- 1Laboratory of Molecular and Functional Neurobiology, Department of Pharmacology, Institute of Biomedical Sciences, University of São Paulo, São Paulo, Brazil
- 2Laboratory of Molecular Neuropharmacology, Department of Pharmacology, Institute of Biomedical Sciences, University of São Paulo, São Paulo, Brazil
The aging process is characterized by a series of molecular and cellular changes over the years that could culminate in the deterioration of physiological parameters important to keeping an organism alive and healthy. Physical exercise, defined as planned, structured and repetitive physical activity, has been an important force to alter physiology and brain development during the process of human beings' evolution. Among several aspects of aging, the aim of this review is to discuss the balance between two vital cellular processes such as autophagy and apoptosis, based on the fact that physical exercise as a non-pharmacological strategy seems to rescue the imbalance between autophagy and apoptosis during aging. Therefore, the effects of different types or modalities of physical exercise in humans and animals, and the benefits of each of them on aging, will be discussed as a possible preventive strategy against neuronal death.
Aging
The aging process is characterized by a series of molecular and cellular changes over the years that could culminate in the deterioration of physiological parameters important to keeping an organism alive and healthy. This widespread loss of body function, or loss of fitness, is extremely variable and can result in increased individual vulnerability, the onset of various illnesses, and death (1, 2). At the cellular level, aging is characterized by aggregation and accumulation of misfolded proteins and disabled organelles in a progressive way that may lead to cell homeostasis interruptions. Therefore, progressive degeneration may occur, increasing the risk of cell death [reviewed by (1, 3)].
In the Central Nervous System (CNS) normal aging is accompanied by alterations in brain structure such as white matter atrophy (4, 5), and functional and cognitive decline. It is still unclear what relationship the cognitive and functional dysfunctions have with the decrease in neurogenesis observed during aging (6). Age-related cognitive decline can reduce the quality of an individual's life and is related to an increased risk of neurodegenerative diseases (7, 8).
Neurogenesis consists in the generation of new neurons from neural stem cells and progenitor cells that reside in germinal niches in the subgranular zone (SGZ) of the hippocampal dentate gyrus and in the subventricular zone (SVZ) of the lateral ventricle (9). In relation to humans, neurogenesis is still a highly controversial topic, because of the inherent difficulty in marking neurogenic niches and newborn cells in vivo (10). Notwithstanding, a recent study raised the debate about the existence of neurogenesis in the human brain and its meaning. Analyzing 37 postmortem and 22 intraoperative tissue samples from human hippocampus, Sorrells et al. concluded that, different to other species, dentate gyrus (DG) proliferating progenitor cells and newborn neurons in humans decline during childhood without being detected in adult brain samples, suggesting a decline in neurogenesis during life (11). Besides, some studies suggest that neurogenesis happens daily in human DG (12–14), whereas others find a decrease in neurogenesis with just a few neurons being generated in adults (15, 16). Indeed, in 2019 new studies supported the evidence of hippocampal neurogenesis in adult humans, with great individual variability (17) up to the ninth decade of life in healthy subjects (18) and also in patients with mild cognitive decline and Alzheimer's Disease (17).
In animal models, physical exercise has been related to increased hippocampal neurogenesis (19, 20) which is reduced in old rodents (21), whereas in humans it is still speculative (22). However, in humans of all ages, physical exercise is related to improved memory function (23–25), as well as reduction in brain atrophy observed during aging in humans (26) and rodents (27). In the face of such an interesting approach against age-related cognitive decline, the focus of this review is to present and discuss some cellular mechanisms by which different kinds of physical exercise can unleash possible benefits in the CNS in human and animal models.
Physical Exercise and Aging
Physical exercise was defined by Caspersen as a planned, structured and repetitive physical activity done with the objective of improving or promoting physical fitness (28). Exercise has been an important force of evolution for the human species in order to hunt for food sources, adapt to the environment and, in consequence, to alter physiology and development of the brain, displaying co-evolution of neuroplasticity signaling pathways (29–32).
The benefits of physical exercise can be observed throughout different stages of life. During pregnancy, supervised moderate exercise attenuates prenatal depression (33). It is also associated with a shorter first stage of labor (34), and newborns whose mothers exercised during pregnancy presented a better auditory memory response related to sound differentiation (35). Thus, an active lifestyle during pregnancy should be encouraged and promoted by public health policies (36). Among adolescents, physical activity may have beneficial effects on attention capacity and cognitive functions (37, 38), and is likely to be effective in reducing depression symptoms amongst both adolescents and young adults (39–42). Meanwhile, different modes of exercise are investigated for the older population, including stretching exercise, such as Pilates (43, 44) and Tai Chi Chuan (45, 46), resistance exercise (26, 47), multimodal exercise (48–50) and aerobic exercise (51). These modes of exercising seem to be similarly effective regarding cognitive improvement (23), but such improvement may not be seen in a short period of time (52).
Some studies and public organs recommend 150 min of moderate physical exercise per week to be sufficient for beneficial outcomes (53, 54). However, few elderly people accomplish the recommendation, especially of moderate to vigorous physical exercise; some believe physical exercise may be potentially harmful or even unnecessary [reviewed by (55)]. A review of nine cohort studies indicated that lower doses of the recommended physical exercise may reduce mortality risk by 22% (56), indicating that, even though it is believed that it is necessary to reach the suggested amount of activity, there is also the need to investigate whether light intensity exercise could ameliorate health or function and motivate the practice of more intense exercise (57).
Among the various interventions that affect aging, physical exercise seems to be the main ally in the prevention of aging-related diseases (58).
Studies regarding the effects of physical activity on elderly people also extend to several types of aging-related disorders, comprising dementia (59), late-life depression (60, 61), frailty syndrome (62, 63), Parkinson's Disease (64) and Alzheimer's Disease (65), through evaluation of epigenetic changes [see (66, 67) for review]. However, studies about the effects of physical exercise on elderly people's epigenetics are still emerging. Lavratti et al. conducted one of the first human studies to demonstrate the relationship between physical exercise and levels of global histone acetylation in schizophrenic patients (68). A meta-analysis on elderly people supports the protective effects of physical activity, a healthy diet and higher educational levels (69); however, in 2018, Gale et al. investigated the effect of physical activity on the epigenetic clock [reviewed in (69)] and found no correlation between them, indicating that exercise alone might not be enough to exert a protective effect in this specific regard.
It has already been demonstrated in the literature that physical exercise can promote neuroprotection. For example, treadmill physical exercise carried out in a mouse model of Alzheimer's disease (69) and voluntary running physical exercise in elderly mice (70) demonstrated, among other effects, that running physical exercise decreased glia activation and amyloid-beta (Aβ) peptide levels, suggesting possible mechanisms for exercise-induced neuroprotection.
The literature brings some ways in which physical exercise promotes its neuroprotective effects. In mice, it has been shown that neuroprotection induced by resistance physical exercise occurs in combination with multiple synergistic neuroprotective pathways: increased neurogenesis, decreased loss of dopaminergic neurons, increased antioxidant capacity, and improved autophagy (70). A study carried out in rats suggested that aerobic physical exercise reverted the synaptic loss in the cortex and hippocampus in old rats, which may be related to the up-regulation of Rho-GTPases (a G protein family, which plays a fundamental role in synaptic morpho-functional changes) (71). In mouse model of Parkinson's disease induced by MPTP, endurance physical exercise promoted neuroprotection possibly due to its contribution to the improvement of mitochondria biogenesis and reduction of apoptosis (72), decrease of pro-inflammatory cytokines and α-synuclein protein (73). In ischemic brain injury rat model, aerobic physical exercise can contribute to neuroprotection by blocking glia activation and preventing neuronal death (74).
Given the data presented here, it is likely that physical exercise is able to promote neuroprotective effects, which seem to depend on the type of physical exercise performed.
In addition to that, in a more general view about the effects of physical exercise in aging, there are many studies regarding cerebrovascular function, gut microbiota, hormone release, sleep quality, and neurotrophic factors production. These topics are quite large and complex and are beyond the scope of this review, however we will briefly mention the main data to contextualize the reader.
Cerebral blood flow (CBF) is the main marker of cerebrovascular function and its decrease, mainly due to detriment of energy depletion or brain ischemia [reviewed by (75)], seems to be related to the generation of cognitive impairment and dementia (72). Disruption of neuronal environmental homeostasis through impaired CBF can be highly related to the decline of the cerebrovascular system during aging (73, 74). In patients with Alzheimer's disease, in addition to decreased brain volume, there is also a decrease in CBF, and it is a potential marker of the severity of the disease (76).
In humans, physical exercise can prevent cognitive impairment by enhancing cerebral vasomotor reactivity, increasing CBF, and consequently increasing cerebrovascular function in older adults (77, 78). Such an increase seems to be dependent on exercise intensity (79). In sedentary older men, aerobic physical exercise was able to increase CBF in the region responsible for regulating cognitive functions, part of this mediated by improvements in glucose metabolism (78). Moreover, another study involving sedentary older men verified the increase in brain function mediated by the regional CBF, increased cognition assessed by memory and executive functions, and the increase in cardiovascular fitness measured by VO2 max, after a protocol of 12-week exercise (80). Otherwise, a study with master athletes ranged in age between 50 and 80 demonstrated that the exercise cessation for a short period of time reduced CBF levels in hippocampus and gray matter regions (81).
In animals, physical exercise through treadmills or running wheels was able to improve endothelium-dependent vasorelaxation as well as increase CBF, reducing functional deficits and protecting the brain from cerebral ischemia and reperfusion (82).
Regarding the gut-brain axis, it is known that gut microbiota plays important roles on metabolic and immunological activities in humans (83). Infrequent bowel movements can decrease gut microbiota, which increases the risk of individuals developing colorectal cancer (84, 85). A combination of moderate physical exercise [≥ 7000 steps/day or 15 min/day at >3 METS (metabolic equivalents)] and lactobacillus ingestion has been shown to decrease infrequent bowel movements in elderly people aged 65–92 (86). In elderly humans, it was shown that 5-week endurance physical exercise was not able to significantly change gut microbiota diversity and composition (87). In the animal model, it has been shown that 11-month-old mice submitted to treadmill physical exercise for 7 months had their gut microbiota diversity augmented, suggesting that physical exercise is able to increase microbiota diversity during the aging process (88). However, Fielding et al. found that mice presented no changes either in their entire lean body mass or in treadmill endurance capacity when treated with human feces coming from elderly people who exercised. Therefore, data regarding physical exercise influence on gut microbiota along aging seems contradictory both in animals and humans, likely depending on duration, intensity and type of physical exercise (89).
It is well-established that growth hormone (GH) secretion decreases during aging process (90–93), which seems to be associated with changes in the organism such as loss of lean mass, gain of fat tissue, diminution in muscle strength, decline in cognitive function, among others [reviewed in (94)]. Physical exercise in the elderly has been described to influence GH level/activity; studies in humans, independently of gender, have demonstrated that regular physical exercise can increase GH levels in plasma (95, 96) or serum (97–103).
However, other studies have not shown an increase of GH levels in elderly marathon runners and sedentary controls (104), in middle-age men (40–50 years old) (105), in old men (47), in old women (106) and in old men and women. The comparison was conducted after subjects had been submitted to heavy resistance training (107), low volume resistance exercise (108), and low intensity physical exercise (109).
Furthermore, experiments done in 21-month-old rats showed that mild physical exercise in treadmill (8 m/min, 1 h/day) in combination with GH administration for 73 days, increased both muscle mass and strength compared with GH by itself (110). However, Marzetti et al. showed that short-term treadmill training attenuates age-related skeletal muscle apoptosis and the same effect was not observed with short-term administration of GH in older rats (111). Studies performed in old rats showed that exercise and GH reduced age-related decay in myocardial relaxation, avoiding diastolic dysfunction (112) and increasing bone strength (113, 114). It is known that GH can augment muscle mass in humans (92). In humans, administration of GH and testosterone together in elderly males produced a gain in lean mass and increased muscle strength, and consequently aerobic endurance (115). Another study about GH supplementation in elderly men did not observe increased muscle strength, and consequently no changes in resistance exercise (116).
Sleep disturbances are common features in older adults, such as sleepiness at daytime, fractionated sleep at night (117–119), among others. It has been shown in the elderly, both men and women, that low to moderate physical activity improved sleep quality (120–133). In animals, sleep derangement related to the aging process also happens (134, 135). It seems that regular moderate physical exercise ameliorates sleep architecture in old rats (136).
A great number of signaling pathways seem to be involved in physical exercise benefits, and one of them is brain-derived neurotrophic factor (BDNF) which is positively induced by physical exercise (137). BDNF is a protein that participates in neuronal proliferation and differentiation, synaptogenesis, synaptic function, plasticity, and neuroendocrine actions [see Review (138)]. In 1995, Neeper et al. measured BDNF mRNA in different brain regions of adult rats with different levels of physical activity, and found a positive correlation between the distance run per night and the BDNF produced in the hippocampus and caudal neocortex of these animals (139). Moreover, BDNF acts as a regulator of the ubiquitin-proteasome system (UPS) as it increases ubiquitin conjugation in synaptic proteins during synaptic remodeling. In addition, the use of a proteasome pharmacological inhibitor prevented BDNF-mediated action and had the same profile as the BDNF signaling block (140).
In summary, the benefits of physical exercise can be observed throughout different stages of life. Especially during aging, neuroprotection is promoted according to type and intensity of physical exercise. In general, it improves cerebrovascular health and gut microbiota diversity, which seems to be related to healthy aging. Furthermore, physical exercise improves quality of sleep and increases BDNF production, and can decrease neuronal death and improve cognitive performance, due to better functioning of the proteostasis system, among many other effects. Besides, data regarding physical exercise influence on gut microbiota, GH, CBF during aging seems contradictory both in animals and humans, likely depending on duration, intensity and type of physical exercise.
Proteostasis and Aging
The mammalian protein pool is subject to a constant quality control system that integrates the pathways related to protein synthesis, folding, unfolding, secretion, trafficking and degradation (Figure 1). This quality control system is known as proteostasis and its failures rely on increased levels of protein aggregates, which contribute to the development of proteinopathies and thus neurodegenerative diseases including Alzheimer's disease, Parkinson's disease, and amyotrophic lateral sclerosis (141, 142). The proteostasis decline is one of the hallmarks of aging (1, 143) and this decline can be explained by increased generation of oxidative damage within the cells (144).
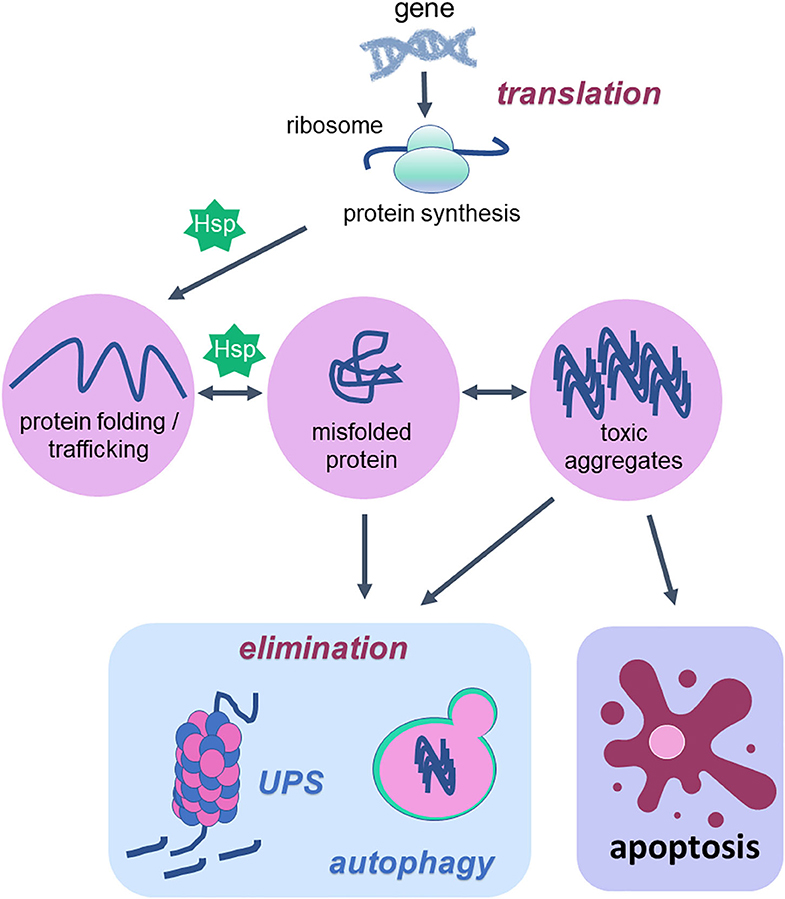
Figure 1. Cellular proteostasis and apoptotic cell death. Proteostasis network is the protein pool quality control system that integrates the pathways related to protein synthesis since translation, protein folding, protein unfolding, secretion, trafficking and degradation or elimination. To succeed in protein folding, unfolding, refolding and trafficking, chaperone proteins (heat shock proteins—Hsp) are of fundamental importance. In the elimination phase that usually happens when misfolded proteins turn into toxic aggregates, degradation of damaged proteins occurs through proteolytic systems: autophagy and ubiquitin-proteasome. The activity of both systems avoids cell death. However, when the proteostatic network cannot avoid protein aggregates accumulation, the cell undergoes apoptosis. Thus, degradation system and apoptosis are both important mechanisms for organism homeostasis due to the elimination of damaged proteins and damaged cells, respectively.
According to a vast literature in the field, the proteostasis network is mediated by the degradation of damaged proteins by proteolytic systems (autophagy and ubiquitin-proteasome) and correction or sequestration by chaperones (141, 145, 146). Although proteostasis involves all of these processes, this review will focus on the balance between two vital cellular processes such as autophagy and programmed cell death, apoptosis, based on the fact that there are several studies done with physical exercise as a non-pharmacological strategy that rescues the lost balance between them during aging (Figure 2).
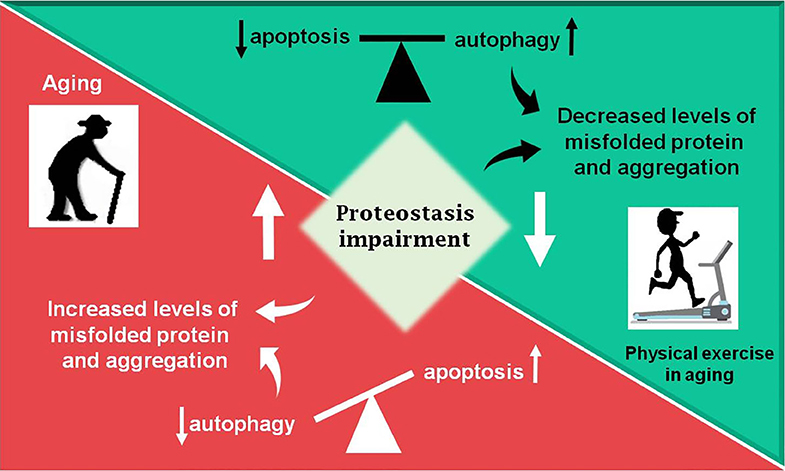
Figure 2. Summary of the review. During aging (red box) an increase in proteostasis impairment is observed in the Central Nervous System, which may lead to increased levels of misfolded proteins, in part due to decreased autophagic process. The balance between apoptosis and autophagy is lost and an increase in programmed cell death is observed. Otherwise, physical exercise in aging (green box) can partially revert the disbalance observed in aging, decreasing proteostasis impairment and improving autophagic process, as well as decreasing levels of misfolded proteins and toxic protein aggregates, which lead to less apoptosis activation.
The ubiquitin-proteasome system (UPS) is extremely important for the maintenance of protein homeostasis in the cytosolic and nuclear compartments. Ubiquitination occurs through catalytic enzymes, E ligases, which activate ubiquitin and covalently bind this polypeptide to the substrate, a tag for proteasome degradation. The proteasome, or 26S, is a multicatalytic complex with a proteolytic core, 20S, flanked by regulatory units that recognize the ubiquitinated substrates, misfolded and damaged proteins or healthy proteins, and lead them to degradation (147, 148). During aging, this system may be compromised due to defective proteasome activity, proteasome damage, proteasome assembly changes and ubiquitination defects (141). Studies with Drosophila melanogaster demonstrated a change from the 26S “activated” proteasome (1–32 days old) to the weakly active 20S form (43–47 days old) during aging, together with decline in ATP levels, highly necessary for the 26S proteasome activity (149). Therefore, UPS is one of the proteolytic systems that degrades damaged proteins regulating the proteostasis network (150, 151).
Autophagy in Aged Brain
During the aging process, organelles and proteins are prone to damage affecting their normal functionality, besides that these dysfunctional proteins, and organelles accumulate in the body progressively, thereby increasing the rate of cell death (1, 3). Studies indicate that the loss of autophagic activity in cell aging contributes to a progressive reduction in cell function and may precipitate cell death by restricting the ability of cells to support a healthy population of proteome and organelles (152, 153). Aging is associated with reduced autophagy potential and it has been shown in the literature that autophagic inhibition may result in premature aging (153).
Autophagy (divided into macroautophagy, microautophagy, and chaperone-mediated autophagy) is an important process of cell renewal in maintaining homeostasis and perfect cellular functionality, characterized by the elimination of non-functional proteins, damaged/defective organelles, and intracellular pathogens. Autophagy is an important cell survival mechanism with an important role in cell maintenance and homeostasis and with a positive influence on useful life and longevity (153–159).
The macroautophagy, here referred to as autophagy, has been the most studied. In summary, when damaged proteins and/or organelles are free in the cytoplasm, a nascent membrane originated from Golgi Complex, endoplasmic reticulum (ER), mitochondria, plasma membrane or endosomes is formed to engulf and sequester these damaged elements. This primordial membrane is called phagophore, which will, in a second step, fuse at its edges forming a double-membrane vesicle called autophagosome. The autophagosomes will undergo a maturation step in which they fuse with acidified lysosomal or endosomal vesicles to finally degrade a damaged element and recycle it (Figure 3A) (160, 161).
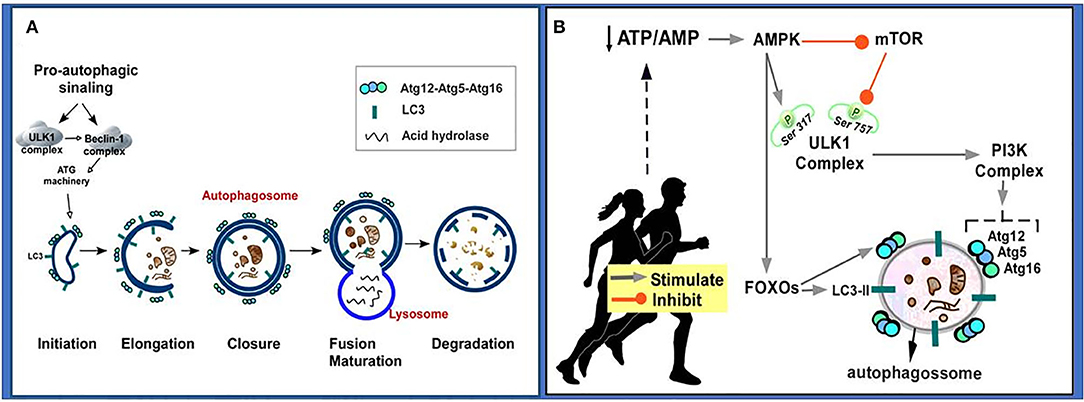
Figure 3. Autophageal mechanism and stages. (A) Pro-autophagic signaling induces ULK1 activation and Beclin-1 complex, activating the ATG machinery, thereby initiating the autophagy stages consisting of initiation, elongation, closure and autophagosome formation, autophagosome fusion with lysosome and degradation. (B) Physical exercise causes a decrease in the ATP/AMP ratio by activating AMPK. AMPK inhibits the mTOR pathway, so ULK Ser757 phosphorylation will be decreased and it may interact with AMPK and be activated by phosphorylation on Ser317. AMPK phosphorylated ULK1 becomes active and may initiate autophagy. PI3K activation occurs culminating in increased expression of autophagy-related proteins (Atg12, Atg5, Atg16). AMPK also promotes signaling of autophagy-related transcription factors (FOXOs), thereby increasing the expression of autophagy-related proteins (Atg12, Atg5, Atg16, LC3-II). Atg, autophagy-related protein; ATP/AMP, adenosine triphosphate/adenosine monophosphate; AMPK, AMP-activated protein kinase; Beclin-1, autophagy-related protein; FOXO, Forkhead box O; LC3II, microtubule-associated protein 1 light chain 3–form II; mTOR, mammalian target of rapamycin; PI3K, Phosphatidylinositol 3-kinase; UKL1, Unc-51-like kinase 1.
Among the main proteins that control the autophagic process, we can mention: autophagy-related (Atg) protein, which is associated with cytosolic component sequestration and autophagosome formation and is crucial for normal autophagic function (162, 163), for example Atg5, Atg12, Atg16; LC3, which participates in the phagophore and autophagosome expansions (164, 165); and Beclin-1, protein which participates in the initiation of the autophagic process by interacting directly with the phosphatidylinositol 3-kinase (PI3K) complex (166, 167). Autophagy is a very tightly controlled process that can adapt cellular metabolism to a stressful situation, such as starvation and growing factors deprivation, and can also be involved in turnover of organelles and long-lived proteins. Thus, autophagy and apoptosis are both important mechanisms for organism homeostasis due to the elimination of damaged or superfluous cellular components and damaged cells, respectively (160).
Autophagy dysfunctions may contribute to neurotoxicity associated with neurodegeneration and aging (168). Decreased age-related autophagy disrupts neuronal homeostasis and may thus promote the process of neurodegenerative disorders (169–171). However, data in the literature indicates that exercise can activate autophagy, thus preventing age-related diseases as well as retarding neurodegenerative processes [see reviews for more information (161, 172)].
Atgs knockout experiments have shown defects associated with aging, such as high accumulation of non-functional organelles (173–175), endoplasmic stress (173) and mitochondrial disorder (174–176). However, it remains unclear whether these Atgs reductions are, in fact, the main reason for age-related autophagic malfunction. Literature data suggests that basal autophagy decay may be mediated by excessive activity of rapamycin complex 1 (TORC1), a protein kinase that negatively regulates autophagy. The literature has shown that inhibition of TORC1 may increase longevity (177–179).
Influence of Physical Exercise in the Autophagy Process in Aged Brain
It has been postulated that regular physical exercise can promote a beneficial effect on the health of individuals and is considered an important autophagic inducer (180–183). It was observed that treadmill exercise (8 weeks) in mice modulated the levels of autophagy-associated proteins, including Beclin1, and improved autophagy (184). Based on literature data, it is suggested that physical exercise can induce autophagy through the following mechanism: exercise induces decreased adenosine triphosphate/adenosine monophosphate (ATP/AMP) in the cell, and this induces AMP-activated protein kinase (AMPK) activation; AMPK activation promotes inhibition of mammalian target of rapamycin (mTOR), leading to Unc-51 kinase 1 (ULK1) disinhibition, which is also phosphorylated and activated by AMPK; the ULK1 complex induces activation of the Phosphatidylinositol 3-kinase (PI3K) complex culminating in increased expression of autophagy-related proteins (Atgs); AMPK also promotes activation of autophagy-related transcription factors such as forkhead box O (FOXO), thereby increasing the expression of LC3-II and Atgs [for more detailed information on these signaling pathways, see (172, 185, 186) (Figure 3B)].
Based on data published in the literature, it is possible to suggest that the induction of autophagy by stimulating physical exercise is regulated according to exercise type, duration and/or intensity-dependent manner (182).
Kou et al. noted that swimming can delay the aging process, rescuing the impaired functional state of autophagy and abnormal mitochondrial dynamics. In addition, Luo et al. observed that 10-week swimming exercise in rats promoted adjustments in lysosomal degradation, activation of autophagy and mitochondrial quality control in the hippocampus, preventing age-associated cognitive decline. These findings indicate that the conservation of cognitive function in older rats by exercise is associated with mitochondrial improvement in the hippocampus, and lysosomal degradation is required in this process, suggesting that exercise and lysosomal degradation may be effective in decreasing age-related cognitive decline (187).
Huang et al. show that 8-week running exercise in mice can activate the autophagy pathway and improve lysosomal biogenesis, suggesting improvement in brain function of mice; besides that, they also observed that prolonged physical exercise promoted nuclear translocation of transcription factor EB (TFEB–main regulator of autophagic and lysosomal biogenesis) in the cortex, positively regulating the transcription of genes associated with autophagy and lysosome (lysosomal degradation is a fundamental step to completing the autophagy process) (188). It has also been observed that moderate exercise contributes to the prevention of early neurodegeneration in the substantia nigra region in aged rats by improving autophagy and mitophagy (189).
Given the data mentioned here, we can conclude that during aging there are dysfunctions in autophagy leading to CNS damage. Physical exercise could attenuate or prevent such autophagic dysfunctions. However, further studies are still necessary to indicate which modality, duration and intensity of physical exercise induce the greatest positive effects on CNS autophagy.
Apoptosis in Aged Brain
Apoptosis is a process of programmed cell death modulated by the B cell leukemia/lymphoma 2 (Bcl-2)/Bcl2 associated X protein (Bax) family and upregulated during the aging process (190, 191), which is important for tissue homeostasis (192). Apoptosis basically occurs in two different pathways: extrinsic and intrinsic. The extrinsic pathway is induced by death receptors and their ligands (Fas/ FasL complex) or via pro-inflammatory marker (tumor necrosis factor (TNF)α), and the intrinsic pathway is regulated by mitochondrial stress which activates caspase 9 and cleaves caspase 3 (Figure 4) (193, 194).
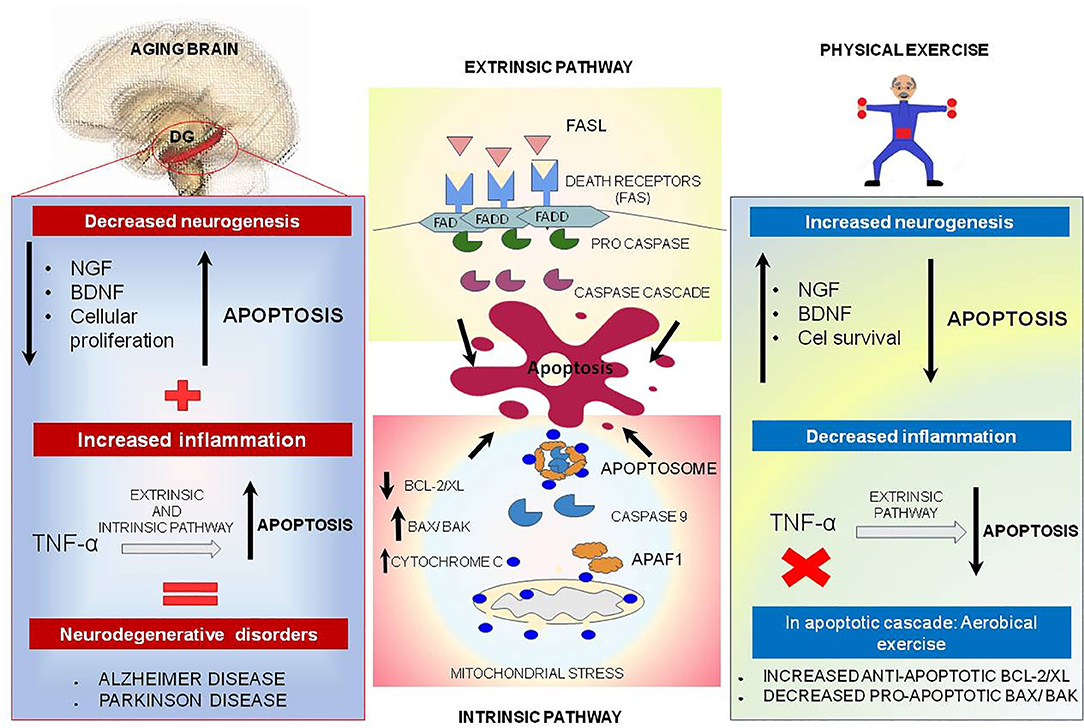
Figure 4. Physical exercise actions against aging brain and the apoptotic process. In the central boxes: Apoptosis can be divided into extrinsic and intrinsic signaling pathways. The extrinsic pathway illustrated in the upper central box is induced by death receptors such as FASL, and their ligands, FAS. On the other hand, the intrinsic pathway illustrated in the central inferior box is induced by signs that cause changes in the mitochondrial membrane, called mitochondrial outer membrane permeabilization (MOMP). This process generates macropores on the mitochondrial surface and a release of Cytochrome C, which once released by mitochondria binds to and induces APAF-1 and pro-caspase 9, forming the Apoptosome complex, which activates caspase 9 causing cell death. This pathway is mediated by anti-apoptotic proteins such as BCL-2 and BCL-XL, and pro-apoptotic proteins such as BAX and BAK. In the left box, brain aging is illustrated, and a decrease of neurotrophic factors such as nerve growth factor (NGF) is observed, and brain-derived neurotrophic factor (BDNF), especially in dentate gyrus located on hippocampus, may be related to decreased cell proliferation and increased apoptosis. In addition, TNF-alpha, a pro-inflammatory cytokine, can increase the apoptosis process by intrinsic and extrinsic pathways and lead to the emergence of neurodegenerative diseases. Physical exercise effects, illustrated in the right box, act in reverse, increasing the amount of NGF and BDNF, increasing cell survival and culminating in decreased apoptosis. In addition, physical exercise inhibits the production of pro-inflammatory cytokine TNF-alpha which, in low quantities, decreases the extrinsic apoptosis process.
The Bcl-2 family is related to apoptotic intrinsic pathway and has a range of 20 different proteins. Each protein of this family has homology domains: BH1 to BH4. Pro-apoptotic proteins are related to BH3 domains called BCL2 antagonist killer 1 (BAK) and BAX. The other domains are related to anti-apoptotic proteins, known as BCL2, BCLXL, BCL2L2, myeloid cell leukemia 1 (MCL1) and BCL2A1 (194–196). In the intrinsic pathway an increased expression of anti-apoptotic Bcl-2 proteins modulates the expression of cell cycle inhibitors and induces cellular senescence, while the expression of pro-apoptotic factors such as Bax and Bak proteins results in macropores formation in the mitochondrial surface, called mitochondrial outer membrane permeabilization (MOMP). This pore allows the exit of cytochrome c from mitochondria, which culminates in activation of caspase cascade causing cell death. Bcl-2 acts as an anti-apoptotic factor that can inhibit the activation of Bax or Bak and inhibits autophagy by beclin-1, which modulates cellular senescence (194–197). Caspase-3 is one of the key proteins of apoptosis and could be responsible for the proteolytic cleavage of many proteins such as poly (ADP-ribose) polymerase (PARP) responsible for DNA repair observed by Cechella et al. in aged rat brains (27).
In the aged brain, there is a reduction in the availability of neurotrophic factors such as nerve growth factor (NGF) and brain-derived neurotrophic factor (BDNF), especially in the hippocampus; therefore, these changes may be linked with the large reduction in cell proliferation and increase in apoptosis in the dentate gyrus (8, 198). Besides, TNF-α, a pro-inflammatory protein, could increase the process of apoptosis via intrinsic and extrinsic pathways, which is more significantly visible in aged brain and could be implicated in neurodegenerative diseases, such as Alzheimer's disease (AD) and Parkinson's disease (PD) (Figure 4) (199–201).
Influence of Physical Exercise in the Apoptosis Process in Aged Brain
Sedentary lifestyle could be a risk factor for cognitive dysfunction and neurodegenerative process, and regular exercise has anti-aging effects, more specifically in the CNS, whose benefits include an increase in hippocampus neurogenesis, which improves learning and memory in aged rodents. Exercise can upregulate BDNF in hippocampal and cortical neurons promoting synaptic remodeling and improving cell survival (202, 203). Physical exercise inhibits the production of the pro-inflammatory cytokine TNF-α, which in low concentration decreases the process of apoptosis via the extrinsic pathway (200).
Aerobic Physical Exercise (APE) is the most common type of training for rodents, such as running and swimming, and they must be conducted repeatedly with an established frequency; however, few studies have compared the effects of many different modes of exercise on cognition (7). APE is able to positively affect the dynamic adaptations of the neuronal terminal zones in aging. Fattoretti et al. observed effects of APE in the hippocampus of old mice (27 months) submitted to a 4-week aerobic training in a treadmill apparatus, five times per week, and found that hippocampal regions are not uniformly influenced by physical training: they show an increase in the number of synapses and synaptic area per μm3 of tissue in CA1 caused by physical exercise protocol in aged animals, while in DG they could only see an increased number in the synaptic area (204).
Moderate intensity APE protocol for 28 days in 18-month-old mice induced an upregulation of hippocalcin, α-spectrin and ovarian tumor domain-containing ubiquitin aldehyde-binding protein 1 (OTUB1) in the hippocampus (6). Hippocalcin, which is a calcium ligand, has been shown to protect neurons against apoptosis by regulating inhibitory proteins (205). Xie et al. using an intracerebral hemorrhage method in rats, verified that OUTB1 colocalized with active caspase 3 and attenuated neuronal apoptosis (206). Besides, hippocalcin and spectrin-α in the hippocampus of old mice could be related to an increase in neurogenesis (7).
Fang et al. tested a 12-week protocol of treadmill aerobic exercise in aged rats, and observed by TUNEL staining and immunohistochemistry that the number of TUNEL positive cells (dead cells) had diminished in cortex and hippocampus after the exercise protocol. Moreover, anti-apoptotic protein Bcl-2 levels were augmented. On the other hand, pro-apoptotic proteins, Bax and cleaved caspase-3 levels were decreased in hippocampus and cortex of these rodents submitted to the APE protocol. Another important evidence for the benefits of APE is the decrease of the cytoskeletal protein tau hyperphosphorylation in the brain of old rodents (197, 207–209).
APE is not restricted only to the treadmill apparatus. Cechella et al. submitted rats to a forced swimming protocol for 4 weeks and one experimental group of rodents were supplemented with diphenyl diselenide ((PhSe)2), a potential antioxidant compound. Both groups, exercise and exercise plus (PhSe)2, could decrease the level of pro-apoptotic proteins in 27-month-old rats. They observed an increase in BDNF levels that has led to a downregulation of pJNK/JNK ratio which induced caspase 3 cleavage. The cleaved caspase 3/caspase 3 ratio was also decreased, while Bcl2 expression was increased in animals submitted to both protocols (27). Conversely, Liu et al. affirmed that, after 10 weeks of treadmill exercise, apoptotic striatum cells in old-aged rats increased by 68.24% in comparison to sedentary animals, showing that intense physical activity might not be beneficial for the organism (210).
Do et al. tested voluntary running in triple transgenic AD mice for 4 and 8 weeks. At the end of this period, they showed a reduction of apoptotic cells in the hypothalamus (211). In addition, 4-week voluntary exercise training reduced pro-inflammatory cytokines TNF-α and IL-6 to the levels compared to the control, which supports previous studies in the literature (212, 213).
There is a great amount of research related to aerobic exercise and apoptosis, but little is known about the effects of resistance training (RT) on the brain. Aerobic exercise may induce events such as cell proliferation, survival and death through distinct mechanisms from those of resistance exercise (214, 215). The RT consisted of bodybuilding exercises like adductor, abductor, leg presses, triceps and biceps exercises, or in a simpler way for rodents, it could be climbing a ladder repeatedly, for example (200, 216, 217). de Smolarek et al. (217) showed that RT could be very suitable for the elderly population because they were able to see an improvement in cognition in elderly women (between 65 and 69 years old) submitted to resistance training for 12 weeks. Another study that used RT protocol in elderly women (between 65 and 75 years old) for 52 weeks showed, after this period, an increase in these women's cognition, mainly in learning, measured by Mini-Mental State Examination and another verbal quiz (26).
There are few studies in the literature showing that RT could be used for hospitalized elderly people who spend most of the time lying on a bed relying exclusively on physiotherapy exercises (218–221). Martínez-Velilla et al. used an RT protocol that includes physical exercises such as line walking, stepping practice, proprioceptive exercises, among others (219). The results showed that RT protocol was efficient in preventing functional decline caused by hospitalization (218). Other clinical trials with RT protocol applied in 65-year-old healthy men and women, consisting of a 3-month body-mass-based exercise program, revealed that only working memory was improved (222).
Frailty is a syndrome characterized by dysregulation of several physiological systems (223, 224). Few studies with physical exercise (not only RT exercise, but also APE) were conducted to evaluate its efficiency in ameliorating frailty phenotype (218, 225). Yoon et al. tested an RT high speed program in elderly people (74 years old) with frailty syndrome for 16 weeks and they observed that RT improved cognitive and physical functions (218). Based on clinical trials data, we can conclude there is a good outcome in using RT protocol in the elderly to improve their cognition (218, 219, 222).
In another perspective, regarding animal studies, Henrique et al. (200) compared two protocols, APE and RT. APE protocol used treadmill running, and RT protocol consisted of a series of eight climbs with a progressively heavier load, both for 7 weeks in 21-month-old rats. They observed that the RT group had a reduction in hippocampal levels of macrophage inflammatory peptide (MIP)-2 protein, a pro-inflammatory mediator which seems to be related to inducing apoptosis (226), so the effects of RT on MIP-2 levels deserve to be more explored.
Vilela et al. (215) also compared APE and RT in 24-month-old rats and observed, in both protocols, an increase of the hippocampal neurotrophin receptor P75 (P75NTR), a transmembrane receptor involved in many cellular functions including apoptosis, cell survival, neurite outgrowth, migration, and cell cycle arrest (227, 228). In this case, the researchers believed that P75NTR was involved in neuroprotection through activation of the apoptosis pathway to induce death process on damaged neurons and to provide a conducive environment for insertion of new cells (215, 229).
In summary, both APE and RT could improve spatial memory or activate different mechanisms that lead to cell survival and induce a decrease of apoptotic cells. RT can be a strategy to protect the brain and maintain healthy cognition during the aging process, while APE could alter intracellular pathways, even though the related mechanisms that explain these effects still remain unknown.
Conclusion and Perspectives
Based on what we cited in this review, we can conclude that during the aging process dysfunctions can occur in several cellular events, such as autophagy and apoptosis, which can culminate in CNS damage. Physical exercise could attenuate or prevent such autophagic or apoptotic dysfunctions. At the same there is controversial data from the literature both in human and animal studies. Therefore, further studies are still necessary to clarify the effects of physical exercise during the aging process and also to demystify underlying mechanisms of physical exercise effects and indicate which modality, duration and intensity of exercise is able to induce the greatest positive effects in the CNS, thus preventing neuronal death.
Author Contributions
EK conceived the review idea and edited the final version of the text and figures. JS, AO, AM, and DA contributed equally by writing the text as well as drawing the figures, and participated in the discussion of the review idea. PM contributed by drafting the review and drawing the figures. All authors contributed to the article and approved the submitted version.
Funding
AM and PM are supported by master and undergraduate fellowships, respectively, from Fundação de Amparo à Pesquisa do Estado de São Paulo (FAPESP). AO is supported by Post-Doctoral fellowship from Coordenação de Aperfeiçoamento de Pessoal de Nível Superior – Brasil (CAPES). EK is research fellow from Conselho Nacional de Desenvolvimento Nacional e Tecnológico-CNPq. This publication was made possible by grants from FAPESP (2016/22996-9, 2016/07427-8).
Conflict of Interest
The authors declare that the research was conducted in the absence of any commercial or financial relationships that could be construed as a potential conflict of interest.
Acknowledgments
We thank Carolina Fernandez and Cecilia Fernandez (English Language CF Advisory) for English revision.
References
1. Lopez-Otin C, Blasco MA, Partridge L, Serrano M, Kroemer G. The hallmarks of aging. Cell. (2013) 153:1194–217. doi: 10.1016/j.cell.2013.05.039
2. Kirkwood TB. Understanding the odd science of aging. Cell. (2005) 120:437–47. doi: 10.1016/j.cell.2005.01.027
3. Escobar KA, Cole NH, Mermier CM, VanDusseldorp AT. Autophagy and aging: maintaining the proteome through exercise and caloric restriction. Aging Cell. (2019) 18:e12876. doi: 10.1111/acel.12876
4. Bennett IJ, Madden JD. Disconnected aging: cerebral white matter integrity and age-related differences in cognition. Neuroscience. (2014) 276:187–205. doi: 10.1016/j.neuroscience.2013.11.026
5. Metzler-Baddeley C, Mole JP, Sims R, Fasano F, Evans J, Jones DK, et al. Fornix white matter glia damage causes hippocampal gray matter damage during age-dependent limbic decline. Sci Rep. (2019) 9:1060. doi: 10.1038/s41598-019-51737-1
6. Kempermann G. Activity dependency and aging in the regulation of adult neurogenesis. Cold Spring Harb Perspect Biol. (2015) 7:a018929. doi: 10.1101/cshperspect.a018929
7. Kim JH, Liu QF, Urnuhsaikhan E, Jeong HJ, Jeon MY, Jeon S. Moderate-intensity exercise induces neurogenesis and improves cognition in old mice by upregulating hippocampal hippocalcin, otub1, and spectrin-α. Mol Neurobiol. (2019) 56:3069–78. doi: 10.1007/s12035-018-1239-x
8. Birch AM, Kelly Á. Lifelong environmental enrichment in the absence of exercise protects the brain from age-related cognitive decline. Neuropharmacology. (2019) 145(Pt A):59–74. doi: 10.1016/j.neuropharm.2018.03.042
9. Kempermann G, Song H, Gage HF. Neurogenesis in the adult hippocampus. Cold Spring Harb Perspect Biol. (2015) 7:a018812. doi: 10.1101/cshperspect.a018812
10. Kumar A, Pareek V, Faiq MA, Ghosh SK, Kumari C. Adult neurogenesis in humans: a review of basic concepts, history, current research, clinical implications. Innov Clin Neurosci. (2019) 16:30–37.
11. Sorrells SF, Paredes MF, Cebrian-Silla A, Sandoval K, Qi D, Kelley KW, et al. Human hippocampal neurogenesis drops sharply in children to undetectable levels in adults. Nature. (2018) 555:377–81. doi: 10.1038/nature25975
12. Boldrini M, Fulmore CA, Tartt AN, Simeon LR, Pavlova I, Poposka V, et al. Human hippocampal neurogenesis persists throughout aging. Cell Stem Cell. (2018) 22:589–99.e5. doi: 10.1016/j.stem.2018.03.015
13. Kempermann G, Gage FH, Aigner L, Song H, Curtis MA, Thuret S, et al. Human adult neurogenesis: evidence and remaining questions. Cell Stem Cell. (2018) 23:25–30. doi: 10.1016/j.stem.2018.04.004
14. Spalding KL, Bergmann O, Alkass K, Bernard S, Salehpour M, Huttner HB, et al. Dynamics of hippocampal neurogenesis in adult humans. Cell. (2013) 153:1219–27. doi: 10.1016/j.cell.2013.05.002
15. Dennis CV, Suh LS, Rodriguez ML, Kril JJ, Sutherland TG. Human adult neurogenesis across the ages: an immunohistochemical study. Neuropathol Appl Neurobiol. (2016) 42:621–38. doi: 10.1111/nan.12337
16. Eriksson PS, Perfilieva E, Björk-Eriksson T, Alborn AM, Nordborg C, Peterson DA, et al. Neurogenesis in the adult human hippocampus. Nat Med. (1998) 4:1313–7. doi: 10.1038/3305
17. Tobin MK, Musaraca K, Disouky A, Shetti A, Bheri A, Honer WG, et al. Human hippocampal neurogenesis persists in aged adults and alzheimer's disease patients. Cell Stem Cell. (2019) 24:974–82.e3. doi: 10.1016/j.stem.2019.05.003
18. Moreno-Jiménez EP, Flor-García M, Terreros-Roncal J, Rábano A, Cafini F, Pallas-Bazarra N, et al. Adult hippocampal neurogenesis is abundant in neurologically healthy subjects and drops sharply in patients with alzheimer's disease. Nat Med. (2019) 25:554–60. doi: 10.1038/s41591-019-0375-9
19. van Praag H, Kempermann G, Gage HF. Running increases cell proliferation and neurogenesis in the adult mouse dentate gyrus. Nat Neurosci. (1999) 2:266–70. doi: 10.1038/6368
20. Leasure JL, Jones M. Forced and voluntary exercise differentially affect brain and behavior. Neuroscience. (2008) 156:456–65. doi: 10.1016/j.neuroscience.2008.07.041
21. Apple DM, Solano-Fonseca R, Kokovay E. Neurogenesis in the aging brain. Biochem Pharmacol. (2017) 141:77–85. doi: 10.1016/j.bcp.2017.06.116
22. Lei X, Wu Y, Xu M, Jones OD, Ma J, Xu X. Physical exercise: bulking up neurogenesis in human adults. Cell Biosci. (2019) 9:74. doi: 10.1186/s13578-019-0337-4
23. Northey JM, Cherbuin N, Pumpa KL, Smee DJ, Rattray B. Exercise interventions for cognitive function in adults older than 50: a systematic review with meta-analysis. Br J Sports Med. (2018) 52:154–60. doi: 10.1136/bjsports-2016-096587
24. Pereira AC, Huddleston DE, Brickman AM, Sosunov AA, Hen R, McKhann GM, et al. An in vivo correlate of exercise-induced neurogenesis in the adult dentate gyrus. Proc Natl Acad Sci USA. (2007) 104:5638–43. doi: 10.1073/pnas.0611721104
25. Suwabe K, Byun K, Hyodo K, Reagh ZM, Roberts JM, Matsushita A, et al. Rapid stimulation of human dentate gyrus function with acute mild exercise. Proc Natl Acad Sci USA. (2018) 115:10487–92. doi: 10.1073/pnas.1805668115
26. Best JR, Chiu BK, Liang Hsu C, Nagamatsu LS, Liu-Ambrose T. Long-term effects of resistance exercise training on cognition and brain volume in older women: results from a randomized controlled trial. J Int Neuropsychol Soc. (2015) 21:745–56. doi: 10.1017/S1355617715000673
27. Cechella JL, Leite MR, Pinton S, Zeni G, Nogueira WC. Neuroprotective benefits of aerobic exercise and organoselenium dietary supplementation in hippocampus of old rats. Mol Neurobiol. (2018) 55:3832–40. doi: 10.1007/s12035-017-0600-9
28. Caspersen CJ, Powell KE, Christenson MG. Physical activity, exercise, and physical fitness: definitions and distinctions for health-related research. Public Health Rep. (1985) 100:126–31.
29. Raichlen DA, Polk DJ. Linking brains and brawn: exercise and the evolution of human neurobiology. Proc R Soc B Biol Sci. (2013) 280:20122250. doi: 10.1098/rspb.2012.2250
30. Wallace IJ, Hainline C, Lieberman ED. Sports and the human brain: an evolutionary perspective. Handb Clin Neurol. (2018) 158:3–10. doi: 10.1016/B978-0-444-63954-7.00001-X
31. Bortz WM II. Physical exercise as an evolutionary force. J Hum Evol. (1985) 14:145–55. doi: 10.1016/S0047-2484(85)80003-8
32. Mattson MP. Evolutionary aspects of human exercise–born to run purposefully. Ageing Res Rev. (2012) 11:347–52. doi: 10.1016/j.arr.2012.01.007
33. Perales M, Refoyo I, Coteron J, Bacchi M, Barakat R. Exercise during pregnancy attenuates prenatal depression. Eval Health Prof. (2015) 38:59–72. doi: 10.1177/0163278714533566
34. Perales M, Calabria I, Lopez C, Franco E, Coteron J, Barakat R. Regular exercise throughout pregnancy is associated with a shorter first stage of labor. Am J Health Promot. (2016) 30:149–57. doi: 10.4278/ajhp.140221-QUAN-79
35. Labonte-Lemoyne E, Curnier D, Ellemberg D. Exercise during pregnancy enhances cerebral maturation in the newborn: a randomized controlled trial. J Clin Exp Neuropsychol. (2017) 39:347–54. doi: 10.1080/13803395.2016.1227427
36. Nascimento SL, Surita FG, Godoy AC, Kasawara KT, Morais SS. Physical activity patterns and factors related to exercise during pregnancy: a cross sectional study. PLoS ONE. (2015) 10:e0128953. doi: 10.1371/journal.pone.0128953
37. Vanhelst J, Béghin L, Duhamel A, Manios Y, Molnar D, De Henauw S, et al. Physical activity is associated with attention capacity in adolescents. J Pediatr. (2016) 168:126–31.e2. doi: 10.1016/j.jpeds.2015.09.029
38. de Greeff JW, Bosker RJ, Oosterlaan J, Visscher C, Hartman E. Effects of physical activity on executive functions, attention and academic performance in preadolescent children: a meta-analysis. J Sci Med Sport. (2018) 21:501–7. doi: 10.1016/j.jsams.2017.09.595
39. Balchin R, Linde J, Blackhurst D, Rauch HL, Schönbächler G. Sweating away depression? The impact of intensive exercise on depression. J Affect Disord. (2016) 200:218–21. doi: 10.1016/j.jad.2016.04.030
40. Sadeghi K, Ahmadi SM, Ahmadi SM, Rezaei M, Miri J, Abdi A, et al. A comparative study of the efficacy of cognitive group therapy and aerobic exercise in the treatment of depression among the students. Glob J Health Sci. (2016) 8:54171. doi: 10.5539/gjhs.v8n10p1
41. Radovic S, Gordon MS, Melvin AG. Should we recommend exercise to adolescents with depressive symptoms? A meta-analysis. J Paediatr Child Health. (2017) 53:214–20. doi: 10.1111/jpc.13426
42. Bailey AP, Hetrick SE, Rosenbaum S, Purcell R, Parker GA. Treating depression with physical activity in adolescents and young adults: a systematic review and meta-analysis of randomised controlled trials. Psychol Med. (2018) 48:1068–83. doi: 10.1017/S0033291717002653
43. Bullo V, Bergamin M, Gobbo S, Sieverdes JC, Zaccaria M, Neunhaeuserer D, et al. The effects of pilates exercise training on physical fitness and wellbeing in the elderly: a systematic review for future exercise prescription. Prev Med. (2015) 75:1–11. doi: 10.1016/j.ypmed.2015.03.002
44. Mello NF, Costa DL, Vasconcellos SV, Lensen CMM, Corazza TS. The effect of the contemporary pilates method on physical fitness, cognition and promotion of quality of life among the elderly. Revis Brasileira Geriatria e Gerontol. (2018) 21:597–603. doi: 10.1590/1981-22562018021.180083
45. Tao J, Chen X, Egorova N, Liu J, Xue X, Wang Q, et al. Tai chi chuan and baduanjin practice modulates functional connectivity of the cognitive control network in older adults. Sci Rep. (2017) 7:41581. doi: 10.1038/srep41581
46. Sungkarat S, Boripuntakul S, Kumfu S, Lord SR, Chattipakorn N. Tai chi improves cognition and plasma BDNF in older adults with mild cognitive impairment: a randomized controlled trial. Neurorehabil Neural Repair. (2018) 32:142–9. doi: 10.1177/1545968317753682
47. Tsai CL, Wang CH, Pan CY, Chen CF. The effects of long-term resistance exercise on the relationship between neurocognitive performance and GH, IGF-1, and homocysteine levels in the elderly. Front Behav Neurosci. (2015) 9:23. doi: 10.3389/fnbeh.2015.00023
48. Nishiguchi S, Yamada M, Tanigawa T, Sekiyama K, Kawagoe T, Suzuki M, et al. A 12-week physical and cognitive exercise program can improve cognitive function and neural efficiency in community-dwelling older adults: a randomized controlled trial. J Am Geriatr Soc. (2015) 63:1355–63. doi: 10.1111/jgs.13481
49. Tait JL, Duckham RL, Milte CM, Main LC, Daly MR. Influence of sequential vs. Simultaneous dual-task exercise training on cognitive function in older adults. Front Aging Neurosci. (2017) 9:368. doi: 10.3389/fnagi.2017.00368
50. Sáez de Asteasu ML, Martínez-Velilla N, Zambom-Ferraresi F, Casas-Herrero Á, Izquierdo M. Role of physical exercise on cognitive function in healthy older adults: a systematic review of randomized clinical trials. Ageing Res Rev. (2017) 37:117–34. doi: 10.1016/j.arr.2017.05.007
51. Jonasson LS, Nyberg L, Kramer AF, Lundquist A, Riklund K, Boraxbekk CJ. Aerobic exercise intervention, cognitive performance, and brain structure: results from the physical influences on brain in aging (PHIBRA) study. Front Aging Neurosci. (2017) 8:336. doi: 10.3389/fnagi.2016.00336
52. Ludyga S, Gerber M, Brand S, Holsboer-Trachsler E, Pühse U. Acute effects of moderate aerobic exercise on specific aspects of executive function in different age and fitness groups: a meta-analysis. Psychophysiology. (2016) 53:1611–26. doi: 10.1111/psyp.12736
53. Physical Activity Guidelines Advisory Committee report, 2008. To the secretary of health and human services. Part A: executive summary. Nutr Rev. (2009) 67:114–20.
54. Arem H, Moore SC, Patel A, Hartge P, Berrington de Gonzalez A, Visvanathan K, et al. Leisure time physical activity and mortality: a detailed pooled analysis of the dose-response relationship. JAMA Intern Med. (2015) 175:959–67. doi: 10.1001/jamainternmed.2015.0533
55. Franco MR, Tong A, Howard K, Sherrington C, Ferreira PH, Pinto RZ, et al. Older people's perspectives on participation in physical activity: a systematic review and thematic synthesis of qualitative literature. Br J Sports Med. (2015) 49:1268–76. doi: 10.1136/bjsports-2014-094015
56. Hupin D, Roche F, Gremeaux V, Chatard JC, Oriol M, Gaspoz JM, et al. Even a low-dose of moderate-to-vigorous physical activity reduces mortality by 22% in adults aged ≥60 years: a systematic review and meta-analysis. Br J Sports Med. (2015) 49:1262–7. doi: 10.1136/bjsports-2014-094306
57. Sparling PB, Howard BJ, Dunstan DW, Owen N. Recommendations for physical activity in older adults. BMJ. (2015) 350:h100. doi: 10.1136/bmj.h100
58. Cotman CW, Berchtold CN. Physical activity and the maintenance of cognition: learning from animal models. Alzheimers Dement. (2007) 3(2 Suppl):S30–7. doi: 10.1016/j.jalz.2007.01.013
59. Cancela JM, Ayán C, Varela S, Seijo M. Effects of a long-term aerobic exercise intervention on institutionalized patients with dementia. J Sci Med Sport. (2016) 19:293–8. doi: 10.1016/j.jsams.2015.05.007
60. Zanetidou S, Belvederi Murri M, Menchetti M, Toni G, Asioli F, Bagnoli L, et al. Physical exercise for late-life depression: customizing an intervention for primary care. J Am Geriatr Soc. (2017) 65:348–55. doi: 10.1111/jgs.14525
61. Huang TT, Liu CB, Tsai YH, Chin YF, Wong CH. Physical fitness exercise versus cognitive behavior therapy on reducing the depressive symptoms among community-dwelling elderly adults: a randomized controlled trial. Int J Nurs Stud. (2015) 52:1542–52. doi: 10.1016/j.ijnurstu.2015.05.013
62. De Labra C, Guimaraes-Pinheiro C, Maseda A, Lorenzo T, Millán-Calenti CJ. Effects of physical exercise interventions in frail older adults: a systematic review of randomized controlled trials. BMC Geriatr. (2015) 15:154. doi: 10.1186/s12877-015-0155-4
63. Mañas A, Del Pozo-Cruz B, Guadalupe-Grau A, Marín-Puyalto J, Alfaro-Acha A, Rodríguez-Mañas L, et al. Reallocating accelerometer-assessed sedentary time to light or moderate- to vigorous-intensity physical activity reduces frailty levels in older adults: an isotemporal substitution approach in the TSHA study. J Am Med Direct Assoc. (2018) 19:185.e1–185.e6. doi: 10.1016/j.jamda.2017.11.003
64. Duchesne C, Lungu O, Nadeau A, Robillard ME, Boré A, Bobeuf F, et al. Enhancing both motor and cognitive functioning in Parkinson's disease: aerobic exercise as a rehabilitative intervention. Brain Cogn. (2015) 99:68–77. doi: 10.1016/j.bandc.2015.07.005
65. Hoffmann K, Sobol NA, Frederiksen KS, Beyer N, Vogel A, Vestergaard K, et al. Moderate-to-high intensity physical exercise in patients with alzheimer's disease: a randomized controlled trial. J Alzheimer's Dis. (2015) 50:443–53. doi: 10.3233/JAD-150817
66. Fernandes J, Arida RM, Gomez-Pinilla F. Physical exercise as an epigenetic modulator of brain plasticity and cognition. Neurosci Biobehav Rev. (2017) 80:443–56. doi: 10.1016/j.neubiorev.2017.06.012
67. Grazioli E, Dimauro I, Mercatelli N, Wang G, Pitsiladis Y, Di Luigi L, et al. Physical activity in the prevention of human diseases: role of epigenetic modifications. BMC Genomics. (2017) 18(Suppl. 8):802. doi: 10.1186/s12864-017-4193-5
68. Lavratti C, Dorneles G, Pochmann D, Peres A, Bard A, De Lima Schipper L, et al. Exercise-induced modulation of histone H4 acetylation status and cytokines levels in patients with schizophrenia. Physiol Behav. (2017) 168:84–90. doi: 10.1016/j.physbeh.2016.10.021
69. Quach A, Levine ME, Tanaka T, Lu AT, Chen BH, Ferrucci L, et al. Epigenetic clock analysis of diet, exercise, education, lifestyle factors. Aging (Albany NY). (2017) 9:419–46. doi: 10.18632/aging.101168
70. Jang Y, Kwon I, Song W, Cosio-Lima LM, Lee Y. Endurance exercise mediates neuroprotection against MPTP-mediated parkinson's disease via enhanced neurogenesis, antioxidant capacity, and autophagy. Neuroscience. (2018) 379:292–301. doi: 10.1016/j.neuroscience.2018.03.015
71. Li Y, Zhao L, Gu B, Cai J, Lv Y, Yu L. Aerobic exercise regulates Rho/cofilin pathways to rescue synaptic loss in aged rats. PLoS ONE. (2017) 12:e0171491. doi: 10.1371/journal.pone.0171491
72. Leeuwis AE, Smith LA, Melbourne A, Hughes AD, Richards M, Prins ND, et al. Cerebral blood flow and cognitive functioning in a community-based, multi-ethnic cohort: the SABRE study. Front Aging Neurosci. (2018) 10:279. doi: 10.3389/fnagi.2018.00279
73. Tarumi T, Zhang R. Cerebral blood flow in normal aging adults: cardiovascular determinants, clinical implications, aerobic fitness. J Neurochem. (2018) 144:595–608. doi: 10.1111/jnc.14234
74. de la Torre JC. Is alzheimer's disease a neurodegenerative or a vascular disorder? Data, dogma, and dialectics. Lancet Neurol. (2004) 3:184–90. doi: 10.1016/S1474-4422(04)00683-0
75. Ogoh S. Relationship between cognitive function and regulation of cerebral blood flow. J Physiol Sci. (2017) 67:345–51. doi: 10.1007/s12576-017-0525-0
76. Leeuwis AE, Benedictus MR, Kuijer JPA, Binnewijzend MAA, Hooghiemstra AM, Verfaillie SCJ, et al. Lower cerebral blood flow is associated with impairment in multiple cognitive domains in alzheimer's disease. Alzheimers Dement. (2017) 13:531–40. doi: 10.1016/j.jalz.2016.08.013
77. Barnes JN, Taylor JL, Kluck BN, Johnson CP, Joyner JM. Cerebrovascular reactivity is associated with maximal aerobic capacity in healthy older adults. J Appl Physiol (1985). (2013) 114:1383–7. doi: 10.1152/japplphysiol.01258.2012
78. Kleinloog JPD, Mensink RP, Ivanov D, Adam JJ, Uludag K, Joris JP. Aerobic exercise training improves cerebral blood flow and executive function: a randomized, controlled cross-over trial in sedentary older men. Front Aging Neurosci. (2019) 11:333. doi: 10.3389/fnagi.2019.00333
79. Barnes JN. Exercise, cognitive function, and aging. Adv Physiol Educ. (2015) 39:55–62. doi: 10.1152/advan.00101.2014
80. Chapman SB, Aslan S, Spence JS, Defina LF, Keebler MW, Didehbani N, et al. Shorter term aerobic exercise improves brain, cognition, and cardiovascular fitness in aging. Front Aging Neurosci. (2013) 5:75. doi: 10.3389/fnagi.2013.00075
81. Alfini AJ, Weiss LR, Leitner BP, Smith TJ, Hagberg JM, Smith CJ. Hippocampal and cerebral blood flow after exercise cessation in master athletes. Front Aging Neurosci. (2016) 8:184. doi: 10.3389/fnagi.2016.00184
82. Endres M, Gertz K, Lindauer U, Katchanov J, Schultze J, Schröck H, et al. Mechanisms of stroke protection by physical activity. Ann Neurol. (2003) 54:582–90. doi: 10.1002/ana.10722
83. Leulier F, MacNeil LT, Lee WJ, Rawls JF, Cani PD, Schwarzer M, et al. Integrative physiology: at the crossroads of nutrition, microbiota, animal physiology, human health. Cell Metab. (2017) 25:522–34. doi: 10.1016/j.cmet.2017.02.001
84. Roberts MC, Millikan RC, Galanko JA, Martin C, Sandler SR. Constipation, laxative use, and colon cancer in a North Carolina population. Am J Gastroenterol. (2003) 98:857–64. doi: 10.1111/j.1572-0241.2003.07386.x
85. Mancabelli L, Milani C, Lugli GA, Turroni F, Mangifesta M, Viappiani A, et al. Unveiling the gut microbiota composition and functionality associated with constipation through metagenomic analyses. Sci Rep. (2017) 7:9879. doi: 10.1038/s41598-017-10663-w
86. Aoyagi Y, Amamoto R, Park S, Honda Y, Shimamoto K, Kushiro A, et al. Independent and interactive effects of habitually ingesting fermented milk products containing. Front Microbiol. (2019) 10:1477. doi: 10.3389/fmicb.2019.01477
87. Taniguchi H, Tanisawa K, Sun X, Kubo T, Hoshino Y, Hosokawa M, et al. Effects of short-term endurance exercise on gut microbiota in elderly men. Physiol Rep. (2018) 6:e13935. doi: 10.14814/phy2.13935
88. Houghton D, Stewart CJ, Stamp C, Nelson A, Aj Ami NJ, Petrosino JF, et al. Impact of age-related mitochondrial dysfunction and exercise on intestinal microbiota composition. J Gerontol A Biol Sci Med Sci. (2018) 73:571–8. doi: 10.1093/gerona/glx197
89. Fielding RA, Reeves AR, Jasuja R, Liu C, Barrett BB, Lustgarten SM. Muscle strength is increased in mice that are colonized with microbiota from high-functioning older adults. Exp Gerontol. (2019) 127:110722. doi: 10.1016/j.exger.2019.110722
90. Finkelstein JW, Roffwarg HP, Boyar RM, Kream J, Hellman L. Age-related change in the twenty-four-hour spontaneous secretion of growth hormone. J Clin Endocrinol Metab. (1972) 35:665–70. doi: 10.1210/jcem-35-5-665
91. Zadik Z, Chalew SA, McCarter RJ, Meistas M, Kowarski AA. The influence of age on the 24-hour integrated concentration of growth hormone in normal individuals. J Clin Endocrinol Metab. (1985) 60:513–6. doi: 10.1210/jcem-60-3-513
92. Rudman D, Feller AG, Nagraj HS, Gergans GA, Lalitha PY, Goldberg AF, et al. Effects of human growth hormone in men over 60 years old. N Engl J Med. (1990) 323:1–6. doi: 10.1056/NEJM199007053230101
93. Coiro V, Volpi R, Capretti L, Caffarri G, Davoli C, Chiodera P. Age-dependent decrease in the growth hormone response to growth hormone-releasing hormone in normally cycling women. Fertil Steril. (1996) 66:230–4. doi: 10.1016/S0015-0282(16)58444-1
94. Bartke C. Growth hormone and aging: updated review. World J Mens Health. (2019) 37:19–30. doi: 10.5534/wjmh.180018
95. Ambrosio MR, Valentini A, Trasforini G, Minuto F, Ghigo E, Cella S, et al. Function of the GH/IGF-1 axis in healthy middle-aged male runners. Neuroendocrinology. (1996) 63:498–503. doi: 10.1159/000127078
96. Chadan SG, Dill RP, Vanderhoek K, Parkhouse SW. Influence of physical activity on plasma insulin-like growth factor-1 and insulin-like growth factor binding proteins in healthy older women. Mech Ageing Dev. (1999) 109:21–34. doi: 10.1016/S0047-6374(99)00017-2
97. Nelson ME, Meredith CN, Dawson-Hughes B, Evans JW. Hormone and bone mineral status in endurance-trained and sedentary postmenopausal women. J Clin Endocrinol Metab. (1988) 66:927–33. doi: 10.1210/jcem-66-5-927
98. Ari Z, Kutlu N, Uyanik BS, Taneli F, Buyukyazi G, Tavli T. Serum testosterone, growth hormone, and insulin-like growth factor-1 levels, mental reaction time, and maximal aerobic exercise in sedentary and long-term physically trained elderly males. Int J Neurosci. (2004) 114:623–37. doi: 10.1080/00207450490430499
99. Coiro V, Volpi R, Gramellini D, Maffei ML, Volta E, Melani A, et al. Effect of physical training on age-related reduction of GH secretion during exercise in normally cycling women. Maturitas. (2010) 65:392–5. doi: 10.1016/j.maturitas.2009.12.020
100. Craig BW, Brown R, Everhart J. Effects of progressive resistance training on growth hormone and testosterone levels in young and elderly subjects. Mech Ageing Dev. (1989) 49:159–69. doi: 10.1016/0047-6374(89)90099-7
101. Kraemer WJ, Häkkinen K, Newton RU, McCormick M, Nindl BC, Volek JS, et al. Acute hormonal responses to heavy resistance exercise in younger and older men. Eur J Appl Physiol Occup Physiol. (1998) 77:206–11. doi: 10.1007/s004210050323
102. Seo DI, Jun TW, Park KS, Chang H, So WY, Song W. 12 Weeks of combined exercise is better than aerobic exercise for increasing growth hormone in middle-aged women. Int J Sport Nutr Exerc Metab. (2010) 20:21–6. doi: 10.1123/ijsnem.20.1.21
103. Im JY, Bang HS, Seo YD. The effects of 12 weeks of a combined exercise program on physical function and hormonal status in elderly korean women. Int J Environ Res Public Health. (2019) 16:4196. doi: 10.3390/ijerph16214196
104. Deuschle M, Blum WF, Frystyk J, Orskov H, Schweiger U, Weber B, et al. Endurance training and its effect upon the activity of the GH-IGFs system in the elderly. Int J Sports Med. (1998) 19:250–4. doi: 10.1055/s-2007-971913
105. Gilbert KL, Stokes KA, Hall GM, Thompson D. Growth hormone responses to 3 different exercise bouts in 18- to 25- and 40- to 50-year-old men. Appl Physiol Nutr Metab. (2008) 33:706–12. doi: 10.1139/H08-034
106. Gordon SE, Kraemer WJ, Looney DP, Flanagan SD, Comstock BA, Hymer CW. The influence of age and exercise modality on growth hormone bioactivity in women. Growth Horm IGF Res. (2014) 24:95–103. doi: 10.1016/j.ghir.2014.03.005
107. Häkkinen K, Pakarinen A. Acute hormonal responses to heavy resistance exercise in men and women at different ages. Int J Sports Med. (1995) 16:507–13. doi: 10.1055/s-2007-973045
108. Kostka T, Patricot MC, Mathian B, Lacour JR, Bonnefoy M. Anabolic and catabolic hormonal responses to experimental two-set low-volume resistance exercise in sedentary and active elderly people. Aging Clin Exp Res. (2003) 15:123–30. doi: 10.1007/BF03324489
109. So WY, Song M, Park YH, Cho BL, Lim JY, Kim SH, et al. Body composition, fitness level, anabolic hormones, and inflammatory cytokines in the elderly: a randomized controlled trial. Aging Clin Exp Res. (2013) 25:167–74. doi: 10.1007/s40520-013-0032-y
110. Andersen NB, Andreassen TT, Orskov H, Oxlund H. Growth hormone and mild exercise in combination increases markedly muscle mass and tetanic tension in old rats. Eur J Endocrinol. (2000) 143:409–18. doi: 10.1530/eje.0.1430409
111. Marzetti E, Groban L, Wohlgemuth SE, Lees HA, Lin M, Jobe H, et al. Effects of short-term GH supplementation and treadmill exercise training on physical performance and skeletal muscle apoptosis in old rats. Am J Physiol Regul Integr Comp Physiol. (2008) 294:R558–67. doi: 10.1152/ajpregu.00620.2007
112. Groban L, Jobe H, Lin M, Houle T, Kitzman DA, Sonntag W. Effects of short-term treadmill exercise training or growth hormone supplementation on diastolic function and exercise tolerance in old rats. J Gerontol A Biol Sci Med Sci. (2008) 63:911–20. doi: 10.1093/gerona/63.9.911
113. Oxlund H, Andersen NB, Ortoft G, Orskov H, Andreassen TT. Growth hormone and mild exercise in combination markedly enhance cortical bone formation and strength in old rats. Endocrinology. (1998) 139:1899–904. doi: 10.1210/endo.139.4.5949
114. Mosekilde L, Thomsen JS, Orhii PB, McCarter RJ, Mejia W, Kalu ND. Additive effect of voluntary exercise and growth hormone treatment on bone strength assessed at four different skeletal sites in an aged rat model. Bone. (1999) 24:71–80. doi: 10.1016/S8756-3282(98)00169-0
115. Sattler FR, Castaneda-Sceppa C, Binder EF, Schroeder ET, Wang Y, Bhasin S, et al. Testosterone and growth hormone improve body composition and muscle performance in older men. J Clin Endocrinol Metab. (2009) 94:1991–2001. doi: 10.1210/jc.2008-2338
116. Taaffe DR, Pruitt L, Reim J, Hintz RL, Butterfield G, Hoffman AR, et al. Effect of recombinant human growth hormone on the muscle strength response to resistance exercise in elderly men. J Clin Endocrinol Metab. (1994) 79:1361–6. doi: 10.1210/jcem.79.5.7525633
117. Foley DJ, Monjan AA, Brown SL, Simonsick EM, Wallace RB, Blazer GD. Sleep complaints among elderly persons: an epidemiologic study of three communities. Sleep. (1995) 18:425–32. doi: 10.1093/sleep/18.6.425
118. Prinz PN. Sleep and sleep disorders in older adults. J Clin Neurophysiol. (1995) 12:139–46. doi: 10.1097/00004691-199503000-00004
119. Yoon IY, Kripke DF, Youngstedt SD, Elliott AJ. Actigraphy suggests age-related differences in napping and nocturnal sleep. J Sleep Res. (2003) 12:87–93. doi: 10.1046/j.1365-2869.2003.00345.x
120. Benloucif S, Orbeta L, Ortiz R, Janssen I, Finkel SI, Bleiberg J, et al. Morning or evening activity improves neuropsychological performance and subjective sleep quality in older adults. Sleep. (2004) 27:1542–51. doi: 10.1093/sleep/27.8.1542
121. Inoue S, Yorifuji T, Sugiyama M, Ohta T, Ishikawa-Takata K, Doi H. Does habitual physical activity prevent insomnia? A cross-sectional and longitudinal study of elderly Japanese. J Aging Phys Act. (2013) 21:119–39. doi: 10.1123/japa.21.2.119
122. Lira FS, Pimentel GD, Santos RV, Oyama LM, Damaso AR, Oller do Nascimento CM, et al. Exercise training improves sleep pattern and metabolic profile in elderly people in a time-dependent manner. Lipids Health Dis. (2011) 10:1–6. doi: 10.1186/1476-511X-10-113
123. Santos RV, Viana VA, Boscolo RA, Marques VG, Santana MG, Lira FS, et al. Moderate exercise training modulates cytokine profile and sleep in elderly people. Cytokine. (2012) 60:731–5. doi: 10.1016/j.cyto.2012.07.028
124. Melancon MO, Lorrain D, Dionne JI. Sleep depth and continuity before and after chronic exercise in older men: electrophysiological evidence. Physiol Behav. (2015) 140:203–8. doi: 10.1016/j.physbeh.2014.12.031
125. Akbari Kamrani A, Shams A, Shamsipour Dehkordi P, Mohajeri R. The effect of low and moderate intensity aerobic exercises on sleep quality in men older adults. Pak J Med Sci. (2014) 30:417–21. doi: 10.12669/pjms.302.4386
126. Karimi S, Soroush A, Towhidi F, Makhsosi BR, Karimi M, Jamehshorani S, et al. Surveying the effects of an exercise program on the sleep quality of elderly males. Clin Interv Aging. (2016) 11:997–1002. doi: 10.2147/CIA.S106808
127. Christie AD, Seery E, Kent AJ. Physical activity, sleep quality, and self-reported fatigue across the adult lifespan. Exp Gerontol. (2016) 77:7–11. doi: 10.1016/j.exger.2016.02.001
128. Endeshaw YW, Yoo W. Association between social and physical activities and insomnia symptoms among community-dwelling older adults. J Aging Health. (2016) 28:1073–89. doi: 10.1177/0898264315618921
129. Hartescu I, Morgan K, Stevinson DC. Sleep quality and recommended levels of physical activity in older people. J Aging Phys Act. (2016) 24:201–6. doi: 10.1123/japa.2014-0061
130. Brandão GS, Gomes SBF G, Callou Sampaio AA, Donner CF, Oliveira LVF, Camelier AA. Home exercise improves the quality of sleep and daytime sleepiness of elderlies: a randomized controlled trial. Multidiscip Respir Med. (2018) 13:2. doi: 10.4081/mrm.2018.74
131. Li J, Yang B, Varrasse M, Ji X, Wu M, Li M, et al. Physical activity in relation to sleep among community-dwelling older adults in China. J Aging Phys Act. (2018) 26:647–54. doi: 10.1123/japa.2017-0270
132. Abd El-Kader SM, Al-Jiffri OH. Aerobic exercise modulates cytokine profile and sleep quality in elderly. Afr Health Sci. (2019) 19:2198–207. doi: 10.4314/ahs.v19i2.45
133. Sonnega A, Leggett A, Pepin R, Assari S. Physical activity and insomnia symptoms over 10 years in a US. National sample of late-middle-age and older adults: age matters. J Aging Phys Act. (2020) doi: 10.1123/japa.2018-0337. [Epub ahead of print].
134. Van Gool WA, Mirmiran M. Age-related changes in the sleep pattern of male adult rats. Brain Res. (1983) 279:394–8. doi: 10.1016/0006-8993(83)90217-2
135. Naylor E, Buxton OM, Bergmann BM, Easton A, Zee PC, Turek WF. Effects of aging on sleep in the golden hamster. Sleep. (1998) 21:687–93. doi: 10.1093/sleep/21.7.687
136. Blanco-Centurion CA, Shiromani JP. Beneficial effects of regular exercise on sleep in old F344 rats. Neurobiol Aging. (2006) 27:1859–69. doi: 10.1016/j.neurobiolaging.2005.10.009
137. Nagahara AH, Merrill DA, Coppola G, Tsukada S, Schroeder BE, Shaked GM, et al. Neuroprotective effects of brain-derived neurotrophic factor in rodent and primate models of alzheimer's disease. Nat Med. (2009) 15:331–7. doi: 10.1038/nm.1912
138. Park H, Poo MM. Neurotrophin regulation of neural circuit development and function. Nat Rev Neurosci. (2013) 14:7–23. doi: 10.1038/nrn3379
139. Neeper SA, Gómez-Pinilla F, Choi J, Cotman C. Exercise and brain neurotrophins. Nature. (1995) 373:109. doi: 10.1038/373109a0
140. Jia JM, Chen Q, Zhou Y, Miao S, Zheng J, Zhang C, et al. Brain-derived neurotrophic factor-tropomyosin-related kinase B signaling contributes to activity-dependent changes in synaptic proteins. J Biol Chem. (2008) 283:21242–50. doi: 10.1074/jbc.M800282200
142. Walker LC, LeVine H. Corruption and spread of pathogenic proteins in neurodegenerative diseases. J Biol Chem. (2012) 287:33109–15. doi: 10.1074/jbc.R112.399378
143. Demontis F, Perrimon N. FOXO/4E-BP signaling in drosophila muscles regulates organism-wide proteostasis during aging. Cell. (2010) 143:813–25. doi: 10.1016/j.cell.2010.10.007
144. Santra M, Dill KA, de Graff RAM. Proteostasis collapse is a driver of cell aging and death. Proc Natl Acad Sci USA. (2019) 116:22173–8. doi: 10.1073/pnas.1906592116
145. Vilchez D, Saez I, Dillin A. The role of protein clearance mechanisms in organismal ageing and age-related diseases. Nat Commun. (2014) 5:5659. doi: 10.1038/ncomms6659
146. Ho CT, Grousl T, Shatz O, Jawed A, Ruger-Herreros C, Semmelink M, et al. Cellular sequestrases maintain basal Hsp70 capacity ensuring balanced proteostasis. Nat Commun. (2019) 10:4851. doi: 10.1038/s41467-019-12868-1
147. Finley D. Recognition and processing of ubiquitin-protein conjugates by the proteasome. Annu Rev Biochem. (2009) 78:477–513. doi: 10.1146/annurev.biochem.78.081507.101607
148. Gaczynska M, Osmulski PA, Ward FW. Caretaker or undertaker? The role of the proteasome in aging. Mech Ageing Dev. (2001) 122:235–54. doi: 10.1016/S0047-6374(00)00246-3
149. Vernace VA, Arnaud L, Schmidt-Glenewinkel T, Figueiredo-Pereira EM. Aging perturbs 26S proteasome assembly in drosophila melanogaster. FASEB J. (2007) 21:2672–82. doi: 10.1096/fj.06-6751com
150. Shemorry A, Hwang CS, Varshavsky A. Control of protein quality and stoichiometries by N-terminal acetylation and the N-end rule pathway. Mol Cell. (2013) 50:540–51. doi: 10.1016/j.molcel.2013.03.018
151. Samant RS, Livingston CM, Sontag EM, Frydman J. Distinct proteostasis circuits cooperate in nuclear and cytoplasmic protein quality control. Nature. (2018) 563:407–11. doi: 10.1038/s41586-018-0678-x
152. Cuervo AM, Macian F. Autophagy and the immune function in aging. Curr Opin Immunol. (2014) 29:97–104. doi: 10.1016/j.coi.2014.05.006
153. Rubinsztein DC, Mariño G, Kroemer G. Autophagy and aging. Cell. (2011) 146:682–95. doi: 10.1016/j.cell.2011.07.030
154. Klionsky DJ, Emr DS. Autophagy as a regulated pathway of cellular degradation. Science. (2000) 290:1717–21. doi: 10.1126/science.290.5497.1717
155. Levine B, Kroemer G. Autophagy in the pathogenesis of disease. Cell. (2008) 132:27–42. doi: 10.1016/j.cell.2007.12.018
156. Madeo F, Tavernarakis N, Kroemer G. Can autophagy promote longevity? Nat Cell Biol. (2010) 12:842–6. doi: 10.1038/ncb0910-842
157. Madeo F, Zimmermann A, Maiuri MC, Kroemer G. Essential role for autophagy in life span extension. J Clin Invest. (2015) 125:85–93. doi: 10.1172/JCI73946
158. Mizushima N. Autophagy: process and function. Genes Dev. (2007) 21:2861–73. doi: 10.1101/gad.1599207
159. Nakamura S, Yoshimori T. Autophagy and longevity. Mol Cells. (2018) 41:65–72. doi: 10.14348/molcells.2018.2333
160. Maiuri MC, Zalckvar E, Kimchi A, Kroemer G. Self-eating and self-killing: crosstalk between autophagy and apoptosis. Nat Rev Mol Cell Biol. (2007) 8:741–52. doi: 10.1038/nrm2239
161. de Mello NP, Orellana AM, Mazucanti CH, de Morais Lima G, Scavone C, Kawamoto ME. Insulin and autophagy in neurodegeneration. Front Neurosci. (2019) 13:491. doi: 10.3389/fnins.2019.00491
162. Rubinsztein DC, Gestwicki JE, Murphy LO, Klionsky JD. Potential therapeutic applications of autophagy. Nat Rev Drug Discov. (2007) 6:304–12. doi: 10.1038/nrd2272
163. Feng Y, He D, Yao Z, Klionsky JD. The machinery of macroautophagy. Cell Res. (2014) 24:24–41. doi: 10.1038/cr.2013.168
164. Romanov J, Walczak M, Ibiricu I, Schüchner S, Ogris E, Kraft C, et al. Mechanism and functions of membrane binding by the Atg5-Atg12/Atg16 complex during autophagosome formation. EMBO J. (2012) 31:4304–17. doi: 10.1038/emboj.2012.278
165. Yang Z, Klionsky JD. Eaten alive: a history of macroautophagy. Nat Cell Biol. (2010) 12:814–22. doi: 10.1038/ncb0910-814
166. Menon MB, Dhamija S. Beclin 1 phosphorylation - at the center of autophagy regulation. Front Cell Dev Biol. (2018) 6:137. doi: 10.3389/fcell.2018.00137
167. Zeng X, Overmeyer JH, Maltese AW. Functional specificity of the mammalian Beclin-Vps34 PI 3-kinase complex in macroautophagy versus endocytosis and lysosomal enzyme trafficking. J Cell Sci. (2006) 119(Pt 2):259–70. doi: 10.1242/jcs.02735
168. Levine B, Mizushima N, Virgin WH. Autophagy in immunity and inflammation. Nature. (2011) 469:323–35. doi: 10.1038/nature09782
169. Cuervo AM, Bergamini E, Brunk UT, Dröge W, Ffrench M, Terman A. Autophagy and aging: the importance of maintaining “clean” cells. Autophagy. (2005) 1:131–40. doi: 10.4161/auto.1.3.2017
170. Wu HJ, Pu JL, Krafft PR, Zhang JM, Chen S. The molecular mechanisms between autophagy and apoptosis: potential role in central nervous system disorders. Cell Mol Neurobiol. (2015) 35:85–99. doi: 10.1007/s10571-014-0116-z
171. Metaxakis A, Ploumi C, Tavernarakis N. Autophagy in age-associated neurodegeneration. Cells. (2018) 7:37. doi: 10.3390/cells7050037
172. Batatinha HAP, Diniz TA, de Souza Teixeira AA, Krüger K, Rosa-Neto CJ. Regulation of autophagy as a therapy for immunosenescence-driven cancer and neurodegenerative diseases: the role of exercise. J Cell Physiol. (2019) 234:1–3. doi: 10.1002/jcp.28318
173. Hartleben B, Gödel M, Meyer-Schwesinger C, Liu S, Ulrich T, Köbler S, et al. Autophagy influences glomerular disease susceptibility and maintains podocyte homeostasis in aging mice. J Clin Invest. (2010) 120:1084–96. doi: 10.1172/JCI39492
174. Komatsu M, Waguri S, Ueno T, Iwata J, Murata S, Tanida I, et al. Impairment of starvation-induced and constitutive autophagy in Atg7-deficient mice. J Cell Biol. (2005) 169:425–34. doi: 10.1083/jcb.200412022
175. Masiero E, Agatea L, Mammucari C, Blaauw B, Loro E, Komatsu M, et al. Autophagy is required to maintain muscle mass. Cell Metab. (2009) 10:507–15. doi: 10.1016/j.cmet.2009.10.008
176. Wu JJ, Quijano C, Chen E, Liu H, Cao L, Fergusson MM, et al. Mitochondrial dysfunction and oxidative stress mediate the physiological impairment induced by the disruption of autophagy. Aging (Albany NY). (2009) 1:425–37. doi: 10.18632/aging.100038
177. Kapahi P, Chen D, Rogers AN, Katewa SD, Li PW, Thomas EL, et al. With TOR. less is more: a key role for the conserved nutrient-sensing TOR pathway in aging. Cell Metab. (2010) 11:453–65. doi: 10.1016/j.cmet.2010.05.001
178. Pani G. From growing to secreting: new roles for mTOR in aging cells. Cell Cycle. (2011) 10:2450–3. doi: 10.4161/cc.10.15.16886
179. Xu S, Cai Y, Wei Y. mTOR signaling from cellular senescence to organismal aging. Aging Dis. (2014) 5:263–73. doi: 10.14336/AD.2014.0500263
180. He C, Sumpter R, Levine B. Exercise induces autophagy in peripheral tissues and in the brain. Autophagy. (2012) 8:1548–51. doi: 10.4161/auto.21327
181. Jiang D, Chen K, Lu X, Gao HJ, Qin ZH, Lin F. Exercise ameliorates the detrimental effect of chloroquine on skeletal muscles in mice via restoring autophagy flux. Acta Pharmacol Sin. (2014) 35:135–42. doi: 10.1038/aps.2013.144
182. Mooren FC, Krüger K. Exercise, autophagy, and apoptosis. Prog Mol Biol Transl Sci. (2015) 135:407–22. doi: 10.1016/bs.pmbts.2015.07.023
183. Moreira OC, Estébanez B, Martínez-Florez S, de Paz JA, Cuevas MJ, González-Gallego J. Mitochondrial function and mitophagy in the elderly: effects of exercise. Oxid Med Cell Longev. (2017) 2017:2012798. doi: 10.1155/2017/2012798
184. Jang YC, Hwang DJ, Koo JH, Um HS, Lee NH, Yeom DC, et al. Association of exercise-induced autophagy upregulation and apoptosis suppression with neuroprotection against pharmacologically induced parkinson's disease. J Exerc Nutrition Biochem. (2018) 22:1–8. doi: 10.20463/jenb.2018.0001
185. Kim J, Kundu M, Viollet B, Guan LK. AMPK and mTOR regulate autophagy through direct phosphorylation of Ulk1. Nat Cell Biol. (2011) 13:132–41. doi: 10.1038/ncb2152
186. Schwalm C, Jamart C, Benoit N, Naslain D, Prémont C, Prévet J, et al. Activation of autophagy in human skeletal muscle is dependent on exercise intensity and AMPK activation. FASEB J. (2015) 29:3515–26. doi: 10.1096/fj.14-267187
187. Luo L, Dai JR, Guo SS, Lu AM, Gao XF, Gu YR, et al. Lysosomal proteolysis is associated with exercise-induced improvement of mitochondrial quality control in aged hippocampus. J Gerontol A Biol Sci Med Sci. (2017) 72:1342–51. doi: 10.1093/gerona/glw242
188. Huang J, Wang X, Zhu Y, Li Z, Zhu YT, Wu JC, et al. Exercise activates lysosomal function in the brain through AMPK-SIRT1-TFEB pathway. CNS Neurosci Ther. (2019) 25:796–807. doi: 10.1111/cns.13114
189. Almeida MF, Silva CM, Chaves RS, Lima NCR, Almeida RS, Melo KP, et al. Effects of mild running on substantia nigra during early neurodegeneration. J Sports Sci. (2018) 36:1363–70. doi: 10.1080/02640414.2017.1378494
190. Higami Y, Shimokawa I. Apoptosis in the aging process. Cell Tissue Res. (2000) 301:125–32. doi: 10.1007/s004419900156
191. Mejías-Peña Y, Estébanez B, Rodriguez-Miguelez P, Fernandez-Gonzalo R, Almar M, de Paz JA, et al. Impact of resistance training on the autophagy-inflammation-apoptosis crosstalk in elderly subjects. Aging (Albany NY). (2017) 9:408–18. doi: 10.18632/aging.101167
192. Ashkenazi A. Targeting the extrinsic apoptotic pathway in cancer: lessons learned and future directions. J Clin Invest. (2015) 125:487–9. doi: 10.1172/JCI80420
193. Svandova EB, Vesela B, Lesot H, Poliard A, Matalova E. Expression of fas, FasL, caspase-8 and other factors of the extrinsic apoptotic pathway during the onset of interdigital tissue elimination. Histochem Cell Biol. (2017) 147:497–510. doi: 10.1007/s00418-016-1508-6
194. Ashkenazi A, Fairbrother WJ, Leverson JD, Souers JA. From basic apoptosis discoveries to advanced selective BCL-2 family inhibitors. Nat Rev Drug Discov. (2017) 16:273–84. doi: 10.1038/nrd.2016.253
195. Kale J, Osterlund EJ, Andrews WD. BCL-2 family proteins: changing partners in the dance towards death. Cell Death Differ. (2018) 25:65–80. doi: 10.1038/cdd.2017.186
196. Singh R, Letai A, Sarosiek K. Regulation of apoptosis in health and disease: the balancing act of BCL-2 family proteins. Nat Rev Mol Cell Biol. (2019) 20:175–93. doi: 10.1038/s41580-018-0089-8
197. Fang G, Zhao J, Li P, Li L, Yu T, Yang X, et al. Long-term treadmill exercise inhibits neuronal cell apoptosis and reduces tau phosphorylation in the cerebral cortex and hippocampus of aged rats. Sci Bull. (2017) 62:755–7. doi: 10.1016/j.scib.2017.05.004
198. Budni J, Bellettini-Santos T, Mina F, Garcez ML, Zugno IA. The involvement of BDNF, NGF and GDNF in aging and alzheimer's disease. Aging Dis. (2015) 6:331–41. doi: 10.14336/AD.2015.0825
199. Deng X, Chen B, Wang B, Zhang J, Liu H. TNF-α mediates the intrinsic and extrinsic pathway in propofol-induced neuronal apoptosis Via PI3K/Akt signaling pathway in rat prefrontal cortical neurons. Neurotox Res. (2017) 32:409–19. doi: 10.1007/s12640-017-9751-8
200. Henrique JS, França EF, Cardoso FDS, Serra FT, de Almeida AA, Fernandes J, et al. Cortical and hippocampal expression of inflammatory and intracellular signaling proteins in aged rats submitted to aerobic and resistance physical training. Exp Gerontol. (2018) 110:284–90. doi: 10.1016/j.exger.2018.06.025
201. Song SH, Jee YS, Ko IG, Lee SW, Sim YJ, Kim DY, et al. Treadmill exercise and wheel exercise improve motor function by suppressing apoptotic neuronal cell death in brain inflammation rats. J Exerc Rehabil. (2018) 14:911–19. doi: 10.12965/jer.1836508.254
202. Norton S, Matthews FE, Barnes DE, Yaffe K, Brayne C. Potential for primary prevention of alzheimer's disease: an analysis of population-based data. Lancet Neurol. (2014) 13:788–94. doi: 10.1016/S1474-4422(14)70136-X
203. Duzel E, van Praag H, Sendtner M. Can physical exercise in old age improve memory and hippocampal function? Brain. (2016) 139:662–73. doi: 10.1093/brain/awv407
204. Fattoretti P, Malatesta M, Cisterna B, Milanese C, Zancanaro C. Modulatory effect of aerobic physical activity on synaptic ultrastructure in the old mouse hippocampus. Front Aging Neurosci. (2018) 10:141. doi: 10.3389/fnagi.2018.00141
205. Park SY, Yoon SN, Kang MJ, Lee Y, Jung SJ, Han JS. Hippocalcin promotes neuronal differentiation and inhibits astrocytic differentiation in neural stem cells. Stem Cell Rep. (2017) 8:95–111. doi: 10.1016/j.stemcr.2016.11.009
206. Xie L, Li A, Shen J, Cao M, Ning X, Yuan D, et al. OTUB1 attenuates neuronal apoptosis after intracerebral hemorrhage. Mol Cell Biochem. (2016) 422:171–80. doi: 10.1007/s11010-016-2817-8
207. Baker LD, Skinner JS, Craft S, Sink KM, Montine T, Hansen A, et al. Aerobic exercise reduces phosphorylated tau protein in cerebrospinal fluid in older adults with mild cognitive impairment. Alzheimer's Dementia J Alzheimer's Assoc. (2015) 11:P324. doi: 10.1016/j.jalz.2015.07.467
208. Daniele S, Pietrobono D, Fusi J, Lo Gerfo A, Cerri E, Chico L, et al. α-Synuclein aggregated with Tau and β-amyloid in human platelets from healthy subjects: correlation with physical exercise. Front Aging Neurosci. (2018) 10:17. doi: 10.3389/fnagi.2018.00017
209. Wang Q, Hu J, Liu Y, Li J, Liu B, Li M, et al. Aerobic exercise improves synaptic-related proteins of diabetic rats by inhibiting FOXO1/NF-κB/NLRP3 inflammatory signaling pathway and ameliorating PI3K/Akt insulin signaling pathway. J Mol Neurosci. (2019) 69:28–38. doi: 10.1007/s12031-019-01302-2
210. Liu W, Wang Z, Xia Y, Kuang H, Liu S, Li L, et al. The balance of apoptosis and autophagy via regulation of the AMPK signal pathway in aging rat striatum during regular aerobic exercise. Exp Gerontol. (2019) 124:110647. doi: 10.1016/j.exger.2019.110647
211. Do K, Laing BT, Landry T, Bunner W, Mersaud N, Matsubara T, et al. The effects of exercise on hypothalamic neurodegeneration of alzheimer's disease mouse model. PLoS ONE. (2018) 13:e0190205. doi: 10.1371/journal.pone.0190205
212. Choi DH, Kwon IS, Koo JH, Jang YC, Kang EB, Byun JE. The effect of treadmill exercise on inflammatory responses in rat model of streptozotocin-induced experimental dementia of alzheimer's type. J Exerc Nutr Biochem. (2014) 18:225. doi: 10.5717/jenb.2014.18.2.225
213. Spielman LJ, Little JP, Klegeris A. Physical activity and exercise attenuate neuroinflammation in neurological diseases. Brain Res Bull. (2016) 125:19–29. doi: 10.1016/j.brainresbull.2016.03.012
214. Cassilhas RC, Tufik S, de Mello TM. Physical exercise, neuroplasticity, spatial learning and memory. Cell Mol Life Sci. (2016) 73:975–83. doi: 10.1007/s00018-015-2102-0
215. Vilela TC, Muller AP, Damiani AP, Macan TP, da Silva S, Canteiro PB, et al. Strength and aerobic exercises improve spatial memory in aging rats through stimulating distinct neuroplasticity mechanisms. Mol Neurobiol. (2017) 54:7928–37. doi: 10.1007/s12035-016-0272-x
216. Neto WK, Silva W, Ciena AP, Anaruma CA, Gama FE. Vertical climbing for rodent resistance training: a discussion about training parameters. Int J Sports Sci. (2016) 6:36–49. doi: 10.5923/s.sports.201601.07
217. de Smolarek Camargo A, Ferreira LH, Mascarenhas LP, McAnulty SR, Varela KD, Dangui MC, et al. The effects of strength training on cognitive performance in elderly women. Clin Interv Aging. (2016) 11:749–54. doi: 10.2147/CIA.S102126
218. Yoon DH, Lee JY, Song W. Effects of resistance exercise training on cognitive function and physical performance in cognitive frailty: a randomized controlled trial. J Nutr Health Aging. (2018) 22:944–51. doi: 10.1007/s12603-018-1090-9
219. Martínez-Velilla N, Casas-Herrero A, Zambom-Ferraresi F, Sáez de Asteasu ML, Lucia A, Galbete A, et al. Effect of exercise intervention on functional decline in very elderly patients during acute hospitalization: a randomized clinical trial. JAMA Intern Med. (2019) 179:28–36. doi: 10.1001/jamainternmed.2018.4869
220. Martínez-Velilla N, Cadore EL, Casas-Herrero Á, Idoate-Saralegui F, Izquierdo M. Physical activity and early rehabilitation in hospitalized elderly medical patients: systematic review of randomized clinical trials. J Nutr Health Aging. (2016) 20:738–51. doi: 10.1007/s12603-016-0683-4
221. Karlsen A, Loeb MR, Andersen KB, Joergensen KJ, Scheel FU, Turtumoeygard IF, et al. Improved functional performance in geriatric patients during hospital stay. Am J Phys Med Rehabil. (2017) 96:e78–84. doi: 10.1097/PHM.0000000000000671
222. Ikudome S, Mori S, Unenaka S, Kawanishi M, Kitamura T, Nakamoto H. Effect of long-term body-mass-based resistance exercise on cognitive function in elderly people. J Appl Gerontol. (2017) 36:1519–33. doi: 10.1177/0733464815625834
223. Feng L, Nyunt MS, Gao Q, Lee TS, Tsoi T, Chong MS, et al. Physical frailty, cognitive impairment, and the risk of neurocognitive disorder in the singapore longitudinal ageing studies. J Gerontol A Biol Sci Med Sci. (2017) 72:369–75. doi: 10.1093/gerona/glw050
224. Stenholm S, Ferrucci L, Vahtera J, Hoogendijk EO, Huisman M, Pentti J, et al. Natural course of frailty components in people who develop frailty syndrome: evidence from two cohort studies. J Gerontol A Biol Sci Med Sci. (2019) 74:667–74. doi: 10.1093/gerona/gly132
225. Cadore EL, Sáez de Asteasu ML, Izquierdo M. Multicomponent exercise and the hallmarks of frailty: considerations on cognitive impairment and acute hospitalization. Exp Gerontol. (2019) 122:10–14. doi: 10.1016/j.exger.2019.04.007
226. Kalehua A, Nagel J, Whelchel L, Gides J, Pyle R, Smith R, et al. Monocyte chemoattractant protein-1 and macrophage inflammatory protein-2 are involved in both excitotoxin-induced neurodegeneration and regeneration. Exp Cell Res. (2004) 297:197–211. doi: 10.1016/j.yexcr.2004.02.031
227. Shu YH, Lu XM, Wei JX, Xiao L, Wang YT. Update on the role of p75NTR in neurological disorders: a novel therapeutic target. Biomed Pharmacother. (2015) 76:17–23. doi: 10.1016/j.biopha.2015.10.010
228. Cheng I, Jin L, Rose LC, Deppmann DC. Temporally restricted death and the role of p75 NTR as a survival receptor in the developing sensory nervous system. Dev Neurobiol. (2018) 78:701–17. doi: 10.1002/dneu.22591
Keywords: aging, exercise, autophagy, apoptosis, brain
Citation: Andreotti DZ, Silva JN, Matumoto AM, Orellana AM, de Mello PS and Kawamoto EM (2020) Effects of Physical Exercise on Autophagy and Apoptosis in Aged Brain: Human and Animal Studies. Front. Nutr. 7:94. doi: 10.3389/fnut.2020.00094
Received: 29 November 2019; Accepted: 22 May 2020;
Published: 28 July 2020.
Edited by:
Yuri Zilberter, INSERM U1106 Institut de Neurosciences des Systèmes, FranceReviewed by:
Francesco Marotta, ReGenera R&D International for Aging Intervention, ItalyVirve Cavallucci, Fondazione Policlinico Universitario A. Gemelli - Università Cattolica del Sacro Cuore, Italy
Copyright © 2020 Andreotti, Silva, Matumoto, Orellana, de Mello and Kawamoto. This is an open-access article distributed under the terms of the Creative Commons Attribution License (CC BY). The use, distribution or reproduction in other forums is permitted, provided the original author(s) and the copyright owner(s) are credited and that the original publication in this journal is cited, in accordance with accepted academic practice. No use, distribution or reproduction is permitted which does not comply with these terms.
*Correspondence: Elisa Mitiko Kawamoto, a2F3YW1vdG9lQGdtYWlsLmNvbQ==
†These authors have contributed equally to this work