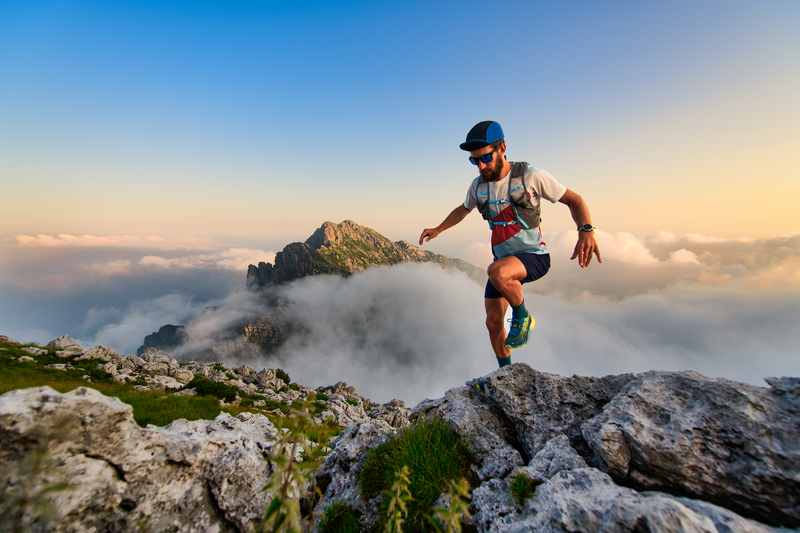
95% of researchers rate our articles as excellent or good
Learn more about the work of our research integrity team to safeguard the quality of each article we publish.
Find out more
REVIEW article
Front. Nutr. , 22 October 2020
Sec. Nutritional Immunology
Volume 7 - 2020 | https://doi.org/10.3389/fnut.2020.570344
This article is part of the Research Topic Beyond Probiotics: Dietary Microbial Modulators of the Immune System - Effects and Mechanisms View all 12 articles
Lactobacilli comprise an important group of probiotics for both human and animals. The emerging concern regarding safety problems associated with live microbial cells is enhancing the interest in using cell components and metabolites derived from probiotic strains. Here, we define cell structural components and metabolites of probiotic bacteria as paraprobiotics and postbiotics, respectively. Paraprobiotics and postbiotics produced from Lactobacilli consist of a wide range of molecules including peptidoglycans, surface proteins, cell wall polysaccharides, secreted proteins, bacteriocins, and organic acids, which mediate positive effect on the host, such as immunomodulatory, anti-tumor, antimicrobial, and barrier-preservation effects. In this review, we systematically summarize the paraprobiotics and postbiotics derived from Lactobacilli and their beneficial functions. We also discuss the mechanisms underlying their beneficial effects on the host, and their interaction with the host cells. This review may boost our understanding on the benefits and molecular mechanisms associated with paraprobiotics and probiotics from Lactobacilli, which may promote their applications in humans and animals.
The genus Lactobacillus is the largest genus among lactic acid bacteria (LAB), consisting of more than 237 species (1), with continuous new species discoveries, such as Lactobacillus metriopterae (2) and Lactobacillus timonensis (3). Some Lactobacillus species are among the most widely used probiotics (4). Accumulating evidences are proposing that probiotic cell components or metabolites which interacting with the host cells may trigger probiotic effects (5–9). The advantages of metabolites and cell components of these probiotic bacteria over probiotic bacteria were clarified (10, 11). Furthermore, it has been reported that not all probiotic bacteria are safe. Concerns associated with live probiotic bacteria administration have been described in case reports, clinical trials and experimental models (12–14). Therefore, the applications of cell components or metabolites derived from probiotic strains are gaining more interest.
Regarding the use of cell components and metabolites of probiotics, different terms have been proposed, such as “paraprobiotics,” “ghost probiotics” “inactivated probiotics” “non-viable microbial cells,” “metabolic probiotics” “postbiotics,” etc. The concept of paraprobiotics was proposed to indicate the use of inactivated microbial cells or cell fractions that confer health benefit to the host (15). In some studies, cell wall components of the probiotics are categorized as paraprobiotics (16). Postbiotics are defined as soluble products or metabolites secreted by probiotics that have physiological benefits to the host (9). Similar definition as “factors resulting from the metabolic activity of a probiotic or any released molecules capable of conferring beneficial effects to the host in a direct or indirect way” was made by other researchers (17). To better differentiate cellular structural components and metabolites of probiotic strains, we define the cell structural components (mainly cell wall components) as paraprobiotics and secretory metabolites/componnets as postbiotics in this review.
The potential health benefits of probiotic Lactobacillus species isolated from the intestine of humans and animals have been documented in a plethora of research publications to date. The terms of paraprobiotics and postbiotics have emerged recently, but they have been adopted rapidly in several study areas including food science, food microbiology, and health and nutrition of human and animals. However, knowledge on the types of paraprobiotics and postbiotics is limited and some aspects related to the bioactivities and the action mechanisms of health-promoting effects of paraprobiotics and postbiotics remain unclear. The present review aims to update the evidence on the paraprobiotics and postbiotics derived from Lactobacilli, their physiological benefits and mechanism of interaction with the host cells.
Scientific evidences showed that there are different methods to isolate and purify paraprobiotics and postbiotics from several Lactobacilli species. Isolation of paraprobiotics and postbiotics from different probiotic bacteria involve cell disruption techniques including thermal treatment (18, 19), enzymatic treatments (60), solvent extraction (20), radiation (ionizing and UV rays) (21), high pressure (22) and sonication (23–26). Several other methods also have the potential to be used for production of paraprobiotics and postbiotics, such as ohmic heating and supercritical CO2, drying, pulsed electric field (PEF), and pH changes (27).
During the production of paraprobiotics from probiotics, it is important to expose the cells to factors (27) without disrupting cell structure (9). On the other hand, to isolate intracellular postbiotics, it is required to disrupt the bacterial membrane via combined treatments in order to obtain the intracellular metabolites (9). Furthermore, extraction and clean-up steps have been applied to help the isolation procedures, such as centrifugation, dialysis, lyophilization and column purification (23, 28–30). Secreted postbiotics by viable cells can be recovered from supernatants, and the viable cells can be eliminated from the medium by centrifugation and/or filtration (31). In most of the time, we can isolate paraprobiotics and postbiotics. However, in some cases it is difficult to separate them, and additional steps such as microfiltration are necessary to isolate the postbiotic fraction. The choice of techniques for isolation of postbiotics and paraprobiotics depend on the characteristics of molecules under study (32). Since the health benefits of paraprobiotics and postbiotics are influenced by their isolation methods, it is important to select the best methods and conditions for probiotic inactivation to obtain paraprobiotics and postbiotics (33).
Studies described that most of the paraprobiotics are located in the bacterial cell-envelope (5, 34). Generally, paraprobiotics consist of a wide range of molecules including peptidoglycans, surface proteins, cell wall polysaccharides, while postbiotics include secreted proteins and peptides, bacteriocins, organic acids, etc (10, 35–37). Furthermore, the paraprobiotics and postbiotics mediate a wide range of positive effects on the host such as immunomodulatory, anti-tumor, barrier-preservation, and antimicrobial properties (24, 38). Different species of Lactobacillus have different types of paraprobiotics and postbiotics. In the following part, we summarized the chemical composition and beneficial functions of paraprobiotics and postbiotics derived from Lactobacilli (Table 1).
Studies confirmed that cell surface components of Lactobacilli are considered as an important part of effector molecules, as this part of the microbial cell is the first to interact with host cells. The cell envelope components of Lactobacilli, here categorized as paraprobiotics, include peptidoglycan, teichoic acid, cell-wall polysaccharides, cell surface-associated proteins, and proteinaceous filaments, which have been reported to mediate beneficial effects to the host (Figure 1).
Figure 1. Schematic representation of the cell surface architecture of Lactobacilli, the bilipidic cell membrane (CM) with embedded proteins is covered by a multilayered peptidoglycan (PG) shell decorated with lipoteichoic acids (LTA), wall teichoic acids (WTA), pili, proteins, and lipoproteins. Exopolysaccharides (EPS) form a thick covering closely associated with PG and are surrounded by an outer envelope of S-layer proteins. The beneficial effects of the paraprobiotics and postbiotics are denoted by numbers. (1) immunomodulatory effects; (2) antagonistic effects against pathogens; (3) anti-tumor effects; (4) preservation of intestinal barrier. Related references are as follows. Pili: immunomodulatory effects (46–48), preservation of intestinal barrier (46, 72). Protein p40/p75: immunomodulatory effects (74), preservation of intestinal barrier (17, 75–78). Aggregation promoting factor (APF) proteins: antagonistic effects against pathogens (79–83). Bacteriocins: immunomodulatory effects (9, 84–87), antagonistic effects against pathogens (88–91). LTA: immunomodulatory effects (7, 92, 93). Peptidoglycan: immunomodulatory effects (39, 94, 95). S-layers proteins: antagonistic effects against pathogens (96–98). Exopolysaccharides (EPS): immunomodulatory effects (99–102), anti-tumor effects (75, 103–106).
The cell wall of Lactobacilli contains a thick peptidoglycan layer, which is a multilayer, cross-linked glycan chain with a repeating pentapeptide unit of β-1,4-linked N-acetylglucosamine and N-acetylmuramic disaccharide units (107) and the fundamental composition of the glycan strands and pentapeptides was strain-specific for Lactobacilli (108). At the time of biosynthesis, assembly, and incorporation of peptidoglycan components, modifications happen in the bacterial peptidoglycan which could enhance the sensitivity to autolysis, hydrophobicity of the cell envelope, and resistance to lysozyme (109).
Peptidoglycan of Lactobacillus casei (L. casei), Lactobacillus johnsonii (L. johnsonii) JCM 2012 and Lactobacillus plantarum ATCC 14917 was reported to suppress interleukin-12 (IL-12) production via Toll-like receptor 2 (TLR2) which have been associated with autoimmune and inflammatory bowel diseases (94). Purified peptidoglycan from Lactobacillus salivarius (L. salivarius) Ls33 also exerted anti-inflammatory properties by inducing IL-10 production. Moreover, Ls33 peptidoglycan stimulated dendritic cell and T-cell regulatory functions upon sensing of nucleotide-binding oligomerization domain protein 2 (NOD2), and rescued mice from colitis induced by trinitrobenzene sulfonic acid (TNBS) (95). Furthermore, peptidoglycan from Lactobacillus rhamnosus (L. rhamnosus) CRL1505 was able to improve innate and systemic adaptive immune responses in mice (39). Notably, strain- or species-specific modifications of the conserved peptidoglycan polymers, including amidation, acetylation, and glycosylation, can lead to specific immunomodulatory capacities, which may contribute to the strain-specificity of probiotic effect.
Teichoic acids (TAs) are the second main constituent of cell walls of Lactobacilli and account for up to half of the cell wall dry weight (110). Due to the anionic polymers nature of the TA, it can be covalently linked to peptidoglycan as wall teichoic acid (WTA) or anchored to the cytoplasmic membrane by their lipid anchors as lipoteichoic acid (LTA) (111).
Plethora studies reported the immunomodulatory characteristics of TA from many species of Lactobacillus (112). L. plantarum LTA (Lp.LTA) attenuated the expression of IL-8 induced by Pam2CSK and exerted anti-inflammatory effects on human intestinal epithelial cells (92). LTA of L. plantarum also showed anti-inflammatory responses in porcine intestinal epithelial cells (7). The anti-inflammatory functions and effects of LTAs are species or strain-specific. For instance, it has been shown that the majority of immunomodulatory properties induced by L. plantarum TA were dependent on D-alanylation (93).
Polysaccharides are common in gram-positive bacteria surface including Lactobacilli. The most studied polysaccharides are exopolysaccharides (EPS). EPS may facilitate the interaction of the bacteria with the environment, mediate adhesion properties, protect against pathogens, and also act as a protective layer (43, 113).
Studies revealed that EPS derived from several species of Lactobacillus has a capacity to modulate systemic and mucosal immune responses, and provide direct health-promoting benefits. Purified EPS produced by L. rhamnosus RW-9595M exhibited immuno-suppressive effect on macrophages by inducing high levels of IL-10 and low or no tumor necrosis factor alpha (TNF-α), IL-6, and IL-12 (99). Moreover, the EPS-producing L. plantarum BGCG11 strain showed anti-inflammatory effect, pointing to an immune-suppressive role of EPS (100). Acidic fraction of EPS produced by L. plantarum 14 was able to decrease the production of pro-inflammatory cytokines (IL-6, IL-8, and MCP-1) in porcine intestinal epithelial cells in response to enterotoxigenic Escherichia coli (E. coli) (ETEC) challenge (101). Apart from the anti-inflammatory effect, EPS can also stimulate the immune response. EPS derived from yogurt fermented with Lactobacillus delbrueckii (L. delbrueckii) subssp. bulgaricus OLL1073R-1 induced interferon gamma (IFN-γ) production and activated natural killer (NK) cells in mice (102), which contributed to anti-viral infection effect (114). EPS can also regulate the energy metabolism of host. The EPS isolated from L. rhamnosus GG inhibited adipogenesis, and deceased the level of triacylglycerols and cholesterol ester in the liver and serum in mice (115).
Besides to the immunoregulatory effect of EPS, studies also described their anti-tumor abilities. In vitro anti-tumor assay of the EPS from L. plantarum YW32 proved their powerful inhibitory activity against colon cancer HT-29 cells (63). EPS isolated from Lactobacillus acidophilus 20079 strain can regulate both apoptotic and nuclear factor kappa B (NF-κB) inflammatory pathways in human colon cancer and have a potentiality to up-regulate the expression of IKbα, P53 and TGF genes (103). EPSs extracted from L. casei M5, L. casei SB27, L. casei X12, and L. casei K11 strains suppressed HT-29 cell growth via induction of G0/G1 cell cycle arrest and apoptosis (104). EPS from L. plantarum NCU116 induced c-Jun dependent Fas/Fasl-mediated apoptosis via TLR2 in mouse CT26 cells (105). Moreover, EPS from L. acidophilus inhibited the expressions of genes involved in tumor angiogenesis and survival of the colon cancer cell lines in vitro (106). Similarly, EPS from L. acidophilus LA1 demonstrated their anti-tumor activity in vivo against Ehrlich ascites carcinoma cells by suppressing the serum levels of malondialdehyde and nitric oxide (116) and EPS of Lactobacillus gasseri strains also showed their capability to inhibit cervical cancer cell growth and modulate immune response (117).
Surface layer proteins are one of the most important components of the outermost cell envelope structures on Lactobacilli cell surface and other probiotic bacteria species. Cell surface proteins are classified as the proteins which are covalently or non-covalently attached to the cell surface. Recent study indicated that many types of surface proteins including LPXTG proteins, S-layer proteins, pili proteins, moonlight proteins are produced by Lactobacillus species including L. plantarum, L. rhamnosus, Lactobacillus helvetics (L. helveticus), and L. acidophilus (118). These proteins play significant positive roles on the host biological processes.
LPXTG protein is one of the proteins covalently attached to the peptidoglycan of bacterial cell wall. These proteins contain a C-terminal LPXTG signal, and are linked to the cell wall by sortase A (SrtA). In Lactobacilli, LPXTG proteins are among the best-known covalent anchored surface proteins. LPXTG proteins were found in many Lactobacillus species including L. plantarum WCFS1, L. johnsonii NCC533, Lactobacillus sakei (L. sakei) 23K and L. salivarius UCC118 (119).
LPXTG proteins from different Lactobacillus species have been shown to bind to mucus and epithelial cells, and play major roles in bacteria-host interaction (120). About 12 proteins containing LPXTG motifs were identified from L. plantarum, which were involved in adhesion activity (120–122). Their major role was adherence to collagen, fibronectin, chitin, or mucus (123). Furthermore, studies with SrtA mutants of L. casei BL23 suggested that SrtA-dependent proteins participated in adhesion of this strain to Caco-2 and HT29 cells (69).
Many Lactobacilli strains, including Lactobacillus cripatus (L. crispatus) ZJ001 and JCM 5810, L. acidophilus ATCC 4356, Lactobacillus buchneri (L. buchneri) CD034, and Lactobacillus brevis ATCC 8287, display a surface coating made of a crystalline, glycoprotein subunits also known as the S-layer (121). S-layer proteins are mostly anchored to peptidoglycan by non-covalent bonds (124). S-layer proteins of Lactobacilli account about 15% of total cell wall proteins and they differ from counterparts of other bacteria in their smaller size (25–71 kDa) and higher isoelectric point values (9.4–10.4) (125). In some species of Lactobacillus, S-layers with distinctive features can be found, such as glycosylated S-layers in L. buchneri and Lactobacillus kefiri (L. kefiri) (96).
Adhesive S-layers proteins of probiotic Lactobacilli can inhibit adherence and infection of pathogenic bacteria. S-layer proteins isolated from Lactobacilli were shown to bind to host cell proteins and extracellular matrix (44, 126). The S-layer protein from L. kefiri CIDCA 8348 improved the response of macrophages to lipopolysaccharide (LPS) (125) and was able to enhance the ovalbumin-specific immune response by triggering maturation of antigen presenting cells through the recognition of glycan moieties in mice (127). Lactobacillus paracasei subp. paracasei, L. rhamnosus, and L. casei strains isolated from natural dairy products are able to inhibit Shigella sonnei adhesion to HT-29 cells via their S-layer proteins (128). Similarly, the S-layer proteins from L. helveticus fb213, L. acidophilus fb116 and L. acidophilus fb214 contributed to the adhesion of the Lactobacillus strains to HT-29 cells and helped to inhibit the adherence and invasion of E. coli ATCC 43893 (96). S-layer proteins of Lactobacillus have also been demonstrated to competitively bind the intestinal epithelium in vivo and inhibit pathogen infection (97, 98).
Pili are elongated protein structures protruding outside bacterial cells. Initially pili were considered as special features of pathogens (129), until they were found in L. rhamnosus. Pili bind to the intestinal mucusa and promote persistence of Lactobacillus strains in GI tract (130, 131). The SpaCBA pili of L. rhamnosus GG were a binding factor to human intestinal mucus, collagen, and intestinal epithelial cell (IEC) lines (46), and SpaC was credited as the major adhesion determinant (71, 107).
Studies also suggested other beneficial effects of pili derived from Lactobacillus strains. Mutant of L. rhamnosus GG devoid of SpaC induced increased mRNA expression of the pro-inflammatory cytokines IL-8 and TNF-α in Caco-2 cells while wild-type L. rhamnosus GG or SpaC alone had little impact on cytokine production (46). The immunomodulatory effect of SpaC was also observed in human fetal intestinal epithelial cell line H4 by modulating TLR-related gene expression (47). Comparative analysis of L. rhamnosus GG wild-type and isogenic pili mutants have shown immunoregulatory function of pilli by interactions with monocytes and dendritic cells (46, 48). Similar comparison also demonstrated that pilli can promote pathogen exclusion including pilliated Enterococcus faecium (132). Furthermore, SpaCBA pilli have been reported to be involved in promotion of cell proliferation in intestinal crypts, and protection against radiological insults (133). The SpaC pilin of L. rhamnosus GG (LGG) has been confirmed to induce the generation of reactive oxygen species (ROS) in epithelium and play a role in stimulating ERK phosphorylation and protecting the gut's epithelial barrier (133).
Moonlighting proteins include various classes of proteins, including translational elongation factors, metabolic enzymes, ribosomal proteins, and molecular chaperones (134–138). They are found in many species of Lactobacillus including L. crispatus, L. plantarum, Lactobacillus reuteri (L. reuteri) and Lactobacillus jensenii (L. jensenii) (135, 139–141).
Moonlighting proteins can mediate the colonization of the probiotic strains in intestinal tract. L. acidophilus used surface GAPDH to colonize the gut (142). Lactobacillus species including L. plantarum, Lactobacillus fermentum (L. fermentum), and L. jensenii were found to use moonlighting proteins in competitive exclusion and displacement of pathogens (140). Furthermore, moonlighting proteins including GAPDH, enolase and EF-Tu were involved in plasminogen/plasmin binding and activation (143), which might interfere with the exploitation of plasminogen by gastrointestinal pathogens that express plasminogen receptors or activators, such as Helicobacter pylori and Salmonella sp. (144).
As postbiotics, different secretory components of probiotic Lactobacillus strains have been reported to mediate beneficial effects, including proteins, peptides, organic acids, and other small molecules. These components can be secreted by live bacteria or released into the host environment after bacteria lysis and confer various physiological benefits to the host.
Protein p40 and p75 were identified from many Lactobacilli species including L. casei, L. paracasei, and L. rhamnosus (145). They are secreted cell wall muramidases and have approximately molecular sizes of 40 and 75 kDa, respectively (74). The positive contribution of these proteins secreted from Lactobacillus species has been described in several studies. The protein p40 from L. rhamnosus GG showed an immunomodulatry action in mice (74). The p40 transactivated the epidermal growth factor receptor (EGFR) in intestinal epithelial cells, inhibited apoptosis and preserved barrier function in the colon, thereby ameliorating intestinal injury and inflammation (17, 75, 76, 78, 109). Besides, p75 purified from L. rhamnosus GG and L. casei BL23 have anti-apoptotic activity by inducing the EGF/Akt pathway (145). Furthermore, the p40 and p75 proteins were able to protect the intestinal epithelial tight junctions and barrier functions by a protein kinase (PKC) and MAP kinase-dependent mechanism (76).
Lactobacillus species have been reported to secrete a number of aggregation promoting factor (APF) proteins, which are extracellular proteins responsible for bridging of conjugal pairs, self-aggregation, maintenance of cellular shape, and co-aggregation with other commensal or pathogenic bacteria (73, 82).
The function of APF from Lactobacilli mainly involves host colonization and pathogen exclusion. Previous studies demonstrated that L. gasseri SBT2055 decreased adhesion and invasion of Campylobacter jejuni (C. jejuni) in vitro and hindered its infection in chickens via co-aggregation with the pathogens, and the co-aggregation was mediated by proteinaceous cell-surface components (79). Similarly, Yungareva and Urshev (81) also confirmed that APF in Lactobacillus delbr (L. delbr). subspp Bulgaricus had co-aggregation property which inhibited the growth of pathogenic bacteria. APF-2 from L. gasseri ATCC 9857 strain contributed to inhibition of the adhesion of Trichomonas vaginalis to human vaginal ectocervical cells (80, 81). Furthermore, the presence of high concentration of intracellular GGDEF protein (DgcA) in L. acidophilus and a serine/threonine-rich APF protein from L. plantarum NCIMB 8826 resulted in increased production of EPS and enhanced the co-aggregation ability (82, 83). The aggregation phenotype enables Lactobacilli strains to colonize the GI tract, and to inhibit adhesion of pathogens by competitive exclusion or by co-aggregation with pathogens (62, 146).
Bacteriocins are a class of powerful small ribosomally synthesized antimicrobial peptides with bactericidal or bacteriostatic functions (147). Various types of bacteriocins were produced by Lactobacilli species, such as lactacin B from L. acidophilus and L. johnsonii, lactocin from L. casei, Lactocin 705 from L. casei, Lactoccin G from L. lactis and plantaricin from L. plantarum (148, 149).
Bacteriocins of probiotic Lactobacilli can mediate inhibitory effect against pathogens. Bacteriocin PJ4 produced by L. helveticus PJ4 isolated from rat gut microflora was active against enteric pathogen (88) and bacteriocin DT24 produced by vaginal L. brevis DT24 was antagonistic against uropathogenic E. coli (89). Pangsomboon et al. (150) reported that bacteriocins from L. paracasei were able to kill P. gingivalis. Moreover, the bacteriocin extracted from probiotic L. acidophilus KS40 was able to inhibit urogenital pathogens such as Gardnerella vaginalis, Streptococcus agalactiae, and Pseudomonas aeruginosa (90). Reuterin produced by L. reuteri (6) exerted antimicrobial effects by modifying thiol groups and inducing oxidative stress in bacterial cells (151). L. salivarius UCC118, a probiotic strain of human origin, produced bacteriocin Abp118, which mediated the inhibitory effect of the probiotic against Listeria monocytogenes infection in mice (91). Additionally, purified bacteriocins from different Lactobacillus species have shown anti-infective functions in mice models, demonstrating that bacteriocins can be a promising alternative against gastrointestinal infections (152).
Besides the antimicrobial effects, bacteriocins produced by Lactobacillus may also affect host immunity. Plantaricin was identified as the factor in L. plantarum WCFS1 that modulate the immune response of DCs (84). Notably, plantaricin can be produced during L. plantarum WCFS1 colonization in mice, thus supporting the function of this bacteriocin under in vivo conditions (85). Phagocytosis activities of macrophage were improved by bacteriocins isolated from L. acidophilus (87). Moreover, bacteriocins can affect the immune function of the host by selectively competing with specific bacterial strains and shaping the microbiota composition (9, 86).
Small molecules differ from the above mentioned paraprobiotics and protein/peptide postbiotics in that they do not have strain-specific differences in the biochemical characteristics and therefore are generally not responsible for strain-specificity of probiotic functionality. Moreover, different from protein/protein postbiotics, they can be produced by strategies independent of the probiotic strains. However, subsets of the probiotic effects are mediated by small molecules. Therefore, in this review, we also categorized small molecules as postbiotics, and summarized their beneficial effects.
SCFAs are produced by gut microbiota from indigestible food components such as fiber, oligosaccharides and polysaccharides via different metabolism channels (153, 154). The SCFAs have a wide range of positive effects on the host, such as providing energy sources for colonic epithelium cells (155), maintaining metabolic homeostasis (156), regulating T regulatory cells (157, 158), and anti-inflammatory effects (159–162). Generally they are essential for the health and well-being of the host when present in sufficient amounts (163). Studies showed that Lactobacillus strains can produce different types of SCFAs. L. rhamnosus GG and L. gasseri PA 16/8 produce propionate (163, 164). Moreover, SCFAs have been associated with the beneficial effects of probiotic Lactobacillus strains in some research. Dhaliwal et al. (165) confirmed that supplementation of mice with L. plantarum showed an increase in acetate and butyrate levels and reduced intestinal permeability and monoamine oxidases in the brain. SCFAs-promoting probiotic L. johnsonii L531 treatment have been shown to control Salmonella infection and maintaining metabolic homeostasis in pig (166). In a screening of LAB to reduce cholesterol levels, the strain of L. plantarum CECT 7529, which produced higher quantities of propionic and butyric acids, showed excellent properties for reducing cholesterol levels (167). Furthermore, probiotic strains L. salivarius FP25 and FP35, and L. reuteri NCIMB exhibited inhibitory effect on colon cancer cell proliferation, which was mediated by the production of SCFAs (168, 169).
Studies showed that many Lactobacillus species are able to synthesize conjugated linoleic acids (CLAs) (170, 171). The ability of L. rhamnosus PL60 to produce cis-9, tra-11 and tra-10, cis-12-CLA in humans was the first report indicating that probiotic bacteria produce CLA (172). Further studies showed that some Lactobacilli species isolated from GI tract of human and animals, including L. rhamnosus, L. acidophilus and L. plantarum, are CLA producers (173, 174).
CLA inhibited the growth of HT-29 and Caco-2 cancer cell lines in vitro (175). Proliferation of MDAMB-231 cells was inhibited by L. plantarum-produced CLA in a dose dependent manner (176). In vivo administration of CLA to rats could decrease the occurrence of colonic tumors and increase the apoptotic indices (177). Moreover, CLA has been shown to reduce the incidence of colonic, skin, mammary, and prostate carcinogenesis in animal models (178).
CLAs produced by probiotic Lactobacillus have remarkable anti-tumor effect. CLA inhibited the growth of HT-29 and Caco-2 cancer cell lines in vitro (175). Proliferation of MDAMB-231 cells was inhibited by L. plantarum-produced CLA in a dose dependent manner (176). In vivo administration of CLA to rats could decrease the occurrence of colonic tumors and increase the apoptotic indices (177). Moreover, CLA has been shown to reduce the incidence of colonic, skin, mammary, and prostate carcinogenesis in animal models (178).
Gut bacteria contribute to the proper function of gut-brain axis by producing neurotransmitters, such as γ-aminobutyric acid (GABA), glutamate, serotonin (5-HT), dopamine (DA), norepinephrine, histamine and acetylcholine (179). Particularly, Lactobacillus can produce multiple neurotransmitters, such as GABA (180–186), serotonin (181), catecholamines (181), dopamine (181), and acetylcholine (187). Different probiotic Lactobacillus strains have been reported to confer beneficial effects on mental health, acting as “psychobiotics,” including L. paracasei (188), L. helveticus (189, 190), L. plantarum (165, 191), and L. rhamnosus (192). Moreover, studies have shown that histamine and dopamine produced by gut commensal Lactobacillus imparted significant role in sleep related disorders and regulates neuronal signaling in depression, anxiety related conditions disease (193), suggesting that the beneficial effects of probiotic Lactobacillus on mental health might be attributable to the neurotransmitters production.
The beneficial effects of paraprobiotics or postbiotics are mediated through an interaction between the microbial products and host. Probiotic Lactobacilli possess conserved MAMPs, including peptidoglycan, LTA, S-layer protein A (SlpA), EPS, and genomic DNA, which can be recognized by pattern recognition receptors (PRRs), induce downstream signaling cascades that confer the beneficial functions (5).
The importance of Toll-like receptors (TLRs) and Nucleotide-binding oligomerization domain-like receptors (NLRs) in mediating differential host interaction with paraprobiotics and probiotics has been widely acknowledged (107, 194). In this review we summarize four types of the PRRs that play principal roles in the regulation of the host's immune response and these different types of PRRs can bind to specific paraprobiotics or postbiotics of Lactobacillus strains (Figure 2).
Figure 2. Interactions of the MAMP of Lactobacillus with PRRs of the epithelial and immune cells of the host. Probiotic Lactobacillus possess conserved microbe-associated molecular patterns (MAMPs), including peptidoglycan, lipoteichoic acids (LTA), S-layer protein A (SlpA), exopolysaccharides (EPS), and genomic DNA which can be recognized by certain pattern recognition receptors (PRRs). Peptidoglycan and LTA interact with TLR2. Moreover, specific components of peptidoglycan, such as meso-DAP and MDP, are recognized by NOD1 and NOD2, respectively. The EPSs of L. delbrueckii TUA4408L, act as TLR2 and TLR4 ligands to exert anti-inflammatory activities by inhibiting the production of IL-6, IL-8, and MCP-1. On the apical side of IECs, CpG-DNA stimulated TLR9 interacts with MYD88 and the inhibitor of NF-κB kinase (IKK) complexes, which may induced IL-10 expression. Binding of SlpA to the DC-SIGN (dendritic cell-specificICAM3-grabbing non integrin) receptor can induce IL-10 production in DCs and development of T cells. IEC, intestinal epithelial cell; DC, dendritic cell; Treg, T regulatory cell; Th, T helper cell; MCP-1, monocyte chemoattranctant protein-1.
TLRs recognize distinct families of MAMPs. For instance, TLR2 recognizes LTA and peptidoglycan; TLR2/TLR4 recognize bacterial EPS with the help of RP105/DM1; TLR9 is responsive to unmethylated CpG oligonucleotide (CpG-ODN) (195) (Table 2). L. reuteri DSM 17938 strain showed a positive effect against necrotizing enterocolitis via TLR2 (203). TLR2 recognized the LTA of L. plantarum, and attenuated Pam2CSK4-induced IL-8 expression (46).
The EPS of L. delbrueckii TUA4408L can act as TLR2 and TLR4 ligands, and exert anti-inflammatory activities in porcine IECs by modulating MAPK and NF-κB signaling pathways (197). L. plantarum N14 EPS reduced inflammation in intestinal epithelial cells depending on RP105/MD1 complex (a member of TLR family). (101). Similarly, L. rhamnosus GG and its components (surface layer protein and EPS) inhibited MAPK and NFκB signaling and alleviated LPS-induced inflammatory cytokines in porcine intestinal epithelial cells by modulating TLR expressions (204).
NLRs constitute a large family of PRRs and includes a number of subfamilies, which can be distinguished depending on the N-terminal effector domains (195). Two well-studied NLR proteins are NOD1 and NOD2. The NOD1 recognizes molecules containing D-Glu-mDAP (205), whereas NOD2 are vital for the regulation of NAM-D-Ala-D-Glu unit of the molecules (206). Recognition of muropeptide from Lactobacilli by NOD2 can induce anti-inflammatory properties and protect mice from colitis development (94). Different types of signaling molecules from Lactobacilli species including the fragments of peptidoglycan were sensed by NODs (207), and this sensing results in the activation of NF-κB and antimicrobial activity (208).
CTLRs recognize carbohydrates molecules, through one or more carbohydrate recognition domains (CRDs) (209). The sugar moieties found in the glycan backbone of the bacterial peptidoglycan bind CTLRs (210). After the ligand recognition, specialized CTLRs trigger or inhibit wide ranges of signaling pathways, thus modulate diverse immune responses (211).
DC-specific ICAM-3-grabbing nonintegrin (DC-SIGN) is a CLR expressed mainly on dendritic cells (DCs) and recognizes mannose- and fucose-containing glycans that are present on many species of Lactobacilli bacterial cell surfaces. DC-SIGN was previously shown to bind L. acidophilus SlpA in vitro (200). SlpA-DC-SIGN interaction induced IL-10 production in DCs promoted of T cells that secrete high amounts of IL-4, thereby decreasing the Th1/Th2 ratio (200). Further, in vivo role of the SlpA-induced protective immune regulation was demonstrated (212).
The best characterized GPCRs are GPR41 and GPR43, which are highly expressed by epithelial cells, adipocytes, enteroendocrine cells and the cells of the sympathetic nervous system (213), and are mainly activated by SCFAs (214). Butyrate and propionate produced by microbiota in the gut acted with GPR43 and regulated the accumulation of Foxp3+ Treg cells (215). The recognition of SCFAs by GPR109A has also been reported. For instance, activation of the GPR109A receptor by butyrate induced the differentiation of regulatory and IL-10-producing T cells, which suppressed colonic inflammation and carcinogenesis by promoting anti-inflammatory properties in colonic macrophages and dendritic cells (216). Furthermore, SCFAs produced by gut microbiota may regulate lipid metabolism, glucose homeostasis and insulin sensitivity through GPCR signaling (156).
Paraprobiotics and postbiotics derived from Lactobacillus species consist of a wide range of effector molecules. These products and byproducts of probiotic Lactobacillus have been found to possess magnificent beneficial functions including preservation of epithelial barrier, anti-tumor effect, immunomodulation, and antagonistic effects against pathogens. Furthermore, they have various advantages compared with probiotics, including clear chemical structures and safety dose parameters, as well as longer shelf life (217, 218). Therefore, the use of paraprobiotics and postbiotics may represent a valid and safer alternative to live probiotic bacteria, and have exhibited good potential to replace probiotics (219, 220).
The mechanisms underlying the beneficial effects have been less known, especially the signaling pathways downstream the interaction of paraprobiotics/postbiotics and PRRs, which deserve more investigation. Furthermore, the structure-activity relationship (SAR) of paraprobiotics and postbiotics will be an interesting topic, which may guide the functional improvement of these probiotic components, by either chemical or biological strategies.
Currently the application of postbiotics and paraprobiotics in human food, animal feed and pharmaceutical industries is increasing and several paraprobiotic and postbiotics products derived from Lactobacill species are commercially available for prevention or treatment of some diseases (221–225). Nevertheless, more evidence is needed to validate the beneficial effects of paraprobiotics and postbiotics. Current advancement of molecular technologies such as multi-omics have been promoting the identification of more paraprobiotics and postbiotics from probiotic Lactobacillus strains. Moreover, novel probiotics from other family or phylum are being discovered and studied, such as commensal bacterium isolated from the intestine of both human and animals (226, 227). The techniques and experience of paraprobiotics and postbiotics discovery from probiotic Lactobacilli may guide the investigation of novel functional components derived from the new probiotics. Collectively, paraprobiotics and postbiotics have good potential as prophylatctic or therapeutic agents as well as functional food or feed additives for human or animal use.
All authors listed have made a substantial, direct and intellectual contribution to the work, and approved it for publication.
This work was supported by the National Science and Technology Major Project of the Ministry of Science and Technology of China (2016ZX08011-005), National Key R&D Program of China (2018YFD0900400), National Natural Science Foundation of China (31925038, 31972807).
The authors declare that the research was conducted in the absence of any commercial or financial relationships that could be construed as a potential conflict of interest.
1. Rossi F, Amadoro C, Colavita G. Members of the Lactobacillus genus complex (LGC) as opportunistic pathogens : a review. Microorganisms. (2019) 7:126. doi: 10.3390/microorganisms7050126
2. Chiba M, Itabashi T, Hirai K, Sakamoto M, Ohkuma M, Ishige T, et al. Lactobacillus metriopterae sp. Nov, a novel lactic acid bacterium isolated from the gut of grasshopper metrioptera engelhardti. Int J Syst Evol Microbiol. (2018) 68:1484–9. doi: 10.1099/ijsem.0.002694
3. Afouda P, Fournier PE, Raoult D, Merhej V. ‘Lactobacillus timonensis' sp. nov, a new bacterial species isolated from the human gut. N Microbes N Infect. (2017) 19:121–2. doi: 10.1016/j.nmni.2017.07.001
4. Bron PA, Tomita S, Mercenier A, Kleerebezem M. Cell surface-associated compounds of probiotic lactobacilli sustain the strain-specificity dogma. Curr Opin Microbiol. (2013) 16:262–9. doi: 10.1016/j.mib.2013.06.001
5. Bron PA, Tomita S, van Swam II, Remus DM, Meijerink M, Wels M, et al. Lactobacillus plantarum possesses the capability for wall teichoic acid backbone alditol switching. Microb Cell Fact. (2012) 11:123. doi: 10.1186/1475-2859-11-123
6. Cicenia A, Scirocco A, Carabotti M, Pallotta L, Marignani M, Severi C. Postbiotic activities of Lactobacilli-derived factors. J Clin Gastroenterol. (2014) 48:S18–22. doi: 10.1097/MCG.0000000000000231
7. Kim KW, Kang SS, Woo SJ, Park OJ, Ahn KB, Song KD, et al. Lipoteichoic acid of probiotic Lactobacillus plantarum attenuates poly I: C-induced IL-8 production in porcine intestinal epithelial cells. Front Microbiol. (2017) 8:1827. doi: 10.3389/fmicb.2017.01827
8. Liu Z, Zhang Z, Qiu L, Zhang F, Xu X, Wei H, et al. Characterization and bioactivities of the exopolysaccharide from a probiotic strain of Lactobacillus plantarum WLPL04. J Dairy Sci. (2017) 100:6895–905. doi: 10.3168/jds.2016-11944
9. Aguilar-Toalá JE, Garcia-Varela R, Garcia HS, Mata-Haro V, González-Córdova AF, Vallejo-Cordoba B, et al. Postbiotics: an evolving term within the functional foods field. Trends Food Sci Technol. (2018) 75:105–14. doi: 10.1016/j.tifs.2018.03.009
10. Shenderov BA. Metabiotics: novel idea or natural development of probiotic conception. Microb Ecol Heal Dis. (2013) 24:1–8. doi: 10.3402/mehd.v24i0.20399
11. De Marco S, Sichetti M, Muradyan D, Piccioni M, Traina G, Pagiotti R, et al. Probiotic cell-free supernatants exhibited anti-inflammatory and antioxidant activity on human gut epithelial cells and macrophages stimulated with LPS. Evid Based Complement Altern Med. (2018) 2018:1756308. doi: 10.1155/2018/1756308
13. Doron S, Snydman DR. Risk and safety of probiotics. Clin Infect Dis. (2015) 60:S129–34. doi: 10.1093/cid/civ085
14. Deshpande G, Athalye-Jape G, Patole S. Para-probiotics for preterm neonates—The next frontier. Nutrients. (2018) 10:871. doi: 10.3390/nu10070871
15. Taverniti V, Guglielmetti S. The immunomodulatory properties of probiotic microorganisms beyond their viability (ghost probiotics : proposal of paraprobiotic concept). Genes Nutr. (2011) 6:261–74. doi: 10.1007/s12263-011-0218-x
16. Posadas GA, Broadway PR, Thornton JA, Carroll JA, Lawrence A, Corley JR, et al. Yeast pro- and paraprobiotics have the capability to bind pathogenic bacteria associated with animal disease. Transl Anim Sci. (2012) 1:60–8. doi: 10.2527/tas2016.0007
17. Tsilingiri K, Rescigno M. Postbiotics: what else? Benef Microbes. (2013) 4:101–7. doi: 10.3920/BM2012.0046
18. Lee MJ, Zang ZL, Choi EY, Shin HK, Ji GE. Cytoskeleton reorganization and cytokine production of macrophages by Bifidobacterial cells and cell-free extracts. J. Microbiol. Biotechnol. (2002) 12:398–405.
19. Chiu YH, Lu YC, Ou CC, Lin SL, Tsai CC, Huang CT, et al. Lactobacillus plantarum MYL26 induces endotoxin tolerance phenotype in Caco-2 cells. BMC Microbiol. (2013) 13:190. doi: 10.1186/1471-2180-13-190
20. Kim HG, Lee SY, Kim NR, Lee HY, Ko MY, Jung BJ. Lactobacillus plantarum lipoteichoic acid down-regulated Shigella flexneri peptidoglycan-induced inflammation. Mol Immunol. (2011) 48:382–91. doi: 10.1016/j.molimm.2010.07.011
21. Kamiya T, Wang L, Forsythe P, Goettsche G, Mao Y, Wang Y, et al. Inhibitory effects of Lactobacillus reuteri on visceral pain induced by colorectal distension in sprague-dawley rats. Gut. (2006) 55:191–6. doi: 10.1136/gut.2005.070987
22. Dinic M, Lukic J, Djokic J, Milenkovic M, Strahinic I, Golic N, et al. Lactobacillus fermentum postbiotic-induced autophagy as potential approach for treatment of acetaminophen hepatotoxicity. Front Microbiol. (2017) 8:594. doi: 10.3389/fmicb.2017.00594
23. Amaretti A, di Nunzio M, Pompei A, Raimondi S, Rossi M, Bordoni A. Antioxidant properties of potentially probiotic bacteria: in vitro and in vivo activities. Appl Microbiol Biotechnol. (2013) 97:809–17. doi: 10.1007/s00253-012-4241-7
24. Nakamura F, Ishida Y, Sawada D, Ashida N, Sakai M, Goto T, et al. Fragmented lactic acid bacterial cells activate peroxisome proliferator- activated receptors and ameliorate dyslipidemia in obese mice. J Agric Food Chem. (2016) 64:1249–59. doi: 10.1021/acs.jafc.5b05827
25. Tiptiri-Kourpeti A, Spyridopoulou K, Santarmaki V, Aindelis G, Tompoulidou E, Lamprianidou EE, et al. Lactobacillus casei exerts anti-proliferative effects accompanied by apoptotic cell death and up-regulation of trail in colon carcinoma cells. PLoS ONE. (2016) 11:e0147960. doi: 10.1371/journal.pone.0147960
26. Aguilar-Toalá JE, Hall FG, Urbizo-Reyes UC, Garcia HS, Vallejo-Cordoba B, GonzálezCórdova AF, et al. In silico prediction and in vitro assessment of multifunctional properties of postbiotics obtained from two probiotic bacteria. Probiot Antimicrobial Proteins. (2019) 12:608–22. doi: 10.1007/s12602-019-09568-z
27. de Almada CN, Almada CN, Martinez RCR, Sant'Ana AS. Paraprobiotics: evidences on their ability to modify biological responses, inactivation methods and perspectives on their application in foods. Trends Food Sci Technol. (2016) 58:96–114. doi: 10.1016/j.tifs.2016.09.011
28. Vidal K, Donnet-Hugues A, Granato D. Lipoteichoic acids from Lactobacillus johnsonii strain La1 and Lactobacillus acidophilus strain La10 antagonize the responsiveness of human intestinal epithelial HT29 cells to lipopolysaccharide and gramnegative bacteria. Infect Immun. (2002) 70:2057–64. doi: 10.1128/IAI.70.4.2057-2064.2002
29. Matsuguchi T, Takagi A, Matsuzaki T, Nagaoka M, Ishikawa K, Yokokura T, et al. Lipoteichoic acids from Lactobacillus strains elicit strong tumor necrosis factor alpha-inducing activities in macrophages through Toll-like receptor 2. Clin Diagn Lab Immunol. (2003) 10:259–66. doi: 10.1128/CDLI.10.2.259-266.2003
30. Zhang S, Liu L, Su Y, Li H, Sun Q, Liang X. Antioxidative activity of lactic acid bacteria in yogurt. Afr J Microbiol Res. (2011) 5:5194–201. doi: 10.5897/AJMR11.997
31. Cuevas-González PF, Liceaga AM, Aguilar-Toalá JE. Postbiotics and paraprobiotics: from concepts to applications. Food Res Int. (2020) 136:109502. doi: 10.1016/j.foodres.2020.109502
32. Shenderov BA, Sinitsa AV, Zakharchenko MM, Lang C. Cellular metabiotics and metabolite metabiotics. In: Shenderov BA, Sinitsa AV, Zakharchenko MM, Lang C, editors. Metabiotics: Present State, Challenges and Perspectives. Cham: Springer (2020). p. 63–75. doi: 10.1007/978-3-030-34167-1_14
33. Barros CP, Guimaraes JT, Esmerino EA, Duarte MCKH, Silva MC, Silva R, et al. Paraprobiotics and postbiotics: concepts and potential applications in dairy products. Curr Opin Food Sci. (2020) 32:1–8. doi: 10.1016/j.cofs.2019.12.003
34. Lebeer S, Bron PA, Marco ML, Van Pijkeren JP, O'Connell Motherway M, Hill C, et al. Identification of probiotic effector molecules: present state and future perspectives. Curr Opin Biotechnol. (2018) 49:217–23. doi: 10.1016/j.copbio.2017.10.007
35. Shenderov BA. Probiotic (symbiotic) bacterial languages. Anaerobe. (2011) 17:490–5. doi: 10.1016/j.anaerobe.2011.05.009
36. Linares DM, Gómez C, Renes E, Fresno JM, Tornadijo ME, Ross RP, et al. Lactic acid bacteria and bifidobacteria with potential to design natural biofunctional health-promoting dairy foods. Front Microbiol. (2017) 8:846. doi: 10.3389/fmicb.2017.00846
37. Silva CCG, Silva SPM, Ribeiro SC. Application of bacteriocins and protective cultures in dairy food preservation. Front Microbiol. (2018) 9:594. doi: 10.3389/fmicb.2018.00594
38. Shin HS, Park SY, Lee DK, Kim SA, An HM, Kim JR, et al. Hypocholesterolemic effect of sonication-killed Bifidobacterium longum isolated from healthy adult Koreans in high cholesterol fed rats. Arch Pharm Res. (2010) 33:1425–31. doi: 10.1007/s12272-010-0917-7
39. Kolling Y, Salva S, Villena J, Alvarez S. Are the immunomodulatory properties of Lactobacillus rhamnosus CRL1505 peptidoglycan common for all Lactobacilli during respiratory infection in malnourished mice? PLoS ONE. (2018) 13:e0194034. doi: 10.1371/journal.pone.0194034
40. Gorska S, Hermanova P, Ciekot J, Schwarzer M, Srutkova D, Brzozowska E, et al. Chemical characterization and immunomodulatory properties of polysaccharides isolated from probiotic Lactobacillus casei LOCK 0919. Glycobiology. (2016) 26:1014–24. doi: 10.1093/glycob/cww047
41. Piqué N, Berlanga M, Miñana-Galbis D. Health benefits of heat-killed (Tyndallized) probiotics: an overview. Int J Mol Sci. (2019) 20:1–30. doi: 10.3390/ijms20102534
42. Slattery C, Cotter PD, O'Toole PW. Analysis of health benefits conferred by Lactobacillus species from kefir. Nutrients. (2019) 11:1–24. doi: 10.3390/nu11061252
43. Castro-Bravo N, Wells JM, Margolles A, Ruas-Madiedo P. Interactions of surface exopolysaccharides from Bifidobacterium and Lactobacillus within the intestinal environment. Front Microbiol. (2018) 9:2426. doi: 10.3389/fmicb.2018.02426
44. Acosta MP, Geoghegan EM, Lepenies B, Ruzal S, Kielian M, Martinez MG. Surface (S) Layer proteins of Lactobacillus acidophilus block virus infection via DC-SIGN interaction. Front Microbiol. (2019) 10:810. doi: 10.3389/fmicb.2019.00810
45. Siciliano RA, Lippolis R, Mazzeo MF. Proteomics for the investigation of surface-exposed proteins in probiotics. Front Nutr. (2019) 6:52. doi: 10.3389/fnut.2019.00052
46. Lebeer S, Claes I, Tytgat HLP, Verhoeven TLA, Marien E, von Ossowski I, et al. Functional analysis of Lactobacillus rhamnosus GG pili in relation to adhesion and immunomodulatory interactions with intestinal epithelial cells. Appl Environ Microbiol. (2012) 78:185–93. doi: 10.1128/AEM.06192-11
47. Ganguli K, Collado MC, Rautava J, Lu L, Satokari R, Von Ossowski I, et al. Lactobacillus rhamnosus GG and its SpaC pilus adhesin modulate inflammatory responsiveness and TLR-related gene expression in the fetal human gut. Pediatr Res. (2015) 77:528–35. doi: 10.1038/pr.2015.5
48. Vargas García CE, Petrova M, Claes IJJ, De Boeck I, Verhoeven TLA, Dilissen E, et al. Piliation of Lactobacillus rhamnosus GG promotes adhesion, phagocytosis, and cytokine modulation in macrophages. Appl Environ Microbiol. (2015) 81:2050–62. doi: 10.1128/AEM.03949-14
49. Hiramatsu Y, Satho T, Hyakutake M, Irie K, Mishima K, Miake F, et al. The anti-inflammatory effects of a high-frequency oligodeoxynucleotide from the genomic DNA of lactobacillus casei. Int Immunopharmacol. (2014) 23:139–47. doi: 10.1016/j.intimp.2014.08.013
50. Zvanych R, Lukenda N, Kim JJ, Li X, Petrof EO, Khan WI, et al. Small molecule immunomodulins from cultures of the human microbiome member Lactobacillus plantarum. J Antibiot. (2014) 67:85–8. doi: 10.1038/ja.2013.126
51. Al-Hassi HO, Mann ER, Sanchez B, English NR, Peake STC, Landy J, et al. Altered human gut dendritic cell properties in ulcerative colitis are reversed by Lactobacillus plantarum extracellular encrypted peptide STp. Mol Nutr Food Res. (2014) 58:1132–43. doi: 10.1002/mnfr.201300596
52. Maldonado Galdeano C, Cazorla SI, Lemme Dumit JM, Vélez E, Perdigón G. Beneficial effects of probiotic consumption on the immune system. Ann Nutr Metab. (2019) 74:115–24. doi: 10.1159/000496426
53. De Giani A, Bovio F, Forcella M, Fusi P, Sello G, Di Gennaro P. Identification of a bacteriocin-like compound from Lactobacillus plantarum with antimicrobial activity and effects on normal and cancerogenic human intestinal cells. AMB Express. (2019) 9:88. doi: 10.1186/s13568-019-0813-6
54. Mahdi LH, Mahdi NZ, Sajet RM, Auda IG, Mater HN, Zwain LAH, et al. Anticariogenic and antibiofilm of purified bacteriocin of Lactobacillus curvatus and immunomodulatory effect of L. curvatus in streptococcal bacteremia. Rev Med Microbiol. (2019) 30:1–10. doi: 10.1097/MRM.0000000000000150
55. Park J, Kim M, Kang SG, Jannasch AH, Cooper B, Patterson J, et al. Short-chain fatty acids induce both effector and regulatory T cells by suppression of histone deacetylases and regulation of the mTOR-S6K pathway. Mucosal Immunol. (2015) 8:80–93. doi: 10.1038/mi.2014.44
56. Moens F, Van den Abbeele P, Basit AW, Dodoo C, Chatterjee R, Smith B, et al. A four-strain probiotic exerts positive immunomodulatory effects by enhancing colonic butyrate production in vitro. Int J Pharm. (2019) 555:1–10. doi: 10.1016/j.ijpharm.2018.11.020
57. Zelante T, Iannitti RG, Cunha C, DeLuca A, Giovannini G, Pieraccini G, et al. Tryptophan catabolites from microbiota engage aryl hydrocarbon receptor and balance mucosal reactivity via interleukin-22. Immunity. (2013) 39:372–85. doi: 10.1016/j.immuni.2013.08.003
58. Vyboh K, Jenabian MA, Mehraj V, Routy JP. HIV and the gut microbiota, partners in crime: breaking the vicious cycle to unearth new therapeutic targets. J Immunol Res. (2015) 2015:614127. doi: 10.1155/2015/614127
59. Peng M, Tabashsum Z, Patel P, Bernhardt C, Biswas D. Linoleic acids overproducing Lactobacillus casei limits growth, survival, and virulence of Salmonella typhimurium and enterohaemorrhagic Escherichia coli. Front Microbiol. (2018) 9:2663. doi: 10.3389/fmicb.2018.02663
60. Li J, Wang W, Xu SX, Magarvey NA, McCormick JK. Lactobacillus reuteri-produced cyclic dipeptides quench agr-mediated expression of toxic shock syndrome toxin-1 in Staphylococci. Proc Natl Acad Sci USA. (2011) 108:3360–5. doi: 10.1073/pnas.1017431108
61. Spinler JK, Auchtung J, Brown A, Boonma P, Oezguen N, Ross CL, et al. Nextgeneration probiotics targeting Clostridium difficile through precursor-directed antimicrobial biosynthesis. Am Soc Microbiol. (2017) 85:e00303–17. doi: 10.1128/IAI.00303-17
62. do Carmo MS, Dos Santos CI, Araújo MC, Girón JA, Fernandes ES, Monteiro-Neto V. Probiotics, mechanisms of action, and clinical perspectives for diarrhea management in children. Food Funct. (2018) 9:5074–95. doi: 10.1039/C8FO00376A
63. Wang J, Zhao X, Yang Y, Zhao A, Yang Z. Characterization and bioactivities of an exopolysaccharide produced by Lactobacillus plantarum YW32. Int J Biol Macromol. (2015) 74:119–26. doi: 10.1016/j.ijbiomac.2014.12.006
64. Tukenmez U, Aktas B, Aslim B, Yavuz S. The relationship between the structural characteristics of Lactobacilli-EPS and its ability to induce apoptosis in colon cancer cells in vitro. Sci Rep. (2019) 9:8268. doi: 10.1038/s41598-019-44753-8
65. Ochoa JJ, Farquharson AJ, Grant I, Moffat L, Heys ED, Wahle KWJ. Conjugated linoleic acids (CLAs) decrease prostate cancer cell proliferation: different molecular mechanisms for cis−9, trans−11 and trans−10, cis−12 isomers. Carcinogenesis. (2004) 25:1185–91. doi: 10.1093/carcin/bgh116
66. Chen DJ, Yan LH, Li Q, Zhang C, Jiao Si CL, Li ZY, et al. Bioconversion of conjugated linoleic acid by Lactobacillus plantarum CGMCC8198 supplemented with acer truncatum bunge seeds oil. Food Sci. Biotechnol. (2017) 26:1595–611. doi: 10.1007/s10068-017-0218-8
67. Wang H, Cheng X, Zhang L, Xu S, Zhang Q, Lu R. A surface-layer protein from Lactobacillus acidophilus NCFM induces autophagic death in HCT116 cells requiring ROS-mediated modulation of mTOR and JNK signaling pathways. Food Funct. (2019) 10:4102–12. doi: 10.1039/C9FO00109C
68. Zhang T, Pan D, Yang Y, Jiang J, Zhang J, Zeng X. et al. Effect of Lactobacillus acidophilus CICC 6074 S-layer protein on colon cancer HT-29 cell proliferation and apoptosis. J Agric Food Chem. (2020) 68:2639–47. doi: 10.1021/acs.jafc.9b06909
69. Muñoz-Provencio D, Rodríguez-Díaz J, Collado MC, Langella P, Bermúdez-Humarán LG, Monedero V. Functional analysis of the Lactobacillus casei BL23 sortases. Appl Environ Microbiol. (2012) 78:8684–93. doi: 10.1128/AEM.02287-12
70. Zhang Y, Xiang X, Lu Q, Zhang L, Ma F, Wang L. Adhesions of extracellular surface-layer associated proteins in Lactobacillus M5-L and Q8-L. J Dairy Sci. (2016) 99:1011–8. doi: 10.3168/jds.2015-10020
71. Kankainen M, Paulin L, Tynkkynen S, Von Ossowski I, Reunanen J, Partanen P, et al. Comparative genomic analysis of Lactobacillus rhamnosus GG reveals pili containing a human-mucus binding protein. Proc Natl Acad Sci USA. (2009) 106:17193–8. doi: 10.1073/pnas.0908876106
72. Hemarajata P, Versalovic J. Effects of probiotics on gut microbiota: Mechanisms of intestinal immunomodulation and neuromodulation. Therap Adv Gastroenterol. (2013) 6:39–51. doi: 10.1177/1756283X12459294
73. Goh YJ, Klaenhammer TR. Functional roles of aggregation-promoting-like factor in stress tolerance and adherence of Lactobacillus acidophilus NCFM. Appl Environ Microbiol. (2010) 76:5005–12. doi: 10.1128/AEM.00030-10
74. Bäuerl C, Pérez-Martínez G, Yan F, Polk DB, Monedero V. Functional analysis of the p40 and p75 proteins from Lactobacillus casei BL23. J Mol Microbiol Biotechnol. (2011) 19:231–41. doi: 10.1159/000322233
75. Wang K, Li W, Rui X, Chen X, Jiang M, Dong M. Characterization of a novel exopolysaccharide with antitumor activity from Lactobacillus plantarum 70810. Int J Biol Macromol. (2014) 63:133–9. doi: 10.1016/j.ijbiomac.2013.10.036
76. Seth A, Yan F, Polk DB, Rao RK. Probiotics ameliorate the hydrogen peroxide-induced epithelial barrier disruption by a PKC- And MAP kinase-dependent mechanism. Am J Physiol Gastrointest Liver Physiol. (2008) 294:1060–70. doi: 10.1152/ajpgi.00202.2007
77. Yan F, Cao HW, Cover TL, Washington MK, Shi Y, Liu LS, et al. Colon-specific delivery of a probiotic-derived soluble protein ameliorates intestinal inflammation in mice through an egfr-dependent mechanism. J Clin Investig. (2011) 121:2242–53. doi: 10.1172/JCI44031
78. Yan F, Liu L, Dempsey PJ, Tsai YH, Raines EW, Wilson CL, et al. A Lactobacillus rhamnosus GG-derived soluble protein, p40, stimulates ligand release from intestinal epithelial cells to transcativate epidermal growth factor receptor. J Bio Chem. (2013) 288:30742–51. doi: 10.1074/jbc.M113.492397
79. Nishiyama K, Seto Y, Yoshioka K, Kakuda T, Takai S, Yamamoto Y, et al. Lactobacillus gasseri SBT2055 reduces infection by and colonization of Campylobacter jejuni. PLoS ONE. (2014) 9:e108827. doi: 10.1371/journal.pone.0108827
80. Phukan N, Brooks AES, Simoes-Barbosa A. A cell surface aggregation promoting factor from Lactobacillus gasseri contributes to inhibition of Trichomonas vaginalis adhesion to human vaginal ectocervical cells. Infect Immun. (2018) 86:e00907–17. doi: 10.1128/IAI.00907-17
81. Yungareva T, Urshev Z. The aggregation-promoting factor in Lactobacillus delbrueckii ssp. bulgaricus confirmation of the presence and expression of the apf gene and in silico analysis of the corresponding protein. World J Microbiol Biotechnol. (2018) 34:97. doi: 10.1007/s11274-018-2480-1
82. Hevia A, Martínez N, Ladero V, Álvarez MA, Margolles A, Sánchez B. An extracellular serine/threonine-rich protein from Lactobacillus plantarum NCIMB 8826 is a novel aggregation-promoting factor with affinity to mucin. Appl Environ Microbiol. (2013) 79:6059–66. doi: 10.1128/AEM.01657-13
83. He J, Ruan W, Sun J, Wang F, Yan W. Functional characterization of c-di-GMP signaling-related genes in the probiotic Lactobacillus acidophilus. Front Microbiol. (2018) 9:1935. doi: 10.3389/fmicb.2018.01935
84. Meijerink M, Van Hemert S, Taverne N, Wels M, de Vos P, Peter A. Identification of genetic loci in Lactobacillus plantarum that modulate the immune response of dendritic cells using comparative genome hybridization. PLoS ONE. (2010) 5:e10632. doi: 10.1371/journal.pone.0010632
85. Marco ML, Bongers RS, de Vos WM, Kleerebezem M. Spatial and temporal expression of Lactobacillus plantarum genes in the gastrointestinal tracts of mice. Appl Environ Microb. (2007) 73:124–32. doi: 10.1128/AEM.01475-06
86. Shaikh AM, Sreeja V. Metabiotics and their health benefits. Intl J Food Ferment. (2017) 6:11–23. doi: 10.5958/2321-712X.2017.00002.3
87. Herawati I, Hilmiprima D, Fauziah N. Effect of lactic acid filtrate and bacteriocins of Lactobacillus Acidophillus on phagocytosis activity of macrophages cell against Enteropathogenic Escherichia coli (EPEC). Microbiol. (2014) 8:183–90. doi: 10.5454/mi.8.4.6
88. Jena PK, Trivedi D, Chaudhary H, Kumar T. Bacteriocin PJ4 active against enteric pathogen produced by Lactobacillus helveticus PJ4 isolated from gut microflora of wistar rat (Rattus norvegicus): partial purification and characterization of bacteriocin. Appl Biochem Biotechnol. (2014) 169:2088–100. doi: 10.1007/s12010-012-0044-7
89. Trivedi D, Jena PK, Patel JK, Seshadri S. Partial purification and characterization of a bacteriocin DT24 produced by probiotic vaginal Lactobacillus brevis DT24 and determination of its anti- uropathogenic Escherichia coli potential. Probiot Antimicro Prot. (2013) 5:142–51. doi: 10.1007/s12602-013-9132-4
90. Gaspar C, Donders GG, Oliveira RP, De Queiroz JA, Tomaz C, De Oliveira JM. Bacteriocin production of the probiotic Lactobacillus acidophilus KS400. AMB Express. (2018) 8:153. doi: 10.1186/s13568-018-0679-z
91. Corr C, Li Y, Riedel CU, Toole PWO, Hill C, Gahan CGM. Bacteriocin production as a mechanism for the antiinfective activity of Lactobacillus salivarius UCC118. Proc Natl Acad Sci USA. (2007) 104:7617–21. doi: 10.1073/pnas.0700440104
92. Noh SY, Kang SS, Yun CH, Han SH. Lipoteichoic acid from Lactobacillus plantarum inhibits Pam2CSK4-induced IL-8 production in human intestinal epithelial cells. Mol Immunol. (2015) 64:183–9. doi: 10.1016/j.molimm.2014.11.014
93. Smelt MJ, de Haan BJ, Bron PA, van Swam I, Meijerink M, Wells JM, et al. The impact of Lactobacillus plantarum WCFS1 teichoic acid D-alanylation on the generation of effector and regulatory T-cells in healthy Mice. PLoS ONE. (2013) 8:e0063099. doi: 10.1371/journal.pone.0063099
94. Shida K, Kiyoshima-Shibata J, Kaji R, Nagaoka M, Nanno M. Peptidoglycan from Lactobacilli inhibits interleukin-12 production by macrophages induced by Lactobacillus casei through toll-like receptor 2-dependent and independent mechanisms. Immunology. (2009) 128:e858–69. doi: 10.1111/j.1365-2567.2009.03095.x
95. Fernandez EM, Valenti V, Rockel C, Hermann C, Pot B, Boneca IG, et al. Anti-inflammatory capacity of selected Lactobacilli in experimental colitis is driven by NOD2-mediated recognition of a specific peptidoglycan-derived muropeptide. Gut. (2011) 60:1050–9. doi: 10.1136/gut.2010.232918
96. Meng J, Zhu X, Gao SM, Zhang QX, Sun Z, Lu RR. Characterization of surface layer proteins and its role in probiotic properties of three Lactobacillus strains. Int J Biol Macromol. (2014) 65:110–4. doi: 10.1016/j.ijbiomac.2014.01.024
97. Chen X, Xu J, Shuai J, Chen J, Zhang Z, Fang W. The S-layer proteins of Lactobacillus crispatus strain ZJ001 is responsible for competitive exclusion against Escherichia coli O157:H7 and Salmonella typhimurium. Int J Food Microbiol. (2007) 115:307–12. doi: 10.1016/j.ijfoodmicro.2006.11.007
98. Johnson-henry KC, Hagen KE, Gordonpour M, Tompkins TA, Sherman PM. Surface-layer protein extracts from Lactobacillus helveticus inhibit enterohaemorrhagic Escherichia coli O157:H7 adhesion to epithelial cells. Cell Microbiol. (2007) 9:356–67. doi: 10.1111/j.1462-5822.2006.00791.x
99. Bleau C, Monges A, Rashidan K, Laverdure JP, Lacroix M, Van Calsteren MR, et al. Intermediate chains of exopolysaccharides from Lactobacillus rhamnosus RW-9595M increase IL-10 production by macrophages. J Appl Microbiol. (2010) 108:666–75. doi: 10.1111/j.1365-2672.2009.04450.x
100. Nikolic M, López P, Strahinic I, Suárez A, Kojic M, Fernández-García M, et al. Characterisation of the exopolysaccharide (EPS)-producing Lactobacillus plantarum BGCG11 and its non-EPS producing derivative strains as potential probiotics. Int J Food Microbiol. (2012) 158:155–62. doi: 10.1016/j.ijfoodmicro.2012.07.015
101. Murofushi Y, Villena J, Morie K, Kanmani P, Tohno M, Shimazu T, et al. The toll-like receptor family protein RP105/MD1 complex is involved in the immunoregulatory effect of exopolysaccharides from Lactobacillus plantarum N14. Mol Immunol. (2015) 64:63–75. doi: 10.1016/j.molimm.2014.10.027
102. Makino S, Sato A, Goto A, Nakamura M, Ogawa M, Chiba Y, et al. Enhanced natural killer cell activation by exopolysaccharides derived from yogurt fermented with Lactobacillus delbrueckii ssp. bulgaricus OLL1073R-1. J Dairy Sci. (2016) 99:915–23. doi: 10.3168/jds.2015-10376
103. El-Deeb NM, Yassin AM, Al-Madboly LA, El-Hawiet A. A novel purified Lactobacillus acidophilus 20079 exopolysaccharide, LA-EPS-20079, molecularly regulates both apoptotic and NF-kB inflammatory pathways in human colon cancer. Microb Cell Fact. (2018) 17:1–15. doi: 10.1186/s12934-018-0877-z
104. Di W, Zhang L, Yi H, Han X, Zhang Y, Xin L. Exopolysaccharides produced by Lactobacillus strains suppress HT - 29 cell growth via induction of G0 / G1 cell cycle arrest and apoptosis. Oncol Lett. (2018) 16:3577–86. doi: 10.3892/ol.2018.9129
105. Zhou X, Hong T, Yu Q, Nie S, Gong D, Xiong T, Xie M. Exopolysaccharides from Lactobacillus plantarum NCU116 induce c-Jun dependent Fas/Fasl-mediated apoptosis via TLR2 in mouse intestinal epithelial cancer cells. Sci. Rep. (2017) 7:14247. doi: 10.1038/s41598-017-14178-2
106. Deepak V, Ramachandran S, Balahmar RM, Pandian SRK, Sivasubramaniam SD, Nellaiah H, et al. In vitro evaluation of anticancer properties of exopolysaccharides from Lactobacillus acidophilus in colon cancer cell lines. Vitr Cell Dev Biol Anim. (2016) 52:163–73. doi: 10.1007/s11626-015-9970-3
107. Lebeer S, Vanderleyden J, De Keersmaecker SCJ. Host interactions of probiotic bacterial surface molecules: comparison with commensals and pathogens. Nat Rev Microbiol. (2010) 8:171–84. doi: 10.1038/nrmicro2297
108. Asong J, Wolfert MA, Maiti KK, Miller D, Boons GJ. Binding and cellular activation studies reveal that toll-like receptor 2 can differentially recognize peptidoglycan from gram-positive and gram-negative bacteria. J Biol Chem. (2009) 284:8643–53. doi: 10.1074/jbc.M806633200
109. Yadav AK, Espaillat A, Cava F. Bacterial strategies to preserve cell wall integrity against environmental threats. Front Microbiol. (2018) 9:2064. doi: 10.3389/fmicb.2018.02064
110. Kleerebezem M, Hols P, Bernard E, Rolain T, Zhou M, Siezen RJ, et al. The extracellular biology of the Lactobacilli. FEMS Microbiol Rev. (2010) 34:199–230. doi: 10.1111/j.1574-6976.2009.00208.x
111. Weidenmaier C, Peschel A. Teichoic acids and related cell-wall glycopolymers in gram-positive physiology and host interactions. Nat Rev Microbiol. (2008) 6:276–87. doi: 10.1038/nrmicro1861
112. Mohamadzadeh M, Pfeiler EA, Brown JB, Zadeh M, Gramarossa M, Managlia E, et al. Regulation of induced colonic inflammation by Lactobacillus acidophilus deficient in lipoteichoic acid. Proc Natl Acad Sci U S A. (2011) 108:4623–30. doi: 10.1073/pnas.1005066107
113. Kim MH, Choi SJ, Il Choi H, Choi JP, Park HK, Kim EK, et al. Lactobacillus plantarum-derived extracellular vesicles protect atopic dermatitis induced by Staphylococcus aureus-derived extracellular vesicles. Allergy Asthma Immunol Res. (2018) 10:516–32. doi: 10.4168/aair.2018.10.5.516
114. Makino S, Ikegami S, Kume A, Horiuchi H, Sasaki H, Orii N. Reducing the risk of infection in the elderly by dietary intake of yoghurt fermented with Lactobacillus delbrueckii ssp. bulgaricus OLL1073R-1. Br J Nutr. (2010) 104:998–1006 doi: 10.1017/S000711451000173X
115. Zhang W, Huang C, Yuan Y, Ma S. Catalytic transient leaving group for atom-economic synthesis of allenes from 2-alkynols. Chem Commun. (2017) 68:3–6. doi: 10.1039/C7CC06866B
116. Ghany KAEL, Elhafez EA, Hamouda RA, Mahrous H, Ahmed FAH, Hamza HA. Evaluation of antioxidant and antitumor activities of Lactobacillus acidophilus bacteria isolated from Egyptian infants. Int J Pharmacol. (2014) 10:282–8. doi: 10.3923/ijp.2014.282.288
117. Sungur T, Aslim B, Karaaslan C, Aktas B. Impact of Exopolysaccharides (EPSs) of Lactobacillus gasseri strains isolated from human vagina on cervical tumor cells (HeLa). Anaerobe. (2017) 47:137–44. doi: 10.1016/j.anaerobe.2017.05.013
118. Liu Q, Yu Z, Tian F, Zhao J, Zhang H, Zhai Q. Surface components and metabolites of probiotics for regulation of intestinal epithelial barrier. Microb Cell Fact. (2020) 19:23. doi: 10.1186/s12934-020-1289-4
119. Call EK, Klaenhammer TR. Relevance and application of sortase and sortase-dependent proteins in lactic acid bacteria. Front Microbiol. (2013) 4:73. doi: 10.3389/fmicb.2013.00073
120. Jensen H, Roos S, Jonsson H, Rud I, Grimmer S, van Pijkeren JP, et al. Role of Lactobacillus reuteri cell and mucusbinding protein A (CmbA) in adhesion to intestinal epithelial cells and mucus in vitro. Microbiol (United Kingdom). (2014) 160:671–81. doi: 10.1099/mic.0.073551-0
121. Sengupta R, Altermann E, Anderson RC, Mcnabb WC, Moughan PJ, Roy NC. The role of cell surface architecture of Lactobacilli in host-microbe interactions in the gastrointestinal tract. Mediators Inflamm. (2013) 2013:237921. doi: 10.1155/2013/237921
122. Zhang B, Zuo F, Yu R, Zeng Z, Ma H, Chen S. Comparative genome-based identification of a cell wall-anchored protein from Lactobacillus plantarum increases adhesion of Lactococcus lactis to human epithelial cells. Sci Rep. (2015) 5:14109. doi: 10.1038/srep14109
123. Boekhorst J, Wels M, Kleeberezem M, Siezen RJ. The predicted secretome of Lactobacillus plantarum WCFS1 sheds light on interactions with its environment. Microbiology. (2006) 152:3175–83. doi: 10.1099/mic.0.29217-0
124. Fagan RP, Fairweather NF. Biogenesis and functions of bacterial S-layers. Nat Rev Microbiol. (2014) 12:211–22. doi: 10.1038/nrmicro3213
125. Malamud M, Carasi P, Freire T, de los Angels Serradell M. S-layer glycoprotein from Lactobacillus kefiri CIDCA 8348 enhances macrophages response to LPS in a Ca+2-dependent manner. Biochem Biophys Res Commun. (2018) 495:1227–32. doi: 10.1016/j.bbrc.2017.11.127
126. Prado Acosta M, Ruzal SM, Cordo SM. S-layer proteins from Lactobacillus sp. inhibit bacterial infection by blockage of DC-SIGN cell receptor. Int J Biol Macromol. (2016) 92:998–1005. doi: 10.1016/j.ijbiomac.2016.07.096
127. Malamud M, Carasi P, Assandri MH, Freire T, Lepenies B, De Los Ángeles Serradell M. S-Layer glycoprotein from Lactobacillus kefiri exerts its immunostimulatory activity through glycan recognition by mincle. Front Immunol. (2019) 10:1422. doi: 10.3389/fimmu.2019.01422
128. Zhang YC, Zhang LW, Tuo YF, Guo CF, Yi HX, Li JY, et al. Inhibition of Shigella sonnei adherence to HT-29 cells by Lactobacilli from Chinese fermented food and preliminary characterization of S-layer protein involvement. Res Microbiol. (2010) 161:667–72. doi: 10.1016/j.resmic.2010.06.005
129. Danne C, Dramsi S. Pili of gram-positive bacteria: roles in host colonization. Res Microbiol. (2012) 163:645–58. doi: 10.1016/j.resmic.2012.10.012
130. Reunanen J, von Ossowski I, Hendrickx APA, Palva A, de Vosa WM. Characterization of the SpaCBA pilus fibers in the probiotic Lactobacillus rhamnosus GG. Appl Environ Microbiol. (2012) 78:2337–44. doi: 10.1128/AEM.07047-11
131. Troge A, Scheppach W, Schroeder BO, Rund SA, Heuner K, Wehkamp J, et al. More than a marine propeller - the flagellum of the probiotic Escherichia coli strain Nissle 1917 is the major adhesin mediating binding to human mucus. Int J Med Microbiol. (2012) 302:304–14. doi: 10.1016/j.ijmm.2012.09.004
132. Tytgat HLP, Reunanen J, Rasinkangas P, Hendrickx APA, Laine PK, Paulin L, et al. Lactobacillus rhamnosus GG outcompetes Enterococcus faecium via mucus-binding pili: evidence for a novel and heterospecific probiotic mechanism. Appl Environ Microbiol. (2016) 82:5756–62. doi: 10.1128/AEM.01243-16
133. Ardita CS, Mercante JW, Kwon YM, Luo L, Crawford ME, Powell DN, et al. Epithelial adhesion mediated by pilin SpaC is required for Lactobacillus rhamnosus GG-induced cellular responses. Appl Environ Microbiol. (2014) 80:5068–77. doi: 10.1128/AEM.01039-14
134. Ayala FJ. Gene sharing and evolution: the diversity of protein functions by jorampiatigorsky 2007 harvard university press. FASEB J. (2009) 23:2022–3. doi: 10.1096/fj.09-0703
135. Kainulainen V, Loimaranta V, Pekkala A, Edelman S, Antikainen J, Kylväjä R, et al. Glutamine synthetase and glucose-6-phosphate isomerase are adhesive moonlighting proteins of Lactobacillus crispatus released by epithelial cathelicidin LL-37. J Bacteriol. (2012) 194:2509–19. doi: 10.1128/JB.06704-11
136. Wang G, Xia Y, Cui J, Gu Z, Song Y, Chen YQ, et al. The roles of moonlighting proteins in bacteria. Curr Issues Mol Biol. (2014) 16:15–22.
137. Waśko A, Polak-Berecka M, Paduch R, Józwiak K. The effect of moonlighting proteins on the adhesion and aggregation ability of Lactobacillus helveticus. Anaerobe. (2014) 30:161–8. doi: 10.1016/j.anaerobe.2014.10.002
138. Jeffery CJ. What is protein moonlighting and why is it important? Moonlighting proteins Nov Virulence Factors. Bact Infect. (2016) 1–19. doi: 10.3389/fgene.2015.00211
139. Saad N, Urdaci M, Vignoles C, Chaignepain S, Tallon R, Schmitter JM, et al. Lactobacillus plantarum 299v surface-bound GAPDH: a new insight into enzyme cell walls location. J Microbiol Biotechnol. (2009) 19:1635–43. doi: 10.4014/jmb.0902.0102
140. Spurbeck RR, Arvidson CG. Lactobacillus jensenii surface-associated proteins inhibit Neisseria gonorrhoeae adherence to epithelial cells. Infect Immun. (2010) 78:3103–11. doi: 10.1128/IAI.01200-09
141. Zhang WM, Wang HF, Gao K, Wang C, Liu L, Liu JX. Lactobacillus reuteri glyceraldehyde-3-phosphate dehydrogenase functions in adhesion to intestinal epithelial cells. Can J Microbiol. (2015) 61:373–80. doi: 10.1139/cjm-2014-0734
142. Patel DK, Shah KR, Pappachan A, Gupta S, Singh DD. Cloning, expression and characterization of a mucin-binding GAPDH from Lactobacillus acidophilus. Int J Biol Macromol. (2016) 91:338–46. doi: 10.1016/j.ijbiomac.2016.04.041
143. Kainulainen V, Korhonen TK. Dancing to another tune-adhesive moonlighting proteins in bacteria. Biology (Basel). (2014) 3:178–204. doi: 10.3390/biology3010178
144. Hurmalainen V, Edelman S, Antikainen J, Baumann M, Lähteenmäki K, Korhonen TK. Extracellular proteins of Lactobacillus crispatus enhance activation of human plasminogen. Microbiology. (2007) 153:1112–22. doi: 10.1099/mic.0.2006/000901-0
145. Bäuerl C, Abitayeva G, Sosa-Carrillo S, Mencher-Beltrán A, Navarro-Lleó N, Coll-Marqués JM, et al. P40 and P75 are singular functional muramidases present in the Lactobacillus casei/paracasei/rhamnosus taxon. Front Microbiol. (2019) 10:1420. doi: 10.3389/fmicb.2019.01420
146. Górska S, Sandstro C, Wojas-Turek J, Ro J. Structural and immunomodulatory differences among Lactobacilli exopolysaccharides isolated from intestines of mice with experimentally induced inflammatory bowel disease. Nat Publ Gr. (2016) 6:37613. doi: 10.1038/srep37613
147. Perez RH, Zendo T, Sonomoto K. Novel bacteriocins from lactic acid bacteria (LAB): various structures and applications. Microb Cell Fact. (2014) 13:1–13. doi: 10.1186/1475-2859-13-S1-S3
148. Nielsen DS, Cho GS, Hanak A, Huch M, Franz CMAP, Arneborg N. The effect of bacteriocin-producing Lactobacillus plantarum strains on the intracellular pH of sessile and planktonic listeria monocytogenes single cells. Int J Food Microbiol. (2010) 141:S53–59. doi: 10.1016/j.ijfoodmicro.2010.03.040
149. Zacharof MP, Lovitt RW. Bacteriocins produced by lactic acid bacteria A. Rev Art APCBEE Proc. (2012) 2:50–6. doi: 10.1016/j.apcbee.2012.06.010
150. Pangsomboon K, Kaewnopparat S, Pitakpornpreecha T, Srichana T. Antibacterial activity of a bacteriocin from Lactobacillus paracasei HL32 against Porphyromonas gingivalis. Arch Oral Biol. (2006) 51:784–93. doi: 10.1016/j.archoralbio.2006.03.008
151. Schaefer L, Auchtung TA, Hermans KE, Whitehead D, Borhan B, Britton RA. The antimicrobial compound reuterin (3- hydroxypropionaldehyde) induces oxidative stress via interaction with thiol groups. Microbiology. (2010) 156:1589–99. doi: 10.1099/mic.0.035642-0
152. Dobson A, Cotter PD, Ross RP, Hill C. Bacteriocin production : Amazon.com probiotic trait? Appl Environ Microbiol. (2012) 78:1–6. doi: 10.1128/AEM.05576-11
153. Layden BT, Angueira AR, Brodsky M, Durai V, Lowe WL Jr. Short chain fatty acids and their receptors: new metabolic targets. Transl Res. (2012) 161:131–40. doi: 10.1016/j.trsl.2012.10.007
154. Tan J, Mckenzie C, Potamitis M, Thorburn AN, Mackay CR, Macia L. The role of short-chain fatty acids in health and disease. Adv Immunol. (2014) 121:91–119. doi: 10.1016/B978-0-12-800100-4.00003-9
155. Pascale A, Marchesi N, Marelli C, Coppola A, Luzi L, Govoni S, et al. Microbiota and metabolic diseases. Endocrine. (2018) 61:357–71. doi: 10.1007/s12020-018-1605-5
156. Canfora EE, Jocken JW, Blaak EE. Short-chain fatty acids in control of body. Nat Publ Gr. (2015) 11:577–91. doi: 10.1038/nrendo.2015.128
157. Smith PM, Howitt MR, Panikov N, Michaud M, Gallini CA, Bohlooly M, et al. The microbial metabolites, short-chain fatty acids, regulate colonic Treg cell homeostasis. Science. (2013) 341:569–73. doi: 10.1126/science.1241165
158. Furusawa Y, Yuuki Obata Y, Shinji Fukuda S, Takaho A, Endo TA, Nakato G, Takahashi D, et al. Commensal microbe-derived butyrate induces the differentiation of colonic regulatory T cells. Nature. (2013) 504:446–50. doi: 10.1038/nature12721
159. Roelofsen H, Priebe MG, Vonk RJ. The interaction of short-chain fatty acids with adipose tissue: relevance for prevention of type 2 diabetes. Beneficial Microbes. (2010) 1:433–7. doi: 10.3920/BM2010.0028
160. D'Souza WN, Douangpanya J, Mu S, Jaeckel P, Zhang M, Maxwell JR, et al. Differing roles for short chain fatty acids and GPR43 agonism in the regulation of intestinal barrier function and immune responses. PLoS ONE. (2017) 12:e0180190. doi: 10.1371/journal.pone.0180190
161. Ferrer-Picón E, Dotti I, Corraliza AM, Mayorgas A, Esteller M, Perales JC, et al. Intestinal inflammation modulates the epithelial response to butyrate in patients with in?ammatory bowel disease. Inflamm Bowel Dis. (2019) 26:43–55. doi: 10.1093/ibd/izz119
162. Sun M, Wu W, Chen L, Yang W, Huang X, Ma C, et al. Microbiota-derived short-chain fatty acids promote Th1 cell IL-10 production to maintain intestinal homeostasis. Nat Commun. (2018) 9:3555. doi: 10.1038/s41467-018-05901-2
163. Leblanc JG, Chain F, Martín R, Humarán LGB, Courau S, Langella P. Beneficial effects on host energy metabolism of short - chain fatty acids and vitamins produced by commensal and probiotic bacteria. Microb Cell Fact. (2017) 16:79. doi: 10.1186/s12934-017-0691-z
164. Sivieri K, Morales MLV, Adorno MAT, Sakamoto IK, Saad SMI, Rossi EA. Lactobacillus acidophilus CRL 1014 improved “gut health” in the SHIME® reactor. BMC Gastroenterol. (2013) 13:100. doi: 10.1186/1471-230X-13-100
165. Dhaliwal J, Singh DP, Singh S, Pinnaka AK, Boparai K, Bishnoi M, et al. Lactobacillus plantarum MTCC 9510 supplementation protects from chronic unpredictable and sleep deprivation-induced behaviour, biochemical and selected gut microbial aberrations in mice. J Appl Microbiol. (2018) 125:257–69. doi: 10.1111/jam.13765
166. He T, Zhu Y, Yu J, Xia B, Liu X, Yang G, et al. Lactobacillus johnsonii L531 reduces pathogen load and helps maintain short- chain fatty acid levels in the intestines of pigs challenged with Salmonella enterica infantis. Vet Microbiol. (2019) 230:187–94. doi: 10.1016/j.vetmic.2019.02.003
167. Bosch M, Fuentes MC, Audivert S, Bonachera MA, Peiró S, Cuñé J. Lactobacillus plantarum CECT 7527, 7528 and 7529: probiotic candidates to reduce cholesterol levels. J Sci Food Agric. (2014) 94:803–9. doi: 10.1002/jsfa.6467
168. Thirabunyanon M, Hongwittayakorn P. Potential probiotic lactic acid bacteria of human origin induce antiproliferation of colon cancer cells via synergic actions in adhesion to cancer cells and short-chain fatty acid bioproduction. Appl Biochem Biotechnol. (2013) 169:511–25. doi: 10.1007/s12010-012-9995-y
169. Kahouli I, Malhotra M, Saha S, Marinescu D, Ls R, Ma A, et al. Screening and in-vitro analysis of Lactobacillus reuteri strains for short chain fatty acids production, stability and therapeutic potentials in colorectal cancer. Bioequivalence Bioavailabil. (2015) 7:39–50. doi: 10.4172/jbb.1000212
170. Dahiya DK, Puniya AK. Isolation, molecular characterization and screening of indigenous Lactobacilli for their abilities to produce bioactive conjugated linoleic acid (CLA). J Food Sci Technol. (2017) 54:792–801. doi: 10.1007/s13197-017-2523-x
171. Dahiya DK, Puniya AK. Optimisation of fermentation variables for conjugated linoleic acid bioconversion by Lactobacillus fermentum DDHI27 in modified skim milk. Int J Dairy Technol. (2018) 71:46–55. doi: 10.1111/1471-0307.12375
172. Lee K, Lee Y. Production of c9,t11- and t10,c12-conjugated linoleic acids in humans by Lactobacillus rhamnosus PL60. J Microbiol Biotechnol. (2009) 19:1617–9. doi: 10.4014/jmb.0907.07010
173. Nieuwenhove CP, Van Cano PG, Pe AB, Gonza SN. Effect of functional buffalo cheese on fatty acid profile and oxidative status of liver and intestine of mice. J Med Food. (2011) 14:420–7. doi: 10.1089/jmf.2010.0061
174. Ribeiro SC, Stanton C, Yang B, Ross RP, Silva CCG. Conjugated linoleic acid production and probiotic assessment of Lactobacillus plantarum isolated from pico cheese. LWT-Food Sci Technol. (2018) 90:403–11. doi: 10.1016/j.lwt.2017.12.065
175. Soel SM, Choi OS, Bang MH, Yoon Park JH, Kim WK. Influence of conjugated linoleic acid isomers on the metastasis of colon cancer cells in vitro and in vivo. J Nutr Biochem. (2007) 18:650–7. doi: 10.1016/j.jnutbio.2006.10.011
176. Kadirareddy RH, GhantaVemuri S, Devi Palempalli UM. Probiotic conjugated linoleic acid mediated apoptosis in breast cancer cells by downregulation of NF-κB. Asian Pac J Cancer Prev. (2016) 17:3395–403. doi: 10.14456/apjcp.2016.107/APJCP.2016.17.7.3395
177. Kim KH, Park HS. Dietary supplementation of conjugated linoleic acid reduces colon tumor incidence in DMH-treated rats by increasing apoptosis with modulation of biomarkers. Nutrition. (2003) 19:772–7. doi: 10.1016/S0899-9007(03)00098-4
178. Belury MA. Inhibition of carcinogenesis by conjugated linoleic acid : potential mechanisms of action. J Nutr. (2002) 132:2995–8. doi: 10.1093/jn/131.10.2995
179. Oleskin AV, El GI, Shenderov BA. Role of neuromediators in the functioning of the human microbiota : “Business Talks” among microorganisms and the microbiota-host dialogue. Microbiology. (2016) 85:1–22. doi: 10.1134/S0026261716010082
180. Siragusa S, De Angelis M, Di Cagno R, Rizzello CG, Coda R, Gobbetti M. Synthesis of γ-aminobutyric acid by lactic acid bacteria isolated from a variety of Italian cheeses. Appl Environ Microbiol. (2007) 73:7283–90. doi: 10.1128/AEM.01064-07
181. Oleskin AV, Zhilenkova OG, Shenderov BA, Amerhanova AM, Kudrin VS, Klodt PM. Lactic-acid bacteria supplement fermented dairy products with human behavior-modifying neuroactive compounds. J Pharm Nutr Sci. (2014) 4:199–206. doi: 10.6000/1927-5951.2014.04.03.5
182. Franciosi E, Carafa I, Nardin T, Schiavon S, Poznanski E, Cavazza A, et al. Biodiversity and γ -aminobutyric acid production by lactic acid bacteria isolated from traditional alpine raw cow's milk cheeses. Biomed Res Int. (2015) 2015:625740. doi: 10.1155/2015/625740
183. Wu Q, Shah NP. Gas release-based prescreening combined with reversed-phase HPLC quantitation for efficient selection of high-γ-aminobutyric acid (GABA)-producing lactic acid bacteria. J Dairy Sci. (2015) 98:790–7. doi: 10.3168/jds.2014-8808
184. Zhao A, Hu X, Pan L, Wang X. Isolation and characterization of a gamma-aminobutyric acid producing strain Lactobacillus buchneri WPZ001 that could efficiently utilize xylose and corncob hydrolysate. Appl Microbiol Biotechnol. (2015) 99:3191–200. doi: 10.1007/s00253-014-6294-2
185. Villegas JM, Brown L, Savoy de Giori G, Hebert EM. Optimization of batch culture conditions for GABA production by Lactobacillus brevis CRL 1942, isolated from quinoa sourdough. LWT Food Sci Technol. (2016) 67:22–26. doi: 10.1016/j.lwt.2015.11.027
186. Pokusaeva K, Johnson C, Luk B, Uribe G, Fu Y, Oezguen N, et al. GABA-producing Bifidobacterium dentium modulates visceral sensitivity in the intestine. Neurogastroenterol Motil. (2017) 29:1–14. doi: 10.1111/nmo.12904
187. Stanaszek PM, Snell JF, Neill JJO. Isolation, extraction, and measurement of acetylcholine from Lactobacillus plantarum. Appl Envron Microbiol. (1977) 34:237–9. doi: 10.1128/AEM.34.2.237-239.1977
188. Wei CL, Wang S, Yen JT, Cheng YF, Liao CL, Hsu CC, et al. Antidepressant-like activities of live and heat-killed Lactobacillus paracasei PS23 in chronic corticosterone-treated mice and possible mechanisms. Brain Res. (2019) 1711:202–13. doi: 10.1016/j.brainres.2019.01.025
189. Liang S, Wang T, Hu X, Luo J, Li W, Wu X, et al. Administration of Lactobacillus helveticus NS8 improves behavioral, congnitive, and biochemical aberrations caused by chronic restraint stress. Neuroscience. (2015) 310:561–77. doi: 10.1016/j.neuroscience.2015.09.033
190. Maehata H, Kobayashi Y, Mitsuyama E, Kawase T, Kuhara T, Xiao JZ, et al. Heat-killed Lactobacillus helveticus strain MCC1848 confers resilience to anxiety or depression-like symptoms caused by subchronic social defeat stress in mice. Biosci Biotechnol Biochem. (2019) 83:1239–47. doi: 10.1080/09168451.2019.1591263
191. Liu Y, Liu W, Wu C, Juan Y, Wu Y, Tsai H, et al. Psychotropic effects of Lactobacillus plantarum PS128 in early life-stressed and naïve adult mice. Brain Res. (2016) 1631:1–12. doi: 10.1016/j.brainres.2015.11.018
192. Bravo JA, Forsythe P, Chew MV, Escaravage E, Savignac HM. Ingestion of Lactobacillus strain regulates emotional behavior and central GABA receptor expression in a mouse via the vagus nerve. Proc Natl Acad Sci U S A. (2011) 108:16050–5. doi: 10.1073/pnas.1102999108
193. Alkasir R, Li J, Li X, Jin M, Zhu B. Human gut microbiota : the links with dementia development. Protein Cell. (2016) 8:90–102. doi: 10.1007/s13238-016-0338-6
194. Li W, Ji J, Chen X, Jiang M, Rui X, Dong M. Structural elucidation and antioxidant activities of exopolysaccharides from Lactobacillus helveticus MB2-1. Carbohydr Polym. (2014) 102:351–9. doi: 10.1016/j.carbpol.2013.11.053
195. Yin Q, Fu T-M, Li J, Wu H. Structural biology of innate immunity. Annu Rev Immunol. (2015) 33:393–416. doi: 10.1146/annurev-immunol-032414-112258
196. Oliveira-Nascimento L, Massari P, Wetzler LM. The role of TLR2 ininfection and immunity. Front Immunol. (2012) 3:79. doi: 10.3389/fimmu.2012.00079
197. Wachi S, Kanmani P, Tomosada Y, Kobayashi H, Yuri T, Egusa S, et al. Lactobacillus delbrueckii TUA4408L and its extracellular polysaccharides attenuate enterotoxigenic Escherichia coli-induced inflammatory response in porcine intestinal epitheliocytes via Toll-like receptor-2 and 4. Mol Nutr Food Res. (2014) 58:2080–93. doi: 10.1002/mnfr.201400218
198. Wang H, Gao K, Wen K, Allen IC, Li G, Zhang W, et al. Lactobacillus rhamnosus GG modulates innate signaling pathway and cytokine responses to rotavirus vaccine in intestinal mononuclear cells of gnotobiotic pigs transplanted with human gut microbiota. BMC Microbiol. (2016) 16:109. doi: 10.1186/s12866-016-0727-2
199. Gao C, Ganesh BP, Shi Z, Shah RR, Fultz R, Major A, et al. Gut microbe–mediated suppression of inflammation-associated colon carcinogenesis by luminal histamine production. Am J Pathol. (2017) 187:2323–36. doi: 10.1016/j.ajpath.2017.06.011
200. Konstantinov SR, Smidt H, De Vos WM, Bruijns SCM, Singh SK, Valence F, et al. S layer protein A of Lactobacillus acidophilus NCFM regulates immature dendritic cell and T cell functions. Proc Natl Acad Sci USA. (2008) 105:19474–9. doi: 10.1073/pnas.0810305105
201. Conterno L, Fava F, Viola R, Tuohy KM. Obesity and the gut microbiota: does up-regulating colonic fermentation protect against obesity and metabolic disease? Genes Nutr. (2011) 6:241–60. doi: 10.1007/s12263-011-0230-1
202. Morrison DJ, Preston T, Morrison DJ, Preston T. Formation of short chain fatty acids by the gut microbiota and their impact on human metabolism. Gut Microbes. (2016) 7:189–200. doi: 10.1080/19490976.2015.1134082
203. Hoang TK, He B, Wang T, Tran DQ, Rhoads JM, Liu Y. Protective effect of Lactobacillus reuteri DSM 17938 against experimental necrotizing enterocolitis is mediated by toll-like receptor 2. Am J Physiol Gastrointest Liver Physiol. (2018) 315:G231–40. doi: 10.1152/ajpgi.00084.2017
204. Gao K, Wang C, Liu L, Dou X, Liu J, Yuan L, et al. Immunomodulation and signaling mechanism of Lactobacillus rhamnosus GG and its components on porcine intestinal epithelial cells stimulated by lipopolysaccharide. J Microbiol Immunol Infect. (2017) 50:700–13. doi: 10.1016/j.jmii.2015.05.002
205. Girardin SE, Boneca IG, Carneiro LAM, Antignac A, Jéhanno M, Viala J, et al. Nod1 detects a unique muropeptide from gram-negative bacterial peptidoglycan. Science. (2003) 300:15847. doi: 10.1126/science.1084677
206. Dagil YA, Arbatsky NP, Alkhazova BI, L'vov VL, Mazurov DV, Pashenkov MV. The dual NOD1/NOD2 agonism of muropeptides containing a meso-diaminopimelic acid residue. PLoS ONE. (2016) 11:e0160784. doi: 10.1371/journal.pone.0160784
207. Keestra-gounder AM, Tsolis RM. NOD1 and NOD2 : beyond peptidoglycan sensing. Trends Immunol. (2017) 38:758–67. doi: 10.1016/j.it.2017.07.004
208. Franchi L, Warner N, Viani K, Nuñez G. Function of Nod-like receptors in microbial recognition and host defense. Immunol Rev. (2009) 227:106–28. doi: 10.1111/j.1600-065X.2008.00734.x
209. Hoving JC, Wilson GJ, Brown GD. Signalling C-type lectin receptors, microbial recognition and immunity. Cell Microbiol. (2014) 16:185–94. doi: 10.1111/cmi.12249
210. Plato A, Willment JA, Brown GD. C-Type lectin-like receptors of the dectin-1 cluster: ligands and signaling pathways. Int Rev Immunol. (2013) 32:134–56. doi: 10.3109/08830185.2013.777065
211. Mayer S, Raulf MK, Lepenies B. C-type lectins: their network and roles in pathogen recognition and immunity. Histochem Cell Biol. (2017) 147:223–37. doi: 10.1007/s00418-016-1523-7
212. Lightfoot YL, Selle K, Yang T, Goh YJ, Sahay B, Zadeh M, et al. SIGNR 3 -dependent immune regulation by Lactobacillus acidophilus surface layer protein A in colitis. EMBO J. (2015) 34:881–95. doi: 10.15252/embj.201490296
213. Vinolo MAR, Rodrigues HG, Nachbar RT, Curi R. Regulation of inflammation by short chain fatty acids. Nutrients. (2011) 3:858–76. doi: 10.3390/nu3100858
214. Brown AJ, Goldsworthy SM, Barnes AA, Eilert MM, Tcheang L, Daniels D, et al. The orphan G protein-coupled receptors GPR41 and GPR43 are activated by propionate and other short chain carboxylic acids. J Biol Chem. (2003) 278:11312–9. doi: 10.1074/jbc.M211609200
215. Frei R, Ferstl R, Konieczna P, Ziegler M, Simon T, Rugeles TM, et al. Histamine receptor 2 modifies dendritic cell responses to microbial ligands. J Allergy Clin Immunol. (2013) 132:194–204.e12. doi: 10.1016/j.jaci.2013.01.013
216. Singh N, Gurav A, Sivaprakasam S, Brady E, Padia R, Shi H, et al. Activation of Gpr109a, receptor for niacin and the commensal metabolite butyrate, suppresses colonic inflammation and carcinogenesis. Immunity. (2014) 40:128–39. doi: 10.1016/j.immuni.2013.12.007
217. Shigwedha N, Sichel L, Jia L, Zhang L. Probiotical cell fragments (PCFs) as “novel nutraceutical ingredients”. J Biosci Med. (2014) 2:43–55. doi: 10.4236/jbm.2014.23007
218. Tomar SK, Anand S, Sharma P, Sangwan V, Mandal S. Role of probiotic, prebiotics, synbiotics and postbiotics in inhibition of pathogens. In: Méndez-Vilas A, editor. The Battle Against Microbial Pathogens: Basic Science, Technological Advances and Educational Programs. Formatex Research Center (2015). p. 717–32.
219. Haileselassie Y, Navis M, Vu N, Qazi KR, Rethi B, Sverremark-Ekstrom E. Postbiotic modulation of retinoic acid imprinted mucosal-like dendritic cells by probiotic Lactobacillus reuteri 17938 in vitro. Front Immunol. (2016) 7:96. doi: 10.3389/fimmu.2016.00096
220. Vieira AT, Fukumori C, Ferreira CM. New insights into therapeutic strategies for gut microbiota modulation in inflammatory diseases. Clin Transl Immunol. (2016) 5:e87. doi: 10.1038/cti.2016.38
221. Liévin-Le Moal V, Sarrazin-Davila L, Servin A. An experimental study and a randomized, double-blind, placebo-controlled clinical trial to evaluate the antisecretory activity of lactobacillus acidophilus strain LB against nonrotavirus diarrhea. Pediatrics. (2007) 120:e795–e803. doi: 10.1542/peds.2006-2930
222. Mehling H, Busjahn A. Non-viable Lactobacillus reuteri DSMZ 17648 (Pylopass) as a new approach to Helicobacter pylori control in humans. Nutrients. (2013) 5:3062–73. doi: 10.3390/nu5083062
223. West R, Roberts E, Sichel L, Sichel J. Improvements in gastrointestinal symptoms among children with autism spectrum disorder receiving the Delpro® probiotic and immunomodulator formulation. J Probiot Health. (2013) 1. doi: 10.4172/jph.1000102
224. Buckley M, Lacey S, Doolan A, Goodbody E, Seamans K. The effect of Lactobacillus reuteri supplementation in Helicobacter pylori infection: a placebo-controlled, single-blind study. BMC Nutrition. (2018) 4:48. doi: 10.1186/s40795-018-0257-4
225. Patil S, Sawant S, Hauff K, Hampp G. Validated postbiotic screening confirms presence of physiologically-active metabolites, such as short-chain fatty acids, amino acids and vitamins in Hylak® Forte. Probiot Antimicrobial Proteins. (2019) 11:1124–31. doi: 10.1007/s12602-018-9497-5
226. Ramírez C, Coronado J, Silva A, Romero J. Cetobacterium is a major component of the microbiome of giant amazonian fish (Arapaima gigas) in ecuador. Animals. (2018) 8:189. doi: 10.3390/ani8110189
Keywords: paraprobiotics, postbiotics, Lactobacilli, metabolites, immunomodulatory effect
Citation: Teame T, Wang A, Xie M, Zhang Z, Yang Y, Ding Q, Gao C, Olsen RE, Ran C and Zhou Z (2020) Paraprobiotics and Postbiotics of Probiotic Lactobacilli, Their Positive Effects on the Host and Action Mechanisms: A Review. Front. Nutr. 7:570344. doi: 10.3389/fnut.2020.570344
Received: 12 June 2020; Accepted: 28 August 2020;
Published: 22 October 2020.
Edited by:
Haruki Kitazawa, Tohoku University, JapanReviewed by:
Muhammad Shahid Riaz Rajoka, Shenzhen University, ChinaCopyright © 2020 Teame, Wang, Xie, Zhang, Yang, Ding, Gao, Olsen, Ran and Zhou. This is an open-access article distributed under the terms of the Creative Commons Attribution License (CC BY). The use, distribution or reproduction in other forums is permitted, provided the original author(s) and the copyright owner(s) are credited and that the original publication in this journal is cited, in accordance with accepted academic practice. No use, distribution or reproduction is permitted which does not comply with these terms.
*Correspondence: Zhigang Zhou, emhvdXpoaWdhbmcwM0BjYWFzLmNu; Chao Ran, cmFuY2hhb0BjYWFzLmNu
†These authors have contributed equally to this work
Disclaimer: All claims expressed in this article are solely those of the authors and do not necessarily represent those of their affiliated organizations, or those of the publisher, the editors and the reviewers. Any product that may be evaluated in this article or claim that may be made by its manufacturer is not guaranteed or endorsed by the publisher.
Research integrity at Frontiers
Learn more about the work of our research integrity team to safeguard the quality of each article we publish.