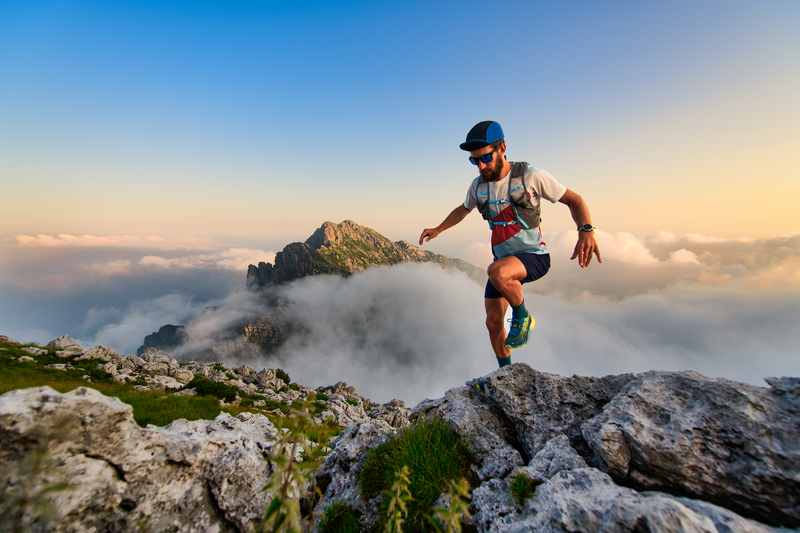
95% of researchers rate our articles as excellent or good
Learn more about the work of our research integrity team to safeguard the quality of each article we publish.
Find out more
ORIGINAL RESEARCH article
Front. Nutr. , 15 April 2021
Sec. Nutrition and Metabolism
Volume 8 - 2021 | https://doi.org/10.3389/fnut.2021.588466
Acetate is one of the main short chain fatty acids produced in the colon when fermentable carbohydrates are digested. It has been shown to affect normal metabolism, modulating mitochondrial function, and fatty acid oxidation. Currently, there is no clear consensus regarding the effects of acetate on tumorigenesis and cancer metabolism. Here, we investigate the metabolic effects of acetate on colon cancer. HT29 and HCT116 colon cancer cell lines were treated with acetate and its effect on mitochondrial proliferation, reactive oxygen species, density, permeability transition pore, cellular bioenergetics, gene expression of acetyl-CoA synthetase 1 (ACSS1) and 2 (ACSS2), and lipid levels were investigated. Acetate was found to reduce proliferation of both cell lines under normoxia as well as reducing glycolysis; it was also found to increase both oxygen consumption and ROS levels. Cell death observed was independent of ACSS1/2 expression. Under hypoxic conditions, reduced proliferation was maintained in the HT29 cell line but no longer observed in the HCT116 cell line. ACSS2 expression together with cellular lipid levels was increased in both cell lines under hypoxia which may partly protect cells from the anti-proliferative effects of reversed Warburg effect caused by acetate. The findings from this study suggest that effect of acetate on proliferation is a consequence of its impact on mitochondrial metabolism and during normoxia is independent of ACCS1/2 expression.
Acetate, one of the main short chain fatty acids produced as a result of ingestion of fermentable carbohydrates has previously been shown to demonstrate anti-tumorigenic effects following its delivery as acetate encapsulated in liposomes (1). However, there is conflicting evidence regarding its effects; acetate has been shown to reduce cell viability of colon cancer cells in vitro (2, 3) and reduce cancer cell proliferation in the liver in vivo (4). Moreover, acetate has also been shown to increase colon cancer cell death in combination with propionate; an effect further increased at a reduced pH (5). Conversely, others have reported no effect of acetate on colon cancer cell lines, despite showing that other short chain fatty acids, butyrate and propionate, reduce cell proliferation (6–9). Although the underlying mechanisms have not been fully elucidated, Jan et al. suggest that acetate (and other SCFAs) induce cell death through mitochondrial changes, such as swelling and increased ROS production (3).
Recent findings have suggested that there may be different mechanisms which account for the effect of acetate on cancer cell proliferation. Long et al. have shown that glyceryl acetate (GTA), an FDA approved food additive, is hydrolyzed in cells to produce free acetate, reducing the growth of malignant brain tumors through cell growth arrest (10). Whereas, Mashimo et al. have reported that acetate is oxidized by tumor cancer cells and increases cytosolic acetyl-CoA synthetase (ACSS2) expression, making tumors more aggressive as silencing these genes is known to reduce malignancy (11). Indeed, Comerford et al. and Schug et al. have shown that acetate is vital for tumor growth since silencing the ACSS2 gene suppresses tumor growth in both liver (12) and prostate cancer cell lines (13).
We have previously reported that acetate has beneficial effects on mitochondria of liver and adipose tissue, improving oxidative phosphorylation (14). As initially observed by Warburg, cancer cells under normoxia convert glucose to lactate and have reduced oxidative phosphorylation and increased glycolysis (15). We have previously shown reduced tumor growth with acetate delivery to tumors in vivo (1), here we investigate the metabolic effects of acetate on colon cancer cell lines HT29 and HCT116 as a possible mechanism for reduced tumor growth.
HT29 and HCT116 colon cancer cell lines were obtained from ATCC (LGC Standards, Middlesex, UK) and grown in DMEM (Sigma-Aldrich, UK) and RPMI (ThermoFisher Scientific, USA) media, respectively, supplemented with 1% glutamate (ThermoFisher Scientific, USA) and penicillin and streptomycin (Sigma-Aldrich, UK) and 10% FBS (ThermoFisher Scientific, USA) at 37°C with 5% CO2. Cells were used for experimental procedures between passage numbers 3 and 18.
For cell growth, cells were seeded in 96-well plates at 20 × 103 cells per well and incubated for 24 h, followed by 24 h treatment with 1 or 10 mM sodium acetate (NaAc). One and 10 mM NaAc were diluted in media from a 1 M stock solution which was prepared by dissolving sodium acetate salt (Sigma-Aldrich, UK) in deionised water, adjusting pH to 7.4 by NaOH and filtering. Cell growth was measured using sulforhodamine B (SRB, Sigma-Aldrich, UK) colorimetric assay as described previously (16). Briefly cells were fixed with 10% (w/v) trichloroacetic acetic acid solution at 4°C for 1 h, rinsed, and stained by 0.4% (w/v) SRB in 0.1% (v/v) acetic acid for 30 min. The plates were washed by 0.1% (v/v) acetic acid to remove unbound SRB dye, and the dye was dissolved by 10 mM Tris base. Absorbance was measured at 500 nm and at 690 nm (as background), using a microplate reader (SPECTROStar Nano, BMG LABTECH, Ortenberg, Germany).
For cell proliferation, cells were seeded 20 × 103 cells per well on a 96-well plate and treated with 10 mM NaAc for 24 h. On the day of the assessment, cells were treated with BrdU, fixed and assay was carried out using a BrdU Cell Proliferation Assay (Millipore) according to the manufacturer's instructions. Plates were read using a SPECTROStar Nano (BMG Labtech, Germany) at 450 and 540 nm.
ATP/ADP ratio was measured to assess the effects of acetate on apoptosis and necrosis. Cells were seeded 20 × 103 cells per well on 96-well plates. On the following day, cells were treated with control, 1 or 10 mM NaAc for 24 h. ATP and ADP levels (n = 4) were measured using ADP/ATP Ratio Assay Kit (Merc, UK) by FLUOstar Optima (BMG Labtech, Germany).
For detection of cellular ROS, cells were seeded 25 × 103 cells per well on a 96-well plate and received either control, 1 or 10 mM NaAc treatment for 15 min or 24 h. Then cells were stained with 2′,7′-dichlorofluorescin diacetate (DCFDA) using DCFDA Cellular ROS Detection Kit according to the manufacturer's instructions (Abcam, USA) and read on a fluorescent plate reader (ex/em: 495/529 nm) (FLUOstar Optima).
To assess mitochondrial density CS activity assay was employed. Cells were seeded 6 × 106 per flask and next day treated with control, 1 or 10 mM acetate for 24 h (n = 3). Cells were then collected, and CS activity was measured with Citrate Synthase Activity Assay Kit (Merc, UK) according to manufacturer's instructions and read for 40 min with 5 min intervals at 412 nm using SPECTROStar Nano.
To assess the opening of mitochondrial permeability transition pore (mPTP), a CRC assay was carried out. Cells were seeded 6 × 106 per flask and next day treated with control, 1 or 10 mM acetate for 24 h (n = 3). Cells were then collected and mitochondria isolated using a Mitochondria Isolation Kit (Abcam, UK). Protein concentration of mitochondria were measured using a Bradford protein assay (Bio-Rad, US). Isolated mitochondria were washed with and reconstituted in mitochondrial assay buffer (MAB) at 2 mg/ml concentration and incubated with 0.7 μM Fluo-4FF (ThermoFisher Scientific, USA) prepared in MAB supplemented with 20 mM glutamate (Merc, UK) and 4 mM malate (Merc, UK) for 10 min in black 96-well plates. After a basal measurement, 100 μM CaCl2 (Merc, UK) was added into each well, this was repeated for 10 times, with 3 min between each dose. Repeated measurements were made in between injections (17) using FLUOstar Optima (ex./em.: 485/570 nm). Data were analyzed as change from minimum and maximum fluorescence values. Area under the CRC curves were also calculated.
Oxygen consumption rate (OCR) and extracellular acidification rate (ECAR) was measured using a XFe24 Extracellular Flux Analyzer (Seahorse Bioscience) and the experiments were performed according to the manufacturer's protocols using 20 × 103 HT29 and 30 × 103 HCT116 cells/well in 24-well plates. For 12 h continuous measurement, cells were treated with acetate after basal measurement. For mitochondrial stress and glycolysis experiments, cells were incubated with acetate for 24 h or received acetate during the experiment. Basal mitochondrial respiration in the presence of acetate (basal cellular respiration minus non-mitochondrial respiration), proton leak (oligomycin inhibited respiration minus non-mitochondrial respiration), ATP turnover-driven respiration (basal respiration minus oligomycin inhibited respiration), maximal mitochondrial respiratory capacity (uncoupler inhibited respiration) and non-mitochondrial respiration (rotenone-antimycin A inhibited respiration) were measured as OCR, as per manufacturer's instructions, by adding oligomycin (Sigma-Aldrick, UK, 1 μM) carbonyl cyanide 4-trifluoromethoxy phenylhydrazone (FCCP, Sigma-Aldrich, UK), HT29: 1 μM, HCT116: 0.5 μM) and rotenone and antimycine mix (Sigma-Aldrich, UK, 0.5 μM). Glycolysis, glycolytic capacity, and glycolytic reserve were measured as ECAR by adding glucose (Sigma, HT29: 4,500 mg/L, HCT116: 2,000 mg/L), oligomycin (using the doses mentioned above) and 2DG (50 mM, Sigma-Aldrich, UK). Metabolic potential was also measured at baseline and under stress by treating with oligomycin and FCCP simultaneously. For mitochondrial function, glycolysis and metabolic potential experiments, OCR and ECAR were normalized with total protein content at the end of the experiment quantified by Pierce™ BCA Protein Assay (ThermoFisher Scientific, USA).
To assess mRNA expression by quantitative RT-PCR, cells were seeded 5 × 106 cells per flasks. Next day cells were treated with control or 10 mM acetate for 24 h. Cells were harvested, and total mRNA was extracted using RNeasy kit (Qiagen, USA) according to manufacturer's instructions. RT reactions were performed using SuperScript™ IV First-Strand Synthesis System (ThermoFisher Scientific, USA) using 1 μg/mL RNA concentration and used in RT-PCR. Quantitative PCR reactions were performed using TaqMan™ Universal Master Mix II, no UNG (ThermoFisher Scientific, USA) (n = 5) using Applied Biosystems 7500 Fast Real-Time PCR System (Life Technologies, USA). Taqman gene expression assays (ThermoFisher Scientific, USA) were used for β-actin (Hs01060665_g1), ACSS1 (Hs00287264_m1), and ACSS2 (Hs00218766_m1).
To assess protein levels of ACSS1 and ACSS2, cells were seeded 6 × 106 cells per flask. The following day cells were treated with control or 10 mM acetate for 24 h (n = 3). Cells were collected and total protein was extracted after three freeze thaw cycles. ELISAs (MyBioSource, USA) were performed according to manufacturer's instructions and read at 450 nm using SPECTROStar Nano.
Cells were seeded in 6 well plates at a density of 500 × 103 cells per well. After 24 h treatment with control or acetate (1 and 10 mM), cells were stained with Oil red O (ORO) (Sigma-Aldrich, UK) following the manufacturer's instructions. Staining was then extracted using 1 ml of isopropanol and measured at 510 nm in cuvettes using a JENWAY 6300 Spectrophotometer (Cole-Parmer, UK) (18).
All statistical analysis was performed using GraphPad Prism (GraphPad Software, USA). Data are presented as mean ± standard deviation (SD) or as mean ± standard error of mean (SEM). Statistical significance was calculated with Student's t-test or one-way ANOVA. Significance was accepted at the level of *p < 0.05, **p < 0.01, ***p < 0.001.
The viability of both HT29 and HCT116 cells was significantly reduced after 24 h treatment with 10 mM acetate (Figures 1A,B), however, there was no effect with 1 mM acetate treatment. A similar effect was observed on cell proliferation, with 10 mM acetate treatment reducing proliferation on both cell lines, whilst 1 mM acetate treatment had no significant effect (Figures 1C,D). No significant change was observed in ATP/ADP ratio of HT29 cells (Control: 9.24 ± 5.06, 1 mM: 12.6 ± 6.28, and 10 mM: 4.01 ± 1.44) or HCT116 cells (0: 6.32 ± 3.9, 1 mM: 8.5 ± 9.36, and 10 mM: 6.07 ± 0.97).
Figure 1. Acetate reduces proliferation. Percent change from control in growth (measured by SRB assay) of HT29 (n = 3) (A) and HCT116 (n = 4) (B) cell lines treated with 1 or 10 mM acetate for 24 h. Percent change of cell proliferation (measured by BRDU assay) from control of HT29 (n = 4) (C) and HCT116 (n = 6) (D) cell lines treated with 1 or 10 mM acetate for 24 h. All data are shown as mean ± SD, *p < 0.05 and **p < 0.01.
ROS levels were significantly increased after 24 h treatment with 10 mM acetate in both HT29 (Figure 2A) and HCT116 cell lines (Figure 2B). However, treatment with 1 mM acetate did not alter ROS levels (Figures 2A,B), nor did a shorter 15 min treatment with either acetate concentration (data not shown).
Figure 2. Acetate increases ROS production. Percent change from control of ROS produced by HT29 (n = 9) (A) and HCT116 (n = 9) (B) cell lines treated with 1 or 10 mM acetate for 24 h. Citrate synthase activity (nmole/min/μL) of HT29 (C) and HCT116 (D) cell lines (n = 3) treated with 1 or 10 mM acetate for 24 h. CRC curve (n = 3) shown as normalized fluorescent intensity of mitochondria isolated from HT29 (E) and HCT116 (F) cell lines treated with 1 and 10 mM acetate for 24 h. Each arrow represents CaCl2 injection. Area under the CRC curves of HT29 (G) and HCT116 (H) cell lines. All data are shown as mean ± SD except from (E,F), which are shown as mean ± SEM, *p < 0.05.
Assessment of CS activity showed that acetate treatment at 10 mM caused no change in either cell lines. However, there was a significant increase in CS activity following treatment with 1 mM acetate in HCT116 cell line, suggesting increased mitochondrial density (Figures 2C,D).
The CRC assay showed a significant increase in HCT116 at 10 mM and a significant decrease at 1 mM suggesting opening of mPTP sooner and later than control, respectively. No change was observed in HT29 cell line (Figures 2E–H).
Acute acetate treatment (1 and 10 mM), where acetate was given to cells during measurements, did not alter basal respiration, ATP production, proton leak, maximal respiration, non-mitochondrial respiration or spare respiration, in either cell line (Supplementary Figures 1, 2). Similarly, no effects were observed after 24 h incubation with acetate (Supplementary Figures 3, 4). Glycolysis was suppressed in a dose dependent manner in both HT29 (Figures 3A–C) and HCT116 (Figures 3D–F) cell lines following both 1 and 10 mM acute acetate treatment.
Figure 3. Acetate reduces glycolysis in a dose dependent manner. (A) glycolysis, (B) glycolytic capacity, and (C) glycolytic reserve of HT29 cell line treated with 1 and 10 mM acetate assessed by ECAR (a representative of lactate production) (n = 4), (D) glycolysis, (E) glycolytic capacity, and (F) glycolytic reserve of HCT116 cell line treated with 1 and 10 mM acetate assessed by ECAR (n = 3). All data are shown as mean ± SD, *p < 0.05, **p < 0.01, and ***p < 0.001.
Time-course study of cells treated with 10 mM acetate showed an increased oxygen consumption rate (OCR) accompanied by a reduced extracellular acidification rate (ECAR) in HT29 (Figures 4A,B) and HCT116 cell lines (Figures 4C,D). OCR of HT29 cell line was significantly increased for the first 200 min but not thereafter (Figure 4A) whereas ECAR was significantly reduced throughout the treatment (Figure 4B). For HCT116 cell line, OCR of acetate treated cells was significantly higher throughout the experiment (Figure 4C) whilst ECAR was significantly reduced during the first 200 min of treatment (Figure 4D).
Figure 4. Acetate causes changes in metabolism (time-course study). Time-course measurement of OCR (pmol/min) and ECAR (pmol/min) for HT29 cell line (A,B), respectively and HCT116 cell line (C,D), respectively, treated with control and 10 mM acetate, measured continuously for 12 h, shown as change from baseline (OCR and ECAR measurements before acetate administration, n = 4 for HT29 and 3 for HCT116). All data are shown as mean ± SEM. Data analyzed by AUC and student's t-test, *p < 0.05 and **p < 0.01.
Under normal oxygen conditions, acetate increased the expression of ACSS2 in the HT29 cell line but not ACSS1 (Figure 5A). In the HCT116 cell line, expression of both enzymes remained unaffected (Figure 5B). Under hypoxia, ACSS1 expression was suppressed in HT29 cell line while ACSS2 expression was increased in both cell lines (Figures 5A,B). No change was observed in protein levels of ACSS1 and ACCS2 in either cell lines under normoxia (Supplementary Figure 5).
Figure 5. Effects of acetate under metabolic stress are modified. (A) Fold change in ACSS1 and ACSS2 mRNA expression of 10 mM acetate treated HT29 cells under normoxia and hypoxia compared to control treated cells (represented by dotted line, n = 3), (B) fold change in ACSS1 and ACSS2 mRNA expression of 10 mM acetate treated HCT116 cells under normoxia and hypoxia compared to control treated cells (represented by dotted line, n = 3), (C) relative change in ORO staining, measured as absorbance at 510 nm of HT29 cell line treated with 10 mM acetate from control (represented by dotted line, n = 5). (D) Relative change in ORO staining, measured as absorbance at 510 nm of HCT116 cell line treated with 10 mM acetate from control (represented by dotted line, n = 5). Percent change from control in growth of HT29 (n = 3) (E) and HCT116 (n = 3) (F) cell lines treated with 1 or 10 mM acetate for 24 h under hypoxia, metabolic potential of HT29 (n = 3) (G) and HCT116 (n = 5) (H) cell lines treated with 1 and 10 mM acetate measured as stressed OCR and ECAR under stressed conditions induced by simultaneous treatment with oligomycin and FCCP. All data represented as mean ± SD, *p < 0.05, **p < 0.01, and ***p < 0.001.
ORO staining showed increased lipid levels following 24 h 10 mM acetate treatment under hypoxic conditions in both cell lines. One millimolar treatment also increased ORO staining in HT29 cell line but did not reach significance in HCT166 cell line. No effect was observed under normal oxygen conditions (Figures 5C,D).
Under hypoxic conditions, 24 h acetate (10 mM) treatment reduced proliferation of HT29 cell line but had no effect on HCT116 cells (Figures 5E,F).
Under stressed conditions both 1 and 10 mM acute acetate treatment reduced the metabolic potential of the ECAR, which indicates the preferred energy pathway of the HT29 cells (Figure 5G). 10 mM acute acetate treatment reduced the metabolic potential of ECAR in HCT116 cells, with no effect observed at the lower acetate concentration (Figure 5H).
In this study we report that acetate increases oxygen consumption and ROS production while reducing glycolysis and cell proliferation. We showed that these effects occurred in the absence of suppressed ACSS2 expression. Under conditions of metabolic stress, in this case hypoxia, acetate's effect on the HT29 cell line was maintained but no longer observed in the HCT116 cell line.
Under normal metabolic conditions, cellular acetate is metabolized into acetyl-CoA by two different enzymes, mitochondrial ACSS1 (19) and cytosolic ACSS2 (20). The ultimate fate of acetate is thought to depend on the enzyme responsible for converting it to acetyl-CoA. Acetyl-CoA produced by ACSS1 is predominantly used for oxidation (20) whilst acetyl-CoA produced by ACSS2 is destined for lipid synthesis (19). Previous work has shown that tumor growth is reduced by suppressing ACSS2 expression in most cancers (12, 13). Cells are more dependent on acetate metabolism when metabolically stressed, including hypoxia and/or nutrients (13), which may explain increased ACSS2 expression in cancer. Indeed, Mashimo et al. reported that ACSS2 expression is increased in brain tumors (11). Although most work has focused on ACSS2, Lakhter et al. showed that suppression of ACSS1 expression results in reduced cell growth, accompanied by increased OXPHOS following acetate treatment (21). Hence, it appears that under some circumstances, endogenous acetate could play a role in cancer progression (12, 13, 21).
Despite this, several studies have shown that acetate can reduce cell growth in stem-like cells and colon cancer (2, 10, 22, 23). Otto Warburg was first to show that cancer cells preferentially display aerobic glycolysis and their growth can be modified by respiration (24), a key hallmark of cancer (25). Schulz et al. demonstrated that growth and colony formation of several colon cancer cell lines can be reduced by reversing the Warburg effect through increasing mitochondrial oxygen consumption (26, 27). We have previously shown that while acetate can enhance the mitochondrial function of non-cancerous cells (14), it can also reduce tumor sizes in xenograph models of colon cancer (1). We therefore hypothesized that the impact of acetate on mitochondrial function could be the underlying mechanism. Although the effect on cell proliferation observed was small, this was in response to acute acetate treatment. Our previous study showed that chronic acetate treatment reduces tumor volume by half (1). Under normoxia, we found no effect at zero or 24 h in mitochondrial function but continuous measurements showed that acetate treatment increased oxygen consumption and reduced glycolysis in both HT29 and HCT116 cells lines, reversing the Warburg effect. Pavlides et al. suggested that the Warburg effect is not limited to cancer cells and stromal cells can switch metabolism to aerobic glycolysis, termed “Reverse Warburg Effect” (28) and that inhibition of glycolysis reduces tumor growth (29). As we observed reduced glycolysis following acetate treatment, it may be worthwhile for further studies to explore whether acetate can be used to reverse the “Reverse Warburg Effect.”
As expected, changes in oxygen consumption and glycolysis were accompanied by increased ROS production, as glycolysis has been shown to be a ROS scavenger (30). Cancer cells are heavily dependent on glycolysis for energy production, as well as generating the macromolecules needed for proliferation and intermediates for redox homeostasis (30). By reducing glycolysis, in our case with exogenous acetate, ROS production is increased thus providing sufficient stress to explain the reduction in tumor growth (31). ROS may cause cell death through the opening of mPTP, which triggers the release of more ROS, which in turn becomes detrimental to mitochondria and the cell (32). We have observed some evidence that acetate causes a biphasic effect on mPTP opening in HCT116 cell line, a lower dose delays its opening, while the higher dose accelerates it. This effect was not observed in HT29 cell line and warrants further exploration to ascertain if chronic treatments or higher doses of acetate would induce an effect.
In the current study, ACSS2 expression, intracellular lipid deposition and cellular proliferation, in the presence of acetate, were clearly dependent on environmental conditions. Under normoxia, cell proliferation was reduced independent of ACSS2 expression, however under hypoxia both ACSS2 expression and lipid levels were increased with proliferation reduced only in the HT29 cell line. ACSS2 is involved in synthesis of lipids and growth of breast cancer cells in hypoxia (13). The importance of lipid metabolism in tumor growth has previously been demonstrated as the blocking of lipid synthesis protects against tumor growth (33) and cancer cells can reduce oxidative stress and cell death by upregulating lipogenesis (34). Gao et al. showed that ACSS1 and ACSS2 are involved in activation of genes involved in lipogenesis through increased histone acetylation under hypoxia (35). Our results suggest that the HCT116 cell line is more glycolytic than the HT29 cell line. As the cells increase glycolysis under hypoxic conditions (36), any suppression that acetate caused may not have been of sufficient magnitude to reduce cell growth in the HCT116 cell line. Therefore, we observed a reduction in cell proliferation under normal oxygen conditions independent of ACSS2 expression while under hypoxic conditions, ACSS2 expression could protect against cell death caused by acetate depending on the glycolytic rate of the cells. Another mechanism by which acetate increases proliferation under hypoxia is through acetylation and activation of Hypoxia Inducible Factor-2 (HIF-2) in response to stressors; knocking down ACSS2 in hypoxia reduces cell proliferation (37). Acetate supplementation has been shown to increase cell proliferation both in vivo and in vitro through ACSS2 and HIF-2 pathway in a fibrosarcoma cell line, where ACSS2 translocates into nucleus and acetyl-CoA increases HIF-2 acetylation thereby increasing growth and proliferation (38). This contradictory finding to our present study may relate to differences in metabolism within the different cell lines used, as well as the dose of acetate used (5 mM).
These results, taken together with findings from previous studies, suggest two opposing functions for endogenous and exogenous acetate. Endogenous acetate is vital for cancer cell proliferation as shown by reduced cell growth in cells where ACSS1/2 is silenced (11–13, 21), whereas exogenous acetate reduces cell proliferation. This was shown previously (1) and confirmed by the current work where reduced cell proliferation was observed despite increased ACSS2 gene expression (Figure 6).
Figure 6. The fate of acetate. Figure summarizing the fate of exogenous acetate in cancer cells under normoxia and hypoxia. In non-cancerous cells, exogenous acetate is taken into mitochondria and increases the rate of TCA cycle and OXPHOS. In cancer cells, mitochondrial function is decreased and lactate production is increased (Warburg effect) so endogenous acetate ends up being made into lipids and used in histone acetylation which can increase cell proliferation. Under normoxic conditions in cancer cells, exogenous acetate increases OXPHOS, reducing glycolysis which results in increased ROS and reduced proliferation. Under hypoxia in cancer cells, exogenous acetate increases lipid synthesis, or it can increase HIF-2 signaling [shown by Chen et al. (38)] both of which can protect the cells from cell death induced by reduced glycolysis.
Although we observed significant changes in cellular bioenergetics which can explain the anti-proliferative effects of acetate, acetate has also been shown to be involved in modifying histone acetylation. In addition to its effects on lipid synthesis and HIF-2 activation (36–38), dietary acetate supplementation in rats was shown to reduce HDAC activity and increase histone acetylation in the brain (39, 40). We have also previously shown that chronic treatment with acetate can reduce expression of various HDACs (1). Here, we show that epigenetic modifications by acetate, such as changes in expression of ACSS2, alone is not enough in explaining its effects and bioenergetic modifications by acetate can help us understand how it reduces proliferation. Mitochondrial proteins are highly acetylated [see review by Baeza et al. (41)] and as cellular energy status modulates mitochondrial protein acetylation [see review by Anderson and Hirschey (42)], acetate can also play a role in acetylation of mitochondrial proteins. Nevertheless, studies combining genetic, epigenetic, and metabolic measurements will be necessary to fully understand the effects of acetate.
In summary, we have shown that exogenous acetate can reduce cell proliferation through modulation of mitochondrial function. It increases OXPHOS, reduces glycolysis and increases ROS which we believe is the mechanism underlying its actions. The effect on cell growth however, depends on the cell line and factors, such as glycolytic rate of the cell and metabolic stressors, such as hypoxia.
The raw data supporting the conclusions of this article will be made available by the authors, without undue reservation.
MS-A designed and performed the experiments, performed the data analyses, and drafted the manuscript. RM and NS performed the continuous cellular bioenergetics and cell proliferation experiments, respectively. All authors contributed to the article and approved the submitted version.
The authors declare that the research was conducted in the absence of any commercial or financial relationships that could be construed as a potential conflict of interest.
HCT116 cell line was a kind gift from Dr. Naveenan Navaratnam Cellular Stress Group (MRC Clinical Sciences Centre, Imperial College London, Hammersmith Hospital, London, UK).
The Supplementary Material for this article can be found online at: https://www.frontiersin.org/articles/10.3389/fnut.2021.588466/full#supplementary-material
1. Brody LP, Sahuri-Arisoylu M, Parkinson JR, Parkes HG, So PW, Hajji N, et al. Cationic lipid-based nanoparticles mediate functional delivery of acetate to tumor cells in vivo leading to significant anticancer effects. Int J Nanomedicine. (2017) 12:6677–85. doi: 10.2147/IJN.S135968
2. Hague A, Elder DJE, Hicks DJ, Paraskeva C. Apoptosis in colorectal tumour cells: induction by the short chain fatty acids butyrate, propionate and acetate and by the bile salt deoxycholate. Int J Cancer. (1995) 60:400–6. doi: 10.1002/ijc.2910600322
3. Jan G, Belzacq AS, Haouzi D, Rouault A, Métivier D, Kroemer G, et al. Propionibacteria induce apoptosis of colorectal carcinoma cells via short-chain fatty acids acting on mitochondria. Cell Death Differ. (2002) 9:179–88. doi: 10.1038/sj.cdd.4400935
4. Bindels LB, Porporato P, Dewulf EM, Verrax J, Neyrinck AM, Martin JC, et al. Gut microbiota-derived propionate reduces cancer cell proliferation in the liver. Br J Cancer. (2012) 107:1337–44. doi: 10.1038/bjc.2012.409
5. Lan A, Lagadic-Gossmann D, Lemaire C, Brenner C, Jan G. Acidic extracellular pH shifts colorectal cancer cell death from apoptosis to necrosis upon exposure to propionate and acetate, major end-products of the human probiotic propionibacteria. Apoptosis. (2007) 12:573–91. doi: 10.1007/s10495-006-0010-3
6. Gamet L, Daviaud D, Denis-Pouxviel C, Remesy C, Murat JC. Effects of short-chain fatty acids on growth and differentiation of the human colon-cancer cell line HT29. Int J Cancer. (1992) 52:286–9. doi: 10.1002/ijc.2910520222
7. McBain JA, Eastman A, Nobel CS, Mueller GC. Apoptotic death in adenocarcinoma cell lines induced by butyrate and other histone deacetylase inhibitors. Biochem Pharmacol. (1997) 53:1357–68. doi: 10.1016/S0006-2952(96)00904-5
8. Siavoshian S, Blottière HM, Le Foll E, Kaeffer B, Cherbut C, Galmiche JP. Comparison of the effect of different short chain fatty acids on the growth and differentiation of human colonic carcinoma cell lines in vitro. Cell Biol Int. (1997) 21:281–7. doi: 10.1006/cbir.1997.0153
9. Comalada M, Bailón E, De Haro O, Lara-Villoslada F, Xaus J, Zarzuelo A, et al. The effects of short-chain fatty acids on colon epithelial proliferation and survival depend on the cellular phenotype. J Cancer Res Clin Oncol. (2006) 132:487–97. doi: 10.1007/s00432-006-0092-x
10. Long PM, Tighe SW, Driscoll HE, Fortner KA, Viapiano MS, Jaworski DM. Acetate supplementation as a means of inducing glioblastoma stem-like cell growth arrest. J Cell Physiol. (2015) 230:1929–43. doi: 10.1002/jcp.24927
11. Mashimo T, Pichumani K, Vemireddy V, Hatanpaa KJ, Singh DK, Sirasanagandla S, et al. Acetate is a bioenergetic substrate for human glioblastoma and brain metastases. Cell. (2014) 159:1603–14. doi: 10.1016/j.cell.2014.11.025
12. Comerford SA, Huang Z, Du X, Wang Y, Cai L, Witkiewicz AK, et al. Acetate dependence of tumors. Cell. (2014) 159:1591–602. doi: 10.1016/j.cell.2014.11.020
13. Schug ZT, Peck B, Jones DT, Zhang Q, Grosskurth S, Alam IS. Acetyl-CoA synthetase 2 promotes acetate utilization and maintains cancer cell growth under metabolic stress. Cancer Cell. (2015) 27:57–71. doi: 10.1016/j.ccell.2014.12.002
14. Sahuri-Arisoylu M, Brody LP, Parkinson JR, Parkes H, Navaratnam N, Miller AD, et al. Reprogramming of hepatic fat accumulation and “browning” of adipose tissue by the short-chain fatty acid acetate. Int J Obes. (2016) 40:955–63. doi: 10.1038/ijo.2016.23
15. Heiden MGV, Cantley LC, Thompson CB. Understanding the Warburg effect: the metabolic requirements of cell proliferation. Science. (2009) 324:1029–33. doi: 10.1126/science.1160809
16. Vichai V, Kirtikara K. Sulforhodamine B colorimetric assay for cytotoxicity screening. Nat Protoc. (2006) 1:1112–6. doi: 10.1038/nprot.2006.179
17. Briston T, Roberts M, Lewis S, Powney B, Staddon JM, Szabadkai G, et al. Mitochondrial permeability transition pore: sensitivity to opening and mechanistic dependence on substrate availability. Sci Rep. (2017) 7:10492. doi: 10.1038/s41598-017-10673-8
18. Ramírez-Zacarías JL, Castro-Muñozledo F, Kuri-Harcuch W. Quantitation of adipose conversion and triglycerides by staining intracytoplasmic lipids with oil red O. Histochemistry. (1992) 97:493–7. doi: 10.1007/BF00316069
19. Fujino T, Kondo J, Ishikawa M, Morikawa K, Yamamoto TT. Acetyl-CoA synthetase 2, a mitochondrial matrix enzyme involved in the oxidation of acetate. J Biol Chem. (2001) 276:11420–6. doi: 10.1074/jbc.M008782200
20. Luong A, Hannah VC, Brown MS, Goldstein JL. Molecular characterization of human acetyl-CoA synthetase, an enzyme regulated by sterol regulatory element-binding proteins. J Biol Chem. (2000) 275:26458–66. doi: 10.1074/jbc.M004160200
21. Lakhter AJ, Hamilton J, Konger RL, Brustovetsky N, Broxmeyer HE, Naidu SR. Glucose-independent acetate metabolism promotes melanoma cell survival and tumor growth. J Biol Chem. (2016) 291:21869–79. doi: 10.1074/jbc.M115.712166
22. Long PM, Tighe SW, Driscoll HE, Moffett JR, Namboodiri AMA, Viapiano MS, et al. Acetate supplementation induces growth arrest of NG2/PDGFRα-positive oligodendroglioma-derived tumor-initiating cells. PLoS ONE. (2013) 8:e80714. doi: 10.1371/journal.pone.0080714
23. Marques C, Oliveira CSF, Alves S, Chaves SR, Coutinho OP, Côrte-Real M, et al. Acetate-induced apoptosis in colorectal carcinoma cells involves lysosomal membrane permeabilization and cathepsin D release. Cell Death Dis. (2013) 4:e507. doi: 10.1038/cddis.2013.29
24. Warburg O. On the origin of cancer cells. Science. (1956) 123:309–14. doi: 10.1126/science.123.3191.309
25. Kroemer G, Pouyssegur J. Tumor cell metabolism: cancer's Achilles' heel. Cancer Cell. (2008) 13:472–82. doi: 10.1016/j.ccr.2008.05.005
26. Schulz TJ, Thierbach R, Voigt A, Drewes G, Mietzner B, Steinberg P, et al. Induction of oxidative metabolism by mitochondrial frataxin inhibits cancer growth: Otto Warburg revisited. J Biol Chem. (2006) 281:977–81. doi: 10.1074/jbc.M511064200
27. Poteet E, Choudhury GR, Winters A, Li W, Ryou MG, Liu R, et al. Reversing the Warburg effect as a treatment for glioblastoma. J Biol Chem. (2013) 288:9153–64. doi: 10.1074/jbc.M112.440354
28. Pavlides S, Whitaker-Menezes D, Castello-Cros R, Flomenberg N, Witkiewicz AK, Frank PG, et al. The reverse Warburg effect: aerobic glycolysis in cancer associated fibroblasts and the tumor stroma. Cell Cycle. (2009) 8:3984–4001. doi: 10.4161/cc.8.23.10238
29. Bonuccelli G, Whitaker-Menezes D, Castello-Cros R, Pavlides S, Pestell RG, Fatatis A, et al. The reverse Warburg effect: glycolysis inhibitors prevent the tumor promoting effects of caveolin-1 deficient cancer associated fibroblasts. Cell Cycle. (2010) 9:1960–71. doi: 10.4161/cc.9.10.11601
30. Brand KA, Hermfisse U. Aerobic glycolysis by proliferating cells: a protective strategy against reactive oxygen species 1. FASEB J. (1997) 11:388–95. doi: 10.1096/fasebj.11.5.9141507
31. Zhai X, Yang Y, Wan J, Zhu R, Wu Y. Inhibition of LDH-A by oxamate induces G2/M arrest, apoptosis and increases radiosensitivity in nasopharyngeal carcinoma cells. Oncol Rep. (2013) 30:2983–91. doi: 10.3892/or.2013.2735
32. Zorov DB, Juhaszova M, Sollott SJ. Mitochondrial reactive oxygen species (ROS) and ROS-induced ROS release. Physiol Rev. (2014) 94:909–50. doi: 10.1152/physrev.00026.2013
33. Sounni NE, Cimino J, Blacher S, Primac I, Truong A, Mazzucchelli G, et al. Blocking lipid synthesis overcomes tumor regrowth and metastasis after antiangiogenic therapy withdrawal. Cell Metab. (2014) 20:280–94. doi: 10.1016/j.cmet.2014.05.022
34. Cheng X, Geng F, Pan M, Wu X, Zhong Y, Wang C, et al. Targeting DGAT1 ameliorates glioblastoma by increasing fat catabolism and oxidative stress. Cell Metab. (2020) 32:229–42.e8. doi: 10.1016/j.cmet.2020.06.002
35. Gao X, Lin SH, Ren F, Li JT, Chen JJ, Yao CB, et al. Acetate functions as an epigenetic metabolite to promote lipid synthesis under hypoxia. Nat Commun. (2016) 7:11960. doi: 10.1038/ncomms11960
36. Eales KL, Hollinshead KER, Tennant DA. Hypoxia and metabolic adaptation of cancer cells. Oncogenesis. (2016) 5:e190. doi: 10.1038/oncsis.2015.50
37. Chen R, Xu M, Nagati JS, Hogg RT, Das A, Gerard RD, et al. The acetate/ACSS2 switch regulates HIF-2 stress signaling in the tumor cell microenvironment. PLoS ONE. (2015) 10:e0123612. doi: 10.1371/journal.pone.0116515
38. Chen R, Xu M, Nagati J, Garcia JA. Coordinate regulation of stress signaling and epigenetic events by Acss2 and HIF-2 in cancer cells. PLoS ONE. (2017) 12:e0190241. doi: 10.1371/journal.pone.0190241
39. Soliman ML, Rosenberger TA. Acetate supplementation increases brain histone acetylation and inhibits histone deacetylase activity and expression. Mol Cell Biochem. (2011) 352:173–80. doi: 10.1007/s11010-011-0751-3
40. Soliman ML, Smith MD, Houdek HM, Rosenberger TA. Acetate supplementation modulates brain histone acetylation and decreases interleukin-1β expression in a rat model of neuroinflammation. J Neuroinflammation. (2012) 9:51. doi: 10.1186/1742-2094-9-51
41. Baeza J, Smallegan MJ, Denu JM. Mechanisms and dynamics of protein acetylation in mitochondria. Trends Biochem Sci. (2016) 41:231–44. doi: 10.1016/j.tibs.2015.12.006
Keywords: acetate, short chain fatty acid, mitochondria, ROS, Warburg effect
Citation: Sahuri-Arisoylu M, Mould RR, Shinjyo N, Bligh SWA, Nunn AVW, Guy GW, Thomas EL and Bell JD (2021) Acetate Induces Growth Arrest in Colon Cancer Cells Through Modulation of Mitochondrial Function. Front. Nutr. 8:588466. doi: 10.3389/fnut.2021.588466
Received: 28 July 2020; Accepted: 15 March 2021;
Published: 15 April 2021.
Edited by:
Cristina Pereira-Wilson, University of Minho, PortugalReviewed by:
Yao Wang, University of California, San Francisco, United StatesCopyright © 2021 Sahuri-Arisoylu, Mould, Shinjyo, Bligh, Nunn, Guy, Thomas and Bell. This is an open-access article distributed under the terms of the Creative Commons Attribution License (CC BY). The use, distribution or reproduction in other forums is permitted, provided the original author(s) and the copyright owner(s) are credited and that the original publication in this journal is cited, in accordance with accepted academic practice. No use, distribution or reproduction is permitted which does not comply with these terms.
*Correspondence: Jimmy D. Bell, ai5iZWxsQHdlc3RtaW5zdGVyLmFjLnVr
Disclaimer: All claims expressed in this article are solely those of the authors and do not necessarily represent those of their affiliated organizations, or those of the publisher, the editors and the reviewers. Any product that may be evaluated in this article or claim that may be made by its manufacturer is not guaranteed or endorsed by the publisher.
Research integrity at Frontiers
Learn more about the work of our research integrity team to safeguard the quality of each article we publish.