009) 25:85–94. doi: 10.1177/0890334408324452
139. Singh R, Kumar M, Mittal A, Mehta PK. Microbial enzymes: industrial progress in 21st century. 3 Biotech. (2016) 6:174. doi: 10.1007/s13205-016-0485-8
140. Cabana MD. The role of hydrolyzed formula in allergy prevention. Ann Nutr Metab. (2017) 70:38–45. doi: 10.1159/000460269
141. Opdenakker G, Rudd PM, Ponting CP, Dwek RA. Concepts and principles of glycobiology. FASEB J. (1993) 7:1330–7. doi: 10.1096/fasebj.7.14.8224606
142. Russell D, Oldham NJ, Davis BG. Site-selective chemical protein glycosylation protects from autolysis and proteolytic degradation. Carbohydr Res. (2009) 344:1508–14. doi: 10.1016/j.carres.2009.06.033
143. Loomes KM. Structural organisation of human bile-salt-activated lipase probed by limited proteolysis and expression of a recombinant truncated variant. Eur J Biochem. (1995) 230:607–13. doi: 10.1111/j.1432-1033.1995.tb20602.x
144. Thangarajah H, Wong A, Chow DC, Crothers JM, Forte JG. Gastric H-K-ATPase and acid-resistant surface proteins. Am J Physiol Liver Physiol. (2002) 282:G953–G61. doi: 10.1152/ajpgi.00399.2001
145. Wang C, Eufemi M, Turano C, Giartosio A. Influence of the carbohydrate moiety on the stability of glycoproteins. Biochemistry. (1996) 35:7299–307. doi: 10.1021/bi9517704
146. Amigo-Benavent M, Clemente A, Ferranti P, Caira S, del Castillo MD. Digestibility and immunoreactivity of soybean β-conglycinin and its deglycosylated form. Food Chem. (2011) 129:1598–605. doi: 10.1016/j.foodchem.2011.06.015
Keywords: human milk oligosaccharides, N-glycans, endo-β-N-acetylglucosaminidase, bifidobacteria, infant formula
Citation: Duman H, Kaplan M, Arslan A, Sahutoglu AS, Kayili HM, Frese SA and Karav S (2021) Potential Applications of Endo-β-N-Acetylglucosaminidases From Bifidobacterium longum Subspecies infantis in Designing Value-Added, Next-Generation Infant Formulas. Front. Nutr. 8:646275. doi: 10.3389/fnut.2021.646275
Received: 25 December 2020; Accepted: 22 February 2021;
Published: 09 April 2021.
Edited by:
David A. Sela, University of Massachusetts Amherst, United StatesReviewed by:
Gianluca Picariello, National Research Council (CNR), ItalySylvie Françoise Rebuffat, Muséum National d'Histoire Naturelle, France
Copyright © 2021 Duman, Kaplan, Arslan, Sahutoglu, Kayili, Frese and Karav. This is an open-access article distributed under the terms of the Creative Commons Attribution License (CC BY). The use, distribution or reproduction in other forums is permitted, provided the original author(s) and the copyright owner(s) are credited and that the original publication in this journal is cited, in accordance with accepted academic practice. No use, distribution or reproduction is permitted which does not comply with these terms.
*Correspondence: Sercan Karav, c2VyY2Fua2FyYXZAY29tdS5lZHUudHI=